- 1Nicholas School of the Environment, Division of Marine Science and Conservation, Duke University, Durham, NC, United States
- 2Department of Environmental Engineering Science, Engineering School for Sustainable Infrastructure and Environment, Center for Coastal Solutions, University of Florida, Gainesville, FL, United States
- 3Alfred-Wegener-Institute Helmholtz Center for Polar and Marine Research (AWI), Bremerhaven, Germany
- 4Commonwealth Scientific Industrial Research Organisation, Environment, Battery Point, TAS, Australia
- 5National Centers for Coastal Ocean Science, National Ocean Service, National Oceanic and Atmospheric Administration, Beaufort, NC, United States
- 6Conservation Ecology, Groningen Institute of Evolutionary Life Sciences GELIFES, University of Groningen, Groningen, Netherlands
- 7Department Coastal Systems, Royal Netherlands Institute for Sea Research and Utrecht University, Den Burg, Netherlands
- 8School of Zoology, Tel Aviv University, Ramat Aviv, Israel
Editorial on the Research Topic
Marine ecosystem restoration (MER) – challenges and new horizons
Widespread loss of coastal ecosystems and the important services they provide severely threatens both biodiversity and human health across the globe (Bayraktarov et al., 2015; He and Silliman, 2019; Saunders et al., 2020). To help combat this threat, the United Nations has declared 2021-2030 the Decade on Ecosystem Restoration. Demand for marine ecosystem restoration in many countries has subsequently increased at exponential rates (United Nations et al., 2020). For this demand to be met and for restoration to increase in efficiency and outcome success, the paradigm of marine restoration science, engineering, and application needs to expand to be more intellectually and socially inclusive of disciplines, sectors and stakeholders. Here, we highlight 10 key concepts that are essential for achieving such inclusivity. Widespread adoption of these concepts will advance the pace and scale of ecosystem restoration, as well as ensure higher and more equitable provisioning of user-inspired, social-ecological outcomes. For example, the restoration paradigm, with its solution-oriented focus, must rapidly factor in emerging technologies, advances in ecological and social science theory and application, diverse cultural and socioeconomic perspectives, broad stakeholder engagement, and advancements from established cultivation sciences and the private sector. Taken together, these concepts, highlight the urgent need to greatly broaden the marine restoration conceptual framework if we are to elevate and globally scale marine ecosystem restoration into an intervention that achieves real-world benefits in our lifetime.
The need to conserve marine ecosystems, biodiversity and habitat-forming species has become a broadly acknowledged societal goal, reflected in international frameworks and national and local policies (Bridgewater et al., 2019; Duarte et al., 2020; Ruckelshaus et al., 2020; Grorud-Colvert et al., 2021; United Nations et al., 2022). Beyond ethical or spiritual motivations, the focus on the tangible benefits marine biodiversity and habitats provide to humans in the form of ecosystem services (e.g., food provisioning and coastal protection) has emerged over the past 20 years as the other, key motivating factor for elevating marine conservation efforts (Saunders et al., 2020; Lester et al.; Bayraktarov et al., 2015; McAfee et al., 2021; Wittmer et al., 2021). Despite increased investments over the past few decades in traditional marine conservation interventions, such as protected areas, marine spatial planning, marine ecosystem management, pollution reduction from point and non-point sources, and fisheries management, the rate of marine ecosystem loss and marine biodiversity declines has continued globally (Saunders et al., 2020). While these traditional, conservation-focused interventions may be slowing the decline, they clearly are not enough. In many places, there remains an urgent need for bigger, more stable, and more productive marine ecosystems than presently exist that can generate multiple ecosystem services (Obura et al., 2023). There is also a need to deepen the inclusion of the communities that may benefit from such conservation and restoration efforts in the design, implementation and management of such interventions to enhance their value and long-term sustainability.
Inspired by the need to do more, and by The United Nations’ declaration that 2021-2030 is the Decade on Ecosystem Restoration, national governments, the private sector and conservation agencies around the world have markedly increased their investment in marine ecosystem restoration over the past 5-10 years (Saunders et al., 2020). Their shared goal is to elevate marine ecosystem restoration so that it is a viable conservation intervention at large spatial scales relevant to achieving significant social, economic and ecological benefits. Increased investment alone, however, will not produce these results, as marine ecosystem restoration has traditionally been considered a less desirable intervention by practitioners because of its relatively high failure rates (62% seagrass, 35% coral, 35% salt marsh failures) and high costs (US $1,600,000 per restored hectare) (Bayraktarov et al., 2015). Instead, fundamental changes in how marine restoration is undertaken are required to achieve the gains that global communities now seek.
Despite the perception that marine ecosystem restoration is prone to failure, recent syntheses have revealed bright spots in marine habitat restoration and shown that restoration projects in marine systems can indeed be: 1) cost effective, 2) successful over large spatial and temporal scales, and 3) provide social and economic benefits to people (Bayraktarov et al., 2015; Saunders et al., 2020). This is especially true in coastal areas where the stressors that historically killed marine foundation species (e.g., pollution or overfishing) have been reduced and regrowth of habitat-forming species is limited by recruitment and presence of positive species interactions (e.g., Silliman et al., 2015; Temmink et al., 2021). Key to expanding these bright spots so that they become the rule rather than the exception is increasing successes in both organismal regrowth and ecosystem service outcomes in marine restoration efforts.
This Research Topic on Marine Ecosystem Restoration (MER) highlights key innovations and challenges that, if revised and overcome, cans greatly increase restoration success, its scale of application, and value to communities. To do so, however, the paradigm for marine ecosystem restoration must greatly expand and be more inclusive of disciplines, sectors and stakeholders not traditionally engaged in restoration planning, design, application and assessment. As a starting point, we highlight and discuss a non-exhaustive list of ten key concepts that should be incorporated into this emerging, new paradigm. The original papers in this Research Topic develop these concepts in more depth and raise many more important factors and issues that should be considered in the quickly evolving MER practice and paradigm. Adopting this expanded paradigm may require profound changes, including ethical and philosophical considerations that address the relationship and responsibility of humans to nature in the sea.
Concept 1. A comprehensive MER approach is a key condition for restoration success
Historically, comprehensive planning and evaluation have been missing from most restoration efforts in marine systems (Lester et al.) and, as a result, managers are less likely to adapt, expand and/or pivot their approaches (Domínguez-Tejo et al., 2016). Therefore, a comprehensive MER approach (Concept #1, or C1), which encompasses a sequence of three distinct phases, should be determined as a precondition of any MER project. The three phases are: 1) pre-launch assessment and planning, 2) restoration interventions, and 3) post-restoration monitoring and long-term adaptive management (Figure 1). The three phases are essential for a sound MER implementation and are designed to maximize the chance for success of any MER project.
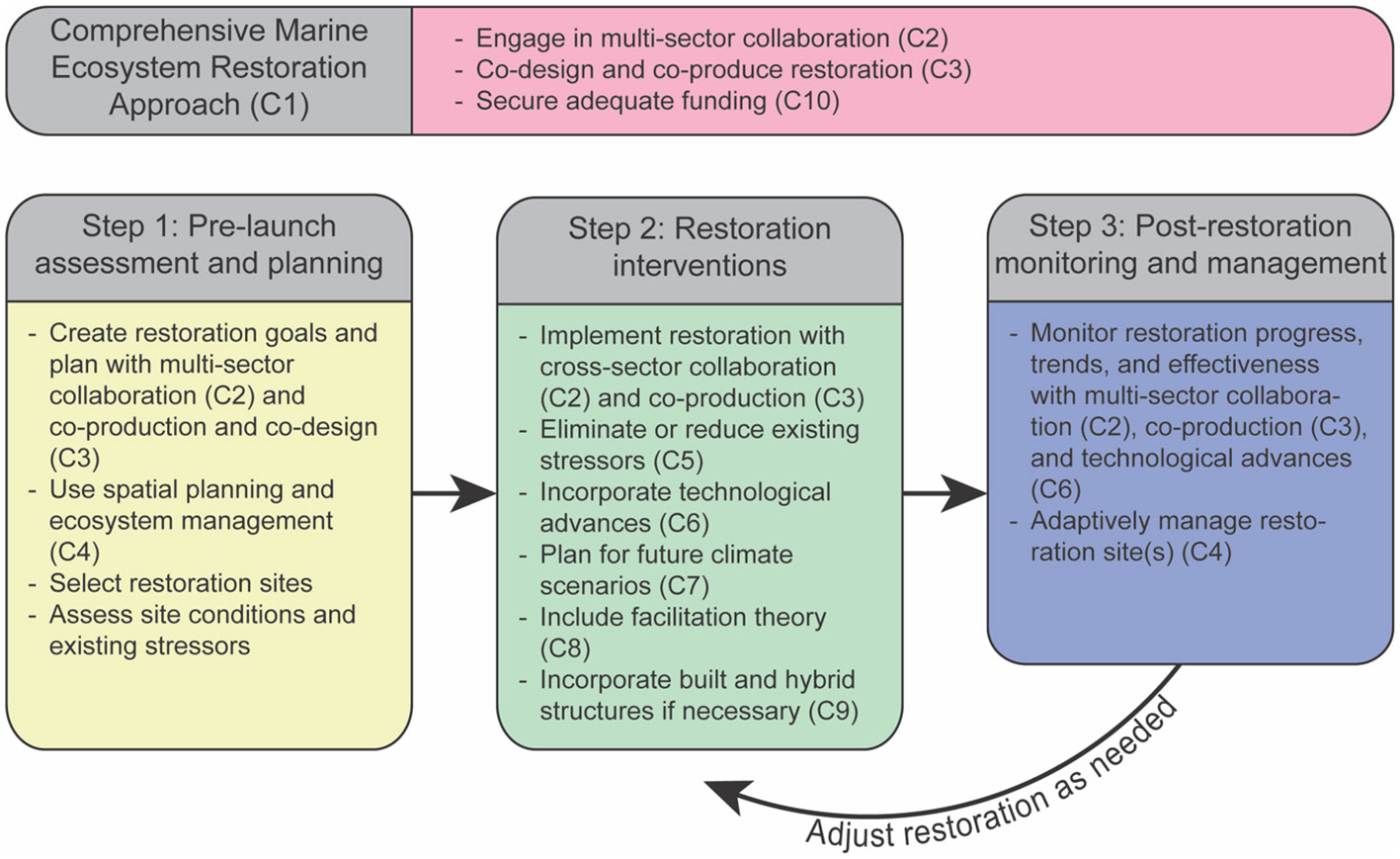
Figure 1 Comprehensive framework for marine ecosystem restoration. Framework incorporates key concepts. Each concept is labeled as “C” and the concept number. For example, Concept #2, which is multi-sector collaboration, is referred to as C2.
The pre-launch assessment and planning phase includes site selection based on ecological and social factors (Abelson et al., 2016), as well as an assessment of the extent of the ecosystem degradation and the underlying conditions at restoration locations (Abelson et al., 2016; Gann et al., 2019). This phase should also include site specific spatial planning and in-depth understanding of specific goals in terms of habitat regrowth and increased provisioning of ecosystem services (Lester et al.). Information gained during this phase will be used to develop a site-specific MER plan that outlines the objectives (and identifies targets and baselines), and the feasible interventions required to restore the ecosystem to its pre-degradation healthy state. If a site is unable to be restored to its pre-degradation state or that goal is beyond reach due to local conditions or budget limits, then the ecosystem can be restored to achieve or provide better ecosystem services, although this may come with tradeoffs. The assessment should use various tools, such as ecological surveys, mapping, and modeling, and engage with local communities and stakeholders in multiple meetings to understand and incorporate their perspectives and concerns.
The restoration interventions phase is based on the planning outcomes of the first phase and may include one or more actions out of a wide range of active management tools of different levels of intervention (Abelson et al., 2016) along the “restorative continuum” (Gann et al., 2019). These include protection and elimination of exogenous stressors (e.g., land-based pollution sources like sewage outlets; Wear et al., 2021; Concept #5) and endogenous stressors (e.g., eradication of invasive or outbreaking species via culling; Guarnieri et al.), and habitat enhancement (e.g., artificial reefs; Paxton et al.; Concept #5), to diverse restocking tools of ecosystem engineers (Doropoulos et al.; Schmidt-Roach et al. et al.; Zhang et al.) and facilitating species (Hammann et al.; Zhang et al.; Concept #8).
The monitoring and long-term maintenance stage involves assessing the progress of the restoration interventions and evaluating the effectiveness of the restoration plan to ensure the sustainability of the restored ecosystem, and implementation of adjustments as needed. At present, monitoring is not typically funded sufficiently to cover the full life span of restoration projects (Bayraktarov et al., 2015; Saunders et al., 2020; Saunders et al., 2022). The long-term segment of phase 3 may be considered a management stage, which may include replenishment, trending, and maintenance that will accompany the restored ecosystem with no deadlines.
We believe that rigorous adoption of the MER comprehensive approach, beyond maximizing the chances of successful restoration outcomes, will prove to be cost-effective in the long-run by reversing degradation, reducing the need for ongoing restoration interventions and improving ecosystem services for local communities as well as national and global conservation entities.
Concept 2. Expanding multi-sector collaboration is essential for scaling-up restoration
For decades, MER has been carried out by a passionate consortium of organizations that vary in the degree to which they coordinate to meet society’s demand for more expansive, productive, and resilient ecosystems. As highlighted in this Research Topic, both improving such coordination and activating the participation of sectors not yet engaged in restoration are vital to achieving major gains in rebuilding and creating marine habitats in the coming decade at large spatial extents (Eger et al.; Schmidt-Roach et al.). In short, large, well-coordinated teams that bring diverse expertise, resources, relationships, and values to bear are essential to pulling off large-scale, high impact restoration projects (Figure 2-C2).
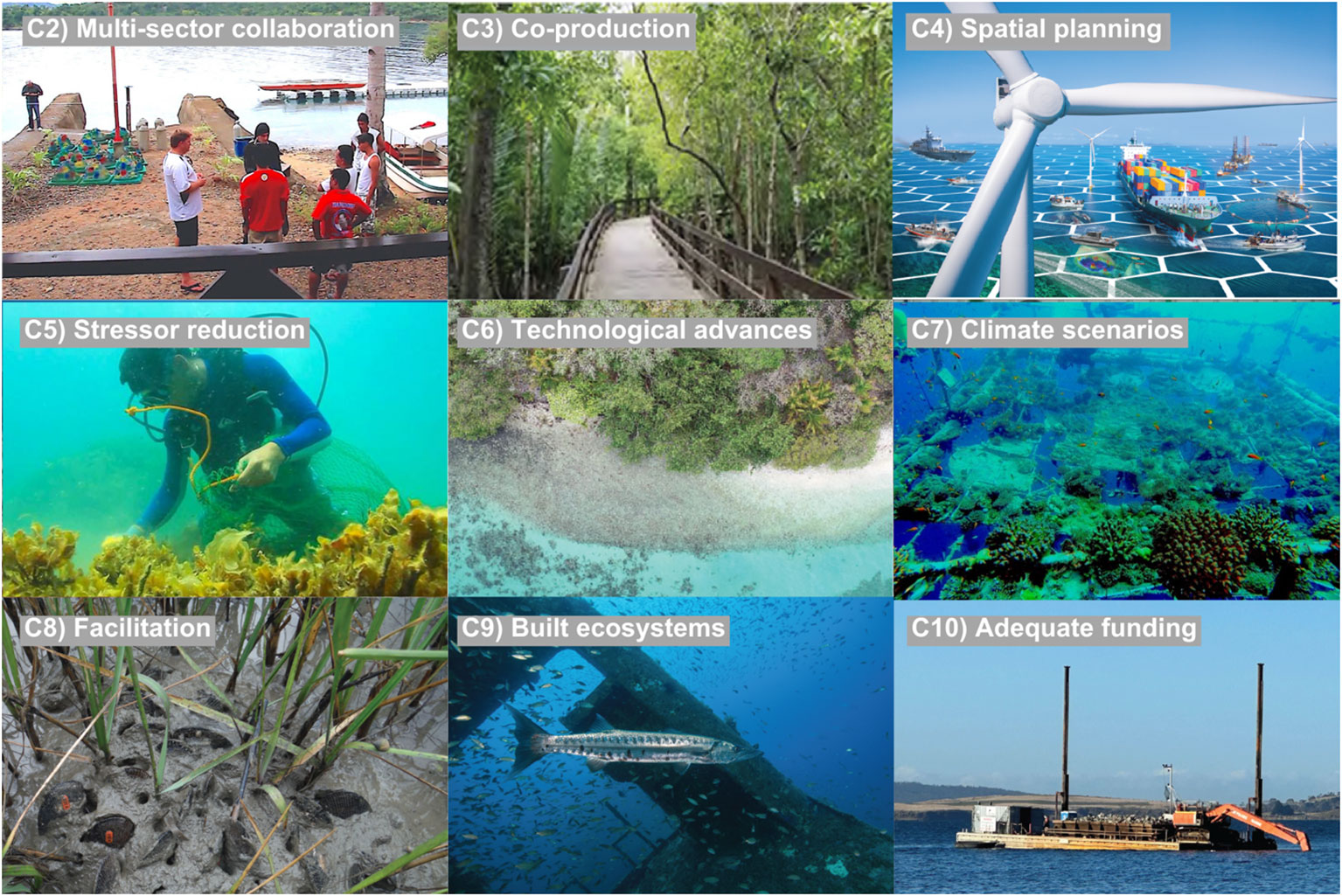
Figure 2 Key concepts to build a more inclusive paradigm for marine ecosystem restoration include: C2) Multi-sector collaboration, C3) Co-production, C4) Spatial planning, C5) Stressor reduction, C6) Technological advances, C7) Climate scenarios, C8) Facilitation theory, C9) Built ecosystems, and C10) Adequate funding. C2) Multi-sector collaboration, such as with artificial reef installation in the Philippines, can lead to successful restoration outcomes Photo credit: Avidgor Abelson. C3) Ellison et al. restored mangroves using co-production that incorporated values of multiple stakeholders and agents. Photo credit: Aaron M. Ellison, CC-BY-NC. C4) Spatial planning can be used to help design successful restorations. Image(s) used under license from Shutterstock.com. Rendering by Dan McDonald. C5) Removing stressors, such as seaweed from reefs, can achieve positive restoration outcomes. Photo credit: Avigdor Abelson. C6) Ridge and Johnston used aerial imaging technologies to monitor restoration outcomes. Photo credit: Duke University Marine Robotics and Remote Sensing Lab. C7) Incorporating climate scenarios, such as those related to coral reefs, can help generate restorations that may persist over longer timescales. Photo credit: Avigdor Abelson. C8)Incorporation of facilitation theory into salt marsh restoration (with mussels) can have positive outcomes. Photo credit: Chistine Angelini. C9) Paxton et al. found that built structures can be successful restoration tools. Photo credit: J. McCord/ECU-CSI. C10) Restoration efforts, such as oyster reef installation, require adequate funding not only for restoration implementation but also for planning, assessment, and adaptive management. Photo credit: Megan Saunders.
For example, in Florida, USA, where coastal habitat mosaics of seagrasses, oyster reefs, coral reefs, dune systems, and vegetated intertidal wetlands are in decline to varying degrees statewide, projects to restore these systems to date have been championed by state agencies, non-governmental organizations (NGOs), city and county governments, engineering firms, and, to a lesser degree, academic institutions (e.g., Bersoza Hernández et al., 2018). Each entity has a unique mission, distinct jurisdictional boundaries and constrained financial capacity. For instance, city governments often oversee coastal wetland and dune restoration projects as a strategy to stabilize shorelines eroding adjacent to critical municipal infrastructure. In contrast, Florida Fish and Wildlife Conservation Commission, a state agency, and Ducks Unlimited, an NGO focused on conserving and restoring wetlands and associated habitats for waterfowl. These disparate approaches yield local, and often isolated, gains in restoring habitats, while comprehensive, region-wide gains in coastal habitat spatial extent, functionality, and service provisioning are not being achieved.
To meaningfully move the needle, entities currently engaged in restoration must coordinate their activities and investments so they systematically build off of one another. For instance, collaboration by restoration practitioners with decision makers and legislators is needed to create effective legislation and permitting that will aid in scaling up restoration (Saunders et al., 2022). Simultaneously, new entities need to be brought to the table, particularly those able to plan larger regional projects (e.g., urban and regional planners), curate long-term funding portfolios (e.g., the financial sector), manage the logistics (e.g., project management specialists), construct at scale (e.g., civil and industrial engineering industries), and assess the functionality of such projects (e.g., sensor technology companies).
Concept 3. Co-production is key for more equitable and relevant outcomes
There has historically been a divide between the generators of restoration knowledge (i.e., researchers) and the users of the knowledge (i.e., practitioners, policy-makers), with little discussion regarding how the transfer of information between these entities should actually take place. This has resulted in a theory-practice gap (alternatively referred to as a research-implementation gap or knowledge-action gap, among others; Cooke et al., 2021). As highlighted in Concept #2, the coordination and engagement of diverse entities will be imperative for scaling up MER practice, but in particular, it will be important to improve communication and break down barriers between groups that are traditionally thought of as knowledge producers versus knowledge users (Saunders et al., 2022; Figure 2-C3). Co-production rejects the idea that scientists alone should be the ones to identify and solve complex problems, but instead that this should be a collaborative process carried out by academics and non-academics alike (Norström et al., 2020). Co-production is context dependent, problem-oriented, and leverages the knowledge and priorities of diverse stakeholders. (e.g., local communities, non-governmental organizations, tribes, etc.).
While co-production has been used in agricultural research for decades (Rocheleau, 1991; Brown, 1996), its application has been relatively limited in ecosystem restoration projects, despite the fact that it can likely enhance implementation and project outcomes and help to focus MER on locally relevant priorities or desired species. For example, Aquatic Habitat Toronto (AHT) is a unique partnership among multiple diverse agencies that are conducting restoration work along the Toronto waterfront in Canada. It includes multiple diverse stakeholders, multiple jurisdictional boundaries, and three levels of government. AHT supports the planning and implementation of various restoration projects conducted for a variety of different reasons and they facilitate knowledge sharing through co-production. The co-production process used by AHT enables early communication among partners about impending projects, broad knowledge sharing, the incorporation of local knowledge, and ultimately more successful restoration projects that incorporate novel techniques and advances (Piczak et al., 2022). However, co-production is not without its potential challenges. If there are practical constraints to the restoration design, they might conflict with stakeholder goals, and unequal power dynamics can compromise the design and implementation process, ultimately resulting in outputs that do not sufficiently meet stakeholder goals (Hastings et al., 2020). Moreover, co-production often requires large time commitments and financial resources and, therefore, securing long-term and stable funding will be critical for ensuring that co-production approaches are feasible and successful (Piczak et al., 2022; see Concept #10).
Concept 4. Spatial planning and ecosystem management must inform restoration design
Scaling up MER must be done using principles from spatial planning (Figure 2-C4). At present, most restoration projects are uncoordinated and site selection is somewhat opportunistic; that is, a location is identified as biophysically suitable and then an opportunity presents itself from a social-governance perspective (e.g., there is political will, a willing landholder, or potentially a loop-hole in permitting which enables a project to proceed; Saunders et al., 2022). Scaling up restoration to larger spatial extents aimed at achieving widespread social, economic, and environmental outcomes will require more systematic approaches to site selection and stronger coordination among projects (Lester et al.; Gleason et al., 2021). Such approaches have been developed over decades for spatial conservation planning and ecosystem management. For example, the design and re-design of Australia’s Commonwealth Marine Parks was done using science-based spatial planning approaches (Day et al., 2019).
Spatial planning involves clearly articulating stated goals and objectives, identifying actions that could be used to address those goals, and then estimating the benefits relative to the objective(s) which could be accrued for a given budget accounting for the likelihood that the project will succeed (Pressey et al., 2007; Gregory et al., 2012; Gleason et al., 2021). Spatial planning is informed by principles from the field of decision science and often includes cost-effectiveness or cost-benefit analyses. This approach is useful as it can help maximize benefits while minimizing costs and can help ensure that portfolios of benefits are accrued instead of all projects being conducted for the same outcome. Decision science can also help with identifying potential trade-offs, such as those between carbon sequestration vs. fisheries outcomes. In Lester et al., the authors review recent scientific peer-reviewed literature for several marine ecosystems to identify how site selection or spatial planning principles have been applied to MER over different spatial scales. Moving forward, there is an opportunity to advance the use and application of spatial planning principles to lead to better recovery of ecosystem function, benefit human communities, result in more efficient use of limited resources, and provide a platform for improved outreach and education.
Concept 5. The stressors that killed ecosystems must be reduced before restoring
Marine and coastal areas around the world are in decline because they are facing a consortium of stressors that are killing off foundational plants, animals, and algae as well as destabilizing food webs (Thomson et al., 2015; Borst et al., 2018). These stressors range from climate-related factors, such as higher water temperatures (Rosenberg and Ben-Haim, 2002; Collier and Waycott, 2014; Shields et al., 2019), more extreme drought (Silliman et al., 2005), more intensive storms (Greening et al., 2006), as well as human activity-related stressors, such as eutrophication (Smith, 2003; Burkholder et al., 2007), sewage pollution (Wear et al., 2021) overfishing (Eriksson et al., 2011), development (Bulleri and Chapman, 2010), boat wakes (Safak et al., 2021), industrial and emerging contaminants (Khan et al., 2022), and intensive recreational use (Hardiman and Burgin, 2010). Without exception, all of our coastal ecosystems are, to some extent, exposed to a suite of these climate- and human-related stressors (He and Silliman, 2019).
In order for coastal habitat restoration to be feasible, the portfolio of stressors must be constrained enough that the species we are seeking to restore can in fact survive, grow, and ideally reproduce (Figure 2-C5). Recent studies suggest that, if local, human-related stressors can be reduced through effective regulation, management, or infrastructure interventions, there is greater potential for habitats undergoing restoration to undergo succession, thereby regaining cover of foundation species and the food webs that depend on and facilitate them (Abelson et al., 2016; He and Silliman, 2019). For example, in coral reefs, experiments simulating the recovery of predatory fish and commensurate reduction in meso-predator snails can facilitate growth of resilience in corals (Shaver et al., 2018). In other regions, there is a huge need to reduce nutrient pollution and excessive sediment delivery to the coast before efforts to restore seagrasses are feasible. For example, Tampa Bay, Florida experienced a tremendous recovery of seagrasses after investments were made to reduce nutrient pollution to the bay, which resulted in increased water clarity and reduced incidence of hypoxia events, enabling seagrasses and their associated food webs to recover (Greening et al., 2011; Tomasko et al., 2018). In other instances, ecosystems can switch into alternative stable states because of the above-mentioned stressors or because of the loss of top-predators. For example, in the Mediterranean Sea, the expansion of urchin populations has changed the macroalgal dominated, rocky subtidal to a “barren” state and the restoration intervention of culling urchins has demonstrated promising results that can facilitate recovery and restoration success (Guarnieri et al.).
Thus, we call for a holistic, more inclusive perspective to MER that simultaneously considers restoration of target species and the systematic reduction of external stressors. However, we caution practitioners on waiting for the “ideal” conditions due to the rapid need for restoration and rather suggest joint efforts to improve conditions while restoring ecosystems. In some cases, environmental conditions, while in need of improvement, are not the limiting factors to successful restoration (see Orth et al., 2012 and Hughes et al., 2013) but rather an ecosystem wide approach that considers stock needs, trophic interactions, and environmental conditions can achieve cascading impacts that exceed handling singular issues at a time. Moving forward, decision science approaches and models (see Concept #4) can be used to assess where active marine restoration, protection, or mitigation of other stressors can best achieve coastal management objectives (Possingham et al., 2015; Saunders et al., 2017)
Concept 6. Technological advances must be rapid for MER to be a viable intervention
Rapid degradation of ecosystems shaped by habitat-forming species such as coral and bivalve reefs, vegetated coastal systems, and the increasing ambitions to halt and reverse these losses call for a rapid development and inclusion of technological advancements into restoration actions (Figure 2-C6). At present, restoration is failure-prone and expensive because the stability of ecosystems often hinges on self-facilitation generated by ‘emergent traits’ from habitat modifiers (Temmink et al., 2020). These are traits that are not expressed by an individual, but emerge at the aggregation level, causing self-facilitation (see Concept #9) to only work beyond certain minimum density thresholds. These emergent traits can be generated more rapidly when positive species interactions are incorporated (Shaver and Silliman, 2017; Reeves et al., 2020; Renzi et al., 2019; Valdez et al.; Zhang et al.). Technological advances using biodegradable structures that mimic valuable emergent traits can amplify restoration success while limiting the need for large amounts of donor material (Temmink et al., 2020; Temmink et al., 2021). For example, the artificial reef structures and built infrastructure in living shorelines can mimic and provide hard substrates needed for the restoration of mussel or oyster reefs (Dafforn et al., 2015; Mayer-Pinto et al., 2017; Temmink et al., 2021; Concept #9) or the facilitation of marsh or seagrasses (Temmink et al., 2020; Marin-Diaz et al., 2021). Additional examples of technologies that improve restoration techniques by ameliorating environmental stressors include the developments of bio-cements seeded with reef forming bivalves such as oysters or biodegradable matrices used to stabilize sediments for plant restoration (Marin-Diaz et al., 2021; Uddin et al., 2021). Moreover, recent work in coral reefs demonstrated how industry-scale restoration based on machinery and techniques derived from oil spill remediation, dredging operations, and aquaculture can yield effective harvesting, culturing, and outplacement of coral recruits (Doropoulos et al.). Another advancement in technology comes from the development and utilization of uncrewed systems. Uncrewed systems have the ability to assist in nearly every phase of a restoration project including site selection and planning, implementation, and monitoring (Ridge and Johnston). Combined, these recent advances highlight how inclusion of novel technologies allow for cross-scale improvements, from simple small-scale design changes to large-scale industry-derived approaches, paving the way towards effective low-cost restoration of marine ecosystems.
Concept 7. Future climate scenarios should permeate and inform restoration planning
It is beyond doubt that marine biodiversity will change in the future due to climate change. Impacts of climate change on marine ecosystems and the diversity they facilitate will occur in multifaceted ways, such as changes in CO2 concentrations, temperature, mixing regimes, and biogeochemical cycles of elements and organic compounds (Hillebrand et al., 2018). In addition, climate change driven modifications of marine organism performance, population size, and species inventory add up to the overall changes in marine biodiversity observed at the community and ecosystem level. For instance, temperature induced losses of a few marine species may cause a cascade of secondary extinctions up to a network collapse to half the initial network size (Jacob et al., 2011). Thus, to understand these causal relationships and their implications for ecosystem functioning, goods, and services, the response of the entire ecological network has to be analyzed (Woodward et al., 2010).
Projections of future climate change play a fundamental role in improving understanding of the climate system as well as characterizing societal risks and response options (Tebaldi et al., 2021). Indeed, modeling can help us to understand the biodiversity consequences of temperature change since range shifts alter regional marine diversity under altered temperature regimes. For example, we are currently observing a massive underwater “refugee crisis”, as species are shifting habitats due to ocean warming (i.e., poleward range shifts; Fogarty et al., 2017). Thus, the early detection of climate-driven range-extending species is important for marine restoration schemes, given the potential for alteration of ecosystem structure and functioning, as well as economic impacts and opportunities (Robinson et al., 2015). Modeling approaches to detect impending range shifts can thus forewarn potential ecosystem changes, identify relevant conservation or restoration strategies, and adapt natural resource management to moderate or take advantage of these effects (Dawson et al., 2011). For instance, it could be shown that increased diversity enhanced ecosystem functions and had a positive impact on ecosystem recovery after climatic extremes (Worm et al., 2006).
All restoration planning must consider future climate conditions at a respective site in order to ensure that the central motivations for MER can actually be met and maintained over thelong-term (Figure 2-C7; Coleman et al). Based on the availability and reliability of future climate data, the MER plan (i.e., promoted adaptation) can be decided (Abelson et al., 2016) either via the “Predict-and-Prescribe” approaches (e.g., assisted evolution and designer reefs; Webster and Reusch, 2017; Darling and Côté, 2018), which are based on predicting future conditions or the “Portfolio” approach, which considers the range of uncertainty of future conditions (Schindler et al., 2015; Webster and Reusch, 2017). It should be noted that whilst the two strategies are distinct, they may serve as complementary tools, to be applied simultaneously to increase the chances of recovery (Abelson et al., 2016).
Concept 8. Inclusion of facilitation theory increases foundation species regrowth
Positive species interactions, such as mutualism and facilitation, are powerful relationships that allow marine foundation species to increase their tolerances to physical stress, resist biological suppression, and recover faster after disturbance. For instance, salt marsh grasses are protected from grazers by predators (Silliman and Bertness, 2002), experience increased resistance to climate change from mussel mounds (Angelini et al., 2016), are protected from wave stress by oyster reefs, and can overcome severe oxygen limitation in saturated soils by benefiting from the infusion of oxygen into the soil around their roots by neighboring plants (Silliman et al., 2015) or burrowing crabs (Bertness, 1985). Every marine foundation species has a list of positive species interactions that underlies their large-scale success over seascapes. These key positive interactions are highlighted for kelp forests (Eger et al.), seagrasses (Valdez et al.), oyster reefs (Reeves et al.), salt marshes (Renzi et al., 2019) and mangroves (Renzi et al., 2019) in this Research Topic, and for coral reefs (Shaver and Silliman, 2017) in previous work.
Despite an understanding of the key role that facilitation plays in the regrowth and sustained success of marine foundation species, MER does not systematically incorporate these beneficial relationships when outplanting foundation species. Indeed, the current paradigm in restoration narrowly focuses on systematically reducing stressors like competition and physical stress before planting (Silliman et al., 2015; Zhang et al.). Experimental work presented in this Research Topic, and in other recent studies, highlights the massive benefit that incorporating positive species interactions into restoration designs can generate. For instance, adding clams to seagrass seed plantings increases their growth and expansion by over ~300% (Zhang et al.). Similarly, planting marsh plants, seagrasses, and mangroves in clumps instead of dispersed designs increases their wave stress and low oxygen tolerance (Silliman et al., 2015; Zhang et al.), and regrowth of seagrasses in the presence of sea otters switches from 100% failure to 100% success (Hughes et al., 2013). There is now overwhelming evidence of the generality of this positive effect across all marine foundation species. Marine ecosystem restoration must immediately expand its paradigm to include systematic inclusion of positive species interactions, or pay an unnecessary penalty of missing out on massive gains in restoration success and decreased costs (Figure 2-C8).
Concept 9. Built and hybrid structures are key tools in the restoration tool chest
Reefs are vital marine habitats as their spatially complex hard structures attenuate current and waves, serve as attachment substrate, and provide shelter, foraging, and nursing grounds for myriad species. These ecological functions are particularly important in otherwise structurally homogeneous soft-sediment systems, where both rocky, geogenic reefs and biogenic reefs, made up of reef-building corals, bivalves, or tubeworms act as biodiversity hotspots. Over recent centuries, reefs have experienced massive losses worldwide (Eddy et al., 2021). Natural reefs declined due to habitat destruction, limiting the influx of natural substrates such as wood via rivers due to damming, pollution, and global warming-related extreme events. At the same time, the abundance of artificial hard substrates from shipwrecks, the construction of offshore oil platforms, dikes, and dams greatly increased (e.g., Petersen and Malm, 2006; Ruiz et al., 2009; Bugnot et al., 2021). Moreover, this growth is expected to continue as coastal defense issues increase due to sea level rise, and offshore windfarm numbers rise to support the renewable energy transition (Bugnot et al., 2021).
When marine ecosystems, such as reefs, are degraded, built and hybrid structures may be necessary to restore such ecosystems (Figure 2-C9). Recent analyses revealed that artificial reefs are generally successful in emulating ecological functions of natural reefs (Paxton et al.). Artificial substrates in many cases serve as suitable settlement surfaces for sessile species in ways similar to natural ones (e.g., Temmink et al., 2021; Dodds et al., 2022). Moreover, when employed based on proper scientific assessments, artificial reefs can also closely mimic natural ones in terms of their fish communities (Paxton et al., 2022), although there is mixed evidence on ecosystem-level effects (Layman and Allgeier, 2020). Hence, artificial reef structures may become a key tool in the marine restoration tool chest to mitigate natural reef declines.
In addition to artificial reef structures, nature-based infrastructure such as living shorelines that incorporate restoration with built infrastructure can incur benefits and improved ecosystem services (Smith et al., 2021), although the terminology and designs can vary widely (Smith et al.). As artificial ecological enhancements may be undesirable in many natural areas and can introduce ecological risks (Heery et al., 2017), we suggest they may become useful and effective as: 1) ecological enhancements as part of coastal defense (Morris et al., 2018) and offshore wind projects (Degraer et al., 2020) where introduction of hard substrates will be unavoidable, and 2) re-introduction of hard substrate in habitat-limited areas (Paxton et al., 2022) or woody substrates to compensate for losses from riverine input (Wohl and Iskin, 2021).
Concept 10. The need for adequate funding to safeguard MER success
It is common knowledge among conservation scientists and practitioners that prevention (i.e., conservation) is substantially better than the cure (i.e., restoration; Layton et al.). The cost of restoration typically far exceeds the cost of implementing protected areas per unit area (Possingham et al., 2015). However, even Marine Protected Areas (MPAs), the main marine conservation tool, fall below the minimal needed area for successful restoration outcomes (O’Leary et al., 2016). Moreover, many MPAs fail to meet thresholds for effective management practices, where the most common shortfalls are in staff and financial resources (Edgar et al., 2014; Gill et al., 2017), and currently, 70 percent of MPAs are underfunded (IMPAC5, 2023). That said, once damage to ecosystems has occurred the implementation of protected areas may not be able to return to the site to a highly functioning state, for instance, where propagule supply is limited, or where physical conditions are no longer suitable. In these instances, active restoration may be the only (and therefore most cost-effective, even if costly on a per ha basis) means of meeting objectives to recover coverage and functioning of the target habitat (See Concept #4, Possingham et al., 2015; Saunders et al., 2017). Due to the relatively high costs there is a need for institutional and financial support in order to overcome environmental and ecological barriers to restore at scale (Eger et al.; Figure 2-C10).
As earlier noted (Concept #1), for restoration projects to be successful they should include three stages. This obligatory MER comprehensive approach may infer that in cases of insufficient budget the proposed MER projects should be discarded. That is, if a restoration project has been approved but with limited funding (i.e., significantly below the required budget), there is a dilemma with three alternative decision-making options for how to proceed. First, to decline the project, as it is predestined to failure. Second, to apply whatever tools, which are not optimal, but fit the budget limitations. The third option, which is the recommended for better chances of improvement, is to start the first MER phase (pre-launch assessment and planning) as well as some basic actions that can alleviate local anthropogenic impact (e.g., MPA designation, sustainable alternative livelihood of local stakeholders, etc.; Abelson et al., 2016). During these initial steps, a plan can be tailored following the below suggested carbon and biodiversity credits.
Beyond the traditional means of fundraising (e.g., grant proposals, donations, and governmental funds), there are two relatively new ways that are in their nascency, but may prove to be gamechanger tools to raise funding for MER projects. The first approach is to adopt the concept of carbon offsetting and the use of carbon credits.This area is already a rapidly expanding concept, which may be relevant for mangrove forests, seagrass meadows and tidal marshes, which are the most effective carbon sinks (Mcleod et al., 2011; Macreadie et al., 2022). Second, a similar and more recent approach with promising MER implications for all marine ecosystems, is biodiversity offsetting and credits. The concept of biodiversity offsetting (and the use of biodiversity credits) is controversial (Droste et al., 2022) and bears some challenges (e.g., difficulties ensuring the offset projects are equivalent to the biodiversity losses; false sense of security that encourages further development and overall loss of biodiversity). However, practically, it is very likely that biodiversity credits, like carbon credits, are to become tools for financing the protection and restoration of marine coastal ecosystems. Taken together and as highlighted in C2 focused on multi-sector collaboration, it is clear that the future capacity to scale MER approaches requires the inclusion of expertise in financial planning to design the framework for funding that can support large-scale and long-term ecosystem rejuvenation efforts.
Concluding remarks
Here, we describe ten key concepts (Figures 1, 2) that together form key pillars of an ‘inclusive MER paradigm.’ These concepts may be categorized into two levels of necessity for success and application generality. The first, obligatory general concepts, refer to basic concepts, the adoption of which is critical to the success of all MER projects (e.g., the MER comprehensive approach [see Concept #1-3]). Second, particular concepts, which refer to concepts that may be essential to the success of certain MER projects under specific ecological and social circumstances and ecosystem types (e.g., “Built structures are a key tool in the MER tool chest;” see Concept #9). We argue that adopting the relevant key concepts for either further development of the scientific basis of MER, or as a general guide in applied projects, can help in accelerating the success of restoration projects as well as the image, and consequently the fundraising options, of MER as a major conservation management approach.
Author contributions
BS conceived the manuscript. BS led the manuscript draft with contributions from all coauthors. AP, JM, AA, and BS made the figures. All authors reviewed and edited the manuscript. All authors read and approved the final manuscript.
Funding
We thank Duke RESTORE, the Stanback Family Foundation, and NSF grants to BS and Prince Albert II of Monaco Foundation to A. Abelson for supporting this manuscript. MS was supported by a Julius Career Award from CSIRO and SV was supported by a NSF graduate research fellowship (DGE- 2139754).
Acknowledgments
We thank T. Barnes, B. Puckett, and L. Poussard for thoughtful reviews of the manuscript. The views and conclusions contained in this document are those of the authors and should not be interpreted as representing the opinions or policies of the US Government, nor does mention of trade names or commercial products constitute endorsement or recommendation for use.
Conflict of interest
The authors declare that the research was conducted in the absence of any commercial or financial relationships that could be construed as a potential conflict of interest.
Publisher’s note
All claims expressed in this article are solely those of the authors and do not necessarily represent those of their affiliated organizations, or those of the publisher, the editors and the reviewers. Any product that may be evaluated in this article, or claim that may be made by its manufacturer, is not guaranteed or endorsed by the publisher.
References
Abelson A., Nelson P. A., Edgar G. J., Shashar N., Reed D. C., Belmaker J., et al. (2016). Expanding marine protected areas to include degraded coral reefs. Conserv. Biol. 30 (6), 1182–1191. doi: 10.1111/cobi.12722
Angelini C., Griffin J. N., van de Koppel J., Lamers L. P. M., Smolders A. J. P., Derksen-Hooijberg M., et al. (2016). A keystone mutualism underpins resilience of a coastal ecosystem to drought. Nat. Commun. 7 (1), Article 1. doi: 10.1038/ncomms12473
Bayraktarov E., Saunders M. I., Abdullah S., Mills M., Beher J., Possingham H. P., et al. (2015). The cost and feasibility of marine coastal restoration. Ecol. Appl. 26 (4), 1055–1074. doi: 10.1890/15-1077
Bersoza Hernández A., Brumbaugh R. D., Frederick P., Grizzle R., Luckenbach M. W., Peterson C. H., et al. (2018). Restoring the eastern oyster: How much progress has been made in 53 years? Front. Ecol. Environ. 16 (8), 463–471. doi: 10.1002/fee.1935
Bertness M. D. (1985). Fiddler crab regulation of spartina alterniflora production on a New England salt marsh. Ecology 66 (3), 1042–1055. doi: 10.2307/1940564
Borst A. C. W., Verberk W. C. E. P., Angelini C., Schotanus J., Wolters J.-W., Christianen M. J. A., et al. (2018). Foundation species enhance food web complexity through non-trophic facilitation. PloS One 13 (8), e0199152. doi: 10.1371/journal.pone.0199152
Bridgewater P., Loyau A., Schmeller D. S. (2019). The seventh plenary of the intergovernmental platform for biodiversity and ecosystem services (IPBES-7): A global assessment and a reshaping of IPBES. Biodiversity Conserv. 28 (10), 2457–2461. doi: 10.1007/s10531-019-01804-w
Brown D. (1996). Beyond farmer first: rural peoples knowledge, agricultural research and extension practice. Edited by Ian Scoones and John Thompson. London: intermediate technology, (1994), pp. 301, paperback £3.95, US28.50, ISBN 1-85339-237-5. Exp. Agric. 32 (1), 103–103. doi: 10.1017/S0014479700025916
Bugnot A. B., Mayer-Pinto M., Airoldi L., Heery E. C., Johnston E. L., Critchley L. P., et al. (2021). Current and projected global extent of marine built structures. Nat. Sustainability 4 (1), Article 1. doi: 10.1038/s41893-020-00595-1
Bulleri F., Chapman M. G. (2010). The introduction of coastal infrastructure as a driver of change in marine environments. J. Appl. Ecol. 47 (1), 26–35. doi: 10.1111/j.1365-2664.2009.01751.x
Burkholder J. M., Tomasko D. A., Touchette B. W. (2007). Seagrasses and eutrophication. J. Exp. Mar. Biol. Ecol. 350 (1), 46–72. doi: 10.1016/j.jembe.2007.06.024
Collier C. J., Waycott M. (2014). Temperature extremes reduce seagrass growth and induce mortality. Mar. Pollut. Bull. 83 (2), 483–490. doi: 10.1016/j.marpolbul.2014.03.050
Cooke S. J., Jeanson A. L., Bishop I., Bryan B. A., Chen C., Cvitanovic C., et al. (2021). On the theory-practice gap in the environmental realm: Perspectives from and for diverse environmental professionals. Socio-Ecological Pract. Res. 3 (3), 243–255. doi: 10.1007/s42532-021-00089-0
Dafforn K. A., Glasby T. M., Airoldi L., Rivero N. K., Mayer-Pinto M., Johnston E. L. (2015). Marine urbanization: An ecological framework for designing multifunctional artificial structures. Front. Ecol. Environ. 13 (2), 82–90. doi: 10.1890/140050
Darling E. S., Côté I. M. (2018). Seeking resilience in marine ecosystems. Science 359 (6379), 986–987. doi: 10.1126/science.aas9852
Dawson T. P., Jackson S. T., House J. I., Prentice I. C., Mace G. M. (2011). Beyond predictions: biodiversity conservation in a changing climate. Science 332 (6025), 53–58. doi: 10.1126/science.1200303
Day J. C., Kenchington R. A., Tanzer J. M., Cameron D. S. (2019). Marine zoning revisited: How decades of zoning the Great Barrier Reef has evolved as an effective spatial planning approach for marine ecosystem-based management. Aquat. Conservation: Mar. Freshw. Ecosyst. 29 (S2), 9–32. doi: 10.1002/aqc.3115
Degraer S., Carey D., Coolen J., Hutchison Z., Kerckhof F., Rumes B., et al. (2020). Offshore wind farm artificial reefs affect ecosystem structure and functioning: A synthesis. Oceanography 33 (4), 48–57. doi: 10.5670/oceanog.2020.405
Dodds K. C., Schaefer N., Bishop M. J., Nakagawa S., Brooks P. R., Knights A. M., et al. (2022). Material type influences the abundance but not richness of colonising organisms on marine structures. J. Environ. Manage. 307, 114549. doi: 10.1016/j.jenvman.2022.114549
Domínguez-Tejo E., Metternicht G., Johnston E., Hedge L. (2016). Marine Spatial Planning advancing the Ecosystem-Based Approach to coastal zone management: A review. Mar. Policy 72, 115–130. doi: 10.1016/j.marpol.2016.06.023
Droste N., Alkan Olsson J., Hanson H., Knaggård Å., Lima G., Lundmark L., et al. (2022). A global overview of biodiversity offsetting governance. J. Environ. Manage. 316, 115231. doi: 10.1016/j.jenvman.2022.115231
Duarte C. M., Agusti S., Barbier E., Britten G. L., Castilla J. C., Gattuso J.-P., et al. (2020). Rebuilding marine life. Nature 580 (7801), Article 7801. doi: 10.1038/s41586-020-2146-7
Eddy T. D., Lam V. W. Y., Reygondeau G., Cisneros-Montemaylor A. M., Greer K., Palomares M. L. D., et al. (2021). Global decline in capacity of coral reefs to provide ecosystem services. One Earth 4, 1278–1285. doi: 10.1016/j.oneear.2021.08.016
Edgar G. J., Stuart-Smith R. D., Willis T. J., Kininmonth S., Baker S. C., Banks S., et al. (2014). Global conservation outcomes depend on marine protected areas with five key features. Nature 506 (7487), Article 7487. doi: 10.1038/nature13022
Eriksson B. K., Sieben K., Eklöf J., Ljunggren L., Olsson J., Casini M., et al. (2011). Effects of altered offshore food webs on coastal ecosystems emphasize the need for cross-ecosystem management. AMBIO 40 (7), 786–797. doi: 10.1007/s13280-011-0158-0
Fogarty H. E., Burrows M. T., Pecl G. T., Robinson L. M., Poloczanska E. S. (2017). Are fish outside their usual ranges early indicators of climate-driven range shifts? Global Change Biol. 23 (5), 2047–2057. doi: 10.1111/gcb.13635
Gann G. D., McDonald T., Walder B., Aronson J., Nelson C. R., Jonson J., et al. (2019). International principles and standards for the practice of ecological restoration. Second edition. Restor. Ecol. 27 (S1), S1–S46. doi: 10.1111/rec.13035
Gill D. A., Mascia M. B., Ahmadia G. N., Glew L., Lester S. E., Barnes M., et al. (2017). Capacity shortfalls hinder the performance of marine protected areas globally. Nature. 543(7647), 665–669. doi: 10.1038/nature21708
Gleason M. G., Caselle J. E., Heady W. N., SaccOmanno V. R., Zimmerman J., McHugh T. A., et al. (2021). A structured approach for kelp restoration and management decisions in California Vol. 2021 (Arlington, Virginia: The Nature Conservancy). 54pp.
Greening H. S., Cross L. M., Sherwood E. T. (2011). A multiscale approach to seagrass recovery in Tampa Bay, Florida. Ecol. Restor. 29 (1–2), 82–93. doi: 10.3368/er.29.1-2.82
Greening H., Doering P., Corbett C. (2006). Hurricane impacts on coastal ecosystems. Estuaries Coasts 29 (6), 877–879. doi: 10.1007/BF02798646
Gregory R., Failing L., Harstone M., Long G., McDaniels T., Ohlson D. (2012). Structured decision making: A practical guide to environmental management choices (Wiley-Blackwell).
Grorud-Colvert K., Sullivan-Stack J., Roberts C., Constant V., Horta E Costa B., Pike E. P., et al. (2021). The MPA Guide: A framework to achieve global goals for the ocean. Science 373 (6560), eabf0861. doi: 10.1126/science.abf0861
Hardiman N., Burgin S. (2010). Recreational impacts on the fauna of Australian coastal marine ecosystems. J. Environ. Manage. 91 (11), 2096–2108. doi: 10.1016/j.jenvman.2010.06.012
Hastings Z., Ticktin T., Botelho M., Reppun N., Kukea-Shultz K., Wong M., et al. (2020). Integrating co-production and functional trait approaches for inclusive and scalable restoration solutions. Conserv. Sci. Pract. 2 (9), e250. doi: 10.1111/csp2.250
He Q., Silliman B. R. (2019). Climate change, human impacts, and coastal ecosystems in the anthropocene. Curr. Biol. 29 (19), R1021–R1035. doi: 10.1016/j.cub.2019.08.042
Heery E. C., Bishop M. J., Critchley L. P., Bugnot A. B., Airoldi L., Mayer-Pinto M., et al. (2017). Identifying the consequences of ocean sprawl for sedimentary habitats. J. Exp. Mar. Biol. Ecol. 492, 31–48. doi: 10.1016/j.jembe.2017.01.020
Hillebrand H., Brey T., Gutt J., Hagen W., Metfies K., Meyer B., et al. (2018). “Climate Change: Warming Impacts on Marine Biodiversity,” in Handbook on Marine Environment Protection: Science, Impacts and Sustainable Management. Eds. Salomon M., Markus T. (Springer International Publishing), 353–373. doi: 10.1007/978-3-319-60156-4_18
Hughes B. B., Eby R., Van Dyke E., Tinker M. T., Marks C. I., Johnson K. S., et al. (2013). Recovery of a top predator mediates negative eutrophic effects on seagrass. Proc. Natl. Acad. Sci. 110 (38), 15313–15318. doi: 10.1073/pnas.1302805110
IMPAC5 (2023). Vancouver BC Canada. Available at: https://www.impac5.ca/.
Jacob U., Thierry A., Brose U., Arntz W. E., Berg S., Brey T., et al. (2011). “The Role of Body Size in Complex Food Webs: A Cold Case,” in Advances in Ecological Research, vol. 45 . Ed. Belgrano A. (Academic Press), 181–223. doi: 10.1016/B978-0-12-386475-8.00005-8
Khan S., Naushad M. U., Govarthanan M., Iqbal J., Alfadul S. M. (2022). Emerging contaminants of high concern for the environment: Current trends and future research. Environ. Res. 207, 112609. doi: 10.1016/j.envres.2021.112609
Layman C. A., Allgeier J. E. (2020). An ecosystem ecology perspective on artificial reef production. J. Appl. Ecol. 57 (11), 2139–2148. doi: 10.1111/1365-2664.13748
Macreadie P. I., Robertson A. I., Spinks B., Adams M. P., Atchison J. M., Bell-James J., et al. (2022). Operationalizing marketable blue carbon. One Earth 5 (5), 485–492. doi: 10.1016/j.oneear.2022.04.005
Marin-Diaz B., Fivash G. S., Nauta J., Temmink R. J. M., Hijner N., Reijers V. C., et al. (2021). On the use of large-scale biodegradable artificial reefs for intertidal foreshore stabilization. Ecol. Eng. 170, 106354. doi: 10.1016/j.ecoleng.2021.106354
Mayer-Pinto M., Johnston E. L., Bugnot A. B., Glasby T. M., Airoldi L., Mitchell A., et al. (2017). Building ‘blue’: An eco-engineering framework for foreshore developments. J. Environ. Manage. 189, 109–114. doi: 10.1016/j.jenvman.2016.12.039
McAfee D., Costanza R., Connell S. D. (2021). Valuing marine restoration beyond the ‘too small and too expensive. Trends Ecol. Evol. 36 (11), 968–971. doi: 10.1016/j.tree.2021.08.002
Mcleod E., Chmura G. L., Bouillon S., Salm R., Björk M., Duarte C. M., et al. (2011). A blueprint for blue carbon: Toward an improved understanding of the role of vegetated coastal habitats in sequestering CO2. Front. Ecol. Environ. 9 (10), 552–560. doi: 10.1890/110004
Morris R. L., Konlechner T. M., Ghisalberti M., Swearer S. E. (2018). From grey to green: Efficacy of eco-engineering solutions for nature-based coastal defence. Global Change Biol. 24 (5), 1827–1842. doi: 10.1111/gcb.14063
Norström A. V., Cvitanovic C., Löf M. F., West S., Wyborn C., Balvanera P., et al. (2020). Principles for knowledge co-production in sustainability research. Nat. Sustainability 3 (3), Article 3. doi: 10.1038/s41893-019-0448-2
Obura D. O., DeClerck F., Verburg P. H., Gupta J., Abrams J. F., Bai X., et al. (2023). Achieving a nature- and people-positive future. One Earth 6 (2), 105–117. doi: 10.1016/j.oneear.2022.11.013
O’Leary B. C., Winther-Janson M., Bainbridge J. M., Aitken J., Hawkins J. P., Roberts C. M. (2016). Effective coverage targets for ocean protection. Conserv. Lett. 9 (6), 398–404. doi: 10.1111/conl.12247
Orth R. J., Moore K. A., Marion S. R., Wilcox D. J., Parrish D. B. (2012). Seed addition facilitates eelgrass recovery in a coastal bay system. Mar. Ecol. Prog. Ser. 448, 177–195. doi: 10.3354/meps09522
Paxton A. B., Steward D. N., Harrison Z. H., Taylor J. C. (2022). Fitting ecological principles of artificial reefs into the ocean planning puzzle. Ecosphere 13 (2), e3924. doi: 10.1002/ecs2.3924
Petersen J. K., Malm T. (2006). Offshore windmill farms: threats to or possibilities for the marine environment. AMBIO: A J. Hum. Environ. 35 (2), 75–80. doi: 10.1579/0044-7447(2006)35[75:OWFTTO]2.0.CO;2
Piczak M. L., Anderton R., Cartwright L. A., Little D., MacPherson G., Matos L., et al. (2022). Towards effective ecological restoration: Investigating knowledge co-production on fish–habitat relationships with Aquatic Habitat Toronto. Ecol. Solutions Evidence 3 (4). doi: 10.1002/2688-8319.12187
Possingham H. P., Bode M., Klein C. J. (2015). Optimal conservation outcomes require both restoration and protection. PloS Biol. 13 (1), e1002052. doi: 10.1371/journal.pbio.1002052
Pressey R. L., Cabeza M., Watts M. E., Cowling R. M., Wilson K. A. (2007). Conservation planning in a changing world. Trends Ecol. Evol. 22 (11), 583–592. doi: 10.1016/j.tree.2007.10.001
Reeves S. E., Renzi** J. J., Fobert E. K., Silliman B. R., Hancock B., DeAngelis B. M., Gillies C. L. (2020). Facilitating better outcomes: How positive species interactions can improve oyster reef restoration. Front. Mar. Sci. 7, 656.
Renzi J. J., He Q., Silliman B. R. (2019). Harnessing positive species interactions to enhance coastal wetland restoration. Structure, Functioning and Conservation of Coastal Vegetated Wetlands, 9.
Robinson L. M., Gledhill D. C., Moltschaniwskyj N. A., Hobday A. J., Frusher S., Barrett N., et al. (2015). Rapid assessment of an ocean warming hotspot reveals “high” confidence in potential species’ range extensions. Global Environ. Change 31, 28–37. doi: 10.1016/j.gloenvcha.2014.12.003
Rocheleau D. E. (1991). Participatory research in agroforestry: Learning from experience and expanding our repertoire. Agroforestry Syst. 15 (2–3), 111–137. doi: 10.1007/BF00120184
Rosenberg E., Ben-Haim Y. (2002). Microbial diseases of corals and global warming. Environ. Microbiol. 4 (6), 318–326. doi: 10.1046/j.1462-2920.2002.00302.x
Ruckelshaus M. H., Jackson S. T., Mooney H. A., Jacobs K. L., Kassam K.-A. S., Arroyo M. T. K., et al. (2020). The IPBES global assessment: pathways to action. Trends Ecol. Evol. 35 (5), 407–414. doi: 10.1016/j.tree.2020.01.009
Ruiz G. M., Freestone A. L., Fofonoff P. W., Simkanin C. (2009). “Habitat Distribution and Heterogeneity in Marine Invasion Dynamics: The Importance of Hard Substrate and Artificial Structure,” in Marine Hard Bottom Communities: Patterns, Dynamics, Diversity, and Change. Ed. Wahl M. (Springer), 321–332. doi: 10.1007/b76710_23
Safak I., Angelini C., Sheremet A. (2021). Boat wake effects on sediment transport in intertidal waterways. Continental Shelf Res. 222, 104422. doi: 10.1016/j.csr.2021.104422
Saunders M. I., Bode M., Atkinson S., Klein C. J., Metaxas A., Beher J., et al. (2017). Simple rules can guide whether land- or ocean-based conservation will best benefit marine ecosystems. PloS Biol. 15 (9), e2001886. doi: 10.1371/journal.pbio.2001886
Saunders M. I., Doropoulos C., Bayraktarov E., Babcock R. C., Gorman D., Eger A. M., et al. (2020). Bright spots in coastal marine ecosystem restoration. Curr. Biol. 30 (24), R1500–R1510. doi: 10.1016/j.cub.2020.10.056
Saunders M., Waltham A. P. N., Cannard T., Sheppard M., Fischer M., Twomey A., et al. (2022). A roadmap for coordinated landscape-scale coastal and marine ecosystem restoration. Report to the Reef and Rainforest Research Centre, Cairns, Queensland.
Schindler D. E., Armstrong J. B., Reed T. E. (2015). The portfolio concept in ecology and evolution. Front. Ecol. Environ. 13 (5), 257–263. doi: 10.1890/140275
Shaver E. C., Burkepile D. E., Silliman B. R. (2018). Local management actions can increase coral resilience to thermally-induced bleaching. Nat. Ecol. Evol. 2 (7), Article 7. doi: 10.1038/s41559-018-0589-0
Shaver E. C., Silliman B. R. (2017). Time to cash in on positive interactions for coral restoration. PeerJ 5, e3499. doi: 10.7717/peerj.3499
Shields E. C., Parrish D., Moore K. (2019). Short-term temperature stress results in seagrass community shift in a temperate estuary. Estuaries Coasts 42 (3), 755–764. doi: 10.1007/s12237-019-00517-1
Silliman B. R., Bertness M. D. (2002). A trophic cascade regulates salt marsh primary production. Proc. Natl. Acad. Sci. 99 (16), 10500–10505. doi: 10.1073/pnas.162366599
Silliman B. R., Schrack E., He Q., Cope R., Santoni A., van der Heide T., et al. (2015). Facilitation shifts paradigms and can amplify coastal restoration efforts. Proc. Natl. Acad. Sci. 112 (46), 14295–14300. doi: 10.1073/pnas.1515297112
Silliman B. R., van de Koppel J., Bertness M. D., Stanton L. E., Mendelssohn I. A. (2005). Drought, snails, and large-scale die-off of southern U.S. Salt marshes. Science 310 (5755), 1803–1806. doi: 10.1126/science.1118229
Smith V. H. (2003). Eutrophication of freshwater and coastal marine ecosystems a global problem. Environ. Sci. pollut. Res. 10 (2), 126–139. doi: 10.1065/espr2002.12.142
Smith C. S., Paxton A. B., Donaher S. E., Kochan D. P., Neylan I. P., Pfeifer T., et al. (2021). Acoustic camera and net surveys reveal that nursery enhancement at living shorelines may be restricted to the marsh platform. Ecol. Eng. 166, 106232. doi: 10.1016/j.ecoleng.2021.106232
Tebaldi C., Debeire K., Eyring V., Fischer E., Fyfe J., Friedlingstein P., et al. (2021). Climate model projections from the Scenario Model Intercomparison Project (ScenarioMIP) of CMIP6. Earth System Dynamics 12 (1), 253–293. doi: 10.5194/esd-12-253-2021
Temmink R. J. M., Angelini C., Fivash G. S., Swart L., Nouta R., Teunis M., et al. (2021). Life cycle informed restoration: Engineering settlement substrate material characteristics and structural complexity for reef formation. J. Appl. Ecol. 58 (10), 2158–2170. doi: 10.1111/1365-2664.13968
Temmink R. J. M., Christianen M. J. A., Fivash G. S., Angelini C., Boström C., Didderen K., et al. (2020). Mimicry of emergent traits amplifies coastal restoration success. Nat. Commun. 11 (1), Article 1. doi: 10.1038/s41467-020-17438-4
Thomson J. A., Burkholder D. A., Heithaus M. R., Fourqurean J. W., Fraser M. W., Statton J., et al. (2015). Extreme temperatures, foundation species, and abrupt ecosystem change: An example from an iconic seagrass ecosystem. Global Change Biol. 21 (4), 1463–1474. doi: 10.1111/gcb.12694
Tomasko D., Alderson M., Burnes R., Hecker J., Leverone J., Raulerson G., et al. (2018). Widespread recovery of seagrass coverage in Southwest Florida (USA): Temporal and spatial trends and management actions responsible for success. Mar. Pollut. Bull. 135, 1128–1137. doi: 10.1016/j.marpolbul.2018.08.049
Uddin M. J., Smith K. J., Hargis C. W. (2021). Development of pervious oyster shell habitat (POSH) concrete for reef restoration and living shorelines. Construction Building Materials 295, 123685. doi: 10.1016/j.conbuildmat.2021.123685
United Nations Convention on Biological Diversity, CONVENTION ON BIOLOGICAL DIVERSITY, IPBES (2022). Kunming-Montreal Global biodiversity framework (Version 1). Montreal, Canada. doi: 10.5281/ZENODO.3831673
Wear S. L., Acuña V., McDonald R., Font C. (2021). Sewage pollution, declining ecosystem health, and cross-sector collaboration. Biol. Conserv. 255, 109010. doi: 10.1016/j.biocon.2021.109010
Webster N. S., Reusch T. B. H. (2017). Microbial contributions to the persistence of coral reefs. ISME J. 11 (10), Article 10. doi: 10.1038/ismej.2017.66
Wittmer H., Krause G., Berghöfer A., Spiering nee Centgraf S., Büttner L., Rode J. (2021). Transformative change for a sustainable management of global commons — biodiversity, forests and the ocean. Recommendations for international cooperation based on a review of global assessment reports and project experience. UFZ-Bericht 3/2021 (Leipzig: Helmholtz Center for Environmental Research GmbH - UFZ) 154 pp. doi: 10.57699/7S83-7Z35
Wohl E., Iskin E. P. (2021). Damming the wood falls. Sci. Adv. 7 (50), eabj0988. doi: 10.1126/sciadv.abj0988
Woodward G., Benstead J. P., Beveridge O. S., Blanchard J., Brey T., Brown L., et al. (2010). Ecological networks in a changing climate. Adv. Ecol. Res. 42, 71–138. doi: 10.1016/S0065-2504
Keywords: adaptive management, ecosystem management, ecosystem restoration, ecosystem services, hybrid ecosystems, marine conservation
Citation: Silliman BR, Angelini C, Krause G, Saunders MI, Smith CS, Valdez SR, McLean JET, Paxton AB, Heide Tvd and Abelson A (2023) Editorial: Marine ecosystem restoration (MER) – a call for a more inclusive paradigm. Front. Mar. Sci. 10:1250022. doi: 10.3389/fmars.2023.1250022
Received: 29 June 2023; Accepted: 28 July 2023;
Published: 12 September 2023.
Edited and Reviewed by:
Cristina Gambi, Marche Polytechnic University, ItalyCopyright © 2023 Silliman, Angelini, Krause, Saunders, Smith, Valdez, McLean, Paxton, Heide and Abelson. This is an open-access article distributed under the terms of the Creative Commons Attribution License (CC BY). The use, distribution or reproduction in other forums is permitted, provided the original author(s) and the copyright owner(s) are credited and that the original publication in this journal is cited, in accordance with accepted academic practice. No use, distribution or reproduction is permitted which does not comply with these terms.
*Correspondence: Brian R. Silliman, QnJpYW4uU2lsbGltYW5AZHVrZS5lZHU=
†ORCID: Brian R. Silliman, orcid.org/0000-0001-6360-650X
Christine Angelini, orcid.org/0000-0002-6669-5269
Gesche Krause, orcid.org/0000-0001-7917-7121
Megan I. Saunders, orcid.org/0000-0002-8549-5609
Carter S. Smith, orcid.org/0000-0002-6652-3010
Stephanie R. Valdez, orcid.org/0000-0002-3497-751X
Avery B. Paxton, orcid.org/0000-0002-4871-9167
Avigdor Abelson, orcid.org/0000-0001-6577-5948