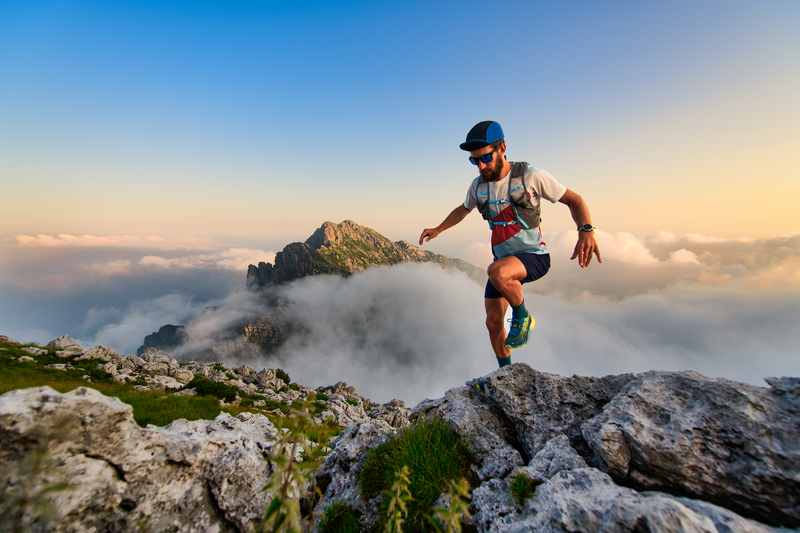
95% of researchers rate our articles as excellent or good
Learn more about the work of our research integrity team to safeguard the quality of each article we publish.
Find out more
ORIGINAL RESEARCH article
Front. Mar. Sci. , 20 November 2023
Sec. Marine Pollution
Volume 10 - 2023 | https://doi.org/10.3389/fmars.2023.1249964
This article is part of the Research Topic Shipping Pressures and Impacts on the Marine Environment View all 8 articles
Shipping is a large industry responsible for atmospheric emissions of hazardous substances including SOX, NOX, and particulate matter. Many ships have installed exhaust gas cleaning systems (scrubbers) to remove primarily SOX from the exhaust, but the hazardous substances are instead transferred to the water used in the scrubbing process. Ships with closed-loop scrubbers recirculate the water but can still discharge around 126-150 m3 directly to the surrounding marine environment every day. The discharged water contains metals and organic substances, such as polycyclic aromatic hydrocarbons, that are known to be toxic to marine zooplankton. Here we show that closed-loop scrubber washwater is toxic to communities of marine mesozooplankton at our lowest tested dilution, 1.5% (v/v), and affects survival, reproduction, diversity, and ability to predate on microzooplankton. The cumulative toxic unit of the undiluted closed-loop scrubber washwater was estimated to 17, which indicates that the water could be toxic at levels below what was tested in this study. Among all detected substances, vanadium, copper, benzo[ghi]perylene, nickel, and zinc were identified as toxicity-driving substances in the order listed. Closed-loop scrubber washwater has been shown to affect development and survival in single species of copepods, but here we find evidence of toxicity at the community level, irrespective of seasonal community structure, and that the exposure has potential to disrupt the interactions between trophic levels in the pelagic food web. We show that the closed-loop scrubber washwater cause both lethal and sublethal effects in marine zooplankton, due to contaminants, some of which are persistent in the marine environment.
Shipping is a large industry responsible for transporting more than 80% of all world trade (UNCTAD, 2021), and given this magnitude, it is important to assess and reduce the impacts it has on the marine environment. With the intention of reducing hazardous emissions to air through the ship exhaust, linked to adverse effects on both environment and on human health (Hassellöv et al., 2013; Jonson et al., 2015), the International Maritime Organization (IMO) has stepwise lowered the allowed sulphur content in marine fuels, most recently in 2020 (IMO, 2008). To comply with these stricter regulations, an increasing number of ships have installed exhaust gas cleaning systems, also known as scrubbers. In a scrubber, the exhausts are sprayed with water to remove sulphur oxides, and thus ships equipped with scrubbers can continue to run on fuels with high sulphur content and still meet the IMO requirements (Ushakov et al., 2020). However, particles and hazardous substances are transferred from the exhaust to the washwater used in the scrubbing process, and this water has been shown to contain sulphur, metals and organic substances including polycyclic aromatic hydrocarbons (PAHs) that originate from fuel combustion (Turner et al., 2017; Teuchies et al., 2020).
Until 2015 there were only a handful of ships equipped with scrubbers, and since then there has been a rapid increase in installations. By the end of 2022, approximately 4800 ships of the global fleet had scrubbers installed, 19% of which are hybrid and closed-loop, and 81% of which are open-loop (DNV-GL, 2023). Open-loop scrubbers use naturally alkaline seawater, which is continuously discharged back to the sea after the scrubbing process, and can discharge volumes of washwater in the range of 24 000-33 000 m3d-1 (based on a medium sized ship, 15 MW) (Hermansson et al., 2021). Closed-loop scrubbers typically use freshwater with an added base (often NaOH), and recirculates the water in the system, whereas the hybrid scrubber can alternate between both modes (Teuchies et al., 2020).
There is a growing concern regarding the impact on the marine environment following wide-scale use of scrubbers (Endres et al., 2018; Ytreberg et al., 2019; Hassellöv et al., 2020). Many ports and states have banned discharge of open-loop scrubber water, which has been shown to be a major contributor to contaminants in certain areas (Hassellöv et al., 2020; Ytreberg et al., 2022), and hence there is now an increased interest in the use of closed-loop scrubbers. Although these systems recirculate the water used in the scrubbing process, most systems have a bleed-off of washwater in the range of 126-150 m3d-1 (based on a medium sized ship, 15 MW) (Hermansson et al., 2021) that generally contain much higher concentrations of metals and organic pollutants than wash water from the open-loop scrubber (Thor et al., 2021). There is no threshold for metal content of the discharged scrubber water, whereas organic chemicals are monitored with a threshold based on the fluorescence of phenanthrene (PAHphe). This works as a proxy for PAH contamination and allows a maximum discharge of 50 µg/l PAHphe above the inlet water (MEPC, 2015).
Mixtures of substances are known to typically cause at least additive toxicity, and sometimes synergistic responses (Nørgaard and Cedergreen, 2010; Cedergreen, 2014). Even when each substance is present below its respective No Observed Effect Concentration (NOEC), a mixture can cause substantial toxicity (Walter et al., 2002). This additive toxicity has been empirically shown with a mixture of several PAHs, including those present in scrubber water, that adversely affected the small crustacean zooplankter Oithona davisae (Barata et al., 2005). One of the models that are routinely used to model toxicity in mixture risk assessment is the concentration addition (CA) model (Kienzler et al., 2016). CA is based on toxic units (TUs), i.e. ratio of concentration to effect concentration of each individual mixture component, and has been found to accurately predict toxicity of chemical mixtures to aquatic organisms within a factor of 2 (Deneer, 2000; Spilsbury et al., 2020).
Zooplankton are some of the organisms that come into direct contact with the discharged scrubber water, particularly close to shipping lanes and ports. Open-loop scrubber washwater has been shown toxic to marine copepods Acartia tonsa and Calanus helgolandicus at 1-10% (v/v) (Koski et al., 2017; Thor et al., 2021), but closed-loop scrubber washwater (CLSW) has been shown to increase mortality rate and reduce development in C. helgolandicus already at 0.1% (v/v) (Thor et al., 2021). These smaller copepod species are common in coastal areas, where they can feed on both microalgae and microzooplankton (Vargas and Gonzáles, 2004; Gismervik, 2006), although some species can switch between them or even prefer ciliates (Wiadnyana and Rassoulzadegan, 1989; Gismervik, 2006).
Mesozooplankton, such as copepods, have been shown to exert a strong predation pressure on microzooplankton, which in the absence of predators can increase quickly in numbers (Calbet and Saiz, 2005; Löder et al., 2011). This top-down control, as well as species-specific preference for ciliates, suggests that the loss or reduction of individual copepod species in the mesozooplankton community can affect the structure of the microzooplankton community, whether it be due to exposure to contaminants like scrubber water or due to other stressors.
Marine zooplankton communities vary in composition during the year (Chiba et al., 2006), and this change in diversity has been found to influence their sensitivity to contaminants (Payne et al., 2014). Feeding strategy in zooplankton species has also been found to influence the accumulation of contaminants, where predatory species accumulate more than herbivores (Hallanger et al., 2011), hence it is important to study the effects on contaminants on entire communities of different seasonal compositions as some species can be more affected than other ones.
The aim of this study was to test the toxicity of the CLSW to marine mesozooplankton communities across several seasons, to characterize the chemical content of the CLSW, and to identify the toxicity-driving substances. We exposed mesozooplankton communities to two concentrations of CLSW (1.5% and 3%) at three different seasons, and tested how their ability to reproduce, predate on microzooplankton, and survive were affected.
We exposed mesozooplankton communities to closed-loop scrubber washwater (CLSW) and collected copepod eggs at the end of the incubation to estimate effects on the community copepod reproduction. After the incubation, we moved the mesozooplankton to seawater (containing microzooplankton) to evaluate how the pre-exposure to CLSW affected their feeding on the ciliates in the microzooplankton community. The experiment was repeated three times during November, March and June to find out if the effects were consistent despite different seasonal species compositions at each trophic level (Table 1). The concentrations chosen reflected a concentration range where effects on single species have occurred.
Table 1 Experimental conditions and start abundances of mesozooplankton (ind/L-1) and microzooplankton (ind L-1) in each of the three experiments.
CLSW was retrieved from a ship equipped with a closed-loop scrubber system in September 2020. The CLSW was stored in a plastic container and kept in dark conditions at approximately 5°C until the end of the third experiment, nine months after receiving the CLSW.
The alkalinity and pH of the undiluted CLSW were determined two months prior to the first experiment. The initial (0 h) alkalinity and pH of each treatment were determined at each experiment from separately prepared bottles with the same concentration as the experimental bottles, but without algae culture and zooplankton (n=5). Finally, alkalinity and pH were determined in all replicates of all treatments at the end of the scrubber exposure phase (72 h). pH measurements were corrected to the total scale (pHT) using standard curves of TRIS (Tris/HCl) and AMP (2-aminopyridine/HCl) buffer solutions with a salinity of 25 PSU. Alkalinity was measured by titration (TA05 plus/TW alpha plus, SI Analytics, Mainz, Germany).
Samples of undiluted CLSW were sent for analysis of metals and organic chemicals (that have previously been detected in CLSW, e.g. Thor et al., 2021) to an accredited laboratory (ALS Scandinavia AB, Danderyd). The CLSW was analyzed prior to each experiment to ensure that the chemical components of the CLSW were stable throughout the storage. Validation samples were collected from at least one replicate of each treatment in each experiment at the start (0 h) and end (72 h) of the respective exposures.
The water was analyzed for metals according to the SS-EN ISO 17294-2:2016 and US EPA Method 200.8:1994 standards (ALS Scandinavia AB, package V-5-bas). The water samples were acidified with 1 ml HNO3 per 100 ml prior to analysis.
The water was also analyzed for organic chemicals with GC-MS (ALS Scandinavia AB, package OV-21H in November and OV-21B in March and June) including aliphatic, aromatic and polyaromatic hydrocarbons. Water from the March and June experiments were also analyzed for additional monoaromatic hydrocarbons that could have been present in the CLSW.
Toxicity data for zooplankton was retrieved from the ECOTOXicology Knowledgebase (accessed on [2022-05-04]) (Olker et al., 2022). Hazard data for freshwater and marine meso and microzooplankton with exposure lengths of<3 days were included, and only concentrations that caused 50% effect on the organisms were included (EC50, ED50, IC50, ID50, LC50, LD50). The mean of each effect concentration, together with the mean detected concentrations of each substance in the undiluted CLSW, was used to calculate a cumulative toxic unit (TU) of the scrubber water. The cumulative (TU) of the scrubber water was defined using the principles of CA by calculating the sum of each TU, i.e. the ratio of concentration () to effect concentration (), for each individual substance () in a mixture. This cumulative ratio indicates whether a mixture is close to a concentration that causes toxicity at the level of chosen effect concentration (). The cumulative TU was defined as:
Experiments were conducted using the same CLSW in November 2020, March 2021, and June 2021 (Table 1) to capture effects related to seasonal differences in composition of zooplankton. The ambient conditions during each of the experiments were selected to reflect the water temperature and the light regime during the respective season (Table 1).
Mesozooplankton communities (0.2-20 mm) were collected from the Gullmar fjord near the monitoring station Släggö (N 58° 15.5’, E 11° 26.0’). The communities were sampled with vertical tows from 0-30 m using a 200 µm plankton net fitted with a plastic bag at the end, and resuspended in filtered seawater (0.2 µm; FSW). The communities were acclimated to the experimental conditions in a thermoconstant room with natural ambient conditions for 24 h prior to the start of each experiment (Table 1). The water was aerated lightly, and the mesozooplankton were fed at excess with a mixture of the cryptophyte Rhodomonas salina (GUMACC, strain no 126) and the diatom Conticribra weissflogii (GUMACC, strain no 162).
Seawater with microzooplankton (20-200 µm) was collected with a 1.7 L Ruttner water sampler (Hydro-X, Swedaq) near the Släggö station from approximately 2 m depth. Subsamples were collected from the water during each of the seasons and checked using a microscope (Leica Leitz Labourlux S) to confirm ciliate presence. The water was gently siphoned through a submerged 200 µm filter to remove the larger zooplankton, and was then stored at the respective experimental conditions for approximately 6 h before the start of the second phase of each experiment.
The mesozooplankton communities were exposed to two levels of CLSW and a negative control in 2 L glass bottles with light aeration for 72 h (Control, 1.5%, 3%, n=5) (Figure 1). The mesozooplankton were carefully concentrated on a 200 µm mesh submerged in FSW, and transferred to a glass bottle from which subsamples were collected using a 10 ml pipette with a wide-opening tip. The subsamples were fixed in ethanol and counted on a stereo microscope in order to determine the approximate copepod concentration in the community, however, this data is not shown and was not used in any analyses in this study. Aliquots of the concentrated community were then distributed to each experimental bottle to ensure a community containing approximately 60-80 copepodites and adults per liter (Table 1).
Figure 1 Overview of the experimental setup during the scrubber exposure phase and the trophic interaction phase of each experiment. CLSW = closed-loop scrubber washwater. The scrubber exposure phase has a duration of 72 h and the trophic interaction phase started directly after this phase and lasted for another 24 h. .
C. weissflogii culture was retrieved from Gothenburg University marine algae culture collection (GUMACC, strain no 162), and the communities were fed at excess with 600 µg carbon/l based on equivalent spherical diameter (11.9 µm; average between the three experiments) at the experimental start. The algae concentration was determined every 24 h from measurements on a particle counter (Beckman Coulter Z2 Particle Cell Counter & Size Analyzer), and new culture was added to maintain a 600 µg carbon/l concentration in each bottle.
The mesozooplankton were carefully filtered out on a 200 µm mesh submerged in FSW after 72 h of exposure. Copepod eggs that were produced by the broadcast spawning copepods during these 72 h were filtered out on a 60 µm mesh from the remaining water, and transferred to plastic petri dishes with FSW. The eggs were left to hatch for another 72 h under the same ambient conditions, and then fixed in 15 ml L-1 acidified Lugol’s solution to estimate the total egg production and hatching success of the broadcast spawning copepods in the community.
After the exposure phase, the mesozooplankton were transferred to 2 L polycarbonate bottles with seawater containing microzooplankton (Figure 1). 1 ml L-1 EDTA was added to each bottle to detoxify metal ions naturally occurring in the seawater to ensure normal microzooplankton growth during the incubation. The bottles were incubated for 23 h on a plankton wheel together with 250 ml control bottles containing microzooplankton only (ciliate controls). At the end of the incubation, the mesozooplankton were stained in neutral red and fixed in a 2:8 glycerol:FSW mix for live/dead assessment and frozen at -18°C (November), or fixed in a 5:95 glycerol:ethanol mix and kept at 5°C (March and June). To ensure a quick fixation of the microzooplankton, 200 ml subsamples were collected in dark bottles pre-filled with 3 ml acidified Lugol’s solution at a final concentration of 15 ml L-1 for later identification of the ciliates. Start samples were collected and fixed from separately prepared bottles of both meso- and microzooplankton communities in order to characterize the communities prior to the exposure.
The neutral red fixed mesozooplankton samples were thawed to room temperature and counted using a Leica Wild M8 stereo microscope on an illuminated stand. Dead and alive individuals were separated based on neutral red uptake and copepods were categorized into male, female, copepodite or nauplius. The ethanol fixed samples were transferred to filtered seawater and counted using the same approach, with the exception that any intact individual was considered alive. Only live individuals were included in the further analyses. Copepod eggs and nauplii were counted using a Leica Wild M8 stereo microscope on an illuminated stand, and the ciliates in the microzooplankton samples were counted in 50 ml Utermöhl chambers with an inverted microscope (Leica Leitz DM IL). All mesozooplankton, copepod eggs, and copeopod nauplii were counted in each replicate, and all ciliates in a 50 ml subsample from each replicate were counted. Community copepod egg production was estimated by adding the number of unhatched eggs and nauplii in each sample, and hatching success was estimated by dividing the number of nauplii by the total number of unhatched eggs and nauplii. Taxonomy of microzooplankton was determined based on reference literature (Enckell, 1980; Gissel Nielsen and Hansen, 1999; Larink and Westheide, 2011) relevant to the Baltic and North Sea, and in dialogue with personnel working with identification of zooplankton species for the Swedish monitoring program. Species identification was made to species level, when possible, or to genus followed by sp. or spp. accordingly.
All data were analyzed using R Version 1.4.1717 (© 2009-2021 RStudio, PBC) with the exception of the SIMPER analyses and Analysis of Similarities (ANOSIMs) that were performed using PRIMER version 7.0.21 (Clarke and Gorley, 2015). The differences between meso- and microzooplankton abundances, copepod egg production and hatching success in control and treatments were analyzed with one-way ANOVAs and Dunnett’s post-hoc tests. The assumptions of residual normality and homogeneity of variances were checked visually from residual and Q-Q plots. The heat plots and clusterings (hierarchical complete-linkage) were performed using the package pheatmap (version 1.0.12). The effects of CLSW exposure on mesozooplankton diversity, and the indirect on microzooplankton diversity were tested using ANOSIMs based on Bray-Curtis dissimilarity. The beta diversity (compositional differences between treatments) was visualized through an NMDS. The SIMPER analysis and the Bray-Curtis distances on which the ANOSIM run were both based on untransformed taxa abundances of live individuals of all life stages.
The undiluted closed-loop scrubber washwater (CLSW) contained ten individual metals and 15 individual organic substances (Table S1) and had a pHT of 6.9 and an alkalinity of 11 030 mmol/l (Table S2). Several aromatic and aliphatic substances could not be individually identified in the CLSW, and were instead grouped based on number of carbon atoms. None of the analyzed organic substances were detected in the control water used in the experiments, whereas all metals except for cadmium and lead were detected, nevertheless at substantially lower levels than in the CLSW (Table S1).
Ecotoxicity data was found for 19 out of the 25 detected individual metals and organic substances in the ECOTOX database, and the cumulative toxic unit (TU) for the combination of these in the undiluted CLSW was estimated to 17 (Figure 2). This corresponds to TUs of 0.26 and 0.5 for the 1.5% and 3% treatments respectively. Vanadium was the main toxicity-driver and contributed with 57% of the total toxicity followed by copper (26%), benzo[ghi]perylene (BghiP) (11%) and nickel (5%). These four substances were together responsible for >99% of the total toxicity of the CLSW based on the detected individual chemicals.
Figure 2 The cumulative toxic unit of the undiluted closed-loop scrubber washwater based on ranked individual toxic units of the detected chemicals. The dashed line marks the total cumulative toxic unit.
The analysis of scrubber water contaminants at the start and end of each experiment generally confirmed the nominal dilutions of 1.5% and 3%, with the exeption of the aromatic substances (Figure S1). Many of these substances were not detected in the diluted treatments since their concentrations in the undiluted CLSW were close to their respective detection limits. The pH and alkalinity in the controls varied between 8.1-8.4 depending on season and was reduced by 0.1-0.2 in the 1.5% treatment and by 0.3-0.5 in the 3% treatment at the start of the exposures (Table S2).
The total copepod community egg production varied depending on season but the relative differences between control and CLSW treatments were similar, except for in March (Figure 3A). The egg production was increased to 127% and 113% in the 1.5% CLSW treatment during November and June and reduced to 78% in March, but none of them were significantly different from the control (November p=0.17, March p=0.41, June p=0.78, Table S3). However, the 3% CLSW treatment had a substantial effect on the community egg production which was reduced to 35-42% (p<0.05, Table S3).
Figure 3 Effects of closed-loop scrubber washwater on copepod reproduction during all three seasons. (A) Copepod community egg production shown as % of control mean (mean ± 95% confidence interval, n=5), and (B) hatching success displayed as % hatched eggs of the total amount of eggs produced during the exposure (mean ± 95% confidence interval, n=5). The mean copepod community egg production was 452, 103, and 734 respectively for the months November, March, and June. The asterisks above the bars indicate significant differences from the respective control (0%) treatments (p-values:<0.001 ‘***’,<0.01 ‘**’,<0.05 ‘*’).
Hatching success of the eggs was between 76-80% in the control during all seasons (Figure 3B). The CLSW reduced the viability of the eggs independent of season starting at 1.5% with a hatching success between 36-57% (p<0.05, Table S3). The viability was even more reduced by 3% CLSW where the hatching success was as low as 3-27% (p<0.05, Table S3).
Survival in the control ranged between 55% (November) and 76% (June), and copepod survival after CLSW exposure was significantly lower than the control at all instances (p<0.05), except after the 1.5% exposure in November where it was lower but not significantly (p=0.12, Table S4). The magnitude of the reduced survival depended on season with 8-43% lower survival than control in the 1.5% treatment, and 19-32% lower than control l in the 3% treatment (Figure 4). The survival was generally lower from 3% exposure than from 1.5% exposure, apart from in March.
Figure 4 Copepod and ciliate densities prior to and after exposure during each season. Densities are displayed as percent of start mean (mean ± 95% confidence interval, n=5) in (A) November, (B) March and (C) June. Start treatment represents abundances prior to exposure, ciliate treatment represents bottles incubated with pure seawater without mesozooplankton, and 0-3% represents the concentration of closed-loop scrubber washwater in the experimental bottles. The mean start concentrations of copepods/l (including copepodites and nauplii) were 63, 208 and 80 respectively for the months November, March, and June. The mean start concentrations of ciliates/ml were 1.8, 3.7, and 2.0 respectively for the months November, March, and June. The asterisks above the bars indicate significant differences from the respective control (0%) treatments (p-values:<0.001 ‘***’,<0.01 ‘**’,<0.05 ‘*’).
Ciliate densities (as % of the mean density prior to exposure) followed an inverse pattern to the abundance of surviving copepods during all three seasons, with the most prominent pattern in November and March (Figure 4). The ciliate abundance was 8-26% higher after pre-exposure to 1.5% CLSW, and 15-61% higher after pre-exposure to 3% CLSW (Figure 4). The increase of ciliates relative to the control was significant during November and March (p<0.05, Table S4), but not during June (1.5% p=0.44, 3% p=0.09, Table S4), although the pattern was similar.
The mesozooplankton diversity was affected from 1.5% CLSW exposure in March and June, and from both 1.5% and 3% CLSW in November (Figures 5A–C, Table S5, Figure S2). The copepod species Paracalanus parvus and Temora longicornis were less sensitive to the CLSW and were present in larger relative proportions after the exposure, whereas Centropages hamatus, Centropages typicus and Calanus spp. were more sensitive and present in smaller proportions (Tables S6–S7). The sensitivity of A.clausi depended on season and was less sensitive in November and more sensitive in June. The sensitivity of each copepod taxa appeared to be closely linked to the developmental stage, as C. typicus, Calanus spp. and A. clausi each had the highest abundance of nauplii or copepodites during the seasons when they were most affected by the CLSW (Figure 6).
Figure 5 NMDS visualizing community diversity during each month for mesozooplankton in (A) November, (B) March, and (C) June, and for microzooplankton in (D) November, (E) March and (F) June. Distances are based on Bray-Curtis dissimilarity using non-transformed percentages of taxa in each sample.
Figure 6 Abundances (ind/L-1) of the respective taxa at the start of the respective experiments. (A) Juveniles in November (B) juveniles in March (C) juveniles in June (D) adults in November (E) adults in March, and (F) adults in June. Bars show mean ± 95% confidence interval.
Changes in mesozooplankton diversity had a moderate effect on microzooplankton diversity. Significant effects were observed for the 1.5% CLSW in November and for the 3% CLSW in March (Figures 5D–F, Table S5, Figure S2). The ciliate Strombidium spp. generally increased in numbers relative to other taxa when mesozooplankton had been pre-exposed to CLSW (Tables S6–S7). The average abundance and variation of each mesozooplankton and microzooplankton taxa prior to and after the incubations can be found in Tables S8–10.
Our findings demonstrate that closed-loop scrubber washwater (CLSW) is toxic to marine mesozooplankton. The cumulative toxic unit (TU) for the undiluted CLSW indicates that the combination of the individually identified substances in the CLSW is 17 times the concentration that affects 50% of a population. However, ecotoxicity data could not be included for all substances in the CLSW, so it cannot be excluded that more substances contribute to the overall toxicity, in which case the cumulative TU would increase.
Vanadium, Ni and Cu are all metals with TUs larger than 1 in the undiluted CLSW, indicating that their respective individual concentrations are high enough for them to be toxic to zooplankton. The concentrations of these metals in the CLSW vary across different studies and are sometimes in the same order of magnitude as what was detected in this study, but sometimes substantially lower (Hermansson et al., 2021; Thor et al., 2021). V, Ni and Cu are all found at high concentrations in particulate matter (PM2.5) in ship exhaust from ships running on residual fuel oils such as intermediate fuel oil (IFO) and heavy fuel oil (HFO) (Celo et al., 2015; Corbin et al., 2018), and can be transferred from the exhaust to the water recirculated in the closed-loop scrubber. Total emission factors for metals such as Cu and Zn have been shown to be higher when using an open-loop scrubber together with HFO, than when running on HFO alone (Hermansson et al., 2021), which may be attributed to use of sacrificial anodes for marine growth protection, lubrication oils and corrosion in the piping system caused by the acidic open-loop scrubber water (Kim and Jeong, 2019). The CLSW is less acidic than the open-loop scrubber water, so the contribution from corrosion should be smaller in comparison to use of open-loop scrubbers.
The only organic substance with a TU above 1 in the undiluted CLSW was BghiP, which is one of many PAHs that are found in the exhaust when ships run on HFO (Sippula et al., 2014). The concentration of BghiP in our study was 1.1 µg/l, which is higher than the 0.031 µg/l and 0.07 µg/l that have been detected in other CLSW (Hermansson et al., 2021; Thor et al., 2021). The differences in both metal and PAH content of the CLSW in our study, relative to other studies, indicate that the composition of the water is highly variable, which could be attributed to engine load (to which capacity the engine is run at) and fuel type (Sippula et al., 2014).
The PAH content in the scrubber water is regulated by the PAHphe threshold, which is based on optical online sensor measurements of UV or fluorescence detection of phenantrene (MEPC, 2015). However, our analysis of the CLSW content shows that this is neither the most abundant PAH nor the most toxic one, which suggests that this type of threshold is unsuitable for ensuring that the scrubber water is safe to discharge. The PAHphe has previously been criticized by GESAMP (Group of Experts on the Scientific Aspects of Marine Environmental Protection) because of its vague definitions and methodology for determination as well as questionable level of protection (Linders et al., 2019).
The pH of undiluted CLSW has been recorded in the range of 3.5-8.2 (Hermansson et al., 2021; Thor et al., 2021), and hence the CLSW used in this study was at the higher end with a pH at 6.9. Similar levels of CO2 induced reductions in pH have caused contrasting effects on mesozooplankton depending on organism type and measured endpoint. Growth and reproduction in Acartia spp. were not affected at pH 7.02-7.4 (Kurihara et al., 2004), whereas hatching success in Calanus finmarchicus exposed to pH 6.9 was severely impaired (Mayor et al., 2007). As for sea urchin larvae, both growth and development have been reduced by pH in the range of CLSW (Kurihara and Shirayama, 2004; Dorey et al., 2013).
While the undiluted CLSW had a pH low enough to potentially cause adverse effects, the diluted CLSW treatments used in this study had pHs that were similar to the control water. The 3% treatment with pH 7.6-7.9 is in fact within the natural range in the Gullmar fjord where we collected the zooplankton used in the experiments (Dorey et al., 2013), and thus we assume that the main driver of effects in this study should be the contaminants rather than acidification.
Copepod reproduction and survival, as well as mesozooplankton predation on ciliates, were affected by the CLSW starting at the lowest tested concentration of 1.5%, all independent of seasonal community structure. Mesozooplankton diversity was affected from 1.5% CLSW, but there was no indirect effect on microzooplankton diversity, which indicates that the mesozooplankton species in the exposed community feed on the same ciliate species as those in the control community, only to a lesser extent.
The community egg production was slightly increased during two out of three seasons in response to 1.5% CLSW exposure, despite the reduced copepod survival, which indicates that copepods experience stress through hormesis (Calabrese, 2008) at this level of exposure. Increased copepod egg production has been observed both at community level, in response to water accommodated fractions of ultra-low sulphur fuel oil (ULSFO) (Jönander and Dahllöf, 2020), and at population level (Acartia bifilosa) in response to a CO2 induced 0.3 reduction in pH (Engström-Öst et al., 2014). When the exposure level increased to 3% CLSW, the remaining surviving copepods could no longer compensate by producing more eggs, and instead the egg production was reduced substantially. Given that both total egg production and hatching rate was impacted at both exposure levels, the effective reproductive output of the community becomes severely reduced. Effects on copepod hatching success from individual PAH exposure has been shown to occur at levels 2-1000 times higher than their respective concentrations in the undiluted CLSW (Bellas and Thor, 2007; Jensen et al., 2008). This suggests a mixture effect of the substances in the CLSW that together causes additive or synergistic toxicity.
Individual exposure to toxicity-driving metals in the CLSW have been shown to cause contrasting toxicities to copepods. Ni reduces hatching success in Acartia pacifica, and nauplii production in Tigriopus japonicus and Apocyclops borneoensis at 10 µg/l, corresponding to 0.3% CLSW (Mohammed et al., 2010). Cu, on the other hand, reduces egg production rate in A. tonsa at 30 µg/l, corresponding to 4% CLSW (Lauer and Bianchini, 2010). Although these studies only cover a fraction of all the substances present in the CLSW, they indicate that metals may have a larger role than PAHs in driving the effects on copepod reproduction.
Copepod survival in the controls relative to start abundances ranged between 55-76%, but part of the mortality can be attributed to the sampling process. Even when sampling with a non-filtering cod end net, there can be 13% mortality due to damage (Elliott and Tang, 2009). Furthermore, field-sampled communities with varying demography means that some individuals will die due to age during the experiments.
The copepod survival was affected starting at 1.5% CLSW across all seasons, similar to what has previously been observed in juvenile C. helgolandicus where mortality rate was increased starting at 0.1% (Thor et al., 2021). Our higher effect concentration could be explained by the fact that we exposed a community of several species with potentially different sensitivities, as well as of different life stages. Furthermore, in both studies the effect was observed at the lowest tested concentration so it cannot be ruled out that survival is affected at even lower concentrations of CLSW.
Mesozooplankton diversity was affected starting at 1.5% CLSW during all three seasons, which indicates that the community always consists of species with varying sensitivity to the CLSW. We also observed that the relative sensitivity of different taxa changed with season, and that those with the most juveniles at the time of each experiment were the most sensitive. Like many other organisms, copepod sensitivity to chemicals is higher among juveniles than adults (Saiz et al., 2009; Heuschele et al., 2022), although the specific mechanisms responsible are unknown for this present study. Copepods molt between each of their 12 life stages, and abnormal molting as well as complete inability to molt has been found in C. helgolandicus copepodites exposed to 0.1% CLSW (Thor et al., 2021), which could play a role in their higher sensitivity.
The reduced survival of the copepods increased the ciliate abundance, which is a clear sign of a reduced predation pressure. Although there were no significant effects on ciliate diversity, Strombidium spp. generally increased more than other taxa, which could indicate a higher growth rate or a better predator avoidance behavior in this group. In accordance with this, Strombiidid ciliates relieved of predation responded quicker than other taxa to increasing food densities in mesocosm studies (Löder et al., 2011). It has been shown that individual mesozooplankton-ciliate interactions is taxa specific (Wiackowski et al., 1994), which confirms that the loss of certain mesozooplankton taxa will likely play an important role in structuring the microzooplankton community. The direct toxicity of CLSW to microzooplankton was not tested in this study, and so it is possible that the interactions between the trophic levels would be different if survival was reduced among the microzooplankton taxa due to direct exposure. Indeed, survival in freshwater ciliates has been reduced at concentrations of Cu and Ni corresponding to 1.5% CLSW (Madoni and Romeo, 2006). Since ciliates are important grazers on small phytoplankton (Löder et al., 2011; Haraguchi et al, 2018), and are known to be sensitive to metals, investigating direct toxicity of CLSW on both copepods and ciliate communities could reveal different indirect effects and interactions between these two trophic levels.
Closed-loop scrubber washwater (CLSW) is toxic to marine mesozooplankton at levels<1.5%, independent of the seasonal species composition. Short-term exposure impairs both copepod reproduction and the ability of the entire mesozooplankton community to predate on microzooplankton and alters community biodiversity. The cumulative toxic unit of 17 for undiluted CLSW, based only on detected substances, suggests that the CLSW can be toxic at levels below what was tested in this study. Given that each single ship equipped with a closed-loop scrubber can discharge 126-150 m3 of CLSW per day (Hermansson et al., 2021), it is likely that substantial adverse effects on the zooplankton will occur near such discharges. Some of the substances in the CLSW are listed as priority substances in the EU Water Framework Directive (Directive 2013/39/EU) and therefore have the potential to cause harm to aquatic systems, as well as reduce the ability to achieve good chemical status in coastal areas. Furthermore, some have half-lives of 100-1000 days in shallow water, and even up to several 1000 days once they reach the sediment (Tansel et al., 2011). This means that the continued use of closed-loop scrubbers without appropriate regulation of discharge limits for more compound groups, will contribute both to adverse effects, and an accumulation of toxic contaminants in areas near shipping routes and ports.
The datasets presented in this study can be found in online repositories. The names of the repository/repositories and accession number(s) can be found below: https://zenodo.org/record/8058781.
The manuscript presents research on animals that do not require ethical approval for their study.
CJ, JE, I-MH, PT, and ID conceptualized and designed the study. CJ organized and managed the project. CJ, JE, and MR performed the experiments and collected the data. CJ and MR identified the organisms in the samples. CJ analyzed the data, performed the statistical analyses, and visualized the results. ID, I-MH, and CJ funded the project. I-MH, PT, and ID supervised the project. CJ prepared the first draft of the manuscript, but all authors helped review and edit the manuscript and approved the final submitted version.
The author(s) declare financial support was received for the research, authorship, and/or publication of this article. Funding was provided by the Swedish research council Formas, contract no. 2017-00432 Copemix, and the University of Gothenburg strategic research initiative FRAM -Centre for Future Chemical Risk Assessment and Management Strategies.
We thank S. Dupont for lending equipment and reagents for alkalinity and pH measurements, and Gothenburg University Marine Algal Culture Collection (GUMACC) for providing algae cultures.
The authors declare that the research was conducted in the absence of any commercial or financial relationships that could be construed as a potential conflict of interest.
All claims expressed in this article are solely those of the authors and do not necessarily represent those of their affiliated organizations, or those of the publisher, the editors and the reviewers. Any product that may be evaluated in this article, or claim that may be made by its manufacturer, is not guaranteed or endorsed by the publisher.
The Supplementary Material for this article can be found online at: https://www.frontiersin.org/articles/10.3389/fmars.2023.1249964/full#supplementary-material
Barata C., Calbet A., Saiz E., Ortiz L., Bayona J. M. (2005). Predicting single and mixture toxicity of petrogenic polycyclic aromatic hydrocarbons to the copepod Oithona davisae. Environ. Toxicol. Chem. 24, 2992–2999. doi: 10.1897/05-189R.1
Bellas J., Thor P. (2007). Effects of selected PAHs on reproduction and survival of the calanoid copepod Acartia tonsa. Ecotoxicology 16, 465–474. doi: 10.1007/s10646-007-0152-2
Calabrese E. J. (2008). Hormesis: why it is important to toxicology and toxicologists. Environ. Toxicol. Chem. 27, 1451–1474. doi: 10.1897/07-541.1
Calbet A., Saiz E. (2005). The ciliate-copepod link in marine ecosystems. Aquat. Microbial Ecology; 38, 157–167. doi: 10.3354/ame038157
Cedergreen N. (2014). Quantifying synergy: a systematic review of mixture toxicity studies within environmental toxicology. PloS One 9, e96580. doi: 10.1371/journal.pone.0096580
Celo V., Dabek-Zlotorzynska E., McCurdy M. (2015). Chemical characterization of exhaust emissions from selected Canadian marine vessels: the case of trace metals and lanthanoids. Environ. Sci. Technol. 49, 5220–5226. doi: 10.1021/acs.est.5b00127
Chiba S., Tadokoro K., Sugisaki H., Saino T. (2006). Effects of decadal climate change on zooplankton over the last 50 years in the western subarctic North Pacific. Global Change Biol. 12, 907–920. doi: 10.1111/j.1365-2486.2006.01136.x
Clarke K., Gorley R. (2015). PRIMER v7: user manual/tutorial (Plymouth, United Kingdom: Primer-E Ltd).
Corbin J. C., Mensah A. A., Pieber S. M., Orasche J., Michalke B., Zanatta M., et al. (2018). Trace metals in soot and PM2. 5 from heavy-fuel-oil combustion in a marine engine. Environ. Sci. Technol. 52, 6714–6722. doi: 10.1021/acs.est.8b01764
Deneer J. W. (2000). Toxicity of mixtures of pesticides in aquatic systems. Pest Manage. Science: formerly Pesticide Science; 56, 516–520. doi: 10.1002/(SICI)1526-4998(200006)56:6<516::AID-PS163>3.0.CO;2-0
Directive 2013/39/EU of the European Parliament and of the Council of 12 August 2013 amending Directives 2000/60/EC and 2008/105/EC as regards priority substances in the field of water policy, Official Journal of the European Union L 226, 1–17.
DNV-GL (2023) Alternative fuels insight: scrubber statistics. Available at: https://afi.dnv.com/Statistics?repId=2s (Accessed 2023-03-23).
Dorey N., Lançon P., Thorndyke M., Dupont S. (2013). Assessing physiological tipping point of sea urchin larvae exposed to a broad range of pH. Global Change Biol. 19, 3355–3367. doi: 10.1111/gcb.12276
Elliott D. T., Tang K. W. (2009). Simple staining method for differentiating live and dead marine zooplankton in field samples. Limnology Oceanography-Methods 7, 585–594. doi: 10.4319/lom.2009.7.585
Endres S., Maes F., Hopkins F., Houghton K., Mårtensson E. M., Oeffner J., et al. (2018). A new perspective at the ship-air-sea-interface: The environmental impacts of exhaust gas scrubber discharge. Front. Mar. Sci. 5. doi: 10.3389/fmars.2018.00139
Engström-Öst J., Holmborn T., Brutemark A., Hogfors H., Vehmaa A., Gorokhova E. (2014). The effects of short-term pH decrease on the reproductive output of the copepod Acartia bifilosa–a laboratory study. Mar. Freshw. Behav. Physiol. 47, 173–183. doi: 10.1080/10236244.2014.919096
Gismervik I. (2006). Top-down impact by copepods on ciliate numbers and persistence depends on copepod and ciliate species composition. J. Plankton Res. 28 (5), 499–507. doi: 10.1093/plankt/fbi135
Gissel Nielsen T., Hansen P. J. (1999). Miljø- og Energiministeriet Danmarks Miljøundersøgelser, (1999) Dyreplankton i danske farvande (Århus: Scanprint as).
Hallanger I. G., Ruus A., Herzke D., Warner N. A., Evenset A., Heimstad E. S., et al. (2011). Influence of season, location, and feeding strategy on bioaccumulation of halogenated organic contaminants in Arctic marine zooplankton. Environ. Toxicol. Chem. 30, 77–87. doi: 10.1002/etc.362
Haraguchi L., Jakobsen H. H., Lundholm N., Carstensen J. (2018). Phytoplankton community dynamic: a driver for ciliate trophic strategies. Front. Mar. Sci. 5. doi: 10.3389/fmars.2018.00272
Hassellöv I.-M., Koski M., Broeg K., Marin-Enriquez O., Tronczynski J., Dulière V., et al. (2020). ICES Viewpoint background document: impact from exhaust gas cleaning systems (scrubbers) on the marine environment (Ad hoc) (Copenhagen, Denmark: ICES Scientific Reports). doi: 10.17895/ices.pub.7487
Hassellöv I.-M., Turner D. R., Lauer A., Corbett J. J. (2013). Shipping contributes to ocean acidification. Geophysical Res. Lett. 40, 2731–2736. doi: 10.1002/grl.50521
Hermansson A. L., Hassellöv I.-M., Moldanová J., Ytreberg E. (2021). Comparing emissions of polyaromatic hydrocarbons and metals from marine fuels and scrubbers. Transportation Res. Part D: Transport Environ. 97, 102912. doi: 10.1016/j.trd.2021.102912
Heuschele J., Lode T., Konestabo H. S., Titelman J., Andersen T., Borgå K. (2022). Drivers of copper sensitivity in copepods: A meta-analysis of LC50s. Ecotoxicology Environ. Saf. 242, 113907. doi: 10.1016/j.ecoenv.2022.113907
IMO (2008). Amendments to the Annex of Protocol of 1997 to amend the International Convention for the Prevention of Pollution from Ships 1973, as modified by the protocol of 1978. (Revised MARPOL Annex VI) (Resolution MEPC.176(58)) 2008), 1–46.
Jensen M. H., Nielsen T. G., Dahllöf I. (2008). Effects of pyrene on grazing and reproduction of Calanus finmarchicus and Calanus glacialis from Disko Bay, West Greenland. Aquat. Toxicol. 87, 99–107. doi: 10.1016/j.aquatox.2008.01.005
Jönander C., Dahllöf I. (2020). Short and long-term effects of low-sulphur fuels on marine zooplankton communities. Aquat. Toxicol. 227, 105592. doi: 10.1016/j.aquatox.2020.105592
Jonson J. E., Jalkanen J.-P., Johansson L., Gauss M., Denier van der Gon H. A. C. (2015). Model calculations of the effects of present and future emissions of air pollutants from shipping in the Baltic Sea and the North Sea. Atmospheric Chem. Phys. 15, 783–798. doi: 10.5194/acp-15-783-2015
Kienzler A., Bopp S. K., van der Linden S., Berggren E., Worth A. (2016). Regulatory assessment of chemical mixtures: requirements, current approaches and future perspectives. Regul. Toxicol. Pharmacol. 80, 321–334. doi: 10.1016/j.yrtph.2016.05.020
Kim M. S., Jeong J.-A. (2019). Corrosion behavior of carbon steel in diluted sulfuric acid based on seawater. Corrosion Sci. Technol. 18, 78–85. doi: 10.14773/cst.2019.18.3.78
Koski M., Stedmon C., Trapp S. (2017). Ecological effects of scrubber water discharge on coastal plankton: potential synergistic effects of contaminants reduce survival and feeding of the copepod Acartia tonsa. Mar. Environ. Res. 129, 374–385. doi: 10.1016/j.marenvres.2017.06.006
Kurihara H., Shimode S., Shirayama Y. (2004). Effects of raised CO2 concentration on the egg production rate and early development of two marine copepods (Acartia steueri and Acartia erythraea). Mar. pollut. Bull. 49, 721–727. doi: 10.1016/j.marpolbul.2004.05.005
Kurihara H., Shirayama Y. (2004). Effects of increased atmospheric CO2 on sea urchin early development. Mar. Ecol. Prog. Ser. 274, 161–169. doi: 10.3354/meps274161
Lauer M. M., Bianchini A. (2010). Chronic copper toxicity in the estuarine copepod Acartia tonsa at different salinities. Environ. Toxicol. Chem. 29, 2297–2303. doi: 10.1002/etc.285
Linders J., Adams E., Behrends B., Dock A., Hanayama S., Luit R., et al. (2019). Exhaust Gas Cleaning Systems - A Roadmap to Risk Assessment. Report of the GESAMP Task Team on exhaust gas cleaning systems. Submitted to PPR 7 as document PPR 7/INF.23. London. IMO., 1–121.
Löder M. G., Meunier C., Wiltshire K. H., Boersma M., Aberle N. (2011). The role of ciliates, heterotrophic dinoflagellates and copepods in structuring spring plankton communities at Helgoland Roads, North Sea. Mar. Biol. 158, 1551–1580. doi: 10.1007/s00227-011-1670-2
Madoni P., Romeo M. G. (2006). Acute toxicity of heavy metals towards freshwater ciliated protists. Environ. pollut. 141, 1–7. doi: 10.1016/j.envpol.2005.08.025
Mayor D. J., Matthews C., Cook K., Zuur A. F., Hay S. (2007). CO2-induced acidification affects hatching success in Calanus finmarchicus. Mar. Ecol. Prog. Ser. 350, 91–97. doi: 10.3354/meps07142
MEPC (2015). RESOLUTION MEPC.259(68) (adopted on 15 may 2015) 2015 guidelines for exhaust gas cleaning systems. International Martime Organisation, IMO, London, UK. 1–25.
Mohammed E. H., Wang G., Jiang J. (2010). The effects of nickel on the reproductive ability of three different marine copepods. Ecotoxicology 19, 911–916. doi: 10.1007/s10646-010-0471-6
Nørgaard K. B., Cedergreen N. (2010). Pesticide cocktails can interact synergistically on aquatic crustaceans. Environ. Sci. pollut. Res. 17, 957–967. doi: 10.1007/s11356-009-0284-4
Olker J. H., Elonen C. M., Pilli A., Anderson A., Kinziger B., Erickson S., et al. (2022). The ECOTOXicology knowledgebase: A curated database of ecologically relevant toxicity tests to support environmental research and risk assessment. Environ. Toxicol. Chem. 41, 1520–1539. doi: 10.1002/etc.5324
Payne S. J., King C. K., Zamora L. M., Virtue P. (2014). Temporal changes in the sensitivity of coastal Antarctic zooplankton communities to diesel fuel: A comparison between single-and multi-species toxicity tests. Environ. Toxicol. Chem. 33, 882–890. doi: 10.1002/etc.2522
Saiz E., Movilla J., Yebra L., Barata C., Calbet A. (2009). Lethal and sublethal effects of naphthalene and 1,2-dimethylnaphthalene on naupliar and adult stages of the marine cyclopoid copepod Oithona davisae. Environ. pollut. 157, 1219–1226. doi: 10.1016/j.envpol.2008.12.011
Sippula O., Stengel B., Sklorz M., Streibel T., Rabe R., Orasche J., et al. (2014). Particle emissions from a marine engine: chemical composition and aromatic emission profiles under various operating conditions. Environ. Sci. Technol. 48, 11721–11729. doi: 10.1021/es502484z
Spilsbury F. D., Warne M. S. J., Backhaus T. (2020). Risk assessment of pesticide mixtures in Australian rivers discharging to the Great Barrier Reef. Environ. Sci. Technology; 54, 14361–14371. doi: 10.1021/acs.est.0c04066
Tansel B., Fuentes C., Sanchez M., Predoi K., Acevedo M. (2011). Persistence profile of polyaromatic hydrocarbons in shallow and deep Gulf waters and sediments: effect of water temperature and sediment–water partitioning characteristics. Mar. pollut. Bull. 62, 2659–2665. doi: 10.1016/j.marpolbul.2011.09.026
Teuchies J., Cox T. J., Van Itterbeeck K., Meysman F. J., Blust R. (2020). The impact of scrubber discharge on the water quality in estuaries and ports. Environ. Sci. Europe 32, 1–11. doi: 10.1186/s12302-020-00380-z
Thor P., Granberg M. E., Winnes H., Magnusson K. (2021). Severe toxic effects on pelagic copepods from maritime exhaust gas scrubber effluents. Environ. Sci. Technol. 55, 5826–5835. doi: 10.1021/acs.est.0c07805
Turner D. R., Hassellöv I.-M., Ytreberg E., Rutgersson A., Miller L. A. (2017). Shipping and the environment: Smokestack emissions, scrubbers and unregulated oceanic consequences Vol. 5 (Elementa: Science of the Anthropocene). doi: 10.1525/elementa.167
Ushakov S., Stenersen D., Einang P. M., Ask T.Ø. (2020). Meeting future emission regulation at sea by combining low-pressure EGR and seawater scrubbing. J. Mar. Sci. Technol. 25, 482–497. doi: 10.1007/s00773-019-00655-y
Vargas C. A., González H.E. (2004). Plankton community structure and carbon cycling in a coastal upwelling system. I. Bacteria, microprotozoans and phytoplankton in the diet of copepods and appendicularians. Aquat. Microb. Ecol 34(2), 151–164. doi: 10.3354/ame034151
Walter H., Consolaro F., Gramatica P., Scholze M., Altenburger R. (2002). Mixture toxicity of priority pollutants at no observed effect concentrations (NOECs). Ecotoxicology 11, 299–310. doi: 10.1023/A:1020592802989
Wiackowski K., Brett M. T., Goldman C. R. (1994). Differential effects of zooplankton species on ciliate community structure. Limnology Oceanography 39, 486–492. doi: 10.4319/lo.1994.39.3.0486
Wiadnyana N. N., Rassoulzadegan F. (1989). Selective feeding of Acartia clausi and Centropages typicus on microzooplankton. Mar. Ecol. Prog. Ser. 53, 37–45. doi: 10.3354/meps053037
Ytreberg E., Hansson K., Hermansson A. L., Parsmo R., Lagerström M., Jalkanen J.-P., et al. (2022). Metal and PAH loads from ships and boats, relative other sources, in the Baltic Sea. Mar. pollut. Bull. 182, 113904. doi: 10.1016/j.marpolbul.2022.113904
Keywords: exhaust gas cleaning system (EGCS), mixture toxicity, shipping, closed-loop, trophic interaction, copepods, ciliates, zooplankton
Citation: Jönander C, Egardt J, Hassellöv I-M, Tiselius P, Rasmussen M and Dahllöf I (2023) Exposure to closed-loop scrubber washwater alters biodiversity, reproduction, and grazing of marine zooplankton. Front. Mar. Sci. 10:1249964. doi: 10.3389/fmars.2023.1249964
Received: 29 June 2023; Accepted: 30 October 2023;
Published: 20 November 2023.
Edited by:
Hernando Bacosa, Iligan Institute of Technology, PhilippinesReviewed by:
Eva Garcia-Vazquez, Universidad de Oviedo Mieres, SpainCopyright © 2023 Jönander, Egardt, Hassellöv, Tiselius, Rasmussen and Dahllöf. This is an open-access article distributed under the terms of the Creative Commons Attribution License (CC BY). The use, distribution or reproduction in other forums is permitted, provided the original author(s) and the copyright owner(s) are credited and that the original publication in this journal is cited, in accordance with accepted academic practice. No use, distribution or reproduction is permitted which does not comply with these terms.
*Correspondence: Ingela Dahllöf, aW5nZWxhLmRhaGxsb2ZAYmlvZW52Lmd1LnNl
Disclaimer: All claims expressed in this article are solely those of the authors and do not necessarily represent those of their affiliated organizations, or those of the publisher, the editors and the reviewers. Any product that may be evaluated in this article or claim that may be made by its manufacturer is not guaranteed or endorsed by the publisher.
Research integrity at Frontiers
Learn more about the work of our research integrity team to safeguard the quality of each article we publish.