- 1Earth, Ocean, and Atmospheric Science Department, Florida State University, Tallahassee, FL, United States
- 2Aix Marseille University, Université de Toulon, CNRS, IRD, MIO Marseille, France
- 3Laboratoire d’Oceanographie de Villefranche, CNRS, Sorbonne University, Villefranche-sur-mer, France
The spatial distribution of marine di-nitrogen (N2) fixation informs our understanding of the sensitivities of this process as well as the potential for this new nitrogen (N) source to drive export production, influencing the global carbon (C) cycle and climate. Using geochemically-derived δ15N budgets, we quantified rates of N2 fixation and its importance for supporting export production at stations sampled near the southwest Pacific Tonga-Kermadec Arc. Recent observations indicate that shallow (<300 m) hydrothermal vents located along the arc provide significant dissolved iron to the euphotic zone, stimulating N2 fixation. Here we compare measurements of water column δ15NNO3+NO2 with sinking particulate δ15N collected by short-term sediment traps deployed at 170 m and 270 m at stations in close proximity to subsurface hydrothermal activity, and the δ15N of N2 fixation. Results from the δ15N budgets yield high geochemically-based N2 fixation rates (282 to 638 µmol N m-2 d-1) at stations impacted by hydrothermal activity, supporting 64 to 92% of export production in late spring. These results are consistent with contemporaneous 15N2 uptake rate estimates and molecular work describing high Trichodesmium spp. and other diazotroph abundances associated with elevated N2 fixation rates. Further, the δ15N of sinking particulate N collected at 1000 m over an annual cycle revealed sinking fluxes peaked in the summer and coincided with the lowest δ15N, while lower winter sinking fluxes had the highest δ15N, indicating isotopically distinct N sources supporting export seasonally, and aligning with observations from most other δ15N budgets in oligotrophic regions. Consequently, the significant regional N2 fixation input to the late spring/summer Western Tropical South Pacific results in the accumulation of low-δ15NNO3+NO2 in the upper thermocline that works to lower the elevated δ15NNO3+NO2 generated in the oxygen deficient zones in the Eastern Tropical South Pacific.
1 Introduction
The biological fixation of dinitrogen (N2) gas, mediated primarily by marine prokaryotes (“diazotrophs”), is the largest source of newly fixed nitrogen (N) to the global ocean (Gruber, 2004; Landolfi et al., 2018), fertilizing primary productivity and supporting carbon (C) export (Dugdale and Goering, 1967; Karl et al., 1997; Capone, 2001; Capone et al., 2005). The global rate and distribution of marine N2 fixation remains uncertain, although geochemical and biological observations indicate significant N2 fixation rates occur in both the Tropical Atlantic (Gruber, 2004; Capone et al., 2005; Mahaffey, 2005; Marconi et al., 2017) as well as the Western Tropical South Pacific (WTSP) (Berthelot et al., 2017; Bonnet et al., 2017; Caffin et al., 2018; Knapp et al., 2018). These high rates of N2 fixation in the Tropical Atlantic are consistent with locations of elevated rates of atmospheric dust deposition (Jickells et al., 2005; Mahowald et al., 2005; Conway and John, 2014; Xu and Weber, 2021), while emerging evidence in the WTSP describes the significance of hydrothermally sourced iron (Fe) (Guieu et al., 2018; Bonnet et al., 2023b) meet the high Fe requirements of diazotrophs (Berman-Frank et al., 2001; Kustka et al., 2003) in the region. Indeed, Fe and phosphorus availability are thought to primarily influence the spatial distribution of marine N2 fixation (Moore et al., 2009; Monteiro et al., 2011; Dutkiewicz et al., 2012; Weber and Deutsch, 2014).
A long-standing goal of marine N2 fixation research is to better characterize the marine diazotroph community and their sensitivity to environmental fluctuations, while relating these to their regional distributions and consequential N2 fixation fluxes (Mahaffey, 2005; Moisander et al., 2010; Sohm et al., 2011). Diazotrophs are identified by the nitrogenase (nifH) gene that encodes for the Fe binding protein of the nifH operon (Zehr et al., 1998; Zehr and Turner, 2001; Turk-Kubo et al., 2012). Common marine proteobacterial (e.g., alpha-, beta- gamma-, delta-) and cyanobacterial diazotroph types include: 1) non-heterocystous filamentous (e.g., Trichodesmium spp.), 2) heterocystous filamentous (e.g., Richelia), and 3) unicellular (e.g., Crocosphaera spp.) (Capone et al., 1997; Luo et al., 2012; Moisander et al., 2014). Historically, high rates of N2 fixation by these and other diazotrophs have been associated with warm (>25 °C), nitrate (NO3-)- and ammonium (NH4+)-depleted, Fe-rich surface waters (Kustka et al., 2003; Staal et al., 2003; Bonnet et al., 2009). The impact Fe has on the intra-basin distribution of N2 fixation rates is particularly evident in the South Pacific, with low Fe supply associated with low rates of N2 fixation in the Eastern Tropical South Pacific (ETSP) (Dekaezemacker et al., 2013; Knapp et al., 2016a). While historically atmospheric deposition has been considered the primary source of Fe fueling marine diazotrophy, the WTSP surface waters appear relatively unique, with the primary Fe supply thought to originate from shallow (≤300 m) hydrothermal vents, particularly in the Lau Basin (Guieu et al., 2018; Tilliette et al., 2022; Bonnet et al., 2023b).
Biological tools have been used to calculate short-term N2 fixation rates (e.g., Montoya et al., 2004; Capone et al., 2005; Mulholland et al., 2019) and identify marine diazotrophic potential (Turk-Kubo et al., 2012; Stenegren et al., 2018; Meiler et al., 2022). However, the contribution of N2 fixation to export production is primarily estimated using a geochemically-derived “δ15N” budget over short timescales (Casciotti et al., 2008; Bourbonnais et al., 2009; White et al., 2013) as well as annual cycles (Böttjer et al., 2017). The “δ15N” budget uses a two end-member mixing model to compare the isotopic composition (δ15N) of exported particulate organic matter captured in a sediment trap (PNsink) to the δ15N of N2 fixation inputs (-1‰) (Hoering and Ford, 1960; Minagawa and Wada, 1986; Carpenter et al., 1997) and subsurface NO3- (measured at each location, where δ15N (‰ vs. air) = [((15N/14N)sample/(15N/14N)AIR)-1]x1000) (Altabet, 1988; Karl et al., 1997; Dore et al., 2002; Casciotti et al., 2008; Bourbonnais et al., 2009; White et al., 2013). Most prior δ15N budgets indicate that N2 fixation supports <20% of export production in oligotrophic regions. In the North Pacific and ETSP, ≤25% of export production is estimated to be supported by N2 fixation (Casciotti et al., 2008; Knapp et al., 2016a; Böttjer et al., 2017), although that 25% is likely not equally distributed over an annual cycle. Specifically, summertime stratification is believed to promote N2 fixation-supported export, while the deepening of the wintertime mixed layer promotes NO3–supported export production (Casciotti et al., 2008; Böttjer et al., 2017). In contrast to other δ15N budgets from oligotrophic regions, a recent study from the WTSP describes particularly large contributions of N2 fixation to export production (>50%) during the late summer and early autumn (Knapp et al., 2018). However, it remains unclear whether N2 fixation supports a meaningful fraction of N export in the WTSP annually. Here we apply a δ15N budget to samples collected in shallow (170 m and 270 m), short-term drifting sediment traps to evaluate the importance of N2 fixation-supported export production during the late spring. Additionally, we use sinking material collected over the course of a year in a deep (1000 m), moored sediment trap to evaluate seasonal trends in the δ15N of exported particulate organic matter relative to the δ15N for sources of new N to surface waters. We compare the geochemically-derived N2 fixation rates from the short-term δ15N budgets with 15N2 incubation-based rates and estimates of diazotroph abundance, and evaluate these results in the context of previous regional and global N2 fixation rate estimates, as well as seasonal trends extracted from the deep, moored trap.
2 Methods
2.1 Sample collection
Sample collection was conducted as part of the GEOTRACES TONGA (shallow hydroThermal sOurces of trace elemeNts: potential impacts on biological productivity and the bioloGicAl carbon pump) research cruises (doi.org/10.17600/18000884) aboard the R/V L’Atalante in November 2019 and R/V Alis in October 2020, with both cruises leaving from and returning to Nouméa, New Caledonia. The 2019 primary cruise collected samples at 13 stations along a roughly zonal transect at ~20° S, sampling Melanesian waters (MW), the Lau Basin (LB), and crossing the Tonga-Kermadec Arc into the deeper South Pacific Gyre (SPG). In 2020, samples were collected at four stations in MW and the LB (Figure 1). On both cruises, water column samples (n=200) for nitrate + nitrite ([NO3-+NO2-]) concentration and δ15N analysis were collected from Niskin or GoFlo bottles deployed on conductivity, temperature, and depth (CTD), TOW (small, classical CTD with 12 Niskin bottles), or trace metal clean (TMC) rosettes equipped with sensors. At discrete depths from each of the casts, 60 mL of 0.2 μm filtered seawater were collected in duplicate and stored in acid and deionized water-washed, sample-rinsed (three times) high-density polyethylene bottles. These samples were then immediately frozen at -20° C and subsequently sent to Florida State University for post cruise analysis.
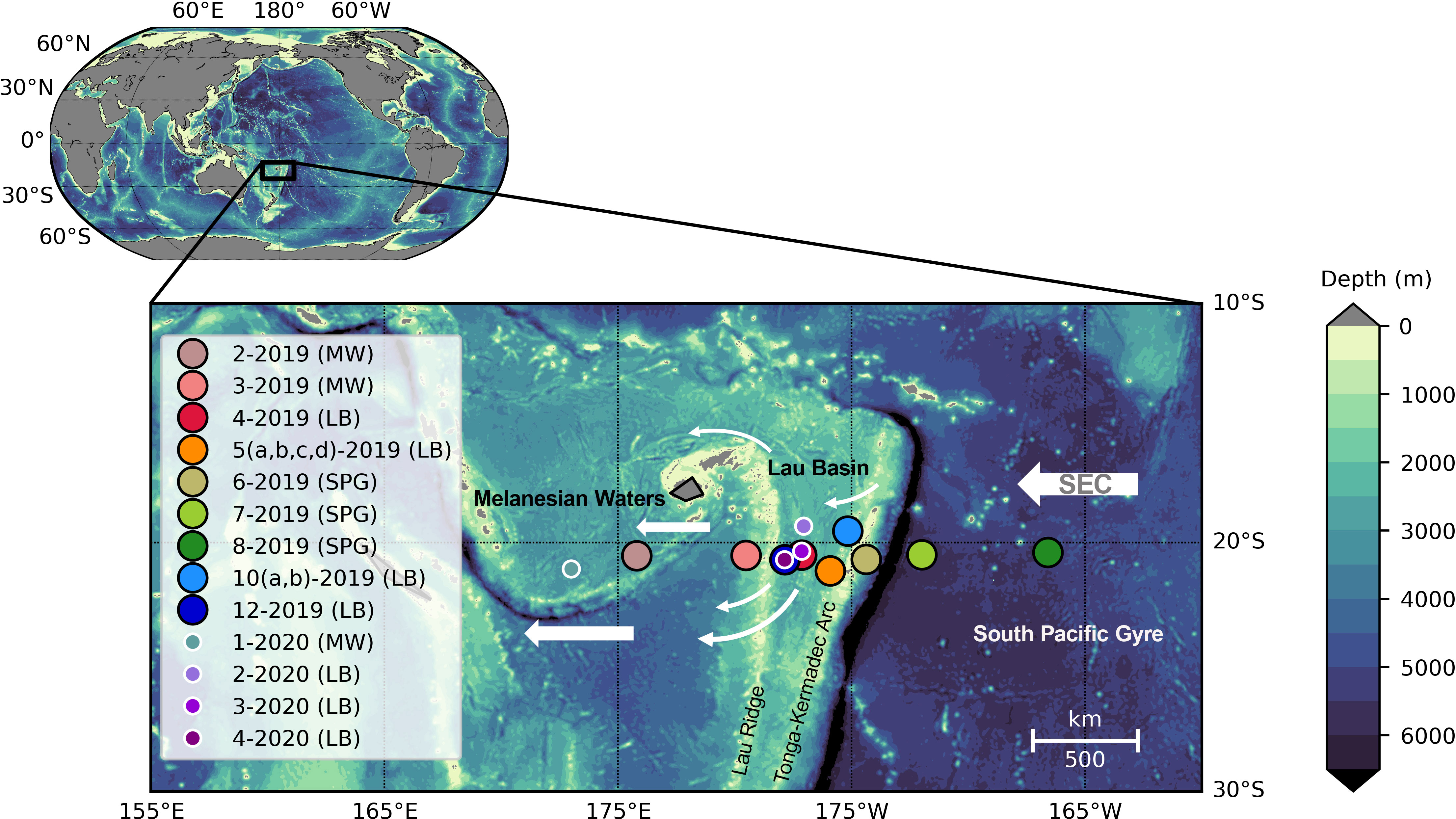
Figure 1 Bathymetry of the southwest Pacific region with stations from the TONGA research cruises shown in larger filled circles with black outlines (2019), and smaller filled circles with white outlines (2020); gray represents areas above sea-level. Stations 2-2019, 3-2019 and 1-2020 were sampled in Melanesian waters (MW), stations 4-2019, 5-2019, 10-2019, 12-2019, 2-2020, 3-2020, and 4-2020 were sampled in the Lau Basin (LB), and stations 6-2019, 7-2019, and 8-2019 were sampled in the South Pacific Gyre (SPG). Stations 5 (a, b, c, d)-2019 and 10 (a, b)-2019 were proximal to the shallow hydrothermal vents ‘Panamax’ and ‘Simone’, respectively. The South Equatorial Current (SEC) and branches thereof are indicated by the white arrows.
Short-term Particle Interceptor Traps [PIT, collecting area of 0.0085 m2, aspect ratio of 6.7, and filled with 0.2 μm filtered seawater with added formaldehyde brine (5% formaldehyde, final concentration) buffered with sodium tetraborate (pH 8)] were deployed on a drifting mooring in close proximity to the hydrothermal vents at 170 m and 270 m at station 5a-2019 for five days and at 270 m at station 10a-2019 for four days during the 2019 cruise, collecting sinking particulate N (“PNsink”). Additionally, a long-term Technicap PPS5 trap (1 m2 collecting area, aspect ratio of 5.3) was deployed (containing the same buffered formaldehyde brine solution described above) at station 12-2019/4-2020 at 1000 m, collecting samples every 14 days (bimonthly) for 12 months from November 2019 to October 2020. Although sediment traps are a standard tool used to capture sinking particles, uncertainties remain in their collection efficiency within the water column and between trap designs (Buesseler et al., 2007; Baker et al., 2020; Tilliette et al., 2023).
2.2 NO3-+NO2- concentration and δ15N analysis
For the 2019 samples, [NO3-+NO2-] was determined using colorimetric analysis (Aminot and Kerouel, 2007) with a detection limit of 0.05 µM and a standard deviation (S.D.) of ± 0.1 μM. Furthermore, the [NO3-+NO2-] for the 2020 samples was measured by chemiluminescence (Braman and Hendrix, 1989) using a Thermo 42i NOx analyzer at Florida State University. Briefly, samples were injected into a heated, acidic vanadium (III) solution that reduces NO3-+NO2- quantitatively to nitric oxide gas (NO(g)). The NO(g) then reacts with ozone inside the analyzer to produce light, the intensity of which is quantitatively related to the amount of NO(g) in the sample and thus the original [NO3-+NO2-]. The range of detection of the instrument was adjusted according to the concentrations of the samples. Sample [NO3-+NO2-] was calibrated using a standard curve that bracketed the range of samples with a lower reporting limit of 0.1 µM and an average S.D. of ± 0.1 μM.
The nitrogen (N) isotopic composition of NO3-+NO2- (δ15NNO3+NO2) was determined using the “denitrifier” method (Sigman et al., 2001; Casciotti et al., 2002; McIlvin and Casciotti, 2011; Weigand et al., 2016). This analysis was performed when sample [NO3-+NO2-] ≥ 0.3 μM. The δ15NNO3+NO2 values were reported when the standard deviation of replicate analyses was <0.5‰. Samples were calibrated with IAEA N3 and USGS 34 as described in McIlvin and Casciotti (2011).
2.3 Sinking particulate N flux and δ15N measurements
The bulk PNsink mass flux, and its associated isotopic composition, “δ15NPNsink”, collected by the sediment traps, was measured using an Elementar Analyser - Isotope Ratio Mass Spectrometer (EA-IRMS) at the Mediterranean Institute of Oceanography (SERCON INTEGRA 2). The Quantification Limit was 7 µg N and the precision was between ± 0.3‰ for highest masses and ± 3.5‰ for masses close to Quantification Limit (k = 2).
2.4 δ15N budget calculations
A two end-member mixing model was used to evaluate the δ15N budgets. This model assumes two quantitatively important “new” N sources to surface waters, subsurface NO3-+NO2- and biological N2 fixation, as well as one loss term, PNsink. The isotopic composition of N2 fixation inputs, “δ15NN2 fix” was assumed to be -1‰ (Hoering and Ford, 1960; Minagawa and Wada, 1986; Carpenter et al., 1997), while the δ15N of subsurface NO3-+NO2- and PNsink were measured. The relative contribution of N2 fixation to export production, “fnfix”, is calculated by the following (Knapp et al., 2018):
which can be rearranged to solve for fnfix:
The depth from which subsurface NO3-+NO2- is sourced likely varies and is difficult to constrain; therefore, the short-term δ15N budgets are evaluated over a range of subsurface δ15NNO3+NO2 source values. These values include the shallowest δ15NNO3+NO2 minima and the δ15NNO3+NO2 of the sample collected immediately below the minima (Knapp et al., 2021). The long-term δ15N budgets are evaluated using the shallowest average δ15NNO3+NO2 minima at station 12-2019/station 4-2020 as well as the average δ15NNO3+NO2 of South Pacific Sub-tropical Under Water (SPSTUW, 150m – 250 m depth) at station 4-2020. Note, sampling resolution at station 12-2019 did not include SPSTUW. These δ15NNO3+NO2 end-members for the moored trap δ15N bugdet were chosen to encompass the range of NO3-+NO2- likely entrained to surface waters over an annual cycle (Moutin et al., 2018). This seasonality is also observed at the station ALOHA, Hawaii (Casciotti et al., 2008; Böttjer et al., 2017). Once the fraction of export supported by N2 fixation is calculated, an N2 fixation rate, “Rnfix” can be calculated by multiplying fnfix by the PNsink mass flux, yielding a geochemically-derived N2 fixation rate.
2.5 Bathymetric data
Bathymetric data from the sampling region were considered to better understand the bathymetric-induced current steering as well as the proximity of the stations to the shallow hydrothermal vents. These bathymetric data were downloaded from NOAA’s National Centers for Environmental Information page (https://www.ngdc.noaa.gov/mgg/global/). The ETOPO1 Global Relief Model was used with the grid version ETOPO1 Bedrock.
3 Hydrography
Water masses along the TONGA transect were identified using temperature, salinity, and potential density (σθ) (Figure 2) and align with those reported in Tilliette et al. (2022). Plots of the water column profiles in temperature-salinity space indicate that all stations were largely influenced by the same water masses (Figure 2), reflecting the dominance of the westward flowing SEC, which impacts waters from 400 to 1000 m across this region (Figure 1) (Talley et al., 2011; Guieu et al., 2018; Tilliette et al., 2022). The SEC divides into branches, particularly in the LB, due to the bathymetric blocking by islands and deep-sea ridges (Webb, 2000; Tilliette et al., 2022). Many of these branches in the LB are observed to have an overall southwestern trajectory before returning to a western trajectory over the center of the LB and maintaining this westward trajectory in MW (Figure 1) (Tilliette et al., 2022). Additionally, the Tonga-Kermadec Arc acts as a physical barrier to deep water masses entering the LB from the SPG, influencing circulation downstream (Tilliette et al., 2022). Surface waters across this transect were turbulent down to ~150 m with a σθ of 23.7 ± 0.2, temperature of 24.4 ± 0.6°C, and salinity of 35.4 ± 0.5, aligning with previous studies (Table 1) (Tilliette et al., 2022). Below that, in the thermocline and extending to ~700 m, two major water masses were present: SPSTUW and Western South Pacific Central Water (WSPCW) (Talley et al., 2011; Lehmann et al., 2018; Tilliette et al., 2022) (Figure 2) (Table 1). Across the transect, SPSTUW had an average (± 1 S.D.) σθ of 25.0 ± 0.2, temperature of 22.5 ± 0.8°C and was further recognized by its characteristic salinity maximum of 35.7 ± 0.1 (Talley et al., 2011; Lehmann et al., 2018; Tilliette et al., 2022) between 150 and 250 m (Table 1). Between 250 and 500 m, WSPCW was identified by a linear temperature-salinity relationship, with an average (± 1 S.D.) σθ of 26.4 ± 0.2, temperature of 12.6 ± 0.9°C and salinity of 34.9 ± 0.2, comparable to values reported by Lehmann et al. (2018) and Tilliette et al. (2022). Notably, between 380 and 400 m South Pacific Subtropical Mode Water (SPSTMW), a subsidiary of WSPCW, was identified by its characteristic σθ of 26.0 (Talley et al., 2011), with an average (± 1 S.D.) temperature of 14.9 ± 0.5°C and salinity of 35.2 ± 0.1 (Table 1). Finally, deep water masses were observed in the SPG east of the Tonga-Kermadec Arc. In particular, Antarctic Intermediate Water (AAIW) was observed at station 8-2019 and station 2-2020 between 630 and 700 m where a σθ of 26.9 ± 0.1 temperature of 6.5 ± 0.1°C and salinity of 34.4 ± 0.0, aligning with previous studies (Table 1) (Talley et al., 2011; Tilliette et al., 2022).
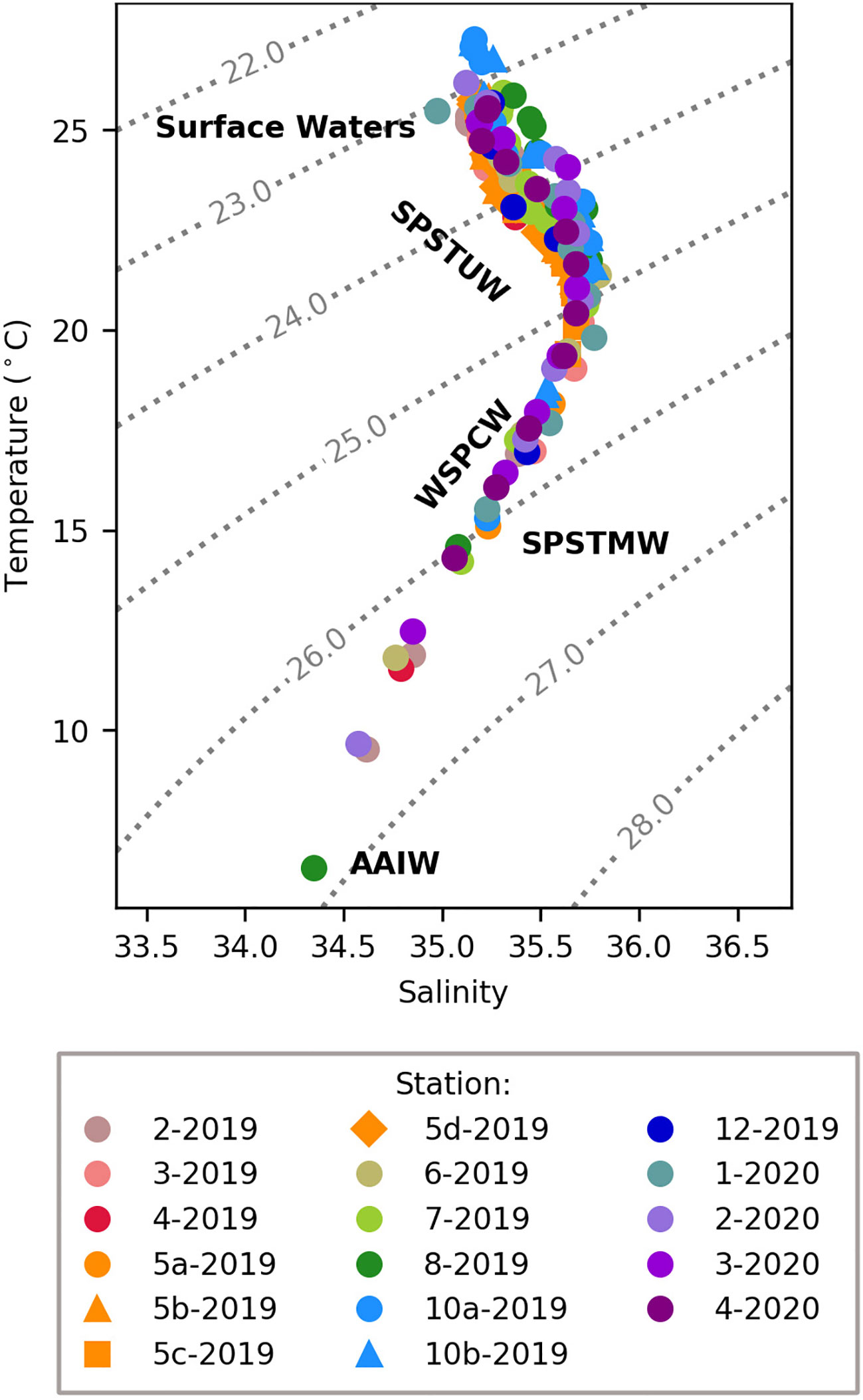
Figure 2 Temperature-salinity plot of samples from the 2019 and 2020 TONGA cruises as well as the prevalent water masses across the transect. The dotted grey lines indicate potential density ( σθ) isopycnals. The water masses include surface waters, South Pacific Subtropical Under Water (SPSTUW), Western South Pacific Central Water (WSPCW), South Pacific Subtropical Mode Water (SPSTMW), a subsidiary of WSPCW and Antarctic Intermediate Water (AAIW).
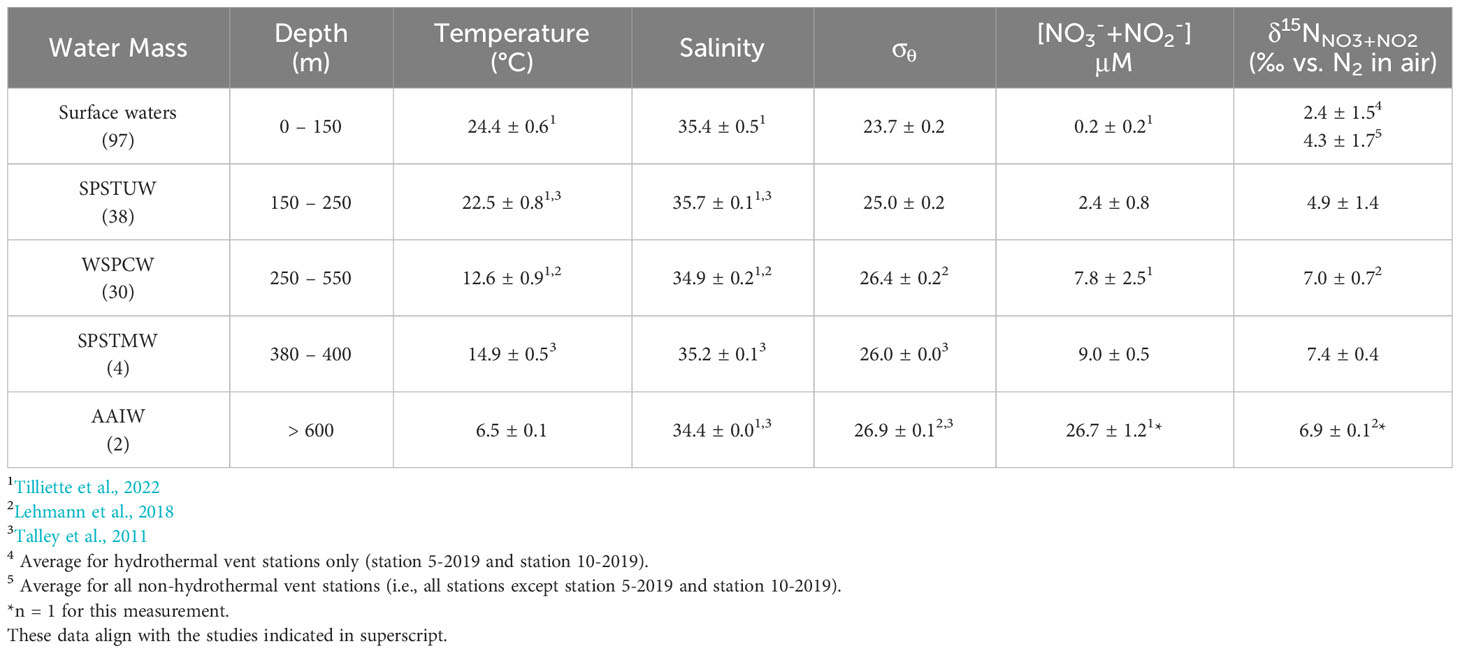
Table 1 Water masses identified from the 2019 and 2020 TONGA cruises and their average (± 1 S.D.) hydrographic and NO3-+NO2- properties and number (n) of samples from each water mass.
4 Results
4.1 NO3-+NO2- concentration and δ15N
The [NO3-+NO2-] in the upper 100 m of the WTSP was ≤0.1 μM except at stations influenced by the hydrothermal vents, i.e., stations 5-2019 and 10-2019, where the [NO3-+NO2-] was 0.2 ± 0.0 μM to 0.7 ± 0.0 μM (average ±1 S.D.) between 60 m and 100 m (Figures 3A, C). At 400 m, the [NO3-+NO2-] increased to between 10 and 15 μM, corresponding to δ15NNO3+NO2 ranging from 6 to 8‰ (Figures 3B, D). This is consistent with the presence of WSPCW (Tilliette et al., 2022) and more particularly, SPSTMW (Lehmann et al., 2018) and aligns with other studies in the region (Knapp et al., 2018). At station 8-2019, the [NO3-+NO2-] increased to 26.7 μM at 630 m with a corresponding δ15NNO3+NO2 of 6.9 ± 0.1‰ (Figures 3A, C), consistent with AAIW (Talley et al., 2011; Lehmann et al., 2018; Tilliette et al., 2022). The majority of stations within MW and the LB (i.e., stations 1-2019 to 5-2019, 10-2019, and 12-2019) had δ15NNO3+NO2 ranging from 4 to 6‰ at 200 m, decreasing shallower in the water column to 2 to 4‰ at 150 m associated with the transition between SPSTUW and surface waters (Figures 3B, D). The SPG stations (stations 6-2019, 7-2019 and 8-2019) had an average δ15NNO3+NO2 of 6.8 ± 0.0‰ at ~200 m characteristic of SPSTUW. This δ15NNO3+NO2 was significantly higher than the δ15NNO3+NO2 at 200 m in MW and LB samples, where the average δ15NNO3+NO2 at 200 m was 5.0 ± 0.6‰ and 5.1 ± 0.6‰, respectively (p<0.5 and p<0.01, respectively, evaluated with the Kruskal-Wallis test; Kruskal and Wallis, 1952). Furthermore, the elevated δ15NNO3+NO2 in SPG SPSTUW corresponded to significantly lower [NO3-+NO2-] at 200 m (2.4 ± 0.8 μM) compared to the LB, where the average [NO3-+NO2-] at 200 m was 3.3 ± 0.6 μM (p<0.01, Kruskal and Wallis, 1952) (Figures 3A, C). Within the surface waters (upper 150 m), the δ15NNO3+NO2 at hydrothermal station 5-2019 was significantly lower between the four casts (5a – d), 1.8 ± 0.9‰, compared to the average δ15NNO3+NO2 in the upper 150 m at hydrothermal station 10 (10 a, b), 4.4 ± 0.6‰ (p<0.01, Kruskal and Wallis, 1952) (Figures 3B, D). The lowest δ15NNO3+NO2, 0.7 ± 0.1‰, was observed at station 5c-2019 at 100 m, above which δ15NNO3+NO2 increased to 1.0 ± 0.0‰ at 95 m (Figures 3A, C). The δ15NNO3+NO2 reported here for the TONGA study are publicly available (Knapp and Forrer, 2023).
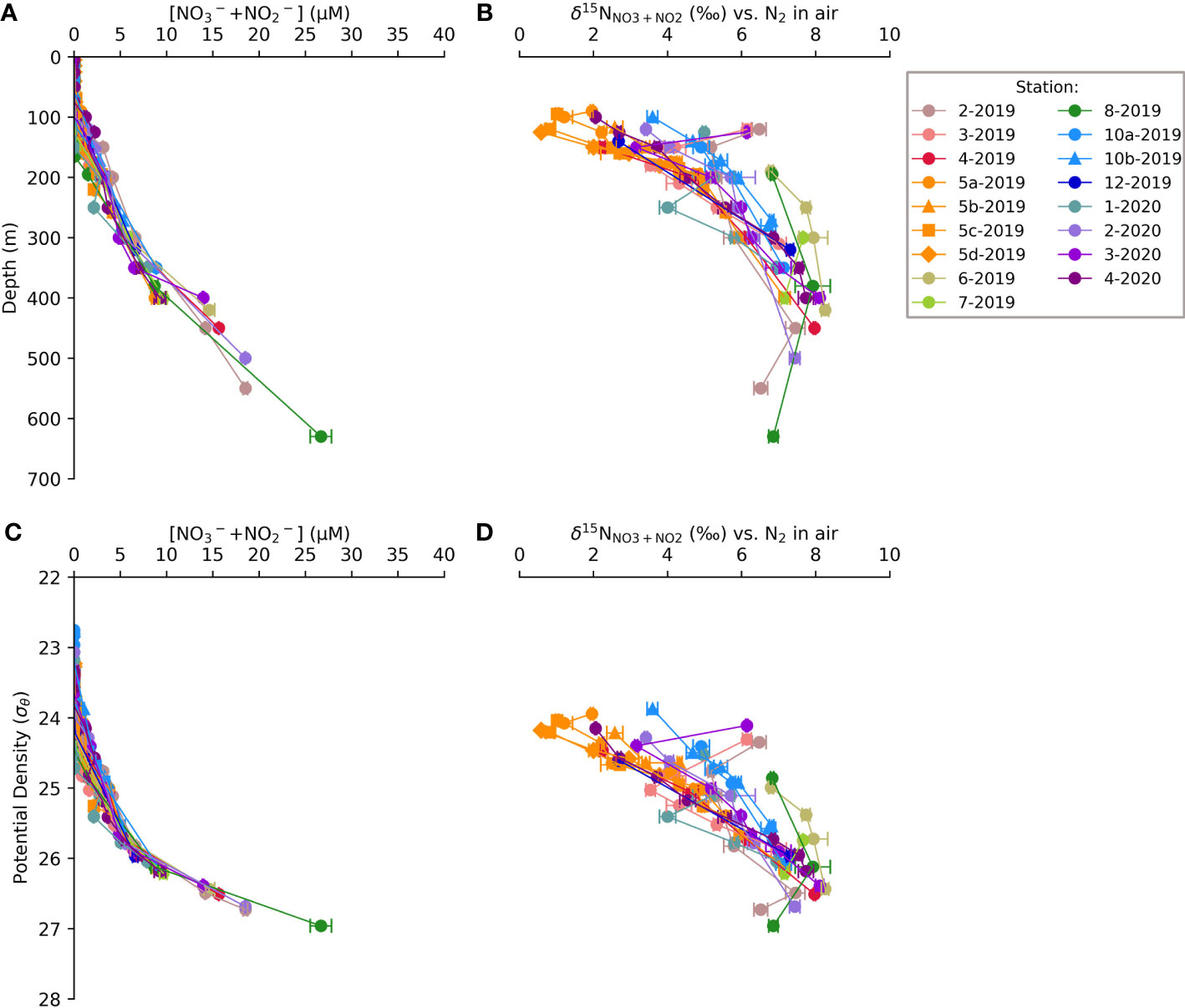
Figure 3 Water column profiles of [NO3-+NO2-] and δ15NNO3+NO2 vs. depth of samples from the 2019 and 2020 TONGA cruises (A, B) and vs. potential density (C, D). Error bars represent ±1 S.D. and are often smaller than the symbol size.
4.2 PNsink flux and δ15N
The PNsink flux collected in the shallow short-term drifting traps was calculated to be 350 µmol N m-2 d-1 (170 m, station 5a-2019), 436 μmol N m-2 d-1 (270 m, station 5a-2019), and 693 μmol N m-2 d-1 (270 m, station 10a-2019) (Table 2). Further, the average (± 1 S.D.) δ15NPNsink was -0.5 ± 3.5‰ and -0.2 ± 1.9‰ at 170 m and 270 m at station 5a-2019, respectively, and -0.6 ± 2.3‰ at 270 m at station 10a-2019 (Table 2) (Figure 4). In comparison, the annual average PNsink flux collected in the long-term 1000 m PPS5 moored trap at station 12-2019/4-2020 was an order of magnitude lower, 16.5 ± 14.3 μmol N m-2 d-1, and had a higher annual mass-weighted average (± 1 S.D.) δ15NPNsink, 3.4 ± 1.9‰, compared to the shallower, short-term traps (Table 2) (Figure 4).
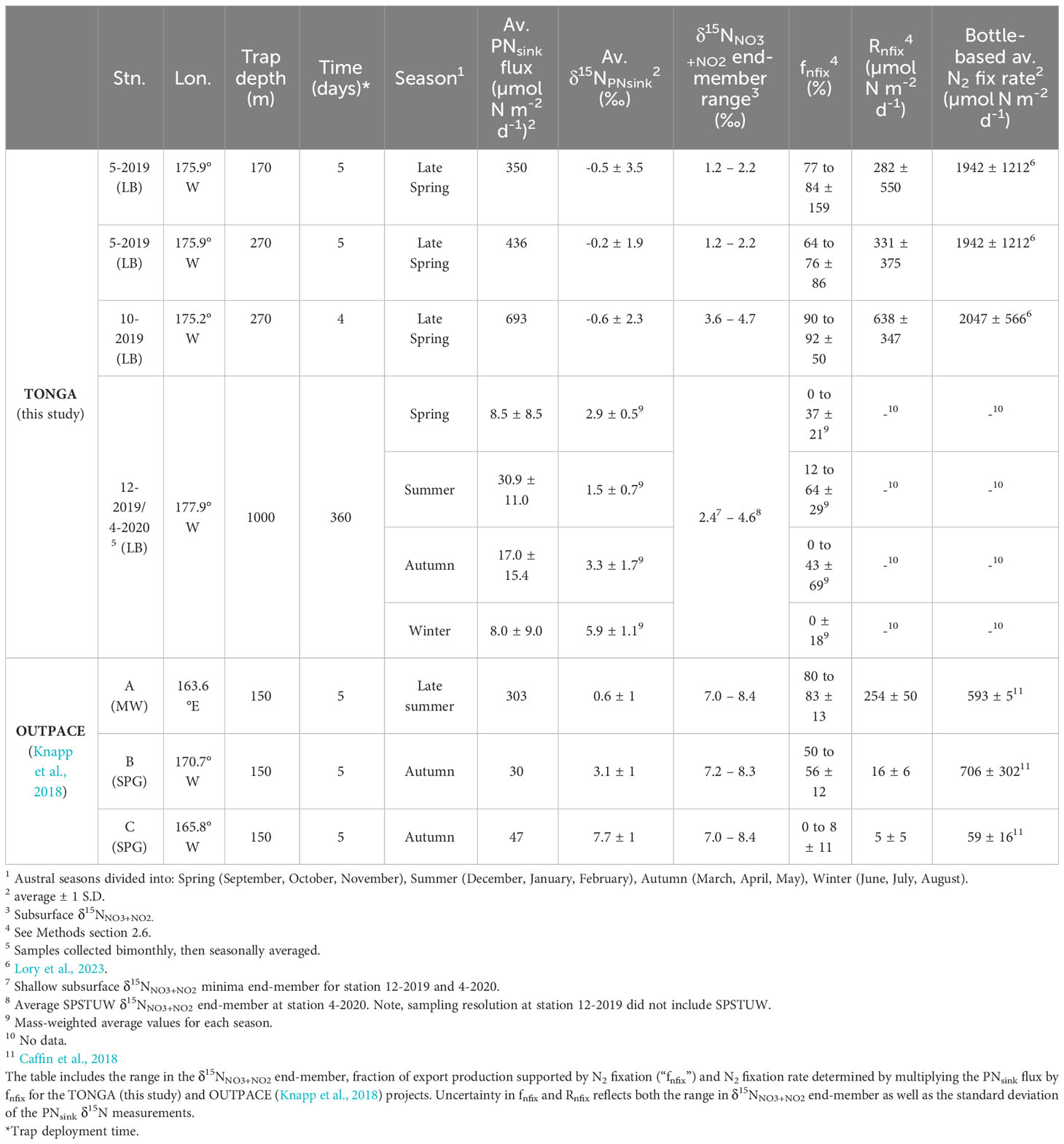
Table 2 The mass and isotopic composition of the sinking particulate N (PNsink) flux captured in the short-term PIT and long-term PPS5 traps, and results of the δ15N budgets.
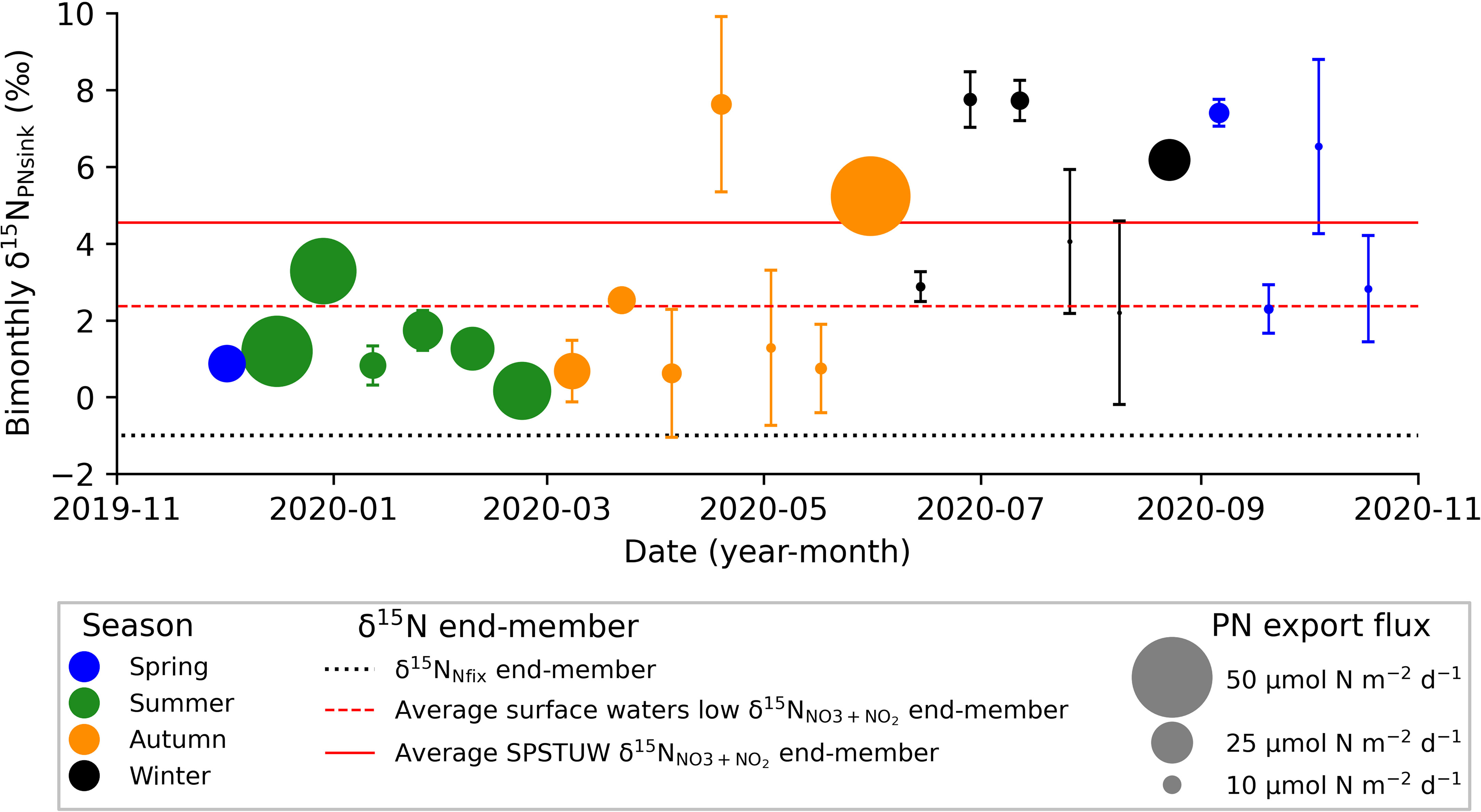
Figure 4 Bimonthly measurements of PNsink flux and δ15NPNsink from the 1000 m trap deployed at station 12-2019/station 4-2020 between November 2019 to October 2020. Circle color corresponds to the season when the majority of the PNsink was collected over bimonthly sampling intervals and circle size corresponds to PNsink flux magnitude (μmol N m-2 d-1). Each measurement is plotted at the end date of the two-week sampling interval. The δ15NNfix end-member (-1‰) is represented by the dotted black line and the low δ15NNO3+NO2 end-member at station 12-2019 and 4-2020 (2.4‰) and average SPSTUW δ15NNO3+NO2 end-member at station 4-2020 (4.6‰) are represented by the dashed and solid lines, respectively. Data available in Supplementary Table S1.
The seasonal average (± 1 S.D.) PNsink fluxes collected in the deep, moored trap were higher in the austral summer and autumn, at 30.9 ± 11.0 μmol N m-2 d-1 and 17.0 ± 15.4 μmol N m-2 d-1, respectively, compared to the average austral winter and spring PNsink fluxes of 8.0 ± 9.0 μmol N m-2 d-1 and 8.5 ± 8.5 μmol N m-2 d-1, respectively (Table 2) (Figure 4). The average, mass-weighted (± 1 S.D.) summer δ15NPNsink from the 1000 m PPS5 moored trap, 1.5 ± 0.7‰, was lower than the wintertime average of 5.9 ± 1.1‰, while the average (± 1 S.D.) spring and autumn mass-weighted δ15NPNsink, 2.9 ± 0.5‰ and 3.3 ± 1.7‰, respectively, were intermediate between summer and winter values.
4.3 Results of the δ15N budget
The δ15N budget described above compares the δ15N of the primary form of N exported from the euphotic zone, δ15NPNsink, with the δ15N of the two input terms, subsurface NO3-+NO2- and N2 fixation. This provides a geochemically-derived estimate of the fractional contribution of N2 fixation to export production (fnfix) as well as rate of N2 fixation (Rnfix). Given that the subsurface δ15NNO3+NO2 source is difficult to constrain, we evaluate the shallow δ15N budgets using the shallowest subsurface δ15NNO3+NO2 minima as well as the sample immediately below the minima (Knapp et al., 2021), while the δ15N budget using the deep trap PNsink flux uses the average subsurface δ15NNO3+NO2 minima at station 12-2019/4-2020 and the average SPSTUW δ15NNO3+NO2 at station 4-2020. At the shallow trap stations in close proximity to the hydrothermal vents, the subsurface δ15NNO3+NO2 ranged from 1.2 to 2.2‰ and 3.6 to 4.7‰ at stations 5-2019 and 10-2019, respectively, while at the deep mooring (station 12-2019/4-2020) the average subsurface δ15NNO3+NO2 minima and SPSTUW δ15NNO3+NO2 were 2.4‰ and 4.6‰, respectively (Table 2). Additional uncertainty in the δ15N budgets includes the standard deviation of the δ15NPNsink analysis (Table 2). The δ15N value for N2 fixation, (δ15NNfix) of -1‰ is based on literature reports of diazotrophic biomass δ15N (Hoering and Ford, 1960; Minagawa and Wada, 1986; Carpenter et al., 1997) (Table 2). Comparing these values with the δ15NPNsink, we calculate that at station 5-2019, the fnfix ranged from 77 to 84 ± 159% for the 170 m trap, while the fnfix ranged from 64 to 76 ± 86% at the 270 m trap (Table 2). At station 10-2019, the fnfix at the 270 m trap ranged from 90 to 92 ± 50% (Table 2). We further calculate a geochemically derived Rnfix of 282 ± 550 µmol N m-2 d-1 and 331 ± 375 µmol N m-2 d-1 for the 170 m and 270 m traps deployed at station 5-2019, respectively, and 638 ± 347 µmol N m-2 d-1 for the 270 m trap deployed at station 10-2019 (Table 2). The δ15N budget calculated for the moored trap at station 12-2019/4-2020 (note that the trap is deeper, i.e. 1000 m instead of 170 – 270 m for the drifting ones) yielded a mass-weighted fnfix of 12 to 64 ± 29% (average = 42 ± 13%) for the summer, while the spring and autumn had similar but lower mass-weighted fnfix of 0 to 37 ± 21% (average = 0 ± 29%) and 0 to 43 ± 69% (average = 12 ± 36%), respectively, and winter had a mass-weighted fnfix of 0 ± 18%. These fnfix values indicate that the highest contribution of N2 fixation to export production collected at 1000 m was during the summer, followed by autumn and spring. Due to significant PNsink flux attenuation with depth (Martin et al., 1987), we do not calculate a Rnfix for the moored trap data.
5 Discussion
5.1 TONGA δ15N budgets reflect high rates of N2 fixation in the WTSP
The results of the short-term δ15N budgets suggest that N2 fixation rates were high (282 to 638 μmol N m-2 d-1) near the hydrothermal vents at the time of the 2019 cruise. While there are notable differences in the magnitude of these geochemically-derived N2 fixation rates and the bottle-based 15N2 uptake rates reported by Lory et al., 2023, we consider these results to be broadly consistent with one another, as well as consistent with estimates of diazotroph abundance measured contemporaneously (Bonnet et al., 2023b). Specifically, bottle-based 15N2 uptake rates were 3 to 7 times higher than the Rnfix estimated from the δ15N budget (Table 2); however, both rate estimates are at the upper-end typically reported by each technique (Gruber, 2004; Capone et al., 2005; Casciotti et al., 2008; Luo et al., 2012; Knapp et al., 2016a) and align with previous work from the region (Montoya et al., 2004; Berthelot et al., 2017; Bonnet et al., 2017; Knapp et al., 2018; Shao et al., 2023). Prior work investigating N2 fixation’s contribution to export production has attributed discrepancies between these two metrics to potential sediment trap under collection of exported material (Knapp et al., 2016a; Böttjer et al., 2017, Knapp et al., 2018), alternate sources of fixed N to the euphotic zone including horizontal advection (Böttjer et al., 2017), phytoplankton bloom stage as well as temporal delay between organic matter formation and capture by the sediment trap (de Verneil et al., 2017; Caffin et al., 2018; Knapp et al., 2018), and bottle-based 15N2 incubations with their associated methodological considerations (i.e., bottle effects) (White et al., 2020). Additionally, our δ15N budgets should be considered a lower bound for estimated N2 fixation rates because of the mixing-model’s inherent assumption that the only fate of newly fixed N is to be balanced by the sinking flux, and that no newly fixed N is released to the dissolved pool, which is likley unrealistic (Capone et al., 1994; Glibert & Bronk, 1994; Mulholland and Capone, 2004; Bonnet et al., 2016; Knapp et al., 2016b). Further, the trap depth used here, 170 m, is below the base of the mixed layer, and thus underestimates new/export production. While euphotic zone nitrification is a source of low-δ15NNO3 that could lead to an overestimation of N2 fixation supported export production, rates of euphotic zone nitrification from this and other similar oligotrophic regions are low (<10 nmol L-1 d-1) (Smith et al., 2014; Raes et al., 2020) compared to the very high rates of N2 fixation using multiple metrics in this study. This therefore suggests that N2 fixation is the dominant mechanism generating the low-δ15NPNSink signal observed.
Regardless of the mechanism(s) driving these discrepancies, both the δ15N budget and 15N2 uptake rate estimates are also consistent with the elevated diazotroph abundances (Bonnet et al., 2023a; Lory et al., 2023) observed on the 2019 TONGA cruise (Figure 5). Here we compare averages of biological and geochemical metrics associated with N2 fixation for the three hydrographic regions, MW, the LB, and the SPG (defined in Section 2.1). While results from the qPCR analysis targeting nifH genes indicate that Trichodesmium spp. and UCYN-A dominated the diazotroph assemblage in the upper 50 m across the TONGA transect, Trichodesmium spp. were most abundant in the LB near the hydrothermal vents (average 1.0x107 gene copies L-1), followed by UCYN-A (average 3.0x106 gene copies L-1) (Figures 5A, B). These exceptionally high abundances of Trichodesmium spp. and UCYN-A were on the order of one to four times higher than previous studies (e.g., Zehr & Turner, 2001; Moisander et al., 2010; Turk-Kubo et al., 2012; Moisander et al., 2014; Benavides et al., 2018; Benavides et al., 2020; Confesor et al., 2022). Notably, the highest regionally-averaged, trapezoidally-integrated upper 50 m 15N2 uptake rates were found in the LB (1038 ± 600 µmol N m-2 d-1), where Trichodesmium spp. were most abundant and where the lowest regionally-averaged δ15NNO3+NO2 subsurface minima of 2.8 ± 1.5‰ (Figure 5F) was observed between 100 and 200 m. This underscores the potential significance of Trichodesmium spp. supporting N2 fixation near the hydrothermal vents. UCYN-A was the dominant diazotroph in the upper 50 m in MW and the SPG, with regional averages of 2.5x108 gene copies L-1 and 3.1x108 gene copies L-1, respectively, followed by Trichodesmium spp., with regional averages of 3.0x105 and 3.7x105 gene copies L-1, respectively. Average abundances of UCYN-B (2.6x104 to 3.2x105 gene copies L-1), UCYN-C (4.3x101 to 1.7x102 gene copies L-1) and Gamma proteobacteria (5.4x103 to 9.9x103 gene copies L-1) remained comparatively low across the transect (Figures 5C–E), but were similar in magnitude to previous studies in the region (Moisander et al., 2014; Benavides et al., 2018; Benavides et al., 2020). Although UCYN-A have extremely high gene abundances and dominate the upper 50 m in MW and the SPG, the trapezoidally-integrated upper 50 m 15N2 uptake rates were lower than in the LB, 713 ± 691 and 537 ± 629 µmol N m-2 d-1, respectively, and were associated with a higher regional average δ15NNO3+NO2 subsurface minima of 4.4 ± 1.2‰ and 5.5 ± 1.9‰, respectively, observed between 100 to 200 m (Figure 5F). While care should be taken when relating nifH gene copies to diazotroph biomass, these gene copy abundances broadly correspond to elevated diazotroph abundances (Meiler et al., 2022) and confirm the significance of diazotrophy in the region.
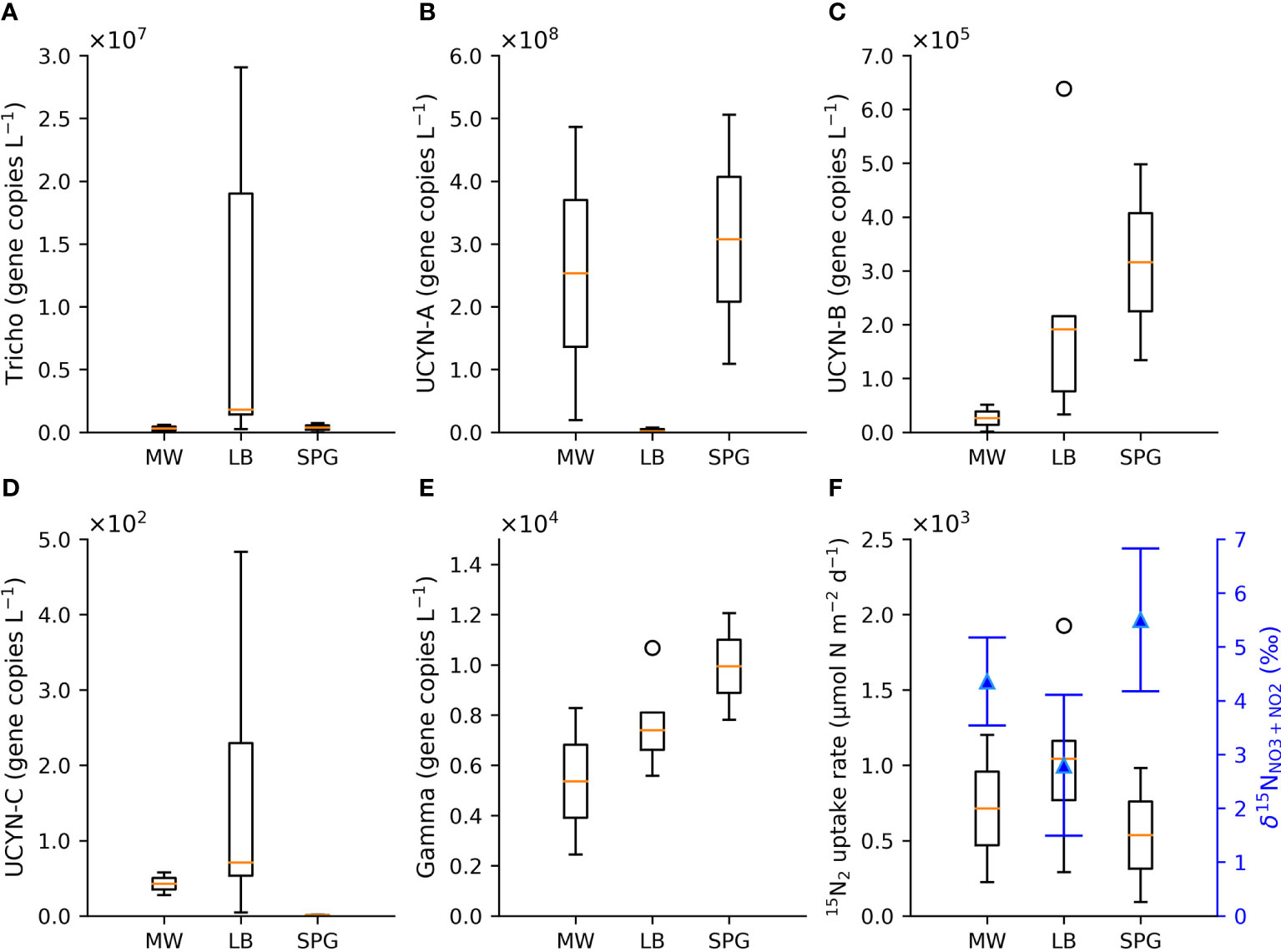
Figure 5 Box plots of the upper 50 m nifH gene abundances (gene copies L-1) from Bonnet et al., 2023a and Lory et al., 2023 for the hydrographic regions of MW, the LB and the SPG for (A) Trichodesmium spp., (B) UCYN-A, (C) UCYN-B, (D) UCYN-C, and (E) Gamma proteobacteria, as well as (F) box plots of the regional average, trapezoidally-integrated upper 50 m 15N2 fixation rates from Lory et al., 2023, with the corresponding regional average 90 to 195 m δ15NNO3+NO2 (blue triangles). The open circles associated with the box plots indicate outliers.
5.2 N2 fixation is an important source of N supporting export production in the WTSP
The agreement between the geochemically-derived N2 fixation rates, 15N2 uptake rates and diazotroph abundances together indicate that export production in the WTSP at the time of this study was driven by N2 fixation (Bonnet et al., 2023b). This is in contrast to prior work in other oligotrophic regions where the majority of export was supported by subsurface NO3-, even when N2 fixation inputs were high (Casciotti et al., 2008; Bourbonnais et al., 2009; White et al., 2013; Böttjer et al., 2017). The shallow sediment traps deployed for the TONGA project indicate that N2 fixation supports a majority of export production (fnfix = 64 to 92%) near the hydrothermal vents, at least in the late spring (Table 2; Figures 6A, B). These fnfix values are similar to those calculated from the previous OUTPACE campaign during the late summer/early autumn in MW (station A, 80 to 83 ± 13%), and near the Tonga-Kermadec Arc (station B, 50 to 56 ± 12%) (Table 2; Figure 6C) (Knapp et al., 2018). The high fnfix values reported here from the short-term traps deployed at TONGA Stations 5 and 10 replicate a geochemical signature that has not been observed outside of the WTSP, underscoring the significance of N2 fixation regionally (Bonnet et al., 2023b). We also emphasize the potential for the fnfix from our δ15N budgets to underestimate the importance of N2 fixation to export due to the mixing-model’s inherent assumption that the only fate of newly fixed N is the PNsink flux captured by the sediment traps, as opposed to being released and persisting as dissolved organic nitrogen (Capone et al., 1994; Glibert and Bronk, 1994).
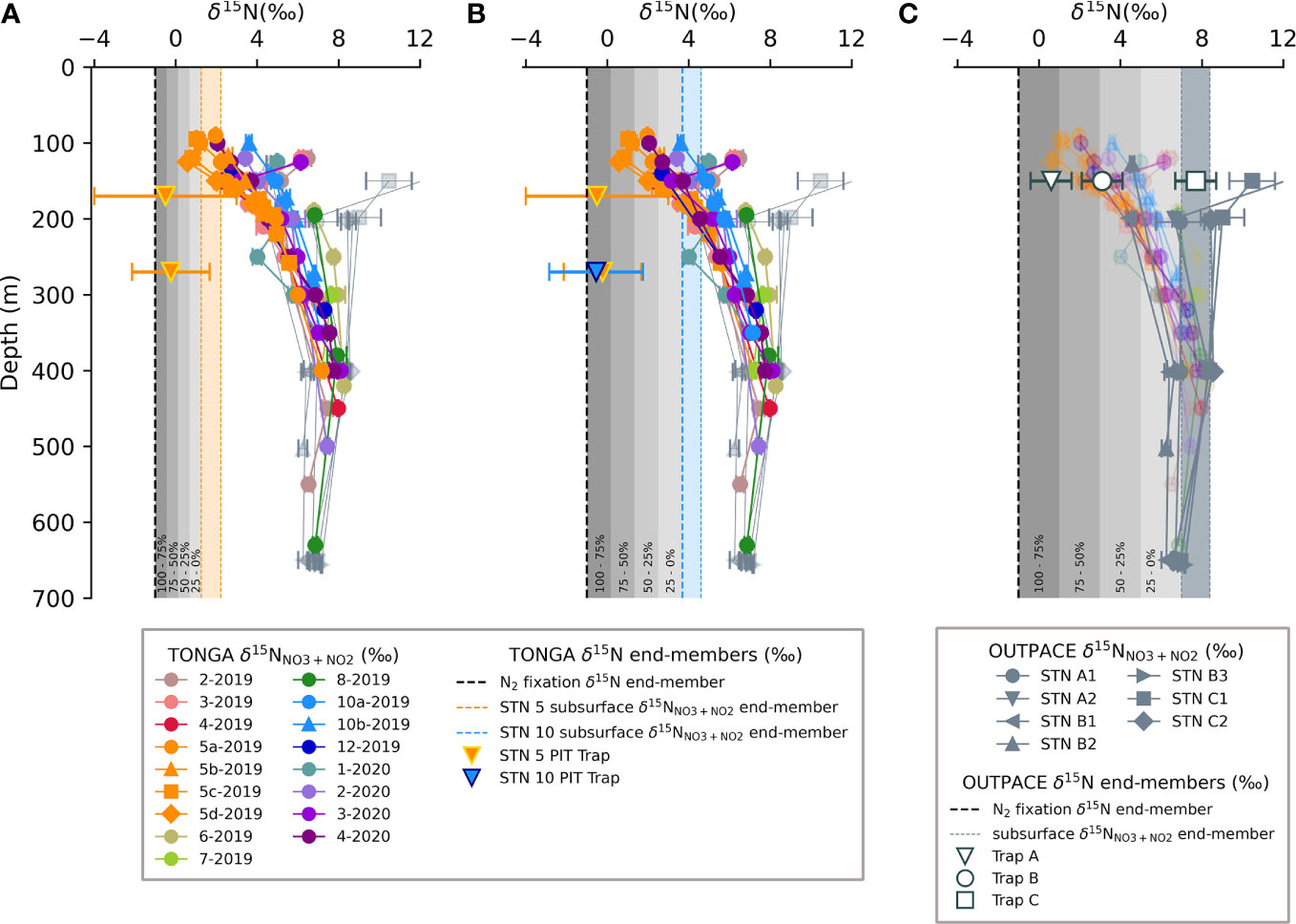
Figure 6 The fraction of N2 fixation-supported export production in the austral spring at (A) TONGA station 5-2019, and, (B) TONGA station 10-2019, compared with (C) results from the austral late summer/early autumn at OUTPACE stations( A-C) Each panel includes water column profiles of δ15NNO3+NO2 for samples from the 2019 and 2020 TONGA cruises in color and the OUTPACE cruise in grey and the associated δ15N budget terms, including the sediment trap δ15NPNsink (inverted triangles and open circle and square), the δ15NNfix (vertical dashed line) and ranges in subsurface δ15NNO3+NO2, which are represented by the orange shaded region in (A), blue shaded region in (B), and steel-grey shaded region in (C) (Table 2). The fractional contribution of N2 fixation to export production is indicated by the grey shading and the corresponding fraction is indicated along the bottom of each panel.
While the results of the short-term δ15N budgets from both the TONGA and OUTPACE campaigns found that N2 fixation supports the majority of export production in the late spring, late summer, and early autumn in the LB and MW, we also consider the δ15NPNsink collected in the deep, moored trap at station 12-2019/4-2020 in the LB to evaluate this trend over an annual cycle (Figure 4). Unsurprisingly, the mass flux collected in the shallower, short-term PIT traps was higher and the δ15NPNsink was lower than that collected in the deeper, moored trap. However, we note that the moored trap was deployed ~200 km west of the shallow traps and hydrothermal vents (Table 2) (Figures 1, 4), and the conical shape of the PPS5 has been observed to undersample (Baker et al., 2020; Tilliette et al., 2023). The δ15NPNsink in the shallow PIT traps collected in the late spring at stations 5-2019 and 10-2019 ranged from -0.2 to -0.6‰ compared to a seasonal average δ15NPNsink of 2.9 ± 0.5‰ and 1.5 ± 0.7‰ observed in the moored PPS5 trap during the spring and summer, respectively. We expect that the higher δ15NPNsink found in the deeper moored trap likely resulted from the collection of PNsink from a larger surface area than the shallow short-term traps, where export production may have been supported by a mixture of N sources with higher δ15N (Siegel and Deuser, 1997; Siegel et al., 2008). Additionally, horizontal advection of particles generated at locations not impacted by the shallow hydrothermal vents potentially decouples the euphotic zone diazotrophic abundance and/or importance from the PN collected in the moored trap (Waniek et al., 2000). Indeed, the exported material in the deep trap was observed to be compositionally different from that captured in the shallow, short-term traps, where the hydrothermal signature of the particles was less evident due to organic matter remineralization while being transported to depth (Tilliette et al., 2023). The associated distance and time components of sinking PN to the deep trap potentially underestimates the importance of N2 fixation to export production. Similar flux and isotopic composition offsets have been observed between shallow and deep sediment traps in the ETSP (Berelson et al., 2015; Knapp et al., 2016a; Tilliette et al., 2023).
Since aerosol inputs to this region are minimal (Guieu et al., 2018), we expect that the seasonal variability of δ15NPNsink in the deep, moored trap reflects shifts in the importance of N2 fixation and subsurface NO3- for supporting export production over seasonal timescales. In the deep trap, annual PNsink fluxes peaked in the summer (30.9 ± 11.0 μmol N m-2 d-1), coinciding with the lowest average mass-weighted δ15NPNsink, 1.5 ± 0.7‰, while the lowest PNsink fluxes were observed in the winter (8.0 ± 9.0 μmol N m-2 d-1) and coincided with the highest average mass-weighted δ15NPNsink, 5.9 ± 1.1‰, indicating that isotopically distinct N sources support export seasonally. Since there are steep gradients in both [NO3-+NO2-] and δ15NNO3+NO2 with depth (Figures 3, 6), a higher winter δ15NPNsink may reflect entrainment of a deeper NO3-+NO2- source (likely SPSTUW) with a higher δ15NNO3+NO2 due to winter mixing (Moutin et al., 2018). The net effect of a higher subsurface δ15NNO3+NO2 end-member would be to raise the estimated fnfix (Table 2) (Böttjer et al., 2017). As a result, the mass-weighted seasonal fnfix values for the deep trap range from 12 to 64 ± 29% in the summer and 0 ± 18% in the winter, describing a largely N2 fixation supported export system in the summer. Further, since the majority of annual export is focused in the summer, and was supported by low-δ15N N sources, we attribute an important fraction of annual export production and deep (>1000 m) long-term C sequestration to N2 fixation at station 12-2019/4-2020 (Figure 4; Table 2).
Considering the elevated chlorophyll a concentrations observed over a large area in this region ranging up to 360,000 km2 (Bonnet et al., 2023b), and given the high Rnfix and fnfix values estimated at station 5a-2019 and 10a-2019, along with the large fraction of N2 fixation supported export production at station 12-2019/4-2020 over an annual timescale, the otherwise oligotrophic WTSP appears to be biogeochemically unique where N2 fixation supports a large fraction of annual export production as a result of the influence of shallow hydrothermal vents. The significance of these regional N2 fixation inputs in the WTSP are further pronounced in the gradients of water column δ15NNO3+NO2 both zonally as well as with depth (Figures 3, 6). In particular, δ15NNO3+NO2 between 150 and 400 m decreases from east to west (SPG to MW) across the zonal transect, and also decreases from ~400 m to shallower depths. These isotopic gradients reflect the accumulation of low-δ15N N inputs in the upper thermocline to the west along this transect that are presumably associated with the remineralization of diazotrophic inputs (Casciotti et al., 2008; Knapp et al., 2008). This accumulation of low-δ15NNO3+NO2 in the upper 400 m of the WTSP erodes the elevated δ15NNO3+NO2 originating from dissimilatory NO3- reduction occurring in the oxygen deficient zones of the ETSP (Bourbonnais et al., 2015; Peters et al., 2018; Casciotti et al., 2013), a geochemical signature that reflects basin-scale compensation of N losses in the east with N inputs in the west that is consistent with peloceanographic records (Brandes and Devol, 2002; Deutsch et al., 2004; Weber and Deutsch, 2014; Knapp et al., 2018).
6 Conclusions
Here we report results of δ15N budgets that compare subsurface δ15NNO3+NO2 with the δ15NPNsink captured in short-term, shallow (170 and 270 m) PIT deployed near the hydrothermal vents of the Tonga-Kermadec Arc and long-term, deep (1000 m) moored PPS5 sediment traps deployed ~200 km west of the Arc. These results are evaluated in the context of 15N2 uptake rates (Lory et al., 2023) and nifH gene abundances (Bonnet et al., 2023b) collected contemporaneously, as well as with prior work from the region (Knapp et al., 2018). Results from the short-term, shallow δ15N budgets indicate that N2 fixation supports the majority, 64 to 92%, of export in late spring in the Lau Basin, while the mass-weighted, seasonally-averaged δ15N budgets from deeper traps suggest that N2 fixation supports 12 to 64% of export production and thus long-term C sequestration in the summer when the highest PNsink fluxes are observed. As the seasons progress into winter, export production becomes increasingly supported by subsurface NO3-. The observations from this cruise as well as from the OUTPACE study (Knapp et al., 2018) are in contrast to other regions explored so far, where even significant N2 fixation inputs do not support the majority of export (Casciotti et al., 2008; Bourbonnais et al., 2009; Böttjer et al., 2017), underscoring the significance of diazotrophy in the WTSP. While diazotroph abundance was high across the transect, Trichodesmium spp. nifH gene copies were highest in the vicinity of the hydrothermal vents, which appear shallow enough to meet the considerable Fe demands of primary productivity in general (Tilliette et al., 2022), and N2 fixation in particular, in the region (Bonnet et al., 2023b), highlighting the sensitivity of N2 fixation to Fe availability. These results suggest that the significant N2 fixation inputs to the WTSP in the late spring, summer, and early autumn work to lower the elevated upper thermocline δ15NNO3+NO2 originating from dissimilatory NO3- reduction in the oxygen deficient zones of the Eastern Tropical South Pacific.
Data availability statement
The datasets presented in this study can be found in online repositories. The names of the repository/repositories and accession number(s) can be found in the article/Supplementary Material.
Author contributions
SB, CG, and AK designed the study. SB and CG carried out the sampling. HF and RT carried out the δ15NNO3+NO2 sample analysis and data aqusition. SB and OG carried out the δ15NPNsink sample analysis and data aqusition. HF and AK wrote the first manuscript draft, which was then revised by all authors. All authors contributed to the article and approved the submitted version.
Funding
The author(s) declare financial support was received for the research, authorship, and/or publication of this article. This research is a contribution to the TONGA project (Shallow hydroThermal sOurces of trace elemeNts: potential impacts on biological productivity and the biological carbon pump; TONGA cruise https://doi.org/10.17600/18000884) funded by the Agence Nationale de la Recherche (grant TONGA ANR-18- CE01-0016), the LEFE-CyBER program (CNRS-INSU), the A-Midex foundation, the TGIR Flotte océanographique française, the Institut de Recherche pour le Développement (IRD). HF acknowledges funding from the Winchester Fund at Florida State University’s EOAS Department. AK acknowledges NSF OCE-1829797 for supporting the nitrate isotopic analyses.
Acknowledgments
The authors warmly thank the captain and crew of the R/V L’Atalante and R/V Alis (both TGIR Flotte, operated by IFREMER) for outstanding shipboard operations. Vincent Taillandier and Nagib Bhairy are thanked for the CTD rosettes management, and data processing, Sandra Nunige for nutrient analyses of the 2019 samples, Mary Dennis for her assistance with the ‘denitrifier method’ at Florida State University, Caroline Lory for her assistance with the 15N2 fixation experiments and data aqusition and Nathalie Leblond (Cellule Piège INSU) for the treatment/splitting of the moored trap.
Conflict of interest
The authors declare that the research was conducted in the absence of any commercial or financial relationships that could be construed as a potential conflict of interest.
Publisher’s note
All claims expressed in this article are solely those of the authors and do not necessarily represent those of their affiliated organizations, or those of the publisher, the editors and the reviewers. Any product that may be evaluated in this article, or claim that may be made by its manufacturer, is not guaranteed or endorsed by the publisher.
Supplementary material
The Supplementary Material for this article can be found online at: https://www.frontiersin.org/articles/10.3389/fmars.2023.1249115/full#supplementary-material
References
Altabet M. A. (1988). Variations in nitrogen isotopic composition between sinking and suspended particles: implications for nitrogen cycling and particle transformation in the open ocean’, Deep Sea Research Part A. Oceanographic Res. Papers 35.4), 535–554. doi: 10.1016/0198-0149(88)90130-6
Aminot A., Kerouel R. (2007). Dosage automatique des nutriments dans les eaux marines: méthodes en flux continu, Ifremer, Plouzané.
Baker C. A., Estapa M. L., Iversen M., Lampitt Buesseler R. K. (2020). Are all sediment traps created equal? An intercomparison study of carbon export methodologies at the PAP-SO site. Prog. Oceanography 184, 102317. doi: 10.1016/j.pocean.2020.102317
Benavides M., Martias C., Elifantz H., Berman-Frank I., Dupouy C., Bonnet S. (2018). Dissolved organic matter influences N2 fixation in the New Caledonian lagoon (Western tropical south pacific). Front. Mar. Sci. 5, 89. doi: 10.3389/fmars.2018.00089
Benavides M., Duhamel S., Van Wambeke F., Shoemaker K. M., Moisander P. H., Salamon E., et al. (2020). Dissolved organic matter stimulates N2 fixation and nifH gene expression in Trichodesmium. FEMS Microbiol. Lett. 367 (4), fnaa034. doi: 10.1093/femsle/fnaa034
Berelson W. M., Haskell W. Z., Prokopenko M., Knapp A. N., Hammond D. E., Rollins N., et al. (2015). Biogenic particle flux and benthic remineralization in the Eastern Tropical South Pacific. Deep Sea Res. Part I: Oceanographic Res. Papers 99, 23–34. doi: 10.1016/j.dsr.2014.12.006
Berman-Frank I., Cullen J. T., Shaked Y., Sherrell R. M., Falkowski P. G. (2001). Iron availability, cellular iron quotas, and nitrogen fixation in Trichodesmium. Limnology Oceanography 46 (6), 1249–1260. doi: 10.4319/lo.2001.46.6.1249
Berthelot H., Benavides M., Moisander P. H., Grosso O., Bonnet S. (2017). High-nitrogen fixation rates in the particulate and dissolved pools in the Western Tropical Pacific (Solomon and Bismarck Seas). Geophysical Res. Lett. 44 (16), 8414–8423. doi: 10.1002/2017GL073856
Bonnet S., Biegala I. C., Dutrieux P., Slemons L. O., Capone D. G. (2009). Nitrogen fixation in the western equatorial Pacific: Rates, diazotrophic cyanobacterial size class distribution, and biogeochemical significance: N2 FIXATION IN THE EQUATORIAL PACIFIC. Global Biogeochemical Cycles 23 (3). doi: 10.1029/2008GB003439
Bonnet S., Berthelot H., Turk-Kubo K., Fawcett S., Rahav E., L’Helguen S., et al. (2016). Dynamics of N2 fixation and fate of diazotroph-derived nitrogen in a low-nutrient, low-chlorophyll ecosystem: results from the VAHINE mesocosm experiment (New Caledonia). Biogeosciences 13 (9), 2653–2673. doi: 10.5194/bg-13-2653-2016
Bonnet S., Caffin M., Berthelot H., Moutin T. (2017). Hot spot of N 2 fixation in the western tropical South Pacific pleads for a spatial decoupling between N 2 fixation and denitrification. Proc. Natl. Acad. Sci. 114 (14). doi: 10.1073/pnas.1619514114
Bonnet S., Benavides M., Le Moigne F. A. C., Camps M., Torremocha A., Grosso O., et al. (2023a). Diazotrophs are overlooked contributors to carbon and nitrogen export to the deep ocean. ISME J. 17 (1), 47–58. doi: 10.1038/s41396-022-01319-3
Bonnet S., Guieu C., Taillandier V., Boulart C., Bouruet-Aubertot P., Gazeau F., et al. (2023b). Natural iron fertilization by shallow hydrothermal sources fuels diazotroph blooms in the ocean. Science 380 (6647), 812–817. doi: 10.1126/science.abq4654
Böttjer D., Dore J. E., Karl D. M., Letelier R. M., Mahaffey C., Wilson S. T., et al. (2017). Temporal variability of nitrogen fixation and particulate nitrogen export at Station ALOHA. Limnology Oceanography 62 (1), 200–216. doi: 10.1002/lno.10386
Bourbonnais A., Lehmann M. F., Waniek J. J., Schulz-Bull D. E. (2009). Nitrate isotope anomalies reflect N 2 fixation in the Azores Front region (subtropical NE Atlantic). J. Geophysical Res. 114 (C3), C03003. doi: 10.1029/2007JC004617
Bourbonnais A., Altabet M. A., Charoenpong C. N., Larkum J., Hu H., Bange H. W., et al. (2015). N-loss isotope effects in the Peru oxygen minimum zone studied using a mesoscale eddy as a natural tracer experiment: N-LOSS ISOTOPE EFFECTS IN AN EDDY. Global Biogeochemical Cycles 29 (6), 793–811. doi: 10.1002/2014GB005001
Braman R. S., Hendrix S. A. (1989). Nanogram nitrite and nitrate determination in environmental and biological materials by vanadium(III) reduction with chemiluminescence detection. Analytical Chem. 61 (24), 2715–2718. doi: 10.1021/ac00199a007
Brandes J. A., Devol A. H. (2002). A global marine-fixed nitrogen isotopic budget: Implications for Holocene nitrogen cycling: NITROGEN ISOTOPIC BUDGET. Global Biogeochemical Cycles 16 (4), 67-1-67–14. doi: 10.1029/2001GB001856
Buesseler K. O., Antia A. N., Chen M., Fowler S. W., Gardner W. D., Gustafsson O., et al. (2007). An assessment of the use of sediment traps for estimating upper ocean particle fluxes. J. Mar. Res. 65 (3), 345–416. doi: 10.1357/002224007781567621
Caffin M., Moutin T., Foster R.A., Bouruet-Aubertot P., Doglioli A.M., Berthelot H., et al. (2018). N2 fixation as a dominant new N source in the western tropical South Pacific Ocean (OUTPACE cruise). Biogeosciences 15 (8), 2565–2585. doi: 10.5194/bg-15-2565-2018
Capone D. G. (2001). Marine nitrogen fixation: what’s the fuss? Curr. Opin. Microbiol. 4 (3), 341–348. doi: 10.1016/S1369-5274(00)00215-0
Capone D. G., Zehr J. P., Paerl H. W., Bergman B., Carpenter E. J. (1997). Trichodesmium , a globally significant marine cyanobacterium. Science 276 (5316), 1221–1229. doi: 10.1126/science.276.5316.1221
Capone D. G., Burns J. A., Montoya J. P., Subramaniam A., Mahaffey C., Gunderson T., et al. (2005). Nitrogen fixation by Trichodesmium spp.: An important source of new nitrogen to the tropical and subtropical North Atlantic Ocean: NITROGEN FIXATION IN THE NORTH ATLANTIC. Global Biogeochemical Cycles 19 (2). doi: 10.1029/2004GB002331
Capone D. G., Ferrier M. D., Carpenter E. J. (1994). Amino acid cycling in colonies of the planktonic marine cyanobacterium trichodesmium thiebautii. Appl. Environ. Microbiol. 60 (11), 3989–3995. doi: 10.1128/aem.60.11.3989-3995.1994
Carpenter E. J., Harvey H. R., Fry B., Capone D. G. (1997). Biogeochemical tracers of the marine cyanobacterium Trichodesmium. Deep Sea Res. Part I: Oceanographic Res. Papers 44 (1), 27–38. doi: 10.1016/S0967-0637(96)00091-X
Casciotti K. L., Buchwald C., McIlvin M. (2013). Implications of nitrate and nitrite isotopic measurements for the mechanisms of nitrogen cycling in the Peru oxygen deficient zone. Deep Sea Res. Part I: Oceanographic Res. Papers 80, 78–93. doi: 10.1016/j.dsr.2013.05.017
Casciotti K. L., Sigman D. M., Böhlke J.K., Hilkert A. (2002). Measurement of the oxygen isotopic composition of nitrate in seawater and freshwater using the denitrifier method. Analytical Chem. 74 (19), 4905–4912. doi: 10.1021/ac020113w
Casciotti K. L., Trull T. W., Glover D. M., Davies D. (2008). Constraints on nitrogen cycling at the subtropical North Pacific Station ALOHA from isotopic measurements of nitrate and particulate nitrogen. Deep Sea Res. Part II: Topical Stud. Oceanography 55 (14–15), 1661–1672. doi: 10.1016/j.dsr2.2008.04.017
Confesor K. A., Selden C. R., Powell K. E., Donahue L. A., Mellett T., Caprara S., et al. (2022). Defining the realized niche of the two major clades of trichodesmium: A study on the west florida shelf. Front. Mar. Sci. 9, 821655. doi: 10.3389/fmars.2022.821655
Conway T. M., John S. G. (2014). Quantification of dissolved iron sources to the North Atlantic Ocean. Nature 511 (7508), 212–215. doi: 10.1038/nature13482
Dekaezemacker J., Bonnet S., Grosso O., Moutin T., Bressac M., Capone D. G. (2013). Evidence of active dinitrogen fixation in surface waters of the eastern tropical South Pacific during El Niño and La Niña events and evaluation of its potential nutrient controls: N 2 FIXATION IN THE ETSP. Global Biogeochemical Cycles 27 (3), 768–779. doi: 10.1002/gbc.20063
Deutsch C., Sigman D. M., Thunell R. C., Meckler A. N., Haug G. H. (2004). Isotopic constraints on glacial/interglacial changes in the oceanic nitrogen budget: GLACIAL/INTERGLACIAL NITROGEN BUDGET. Global Biogeochemical Cycles 18 (4). doi: 10.1029/2003GB002189
de Verneil A., Rousselet L., Doglioli A. M., Petrenko A. A., Moutin T. (2017). The fate of a southwest Pacific bloom: gauging the impact of submesoscale vs. mesoscale circulation on biological gradients in the subtropics. Biogeosciences 14 (14), 3471–3486. doi: 10.5194/bg-14-3471-2017
Dore J. E., Brum J. R., Tupas L. M., Karl D. M. (2002). Seasonal and interannual variability in sources of nitrogen supporting export in the oligotrophic subtropical North Pacific Ocean. Limnology Oceanography 47 (6), 1595–1607. doi: 10.4319/lo.2002.47.6.1595
Dugdale R. C., Goering J. J. (1967). Uptake of new and regenerated forms of nitrogen in primary productivity1: uptake of nitrogen in primary productivity. Limnology Oceanography 12 (2), 196–206. doi: 10.4319/lo.1967.12.2.0196
Dutkiewicz S., Ward B. A., Monteiro F., Follows M. J. (2012). Interconnection of nitrogen fixers and iron in the Pacific Ocean: Theory and numerical simulations: MARINE NITROGEN FIXERS AND IRON. Global Biogeochemical Cycles 26 (1). doi: 10.1029/2011GB004039
Glibert P. M., Bronk D. A. (1994). Release of dissolved organic nitrogen by marine diazotrophic cyanobacteria, trichodesmium spp.t. Appl. Environ. Microbiol. 60, 3996–4000. doi: 10.1128/aem.60.11.3996-4000.1994
Gruber N. (2004). “The dynamics of the marine nitrogen cycle and its influence on atmospheric CO2 variations,” in The ocean carbon cycle and climate. Eds. Follows M., Oguz T. (Dordrecht: Springer Netherlands), 97–148. doi: 10.1007/978-1-4020-2087-2_4
Guieu C., Bonnet S., Petrenko A., Menkes C., Chavagnac V., Desboeufs K., et al. (2018). Iron from a submarine source impacts the productive layer of the Western Tropical South Pacific (WTSP). Sci. Rep. 8 (1), 9075. doi: 10.1038/s41598-018-27407-z
Hoering T. C., Ford H. T. (1960). The isotope effect in the fixation of nitrogen by azotobacter. J. Am. Chem. Soc. 82 (2), 376–378. doi: 10.1021/ja01487a031
Jickells T. D., An Z. S., Andersen K. K., Baker A. R., Bergametti G., Brooks N., et al. (2005). Global iron connections between desert dust, ocean biogeochemistry, and climate. Science 308 (5718), 67–71. doi: 10.1126/science.1105959
Karl D., Letelier R., Tupas L., Dore J., Christian J., Hebel D. (1997). The role of nitrogen fixation in biogeochemical cycling in the subtropical North Pacific Ocean. Nature 388 (6642), 533–538. doi: 10.1038/41474
Knapp A. N., DiFiore P. J., Deutsch C., Sigman D. M., Lipschultz F. (2008). Nitrate isotopic composition between Bermuda and Puerto Rico: Implications for N 2 fixation in the Atlantic Ocean: SARGASSO SEA NITRATE ISOTOPES. Global Biogeochemical Cycles 22 (3). doi: 10.1029/2007GB003107
Knapp A. N., Casciotti K. L., Berelson W. M., Prokopenko M. G., Capone D. G. (2016a). Low rates of nitrogen fixation in eastern tropical South Pacific surface waters. Proc. Natl. Acad. Sci. 113 (16), 4398–4403. doi: 10.1073/pnas.1515641113
Knapp A. N., Fawcett S.E., Martínez-Garcia A., Leblond N., Moutin T., Bonnet S. (2016b). Nitrogen isotopic evidence for a shift from nitrate- to diazotroph-fueled export production in the VAHINE mesocosm experiments. Biogeosciences 13 (16), 4645–4657. doi: 10.5194/bg-13-4645-2016
Knapp A. N., McCabe K.M., Grosso O., Leblond N., Moutin T., Bonnet S. (2018). Distribution and rates of nitrogen fixation in the western tropical South Pacific Ocean constrained by nitrogen isotope budgets. Biogeosciences 15 (9), 2619–2628. doi: 10.5194/bg-15-2619-2018
Knapp A. N., Thomas R. K., Stukel M.R., Kelly T.B., Landry M.R., Selph K.E., et al. (2021). Constraining the sources of nitrogen fueling export production in the Gulf of Mexico using nitrogen isotope budgets. J. Plankton Res. 44 (5), 692–710. doi: 10.1093/plankt/fbab049
Knapp A. N., Forrer H. J. (2023). Water column nitrate plus nitrite d15N measurements from seawater collected in November 2019 and November 2020 in the Western Tropical South Pacific. Biol. Chem. Oceanography Data Manage. Office (BCO-DMO). doi: 10.26008/1912/bco-dmo.869963.2
Kruskal W. H., Wallis W. A. (1952). Use of ranks in one-criterion variance analysis. J. Am. Stat. Assoc. 47, 583–621. 40. doi: 10.1080/01621459.1952.10483441
Kustka A., Saudo-Wilhelmy S., Carpenter E. J., Capone D. G., Raven J. A. (2003). A revised estimate of the iron use efficiency of nitrogen fixation, with special reference to the marine cyanobacterium trichodesmium spp. (cyanophyta) 1. J. Phycology 39 (1), 12–25. doi: 10.1046/j.1529-8817.2003.01156.x
Landolfi A., Kähler P., Koeve W., Oschlies A. (2018). Global marine N2 fixation estimates: from observations to models. Front. Microbiol. 9, 2112. doi: 10.3389/fmicb.2018.02112
Lehmann N., Granger J., Kienast M., Brown K. S., Rafter P. A., Martínez-Méndez G., et al. (2018). Isotopic evidence for the evolution of subsurface nitrate in the western equatorial pacific. J. Geophysical Research: Oceans 123 (3), 1684–1707. doi: 10.1002/2017JC013527
Lory C., Guieu C., Rodier M., Planquette H., Gonzales Santana D., Sarthou G., et al. Environmental drivers of diazotrophs biogeography in the subtropical South Pacific Ocean. Front Mar Sci (2023). Available at: https://campagnes.flotteoceanographique.fr/campagnes/18000884/.
Luo Y.-W., Doney S. C., Anderson L. A., Benavides M., Berman-Frank I., Bode A., et al. (2012). Database of diazotrophs in global ocean: abundance, biomass and nitrogen fixation rates. Earth System Sci. Data 4 (1), 47–73. doi: 10.5194/essd-4-47-2012
Mahaffey C. (2005). The conundrum of marine N2 fixation. Am. J. Sci. 305 (6–8), 546–595. doi: 10.2475/ajs.305.6-8.546
Mahowald N. M., Baker A. R., Bergametti G., Brooks N., Duce R. A., Jickells T. D., et al. (2005). Atmospheric global dust cycle and iron inputs to the ocean: ATMOSPHERIC IRON DEPOSITION. Global Biogeochemical Cycles 19 (4). doi: 10.1029/2004GB002402
Marconi D., Sigman D. M., Casciotti K. L., Campbell E. C., Alexandra Weigand M., et al. (2017). Tropical dominance of N 2 fixation in the north atlantic ocean: tropical lead of atlantic N 2 fixation. Global Biogeochemical Cycles 31 (10), 1608–1623. doi: 10.1002/2016GB005613
Martin J. H., Knauer G. A., Karl D. M., Broenkow W. W. (1987). VERTEX: carbon cycling in the northeast Pacific. Deep Sea Res. Part A. Oceanographic Res. Papers 34 (2), 267–285. doi: 10.1016/0198-0149(87)90086-0
McIlvin M. R., Casciotti K. L. (2011). Technical updates to the bacterial method for nitrate isotopic analyses. Analytical Chem. 83 (5), 1850–1856. doi: 10.1021/ac1028984
Meiler S., Britten G. L., Dutkiewicz S., Gradoville M. R., Moisander P. H., Jahn O., et al. (2022). Constraining uncertainties of diazotroph biogeography from nifH gene abundance. Limnology Oceanography 67 (4), 816–829. doi: 10.1002/lno.12036
Minagawa M., Wada E. (1986). Nitrogen isotope ratios of red tide organisms in the East China Sea: A characterization of biological nitrogen fixation. Marine Chem. 19 (3), 245–259. doi: 10.1016/0304-4203(86)90026-5
Moisander P. H., Beinart R.A., Hewson I., White A. E., Johnson K. S., Carlson C. A., et al. (2010). Unicellular cyanobacterial distributions broaden the oceanic N 2 fixation domain. Science 327 (5972), 1512–1514. doi: 10.1126/science.1185468
Moisander P. H., Serros T., Paerl R. W., Beinart R. A., Zehr J. P. (2014). Gammaproteobacterial diazotrophs and nifH gene expression in surface waters of the South Pacific Ocean. ISME J. 8 (10), 1962–1973. doi: 10.1038/ismej.2014.49
Monteiro F. M., Dutkiewicz S., Follows M. J. (2011). Biogeographical controls on the marine nitrogen fixers: CONTROLS ON MARINE NITROGEN FIXERS. Global Biogeochemical Cycles 25 (2). doi: 10.1029/2010GB003902
Montoya J. P., Holl C. M., Zehr J. P., Hansen A., Villareal T. A., Capone D. G. (2004). High rates of N2 fixation by unicellular diazotrophs in the oligotrophic Pacific Ocean. Nature 430 (7003), 1027–1031. doi: 10.1038/nature02824
Moore Mills M. M., Achterberg E. P., Geider R. J., LaRoche J., Lucas M. I., McDonagh E. L., et al. (2009). Large-scale distribution of Atlantic nitrogen fixation controlled by iron availability. Nat. Geosci. 2 (12), 867–871. doi: 10.1038/ngeo667
Moutin T., Wagener T., Caffin M., Fumenia A., Gimenez A., Baklouti M., et al. (2018). Nutrient availability and the ultimate control of the biological carbon pump in the western tropical South Pacific Ocean. Biogeosciences 15, 2961–2989. doi: 10.5194/bg-2017-565
Mulholland B., Capone D. (2004). Dinitrogen fixation and release of ammonium and dissolved organic nitrogen by Trichodesmium IMS101. Aquat. Microbial Ecol. 37, 85–94. doi: 10.3354/ame037085
Mulholland M. R., Bernhardt P. W., Widner B. N., Selden C. R., Chappell P. D., Clayton S., et al. (2019). High rates of N 2 fixation in temperate, western north atlantic coastal waters expand the realm of marine diazotrophy. Global Biogeochemical Cycles 33 (7), 826–840. doi: 10.1029/2018GB006130
Peters B., Horak R., Devol A., Fuchsman C., Forbes M., Mordy C. W., et al. (2018). Estimating fixed nitrogen loss and associated isotope effects using concentration and isotope measurements of NO3–, NO2–, and N2 from the Eastern Tropical South Pacific oxygen deficient zone. Deep Sea Res. Part II: Topical Stud. Oceanography 156, 121–136. doi: 10.1016/j.dsr2.2018.02.011
Raes E. J., van de Kamp J., Bodrossy L., Fong A. A., Riekenberg J., Holmes B. H., et al. (2020). N2 fixation and new insights into nitrification from the ice-edge to the equator in the south pacific ocean. Front. Mar. Sci. 7, 389. doi: 10.3389/fmars.2020.00389
Shao Z., Xu Y., Wang H., Luo W., Wang L., Huang Y., et al. (2023). Global oceanic diazotroph database version 2 and elevated estimate of global oceanic N2 fixation. Earth System Science Data 15 (8), 3673–3709. doi: 10.5194/essd-15-3673-2023
Siegel D. A., Deuser W. G. (1997). Trajectories of sinking particles in the Sargasso Sea: modeling of statistical funnels above deep-ocean sediment traps. Deep Sea Res. Part I: Oceanographic Res. Papers 44 (9–10), 1519–1541. doi: 10.1016/S0967-0637(97)00028-9
Siegel D. A., Fields E., Buesseler K. O. (2008). A bottom-up view of the biological pump: Modeling source funnels above ocean sediment traps. Deep Sea Res. Part I: Oceanographic Res. Papers 55 (1), 108–127. doi: 10.1016/j.dsr.2007.10.006
Sigman D. M., Casciotti K. L., Andreani M., Barford C., Galanter M., Böhlke J. K. (2001). A bacterial method for the nitrogen isotopic analysis of nitrate in seawater and freshwater. Anal. Chem. 73 (17), 4145–4153. doi: 10.1021/ac010088e
Smith J. M., Chavez F. P., Francis C. A. (2014). Ammonium uptake by phytoplankton regulates nitrification in the sunlit ocean. PloS One 9 (9), e108173. doi: 10.1371/journal.pone.0108173
Sohm J. A., Webb E. A., Capone D. G. (2011). Emerging patterns of marine nitrogen fixation. Nat. Rev. Microbiol. 9 (7), 499–508. doi: 10.1038/nrmicro2594
Staal M., Meysman F. J. R., Stal L. J. (2003). Temperature excludes N2-fixing heterocystous cyanobacteria in the tropical oceans. Nature 425 (6957), 504–507. doi: 10.1038/nature01999
Stenegren M., Caputo A., Berg C., Bonnet S., Foster R. A. (2018). Distribution and drivers of symbiotic and free-living diazotrophic cyanobacteria in the western tropical South Pacific. Biogeosciences 15 (5), 1559–1578. doi: 10.5194/bg-15-1559-2018
Talley L. D., Pickard G. L., Emery W. J., Swift J. H. (2011). Descriptive physical oceanography: an introduction. 6th ed (Amsterdam ; Boston: Academic Press).
Tilliette C., Gazeau F., Chavagnac V., Leblond N., Montanes M., Leblanc K., et al. (2023). Temporal and spatial variability in the hydrothermal signature of sinking particles and sediments in the Western Tropical South Pacific Ocean. ESS Open Archive. doi: 10.22541/essoar.167898492.26733124/v1
Tilliette C., Taillandier V., BouruetAubertot P., Grima N., Maes C., Montanes M., et al. (2022). Dissolved iron patterns impacted by shallow hydrothermal sources along a transect through the Tonga-kermadec arc. Global Biogeochemical Cycles 36, e2022GB007363. doi: 10.1029/2022GB007363
Turk-Kubo K. A., Achilles K. M., Serros T. R. C., Ochiai M., Montoya J. P., Zehr J. P., et al. (2012). Nitrogenase (nifH) gene expression in diazotrophic cyanobacteria in the Tropical North Atlantic in response to nutrient amendments. Front. Microbiol. 3. doi: 10.3389/fmicb.2012.00386
Waniek J., Koeve W., Prien R. D. (2000). Trajectories of sinking particles and the catchment areas above sediment traps in the northeast Atlantic. J. Mar. Res. 58 (6), 983–1006. doi: 10.1357/002224000763485773
Webb D. J. (2000). Evidence for shallow zonal jets in the south equatorial current region of the southwest pacific. J. Phys. Oceanography 30, 15. doi: 10.1175/1520-0485(2000)030<0706:EFSZJI>2.0.CO;2
Weber T., Deutsch C. (2014). Local versus basin-scale limitation of marine nitrogen fixation. Proc. Natl. Acad. Sci. 111 (24), 8741–8746. doi: 10.1073/pnas.1317193111
Weigand M. A., Foriel J., Barnett B., Oleynik S., Sigman D. M. (2016). Updates to instrumentation and protocols for isotopic analysis of nitrate by the denitrifier method. Rapid Commun. Mass Spectrometry 30 (12), 1365–1383. doi: 10.1002/rcm.7570
White A. E., Foster R. A., Benitez-Nelson C. R., Masqué P., Verdeny E., Popp B. N., et al. (2013). Nitrogen fixation in the gulf of california and the eastern tropical north pacific. Prog. Oceanography 109, 1–17. doi: 10.1016/j.pocean.2012.09.002
White A. E., Granger J., Selden C., Gradoville M.R., Potts L., Bourbonnais A., et al. (2020). A critical review of the 15 N 2 tracer method to measure diazotrophic production in pelagic ecosystems. Limnology Oceanography: Methods 18 (4), 129–147. doi: 10.1002/lom3.10353
Xu H., Weber T. (2021). Ocean dust deposition rates constrained in a data-assimilation model of the marine aluminum cycle. Global Biogeochemical Cycles 35 (9). doi: 10.1029/2021GB007049
Zehr J. P., Mellon M. T., Zani S. (1998). New nitrogen-fixing microorganisms detected in oligotrophic oceans by amplification of nitrogenase (nifH) genes. Appl. Environ. Microbiol. 64 (9), 3444–3450. doi: 10.1128/AEM.64.9.3444-3450.1998
Zehr J. P., Turner (2001) Nitrogen fixation: Nitrogenase genes and gene expression - ScienceDirect. Available at: https://www.sciencedirect.com/science/article/abs/pii/S0580951701300491?via%3Dihub (Accessed 14 February 2023).
Keywords: N2 fixation, nitrate d15N, Tonga Arc, South Pacific, hydrothermal vents, d15N budget
Citation: Forrer HJ, Bonnet S, Thomas RK, Grosso O, Guieu C and Knapp AN (2023) Quantifying N2 fixation and its contribution to export production near the Tonga-Kermadec Arc using nitrogen isotope budgets. Front. Mar. Sci. 10:1249115. doi: 10.3389/fmars.2023.1249115
Received: 28 June 2023; Accepted: 12 October 2023;
Published: 27 October 2023.
Edited by:
Margarita Fernández Tejedor, Institute of Agrifood Research and Technology (IRTA), SpainReviewed by:
Dengzhou Gao, East China Normal University, ChinaAnnie Bourbonnais, University of South Carolina, United States
Copyright © 2023 Forrer, Bonnet, Thomas, Grosso, Guieu and Knapp. This is an open-access article distributed under the terms of the Creative Commons Attribution License (CC BY). The use, distribution or reproduction in other forums is permitted, provided the original author(s) and the copyright owner(s) are credited and that the original publication in this journal is cited, in accordance with accepted academic practice. No use, distribution or reproduction is permitted which does not comply with these terms.
*Correspondence: Heather J. Forrer, aGZvcnJlckBmc3UuZWR1