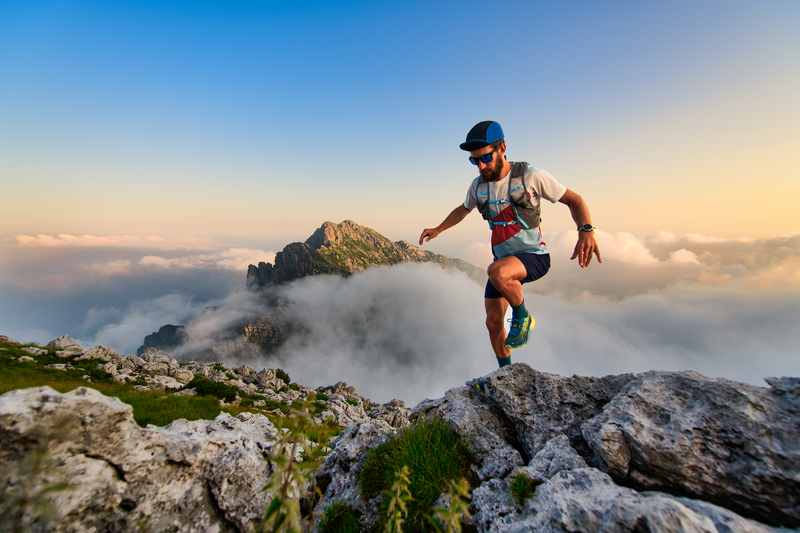
95% of researchers rate our articles as excellent or good
Learn more about the work of our research integrity team to safeguard the quality of each article we publish.
Find out more
ORIGINAL RESEARCH article
Front. Mar. Sci. , 28 August 2023
Sec. Marine Biogeochemistry
Volume 10 - 2023 | https://doi.org/10.3389/fmars.2023.1243780
This article is part of the Research Topic Broadband Seafloor Sediment Acoustic Property and Multi-Parameter Geoacoustic Model View all 11 articles
The acoustic and physical properties of two valuable marine cold spring carbonate rock samples gathered from the Chaoshan Depression in the South China Sea were measured. The Wyllie time-average equation was applied to analyze the measured sound speeds and their trend under different porosities, and the sound speeds of marine cold spring carbonate rocks were found to be consistent with those of terrestrial carbonate rocks. The Voigt model, Reuss model, and Voigt-Reuss-Hill model were used to predict the characteristics of the sound speed for four states of seafloor sediments containing cold spring carbonate mineral particles or rocks. For these four states of marine cold spring carbonate mineral particles existing on or in seafloor sediments, the sound speed and reflection coefficient of a mixture of seafloor surface sediments containing cold spring carbonate mineral particles or rocks decrease with an increase in the volume ratio of the seafloor sediment. This method for predicting the reflection coefficient provides evidence to explain the high and low reflection coefficients observed in Chirp sub-bottom profiles of cold spring seepage areas.
Since 12 Ma BP, the Earth’s climate has been marked by frequent cold spring activities (Callender and Powell, 1999). Cold springs characterized by a sufficient gas source and continuous overflow lead to the formation of chemoautotrophic communities, which are recorded in carbonate sediments. Therefore, the deposition of carbonate rocks indicates the existence of natural gas hydrates and the occurrence and persistence of natural gas seepage (Chen et al., 2007; Wu et al., 2015; Wang et al., 2019; Shan et al., 2020). Cold spring carbonate rocks are usually deposited on the seafloor surface with angular, spherical, and ellipsoidal shapes and massive, lamellar, tubular and columnar habits. These rocks generally reach sizes of 3 to 8 cm but can sometimes be as large as 22 cm (Chen et al., 2008; Tong et al., 2012; Xue and Huang, 2016; Xi et al., 2017), and some grow even larger in the form of different structures that accumulate on the seafloor (Wang et al., 2019; Shan et al., 2020).
Cold spring carbonate rocks have been mainly studied by biogeochemical methods with a focus on their lithological characteristics (Chen et al., 2005; Chen et al., 2007; Chen et al., 2008; Han et al., 2008; Wu et al., 2009; Xue and Huang, 2016; Liu et al., 2017; Lu et al., 2020; Zhao et al., 2020). These rocks are directly related to the existence of seepage-associated natural gas hydrates and cold springs. For example, carbonate rocks are distributed across the seafloor of the Shenhu Sea area and Dongsha Island in the northern South China Sea, and drilling yielded gas hydrate samples at a depth of 183 m in the Shenhu Sea area (Zhang et al., 2007). Yan et al. (2011) found evidence of seepage-associated hydrates based on sub-bottom geophysical surveys across a suspected mud diapir area of the Baiyun Depression in the northern South China Sea. Based on the analysis of these sub-bottom profiles, the reflections from most areas of the seafloor are weak, but there are obvious abnormal reflection characteristics in some areas with strong reflection intensities (Yan et al., 2014; Liu et al., 2015; Chen et al., 2016; Shan et al., 2020). The samples obtained in this area were found to contain carbonate rock fragments, which indicates that these strong reflections may originate from cold spring carbonate rocks. The reflection coefficients also indicate the different states under which these cold spring carbonate rocks exist. Unlike sand, silt, clay, and mixed-type seafloor surface sediments, cold spring carbonate rocks form clumps because of their crystalline and compacted structure, and their acoustic characteristics typically show high sound speeds and low attenuation. However, due to their special natural shapes and varying structures, it is difficult to obtain proper samples of marine cold spring carbonate rocks to carry out acoustic measurements. As a result, few direct measurements of the acoustic characteristics of marine cold spring carbonate rocks have been reported.
In this article, based on two valuable marine cold spring carbonate rock samples gathered from the Chaoshan Depression, we measured some of the physical properties and sound speeds of the two samples, compared them with the properties of terrestrial carbonate rocks, analyzed the relationship between the sound speed and porosity of cold spring carbonate rocks using the Wyllie time-average equation, and predicted the acoustic characteristics of seafloor surface sediments containing cold spring carbonate rocks. Detecting and predicting the acoustic characteristics of marine cold spring carbonate rocks both on the seafloor and in seafloor surface sediments can provide evidence to explain the strong reflection characteristics of cold spring carbonate rocks and help develop an effective method for the exploration of seepage-associated natural gas hydrates.
The samples of marine cold spring carbonate rocks were gathered from the Chaoshan Depression (Figure 1), the largest Mesozoic residual basin in the northern South China Sea with a sedimentary thickness exceeding 6000 m. Our samples were extracted from the seafloor surface sediments at a seawater depth of approximately 470 m. Their main component was determined to be ferruginous dolomite (Chen et al., 2006; Yan et al., 2014). Based on a Chirp sub-bottom profiling survey near Dongsha Island in the northern South China Sea, Chen et al. (2016) found reflection coefficients of the seabed ranging from 0.1 to 0.8.
Figure 1 Samples of cold spring carbonate rocks from the northern South China Sea. The red dot is the sample of TSYC1, and the bule dot is the sample TSYC2.
The two samples used in this article are named TSYC1 and TSYC2. Both samples (Figure 1) were cut into two parts. One part of each sample was used to measure the physical and acoustic properties reported in this study. These parts were split at both ends, and the two edges of the main part were cut flat for acoustic measurements, and the cut segments were used to detect the physical properties. The samples were very dense overall, with an approximately 3 mm thick brown coating on the surface. The sample interior was gray and displayed an uneven texture with a small amount of unconsolidated soil. Miniscule individual pores were identified on the cutting surface of each sample.
The samples were preserved and saturated in seawater. The cut segments were used to measure the wet weight and volume by using the volume product method. Then, the cut segments were dried in an oven at 108°C for 24 hours to measure the dry weight by using the weight removal method. An electronic balance with an accuracy of 0.01 g was used to record the weight, and a high-precision measuring cylinder with an accuracy of ±0.1 ml was used for bulk measurements. Then, the wet density and porosity were calculated 2.239 g/cm3 and 3.904%, respectively, for TSYC1 and 2.748 g/cm3 and 10.027%, respectively, for TSYC2.
The lengths of the main segments employed for the acoustic measurements were 100.92 mm and 139.89 mm for TSYC1 and TSYC2, respectively. The acoustic measurement principle is based on the time-of-fight method, as shown in Figure 2. A DB4 ultrasonic apparatus was used to excite electrical signals, drive the acoustic emitter, amplify the signal of the acoustic receiver, and store the sound pressure signals. The sampling frequency of the acoustic instrument was 5 MHz. A Lenovo laptop with a good human-computer interface was employed as the control and analysis system, and the data were analyzed in MATLAB 2009. The length of each sample was measured by a Vernier caliper with an accuracy of 0.02 mm. Transducers with dominant frequencies of 20 kHz, 40 kHz and 100 kHz were applied to study the characteristics of the longitudinal wave velocity (hereinafter referred to as the sound speed) under both seawater- and air-saturated conditions. The total absolute acoustic measurement precision was less than 1.2%.
Figure 2 Schematic diagram of acoustic measurements in the laboratory for the samples of marine cold spring carbonate rocks.
The measured sound speeds of the marine cold spring carbonate rocks (shown in Table 1) are 166 m/s on average higher in the seawater-saturated rocks than in the air-saturated rocks. In addition, the sound speeds of the marine cold spring carbonate rocks exhibit dispersion characteristics, with the sound speed of the seawater-saturated samples being 261 m/s on average higher at 100 kHz than at 20 kHz and 162 m/s on average higher than at 40 kHz. Because the acoustic properties of marine carbonate rocks have not been reported, we compare the above measured data with those of terrestrial carbonate rocks. Liu (1985) pointed out that the sound speeds of terrestrial liquid-saturated carbonates range from 3,200 to 7,000 m/s due to differences in the sample area and formation and are higher than those of air-saturated carbonates by 300 to 2,000 m/s. The sound speeds of dolomite (a carbonate rock) from Sichuan and other regions of China range from 4,991.41 to 7,019.84 m/s (Hang et al., 2004). Moreover, carbonate rocks are characterized by obvious anisotropy, and thus, the sound speed varies in different directions from 4,590 to 7,230 m/s (Chen et al., 2017). Both water- and air-saturated rocks usually display dispersion characteristics (Wei et al., 2015; Guo et al., 2018; Ma et al., 2019), and carbonate rocks differ in terms of their lithology, diagenetic history, structure and degree of saturation. Therefore, the sound speeds of the marine cold spring carbonate rocks are in the range of those of terrestrial carbonates.
The sound speed-porosity relationships of the two air-saturated cold spring carbonate rock samples at different frequencies with those of terrestrial limestone and dolomite measured by Rafavich et al. (1984) and Sayers (2008), respectively. The sound speeds of TSYC1 and TSYC2 are consistent with that of the terrestrial carbonate rocks, while the sound speed of TSYC1 is slightly lower. The sound speeds of the marine cold spring carbonate rocks seem to be similar to those of terrestrial carbonate rocks. Baechle et al. (2008) pointed out that microporosity (proportion of micropores to total pores) scatters the sound speed of terrestrial carbonate rocks. As shown in Figure 3, the higher the overall porosity is, the lower the sound speed; likewise, the higher the microporosity is, the lower the sound speed. These properties suggest that the microporosity of TSYC1 may be higher than that of TSYC2. In fact, some small unevenly distributed microcracks were found on the cutting surfaces of the two samples, and some clay holes with a low degree of consolidation were also identified. In another respect, the anisotropic characteristics in three different directions of terrestrial water-saturated carbonate rocks changed in the anisotropy coefficient of the wave velocity ranging from 1.671% to 24.699%(Chen et al., 2017). These two cold spring carbonate rock samples also appeared slight anisotropy in the structure.
Figure 3 Comparison of the sound speeds between water-saturated cold spring carbonate rocks and terrestrial carbonate rocks. The dots are the porosity and these different colors of the dots represent different percentages of micropores (Baechle et al., 2008).
According to the Wyllie time-average equation (Wyllie et al., 1956; Ma et al., 2010), the acoustic interval transit time of a rock can be expressed as follows:
where is the interval transit time; is the porosity; and the subscripts ma and f denote the rock skeleton and pore fluid, respectively.
When analyzing the sound speed of marine cold spring carbonate rocks, Eq. (1) can be rewritten as follows:
The sound speed of the interior of the frame is expressed as
where , are the bulk modulus, shear modulus, and density of the frame, respectively.
Moreover, the density of marine cold spring carbonate rocks can be written as
The marine cold spring carbonate rocks near Dongsha Island contain ferriferous dolomite, siderite, and small amounts of aragonite and calcite (Chen et al., 2008). However, due to the lack of details on the mineral composition, for the analyses of the acoustic and other physical properties, the frame is presumed to be made entirely of either dolomite or calcite. The elastic properties of the minerals (Sayers, 2008) and seawater are shown in Table 2.
Table 2 Elastic moduli and density of minerals (Sayers, 2008) and seawater.
The Wyllie time-average equation is used to calculate the relationship between the sound speed and the porosity of the cold spring carbonate rocks. As shown in Figure 4, the sound speeds of the cold spring carbonate rocks decrease with increasing porosity. Porosity is the main factor affecting the acoustic characteristics of carbonate rocks (Li et al., 2002). The measured properties of the marine cold spring carbonate rocks in the northern South China Sea, that is, the measured sound speeds of samples TSYC1 and TSYC2, are close to the theoretically calculated values.
Figure 4 Comparison between the theoretically calculated and actually measured properties of the cold spring carbonate rock samples.
According to the existing states of natural deposits of sediment and authigenic carbonate minerals (Chen et al., 2007; Wang et al., 2019), the mixed states of marine cold spring carbonate rocks and seafloor sediments can be simplified into four states: a) seafloor sediments without cold spring carbonate minerals (named SA); b) a scattered distribution of small cold spring carbonate minerals in seafloor sediments (named SB); c) an accumulation of cold spring carbonate minerals in seafloor sediments (named SC); and d) consolidated marine cold spring carbonate rocks isolated from seafloor sediments (named SD). For simplification, spherical particles are used to represent the most general from of the cold spring carbonate rocks and the four states are illustrated in Figure 5.
SA is the basic state of seafloor sediment without marine cold spring carbonate rocks (Chen et al., 2016), while SD is the diagenetic state of large marine cold spring carbonate rocks (Wang et al., 2019). SB and SC signify the growth and accumulation states of authigenic carbonate minerals, respectively, in seafloor sediments (Wang et al., 2019). For SB and SC, carbonate minerals may grow into small- or medium-sized carbonate rocks. When samples TSYC1 and TSYC2 were separately measured in the laboratory, they were in the state of SD; when they were distributed on the seafloor, they were in the state of either SB or SC; and when they grew into a large carbonate rock on the seafloor, they were in the state of SD.
The seafloor sediment sample collected from the marine cold spring carbonate rock area is a clayey silt. The sound speed measured at 40 kHz is 1,578 m/s, and its main physical parameters are a porosity of 41.46%, a wet density of 1.88 g/cm3, an average grain size of 7.685 and a silt content of 60.76%. The parameters of TSYC2 are selected to represent the marine cold spring carbonate rocks in this area with a sound speed of 5,813 m/s at 40 kHz, a porosity of 10.027%, and a density of 2.748 g/cm3. Because the measured temperature of the marine cold spring carbonate rocks and sediments was 24°C, this temperature is used for the pore water in the following calculations.
For SA, the sound speed of the clayey silt without marine cold spring carbonate rocks was measured to be 1,578 m/s. In contrast, SD represents marine cold spring carbonate rocks. Due to differences in their diagenetic history, these rocks may have different degrees of cementation and thus different porosities and fracture networks. The measured porosity of a natural terrestrial carbonate rock does not exceed 55% (Baechle et al., 2008), so the porosity of the marine cold spring carbonate rocks is also set to be no greater than 55%. Eqs. (2) and (3) are used to predict the sound speed. As seen in Figure 4, the sound speed of the marine cold spring carbonate rock decreases as its porosity increases. When the porosity (pure dolomite) increases from 0 to 55%, its sound speed is predicted to decrease from 7,051 m/s to 2,367 m/s. The sound speeds of both TSYC1 and TSYC2 are within this range.
The mixture of seafloor sediments with marine cold spring carbonate rocks can be considered equivalent to a stratified model of two components according to the Wyllie time-average equation. Because seafloor sediments are much softer than cold spring carbonate rocks, the former component is assumed to be the fluid filling the pore space between cold spring carbonate minerals or rocks. When the volume ratio of seafloor sediments in the entire mixture varies, the mixture exhibits different acoustic and other physical properties.
For SB and SC, the mixing form of marine cold spring carbonate mineral particles (or rocks) in the seafloor sediments is more complex because of the different porosities, shapes, sizes and ratios of the cold spring carbonate mineral particles (or rocks) in the seafloor sediment. Hence, for the following calculations, the equivalent elastic modulus is adopted as a necessary parameter. To predict the equivalent elastic modulus of a mixture of mineral particles and sediments, it is usually necessary to know the volume content, elastic moduli, and spatial and geometric distributions of each component. However, marine cold spring carbonate rocks have rarely been studied, and thus, their sizes and spatial and geometric distributions are not fully understood. Hill (1952) developed a method to average the upper and lower limits calculated by the Voigt and Reuss models to obtain the Voigt-Reuss-Hill model, which can be used to calculate the elastic properties of mixtures such as SB and SC. The equivalent elastic modulus EHof sediments containing cold spring carbonate mineral particles (or rocks) can be written as
where and are the equivalent elastic moduli calculated by the Voigt and Reuss models, respectively; is the volume ratio of seafloor sediments in the entire mixture; and and are the equivalent elastic moduli of seafloor sediments and cold spring carbonate mineral particles (or rocks), respectively.
The equivalent density seafloor sediments containing cold spring carbonate rocks is calculated as follows:
where and are the densities of cold spring carbonate rocks and seafloor sediments, respectively.
Then, the sound speed of the seafloor sediments containing cold spring carbonate mineral particles (or rocks) can be calculated as
The sound speeds of seafloor sediments containing cold spring carbonates calculated with the Voigt, Reuss, and Voigt-Reuss-Hill models (Eqs. (5–7)) are shown in Figure 6. When seafloor sediments do not contain cold spring carbonate mineral particles (or rocks), that is, when the volume ratio of seafloor sediment is 100% (SA), the sound speed depends entirely on the acoustic properties of the seafloor sediment. In this case, the sediment is equivalent of clayey silt. When the volume ratio of seafloor sediment is zero (SD), the sound speed depends entirely on the acoustic properties of the marine cold spring carbonate rocks. In this case, the rock is equivalent to sample TSYC2. When the volume ratio is small or moderate, authigenic cold spring carbonate mineral particles accumulate in the seafloor sediments, which fill in the pore space of the cold spring carbonate mineral skeleton (SC). As the consolidation of authigenic mineral particles continues, the mineral particles contact each other and grow into small- and large-sized cold spring carbonate rocks (with larger rocks yielding SD), although some small particles may remain separated by sediments (such as in SB). When the volume ratio is large, marine cold spring carbonate mineral particles are distributed and wrapped mainly in seafloor sediments (SB). Based on the Voigt and Reuss models, the upper and lower limits of the possible sound speed of seafloor sediments containing cold spring carbonate rocks are predicted. Based on the Voigt-Reuss-Hill model, the intermediate sound speed characteristics of seafloor sediments containing cold spring carbonate rocks are also predicted. However, when considering an uneven pore distribution, complex cold spring carbonate rock states, shapes and structures, and different seafloor sediment types, the sound speed distribution becomes highly complicated and should be studied in greater detail and depth in future research.
Figure 6 Relationship between the sound speed and volume percentage of seafloor sediments containing cold spring carbonate rocks.
When both the incident angle and the reflection angle are 0° (representing vertical incidence), the reflection coefficient can be written as Eq.(8), which is seafloor Rayleigh reflection coefficient.
where and the subscript w denotes bottom seawater.
For SA, the reflection coefficient of clayey silt without cold spring carbonate rocks is calculated as 0.308. In contrast, SD represents the encrustation of cold spring carbonate rocks. According to the analysis of the sound speed, the porosity of the cold spring carbonate rock encrustation is no more than 55%. As shown in Figure 7, upon calculating the sound speed using Eqs. (2) and (3), the density using Eq. (4), and the reflection coefficient using Eq. (8), the predicted reflection coefficient of the cold spring carbonate rock (with dolomite as the constituent mineral) decreases from 0.856 to 0.473 as its porosity increases from 0 to 55%. The reflection coefficient of TSYC2 is 0.821, which is within this range.
Figure 7 Reflection characteristics of a cold spring carbonate rock encrustation based on the Wyllie time-average equation.
Based on the Voigt, Reuss, and Voigt-Reuss-Hill models, the reflection coefficients of seafloor sediments containing marine cold spring carbonate mineral particles (or rocks) are analyzed. As shown in Figure 8A, when the volume ratio is close to 0, the sediment contains only cold spring carbonate rocks (TSYC2), and the reflection coefficient reaches as high as 0.821. When the volume ratio is close to 1, the sediment contains only clayey silt, and the reflection coefficient is only 0.308. When the volume ratio is between 0 and 1, namely, when cold spring carbonate mineral particles (or rocks) and seafloor sediments are mixed together, the reflection coefficient varies from 0.308 to 0.821. Considering that the abovementioned porosity of natural carbonate rocks cannot exceed 55%, the reflection coefficient of seawater-saturated cold spring carbonate rocks (composed mainly of dolomite) must be greater than 0.473, as illustrated in Figure 7. On the other hand, the reflection coefficient of seafloor surface sediments on continental slopes without cold spring carbonate mineral particles is usually no greater than the reflection coefficient of coarse-grained sand (0.410) (Liu et al., 2015). Consequently, seafloor sediments with a reflection coefficient between 0.410 and 0.473 in cold spring seepage areas are likely to contain cold spring carbonate mineral particles (or rocks) in an accumulated or suspended state.
Figure 8 Reflection characteristics and porosities of the seafloor sediments containing cold spring carbonate rocks. The (A) is the relationship between the reflection coefficient and porosity, the (B, C) is the inverted reflection coefficient and porosity from the Chirp sub-bottom data, respectively.
Based on a sub-bottom profiling survey near the Baiyun Depression in the northern South China Sea, the reflection coefficient inverted from Chirp sub-bottom data ranges from 0.2 to 0.8 as shown in Figure 8B (Chen et al., 2016). And the porosity inversion is illustrated in Figure 8C. The inversion results predicted the presence of obvious high-velocity, high-density, and low-porosity seafloor sediments inside the Mud Volcano Zone and low-velocity, low-density, and high-porosity seafloor sediments outside the Mud Volcano Zone. It can be explained by the mixture of cold spring carbonate rock and seafloor sediment as the kind of SA and SD shown in Figure 7 and the kind of SB and SC shown in Figure 8A. Clayey silt containing carbonate rock fragments and cold spring carbonate rock encrustations was collected at several nearby sampling points, so cold spring carbonate mineral particles must be mixed into the seafloor sediments (as in SB) when the reflection coefficient is between 0.41 and 0.473. When the reflection coefficient ranges from 0.473 to 0.821, the cold spring carbonate mineral particles are very likely to grow into cold spring carbonate rocks, which may form a mixture (SB), an accumulation (SC) or an encrustation (SD).
An increasing number of cold spring carbonate mineral particles and rocks have been found in different states throughout the northern South China Sea. These cold spring carbonate rocks can be regarded as a special type of sediment distributed on or in seafloor surface sediments.
When cold spring carbonate mineral particles exist in seafloor sediments, the sound speed of the mixture is less than that of cold spring carbonate encrustations and cold spring carbonate rocks but greater than that of the clayey silt sediment. The same is true for the Rayleigh reflection coefficient of the mixture. Upon analyzing the measurement and calculation results with the Wyllie time-average equation and Voigt-Reuss-Hill model, the sound speeds and Rayleigh reflection coefficients of the seafloor sediments containing cold spring carbonate rocks are predicted. Some meaningful conclusions are as follows:
1) The relationship between the sound speed and porosity of marine cold spring carbonate rocks is consistent with that of terrestrial carbonate rocks. Taking sample TSYC2 as a reference, the measured sound speed of the seawater-saturated marine cold spring carbonate rock sample is 5,813 m/s, and the Rayleigh reflection coefficient is 0.821 at 40 kHz.
2) The sound speeds in marine cold spring carbonate rocks are dispersive, with the sound speed at 100 kHz being 261 m/s on average higher than that at 20 kHz, and the sound speed of seawater-saturated cold spring carbonate rocks is 166 m/s on average higher than that of air-saturated cold spring carbonate rocks.
3) For marine cold spring carbonate rocks, the sound speed decreases as the porosity increases. When the porosity (with dolomite as the constituent mineral) of seawater-saturated marine cold spring carbonate rocks increases from 0 to 55%, its sound speed is predicted to decrease from 7,051 m/s to 2,367 m/s.
4) For the four states of marine cold spring carbonate mineral particles existing on or in seafloor sediments, the sound speed and the Rayleigh reflection coefficient of a mixture of seafloor surface sediments containing cold spring carbonate mineral particles or rocks decrease with an increase in the volume ratio of the seafloor sediment. The reflection coefficient is predicted to vary from 0.308 (clayey silt) to 0.821 (marine cold spring carbonate rocks).
The datasets presented in this study can be found in online repositories. The names of the repository/repositories and accession number(s) can be found in the article/supplementary material.
YT: Writing – original draft. DZ: Writing – review & editing. ZC: Review & editing. CF: Review & editing. PY: Review & editing. YJ: Review & editing. LW: Review & editing. All authors contributed to the article and approved the submitted version.
We are also thankful for the financial support of the National Natural Science Foundation of China (42227803, 41976065, 91855101 and 42006071), Special Support Program for Cultivating High-level Talents in Guangdong Province (2019BT02H594), and 2020 Research Program of Sanya Yazhou Bay Science and Technology City (SKJC-2020-01-012), National Key R&D Program of China (2021YFC3100600).
We wish to thank the other members of the Guangdong University of Technology and the South China Sea Institute of Oceanology, Chinese Academy of Sciences, for carrying out the measurements and experiments, analyzing the datasets, and discussing this research.
The authors declare that the research was conducted in the absence of any commercial or financial relationships that could be construed as a potential conflict of interest.
All claims expressed in this article are solely those of the authors and do not necessarily represent those of their affiliated organizations, or those of the publisher, the editors and the reviewers. Any product that may be evaluated in this article, or claim that may be made by its manufacturer, is not guaranteed or endorsed by the publisher.
Baechle G. T., Colpaert A., Eberli G. P., Weger R. J. (2008). Effects of microporosity on sonic velocity in carbonate rocks. Leading Edge. 27, 1012–1018. doi: 10.1190/1.2967554
Callender W. R., Powell E. N. (1999). Why did ancient chemosynthetic seep and vent assemblages occur in shallower water than they do today? Int. J. Earth Sci. 88, 377–391. doi: 10.1007/s005310100196
Chen D. F., Huang Y. Y., Yuan X. L., Cathles III, L. M. (2005). Seep carbonates and preserved methane oxidizing archaea and sulfate reducing bacteria fossils suggest recent gas venting on the seafloor in the Northeastern South China Sea. Mar. petroleum Geology. 22, 613–621. doi: 10.1016/j.marpetgeo.2005.05.002
Chen C., Lou Z. H., Jin A. M. (2017). Acoustic anisotropy of water-saturated and desiccated carbonate rocks. Lithologic Reservoirs. 29, 131–137. doi: 10.3969/j.issn.1673-8926.2017.04.016
Chen Z., Yan W., Chen M. H., Wang S. H. (2006). Discovery of seep authigenic carbonate nodules on northern continental slope of South China Sea: new evidence of gas hydrate. J. Trop. Oceanography. 25, 83–83.
Chen S., Yan P., Wang Y. L. (2016). Inversion of the physical properties of the seabed using Chirp sub-bottom data in Mud Volcanoes field of the Southwest of Dongsha Islands. Earth Science. 41, 425–432. doi: 10.3799/dpkx.2016.034in Chinese with English abstract
Chen Z., Yang H. P., Huang Q. Y., Lu J., Yan W. (2007). Characteristics of cold seeps and structures of chemoauto-synthesis-based communities in seep sediments. J. Trop. Oceanography. 26, 73–82.
Chen Z., Yang H. P., Huang Q. Y., Yan W., Lu J. (2008). Diagenetic environment and implication of seep carbonate precipitations from the southwestern Dongsha area South China Sea. Geoscience. 22, 382–389.
Guo M. Q., Ba. J., Ma. N. P., Chen T. S., Zhang L., Pang M. Q., et al. (2018). P-wave velocity dispersion and attenuation in fluid-saturated tight sandstones: Characteristics analysis based on a double double-porosity structure model description. Chin. J. Geophysics. 61, 1053–1068. doi: 10.6038/cjg2018L0678
Han X., Suess E., Huang Y., Wu N., Bohrmann G., Su X., et al. (2008). Jiulong methane reef: microbial mediation of seep carbonates in the South China Sea. Mar. Geology. 249, 243–256. doi: 10.1016/j.margeo.2007.11.012
Hang L. J., Li Z. K., Yan J., Sun J. J., Geng Y. C., Shi H. J., et al. (2004). Test and application of sonic properties of carbonate rock. Chin. J. Rock Mechanics Eng. 14, 2444–2447.
Hill R. (1952). The elastic behaviour of a crystalline aggregate. Proc. Phys. Soc. Section A. 65, 349–355. doi: 10.1088/0370-1298/65/5/307
Li Y. H., Chu Z. H., Wang H. (2002). On the experimental relation between clay-bearing carbonate rock acoustical and lithological characters. Well Logging Technology. 26, 269–227. doi: 10.16489/j.issn.1004-1338.2002.04.002
Liu D. H. (1985). Testing of carbonate reservoir and its acoustic characteristics. Oil Explor. Ser. 06, 49–56.
Liu B. R., Song H. B., Guan Y. X., Bai Y., Chen J. X., Geng M. H., et al. (2015). Characteristics and formation mechanism of cold seep system in the northeastern continental slope of South China Sea from sub-bottom profiler data. Chin. J. Geophysics. 58, 247–256. doi: 10.6038/cjg20150122 (in Chinese with English abstract
Liu X. J., Tang D. H., Yan P., Ge C. D., Wang Y. L. (2017). Characteristics of authigenic carbonates from a mega-pockmark on the eastern side of Baiyun Sag, South China Sea and their geological significance. Mar. Geo. Quaternary Geo. 37, 119–127. doi: 10.16562/j.cnki.0256-1492.2017.06.013
Lu Y., Chu F. Y., Dong Y. H., Zhu Z. M., Zhu J. H., Lu J. G. (2020). Formation of nodules on continental slopes in the northeast of the South China Sea and its implications for cold seep. J. Mar. Sci. 38, 16–25. doi: 10.3969/j.issn.1001-909X.2020.02.03
Ma R. P., Ba J., Carcione J. M., Zhou X., Li F. (2019). Dispersion and attenuation of compressional waves in tight oil reservoirs: Experiments and simulations. Appl. Geophysics. 16, 33–45. doi: 10.1007/s11770-019-0748-3
Ma S. F., Han D. K., Gan L. D., Yang H. (2010). A review of seismic rock physics models. Prog. Geophys 2, 460–471. doi: 10.3969/j.issn.1004-2903.2010.02.012
Rafavich F., Kendall C. S. C., Todd T. P. (1984). The relationship between acoustic properties and the petrographic character of carbonate rocks. Geophysics. 49, 1622–1636. doi: 10.1190/1.1441570
Sayers C. M. (2008). The elastic properties of carbonates. Leading Edge. 8, 1020–1024. doi: 10.1190/1.2967555
Shan C. C., Deng X. G., Wen M. M., Feng Q. Q., Syed W. H., Huang W. (2020). Application of parametric sub-bottom profile in gas plumes detection: ATLAS P70 in Makran area as an example. Prog. Geophysics. 35, 1183–1190. doi: 10.6038/pg2020DD0148
Tong H. P., Feng D., Chen D. F. (2012). Progresses on petrology, mineralogy and geochemistry of cold seep carbonates in the northern South China Sea. J. Trop. Oceanography. 31, 45–56. doi: 10.3969/j.issn.1009-5470.2012.05.007
Wang B., Luan Z. D., Zhang X., Xi S. C., Li L. F., Lian C., et al. (2019). Topographic and geomorphological features of the Formosa Ridge cold seep system, the Southwestern Taiwan Island. Mar. Sci. 43, 51–59. doi: 10.11759/hykx20171120003
Wei X., Wang S. X., Zhao J. G. (2015). Laboratory study of velocity dispersion of the seismic wave in fluid-saturated sandstones. Chin. J. Geophysics. 58, 3380–3388. doi: 10.6038/cjg20150930
Wu X. T., Liu L. H., Wu N. Y., Cheng J. W. (2015). Geochemistry of early diagenesis in marine sediments: research progress. Mar. Geology Frontiers. 31, 17–26. doi: 10.16028/j.1009-2722.2015.12003
Wu D. D., Wu N. Y., Ye Y., Han X. Q., Huang Y. Y., Suess E. (2009). Petrographic characteristics of authigenic carbonates from Jiulong methane reef of northern South China Sea. J. Trop. Oceanography, 74–81.
Wyllie M. R. J., Gregory A. R., Gardner L. W. (1956). Elastic wave velocities in heterogeneous and porous media. Geophysics. 21, 41–70. doi: 10.1190/1.1438217
Xi S. C., Zhang X., Wang B., Luan Z. D., Chen C. A., Yan J. (2017). The indicators of seabed cold seep and comparison among main distribution areas. Mar. Geol. Front. 33, 7–18. doi: 10.16028/j.1009-2722.2017.02002
Xue Y. S., Huang J. H. (2016). Advances in study of cold seep deposition and palaeoclimatic and palaeoenviromental significance. Geological Sci. Technol. Information. 35, 97–104.
Yan P., Wang Y. L., Zheng H. B. (2011). Characteristics of deep water sedimentation revealed by sub-bottom profiler survey over the Baiyun Sag-Southwest Dongsha Island Waters in the norther South China Sea. J. Trop. Oceanogr. 30, 115–122.
Yan P., Wang Y. L., Zheng H. B., Zou D. P., Chen Z. (2014). Geophysical features of mud volcanoes in the waters southwest of Dongsha Islands. Acta Oceanologica Sinica. 36, 142–148. doi: 10.3969/j.issn.0253-4193.2014.07.016 (in Chinese with English abstract
Zhang H., Yang S., Wu N. Y., Su X., Holland M., Schultheiss P., et al. (2007). Successful and surprising results for China first gas hydrate drilling expedition. Fire Ice 7, 6–9.
Keywords: cold spring, carbonate rocks, seafloor sediments, sound speed, reflection coefficient
Citation: Tian Y, Wu L, Zou D, Chen Z, Jiang Y, Yan P and Fan C (2023) Predicting the acoustic characteristics of seafloor sediments containing cold spring carbonate rocks. Front. Mar. Sci. 10:1243780. doi: 10.3389/fmars.2023.1243780
Received: 21 June 2023; Accepted: 07 August 2023;
Published: 28 August 2023.
Edited by:
Guangming Kan, Ministry of Natural Resources, ChinaReviewed by:
Yanliang Pei, Ministry of Natural Resources, ChinaCopyright © 2023 Tian, Wu, Zou, Chen, Jiang, Yan and Fan. This is an open-access article distributed under the terms of the Creative Commons Attribution License (CC BY). The use, distribution or reproduction in other forums is permitted, provided the original author(s) and the copyright owner(s) are credited and that the original publication in this journal is cited, in accordance with accepted academic practice. No use, distribution or reproduction is permitted which does not comply with these terms.
*Correspondence: Dapeng Zou, YW50aG9ueXpvdUBnZHV0LmVkdS5jbg==; Zhong Chen, Y2h6aHNvdXRoQHNjc2lvLmFjLmNu
Disclaimer: All claims expressed in this article are solely those of the authors and do not necessarily represent those of their affiliated organizations, or those of the publisher, the editors and the reviewers. Any product that may be evaluated in this article or claim that may be made by its manufacturer is not guaranteed or endorsed by the publisher.
Research integrity at Frontiers
Learn more about the work of our research integrity team to safeguard the quality of each article we publish.