- 1Norwegian Polar Institute, Fram Centre, Tromsø, Norway
- 2Department of Arctic and Marine Biology, Faculty of Biosciences, Fisheries and Economics, UiT The Arctic University of Norway, Tromsø, Norway
- 3Department of Arctic Biology, The University Centre in Svalbard (UNIS), Longyearbyen, Norway
- 4Ecological Chemistry, Alfred-Wegener Institute Helmholtz Centre for Polar and Marine Research, Bremerhaven, Germany
The Arctic region is undergoing rapid and significant changes, characterized by high rates of acidification and warming. These transformations prompt critical questions about the resilience of marine communities in the face of environmental change. In the Arctic, marine zooplankton and in particular calanoid copepods play a vital role in the food web. Changes in environmental conditions could disrupt zooplankton communities, posing detrimental consequences for the entire ecosystem. Copepod early-life stages have been shown to be particularly sensitive to environmental stressors since they represent a bottleneck in the life cycle. Here, we investigated the responses of 4-day old Calanus hyperboreus nauplii when exposed to acidification (pH 7.5 and 8.1) and warming (0 and 3°C), both independently and in combination. Naupliar respiration rates increased when exposed to a combination of acidification and warming, but not when exposed to the stressors individually. Moreover, we found no discernible differences in lipid content and fatty acid (FA) composition of the nauplii across the different experimental treatments. Wax esters accounted for approximately 75% of the lipid reserves, and high amounts of long chain fatty acids 20:1 and 22:1, crucial for the reproduction cycle in copepods, were also detected. Our results indicate a sensitivity of these nauplii to a combination of acidification and warming, but not to the individual stressors, aligning with a growing body of evidence from related studies. This study sheds light on the potential implications of global change for Arctic copepod populations by elucidating the responses of early-life stages to these environmental stressors.
1 Introduction
The Arctic region is rapidly changing, experiencing the highest rates of ocean acidification (OA) and warming on a global scale (AMAP, 2018). The occurrence of these environmental stressors frequently amplifies the impact of pre-existing pressures in the region, such as fluctuations in salinity or the discharge of environmental contaminants (Gunderson et al., 2016; AMAP, 2021). The physical changes resulting from acidification, solar radiation and warming can trigger changes in natural marine communities (e.g. changes in community composition, altered recruitment processes), potentially leading to cascading effects at the ecosystem level. In the Arctic region, many studies investigating the impact of climate change on ecosystem structure and functioning have focused on coastal ecosystems around Svalbard (Hop et al., 2019a, Hop et al., 2019b), as these areas experience the greatest variability in physical parameters. However, fewer studies have examined the responses of open-ocean organisms in Arctic waters to environmental changes (Ramondenc et al., 2022).
In the Arctic, marine zooplankton are a crucial element of the food web, connecting primary producers and predators, and any changes in their community composition and biology could have far-reaching consequences for the energy flow towards higher trophic levels (Falk-Petersen et al., 2007). Arctic copepods represent a critical food source for carnivorous zooplankton and fish, such as polar cod (Boreogadus saida), capelin (Mallotus villosus) and Arctic char (Salvelinus alpinus), and seabirds such as little auk (Alle alle)(Karnovsky et al., 2003; Falk-Petersen et al., 2007; Hop and Gjøsæter, 2013). The predominantly herbivorous copepods of the genus Calanus dominate the zooplankton biomass in the Arctic (Falk-Petersen et al., 2009; Daase et al., 2021). Calanus hyperboreus is the most important zooplankton species in terms of biomass in the Central Arctic Ocean (CAO, Ershova et al., 2021), although it is also present in marginal seas, such as the northern Barents Sea.
To endure the characteristic prolonged periods of food limitation associated with the Polar Night, Arctic copepods can undergo seasonal vertical migrations (diapause) and have multi-year life cycles (Daase et al., 2021). Successful reproduction of C. hyperboreus depends on energy reserves that the females pass onto their offspring via egg development. This species is a capital breeder, meaning that it relies entirely on its internal lipid reserves to fuel its reproduction (Falk-Petersen et al., 2009; Varpe, 2012). Calanus hyperboreus accumulates sufficient lipid stores to overwinter and can reproduce during winter, independent of the ice-algae and phytoplankton spring blooms (Hirche, 1997; Halvorsen, 2015; Daase et al., 2021). Egg production can occur from November to May (Halvorsen, 2015). At hatching, nauplii rely on stored lipid energy reserves to cover their metabolic costs during non-feeding stages (nauplii I to II) and will utilize the spring or summer blooms for growth and further development once these become available (Falk-Petersen et al., 2009; Jung-Madsen et al., 2013).
Early-life stages of marine invertebrates are known to be particularly vulnerable to environmental changes, and any impacts on these stages can have significant consequences for entire populations (Byrne, 2011). To fully comprehend the fate of marine ecosystems under future scenarios of change, it is crucial to understand how early-life stages respond to environmental stressors (Byrne, 2012). In the Arctic, changes in environmental parameters could alter the conditions that C. hyperboreus nauplii encounter after hatching, with potentially negative consequences for their survival. This could happen either directly, through changes in larval physiology or metabolism, or indirectly, through alterations in the timing and availability of their preferred food (Espinel-Velasco et al., 2018). This is especially important if changes in spring bloom phenology are expected in future scenarios (Falk-Petersen et al., 2007). Depletion of larval energy reserves prior to reaching feeding stages or before the food becomes available could change recruitment patterns, potentially leading to significant consequences for the ecology of the species. For example, C. hyperboreus nauplii from regions where the females overwinter at depths greater than 2000 m, such as the Fram Strait or the CAO, will need to actively swim upwards in order to reach the surface in time for the spring bloom (Jung-Madsen et al., 2013). Changes in metabolism or lipid energy reserves could compromise the timing of reaching the surface, potentially leading to adverse impacts on survival.
Arctic Calanus species have been the focus of numerous studies examining the effects of OA and warming on their physiology, including works by Lewis et al. (2013); Hildebrandt et al. (2014); Thor et al. (2016); Bailey et al. (2017a); Bailey et al. (2017b) and Thor et al. (2018). Although most research on calanoid copepods in the Arctic has focused on adult and late larval stages (CIV and CV), a few studies have explored the impacts of environmental stressors on naupliar stages and have indicated sensitivity to either acidification (Lewis et al., 2013) or warming (Jung-Madsen et al., 2013), individually, and only a handful of observations revealed interactive effects (e.g. warming and pyrene; Jortveit, 2022).
In this study, we conducted an exploratory assay to investigate the interactive effects of acidification, singularly and in combination with warming on 4-day old C. hyperboreus nauplii from females collected in the Barents Sea. We hypothesized that C. hyperboreus nauplii would be sensitive to both acidification and warming, and that this would be evident through altered respiration rates. To our knowledge, this is the first study investigating the responses of C. hyperboreus naupliar stages to multiple environmental stressors.
2 Materials and methods
2.1 Copepod collection and nauplii hatching
Mesozooplankton, including C. hyperboreus, was collected using a Bongo net (with opening 0.28 m2 and mesh size 180 μm) equipped with a non-filtering cod end. During The Nansen Legacy seasonal cruise Q1 in March 2021, vertical net hauls were conducted at station P4 (79.75°N, 34.00°E) on the shelf (Figure 1), at a depth of ~300 m (bottom depth 332 m), as reported by Gerland et al. (2022). The collected samples were placed in a 60 L bucket filled with sea water acclimatized to the in-situ temperature. Gravid females of C. hyperboreus were carefully extracted from subsamples using tweezers and transferred into separate petri dishes filled with 15 mL of filtered seawater. These females were incubated in the dark for 10 days at 0°C. Every 48 h, half of the water in the petri dishes was replaced with filtered seawater at the same temperature. Eggs produced during the incubation period were counted every 24 h and transferred to new petri dishes until hatching (The Nansen Legacy, 2022). The nauplii hatched over a 4-day period were collected and pooled in a larger (2 L) glass jar with filtered seawater until the start of the incubation experiments. By that time, the nauplii were most likely N1 (with mean developmental time = 2.8 - 3.1 days, stage duration 2.3 - 2.5 days at 5°C; Jung-Madsen et al., 2013). The first two naupliar stages of C. hyperboreus do not feed, therefore no feeding took place prior to the start of the incubations. No abnormal nauplii were detected nor removed from the pool of larvae for the subsequent experiments.
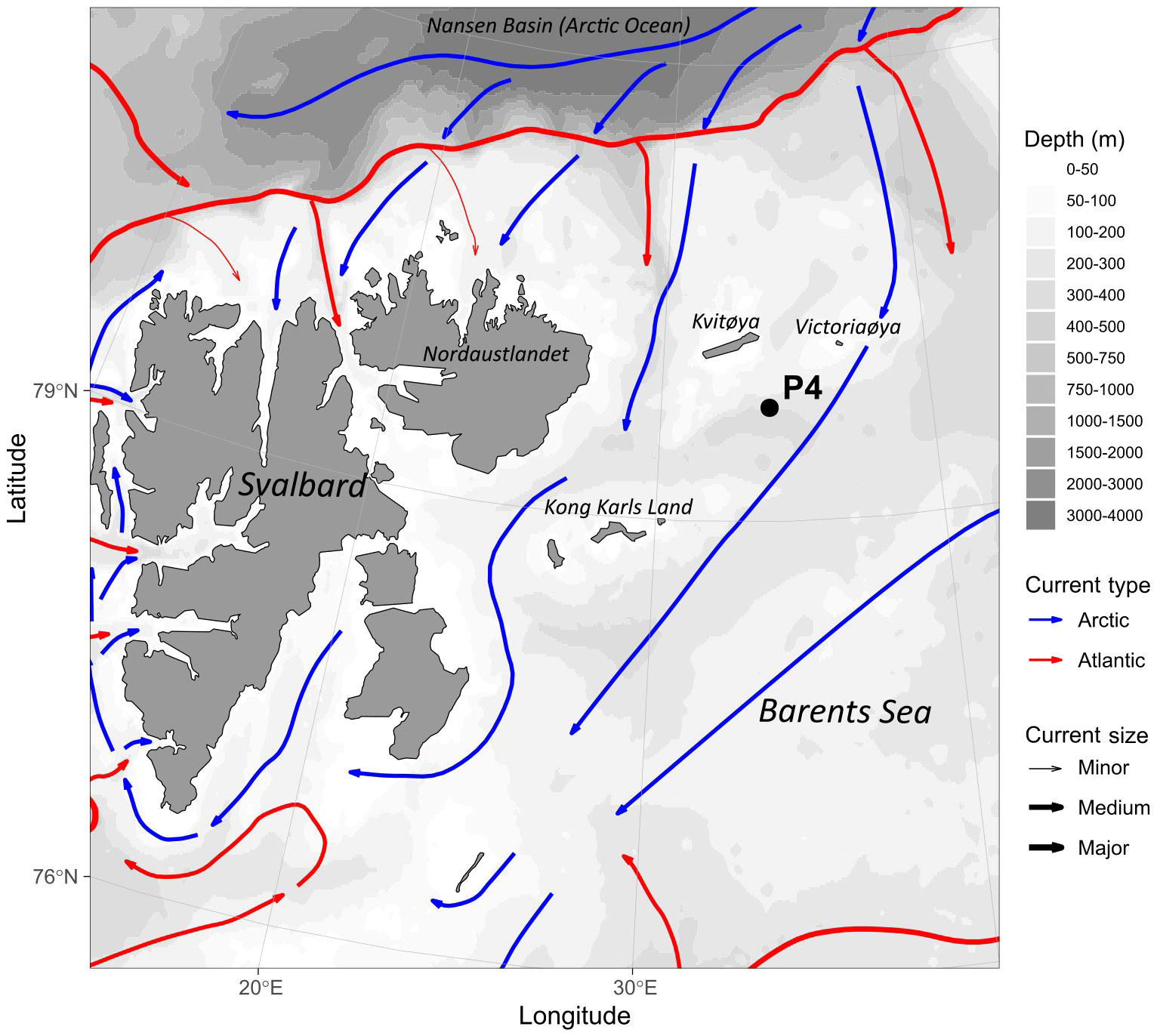
Figure 1 Location of the P4 station in the Barents Sea sampled during The Nansen Legacy seasonal cruise in March 2021, where Calanus hyperboreus females were collected for this study. The coloured lines indicate the type and direction of water masses (blue: Arctic; red: Atlantic), whereas the grey shadowing indicates depth. The current data were plotted in R using GGOceanmaps package and shapefiles from Natural Earth Data (Vihtakari et al., 2019; Vihtakari, 2022) and bathymetry data from NOAA (Amante and Eakins, 2009; NOAA, 2009).
2.2 Measurements of metabolic rates and closed-bottle incubations
Using the pool of hatched larvae, we conducted parallel measurements of metabolic rates as well as short-term bottle incubations to test the responses of the nauplii to acidification and warming. The experimental treatments consisted of a full factorial combination of pH and temperature as follows: i) low pH (7.5) + low/ambient temperature (0°C), ii) high/ambient pH (8.1) + low/ambient temperature (0°C), iii) low pH (7.5) + high temperature (3°C), iv) high/ambient pH (8.1) + high temperature (3°C). The pH treatments were achieved by manually introducing gaseous CO2 into filtered seawater and corrected with filtered seawater at ambient pH until reaching the desired pH for each of the target temperatures, previously to introducing the larvae. Salinity, pH and temperature were manually checked with a handheld probe (Hanna pH meter HI98191).
To measure metabolic rates, 240 randomly-selected individuals were chosen from the pool of hatched nauplii. Respiration measurements were conducted using the Loligo® Microplate Respirometry System (MicroPlate™ software version 1.0.4) with 24 × 500 μL multiwell plates (Loligo Systems, Denmark). The plates were previously calibrated with filtered (0.2 μm) seawater at the target temperature and salinity 34. Calibration was carried out using water supersaturated in oxygen (100% O2 air-saturation content) and water depleted of O2 through the addition of sodium sulphite (0% air saturation). For the measurements, three nauplii were introduced into each well with water at the target treatment by means of a small pipette. Four wells in each plate served as a control, containing only filtered seawater, to calculate the background rate. The metabolic rate measurements were performed in the dark in incubators at a constant target temperature (0°C or 3°C) for 12 h. After measuring the metabolic rates, we visually inspected nauplii from each plate (treatment) for survival based on movement.
For the incubation assay we randomly selected 1440 nauplii from the pool of hatched individuals. Short-term incubations were conducted in triplicate using 200 mL brown glass bottles, representing one of the four experimental treatments. Nauplii were added to each replicate at a density of ~0.6 ind. mL-1. The bottles were kept in the dark in incubators at the target temperature for 24 h. After the incubation, all larvae from each treatment were extracted and checked for survival, and the remaining individuals were stored in Eppendorf tubes, freeze-dried, and kept at -80°C for lipid content analysis. To assess their body condition (i.e., level of stored lipids), the lipid content and fatty acid composition of the copepod nauplii were analyzed at the Alfred Wegener Institute in Bremerhaven, Germany (see Supplementary Material and supporting data: 10.21334/npolar.2023.edc957ac; Espinel-Velasco et al., 2023a). The relative proportions of wax esters (main storage lipids of Calanus spp.) were estimated from the relation of fatty acids to fatty alcohols.
2.3 Statistical analyses
Data visualization and statistical analyses were performed with R v. 4.0.3. and RStudio v.1.4.1103 (R Core Team, 2021). The analysis and calculation of the metabolic rates were performed using the RespR package (Harianto et al., 2019). The main effects and interactions of pH and temperature on naupliar respiration were tested with a two-way ANOVA followed by a post-hoc Tukey’s test (p < 0.05 was considered significant). The Levene’s test was used to test for homogeneity of variances (conditions met).
3 Results
Individual naupliar respiration rates ranged from 0.055 ngO2 ind.-1 h-1 (low pH and low temperature treatment) to 11.37 ngO2 ind.-1 h-1 (low pH and high temperature treatment; Figure 2 and supporting data 10.21334/npolar.2023.ece3e9bb; Espinel-Velasco et al., 2023b).
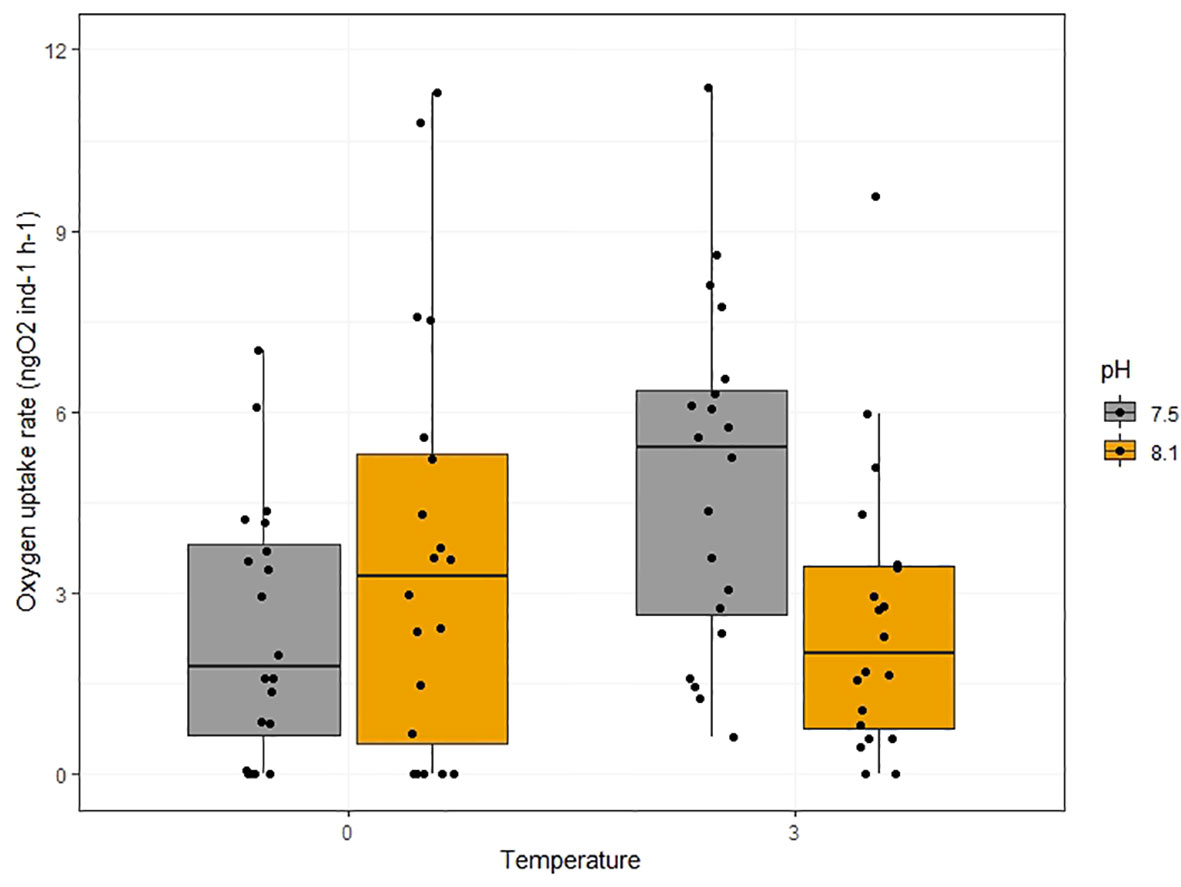
Figure 2 Individual oxygen uptake rates of Calanus hyperboreus nauplii (in ngO2 h-1) relative to the pH (colors) and water temperature (°C). The box-and-whisker plot displays the median (horizontal bar), interquartile range (box), and minimum/maximum values excluding outliers (whiskers). Outliers were defined as values beyond 1.5 times the interquartile range. The sample size was n = 240. The individual data points have been added on top of the boxplots and have been slightly offset for clarity.
Individual naupliar respiration rates were not significantly influenced by pH (F1 = 0.799, p = 0.374) or temperature alone (F1 = 1.352, p = 0.248). The statistical analyses point towards a significant effect of the interaction of both factors in the observed respiration rates (F1 = 8.784, p = 0.004). Metabolic rates measured in nauplii kept at pH 7.5 and 3°C were significantly higher compared to their counterparts in 0°C (p = 0.023) and their counterparts in pH 8.1 and 3°C (p = 0.038).
4 Discussion
The results of our investigation indicated increased respiration rates of C. hyperboreus nauplii when exposed to a combination of warming and acidification, but not when exposed to the stressors individually. This suggests a sensitivity to the interactive effect while being more resilient to each of the stressors alone. To our knowledge, very few studies investigate the responses of early naupliar stages of Arctic Calanus copepods to multiple stressors. While there have been some investigations on the effects of acidification (Lewis et al., 2013; Bailey et al., 2017b) or temperature (Jung-Madsen et al., 2013) on nauplii, there is a scarcity of studies that look at the effect of multiple stressors on these nauplii.
The responses of calanoid copepods to ocean acidification appear to be dependent on the taxa and life-stage (Wang et al., 2018). For example, hatching rates in Acartia steueri and Acartia erythraea decrease following an exposure to OA (Kurihara et al., 2004), while vertically migrating adult Calanus spp. seem to be only marginally affected by high pCO2 (Lewis et al., 2013). In Calanus finmarchicus females, OA did not affect egg production or cause biomass loss, but impacted naupliar hatching rate (Mayor et al., 2007). Studies focusing on Calanus glacialis have shown that ocean acidification increases metabolic rates and decreases ingestion rates in copepodite stage IV, but not in stage V (390 and 3000 µatm pCO2, Hildebrandt et al., 2014; ~ 800 µatm pCO2, Thor et al., 2016). In contrast, in C. glacialis females, no negative responses have been shown on metabolic rates, gonad maturation rate, or mortality after long term incubations (several months at 390 and 3000 µatm pCO2; Hildebrandt et al., 2014). However, OA can cause delayed hatching and reduced overall hatching success, although egg production remains unchanged (pH ~ 6.9, Weydmann et al., 2012).
Increased temperatures can also have various effects on calanoid copepods, including altered fecundity (5 to 25°C; Hirst and Kiørboe, 2002; Bunker and Hirst, 2004), reduced grazing rates (10 and 14°C in female C. finmarchicus; Van Dinh et al., 2019), and increased oxygen consumption rates and sublethal stress (in diapausing C. hyperboreus females at 0, 5 and 10°C; Hildebrandt et al., 2014). These responses also seem to be taxa- and stage-specific. For example, increased temperature (5, 10 and 15°C) upregulates heat shock proteins in C. finmarchicus but not in C. glacialis (Smolina et al., 2015).
In contrast to the straightforward reactions to individual stressors, the way marine species respond to multiple stressors can be intricate and dependent on the specific taxon, on life stage and on the strength of the stressor itself (e.g. concentration level). Numerous studies have suggested that acidification and warming can produce synergistic effects, distinct from the additive responses observed in single-stressor situations. Multiple studies have explored the impacts of various stressors, such as warming, acidification, and increased pollution, on copepod survival, growth, development, fecundity, egg production rate, hatching success, and feeding (e.g. Zervoudaki et al., 2014; Garzke et al., 2016; Horn et al., 2016). Although a plethora of research has been conducted in this field, Arctic species have received relatively little attention. From the literature available, it is apparent that these stressors can have synergistic effects on copepod populations, resulting in negative impacts on reproduction and population growth rates. For instance, long-term investigations on adult C. glacialis and C. hyperboreus revealed a synergistic effect of ocean acidification (390 and 3000 µatm pCO2) with ocean warming (0, 5 and 10°C), but no effect of acidification alone (Hildebrandt et al., 2014). Another study explored the combined effects of ocean warming and exposure to the oil compound pyrene on C. finmarchicus and found that the combined stressors negatively impacted the copepod’s survival and growth (100 nM pyrene at 0 and 10°C, Grenvald et al., 2013).
Our observations showed no clear response to acidification, concurrent with previous studies on Calanus nauplii: Bailey et al. (2017b) studied naupliar development in wild populations of C. glacialis and found unaffected respiration rates under ocean acidification (pCO2 320 to 1700 µatm), as well as development and growth, although this was compensated by altered gene expression (Bailey et al., 2017a). However, Lewis et al. (2013) found lower survival and seemingly greater sensitivity to manipulated OA conditions (pCO2 1000 μatm) predicted for the year 2100 in Calanus spp. nauplii compared to the adults in a short-term exposure experiment. Similarly, exposure to acidification (over 5000 ppm CO2) resulted in an increase in naupliar mortality in the copepods Acartia steueri and A. erythraea (Kurihara et al., 2004). Reasons behind these discrepancies include differences in experimental design, geographical variations (European vs. Canadian Arctic), and variations in the parameters used to measure responses.
Conversely, our observations of unchanged respiration as a response to warming contrast with a study on C. hyperboreus nauplii respiration under warming conditions, where authors found a clear sensitivity to warming alone in early-larval stages, which might affect future recruitment (Jung-Madsen et al., 2013). One explanation for these discrepancies could be differences in the experimental design (we tested 0°C and 3°C, while they tested 0, 5 and 10°C; we tested short-term exposure [24h] while they tested long-term exposure [40 days]), as well as geographical differences (we used C. hyperboreus collected in the northern Barents Sea, whereas they tested individuals collected in Eastern Greenland). Different populations of copepods may have varying degrees of tolerance to environmental stressors. For example, geographically-distinct populations of C. glacialis showed physiological differences in OA responses (Thor et al., 2018).
Our results indicated a sensitivity of the nauplii to the combined exposure to acidification and warming through a significant impact on the metabolic rate of the nauplii, as demonstrated by increased oxygen uptake. This could be due to increased energy requirements when exposed to warming, leading to less energy available to cope with acidification. These observations support the notion that responses to multiple stressors in marine invertebrates, particularly during early-life stages are more complex than just additive. For example, in a recent multiple-stressor study by Jortveit (2022), it was observed that temperature (5°C) increased the lethal sensitivity of C. glacialis nauplii to pyrene, while other exposure combinations did not significantly affect naupliar survival. However, since our study was focused on short-term exposure, we did not measure survival as an endpoint, and it is therefore not possible to compare both studies.
Negative impacts on physiological endpoints, such as changes in metabolic rates from exposure to stressors such as acidification, may be attributed to constraints in the energy budget (Pedersen et al., 2014). As a result, potential scenarios of environmental change may increase energy demands, which could affect the natural communities of Calanus copepods with potential negative effects on higher trophic levels.
As most of the copepod congeners, C. hyperboreus stores lipids primarily in the form of wax esters (Falk-Petersen et al., 2009; Supporting data 10.21334/npolar.2023.edc957ac; Espinel-Velasco et al., 2023a). Nauplii have large oil droplets at hatching, which sustain them during their non-feeding stages until the spring bloom. In contrast to other studies (e.g. pteropods: Lischka et al., 2022), our study found no differences in lipid content and fatty acid (FA) composition of the nauplii between the different experimental treatments. This is expected since lipid-reserve mobilization in Calanus nauplii is slow after a long period of starvation (Daase et al., 2011), and our exposure duration was short. The FA composition observed in our nauplii is consistent with previous investigations on Arctic C. hyperboreus, with wax esters comprising ~75% of the lipid reserves. This observation concurs with analyses of C. hyperboreus nauplii collected east of Greenland (85-90% WE; Jung-Madsen et al., 2013). Moreover, we observed high relative proportions of long chained fatty acids 20:1 and 22:1, which are key elements for the reproduction cycle in copepods (see supporting data: 10.21334/npolar.2023.edc957ac; Espinel-Velasco et al., 2023a). Given that the decrease in lipid during the naupliar development is most likely due to the nauplii metabolizing the lipid reserves to cover their energy requirements, changes in the amount of lipids passed on from the mothers, or changes in energy requirements of the larvae, could have significant implications for the recruitment of the species. A warmer Arctic ocean with smaller mothers (Halvorsen, 2015) could lead to earlier spawning and smaller nauplii. Moreover, warmer temperatures alter the larval development and increase the metabolism (increasing carbon requirements), as well as decrease the duration of starvation that the nauplii can survive, potentially affecting the timing to reach the spring bloom. Therefore, a synergistic effect of warming and acidification could imply that nauplii may not survive a potential mismatch with the ice break-up and resulting phytoplankton bloom, which would have detrimental effects on the species recruitment (Søreide et al., 2010; Daase et al., 2013).
In recent years, molecular techniques such as metabolomics have emerged as a powerful tool to study the physiological mechanisms that organisms use to cope with environmental stressors. Combining these new techniques with traditional measurements can provide a more comprehensive understanding of the potential responses of Arctic zooplankton communities to future changes. For instance, a recent study by Thor et al. (2022) has demonstrated the usefulness of metabolomics in elucidating the metabolic pathways and responses of copepods to environmental stressors (through changes in cellular metabolism). Therefore, incorporating these molecular techniques into future experimental investigations could shed light on the underlying mechanisms that govern the physiological responses of Arctic organisms to multiple stressors.
While there is a growing body of literature on the responses of early life stages of Arctic zooplankton to combined stressors, many questions remain unanswered. The limited research on the effects of combined environmental stressors on Arctic copepods, including C. hyperboreus, highlights the need for further experimental investigations to fully comprehend their physiological responses in potential future Arctic scenarios. Future work should include long-term investigations that could help understand not only responses, but also discern resilience of the Arctic ecosystems (Griffith et al., 2019). Early-life stages remain an important element to consider, as they can act as a bottleneck for the development of the species. By furthering our understanding of how these organisms respond to environmental stressors, we may be better equipped to mitigate the effects of climate change on these vital ecosystems.
Data availability statement
Supporting data for this study is available at 10.21334/npolar.2023.edc957ac (fatty acid data; Espinel-Velasco et al., 2023a) and 10.21334/npolar.2023.ece3e9bb (oxygen uptake rates; Espinel-Velasco et al., 2023b).
Ethics statement
The manuscript presents research on animals that do not require ethical approval for their study.
Author contributions
NE-V, CG and VP contributed to the conception and design of the study. NE-V, CG and VP carried out the experimental investigation. DK and MG carried out the lipid laboratory analyses. NE-V performed the analysis of the respiration data and the statistical analysis. NE-V wrote the first draft of the manuscript and subsequent versions. CG and DK wrote sections of the manuscript. All authors contributed to manuscript revision, read, and approved the submitted version.
Funding
This work was funded by the Research Council of Norway through the project The Nansen Legacy (RCN # 276730).
Acknowledgments
We thank Anette Wold, Norwegian Polar Institute, for her invaluable assistance during the sampling process, as well as the crew on board RV Kronprins Haakon.
Conflict of interest
The authors declare that the research was conducted in the absence of any commercial or financial relationships that could be construed as a potential conflict of interest.
Publisher’s note
All claims expressed in this article are solely those of the authors and do not necessarily represent those of their affiliated organizations, or those of the publisher, the editors and the reviewers. Any product that may be evaluated in this article, or claim that may be made by its manufacturer, is not guaranteed or endorsed by the publisher.
Supplementary material
The Supplementary Material for this article can be found online at: https://www.frontiersin.org/articles/10.3389/fmars.2023.1240673/full#supplementary-material
References
Amante C., Eakins B. W. (2009). ETOPO1 1 arc-minute global relief model: procedures, data sources and analysis. NOAA technical memorandum NESDIS NGDC-24 (National Geophysical Data Center, NOAA), p. 19. doi: 10.7289/V5C8276M
AMAP (2018). AMAP assessment 2018: Arctic ocean acidification (Tromsø, Norway: Arctic Monitoring and Assessment Programme (AMAP).
AMAP (2021). Arctic climate change update 2021: key trends and impacts. Summary for policy-makers (Tromsø, Norway: Arctic Monitoring and Assessment Programme (AMAP).
Bailey A., De Wit P., Thor P., Browman H. I., Bjelland R., Shema S., et al. (2017a). Regulation of gene expression is associated with tolerance of the Arctic copepod Calanus glacialis to CO2-acidified sea water. Ecol. Evol. 7, 7145–7160. doi: 10.1002/ece3.3063
Bailey A., Thor P., Browman H. I., Fields D. M., Runge J., Vermont A., et al. (2017b). Early life stages of the Arctic copepod Calanus glacialis are unaffected by increased seawater pCO2. ICES J. Mar. Sci. 74, 996–1004. doi: 10.1093/icesjms/fsw066
Bunker A. J., Hirst A. G. (2004). Fecundity of marine planktonic copepods: global rates and patterns in relation to chlorophyll a, temperature and body weight. Mar. Ecol. Prog. Ser. 279, 161–181. doi: 10.3354/meps279161
Byrne M. (2011). Impact of ocean warming and ocean acidification on marine invertebrate life history stages: vulnerabilities and potential for persistence in a changing ocean. Oceanogr. Mar. Biol. Ann. Rev. 49, 1–42. doi: 10.1016/j.marenvres.2011.10.00
Byrne M. (2012). Global change ecotoxicology: Identification of early life history bottlenecks in marine invertebrates, variable species responses and variable experimental approaches. Mar. Environ. Res. 76, 3–15. doi: 10.1016/j.marenvres.2011.10.004
Daase M., Berge J., Søreide J. E., Falk-Petersen S. (2021). “Ecology of Arctic pelagic communities,” in Arctic ecology. Ed. Thomas D. N., 231–259. doi: 10.1002/9781118846582.ch9
Daase M., Falk-Petersen S., Varpe Ø., Darnis G., Søreide J. E., Wold A., et al. (2013). Timing of reproductive events in the marine copepod Calanus glacialis: A pan-Arctic perspective. Can. J. Fish. Aquat. Sci. 70, 871–884. doi: 10.1139/cjfas-2012-0401
Daase M., Søreide J. E., Martynova D. (2011). Effects of food quality on naupliar development in Calanus glacialis at subzero temperatures. Mar. Ecol. Prog. Ser. 429, 111–124. doi: 10.3354/meps09075
Ershova E. A., Kosobokova K. N., Banas N. S., Ellingsen I., Niehoff B., Hildebrandt N., et al. (2021). Sea ice decline drives biogeographical shifts of key Calanus species in the central Arctic Ocean. Glob. Change Biol. 27, 2128–2143. doi: 10.1111/gcb.15562
Espinel-Velasco N., Gawinski C., Kohlbach D., Pitusi V., Graeve M., Hop H. (2023a). Fatty acid composition (relative proportions in %) of 4-day old Calanus hyperboreus nauplii from mothers collected in the Northern Barents Sea (Norwegian Polar Institute). doi: 10.21334/npolar.2023.edc957ac
Espinel-Velasco N., Gawinski C., Kohlbach D., Pitusi V., Graeve M., Hop H. (2023b). Individual oxygen uptake rates of 4-day old Calanus hyperboreus nauplii when exposed to ocean acidification and warming (12 hour measurements) (Norwegian Polar Institute). doi: 10.21334/npolar.2023.ece3e9bb
Espinel-Velasco N., Hoffmann L., Agüera A., Byrne M., Dupont S., Uthicke S., et al. (2018). Effects of ocean acidification on the settlement and metamorphosis of marine invertebrate and fish larvae: a review. Mar. Ecol. Prog. Ser. 606, 237–257. doi: 10.3354/meps12754
Falk-Petersen S., Mayzaud P., Kattner G., Sargent J. R. (2009). Lipids and life strategy of Arctic Calanus. Mar. Biol. Res. 5, 18–39. doi: 10.1080/17451000802512267
Falk-Petersen S., Pavlov V., Timofeev S., Sargent J. R. (2007). “Climate variability and possible effects on arctic food chains: The role of Calanus,” in Arctic alpine ecosystems and people in a changing environment. Eds. Ørbæk J. B., Kallenborn R., Tombre I., Hegseth E. N., Falk-Petersen S., Hoel A. H. (Berlin: Springer-Verlag), 147–166. doi: 10.1007/978-3-540-48514-8_9
Garzke J., Hansen T., Ismar S. M. H., Sommer U. (2016). Combined effects of ocean warming and acidification on copepod abundance, body size and fatty acid content. PloS One 11, 1–22. doi: 10.1371/journal.pone.0155952
Gerland S., Wold A., Altuna N. E., Anglada-Ortiz G., Arumi M. A., Bodur Y., et al. (2022). “Seasonal cruise Q1 2021: Cruise report,” in The Nansen Legacy report series. doi: 10.7557/nlrs.6464
Grenvald J. C., Nielsen T. G., Hjorth M. (2013). Effects of pyrene exposure and temperature on early development of two co-existing Arctic copepods. Ecotoxicology 22, 184–198. doi: 10.1007/s10646-012-1016-y
Griffith G. P., Hop H., Vihtakari M., Wold A., Kalhagen K., Gabrielsen G. W. (2019). Ecological resilience of Arctic marine food webs to climate change. Nat. Clim. Change 9, 868–872. doi: 10.1038/s41558-019-0601-y
Gunderson A. R., Armstrong E. J., Stillman J. H. (2016). Multiple stressors in a changing world: The need for an improved perspective on physiological responses to the dynamic marine environment. Ann. Rev. Mar. Sci. 8, 357–378. doi: 10.1146/annurev-marine-122414-033953
Halvorsen E. (2015). Significance of lipid storage levels for reproductive output in the Arctic copepod Calanus hyperboreus. Mar. Ecol. Prog. Ser. 540, 259–265. doi: 10.3354/meps11528
Harianto J., Carey N., Byrne M. (2019). respR—An R package for the manipulation and analysis of respirometry data. Methods Ecol. Evol. 10, 912–920. doi: 10.1111/2041-210X.13162
Hildebrandt N., Niehoff B., Sartoris F. J. (2014). Long-term effects of elevated CO2 and temperature on the Arctic calanoid copepods Calanus glacialis and C. hyperboreus. Mar. Pollut. Bull. 80, 59–70. doi: 10.1016/j.marpolbul.2014.01.050
Hirche J. (1997). Life cycle of the copepod Calanus hyperboreus in the Greenland Sea. Mar. Biol. 128, 607–618. doi: 10.1007/s002270050127
Hirst A., Kiørboe T. (2002). Mortality of marine planktonic copepods: global rates and patterns. Mar. Ecol. Prog. Ser. 230, 195–209. doi: 10.3354/meps230195
Hop H., Assmy P., Wold A., Sundfjord A., Daase M., Duarte P., et al. (2019a). Pelagic ecosystem characteristics across the Atlantic Water Boundary Current from Rijpfjorden, Svalbard, to the Arctic ocean during summer, (2010-2014). Front. Mar. Sci. 6. doi: 10.3389/fmars.2019.00181
Hop H., Gjøsæter H. (2013). Polar cod (Boreogadus saida) and capelin (Mallotus villosus) as key species in marine food webs of the Arctic and the Barents Sea. Mar. Biol. Res. 9, 878–894. doi: 10.1080/17451000.2013.775458
Hop H., Wold A., Vihtakari M., Daase M., Kwasniewski S., Gluchowska M., et al. (2019b). “Zooplankton in Kongsfjorden, (1996–2016) in relation to climate change,” in The ecosystem of Kongsfjorden, Svalbard. Eds. Hop H., Wiencke C. (Cham: Springer), 229–300. Adv. Polar Ecol. 2. doi: 10.1007/978-3-319-46425-1_7
Horn H. G., Boersma M., Garzke J., Lo M. G. J., Sommer U., Aberle N. (2016). Effects of high CO2 and warming on a Baltic Sea microzooplankton community. ICES J. Mar. Sci. 73, 772–782. doi: 10.1093/icesjms/fsv198
Jortveit A. (2022). Interactive effects of temperature, ocean acidification, and pyrene on Calanus glacialis nauplii. master’s thesis. (University of Oslo), 35.
Jung-Madsen S., Nielsen T. G., Grønkjær P., Hansen B. W., Møller E. F. (2013). Early development of Calanus hyperboreus nauplii: Response to a changing ocean. Limnol. Oceanogr. 58, 2109–2121. doi: 10.4319/lo.2013.58.6.2109
Karnovsky N. J., Kwaśniewski S., Węsławski J. M., Walkusz W., Beszczyńska-Möller A. (2003). Foraging behavior of little auks in a heterogeneous environment. Mar. Ecol. Prog. Ser. 253, 289–303. doi: 10.3354/meps253289
Kurihara H., Shimode S., Shirayama Y. (2004). Effects of raised CO2 concentration on the egg production rate and early development of two marine copepods (Acartia steueri and Acartia erythraea). Mar. Pollut. Bull. 49, 721–727. doi: 10.1016/j.marpolbul.2004.05.005
Lewis C. N., Brown K. A., Edwards L. A., Cooper G., Findlay H. S. (2013). Sensitivity to ocean acidification parallels natural pCO2 gradients experienced by Arctic copepods under winter sea ice. Proc. Natl. Acad. Sci. U. S. A. 110 (51), E4960-7. doi: 10.1073/pnas.1315162110
Lischka S., Greenacre M. J., Riebesell U., Graeve M. (2022). Membrane lipid sensitivity to ocean warming and acidification poses a severe threat to Arctic pteropods. Front. Mar. Sci. 9. doi: 10.3389/fmars.2022.920163
Mayor D. J., Matthews C., Cook K., Zuur A. F., Hay S. (2007). CO2-induced acidification affects hatching success in Calanus finmarchicus. Mar. Ecol. Prog. Ser. 350, 91–97. doi: 10.3354/meps07142
NOAA National Geophysical Data Center (2009) ETOPO1 1 arc-minute global relief model (NOAA National Centers for Environmental Information) (Accessed 05/06/2023).
Pedersen S. A., Håkedal O. J., Salaberria I., Tagliati A., Gustavson L. M., Jenssen B. M., et al. (2014). Multigenerational exposure to ocean acidification during food limitation reveals consequences for copepod scope for growth and vital rates. Environ. Sci. Technol. 48, 12275–12284. doi: 10.1021/es501581j
Ramondenc S., Nöthig E. M., Hufnagel L., Bauerfeind E., Busch K., Knüppel N., et al. (2022). Effects of Atlantification and changing sea-ice dynamics on zooplankton community structure and carbon flux between 2000 and 2016 in the eastern Fram Strait. Limnol. Oceanogr. 2012, 1–15. doi: 10.1002/lno.12192
R Core Team (2021) R: A language and environment for statistical computing. Available at: https://www.r-project.org/.
Smolina I., Kollias S., Møller E. F., Lindeque P., Sundaram A. Y. M., Fernandes J. M. O., et al. (2015). Contrasting transcriptome response to thermal stress in two key zooplankton species, Calanus finmarchicus and C. glacialis. Mar. Ecol. Prog. Ser. 534, 79–93. doi: 10.3354/meps11398
Søreide J. E., Leu E. V. A., Berge J., Graeve M., Falk-Petersen S. (2010). Timing of blooms, algal food quality and Calanus glacialis reproduction and growth in a changing Arctic. Glob. Change Biol. 16, 3154–3163. doi: 10.1111/j.1365-2486.2010.02175.x
The Nansen Legacy (2022). Sampling protocols: version 10. The nansen legacy report series. doi: 10.7557/nlrs.6684
Thor P., Bailey A., Dupont S., Calosi P., Søreide J. E., De Wit P., et al. (2018). Contrasting physiological responses to future ocean acidification among Arctic copepod populations. Glob. Change Biol. 24, 365–377. doi: 10.1111/gcb.13870
Thor P., Bailey A., Halsband C., Guscelli E., Gorokhova E., Fransson A. (2016). Seawater pH predicted for the year 2100 affects the metabolic response to feeding in copepodites of the Arctic copepod Calanus glacialis. PloS One 11 (12), e0168735. doi: 10.1371/journal.pone.0168735
Thor P., Vermandele F., Bailey A., Guscelli E., Sartrou L. L., Dupont S., et al. (2022). Ocean acidification causes fundamental changes in the cellular metabolism of the Arctic copepod Calanus glacialis as detected by metabolomic analysis. Sci. Rep. 12, 1–14. doi: 10.1038/s41598-022-26480-9
Van Dinh K., Olsen M. W., Altin D., Vismann B., Nielsen T. G. (2019). Impact of temperature and pyrene exposure on the functional response of males and females of the copepod Calanus finmarchicus. Environ. Sci. Pollut. Res. 26, 29327–29333. doi: 10.1007/s11356-019-06078-x
Varpe Ø. (2012). Fitness and phenology: Annual routines and zooplankton adaptations to seasonal cycles. J. Plankton Res. 34, 267–276. doi: 10.1093/plankt/fbr108
Vihtakari M. (2022). ggOceanMaps: Plot Data on Oceanographic Maps using 'ggplot2' (R package version 1.3.7). Available at: https://mikkovihtakari.github.io/ggOceanMaps/.
Vihtakari M., Sundfjord A., de Steur L. (2019). Barents Sea ocean-current arrows modified from Eriksen et al., (2018) (Norwegian Polar Institute and Institute of Marine Research). Available at: https://github.com/MikkoVihtakari/Barents-Sea-currents.
Wang M., Jeong C. B., Lee Y. H., Lee J. S. (2018). Effects of ocean acidification on copepods. Aquat. Toxicol. 196, 17–24. doi: 10.1016/j.aquatox.2018.01.004
Weydmann A., Søreide J. E., Kwasniewski S., Widdicombe S. (2012). Influence of CO2-induced acidification on the reproduction of a key Arctic copepod Calanus glacialis. J. Exp. Mar. Bio. Ecol. 428, 39–42. doi: 10.1016/j.jembe.2012.06.002
Keywords: Arctic copepods, early-life stages, metabolism, energy reserves, Barents Sea, environmental drivers, climate change, The Nansen Legacy
Citation: Espinel-Velasco N, Gawinski C, Kohlbach D, Pitusi V, Graeve M and Hop H (2023) Interactive effects of ocean acidification and temperature on oxygen uptake rates in Calanus hyperboreus nauplii. Front. Mar. Sci. 10:1240673. doi: 10.3389/fmars.2023.1240673
Received: 15 June 2023; Accepted: 12 October 2023;
Published: 26 October 2023.
Edited by:
Guang Yang, Chinese Academy of Sciences (CAS), ChinaReviewed by:
William Froneman, Rhodes University, South AfricaPeter Thor, Swedish University of Agricultural Sciences, Sweden
Copyright © 2023 Espinel-Velasco, Gawinski, Kohlbach, Pitusi, Graeve and Hop. This is an open-access article distributed under the terms of the Creative Commons Attribution License (CC BY). The use, distribution or reproduction in other forums is permitted, provided the original author(s) and the copyright owner(s) are credited and that the original publication in this journal is cited, in accordance with accepted academic practice. No use, distribution or reproduction is permitted which does not comply with these terms.
*Correspondence: Nadjejda Espinel-Velasco, bmFkamVqZGEuZXNwaW5lbEBndS5zZQ==
†Present address: Nadjejda Espinel-Velasco, Department of Marine Sciences, Tjärnö Marine Laboratory, University of Gothenburg, Strömstad, Sweden
Doreen Kohlbach, Department of Arctic and Marine Biology, Faculty of Biosciences, Fisheries and Economics, UiT The Arctic University of Norway, Tromsø, Norway
Vanessa Pitusi, The Arctic University Museum of Norway (UMAK), UiT The Arctic University of Norway, Tromsø, Norway