- 1Marine Invasive Species Program, California State Lands Commission, Long Beach, CA, United States
- 2Hazardous Substances and New Organisms Group, Environmental Protection Authority, Wellington, New Zealand
- 3Safinah Group, Gateshead, United Kingdom
- 4Chesapeake Biological Laboratory, University of Maryland Center for Environmental Science, Solomons, MD, United States
Effective environmental policy often involves introducing and maintaining important activities with positive outcomes while minimizing environmental consequences; essentially decoupling a positive activity from its negative impacts. In-water cleaning (IWC) of biofouling from ships’ submerged surfaces is an example of an activity with positive outcomes (e.g., maintaining optimal ship energy efficiency and decreased biosecurity risk) and unintended negative consequences (e.g., release of living organisms, biocides, and microplastics). Several approaches exist to mitigate these negative consequences, including debris capture, with primary and secondary treatment of removed particulate and dissolved materials. However, it is unlikely that these approaches will eliminate environmental risk. Policy makers should be aware of the full suite of risks related to ship IWC and the tradeoffs to consider when balancing mitigation approaches.
1 Introduction
The overarching goal of environmental protection regulations is to prevent or minimize environmental harm (i.e., perverse outcomes) associated with certain activities. When developing environmental protection regulations, policymakers are often encouraged to consider ‘decoupling’ as one of the main principles of sustainable development (United Nations Environment Programme [UNEP], 2011). The UNEP (2011) distinguishes between two types of decoupling: 1) resource decoupling defined as the reduction in the rate of use of resources per unit of economic activity; and 2) impact decoupling defined as the maintenance of economic output while minimizing the environmental impact of such activities.
Finding a balance between these objectives is challenging, as several additional factors and potential ‘rebound’ effects need to be considered from multi- and trans-disciplinary perspectives. As a result, policy adoption may lead to both direct and indirect negative consequences for the environment, economy, and industry. An example of an environmental protection policy with serious unintended consequences is the 2003 European Union Directive on the Promotion of the Use of Biofuels and other Renewable Fuels in Transport (Directive 2003/03/EC) that aimed to reduce greenhouse gas (GHG) emissions by introducing targets for the proportion of biofuel in transport fuel (EU, 2003; Lange, 2013). Modelling later demonstrated that biofuel crops were likely to displace less valuable food crops, which would lead to converting forests and grasslands into farmland to satisfy the demand for food production (Searchinger et al., 2008). Other negative effects linked to the policy included increased water use (Gerbens-Leenes et al., 2009), uncertainty in food prices due to fluctuations in food production capacity, and impacts on biodiversity due to projected habitat loss (Gallagher, 2008; Suckling et al., 2021).
Types of policy-related negative consequences commonly highlighted in the literature include:
● Unilateral actions that cause unintended environmental harm to other regions (Jalilov et al., 2013; Suckling et al., 2021);
● Non-uniform frameworks that create opportunities for economic gain at the expense of the environment (Levinson and Taylor, 2008; Dechezleprêtre and Sato, 2017); and
● Limits on innovation (Gurtoo and Antony, 2007).
Managing biosecurity risks in the marine environment is complex due to the number of vectors contributing to potential introductions of nonindigenous species (NIS), in combination with ocean biogeochemical changes leading to shifts in species composition, abundance, and biomass production (Bindoff et al., 2022). The main identified pathways for the translocation of marine NIS are the uptake and release of ballast water and biofouling accumulated on the submerged surfaces of commercial ships (Bailey, 2015; Chan and Briski, 2017; Costello et al., 2022). These are particularly complex global pathways to regulate and as such are likely susceptible to unintended consequences. For example, ships using ballast water management systems that rely on sodium hypochlorite or other active substances to kill entrained organisms could discharge unacceptable residual byproducts, even after the neutralization of biocides (e.g., Ziegler et al., 2019).
Discussions regarding international regulatory solutions to manage biofouling pathways are ongoing at the International Maritime Organization (IMO), representing an opportunity to strategically manage associated risks in a holistic manner. As a parallel to ballast water management systems, the use of in-water cleaning (IWC) systems has been identified as a potential management activity for ship associated NIS. Such systems are commonly used by industry to prevent or remove various levels and forms of biofouling (from microbes found in biofilms to macrofouling, such as barnacles, tubeworms, bryozoans, and bivalves) from submerged surfaces (Scianni and Georgiades, 2019; Tamburri et al., 2021). Thus, the environmental and industry benefits associated with IWC are widely recognized. These benefits include minimizing biosecurity risks and GHG emissions associated with ship operations while reducing fuel costs associated with biofouling accumulation (NAVSEA, 2006; IMO, 2011; Schultz et al., 2011; Farkas et al., 2018; Pagoropoulos et al., 2018; Kim, 2021; GEF-UNDP-IMO, 2022). However, IWC may also lead to unintended consequences.
This policy brief aims to draw attention to the consequences related to different IWC approaches, the extent to which the negative impacts can be mitigated, and the tradeoffs that must be appreciated as part of the policy making process dedicated to biofouling management. Considering unintended consequences related to IWC prior to local, regional, or global regulatory action is in line with the concept of impact decoupling and increases the chances of avoiding pitfalls that may cause unintended harm to the environment, economy, industry, and socio-cultural values.
2 Consequences of IWC
There are several potentially significant environmental and human health consequences related to cleaning ships while they remain in water. Human health consequences typically relate to diver safety (e.g., cleaning in areas of high shipping traffic, low ship clearance, the open ocean, or ship recesses), while the most obvious environmental consequence is the release of removed organisms, including viable fragments, larvae or other propagules, and human or wildlife parasites or pathogens into the receiving water (Morrisey et al., 2013; Scianni and Georgiades, 2019; Georgiades et al., 2021).
The accelerated and pulsed release of biocides from antifouling coatings represents another well-known environmental consequence (Morrisey et al., 2013; Scianni and Georgiades, 2019; Tamburri et al., 2020; Soon et al., 2021; Shin et al., 2023). Most commercial ships use antifouling coatings with one or more biocides (e.g., cuprous oxide) to limit biofouling accumulation (Scianni et al., 2021; Mihaylova and Barnes, 2023; Thompson et al., 2023). Most of these antifouling coatings are designed to slowly release primary and co-biocides over the typical three-to-five-year in-service period while the ship is between dry dockings (Arndt et al., 2021). Although biocides will normally be released at specific rates into the ocean to ensure they meet their specified in-service period, IWC can accelerate chemical release, resulting in pulses of biocides introduced where the ship is being cleaned (e.g., coastal port, anchorage). As the consequences associated with tributyl-tin (TBT) based coatings became globally recognized, IWC became forbidden in many regions (e.g., ANZECC, 1997). The IMO ultimately banned the use of TBT through the International Convention on the Control of Harmful Antifouling Systems on Ships (IMO, 2001) that was adopted in 2001 and entered into force in 2008. As a result, IWC became more acceptable when performed under specific conditions (e.g., DOE and MPI, 2013). Additionally, Morrisey et al. (2013) modelled the environmental risk of biocide release via IWC to inform environmentally safe IWC practices. However, the authors noted the following precautions: 1) a number of assumptions were required to deliver the outputs, as measured data were not readily available; 2) the model outputs did not factor the entry of additional contaminants, including copper from land-based sources, into the receiving environment; and 3) the modeling conducted did not account for the accumulation of biocides within the sediments.
Accumulation of antifouling coating biocides and co-biocides in sediments can have long-term consequences to receiving environments (Singh and Turner, 2009; Batista-Andrade et al., 2018; Muller-Karanassos et al., 2019; Richir et al., 2021; Turner, 2022). In addition, dredging activities to maintain water depth in channels, ports, and marinas may lead to the resuspension of contaminants in the water column (Norén et al., 2020; Polrot et al., 2021). Removal of dredge spoil from these areas may lead to wider distribution of contaminants in addition to physical impacts of disposal to the receiving environment (Norén et al., 2020; Warford et al., 2022). An unintended consequence of more frequent IWC may be the need for more frequent dredging as permits are often restricted to materials with contaminant concentrations below pre-defined limits (Norén et al., 2020). Increased dredging and disposal have implications for both GHG emissions and biosecurity (i.e., movement of contaminated dredging vessels and equipment between ports and the spread of benthic NIS from enclosed areas to open ocean environments).
A pulsed release of microplastics from antifouling coatings (containing 10-90% polymers as binding agents) may also occur during IWC operations (Tamburri et al., 2022). Additionally, IWC technologies (e.g., nylon brushes) may themselves be a source of microplastics during IWC events (Tamburri et al., 2022). Several routine processes can result in microplastic releases from ship coatings, including coating application, in-service use, routine ship maintenance (e.g., submerged surface and equipment inspections and testing; replacing anodes; pipe and valve maintenance), and especially end-of-life (i.e., coating removal and disposal or ship decommissioning/scrapping) if not carried out with due diligence in terms of waste disposal (Tamburri et al., 2022). In addition to these routine inputs IWC may release a larger plume directly into the marine environment. The potential for larger plumes of microplastics is similar to the potential for larger plumes of biocides released in an accelerated fashion through the actions of the IWC system.
Some less obvious consequences of IWC include the creation of conditions conducive to NIS establishment [e.g., copper contaminated ports or marinas favoring survival of copper tolerant species (Piola and Johnston, 2006; Piola and Johnston, 2007)], premature deterioration of biocidal antifouling coatings leading to a higher likelihood of refouling and the acceleration of re-cleaning cycles (Earley et al., 2014; Oliveira and Granhag, 2020; Swain et al., 2022), and a focus on cleaning the main hull at the expense of managing all submerged surfaces, including niche areas (Growcott et al., 2019; Davidson et al., 2023). Focusing attention away from niche areas is particularly troubling, as these areas are important hotspots for the accumulation and transfer of NIS (Coutts and Dodgshun, 2007; Frey et al., 2014).
3 Existing approaches to mitigate or prevent IWC consequences
There are several approaches to limiting unintended consequences associated with IWC, somewhat decoupling the action of IWC from the negative impact as suggested by UNEP (2011). One approach is to capture, separate, and retain all the particulate and dissolved material removed during cleaning operations (Scianni and Georgiades, 2019; Tamburri et al., 2020). By capturing and retaining the removed organic and inorganic debris during treatment stages (Figure 1), the release of these materials into receiving waters can be reduced. However, the extent to which releases are minimized depends on the capture efficiency at the cleaning unit and the retention or removal efficiency during the waste treatment stage (Tamburri et al., 2020; Tamburri et al., 2021). These reductions are largely dependent on the term ‘capture’ that currently lacks a clear and broadly accepted definition in the context of IWC (see Table 1 for proposed comprehensive definition).

Figure 1 Conceptual diagram of an in-water cleaning (IWC) system with capture, including primary and secondary treatment, of the dissolved and particulate materials released from the ship’s underwater surfaces.

Table 1 Proposed comprehensive definitions of key in-water cleaning (IWC) terms that currently lack consistent meaning.
The importance of debris capture during IWC is illustrated by Tamburri et al. (2020). This comprehensive independent evaluation of a reactive IWC system recorded total copper concentrations as high as 703 (39.8 SE) µg/L following capture and primary treatment set at 5 µm. This concentration was well above the local allowable discharge limit of 100 µg/L (i.e., uncontaminated site) and vastly exceeds those set to protect contaminated sites (e.g., 3.1 µg/L) or proposed by other jurisdictions (e.g., 1.3 µg/L; see United States Environmental Protection Agency [USEPA], 2000; DAWE, 2020; Rao et al., 2021).
Secondary treatment, as part of the capture process, can also be employed to further minimize the unintended consequences of IWC. These secondary treatments may be targeted at limiting the release of viable microorganisms (e.g., ultraviolet radiation, heat, sodium hypochlorite, or other biocides; Georgiades et al., 2021) or at limiting coating biocide concentrations through use of selective media separation (e.g., organoclays to bind metals). Such secondary treatments have been effective at reducing total copper concentrations in the treated effluent to 20 µg/L (Terraphase, 2012). To the authors’ knowledge, the secondary treatment of biocides or co-biocides other than copper has yet to be studied. In the context of IWC, the term ‘treatment’ also currently lacks a clear and broadly accepted definition (see Table 1).
Proactive IWC without capture has been discussed as an alternative approach to minimize unintended consequences (e.g., Swain et al., 2022). Proactive IWC involves periodic cleaning to restrict biofouling at the biofilm/microfouling level, or in some cases to limit the development of initial biofilms (Scianni and Georgiades, 2019; Georgiades et al., 2023). Because proactive IWC does not remove established macrofouling (i.e., visible invertebrates and macroalgae), the cleaning operations can be less abrasive than reactive IWC and may, thus, result in lower concentrations of biocides and microplastics being released from antifouling coatings during cleaning. However, a paucity of independent data exists on the performance and environmental safety of proactive IWC systems. To our knowledge, the only independent test of a proactive IWC system (without capture) on a ship was conducted by ACT/MERC (2022a; Table 2), showing elevated copper concentrations of 30.04 (0.39 SD) µg/L directly above the cleaning brushes. Additional comprehensive testing of proactive IWC systems on ships is necessary to better understand unintended consequences associated with these cleaning operations.
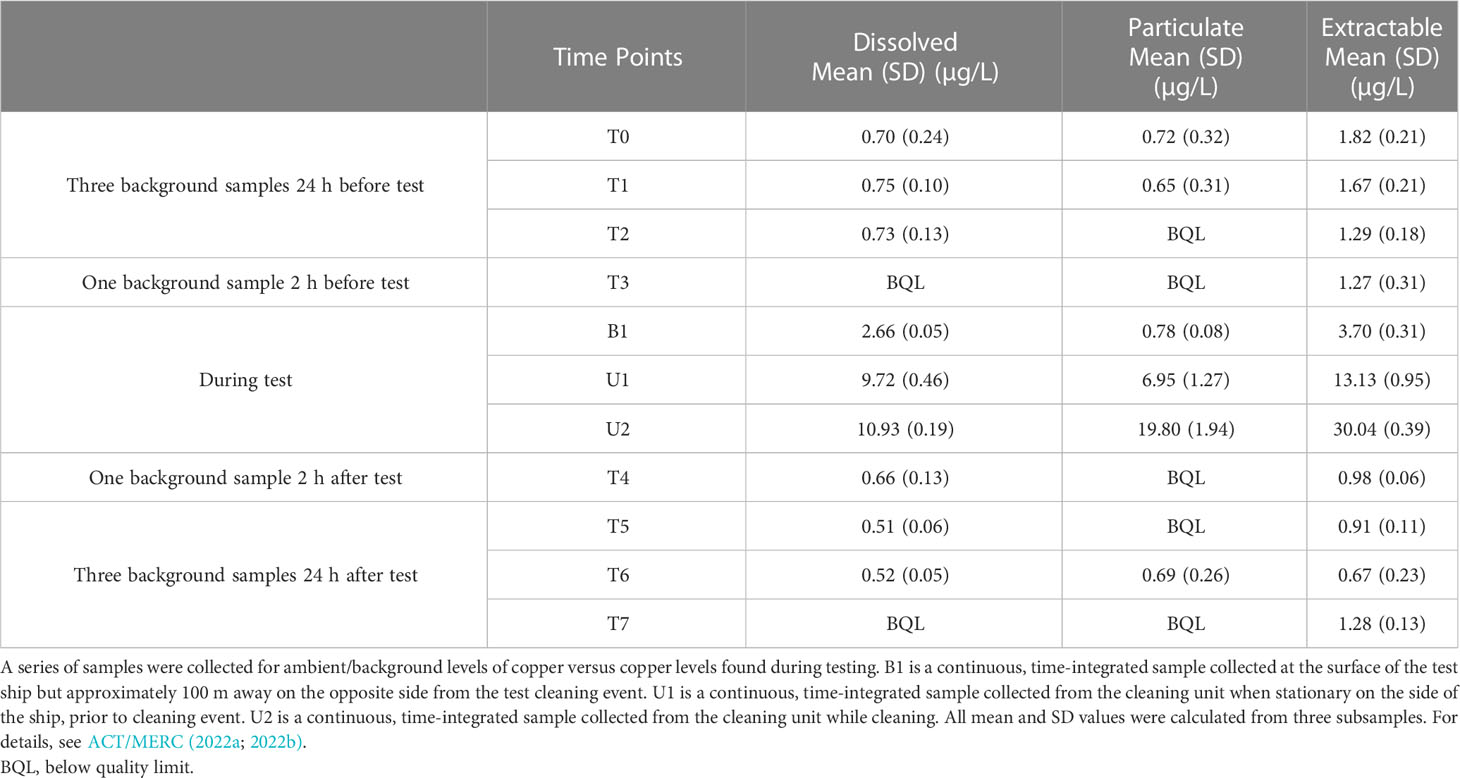
Table 2 Mean (SD) concentration of copper in dissolved, particulate, and extractable form from a proactive IWC in Baltimore, MD.
An alternative approach to avoid negative environmental consequences near coastal areas is to conduct reactive IWC offshore (e.g., prior to entry into territorial waters). Such an approach, however, has implications for diver and crew health and safety and raises questions regarding the ability to achieve cleaning/treatment efficacy of external and internally submerged areas in a non-sheltered open ocean environment. Systems capable of in-transit proactive cleaning without capture also exist, however, these may have limitations in terms of the areas of the ship that can be serviced. Further, the environmental implications of non-capture technologies require specific attention from both biosecurity and environmental contamination perspectives, particularly considering the length of larval dispersal phases of some biofouling species (e.g., Bell et al., 2011; Hewitt et al., 2011; Vinagre et al., 2020), release and long distance dispersal of biocide debris plumes (Soon et al., 2021), onshore currents, and the vicinity to other anthropogenic structures (e.g., oil rigs, wind farms, aquaculture).
Arguably, the greatest mitigation of chemical contamination due to the release of biocides would be to emphasize the use of biocide-free coatings and proactive IWC of hull surfaces (DOE and MPI, 2013; Kim, 2021). However, transitioning to biocide free technologies may result in unintended environmental consequences, such as the increased release of potentially toxic components (Piazza et al., 2018; Lagerström et al., 2022) and the need for more frequent interventions. The frequency of interventions is likely to be affected by current limitations in global capacity and the number of locations where such interventions are allowed. The discussion of whether more frequent IWC actions would result in an overall benefit to the environment is challenging at present given the limited availability of data on the effect of current systems on different types of coatings and the fact that many solutions are still in early stages of development. Therefore, a structured approach to coating selection and specification, and ensuring that any potential IWC actions are carried out with suitable equipment that will not compromise the long-term effectiveness of the coating are critical for minimizing the likelihood of biofouling accumulation over the drydocking period (Georgiades et al., 2018; Mihaylova et al., 2022).
Niche areas (relatively small subsections of ships’ submerged surfaces with complex geometries, including curves, edges, protrusions, indentations, gratings, etc.) remain a major biosecurity gap, particularly internal niches, where IWC system efficacy and capture is either untested (e.g., using the testing approach of Growcott et al., 2019) or unproven (Jones and McClary, 2021). More data are becoming available on the operational, economic, and biosecurity consequences associated with biofouling accumulated in internal niches (e.g., Pamitran et al., 2016; Ceylan et al., 2022; Davidson et al., 2023) and automated technologies are being developed and tested with biosecurity concerns in mind (e.g., Park et al., 2023). For niche areas, a combination of biocidal coatings and marine growth prevention systems (MGPS) may still offer the best chance of fouling prevention, where applicable (Lewis, 2016; Georgiades et al., 2018). However, the efficacy of MGPS has long been questioned (Lewis and Smith, 1991; Frey et al., 2014; Lewis, 2016; MPI, 2020), and thus should also be subject to independent testing.
4 Policy implications
Like most environmental protection challenges, none of the solutions discussed in this paper represent a panacea. While each option offers some level of mitigation toward the primary issue of ship biofouling, they all have one or more negative environmental tradeoffs. In most cases, the immediate solution of reducing the amount of biofouling on ship wetted surfaces comes with the associated release of organisms/propagules (including pathogens), biocides, and microplastics into the receiving environment. This situation is also representative of the difficulty of establishing environmental protection systems retroactively to existing large scale maritime processes (e.g., aquaculture; Georgiades et al., 2016) or pathways (e.g., ballast water; Čampara et al., 2019).
Some of the unintended consequences of ship IWC can be mitigated to various degrees through debris capture, with removal and/or treatment, noting that the efficacy of these systems remains largely unproven (Tamburri et al., 2020; Jones and McClary, 2021; Tamburri et al., 2021; Park et al., 2023). While these measures can further minimize, but likely not eliminate, risks, they may be technologically complex and can add time and costs to IWC operations that are already under tight time constraints and cost points (Inglis et al., 2012; Pagoropoulos et al., 2018). For example, policies that require IWC at a greater frequency can lead to issues with constricted arrival windows and trade disruptions. IWC systems offer different theoretical cleaning rates, which in service depend on factors such as environmental conditions, the type and extent of biofouling, and the size and complexity of the area. Therefore, the time required to service the submerged areas of a ship will vary, making planning challenging. There are also issues with available IWC capacity (i.e., geographic coverage), which is partially linked to existing local and regional IWC policies, restrictions, and/or bans (GEF-UNDP-IMO GloFouling Partnerships Project and GIA for Marine Biosafety, 2022). Given these constraints, as well as those associated with available technologies and capacity, there needs to be careful planning and implementation of policies that require ships to conduct frequent interventions or to use different types of coatings that may be less effective at controlling biofouling accumulation over time. If this is not the case, the unintended consequences of delays and supply chain issues may lead to additional GHG emissions and biosecurity risks.
It is becoming increasingly evident that, given the current toolbox, tradeoffs will need to be made to minimize the biosecurity risk associated with ship-borne pathways. In some cases, the tradeoffs are costs and time, as unintended releases can be reduced with current technologies if enough resources are employed. In other cases, the tradeoffs include some release of organisms, biocides, and microplastics during IWC events. This type of tradeoff is not new to the world of biofouling management. Biocidal antifouling coatings (approximately 90-95% of all biofouling management coatings used; Scianni et al., 2021; Thompson et al., 2023) are designed to release biocides over the in-service period. The great majority of those biocides will enter the marine environment during this in-service period, as the benefits are deemed to outweigh the costs [e.g., New Zealand Environmental Protection Authority (2013)]. These biocides, however, are released slowly over the in-service period everywhere a ship trades, with much of this release occurring in the open ocean where the environmental impacts are minimized to a degree. In contrast, IWC accelerates the release of biocides in large pulses in a single location, typically in sheltered, low flushing coastal environments (e.g., ports and marinas) that are under many other pollution pressures (Morrisey et al., 2013; Richir et al., 2021; Tuholske et al., 2021; Hermansson et al., 2023). Dependent on location, even reduced biocide release on a per-cleaning basis can still result in long-term impacts from cumulative inputs over time. Biocidal paints are coming under increasing scrutiny (e.g., European Commission, 2007; European Commission, 2016; New Zealand Environmental Protection Authority, 2013), with controls recently put into place by the IMO’s Marine Environmental Protection Committee to ban the use of the biocide cybutryne from 1 January 2023 (IMO, 2021).
From an ethical standpoint, developed nations should actively shepherd the development and uptake of environmentally acceptable IWC rather than create conditions for the establishment of questionable IWC practices in regions with less stringent health, safety, and environmental standards. This approach not only protects more vulnerable areas but provides an incentive for the industry to develop and use sound/proven IWC systems (Morrisey et al., 2013).
5 Conclusions and recommendations
Commercial shipping is fundamental to the global economy, but it comes with known and unknown environmental consequences, including biofouling-mediated NIS establishments and GHG emissions. While complete elimination of these risks is unrealistic, they can be considerably reduced by biofouling management processes including existing and developing technologies. While some of the unintended consequences of current IWC systems have been identified, it may be necessary to make strategic tradeoffs when setting policy.
We recommend that policymakers attempt to quantify or otherwise understand the full suite of risks (e.g., biosecurity, greenhouse gas emissions, biocides, microplastics, human health) associated with the various approaches to IWC (including prohibitions) to ensure that fully informed decisions are made. While tradeoffs may be necessary, it is important to appreciate that embracing gains now based on current technologies can reduce risks in real time. Equally important is the need to continue making progress to further reduce risks by incentivizing innovation and considering innovative approaches like proactive IWC of biocide-free coatings.
Author contributions
The authors contributed equally to the conception and authorship of the article. All authors contributed to the article and approved the submitted version.
Funding
This effort was supported, in part, by the California State Lands Commission and the U.S. Department of Transportation Maritime Administration.
Acknowledgments
The authors wish to thank Mr Alan Guy (Safinah Group) whose contribution greatly improved the clarity of the manuscript. Special thanks to the two reviewers who provided thoughtful feedback and constructive suggestions for improving the manuscript.
Conflict of interest
Author RM is employed by the company Safinah Ltd.
The remaining authors declare that the research was conducted in the absence of any commercial or financial relationships that could be construed as a potential conflict of interest.
Publisher’s note
All claims expressed in this article are solely those of the authors and do not necessarily represent those of their affiliated organizations, or those of the publisher, the editors and the reviewers. Any product that may be evaluated in this article, or claim that may be made by its manufacturer, is not guaranteed or endorsed by the publisher.
Supplementary material
The Supplementary Material for this article can be found online at: https://www.frontiersin.org/articles/10.3389/fmars.2023.1239723/full#supplementary-material
References
ACT/MERC. (2022a). Evaluation of the Jotun Hull Skating Solution: A proactive ship biofouling in-water cleaning system. Solomons, MD: ACT/MERC, TS-786-22. CBL/UMCES 2023-014.
ACT/MERC. (2022b). Guidelines for testing ship biofouling in-water cleaning systems. Solomons, MD: ACT/MERC, TS-788-22, CBL/UMCES 2023-017.
ANZECC. (1997). Code of Practice for Antifouling and In-water Hull Cleaning and Maintenance (Canberra: Australian and New Zealand Environment Conservation Council).
Arndt E., Robinson A., Hester S. (2021) Factors that Influence Vessel Biofouling and its Prevention and Management. Final report for CEBRA Project 190803. Available at: https://cebra.unimelb.edu.au/:data/assets/pdf_file/0010/3822922/Endorsed-CEBRA-190803-Final-Report.pdf (Accessed May 2023).
Bailey S. A. (2015). An overview of thirty years of research on ballast water as a vector for aquatic invasive species to freshwater and marine environments. Aquat. Ecosyst. Health Manag. 18, 261–268. doi: 10.1080/14634988.2015.1027129
Batista-Andrade J. A., Caldas S. S., Batista R. M., Castro I. B., Fillmann G., Primel E. G. (2018). From TBT to booster biocides: Levels and impacts of antifouling along coastal areas of Panama. Environ. pollut. 234, 243–252. doi: 10.1016/j.envpol.2017.11.063
Bell A., Phillips S., Denny C., Georgiades E., Kluza D. (2011) Risk Analysis: Vessel Biofouling (Wellington: Ministry of Agriculture and Forestry Biosecurity New Zealand). Available at: https://www.mpi.govt.nz/dmsdocument/2863 (Accessed May 2023).
Bindoff N. L., Cheung W. W. L., Kairo J. G., Arístegui J., Guinder V. A., Hallberg R., et al. (2022). “Changing ocean, marine ecosystems, and dependent communities,” in IPCC Special Report on the Ocean and Cryosphere in a Changing Climate. Eds. Pörtner H.-O., Roberts D. C., Masson-Delmotte V., Zhai P., Tignor M., Poloczanska E., et al (Cambridge, UK and New York, NY, USA: Cambridge University Press), 447–587. doi: 10.1017/9781009157964.007
Čampara L., Frančić V., Maglić L., Hasanspahić N. (2019). Overview and comparison of the IMO and the US Maritime Administration ballast water management regulations. J. Mar. Sci. Eng. 7 (9), 283. doi: 10.3390/jmse7090283
Ceylan B. O., Karatuğ Ç., Ejder E., Uyanık T., Arslanoğlu Y. (2022). Risk assessment of sea chest fouling on the ship machinery systems by using both FMEA method and ERS process. Aust. J. Marit. Ocean Aff., 1–20. doi: 10.1080/18366503.2022.2104494
Chan F. T., Briski E. (2017). An overview of recent research in marine biological invasions. Mar. Biol. 164, 121. doi: 10.1007/s00227-017-3155-4
Costello K. E., Lynch S. A., McAllen R., O’Riordan R. M., Culloty S. C. (2022). Assessing the potential for invasive species introductions and secondary spread using vessel movements in maritime ports. Mar. pollut. Bull. 177, 113496. doi: 10.1016/j.marpolbul.2022.113496
Coutts A. D. M., Dodgshun T. J. (2007). The nature and extent of organisms in vessel sea-chests: A protected mechanism for marine bioinvasions. Mar. pollut. Bull. 54, 875–886. doi: 10.1016/j.marpolbul.2007.03.011
Davidson I., Cahill P., Hinz A., Major R., Kluza D., Scianni C., et al. (2023). Biofouling occlusion of ships’ internal seawater systems: Operational, economic, and biosecurity consequences. Biofouling. 39 (4), 410–426. doi: 10.1080/08927014.2023.2225411
Dechezleprêtre A., Sato M. (2017). The impacts of environmental regulations on competitiveness. Rev. Environ. Econ. Policy 11 (2), 183–206. doi: 10.1093/reep/rex013
Department of Agriculture, Water, and the Environment (DAWE) (2020). Australian in-water cleaning standards: consultation draft (Canberra: Department of Agriculture, Water and the Environment).
Department of the Environment [DOE] and New Zealand Ministry for Primary Industries [MPI]. (2013). Antifouling and in-Water Cleaning Guidelines (Canberra: Department of Agriculture).
Earley P. J., Swope B. L., Barbeau K., Bundy R., McDonald J. A., Rivera-Duarte I. (2014). Life cycle contributions of copper from vessel painting and maintenance activities. Biofouling 30 (1), 51–68. doi: 10.1080/08927014.2013.841891
European Commission. (2007). 2007/565/EC: Commission Decision of 14 August 2007 concerning the non-inclusion in Annex I, IA or IB to Directive 98/8/EC of the European Parliament and of the Council concerning the placing of biocidal products on the market of certain substances to be examined under the 10-year work programme referred to in Article 16(2) thereof (notified under document number C(2007) 3846). Available at: http://data.europa.eu/eli/dec/2007/565/oj (Accessed May 2023).
European Commission. (2016). Commission implementing decision (EU) 2016/107 of 27 January 2016 Not approving Cybutryne as an existing active substance for use in biocidal products for product-type 21 (Accessed May 2023).
European Union. (2003). EU Directive on the promotion of the use of biofuels and other renewable fuels in transport (Directive 2003/30/EC). Available at: https://eur-lex.europa.eu/eli/dir/2009/28/oj (Accessed May 2023).
Farkas A., Degiuli N., Martić I. (2018). Towards the prediction of the effect of biofilm on the ship resistance using CFD. Ocean Eng. 167, 169–186. doi: 10.1016/j.oceaneng.2018.08.055
Frey M. A., Simard N., Robichaud D. D., Martin J. L., Therriault T. W. (2014). Fouling around: vessel sea-chests as a vector for the introduction and spread of aquatic invasive species. Manage. Biol. Invasions 5 (1), 21–30. doi: 10.3391/mbi.2014.5.1.02
Gallagher E. (2008). The Gallagher Review of the Indirect Effects of Biofuels Production (St Leonards-on-Sea: Renewable Fuels Agency).
GEF-UNDP-IMO GloFouling Partnerships Project and GIA for Marine Biosafety (2022). Compilation and Comparative Analysis of Existing and Emerging Regulations (London, England: Standards and Practices Related to Ships’ Biofouling Management).
GEF-UNDP-IMO GloFouling Partnerships Project and GIA for Marine Biosafety. (2022). Analysing the Impact of Marine Biofouling on the Energy Efficiency of Ships and the GHG Abatement Potential of Biofouling Management Measures. Available at: https://www.glofouling.imo.org/_files/ugd/34a7be_afd9d183df9a4526bd088007436c1079.pdf (Accessed May 2023).
Georgiades E., Fraser R., Jones B. (2016). Options to strengthen on-farm biosecurity management for commercial and non-commercial aquaculture. Technical Paper No. 2016/47. Wellington: Ministry for Primary Industries.
Georgiades E., Growcott A., Kluza D. (2018). Technical Guidance on Biofouling Management for Vessels Arriving to New Zealand (Wellington: Ministry for Primary Industries). Available at: https://mpi.govt.nz/dmsdocument/27726-technical-guidance-on-biofouling-management-for-vessels-arriving-to-new-zealand (Accessed May 2023).
Georgiades E., Scianni C., Davidson I., Tamburri M. N., First M. R., Ruiz G., et al. (2021). The role of vessel biofouling in the translocation of marine pathogens: management considerations and challenges. Front. Mar. Sci. 8. doi: 10.3389/fmars.2021.660125
Georgiades E., Scianni C., Tamburri M. N. (2023). Biofilms associated with ship submerged surfaces: Implications for ship biofouling management and the environment. Front. Mar. Sci. 10. doi: 10.3389/fmars.2023.1197366
Gerbens-Leenes P. W., Hoekstra A. Y., van der Meer T. (2009). The water footprint of energy from biomass: a quantitative assessment and consequences of an increasing share of bio-energy in energy supply. Ecol. Econ. 68, 1052–1060. doi: 10.1016/j.ecolecon.2008.07.013
Growcott A., Kluza D., Georgiades E. (2019). Technical advice: Evaluation of in-water systems to reactively treat or remove biofouling within vessel internal niche areas (Wellington: Ministry for Primary Industries). Available at: https://www.mpi.govt.nz/dmsdocument/33594-technical-advice-evaluation-of-in-water-systems-to-reactively-treat-or-remove-biofouling-within-vessel-internal-niche-areas (Accessed May 2023).
Gurtoo A., Antony S. J. (2007). “Environmental regulations: Indirect and unintended consequences on economy and business”, Management of Environmental Quality. 18 (6), 626–642. doi: 10.1108/14777830710826676
Hermansson A. L., Hassellöv I. M., Jalkanen J. P., Ytreberg E. (2023). Cumulative environmental risk assessment of metals and polycyclic aromatic hydrocarbons from ship activities in ports. Mar. pollut. Bull. 189, 114805. doi: 10.1016/j.marpolbul.2023.114805
Hewitt C. L., Campbell M. L., Coutts A. D. M., Dahlstrom A., Valentine J., Shields D. (2011) Species Biofouling Risk Assessment. The Department of Agriculture, Fisheries and Forestry (DAFF). Available at: https://www.agriculture.gov.au/sites/default/files/sitecollectiondocuments/animal-plant/pests-diseases/marine-pests/biofouling-consult/species-biofouling-risk-assessment.doc (Accessed May 2023).
Inglis G. J., Floerl O., Woods C. (2012). Scenarios of Vessel Biofouling Risk and their Management: An Evaluation of Options (Wellington: New Zealand: Ministry of Agriculture and Forestry). Available at: https://mpi.govt.nz/dmsdocument/4029-scenarios-of-vessel-biofouling-risk-and-their-management (Accessed May 2023).
International Maritime Organization. (2001). International Convention on the Control of Harmful Antifouling Systems on Ships (London: International Maritime Organization).
International Maritime Organization. (2023). Revised Guidelines for the Control and Management of SHip Biofouling to Minimize the Transfer of Invasive Aquatic Species (London: International Maritime Organization).
International Maritime Organization. (2021). Marine Environment Protection Committee (MEPC 76), 10 to 17 June 2021 (London: International Maritime Organization) (Accessed May 2023).
Jalilov S.-M., Amer S. A., Ward F. A. (2013). Water, food, and energy security: An elusive search for balance in Central Asia. Water Resour. Manage. 27 (11), 3959–3397. doi: 10.1007/s11269-013-0390-4
Jones E., McClary D. (2021). Summary – Testing of Reactive In-Water Cleaning Systems for Removal of Vessel Biofouling. Biosecurity New Zealand Technical Paper No: 2021/11 (Wellington: Biosecurity New Zealand).
Kim H. J. (2021). Strategic actions for sustainable vessel hull coatings in line with the UN SDGs. J. Adv. Mar. Eng. Technol. 45 (4), 231–242. doi: 10.5916/jamet.2021.45.4.231
Lagerström M., Wrange A. L., Oliveira D. R., Granhag L., Larsson A. I., Ytreberg E. (2022). Are silicone foul-release coatings a viable and environmentally sustainable alternative to biocidal antifouling coatings in the Baltic Sea region? Mar. pollut. Bull. 184, 114102. doi: 10.1016/j.marpolbul.2022.114102
Lange M. (2013). The GHG balance of biofuels taking into account land use change. Energy Policy 39, 2373–2385. doi: 10.1016/j.enpol.2011.01.057
Levinson A., Taylor M. (2008). Unmasking the pollution haven effect. Int. Econ. Rev. 49 (1), 223–254. doi: 10.1111/j.1468-2354.2008.00478.x
Lewis J. A. (2016) Assessment of Preventative Biofouling Management Measures. Available at: http://mpi.govt.nz/document-vault/14530 (Accessed May 2023).
Lewis J. A., Smith B. S. (1991). “Hydroides settlement in Sydney Harbour (Australia) and its control in sea-water cooling systems,” in Biodeterioration and Biodegradation 8. Ed. Rossmoore H. W. (London: Elsevier Applied Science), 464–466.
Mihaylova R., Barnes C. (2023). The importance of hull coating specifications. J. Prot. Coat. Linings 40 (4), 17–21.
Mihaylova R., Barnes C., Porsbjerg M. (2022). Energy Efficiency: Data-driven Approaches to Hull Coating Specification and Selection. Scaling Decarbonisation Solutions: Reducing Emissions by 2030 (Rotterdam, The Netherlands: Royal Institution of Naval Architects).
Ministry for Primary Industries (2020) Request for Tender 406291 - Evaluating Efficacy of Marine Growth Prevention Systems. Available at: https://www.gets.govt.nz/MPI/ExternalTenderDetails.htm?id=23737120 (Accessed May 2023).
Morrisey D., Gadd J., Page M., Lewis J., Bell A., Georgiades E. (2013) In-Water Cleaning of Vessels: Biosecurity and Chemical Contamination Risks. New Zealand Ministry for Primary Industries Technical Paper No. 2013/11 (Wellington: Ministry for Primary Industries). Available at: https://www.mpi.govt.nz/dmsdocument/4092-in-water-cleaning-of-vessels-biosecurity-and-chemical-contamination-risks (Accessed May 2023).
Muller-Karanassos C., Turner A., Arundel W., Vance T., Lindeque P. K., Cole M. (2019). Antifouling paint particles in intertidal estuarine sediments from southwest England and their ingestion by the harbour ragworm, Hediste diversicolor. Environ. pollut. 249, 163–170. doi: 10.1016/j.envpol.2019.03.009
Naval Sea Systems Command [NAVSEA]. (2006). Naval Ships’ Technical Manual Chapter 081. Waterborne Underwater Hull Cleaning of Navy Ships, Revision 5 (Washington, DC: Naval Sea Systems Command).
New Zealand Environmental Protection Authority (2013). Evaluation and review report for the reassessment of antifouling paints (APP201051). Wellington: New Zealand Environmental Protection Authority).
Norén A., Fedje K. K., Strömvall A. M., Rauch S., Andersson-Sköld Y. (2020). Integrated assessment of management strategies for metal-contaminated dredged sediments–What are the best approaches for ports, marinas, and waterways? Sci. Total Environ. 716, 135510. doi: 10.1016/j.scitotenv.2019.135510
Oliveira D. R., Granhag L. (2020). Ship hull in-water cleaning and its effects on fouling-control coatings. Biofouling 36 (3), 332–350. doi: 10.1080/08927014.2020.1762079
Pagoropoulos A., Kjaer L. L., Dong Y., Birkved M., McAloone T. C. (2018). Economic and environmental impact trade-offs related to in-water hull cleanings of merchant vessels. J. Ind. Ecol. 22 (4), 916–929. doi: 10.1111/jiec.12627
Pamitran A. S., Adam S. A., Alhamid M. I. (2016). Cost estimation study for the effect of biofouling on engine cooling system performance with an 8000 BHP vessel. Appl. Mech. Mater. 819, 427–431. doi: 10.4028/www.scientific.net/AMM.819.427
Park D., Han J. B., Yeu T., Cho S. G., Kim S., Kim H., et al. (2023). Development of an autonomous cleaning robot with a hydraulic manipulator arm for the cleaning of niche areas of a ship hull. J. Mar. Sci. Eng. 11 (5), 973. doi: 10.3390/jmse11050973
Piazza V., Gambardella C., Garaventa F., Massanisso P., Chiavarini S., FaiMali M. (2018). A new approach to testing potential leaching toxicity of fouling release coatings (FRCs). Mar. Environ. Res. 141, 305–312. doi: 10.1016/j.marenvres.2018.09.024
Piola R. F., Johnston E. L. (2006). Differential tolerance of metals among populations of the introduced bryozoan Bugula neritina. Mar. Biol. 148, 997–1010. doi: 10.1007/s00227-005-0156-5
Piola R. F., Johnston E. L. (2007). Pollution reduces native diversity and increases invader dominance in marine hard-substrate communities. Diversity Distrib. 14 (2), 329–342. doi: 10.1111/j.1472-4642.2007.00430.x
Polrot A., Kirby J. R., Birkett J. W., Sharples G. P. (2021). Combining sediment management and bioremediation in muddy ports and harbours: A review. Environ. pollut. 289, 117853. doi: 10.1016/j.envpol.2021.117853
Rao A. S., Björklund K., LeNoble J., Lilley. P. (2021) Water Quality Assessment and Proposed Objectives for Burrard Inlet: Copper Technical Report. Prepared for Tsleil-Waututh Nation and the Province of B.C. Available at: https://www2.gov.bc.ca/assets/gov/environment/air-land-water/water/waterquality/water-quality-objectives/burrard_inlet_water_quality_objectives_copper_aug_11_2021.pdf (Accessed May 2023).
Richir J., Bray S., McAleese T., Watson G. J. (2021). Three decades of trace element sediment contamination: the mining of governmental databases and the need to address hidden sources for clean and healthy seas. Environ. Int. 149, 106362. doi: 10.1016/j.envint.2020.106362
Schultz M. P., Bendick J. A., Holm E. R., Hertel W. M. (2011). Economic impact of biofouling on a naval surface ship. Biofouling 27, 87–98. doi: 10.1080/08927014.2010.542809
Scianni C., Georgiades E. (2019). Vessel in-water cleaning or treatment: Identification of environmental risks and science needs for evidence-based decision making. Front. Mar. Sci. 6, 467. doi: 10.3389/fmars.2019.00467
Scianni C., Lubarsky K., Ceballos-Osuna L., Bates T. (2021). Yes, we CANZ: Initial compliance and lessons learned from regulating vessel biofouling management in California and New Zealand. Manage. Biol. Invasions 12, 727–746. doi: 10.3391/mbi.2021.12.3.14
Searchinger T., Heimlich R., Houghton R. A., Dong F., Elobeid A., Fabiosa J., et al. (2008). Use of U.S. croplands for biofuels increases greenhouse gases through emissions from land-use change. Science 319, 1238–1240. doi: 10.1126/science.1151861
Shin D., Choi Y., Soon Z. Y., Kim M., Jang M. C., Seo J. Y., et al. (2023). Chemical hazard of robotic hull in-water cleaning discharge on coastal embryonic fish. Ecotoxicol. Environ. Saf. 253, 114653. doi: 10.1016/j.ecoenv.2023.114653
Singh N., Turner A. (2009). Leaching of copper and zinc from spent antifouling paint particles. Environ. pollut. 157 (2), 371–376. doi: 10.1016/j.envpol.2008.10.003
Soon Z. Y., Jung J. H., Loh A., Yoon C., Shin D., Kim M. (2021). Seawater contamination associated with in-water cleaning of ship hulls and the potential risk to the marine environment. Mar. pollut. Bull. 171, 112694. doi: 10.1016/j.marpolbul.2021.112694
Suckling J., Hoolohan C., Soutar I., Druckman A. (2021). Unintended consequences: Unknowable and unavoidable, or knowable and unforgivable? Front. Climate 124. doi: 10.3389/fclim.2021.737929
Swain G., Erdogan C., Foy L., Gardner H., Harper M., Hearin J., et al. (2022). Proactive in-water ship hull grooming as a method to reduce the environmental footprint of ships. Front. Mar. Sci 8, 808549. doi: 10.3389/fmars.2021.808549
Tamburri M. N., Davidson I. C., First M. R., Scianni C., Newcomer K., Inglis G. J., et al. (2020). In-water cleaning and capture to remove ship biofouling: An initial evaluation of efficacy and environmental safety. Front. Mar. Sci. 7, 437. doi: 10.3389/fmars.2020.00437
Tamburri M. N., Georgiades E. T., Scianni C., First M. R., Ruiz G. M., Junemann C. E. (2021). Technical considerations for development of policy and approvals for in-water cleaning of ship biofouling. Front. Mar. Sci. 8, 804766. doi: 10.3389/fmars.2021.804766
Tamburri M. N., Soon Z. Y., Scianni C., Øpstad C. L., Oxtoby N. S., Doran S., et al. (2022). Understanding the potential release of microplastics from coatings used on commercial ships. Front. Mar. Sci. 9, 1074654. doi: 10.3389/fmars.2022.1074654
Terraphase Engineering Inc. (2012). In-Water Hull Cleaning Summary Report (Alameda, CA: US-DOT – Maritime Administration).
Thompson J., Ceballos-Osuna L., Ko T., Mackay J., Nedelcheva R., Scianni C. (2023). 2023 Biennial report on the California Marine Invasive Species Program. Produced for the California Legislature. Available at: https://slcprdwordpressstorage.blob.core.windows.net/wordpressdata/2022/12/MISPBiennial-2023.pdf (Accessed May 2023).
Tuholske C., Halpern B. S., Blasco G., Villasenor J. C., Frazier M., Caylor K. (2021). Mapping global inputs and impacts from of human sewage in coastal ecosystems. PloS One 16 (11), e0258898. doi: 10.1371/journal.pone.0258898
Turner A. (2022). Metal contamination of intertidal sediment and macroalgae in an area impacted by paint from abandoned boats. Mar. pollut. Bull. 182, 113958. doi: 10.1016/j.marpolbul.2022.113958
United Nations Environment Programme [UNEP]. (2011). Decoupling natural resource use and environmental impacts from economic growth. Available at: https://wedocs.unep.org/20.500.11822/9816 (Accessed May 2023).
United States Environmental Protection Agency [USEPA]. (2000). California Toxics Rule. Title 40, Code of Federal Regulations Section 131.38. Available at: https://www.ecfr.gov/current/title-40/chapter-I/subchapter-D/part-131/subpart-D/section-131.38 (Accessed May 2023).
Vinagre P. A., Simas T., Cruz E., Pinori E., Svenson J. (2020). Marine biofouling: A European database for the marine renewable energy sector. J. Mar. Sci. Eng. 8 (7), 495. doi: 10.3390/jmse8070495
Warford L., Mason C., Lonsdale J., Bersuder P., Blake S., Evans N., et al. (2022). A reassessment of TBT action levels for determining the fate of dredged sediments in the United Kingdom. Mar. pollut. Bull. 176, 113439. doi: 10.1016/j.marpolbul.2022.113439
Ziegler G., Gonsior M., Fisher D. J., Schmitt-Kopplin P., Tamburri M. N. (2019). Formation of brominated organic compounds and molecular transformations in dissolved organic matter (DOM) after ballast water treatment with sodium dichloroisocyanurate dihydrate (DICD). Environ. Sci. Technol. 53 (14), 8006–8016. doi: 10.1021/acs.est.9b01064
Keywords: ship biofouling, in-water cleaning, shipping, environmental risks, biosecurity, impact decoupling
Citation: Scianni C, Georgiades E, Mihaylova R and Tamburri MN (2023) Balancing the consequences of in-water cleaning of biofouling to improve ship efficiency and reduce biosecurity risk. Front. Mar. Sci. 10:1239723. doi: 10.3389/fmars.2023.1239723
Received: 13 June 2023; Accepted: 21 July 2023;
Published: 11 August 2023.
Edited by:
Cathryn Murray, Fisheries and Oceans Canada (DFO), CanadaReviewed by:
Noa Shenkar, Tel Aviv University, IsraelEmily Ralston, Florida Institute of Technology, United States
Copyright © 2023 Scianni, Georgiades, Mihaylova and Tamburri. This is an open-access article distributed under the terms of the Creative Commons Attribution License (CC BY). The use, distribution or reproduction in other forums is permitted, provided the original author(s) and the copyright owner(s) are credited and that the original publication in this journal is cited, in accordance with accepted academic practice. No use, distribution or reproduction is permitted which does not comply with these terms.
*Correspondence: Chris Scianni, Chris.Scianni@slc.ca.gov