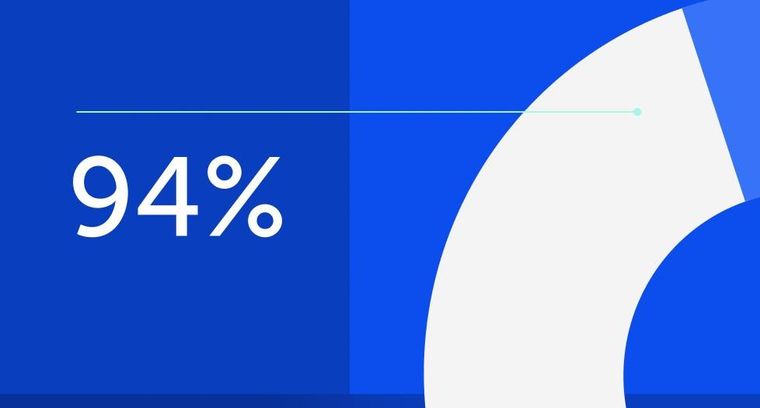
94% of researchers rate our articles as excellent or good
Learn more about the work of our research integrity team to safeguard the quality of each article we publish.
Find out more
ORIGINAL RESEARCH article
Front. Mar. Sci., 20 November 2023
Sec. Coral Reef Research
Volume 10 - 2023 | https://doi.org/10.3389/fmars.2023.1236164
Global warming of the oceans poses a double threat to benthic organisms as they reach their thermal limits and starve to death due to declines in plankton abundance during heat waves. Under these circumstances, dissolved nutrients become an important alternative food source, especially in temperate regions where they can reach high concentrations. Although octocorals play a central role in temperate Marine Animal Forests and benthic-pelagic coupling, our knowledge of their feeding ecology is still limited. We used 13C and 15N isotope labeling to investigate feeding rates on dissolved inorganic (carbon, ammonium, nitrate) and organic (urea, amino acids) nutrients of the two most common Mediterranean gorgonians, the mixotrophic species Eunicella singularis and the heterotrophic species Paramuricea clavata. We also measured the uptake of natural dissolved organic matter (DOM) provided at different concentrations. Measurements were conducted at 17°C and 24°C to anticipate the effects of ocean warming. Our findings indicate that gorgonians exhibit notably low uptake rates of dissolved inorganic and organic nutrients, both at 17°C and 24°C. At 24°C, gorgonians experienced heat stress, as evidenced by elevated respiration rates, the loss of symbionts in E. singularis, and decreased lipid reserves in P. clavata. However, we did not observe a significant increase in the uptake rates of dissolved inorganic or organic nutrients, except for dissolved organic carbon in E. singularis. This study provides valuable insight into the nutritional requirements of temperate octocorals. The results demonstrate that dissolved nutrients, in particular nitrogen, do not play a substantial role in the diet of gorgonians, suggesting that these organisms primarily rely on the capture of plankton and detrital material for their nitrogen requirements. Therefore, temperate octocorals may be at high risk of severe starvation in the future.
Carbon (C) and nitrogen (N) elements are essential to any biological system, as they enter into the composition of important cellular components such as proteins, genetic material or enzymes. In various living organisms, their metabolisms are therefore closely linked, and an excess or shortage of one element strongly affects the metabolism of the other (Zhang et al., 2018). In the oceans, N is often a limiting nutrient for animal and plant growth (Howarth, 1988). Therefore, the ability of organisms to efficiently use the various available N sources also affects their distribution and abundance in a given environment (Klais et al., 2017). To cope with N limitation, many benthic marine organisms form holobionts (sensus Rohwer et al., 2002), in which the animal host lives in symbiosis with a variety of microorganisms, including protists, fungi, bacteria, archaea, and viruses (Knowlton and Rohwer, 2003). Each partner makes a specific contribution to the N requirements of the holobiont. The animal host feeds heterotrophically on particulate and dissolved organic nitrogen (PON and DON respectively), found in plankton, or in the form of dissolved free amino acids and urea (Grover et al., 2006; Rix et al., 2020). Microbial symbionts such as diazotrophs can provide N to the holobiont through N fixation (Bednarz et al., 2012). Other microbes, such as photosynthetic dinoflagellates from the Symbiodininiaceae family fix dissolved inorganic carbon (DIC) and nitrogen (DIN; ammonium, nitrate) to produce organic compounds that they share with the host to meet its nutrient needs (Rädecker et al., 2015; Freeman et al., 2016). However, the contribution of each partner to the N requirements of the holobiont is species-specific and varies greatly depending on dissolved N concentrations, seawater temperature or other environmental conditions (Baker et al., 2013; Pupier et al., 2021).
Corals are one of the most widespread holobionts in coastal benthic ecosystems. They can form large marine animal forests (MAFs, sensu Rossi et al., 2017), such as coral reefs and coralligenous assemblages, which are probably among the most widespread ecosystems on Earth, and provide services of great economic value to hundreds of millions of people worldwide (Rossi and Rizzo, 2020). Nitrogen availability is a key factor in coral functioning and health, as it determines their ability to grow, reproduce and survive (Rädecker et al., 2015). However, the acquisition and allocation of N in coral holobionts is not well understood, especially in octocorals (Baker et al., 2011; Pupier et al., 2019a; Pupier et al., 2021), despite the fact that octocorals play an important structural and functional role in MAFs, and are key players in the benthic-pelagic energy fluxes through the uptake and release of nutrients (Gili and Coma, 1998; Schubert et al., 2016; Rossi and Rizzo, 2021). Coral feeding ecology has indeed mainly focused on the Hexacorallia subclass, which consists of stony corals (76% of studies; Santos et al., 2020), while only 22% of studies were dedicated to Octocorallia. Moreover, half of the studies were related to feeding behaviour (Santos et al., 2020), so the uptake rates and assimilation of nutrients such as dissolved C and N by Octocorallia are still very poorly understood (Baker et al., 2015; Pupier et al., 2019b; Pupier et al., 2021).
In temperate coastal waters of the Mediterranean Sea, gorgonian corals can reach very high densities in both shallow and deep waters (Gori et al., 2011a; Gori et al., 2011b), and contribute greatly to the biological and economic value of the ecosystems they form. Gorgonians (with an average size of 20-40 cm height) can indeed reach densities from 20 to more than 60 colonies m-2 in some locations (Sini et al., 2015; Grinyó et al., 2016). Our understanding of their ecology, distribution patterns or reproductive strategies has increased in recent decades (Schubert et al., 2016). However, as with all Octocorallia, much less is known about their feeding strategies for dissolved organic and inorganic C and N. This lack of knowledge becomes particularly concerning in the face of climate change, which poses a serious threat to the survival of these octocorals inhabiting shallow waters (Garrabou et al., 2022). Indeed, the Mediterranean Sea has been suffering from repeated heat waves for the last decades, leading to mass mortality of gorgonians that thrive in shallow waters (Garrabou et al., 2022). In the summer of 2022, which was one of the hottest ever observed in this region, seawater temperature rose above 25°C at depths of 20-30 m, where most gorgonians thrive, reaching threshold for these organisms (Pey et al., 2013; Tignat-Perrier et al., 2022).
To fill the knowledge gaps on N requirements of temperate octocorals both under normal growth conditions and heat stress, the uptake rates of DIN and DON were measured in the two most abundant gorgonian species of the western Mediterranean: the only symbiotic species Eunicella singularis (Esper, 1794), and the non-symbiotic species Paramuricea clavata (Risso, 1826) (Weinberg, 1976). In parallel, measurements of DIC and DOC uptake rates were also carried out, to link the uptake rates of C and N. Measurements were performed under normal growth temperature (17°C) and under heat stress (24°C) (Garrabou et al., 2022). To achieve our objectives, we used the techniques of stable isotopes tracers of N and C (15N and 13C respectively), as well as an approach based on the measurement of nutrient depletion in seawater. Our study addresses several questions. First, to what extent Mediterranean gorgonians rely on dissolved nutrients? Second, how does the existence of a symbiosis with photosynthetic dinoflagellates influence the nutrition in temperate gorgonians? Third, how do their nutritional capacities change with heat stress? We hypothesized that (1) both species can assimilate dissolved C and N; (2) the symbiotic relationship in E. singularis allows for greater dissolved N uptake through the uptake of DIN. Finally, we hypothesized that (3) heat stress had a different effect on the symbiotic than on the non-symbiotic gorgonian. By assessing how gorgonians take up dissolved organic and inorganic nutrients in different seawater temperature conditions, this comparative study will provide a better understanding of the nutritional factors, which regulate the distribution of symbiotic versus non-symbiotic gorgonians in the Mediterranean Sea. In addition, understanding the nutritional requirements of these gorgonians can help inform management practices aimed at improving their health and resilience, particularly in the face of stressors such as climate change and ocean acidification.
Experiments were carried out with two Mediterranean gorgonians: the symbiotic species Eunicella singularis (Esper, 1794), which is mixotroph, and the asymbiotic species Paramuricea clavata (Risso, 1826), which is only heterotroph. Ten colonies of each species were collected in May 2022 in Marseille, France at 30 meters depth by SCUBA diving and transported directly to the aquarium facilities of the laboratory where experiments were performed. For each species, a total of 100 nubbins (8 cm) were generated (10 nubbins per colony) and evenly distributed among ten 20-L aquaria for acclimation (10 nubbins from different colonies per aquarium). The aquaria were continuously supplied with Mediterranean seawater, at a flow rate of 20 L h-1. Seawater temperature was kept constant at 17 ± 0.1°C using heaters (Visi-Therm VTX 300 W, Aquarium Systems, France) connected to temperature controllers (Ponsel, France). The gorgonians received a photosynthetically active radiation (PAR) of 120 µmol photons m-2 s-1 (on a 12:12 photoperiod) provided by HQI lamps (Tiger 230V/50Hz 250 W, Faeber, Italy). The chosen PAR corresponded to the summer average irradiance received by the gorgonians in their natural environment (Gori et al., 2012). During the acclimation phase (3 weeks), nubbins were fed a mixture of live Artemia salina nauplii and frozen plankton five times per week. After this acclimation phase, half of the aquaria were subjected to heat stress, with temperature increasing at a rate of 0.3°C per day to reach 24°C in 3 weeks, while the other aquaria remained at 17°C as controls. Therefore, each gorgonian colony had nubbins exposed to both temperatures. Feeding was then stopped in order to recreate natural summer conditions, during which the thermocline depletes the upper water column with nutrients and particulate food.
Measurements started two weeks after the temperature of 24°C was reached in the aquaria, when visible signs of heat stress (tissue loss) could be observed in few nubbins of P. clavata (n=2). These nubbins were discarded from measurements, which were therefore performed on the remaining ones. Six nubbins per species and temperature, coming from 6 different colonies and different aquaria, were directly frozen at -20°C for protein and lipid content measurements. The other nubbins were used to assess their feeding strategy on C and N as described in detail in the following chapters. For C, two sources were considered: i) the dissolved organic carbon (DOC) acquired heterotrophically through the uptake of natural dissolved organic matter (DOM), and ii) the dissolved inorganic carbon (DIC) acquired through photosynthesis. For N, several sources were considered: i) dissolved organic nitrogen (DON) acquired from a natural DOM mixture or specifically from urea and dissolved free amino acids (DFAAs), and ii) dissolved inorganic nitrogen (DIN) acquired specifically from ammonium and nitrate.
Measurements of net photosynthesis (Pn) for E. singularis and respiration rates (R) for both species were performed in the dark and at 120 µmol photons m-2 s-1, respectively, on 6 nubbins per species and temperature, from 6 different colonies and aquaria. Nubbins were individually placed in 50 mL transparent Plexiglas chambers, hermetically sealed and filled with 0.45-µm filtered seawater (FSW). Seawater mixing was ensured with magnetic stirrers. The chambers were equipped with a Unisense optode (oxygen-sensitive mini sensor) connected to the Oxy-4 software (Chanel fiber-optic oxygen-meter, Presens®, Regensburg, Germany). The optodes were calibrated before each measurement using nitrogen-saturated and air-saturated seawater for 0 and 100% oxygen, respectively. Pn and R were then estimated using a regression of the oxygen data against time, and gross photosynthesis (Pg) was calculated as the sum of the absolute values of R and Pn. Finally, we assumed a mole-to-mole relationship from CO2 consumed to O2 produced during photosynthesis, and inversely from O2 consumed to CO2 produced during respiration. For E. singularis, the contribution of photosynthesis to the respiratory needs of the holobiont (P:R) was determined as P:R=(Pg×12)/(R×24). We considered 12 h of C acquisition from photosynthesis and 24 h of C consumed through the respiration. Samples were then frozen at -20°C for the latter determination of their chlorophyll content and symbiont density (E. singularis), and ash-free dry weight (AFDW; both species) as described below.
To evaluate the acquisition of DOC and DON, a new set of incubations was carried out with the two species and for each temperature conditions (17°C and 24°C), using 3 different concentrations of DOM: ambient seawater DOM concentration (80 µmol C L-1 + 10 µmol N L-1, [DOMnat]); a medium DOM enrichment (3 x [DOMnat]); and a high DOM enrichment (6 x [DOMnat]). Each combination of temperature and DOM concentration was performed at 120 µmol photons-1 m-2 s-1. DOM used for the enrichment was obtained from frozen microplankton (Ocean nutrition™, Switzerland) homogenized at 20,500 rpm, sonicated and centrifuged. The supernatant was then filtered through a 0.20-µm filter before being stored at -20°C.
At the beginning of the incubation, nubbins were individually transferred into 400 mL beakers placed beforehand in the aquaria. This prevented gorgonians to stress by being in contact with air. The beakers were then removed from the aquaria and put on a stirring plate with a magnetic stirrer at the correct temperature and light conditions. Nubbins were incubated for 7 h at the three DOM concentrations. In addition, each series of incubations included three control beakers (without nubbins), whose average DOM fluxes was subtracted from the fluxes calculated in the other beakers, to account for changes related to microbial activity and C and N exchanges at the air-water interface.
At the beginning and end of the incubation, 10 mL seawater samples were collected in duplicate, filtered on a 0.45-µm sterile syringe filter and stored at -20°C to determine the DIN concentrations in the beakers using a Xenius spectrophotometer (SAFAS®, Monaco) (Table S1; full description in the Supplementary material). In addition, 30 mL of seawater were collected in duplicate using a sterile syringe, then transferred to a pre-burnt (4 h, 480°C) Pyrex tube, and stored at -20°C for the determination of DOC and dissolved nitrogen (DN) concentrations using a TOC-L analyzer (Shimadzu, Japan) (full description in the Supplementary Material). At the end of the incubations, nubbins were frozen and stored at -20°C for data normalization.
DON concentrations were calculated from the difference between DN and DIN concentrations. DOC and DON fluxes were then assessed through the difference in DOC and DON concentrations measured at the beginning and end of the incubation, and normalized to the AFDW of each nubbin. Negative fluxes indicated a net uptake of DOC or/and DON, whereas positive fluxes indicated a net release by the gorgonians. Finally, the contribution of DOC heterotrophy to the animal respiration (CHARDOC, %), was calculated according to:
where R is the respiration rate previously calculated (see 2.2).
15N stable isotope tracers were used to assess the uptake rates of the different DIN (ammonium and nitrate, NH4+ and NO3- respectively) and DON (urea, DFAAs) sources by gorgonians, according to Grover et al (2002; 2003). Therefore, 15NH4Cl, Na15NO3, 15N-urea (all 98 atomic %15N, Sigma-Aldrich, USA) or 15N-DFAAs (98 atomic %15N, Algal Amino Acid mixture, CortecNet, France) were used at a final concentration of 2 µM (note that since the urea molecule is composed of two N atoms, the enrichment concentration of N-urea is 4 µM). This concentration was chosen as it is in the same order of magnitude as the in-situ concentrations measured in the Mediterranean Sea (Doval et al., 1999; Ribes et al., 1999b; Reinthaler et al., 2008; Segura-Noguera et al., 2016). In addition, 13C-bicarbonate (NaH13CO3) tracer was also used to estimate the rates at which DIC was fixed by E. singularis and P. clavata nubbins. For this purpose, 0.5 mM of NaH13CO3 (98 atomic %13C, Sigma-Aldrich, USA) was added to the beakers enriched with 15NH4+ or 15NO3-.
For each N source, 6 nubbins per species and temperature, coming from 6 different colonies, were incubated under magnetic stirring for 7 h in individual beakers placed in a thermostatic bath and containing 220 mL of 0.45-µm FSW enriched with the 15N and 13C tracers. Incubations were carried out at 17°C and 24°C, and at 120 µmol photons m-2 s-1. At the end of the incubation, nubbins were rinsed in non-enriched FSW and frozen at -20°C until further analysis. To determine the natural isotopic abundance of 15N and 13C of the gorgonians, three control nubbins of each species were incubated in 220 mL of 0.45-µm FSW in the same conditions.
In order to distinguish uptake of 15N by the gorgonian host from uptake by the mucus-associated microbes, an additional experiment was performed at the control temperature. Ten nubbins of each species were incubated for 10 h in individual beakers containing 200 mL of 0.45-µm FSW under magnetic stirring, to let them release mucus. Three control beakers filled with 0.45-µm FSW without gorgonians were also added. After 10 h, nubbins were removed from the beakers leaving mucus behind. Four beakers per species were used to measure the natural isotopic abundance of the mucus. The remaining 6 beakers per species, as well as the 3 control beakers, were enriched with 15NH4Cl to a final concentration of 2 µM and incubated for 7 h. The mucus from each beaker, as well as the seawater in control beakers, were then filtered onto glass microfiber filters grade GF/F (Whatman®, United Kingdom) pre-burned for 4 h at 480°C. Filters were frozen at -20°C before being freeze-dried. The control beakers were used to estimate if some 15N was taken up by the few particles which could have remained in the filtered seawater. The nubbins used were also frozen and stored at -20°C for data normalization.
The surface area of all gorgonian samples was first determined using a caliper to measure the length and width of each gorgonian nubbin and considering that each nubbin was a cylinder. The samples were then freeze-dried, weighted to determine their dry weight (DW), and the tissue was separated from the axial skeleton with a scalpel. The tissue was then ground to a powder in a mortar, a fraction was taken for the AFDW determination according to Pupier et al. (2018), while the remaining powder was homogenized with 10 mL of Milli-Q water and centrifuged at 20 x g for 1 minute (adapted from Shirur et al., 2014). This gentle centrifugation allowed the separation of the soft tissue from the sclerites, which remained in the pellet. For P. clavata nubbins, the supernatant, considered as the host fraction, was immediately collected and freeze-dried for the corresponding analysis. For E. singularis nubbins, the supernatant was either directly freeze-dried (for protein and lipid content measurement) or was centrifuged at 1750 x g for 2 min to separate the soft tissue (supernatant; host fraction) from the symbionts (pellet; washed twice with Milli-Q water) for the other measurements. Both fractions were then freeze-dried, and different measurements were performed according to the samples considered.
Symbiont density and total chlorophyll content of E. singularis were measured from nubbins used for the measurements of photosynthesis and respiration. For this purpose, from each nubbin, symbiont pellets were resuspended in 6 mL FSW. A subsample of 500 µL was used for the determination of symbiont density by microscope counting via 8 replicate haemocytometer counts (Neubauer haemocytometer, Marienfeld, Germany). Another subsample of 5 mL was taken for the determination of the total chlorophyll (a + c2) quantification. The subsample was centrifuged at 8,000 x g for 10 min at 4°C. The supernatant was removed, and the pellet was washed twice with Milli-Q water, resuspended into 5 mL of acetone (>99%, Sigma-Alrich, USA) and stored at 4°C for 24 h. After a centrifugation at 11,000 x g for 15 min at 4°C, absorbances at 616 nm and 663 nm were measured using a Xenius spectrophotometer (SAFAS®, Monaco) and the total chlorophyll content was assessed according to Jeffrey and Humphrey (1975).
Measurements of protein and lipid content were carried out on 6 additional nubbins per species and temperature. After elimination of the spicules, 5 mg of freeze-dried tissue was resuspended in 200 µL of NaOH 1M and incubated in a dry bath for 30 min at 90°C. Protein content was then quantified using a BC assay kit (Interchim, France) (Smith et al., 1985) and protein standards (from 0 to 2000 µg mL-1) were prepared using bovine serum albumin (Interchim, France). The absorbance was measured at 562 nm by a Xenius spectrophotometer (SAFAS®, Monaco).
For total lipid content determination, 10 mg of freeze-dried tissue was added to 1.5 mL of chloroform/methanol (2v:1v) in a Pyrex tube and put on an orbital shaker (400 rpm) for 20 min. After a 5 min centrifugation at 1050 x g at 4°C, 150 µL of the supernatant were sampled and placed on a quartz microplate. Lipid standards (from 0 to 160 µg) were prepared using a cholesterol stock solution. Then lipid content was assessed according to Cheng et al. (2011). Briefly, the microplate was incubated for 10 min in a dry bath at 90°C for solvent evaporation, before adding 100 µL of sulfuric acid and incubating for 20 min at 90°C. The background absorbance at 540 nm was then measured using a Xenius spectrophotometer (SAFAS®, Monaco). Then, 50 µL of vanillin-phosphoric acid reagent was added for color developing and the absorbance at 540 nm was measured again. Finally, the background absorbance was subtracted from the second measured absorbance to avoid bias from the color developed by sulfuric acid.
These data were acquired from nubbins used in the 13C and 15N incubations. For this purpose, tin capsules containing approximately 600 µg of freeze-dried material, were prepared and analyzed using an isotopic ratio mass spectrometer (IRMS Delta Plus, ThermoFisher Scientific, Bremen, Germany) coupled to a C/N analyzer (Flash EA, ThermoFisher Scientific). Phosphorus (P) content in symbionts and host fractions was also determined using a Xenius spectrophotometer (SAFAS®, Monaco) (see detailed protocol in Supplementary Material). For the experiment regarding 15N enrichment of the mucus, the whole filter was analyzed in a tin capsule using an Integra II mass spectrometer (Sercon®, United Kingdom).Uptake rates of each form of DIN (N-NH4+, N-NO3-) and DON (N-urea and N-DFAAs) were calculated according to Grover et al (2002; 2003). (see Table 1 for details):
The same formulas were adapted and used for the uptake rates of 13C – DIC (see Supplementary material 1.2.2, Table S6).
Analyses were performed using R software (R Foundation for Statistical Computing, version 4.0.2). All data were expressed as mean ± standard deviation. A preliminary identification of outliers was performed by Grubb’s test, and values were excluded if the p-value was significant. The assumptions of normality and homoscedasticity of variances were verified by Shapiro’s and Bartlett’s test as well as graphical analysis of the residuals. When they were satisfied, a two-way analysis of variance (ANOVA) was performed to test the effect of species and temperature. When there were significant differences between treatments, Tukey’s HSD test was performed. Otherwise, a Kruskal-Wallis test was used, looking separately at the effect of temperature and species. When the effect was significant, Dunn’s test was performed. For the uptake rates of C, N using the stable isotope tracers technique, and the cellular CNP content, the effect of the fraction (host or symbiont) was also considered. Regarding the uptake rates of 15N, the potential effect of the N source (NH4+, NO3-, urea or DFAAs) was considered as well. Similarly, the separate effect of DOC and DON concentration on DOC and DON fluxes was also looked at. Finally, a Student T-test was performed to test the effect of temperature on E. singularis symbiosis-related parameters (Pn, Pg, symbiont density, total chlorophyll content and P:R). Differences were considered significant when p< 0.05. The data were normalized both by the nubbin’s AFDW and surface (cm2) and the statistical results obtained were essentially the same. Only the data normalized by AFDW will be used to discuss the results, and the data normalized by surface are available as Supplementary Information (Figure S1; Tables S2-4).
The results of the statistical tests are reported in the Supplementary Material in Table S7 for symbiotic - related parameters of E. singularis and in Table S8 for physiological and tissue parameters.
In E. singularis, heat stress induced a 40% decrease in symbiont density (Figure 1A; p< 0.05), although there was no significant decrease in chlorophyll content (Figure S2). As a result, net photosynthetic rates decreased by nearly 20% (Figure 1B; p< 0.05), without any change in the gross photosynthesis (Pg; Figure 1C), but decreased P:R ratios by 19% (Figure 1D, p< 0.05). Therefore, photosynthesis could not sustain respiration at 24°C (P:R< 1). Heat stress did not change the lipid or protein content (Figures 2A, B), but increased respiration rates by 47% (Figure 2C; p< 0.01). It also induced a 14, 21 and 30% decrease in C, N and P content, in the symbiont fraction (p< 0.01, p< 0.01 and p< 0.01, respectively), as well as a 20% decrease in both N and P content in the host fraction (Figures 3A-C; p< 0.0001 and p< 0.01, respectively). As a result, C:N and C:P ratios significantly increased in both fractions (p< 0.01 and p< 0.0001, respectively), with also an increase of the N:P ratio in the host fraction (Figures 3D–F; p< 0.05).
Figure 1 Symbiotic-related physiological parameters of E. singularis at 17°C (blue plots) and 24°C (red plots). The boxplot represent symbiont density (107 cells g-1 AFDW) (A), net (B) and gross (C) photosynthesis rates (µmol O2 g-1 AFDW h-1) and P:R (D) (n = 6). AFDW, ash-free dry weight; P:R, contribution of photosynthesis to the holobiont respiratory requirements. Significant differences are displayed with asterisks (p value<0.05).
Figure 2 Gorgonian physiological parameters at 17°C (blue plots) and 24°C (red plots). The boxplots represent total lipid (A) and protein (B) content (mg g-1 AFDW), and respiration rates (µmol O2 g-1 AFDW h-1) (C) (n = 6). AFDW, ash-free dry weight. Significant differences between temperatures for each species are displayed with asterisks (p value<0.05). Significant differences between species are distinguished with letters, for each temperature separately.
Figure 3 Gorgonian tissue parameters at 17°C (blue plots) and 24°C (red plots). The boxplot represent carbon (A), nitrogen (B), phosphorus (C) content in tissue (mg g-1 AFDW), and C:N (D), C:P (E) N:P (F) ratio in tissue (n = 6). AFDW, ash-free dry weight. Significant differences between temperatures for each species are displayed with asterisks (p value<0.05). Significant differences between species and/or fractions are distinguished with letters, for each temperature separately.
In P. clavata, heat stress increased respiration rates by 73% (p< 0.01), along with a 30% decrease in lipid reserves (Figures 2A, B; p< 0.05). These parameters were however significantly lower in P. clavata than in E. singularis (p< 0.01). On the contrary, its protein content was two times higher than E. singularis (p< 0.001). Regarding tissue content, heat stress only impacted the C content of P. clavata (p< 0.01), which induced a decrease in the C:N ratio (p< 0.01). On the contrary, the N:P ratio increased with heat stress (p< 0.001).
The results of the statistical tests are reported in the Supplementary Material in Table S9 for DOC fluxes, in Table S10 for CHARDOC and in Table S11 for DIC uptake.
At 17°C, DOC fluxes were positive for ambient DOM conditions ([DOMnat]) for both species, suggesting a net release of DOC (Figure 4A). However, under DOM enrichment, there was a significant DOC uptake for both species (p< 0.05). Similarly, CHARDOC significantly increased with DOM enrichment for both species (Table 2; p< 0.05 for E. singularis and p< 0.01 for P. clavata), although DOM could not supply 100% of the respiratory needs of both species in any condition. Heat stress increased DOC uptake rates of E. singularis, at both 3 x [DOMnat] and 6 x [DOMnat] (p< 0.01), leading to a significant increase of its CHARDOC above 100% of the respiratory needs (p< 0.05). On the contrary, heat stress increased DOC release rates of P. clavata at [DOMnat] (p< 0.01) and had no significant impact at higher DOM concentrations. Finally, under heat stress and DOM enrichment, DOC uptake rates of E. singularis were significantly higher than those of P. clavata (p< 0.05).
Figure 4 Assimilation of dissolved carbon by gorgonians at 17°C (blue bars) and 24°C (red bars). The boxplots represent the DOC fluxes (µmol C g-1 AFDW h-1) using a mixture of natural DOM at different concentrations (A) (n = 6) and DIC uptake rates (µmol C g-1 AFDW h-1) of each fraction of the gorgonians, using labelled 13C (B) (n = 12). AFDW, ash-free dry weight; DOC, dissolved organic carbon; DIC, dissolved inorganic carbon; DOM, dissolved organic matter. For the graph (A), a negative flux implies an uptake of DOC, whereas a positive flux implies a release of DOC. Significant differences in DOC fluxes between the lowest and highest DOC concentration (A; [DOMnat] and 6 x [DOMnat], respectively) are displayed with an arrow. For the graph (B), significant differences in DIC uptake rates between fractions are distinguished with letters, for each temperature separately. For both graphs, significant differences between temperatures are displayed with asterisks (p value<0.05).
Table 2 Contribution of DOC heterotrophy relative to the holobiont respiration (CHARDOC, %) regarding species, temperature, and DOC concentration.
In contrast to P. clavata, E. singularis could also use autotrophic C through the fixation of DIC by the symbionts (Figure 4B). Measurements performed with 13C-bicarbonate showed that the C taken up by E. singularis after 7 h incubation was mainly found in the symbionts (80% assimilated from a total of 13.2 ± 3.4 µmol C g-1 AFDW h-1; Figure S3A). Under heat stress, DIC uptake rates of the holobiont remained constant (Figure S3B) but the contribution of each fraction was different. Indeed, heat stress increased the DIC uptake rates by the host fraction (36% of total assimilated C; p< 0.01), while uptake by the symbiont fraction decreased to 64% (p< 0.05).
The results of the statistical tests are reported in the Supplementary Material in Table S9 for DON fluxes and in Table S11 for N uptake using labelled 15N-compound.
Measurements of DON fluxes showed that, at 17°C, there was no DON net uptake by the gorgonians, even under high enrichment (Figure 5A), suggesting that excretion of DON by the gorgonians exceeded the DON uptake. Indeed, there was a constant net release of DON by E. singularis, independently of DOM enrichments, while DOM enrichment slightly reduced the release of DON by P. clavata (p< 0.01). Heat stress increased DON release for both species at [DOMnat] (p< 0.05), as well as at 3 x [DOMnat] for P. clavata (p< 0.01).
Figure 5 Assimilation of dissolved nitrogen by gorgonians at 17°C (blue bars) and 24°C (red bars). The boxplots represent the DON fluxes (µmol N g-1 AFDW h-1) using a mixture of natural DOM at different concentrations (A) (n = 6) and DN uptake rates according to different nitrogen sources (nmol N g-1 AFDW h-1) for each fraction of the two gorgonian species, using labelled 15N (B) (n = 6). AFDW, ash-free dry weight; DFAAs, dissolved free amino acids; DON, dissolved organic nitrogen; DOM, dissolved organic matter. For the graph (A), a negative flux implies an uptake of DON, whereas a positive flux implies a release of DON. For the graph (B), for each fraction and temperature independently, significant differences in DN uptake rates between nitrogen sources are distinguished with letters. For both graphs, significant differences between temperatures are displayed with asterisks (p value<0.05).
Experiments with labelled 15N-compounds showed a significant uptake of all sources of dissolved N by both gorgonian species, with a higher uptake of N-NH4+ and N-DFAAs compared to the other forms (Figure 5B). Note that, for the urea source, a given N uptake rate implies an uptake of urea molecules twice as low. In E. singularis, symbionts contributed more than 50% to N-NH4+ uptake and 80% to N-DFAAs uptake (Figure 5B; S4A). On the contrary, they assimilated less N-NO3- and N-urea than the host (p< 0.01 and p< 0.05, respectively). At the holobiont level, there was no major difference in total dissolved N uptake between species. While E. singularis holobiont assimilated twice more N-NH4+ than P. clavata (ca 120 nmol N g-1 AFDW h-1; Figure S4B; p< 0.01), it assimilated less DON (p< 0.01). The additional investigation of N uptake by isolated mucus of gorgonians showed that only 7% to 8% of N-NH4+ uptake by the holobiont (for P. clavata and E. singularis, respectively; Table S5) was carried out by the mucus-associated microbes.
Under heat stress, N-NH4+ uptake rates decreased in the symbionts of E. singularis, while N-NO3- uptake rates increased (Figure 5B; p< 0.05 and p< 0.05, respectively). The host fraction, however, increased its N-DFAAs uptake, thus reducing the symbionts relative contribution to total N-DFAAs uptake (Figure 5B and S4A; p< 0.05 and p< 0.05, respectively). At the holobiont level, E. singularis assimilated more N-DFAAs and N-NO3- than under normal temperature (Figure S4B; p< 0.05 and p< 0.01, respectively), while P. clavata stopped assimilating N-urea and N-NO3- (p< 0.01 and p< 0.05, respectively). However, E. singularis total N-DFAAs uptake was still lower than for P. clavata, along with a higher N-NH4+ uptake (p< 0.05 and p< 0.01, respectively).
Understanding the nutritional requirements of Mediterranean gorgonians, which are essential in forming large Marine Animal Forests (Rossi et al., 2017), is critical for developing effective management practices that can improve gorgonian health and resilience, especially in the face of climate change stressors. Despite high concentrations of dissolved inorganic and organic nitrogen (DIN, DON) in the mesotrophic Mediterranean Sea (Coste et al., 1988; Bethoux et al., 1992), the extent to which gorgonians rely on these nutrients is unknown. The main results of the study, summarized in Table 3 and discussed below, indicate that temperate gorgonians can assimilate all forms of DIN and DON, but to a much lesser extent than tropical octocorals and scleractinian corals, despite being exposed to higher nutrient concentrations. As a result, dissolved N does not contribute significantly to their diet. In addition, at natural concentrations, they do not rely on dissolved organic matter (DOM) to feed, but they can use it when concentrations of DOM are increased. The asymbiotic gorgonian Paramuricea clavata was also able to take up relatively high amounts of ammonium (NH4+), despite the absence of Symbiodiniaceae, which are usually the main drivers of NH4+ uptake in many coral species (Muscatine et al., 1979; Grover et al., 2002; Grover et al., 2003; Pernice et al., 2012). Finally, an increase in temperature to 24°C for 2 weeks significantly affected the health of the gorgonians and their ability to take up nutrients.
Table 3 Recapitulative table of the effect of temperature and DOM concentration on all parameters measured, for each species.
The two gorgonian species studied, the asymbiotic P. clavata and the symbiotic E. singularis, thrive in mesotrophic waters with high concentrations of DON (up to 14 µM, Morganti et al., 2017) and DIN (1-3 µM); that vary depending on the season (Ribes et al., 1999b; Aminot and Kérouel, 2004; Segura-Noguera et al., 2016). These values are significantly higher than those typically found in reef waters (DON: 3.8-5.6 µM (Tanaka et al., 2011), DIN: 1-2 µM). Despite being able to take up DIN, mainly in the form of NH4+ (at rates of 60-120 nmol N g-1 AFDW h-1), the uptake rates of both gorgonian species were lower than those of tropical scleractinian corals (in the µmol range, Grover et al., 2002; Pupier et al., 2021) and tropical octocorals of the suborder Alcyonaceae (Pupier et al., 2021, ranging from 150 to 640 nmol N g-1 AFDW h-1, depending on the species). The most meaningful comparison can be made between E. singularis, which lives in symbiosis with Symbiodiniaceae dinoflagellates (LaJeunesse et al., 2018), and the tropical species mentioned above, all of which live symbiotically. On the contrary to tropical soft corals, gorgonian tissue is thin and cannot act as a barrier to N diffusion (Pupier et al., 2021). Therefore the lower uptake rates observed in E. singularis compared to tropical octocorals could be due to the lower irradiance it receives (50 – 150 µmol photons m-2 s-1 (Gori et al., 2012), compared to > 400 µmol photons m-2 s-1 for tropical species). Indeed, a positive correlation has been demonstrated between irradiance, symbiont photosynthesis, and N uptake in corals, with increased photosynthesis and N uptake under high light conditions (Grover et al., 2002). In addition, the Mediterranean seawater temperature varies between 12°C and 24°C depending on the season, compared to a minimum of 25°C for tropical species. Therefore, E. singularis may have a lower metabolism than tropical species due to the lower temperatures at which it thrives (Previati et al., 2010).
An interesting observation is that the asymbiotic gorgonian P. clavata exhibited the same rates of NH4+ uptake into host tissue as the symbiotic E. singularis. Normally, the first site of NH4+ uptake in corals is the Symbiodiniaceae component, which assimilates 90% of the NH4+ taken up via the glutamine synthetase/glutamine oxoglutarate aminotransferase pathway (GS/GOGAT) (Pernice et al., 2012; Roberty et al., 2020). Although the coral host can also assimilate NH4+ via two pathways (GS/GOGAT and glutamate dehydrogenase pathway), and has ammonium transporters (Capasso et al., 2022), direct NH4+ uptake is generally low (Pernice et al., 2012), except under certain stress conditions (Tang et al., 2020; Xiang et al., 2020). Unlike Symbiodiniaceae-bearing corals, non-symbiotic corals are generally unable to assimilate DIN (Muscatine et al., 1979) and instead excrete NH4+ and urea into the surrounding water (Rahav et al., 1989). Since microbes in the mucus layer accounted for only 7% of the total NH4+ uptake of gorgonians, P. clavata must have evolved specific adaptations to assimilate NH4+ and benefit from this additional N source. Alternatively, NH4+ uptake may derive from the activity of microbes inside the gorgonian tissue, although the measured uptake rates are very high for such a presumed tiny microbial biomass. In addition to NH4+ uptake, there was also very low uptake of nitrate (NO3-) in both gorgonians (although 8 to 20 times lower than NH4+, which in turn is lower than in tropical corals). This uptake may be attributed to the microbes associated with the gorgonians, in particular from the bacterial symbiont Endozoicomonas. This bacteria is very abundant in Mediterranean gorgonians (Van De Water et al., 2017; Van De Water et al., 2018) and can take up NO3- and reduce it into NH4+ (Neave et al., 2014; Neave et al., 2017).
Gorgonians were also able to assimilate labile DON, especially dissolved free amino acids (DFAAs). However, uptake rates were relatively low, in the nanomolar range (20 to 50 nmol N g-1 AFDW h-1) and were at the lower end of rates measured for tropical soft corals (20 to 200 nmol N g-1 AFDW h-1), themselves lower than for scleractinian corals (Grover et al., 2008; Pupier et al., 2021). DON uptake rates were however higher in the host tissue of the heterotrophic gorgonian P. clavata than in the symbiotic E. singularis, suggesting that P. clavata had to compensate for lower DIN uptake due to the absence of Symbiodiniaceae (total DN uptake of P. clavata: 120 nmol N g-1 AFDW h-1 vs 160 for E. singularis holobiont). The uptake of nanomolar amounts of DFAAs suggests that the gorgonians either do not have a large requirement for DON or are unable to assimilate large amounts of DON. In addition, such nanomolar uptake of DFAAs cannot be measured through the incubations with natural DOM, which contains both labile and nonlabile DON at concentrations of 10 µmol N L-1. This differs from tropical octocorals, which are able to assimilate large amounts of DON when it is present in seawater (Lange et al., 2023). Considering the low uptake rates of DIN and DON, our results suggest that Mediterranean gorgonians have a low capacity to feed on dissolved N sources. However, their low C:N ratio of 5-6 indicates that gorgonians are not limited in N. In fact, the C:N ratio is in the same range as the Redfield ratio (C:N = 6.6) and much lower than the C:N ratio of N-depleted phytoplankton or of deep-sea corals (C:N > 10, Losh et al., 2012; Ferrier-Pagès et al., 2022). Mediterranean gorgonians should therefore meet their N requirements through heterotrophic feeding on particulate organic material (plankton). To our knowledge, no previous study has assessed the amount of N supplied to gorgonians through heterotrophy. However, if we assume a C:N ratio of 6 for plankton and a heterotrophic uptake of 450 µg C g-1 AFDW h-1 measured for the Mediterranean gorgonian Leptogorgia sarmentosa (Ribes et al., 2003), the amount of N that can be obtained by heterotrophy may be ca. 75 µg N g-1 AFDW h-1 or 5.4 µmol N g-1 AFDW h-1, which is in fact more than 30 times the amount of N obtained by the uptake of DIN and DON.
From the measurements of the dissolved organic carbon (DOC) fluxes, it appears that, as with N, the gorgonians release more DOC than they take up from the natural DOM at ambient concentration (80 µmol C L-1), which is consistent with a previous study on Mediterranean gorgonians (Ribes et al., 1999a; Ribes et al., 2003). The ratio between the release of DOC and the net photosynthetic rate of E. singularis (13%) is in the same range as previous measurements on tropical corals (Ferrier-Pagès et al., 1998; Tanaka et al., 2009). Nevertheless, our results show that gorgonians were able to take up significant amounts of DOC under enrichment conditions (3 and 6 x [DOMnat]), suggesting that they require a certain threshold for DOC utilization, as previously observed in sponges (Wooster et al., 2019; Ribes et al., 2023). Such high concentrations can be observed in coastal areas receiving DOM from rivers (Cauwet et al., 1990; Ejarque et al., 2017; Sánchez-Pérez et al., 2020). Eunicella singularis can also acquire C as DIC thanks to the presence of Symbiodiniaceae in its tissues. However, although E. singularis had similar concentrations of symbionts and chlorophyll as many tropical soft corals and gorgonians (Rossi et al., 2018; Pupier et al., 2019b; Rossi et al., 2020), its C fixation rate was 10-fold lower except when compared to tropical sea rods (Rossi et al., 2018). This may be related to the polyp size of E. singularis, which is also comparable to sea rods, as a correlation between polyp size and primary productivity has been demonstrated in tropical gorgonians (Baker et al., 2015; Rossi et al., 2018). However, the amount of C supplied to E. singularis by photosynthesis under our experimental conditions (120 µmol photons m-2 s-1, corresponding to the summer season) is lower than the amount of C obtained by heterotrophy (Ribes et al., 1999a; Ribes et al., 2003). These measurements confirm that Mediterranean gorgonians rely more on the uptake of particulate food, such as pico- and nanoplankton, than on the uptake of dissolved material.
The health of gorgonian species was significantly affected when the temperature was raised to 24°C for two weeks. A temperature of 24°C - 25°C is a threshold for many Mediterranean corals (Rodolfo-Metalpa et al., 2006; Tignat-Perrier et al., 2022), which was not often reached except at very shallow depths before 2011, but since then has been increasingly measured down to 40 m depth (Cerrano et al., 2000; Garrabou et al., 2009; Garrabou et al., 2022). After several weeks (5 to 8 weeks) at 25°C, gorgonians generally show tissue necrosis and microbial diseases (Tignat-Perrier et al., 2022). In this study, we did not aim to reach this final health state, but only to induce heat stress with reversible effects on gorgonian health. In E. singularis, bleaching and increased respiration rate resulted in a significant loss of cellular C, N and P content, as observed previously (Baker et al., 2018). In P. clavata, the increased respiration rates resulted in a significant decrease in lipid content and thus cellular C content, consistent with Tignat-Perrier et al. (2022). To compensate for the higher respiration rates and energy costs, E. singularis significantly increased its heterotrophic diet on DOC. Indeed, feeding on 3x and 6x [DOMnat] allowed E. singularis to meet more than 100% of its respiration requirements, consistent with previous findings that some corals can rely on DOC to compensate for loss of autotrophy (Levas et al., 2016). However, DOC uptake was not accompanied by major increase of DON or DIN uptake in either gorgonian. Overall, these changes resulted in a significant increase in the C:N ratio (and C:P ratio) of symbionts and host tissues of E. singularis, suggesting that this species may become N and P limited in the long term under heat stress. Paramuricea clavata also lost N in the form of DON. This release may be due to either increased release of mucoid exudates or increased release of coral cellular material (e.g., detachment of host cells) following heat stress (Tanaka et al., 2009). The last hypothesis seems to be the most likely, since it has indeed been observed that P. clavata is subjected to severe oxidative stress at high temperatures, leading to tissue necrosis after several weeks of stress (Tignat-Perrier et al., 2022). Overall, these observations strongly indicate that gorgonians are highly vulnerable to starvation during marine heat waves (MHWs). During the summer months, the stratification of the water column leads to a significant decline in zooplankton abundance in surface waters (Roemmich and McGowan, 1995), which directly impacts filter feeders like gorgonians by depriving them of a vital food source. Unlike tropical corals, Mediterranean gorgonians lack the ability to rely on the uptake of dissolved inorganic and organic nutrients, exacerbating their susceptibility to food scarcity.
The diet of Mediterranean gorgonians appears to rely more on the capture of plankton prey than on dissolved inorganic and organic nutrients. Previous studies has shown that P. clavata predominantly ingests particulate detritus (Ribes et al., 1999a) and captures a large number of nauplii and small prey items (< 200 µm) during periods of high prey abundance in autumn and spring (Coma et al., 1994). In E. singularis, symbionts can however bring a significant amount of C to the symbiosis, especially during summer, when light is maximal, and plankton abundance is minimal. We studied here the only symbiotic octocoral gorgonian of the Mediterranean Sea (Eunicella singularis) and one widespread asymbiotic gorgonian, Paramuricea clavata. As the response may differ across species, there may be other species-specific differences aside from symbiosis vs non-symbiosis that remains to be investigated. In addition, in tropical reefs, sponges are known to play a key role in processing DOM before returning it to corals in the form of particulate organic matter (the “sponge loop”, de Goeij et al., 2013). Given the high abundance of sponges in the coralligenous assemblages of the Mediterranean Sea, it would be valuable to investigate the potential importance of the sponge loop for gorgonian’s nutrition.
The original contributions presented in the study are included in the article/Supplementary Material. Further inquiries can be directed to the corresponding author.
The manuscript presents research on animals that do not require ethical approval for their study.
KL, SR and CF-P conceived and designed the experiment. KL performed the experiments. J-FM performed the mass spectrometry analyses. KL analyzed the data. All authors contributed to the article and approved the submitted version.
The author(s) declare financial support was received for the research, authorship, and/or publication of this article. This study was funded by the Scientific Center of Monaco.
The authors would like to thank MI. Marcus and L. Millet for laboratory assistance, as well as R. Grover for fruitful discussions.
The authors declare that the research was conducted in the absence of any commercial or financial relationships that could be construed as a potential conflict of interest.
All claims expressed in this article are solely those of the authors and do not necessarily represent those of their affiliated organizations, or those of the publisher, the editors and the reviewers. Any product that may be evaluated in this article, or claim that may be made by its manufacturer, is not guaranteed or endorsed by the publisher.
The Supplementary Material for this article can be found online at: https://www.frontiersin.org/articles/10.3389/fmars.2023.1236164/full#supplementary-material
Aminot A., Kérouel R. (2004). Dissolved organic carbon, nitrogen and phosphorus in the N-E Atlantic and the N-W Mediterranean with particular reference to non-refractory fractions and degradation. Deep Sea Res. Part Oceanogr. Res. Pap. 51, 1975–1999. doi: 10.1016/j.dsr.2004.07.016
Baker D. M., Andras J. P., Jordán-Garza A. G., Fogel M. L. (2013). Nitrate competition in a coral symbiosis varies with temperature among Symbiodinium clades. ISME J. 7, 1248–1251. doi: 10.1038/ismej.2013.12
Baker D. M., Freeman C. J., Knowlton N., Thacker R. W., Kim K., Fogel M. L. (2015). Productivity links morphology, symbiont specificity and bleaching in the evolution of Caribbean octocoral symbioses. ISME J. 9, 2620–2629. doi: 10.1038/ismej.2015.71
Baker D. M., Freeman C. J., Wong J. C. Y., Fogel M. L., Knowlton N. (2018). Climate change promotes parasitism in a coral symbiosis. ISME J. 12, 921–930. doi: 10.1038/s41396-018-0046-8
Baker D. M., Kim K., Andras J. P., Sparks J. P. (2011). Light-mediated 15N fractionation in Caribbean gorgonian octocorals: implications for pollution monitoring. Coral Reefs 30, 709–717. doi: 10.1007/s00338-011-0759-x
Bednarz V. N., Naumann M. S., Niggl W., Wild C. (2012). Inorganic nutrient availability affects organic matter fluxes and metabolic activity in the soft coral genus Xenia. J. Exp. Biol. 215, 3672–3679 doi: 10.1242/jeb.072884
Bethoux J. P., Morin P., Madec C., Gentili B. (1992). Phosphorus and nitrogen behaviour in the Mediterranean Sea. Deep Sea Res. Part Oceanogr. Res. Pap. 39, 1641–1654. doi: 10.1016/0198-0149(92)90053-V
Capasso L., Aranda M., Cui G., Pousse M., Tambutté S., Zoccola D. (2022). Investigating calcification-related candidates in a non-symbiotic scleractinian coral, Tubastraea spp. Sci. Rep. 12, 13515. doi: 10.1038/s41598-022-17022-4
Cauwet G., Gadel F., de Souza Sierra M. M., Donard O., Ewald M. (1990). Contribution of the Rhône River to organic carbon inputs to the northwestern Mediterranean Sea. Cont. Shelf Res. 10, 1025–1037. doi: 10.1016/0278-4343(90)90073-U
Cerrano C., Bavestrello G., Bianchi C. N., Cattaneo-vietti R., Bava S., Morganti C., et al. (2000). A catastrophic mass-mortality episode of gorgonians and other organisms in the Ligurian Sea (North-western Mediterranean), summer 1999. Ecol. Lett. 3, 284–293. doi: 10.1046/j.1461-0248.2000.00152.x
Cheng Y., Zheng Y., VanderGheynst J. S. (2011). Rapid quantitative analysis of lipids using a colorimetric method in a microplate format. Lipids 46, 95–103. doi: 10.1007/s11745-010-3494-0
Coma R., Gili J.-M., Zabala M., Riera T. (1994). Feeding and prey capture cycles in the aposymbiontic gorgonian Paramuricea clavata. Mar. Ecol. Prog. Ser. 115, 257–270. doi: 10.3354/meps115257
Coste B., Corre P. L., Minas H. J. (1988). Re-evaluation of the nutrient exchanges in the strait of Gibraltar. Deep Sea Res. Part Oceanogr. Res. Pap. 35, 767–775. doi: 10.1016/0198-0149(88)90029-5
de Goeij J. M., van Oevelen D., Vermeij M. J. A., Osinga R., Middelburg J. J., de Goeij A. F. P. M., et al. (2013). Surviving in a marine desert: the sponge loop retains resources within coral reefs. Science 342, 108–110. doi: 10.1126/science.1241981
Doval M. D., Pérez F. F., Berdalet E. (1999). Dissolved and particulate organic carbon and nitrogen in the Northwestern Mediterranean. Deep Sea Res. Part Oceanogr. Res. Pap. 46, 511–527. doi: 10.1016/S0967-0637(98)00072-7
Ejarque E., Freixa A., Vazquez E., Guarch A., Amalfitano S., Fazi S., et al. (2017). Quality and reactivity of dissolved organic matter in a Mediterranean river across hydrological and spatial gradients. Sci. Total Environ., 599–600. doi: 10.1016/j.scitotenv.2017.05.113
Ferrier-Pagès C., Bednarz V., Grover R., Benayahu Y., Maguer J., Rottier C., et al. (2022). Symbiotic stony and soft corals: Is their host-algae relationship really mutualistic at lower mesophotic reefs? Limnol. Oceanogr. 67, 261–271. doi: 10.1002/lno.11990
Ferrier-Pagès C., Gattuso J.-P., Cauwet G., Jaubert J., Allemand D. (1998). Release of dissolved organic carbon and nitrogen by the zooxanthellate coral Galaxea fascicularis. Mar. Ecol. Prog. Ser. 172, 265–274. doi: 10.3354/meps172265
Freeman C., Stoner E., Easson C., Matterson K., Baker D. (2016). Symbiont carbon and nitrogen assimilation in the Cassiopea–Symbiodinium mutualism. Mar. Ecol. Prog. Ser. 544, 281–286. doi: 10.3354/meps11605
Garrabou J., Coma R., Bensoussan N., Bally M., Chevaldonné P., Cigliano M., et al. (2009). Mass mortality in Northwestern Mediterranean rocky benthic communities: effects of the 2003 heat wave. Glob. Change Biol. 15, 1090–1103. doi: 10.1111/j.1365-2486.2008.01823.x
Garrabou J., Gómez-Gras D., Medrano A., Cerrano C., Ponti M., Schlegel R., et al. (2022). Marine heatwaves drive recurrent mass mortalities in the Mediterranean Sea. Glob. Change Biol. 28, 5708–5725. doi: 10.1111/gcb.16301
Gili J.-M., Coma R. (1998). Benthic suspension feeders: their paramount role in littoral marine food webs. Trends Ecol. Evol. 13, 316–321. doi: 10.1016/S0169-5347(98)01365-2
Gori A., Rossi S., Berganzo E., Pretus J. L., Dale M. R. T., Gili J.-M. (2011a). Spatial distribution patterns of the gorgonians Eunicella singularis, Paramuricea clavata, and Leptogorgia sarmentosa (Cape of Creus, Northwestern Mediterranean Sea). Mar. Biol. 158, 143–158. doi: 10.1007/s00227-010-1548-8
Gori A., Rossi S., Linares C., Berganzo E., Orejas C., Dale M. R., et al. (2011b). Size and spatial structure in deep versus shallow populations of the Mediterranean gorgonian Eunicella singularis (Cap de Creus, northwestern Mediterranean Sea). Mar. Biol. 158, 1721–1732. doi: 10.1007/s00227-011-1686-7
Gori A., Viladrich N., Gili J.-M., Kotta M., Cucio C., Magni L., et al. (2012). Reproductive cycle and trophic ecology in deep versus shallow populations of the Mediterranean gorgonian Eunicella singularis (Cap de Creus, northwestern Mediterranean Sea). Coral Reefs 31, 823–837. doi: 10.1007/s00338-012-0904-1
Grinyó J., Gori A., Ambroso S., Purroy A., Calatayud C., Dominguez-Carrió C., et al. (2016). Diversity, distribution and population size structure of deep Mediterranean gorgonian assemblages (Menorca Channel, Western Mediterranean Sea). Prog. Oceanogr. 145, 42–56. doi: 10.1016/j.pocean.2016.05.001
Grover R., Maguer J.-F., Allemand D., Ferrier-Pagés C. (2003). Nitrate uptake in the scleractinian coral Stylophora pistillata. Limnol. Oceanogr. 48, 2266–2274. doi: 10.4319/lo.2003.48.6.2266
Grover R., Maguer J.-F., Allemand D., Ferrier-Pagès C. (2006). Urea uptake by the scleractinian coral Stylophora pistillata. J. Exp. Mar. Biol. Ecol. 332, 216–225. doi: 10.1016/j.jembe.2005.11.020
Grover R., Maguer J.-F., Allemand D., Ferrier-Pagès C. (2008). Uptake of dissolved free amino acids by the scleractinian coral Stylophora pistillata. J. Exp. Biol. 211, 860–865. doi: 10.1242/jeb.012807
Grover R., Maguer J.-F., Reynaud-Vaganay S., Ferrier-Pagès C. (2002). Uptake of ammonium by the scleractinian coral Stylophora pistillata: Effect of feeding, light, and ammonium concentrations. Limnol. Oceanogr. 47, 782–790. doi: 10.4319/lo.2002.47.3.0782
Howarth R. W. (1988). Nutrient limitation of net primary production in marine ecosystems. Annu. Rev. Ecol. Syst. 19, 89–110. doi: 10.1146/annurev.es.19.110188.000513
Jeffrey S. W., Humphrey G. F. (1975). New spectrophotometric equations for determining chlorophylls a, b, c1 and c2 in higher plants, algae and natural phytoplankton. Biochem. Physiol. Pflanz. 167, 191–194. doi: 10.1016/S0015-3796(17)30778-3
Klais R., Norros V., Lehtinen S., Tamminen T., Olli K. (2017). Community assembly and drivers of phytoplankton functional structure. Funct. Ecol. 31, 760–767. doi: 10.1111/1365-2435.12784
Knowlton N., Rohwer F. (2003). Multispecies microbial mutualisms on coral reefs: the host as a habitat. Am. Nat. 162, S51–S62. doi: 10.1086/378684
LaJeunesse T. C., Parkinson J. E., Gabrielson P. W., Jeong H. J., Reimer J. D., Voolstra C. R., et al. (2018). Systematic revision of symbiodiniaceae highlights the antiquity and diversity of coral endosymbionts. Curr. Biol. 28, 2570–2580.e6. doi: 10.1016/j.cub.2018.07.008
Lange K., Reynaud S., De Goeij J., Ferrier-Pagès C. (2023). Data from: The effects of dissolved organic matter supplements on the metabolism of corals under heat stress. Dryad Digital Repository. doi: 10.5061/dryad.np5hqc00m
Levas S., Grottoli A. G., Schoepf V., Aschaffenburg M., Baumann J., Bauer J. E., et al. (2016). Can heterotrophic uptake of dissolved organic carbon and zooplankton mitigate carbon budget deficits in annually bleached corals? Coral Reefs 35, 495–506. doi: 10.1007/s00338-015-1390-z
Losh J., Morel F., Hopkinson B. (2012). Modest increase in the C:N ratio of N-limited phytoplankton in the California Current in response to high CO2. Mar. Ecol. Prog. Ser. 468, 31–42. doi: 10.3354/meps09981
Morganti T., Coma R., Yahel G., Ribes M. (2017). Trophic niche separation that facilitates co-existence of high and low microbial abundance sponges is revealed by in situ study of carbon and nitrogen fluxes: Trophic niche separation in Sponges. Limnol. Oceanogr. 62, 1963–1983. doi: 10.1002/lno.10546
Muscatine L., Masuda H., Burnap R. (1979). Ammonium uptake by symbiotic and aposymbiotic reef corals. Bull. Mar. Sci. 29, 572–575.
Neave M. J., Michell C. T., Apprill A., Voolstra C. R. (2014). Whole-genome sequences of three symbiotic endozoicomonas strains. Genome Announc. 2, e00802–e00814. doi: 10.1128/genomeA.00802-14
Neave M. J., Michell C. T., Apprill A., Voolstra C. R. (2017). Endozoicomonas genomes reveal functional adaptation and plasticity in bacterial strains symbiotically associated with diverse marine hosts. Sci. Rep. 7, 40579. doi: 10.1038/srep40579
Pernice M., Meibom A., Van Den Heuvel A., Kopp C., Domart-Coulon I., Hoegh-Guldberg O., et al. (2012). A single-cell view of ammonium assimilation in coral–dinoflagellate symbiosis. ISME J. 6, 1314–1324. doi: 10.1038/ismej.2011.196
Pey A., Catanéo J., Forcioli D., Merle P.-L., Furla P. (2013). Thermal threshold and sensitivity of the only symbiotic Mediterranean gorgonian Eunicella singularis by morphometric and genotypic analyses. C. R. Biol. 336, 331–341. doi: 10.1016/j.crvi.2013.06.008
Previati M., Scinto A., Cerrano C., Osinga R. (2010). Oxygen consumption in Mediterranean octocorals under different temperatures. J. Exp. Mar. Biol. Ecol. 390, 39–48. doi: 10.1016/j.jembe.2010.04.025
Pupier C. A., Bednarz V. N., Ferrier-Pagès C. (2018). Studies with soft corals – recommendations on sample processing and normalization metrics. Front. Mar. Sci. 5. doi: 10.3389/fmars.2018.00348
Pupier C. A., Bednarz V. N., Grover R., Fine M., Maguer J.-F., Ferrier-Pagès C. (2019a). Divergent capacity of scleractinian and soft corals to assimilate and transfer diazotrophically derived nitrogen to the reef environment. Front. Microbiol. 10, 1860. doi: 10.3389/fmicb.2019.01860
Pupier C. A., Fine M., Bednarz V. N., Rottier C., Grover R., Ferrier-Pagès C. (2019b). Productivity and carbon fluxes depend on species and symbiont density in soft coral symbioses. Sci. Rep. 9, 17819. doi: 10.1038/s41598-019-54209-8
Pupier C. A., Grover R., Fine M., Rottier C., van de Water J. A. J. M., Ferrier-Pagès C. (2021). Dissolved nitrogen acquisition in the symbioses of soft and hard corals with symbiodiniaceae: A key to understanding their different nutritional strategies? Front. Microbiol. 12. doi: 10.3389/fmicb.2021.657759
Rädecker N., Pogoreutz C., Voolstra C. R., Wiedenmann J., Wild C. (2015). Nitrogen cycling in corals: the key to understanding holobiont functioning? Trends Microbiol. 23, 490–497. doi: 10.1016/j.tim.2015.03.008
Rahav O., Dubinsky Z., Achituv Y., Falkowski P. G. (1989). Ammonium metabolism in the zooxanthellate coral, Stylophora pistillata. Proc. R. Soc Lond. B. Biol. Sci. 236, 325–337. doi: 10.1098/rspb.1989.0026
Reinthaler T., Sintes E., Herndl G. J. (2008). Dissolved organic matter and bacterial production and respiration in the sea-surface microlayer of the open Atlantic and the western Mediterranean Sea. Limnol. Oceanogr. 53, 122–136. doi: 10.4319/lo.2008.53.1.0122
Ribes M., Coma R., Gili J.-M. (1999a). Heterogeneous feeding in benthic suspension feeders:the natural diet and grazing rate of the temperate gorgonian Paramuricea clavata (Cnidaria:Octocorallia) over a year cycle. Mar. Ecol. Prog. Ser. 183, 125–137. doi: 10.3354/meps183125
Ribes M., Coma R., Gili J.-M. (1999b). Seasonal variation of particulate organic carbon, dissolved organic carbon and the contribution of microbial communities to the live particulate organic carbon in a shallow near-bottom ecosystem at the Northwestern Mediterranean Sea. J. Plankton Res. 21, 1077–1100. doi: 10.1093/plankt/21.6.1077
Ribes M., Coma R., Rossi S. (2003). Natural feeding of the temperate asymbiotic octocoral-gorgonian Leptogorgia sarmentosa (Cnidaria: Octocorallia). Mar. Ecol. Prog. Ser. 254, 141–150. doi: 10.3354/meps254141
Ribes M., Yahel G., Romera-Castillo C., Mallenco R., Morganti T. M., Coma R. (2023). The removal of dissolved organic matter by marine sponges is a function of its composition and concentration: An in situ seasonal study of four Mediterranean species. Sci. Total Environ. 871, 161991. doi: 10.1016/j.scitotenv.2023.161991
Rix L., Ribes M., Coma R., Jahn M. T., de Goeij J. M., van Oevelen D., et al. (2020). Heterotrophy in the earliest gut: a single-cell view of heterotrophic carbon and nitrogen assimilation in sponge-microbe symbioses. ISME J. 14, 2554–2567. doi: 10.1038/s41396-020-0706-3
Roberty S., Béraud E., Grover R., Ferrier-Pagès C. (2020). Coral productivity is co-limited by bicarbonate and ammonium availability. Microorganisms 8, 640. doi: 10.3390/microorganisms8050640
Rodolfo-Metalpa R., Richard C., Allemand D., Ferrier-Pagès C. (2006). Growth and photosynthesis of two Mediterranean corals, Cladocora caespitosa and Oculina patagonica , under normal and elevated temperatures. J. Exp. Biol. 209, 4546–4556. doi: 10.1242/jeb.02550
Roemmich D., McGowan J. (1995). Climatic warming and the decline of zooplankton in the California current. Science 267, 1324–1326. doi: 10.1126/science.267.5202.1324
Rohwer F., Seguritan V., Azam F., Knowlton N. (2002). Diversity and distribution of coral-associated bacteria. Mar. Ecol. Prog. Ser. 243, 1–10. doi: 10.3354/meps243001
Rossi S., Bramanti L., Gori A., Orejas C. (2017). “An overview of the animal forests of the world,” in Marine Animal Forests: The Ecology of Benthic Biodiversity Hotspots. Eds. Rossi S., Bramanti L., Gori A., Orejas C. (Cham: Springer International Publishing), 1–26. doi: 10.1007/978-3-319-17001-5_1-1
Rossi S., Rizzo L. (2020). “Marine animal forests as carbon immobilizers or why we should preserve these three-dimensional alive structures,” in Perspectives on the Marine Animal Forests of the World. Eds. Rossi S., Bramanti L. (Cham: Springer International Publishing), 333–400. doi: 10.1007/978-3-030-57054-5_11
Rossi S., Rizzo L. (2021). The importance of food pulses in benthic-pelagic coupling processes of passive suspension feeders. Water 13, 997. doi: 10.3390/w13070997
Rossi S., Schubert N., Brown D., Gonzalez-Posada A., Soares M. O. (2020). Trophic ecology of Caribbean octocorals: autotrophic and heterotrophic seasonal trends. Coral Reefs 39, 433–449. doi: 10.1007/s00338-020-01906-w
Rossi S., Schubert N., Brown D., Soares M., de O., Grosso V., et al. (2018). Linking host morphology and symbiont performance in octocorals. Sci. Rep. 8, 12823. doi: 10.1038/s41598-018-31262-3
Sánchez-Pérez E. D., Pujo-Pay M., Ortega-Retuerta E., Conan P., Peters F., Marrasé C. (2020). Mismatched dynamics of dissolved organic carbon and chromophoric dissolved organic matter in the coastal NW Mediterranean Sea. Sci. Total Environ. 746, 141190. doi: 10.1016/j.scitotenv.2020.141190
Santos T. B., Reimer J. D., Acuña F. H., Stampar S. N. (2020). Diversity of feeding in anthozoa (Cnidaria): A systematic review. Diversity 12, 405. doi: 10.3390/d12100405
Schubert N., Brown D., Rossi S. (2016). “Symbiotic versus non-symbiotic octocorals: physiological and ecological implications,” in Marine Animal Forests. Eds. Rossi S., Bramanti L., Gori A., Orejas Saco del Valle C. (Cham: Springer International Publishing), 1–32. doi: 10.1007/978-3-319-17001-5_54-1
Segura-Noguera M., Cruzado A., Blasco D. (2016). The biogeochemistry of nutrients, dissolved oxygen and chlorophyll a in the Catalan Sea (NW Mediterranean Sea). Sci. Mar. 80, 39–56. doi: 10.3989/scimar.04309.20A
Shirur K. P., Ramsby B. D., Iglesias-Prieto R., Goulet T. L. (2014). Biochemical composition of Caribbean gorgonians: Implications for gorgonian — Symbiodinium symbiosis and ecology. J. Exp. Mar. Biol. Ecol. 461, 275–285. doi: 10.1016/j.jembe.2014.08.016
Sini M., Kipson S., Linares C., Koutsoubas D., Garrabou J. (2015). The Yellow Gorgonian Eunicella cavolini: Demography and Disturbance Levels across the Mediterranean Sea. PloS One 10, e0126253. doi: 10.1371/journal.pone.0126253
Smith P. K., Krohn R. I., Hermanson G. T., Mallia A. K., Gartner F. H., Provenzano M. D., et al. (1985). Measurement of protein using bicinchoninic acid. Anal. Biochem. 150, 76–85. doi: 10.1016/0003-2697(85)90442-7
Tanaka Y., Miyajima T., Umezawa Y., Hayashibara T., Ogawa H., Koike I. (2009). Net release of dissolved organic matter by the scleractinian coral Acropora pulchra. J. Exp. Mar. Biol. Ecol. 377, 101–106. doi: 10.1016/j.jembe.2009.06.023
Tanaka Y., Miyajima T., Watanabe A., Nadaoka K., Yamamoto T., Ogawa H. (2011). Distribution of dissolved organic carbon and nitrogen in a coral reef. Coral Reefs 30, 533–541. doi: 10.1007/s00338-011-0735-5
Tang J., Ni X., Wen J., Wang L., Luo J., Zhou Z. (2020). Increased Ammonium Assimilation Activity in the Scleractinian Coral Pocillopora damicornis but Not Its Symbiont After Acute Heat Stress. Front. Mar. Sci. 7. doi: 10.3389/fmars.2020.565068
Tignat-Perrier R., van de Water J. A. J. M., Guillemain D., Aurelle D., Allemand D., Ferrier-Pagès C. (2022). The effect of thermal stress on the physiology and bacterial communities of two key mediterranean gorgonians. Appl. Environ. Microbiol. 88, e02340–e02321. doi: 10.1128/aem.02340-21
Van De Water J. A. J. M., Melkonian R., Voolstra C. R., Junca H., Beraud E., Allemand D., et al. (2017). Comparative assessment of mediterranean gorgonian-associated microbial communities reveals conserved core and locally variant bacteria. Microb. Ecol. 73, 466–478. doi: 10.1007/s00248-016-0858-x
Van De Water J. A. J. M., Voolstra C. R., Rottier C., Cocito S., Peirano A., Allemand D., et al. (2018). Seasonal stability in the microbiomes of temperate gorgonians and the red coral corallium rubrum across the mediterranean sea. Microb. Ecol. 75, 274–288. doi: 10.1007/s00248-017-1006-y
Weinberg S. (1976). Revision of the common Octocorallia of the Mediterranean circalittoral. I. Gorgonacea. Beaufortia 24, 63–104.
Wooster M. K., McMurray S. E., Pawlik J. R., Morán X. A. G., Berumen M. L. (2019). Feeding and respiration by giant barrel sponges across a gradient of food abundance in the Red Sea. Limnol. Oceanogr. 64, 1790–1801. doi: 10.1002/lno.11151
Xiang T., Lehnert E., Jinkerson R. E., Clowez S., Kim R. G., DeNofrio J. C., et al. (2020). Symbiont population control by host-symbiont metabolic interaction in Symbiodiniaceae-cnidarian associations. Nat. Commun. 11, 108. doi: 10.1038/s41467-019-13963-z
Keywords: carbon, dissolved nutrients, gorgonians, heat stress, heterotrophy, marine animal forest, nitrogen, symbiosis
Citation: Lange K, Maguer J-F, Reynaud S and Ferrier-Pagès C (2023) Nutritional ecology of temperate octocorals in a warming ocean. Front. Mar. Sci. 10:1236164. doi: 10.3389/fmars.2023.1236164
Received: 07 June 2023; Accepted: 30 October 2023;
Published: 20 November 2023.
Edited by:
Linda Wegley Kelly, University of California, San Diego, United StatesReviewed by:
Randi D. Rotjan, Boston University, United StatesCopyright © 2023 Lange, Maguer, Reynaud and Ferrier-Pagès. This is an open-access article distributed under the terms of the Creative Commons Attribution License (CC BY). The use, distribution or reproduction in other forums is permitted, provided the original author(s) and the copyright owner(s) are credited and that the original publication in this journal is cited, in accordance with accepted academic practice. No use, distribution or reproduction is permitted which does not comply with these terms.
*Correspondence: Christine Ferrier-Pagès, ZmVycmllckBjZW50cmVzY2llbnRpZmlxdWUubWM=
Disclaimer: All claims expressed in this article are solely those of the authors and do not necessarily represent those of their affiliated organizations, or those of the publisher, the editors and the reviewers. Any product that may be evaluated in this article or claim that may be made by its manufacturer is not guaranteed or endorsed by the publisher.
Research integrity at Frontiers
Learn more about the work of our research integrity team to safeguard the quality of each article we publish.