- 1Stazione Zoologica Anton Dohrn, Department of Ecosustainable Marine Biotechnology, Napoli, Italy
- 2Department of Biology, University of Naples Federico II, Complesso Universitario di Monte Sant’Angelo, Naples, Italy
- 3Department of Earth, Environmental and Life Sciences (DISTAV), University of Genoa, Genova, Italy
- 4Institute for Environmental Protection and Research (ISPRA), Rome, Italy
- 5Stazione Zoologica Anton Dohrn, Department of Ecosustainable Marine Biotechnology, Ischia Marine Centre, Naples, Italy
Sponge farming has been experimentally performed for more than 100 years, with early attempts mainly devoted for the supply of bath sponges and for ornamental purposes. During the last decades, sponge farming has been proposed to produce biomass specifically for those species from which many structurally diverse bioactive compounds were isolated, frequently present in a low concentration that limits their commercial production. This point is very important because it offers an environmental-friendly approach for the use of sponges as a source of natural compounds for pharmacological, cosmeceutical, and nutraceutical industries. In addition, sponges can have an ecological role as filter-feeding animals with a great significance in marine benthic communities. Thanks to their aquiferous system, they can filter large amounts of sea water, retaining up to 80% of suspended particles, resulting in a good system to bioremediate the marine environment from different contaminants. Remarkably, few attempts at integrating aquaculture systems were performed by combining the increase in sponge biomass and their use for bioremediation, showing impressive results and opening new possibilities in the aquaculture sector. This review concerns both in situ and lab-based aquaculture methods for the production of sponge biomass and for the sponge-related bioremediation of the marine environment focusing on microorganisms and contaminants (heavy metals, pesticides, microplastics, and others). Moreover, a first overview about integrated aquaculture combining biomass increase and bioremediation, as a challenging perspective for marine biotechnologies, is included.
1 Introduction
Marine sponges are pluricellular, benthic, mostly sessile, and filter-feeding organisms that use flagellated cells (called choanocytes) to pump a unidirectional water current through its body. Sponges exhibit multiple shapes, can colonize any aquatic environment (marine, brackish, and fresh waters), and have a wide distribution, in terms of both geographic distribution (from polar to tropical waters) and bathymetric extension (from intertidal zones to thousands of meters depths). The life of a sponge centers around pumping a high volume of water through the pores (ostia), canals, and chambers (leuconoid condition), which conduct the water current from the inhalant sponge surface to the exhalant apertures called oscules (Figure 1) (Webster, 2007).
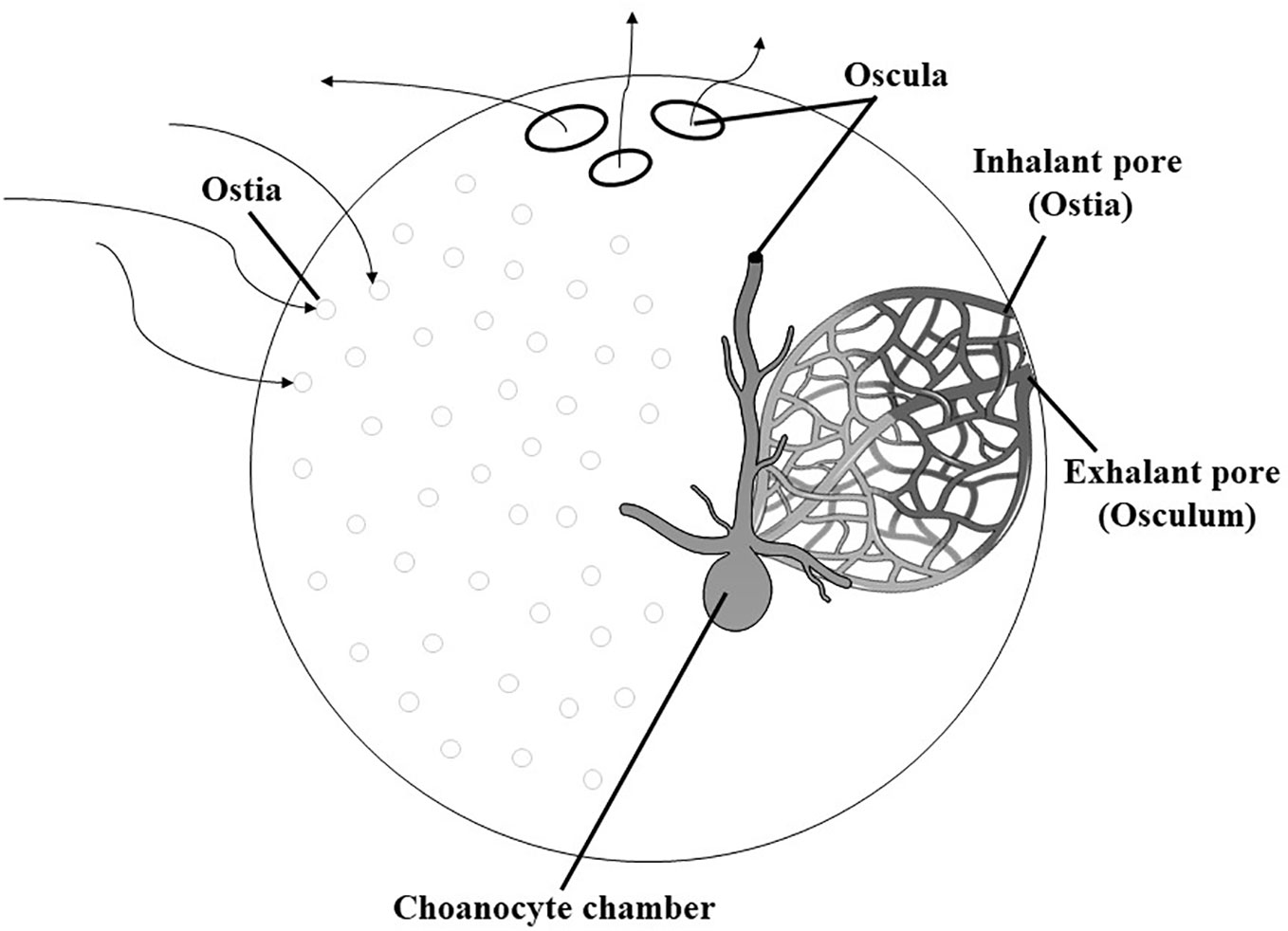
Figure 1 Morphology and aquiferous system of sponge (leuconoid condition) (modified from Webster, 2007).
Marine sponges have extremely high capability to filter huge amounts of sea water, ranging from 0.002 to 0.84 mL/s cm3 of sponge tissue, through their aquiferous canal system (Osinga et al., 1999). They also have a good capability to retain particles, such as dissolved organic matter, phytoplankton, bacteria, and various pollutants, with a wide size range (between 0.1 and 50 µm) (Fu et al., 2007). For these reasons, the use of these marine organisms in aquaculture system represents an efficient method to bioremediate from Total Organic Carbon (TOC). Bioremediation is an option that provides the ability to destroy or neutralize various contaminants using natural biological activity. Despite the limits of this procedure as the restricted range of contaminants and period of action are relatively long, the methods used for bioremediation are inexpensive and suitable to be performed on-site (Kensa, 2011).
There are various mechanisms for bioremediation: (i) bioadsorption by sponge-associated bacteria, which are capable of chelating heavy metals as copper, zinc, cadmium, and lead (Satyanarayana et al., 2012; Marzuki et al., 2020; Tanvi et al., 2020; Marzuki et al., 2023); (ii) degradation of plastics by bacteria associated with sponges (free living or associated with sponges) through intracellular and extracellular depolymerase enzymes that help to break down the plastic polymer into shorter and water soluble chains, while these chains enter the microbial cell and are metabolized by the intracellular enzymes (Bano et al., 2017; Fallon and Freeman, 2021; Fu et al., 2023; Krikech et al., 2023); and (iii) bioaccumulation and biodegradation by microalgae associated with sponges to remove pesticides through the production of antioxidant enzymes that activate the detoxification protection mechanisms of microalgae (Chu et al., 2023).
Marine sponges, together with their sponge-associated microbiota, represent a huge source of compounds with various biological and biotechnological activities such as antiviral, anticancer, antifouling, antimicrobial, antiplasmodial, antifungal, antioxidant, antiaging, skin whitening, and wound healing as reviewed in Esposito et al (2021; 2022a; 2022b). and Amelia et al. (2022). In particular, sponges evolved a sophisticated chemical communication and defense system based on secondary metabolites (Müller et al., 2004; Hogg et al., 2010), which safeguard them for example against fouling (Proksch, 1994), predation (Becerro, 1997), and microbial infection (Sipkema et al., 2005a). For most sponge-derived bioactive compounds, it is not clear whether they are produced by the sponge itself [as, for example, the avarol isolated from the choanocytes of Dysidea avara (Schmidt, 1862) (Uriz et al., 1996) and the stevensine from the archaeocytes of Axinella corrugata (George & Wilson, 1919) (ex Teichaxinella morchella) (Andrade et al., 1999), both used in medicine as antitumor and antibacterial agents], by the associated microorganisms (which can contribute up to 40% of the sponge volume), or by the interaction sponge-associated microbial communities (Li et al., 2023).
Once a bioactive compound for biotechnological applications in pharmacological, nutraceutical, and cosmeceutical fields has been isolated and identified from sponges, one of the major limitations in its use consists in obtaining sufficient material for preclinical and clinical trials leading to its commercialization (Steinert et al., 2018). Since many marine compounds are found in nature in small amounts, tons or thousands of tons of fresh biomass can be required to provide adequate amounts. It is obvious that such large quantities of biomass can never be harvested from nature without risking serious damage to the sponge population or even the extinction of the respective species (Wijffels, 2008).
The controlled chemical synthesis of the bioactive molecules (or their analogs) is the preferred production method for pharmaceutical application, but most bioactive natural compounds have chemically complex structures, which cannot be synthesized easily (Lindequist, 2016). Investments in establishing synthetic pathways for complex molecules are usually not made until the potency of the compound has been fully demonstrated (i.e., after completion of development studies or preclinical and clinical trials). In the case of many bioactive compounds isolated from marine sponges, for instance, cytarabine was isolated from Tethya crypta (Cappello and Nieri, 2021).
The culture of marine sponges would be another alternative to produce the bioactive compounds to overcome this supply issue (e.g., Dumdei et al., 1998; Mendola, 2003; Mendola et al., 2008; Gökalp et al., 2021; Maslin et al., 2021; Urban-Gedamke et al., 2021; Hesp et al., 2023), but, until now, many marine sponges are considered unculturable or difficult to cultivate. This paradox, which is often referred to as “The Supply Issue” (e.g., Munro et al., 1999; Osinga et al., 1999; Faulkner, 2000; Kobayashi and Fusetani, 2000), emphasizes the need to implement culture techniques. Since biological production is particularly essential for early drug development, the main challenge for marine biotechnologists is to establish common techniques for efficient biomass production of marine sponges on small and medium scales. Depending on the product of interest, different cultivation approaches may be applied to overcome “The Supply Issue”. When product concentrations are high inside the sponge or if the sponge itself is the product (e.g., bath sponges), cultivation of adult sponges becomes the best option (Sipkema et al., 2005b).
In this review, we focused on different usages of marine sponges: (i) aquaculture methods, aiming to produce sponge biomass; (ii) use of sponges to bioremediate the marine environment from pathogen microorganisms and contaminants [i.e., heavy metals; pesticides; microplastics (MPs), so defined because of their size of less than 5 mm; and other contaminants]; (iii) first attempts of integrated aquaculture to combine biomass increase and bioremediation. Our bibliographic research revealed that in the last years, the sponges were extensively used for bioremediation of marine environment. In addition, there were several research projects aiming to cultivate sponges in situ and few attempts were performed to cultivate sponges in laboratory, mainly due to the very different environmental conditions in which they live (Figure 2).
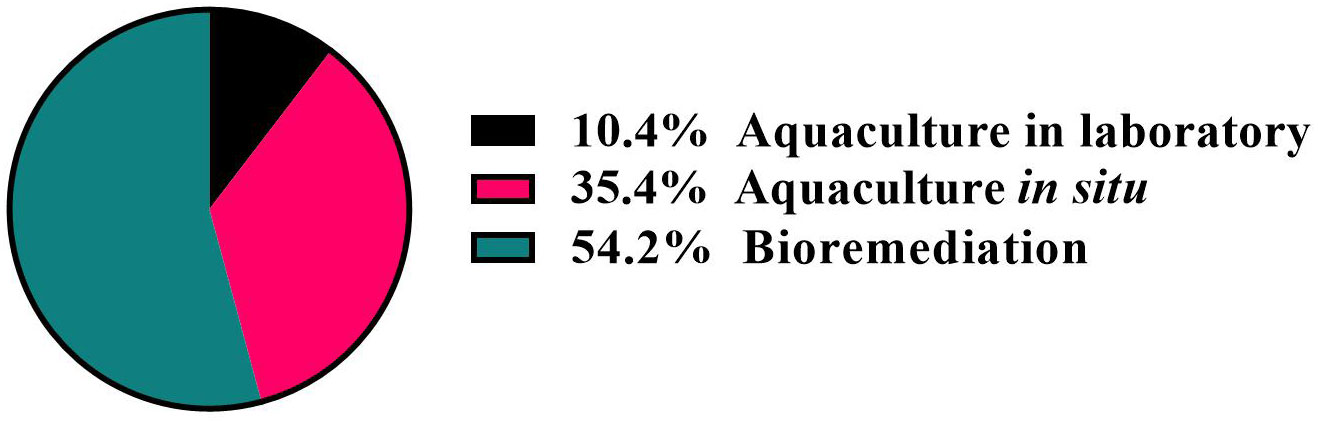
Figure 2 Results of bibliographic research on the use of marine sponges for bioremediation as well as in aquaculture in situ and in laboratory.
2 Aquaculture of sponges
Sponge cultivation was firstly thought to be a really hard objective because of the different environmental conditions in which the sponges live. Captivity culture could be a game changer when it comes to understanding which factors trigger the best growth of the sponges, because in a closed system, scientists can keep different parameters under control, such as salinity, temperature, water flow, light, nutrients, rapid spreading of microbes, and eventual diseases, also changing one parameter per time (Maslin et al., 2021). Many culture approaches have been used in recent years; for instance, sponge samples might be cut into smaller pieces (called “explants”) or cultured as whole individuals without any fragmentation and eventually the pieces were attached to a variety of substrates. Furthermore, we can distinguish among two main culture conditions: ex situ when sponges are cultured in captivity and in situ when individuals are grown in nature.
2.1 Cultivation in laboratory
Among sponge species, the first to be cultured in laboratory were Halichondria (Halichondria) panicea (Pallas, 1766), thanks to its ability to adapt filtration rates and oscula dynamics to the change flow and feeding conditions (Langenbruch, 1983; Maslin et al., 2021). A continuous-flow system in laboratory was set up for growing the freshwater sponges Ephydatia fluviatilis (Linnaeus, 1759) and Spongilla alba (Carter, 1849) from gemmules (Poirrier et al., 1981). The last two freshwater sponges were kept in continuous water flow at which known quantity of the bacteria Escherichia coli was added every 12 h at a concentration of 5 × 105 cells/mL. Not surprisingly, an increase in bacterial concentration also led to an increase in sponge growth (Simpson, 1963; Poirrier et al., 1981).
Microalgae are among the most widely used organisms as live feed for sponges, even if very motile cells (such as Dunaliella sp.) seem not to be adequate as feed for sponge fragments, which have been just excised from the wild donors since their aquiferous system has to be rearranged to be fully functional (Nickel and Brümmer, 2003) (Table 1). This process takes place because, depending on the position from which the fragment is excised (sponge cortex or choanosome), an efficient filtering system has to be reorganized in order to have a correct filtration. In fact, the reorganization of the aquiferous system is a key element in the sponge’s asexual reproduction (Ereskovsky, 2003; Nickel and Brümmer, 2003).
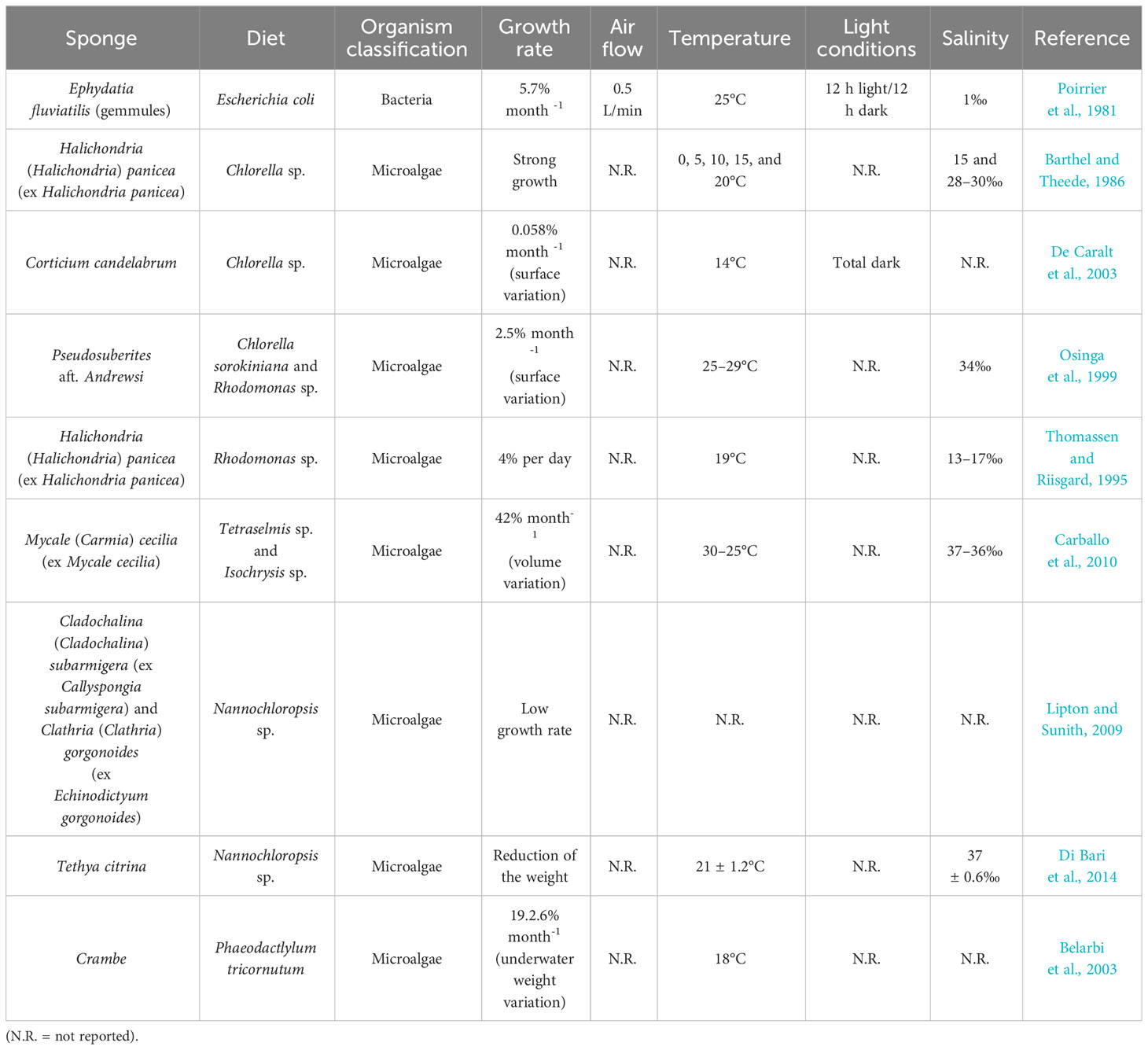
Table 1 Sponge species cultivated in laboratory, different diets used, growth (in percentage, only when reported in the reference), and references.
Between the first attempts using microalgae as sponge feed, there is one conducted in 1986 on the species Halichondria panicea. In this experiment, the concentrations of 2 × 107 and 3 × 107 cells per mL of Chlorella sp. were used as feed. Some cuttings were tied up to a nylon thread and others were tied by a nylon thread to glass slides. The best results were obtained when sponges were cultivated on the nylon threads, probably because this method is less manipulative compared to the other one, both in laboratory and in situ experiments (Barthel and Theede, 1986). Similarly, in 2003, the same feed (Chlorella sp.) at lower concentration (105 cells per mL) was used for the growth of Corticium candelabrum (Schmidt, 1862), a Mediterranean Demospongiae capable of asexual reproduction (De Caralt et al., 2003).
Osinga et al. (1999), instead of just one species, used a mixture of two species, Chlorella sorokiniana and Rhodomonas sp., in the concentrations of 107 cells/mL and 106 cells/mL, respectively. In the same study, it has been observed that the microalgal cells were absorbed and then digested by sponge cells, confirming previous observations already made in two other studies in which the same algae were used as feed separately (Barthel and Theede, 1986; Thomassen and Riisgard, 1995; Osinga et al., 1999). A similar approach was used by Carballo et al. (2010), where a mixture of two microalgae, Tetraselmis sp. and Isochrysis sp. and powdered yeast Saccharomyces cerevisiae, was used to grow specimens of Mycale (Carmia) cecilia (de Laubenfels, 1936) (ex Mycale cecilia). Specimens were cultured both in tanks and in situ. Despite the variety of feeds provided in the laboratory, the explants kept in natural conditions grew better. In fact, an increase of 207% of the volume was recorded compared to 65% growth recorded in the tanks in a 60-day trial (Carballo et al., 2010).
Nannochloropsis sp. demonstrated to be a valid candidate as microalgal feed for sponge species Callyspongia (Cladochalina) subarmigera (Ridley, 1884) (ex Callyspongia subarmigera) and Clathria (Clathria) gorgonoides (Dendy, 1916) (ex Echinodictyum gorgonoides) at the concentration of 3.5 × 106 cells (Lipton and Sunith, 2009). Some years later, in 2014, the same microalgae Nannochloropsis sp. was utilized as feed for the sponge Tethya citrina (Sarà & Melone, 1965), for which a solution of 300 mL of algae culture at the concentration of 4 × 107 cells/mL was daily supplied. These culture conditions caused a rearrangement of the aquiferous system due to the stress condition. Moreover, the sponge Tethya citrina showed an increase in the production of asexual buds probably because this diet was inadequate for this sponge species (Di Bari et al., 2014).
Interesting results were obtained using the diatom Phaeodactylum tricornutum as feed for the sponge Crambe crambe (Schmidt, 1862), where an increase of 1,380% of the initial volume was recorded in a continuous flow-through system in a 22- to 45-day trial, whereas the volume of the same explants grew 322% in 102–210 days with the same diet in a discontinuous flow-through system (Belarbi et al., 2003). Overall, as demonstrated by the different experiments, growth ex situ could be useful in sponge culture but only for some species, depending from their structure. For this reason, it was difficult until now to propose a standardized protocol to use. However, microalgae have revealed to be a promising feed for the culture in captivity of marine sponges.
2.2 Cultivation in situ
Many scientists attempted the culture in situ of sponges with different approaches, sometimes successful, while others give important advice on which can be the best culture method for each sponge species (Koopmans et al., 2009; Bierwirth et al., 2022). There are many methods based on culture on horizontal and vertical grids, in soft meshes, on threads or ropes, attached to a variety of substrates, and many others, which we will be described below (Figure 3). Various factors can influence the efficiency of the cultivation methods used, including water flow and temperature, adhesion substrates, and their position and orientation, as well as the cultivation site. Remarkably, sponge growth can be influenced by a combination of different factors, as demonstrated by several experiments reviewed below.
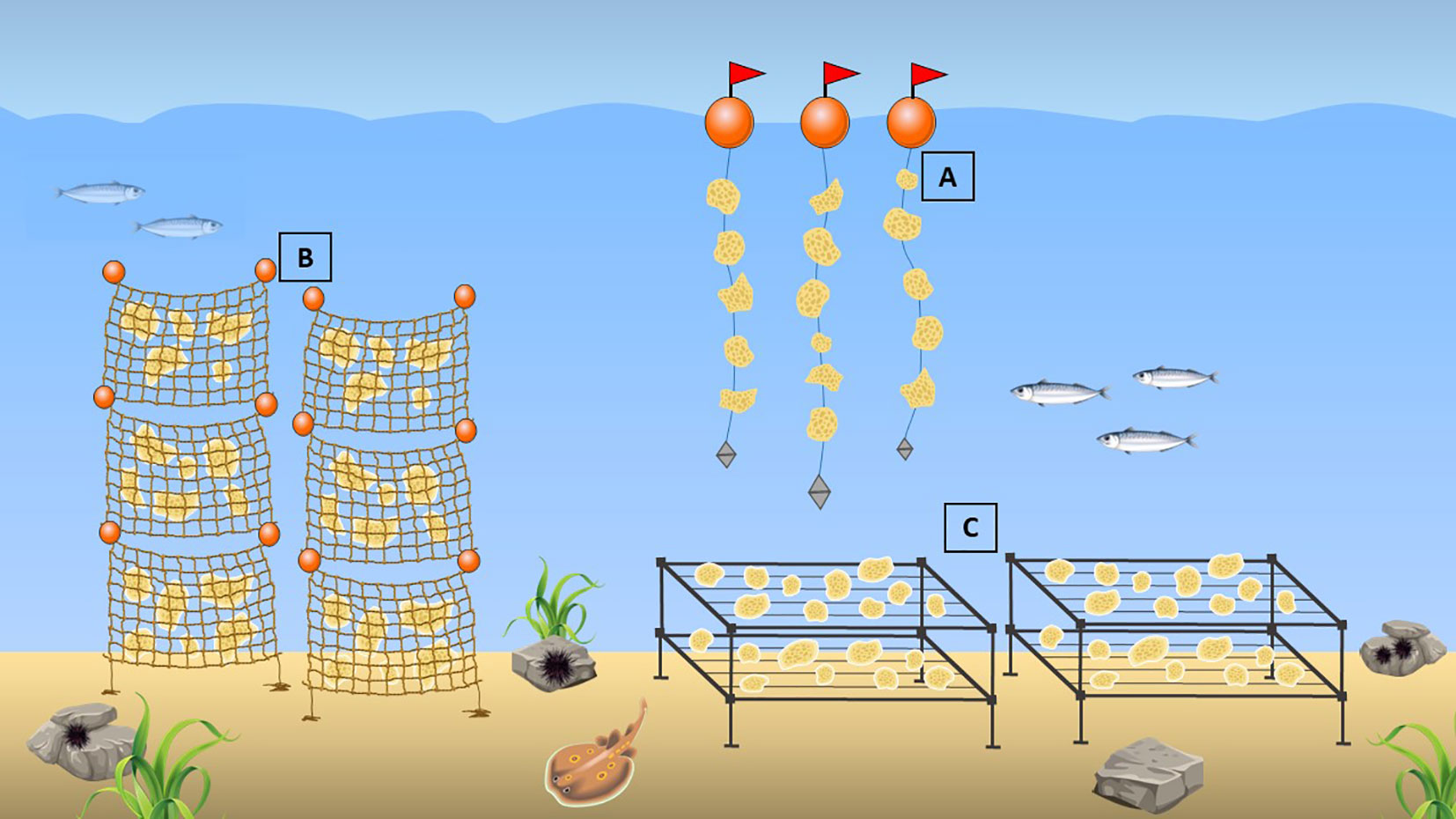
Figure 3 Aquaculture methods: (A) culture of sponges on suspended threads/ropes; (B) culture on vertical soft mesh bags; (C) culture on horizontal grids.
In the case of the water flow, it can increase the food availability in the water column. In addition, a stronger water flow on the sponge might bring more food, but this is not correlated linearly. Nonetheless, a faster water flow might stimulate the sponge to invest its energies in the enhancement of the skeletal structures to resist the perturbation, instead of investing them in the overall body growth (Palumbi, 1984; Duckworth, 2009). However, this is not always true. In fact, as demonstrated by Duckworth et al. (2004), the samples of Latrunculia (Biannulata) wellingtonensis (Alvarez et al., 2002) (ex Latrunculia wellingtonensis) and Polymastia crocea (Kelly-Borges & Bergquist, 1997) (ex Polymastia croceus) exposed to high-flow conditions grew better compared to the sites exposed to medium and slow flow sites. The results obtained for Latrunculia (Biannulata) wellingtonensis were of 103%, 93%, and 90% in the strong, medium, and slow flow sites, respectively. In contrast, for the sponge Polymastia crocea, the growth was slightly higher but the best culture conditions were obtained in the fast flow sites if compared to the medium and slow flow sites (125%, 102%, and 104%, respectively) (Duckworth et al., 2004). The different growth can be linked to the space and local hydrodynamic regime (Corriero et al., 2004). Interestingly, in the same study by Corriero et al. (2004), it was reported that mean growth was highly variable among cuttings and this is probably due to the different age of the wild donor: younger sponges grow faster than older ones (Corriero et al., 2004).
Regarding the substrates, they can vary according to the different species to be cultivated, and also their orientation can affect the outcomes of the experiments. Various methods were set up, such as attachment of sponge specimens to rocks, wooden boxes, or being stabbed with bamboo sticks fixed to a wheel kept in continuous movement (Moore, 1910). Usually, to start aquaculture trials in situ, the most diffuse approach was to pick up wild specimens and use them as donor sponges, from which smaller fragments were cut out and called “explants”. Once these were obtained, the cultivation can be started as they can be attached to a wide variety of artificial substrates, suspended on threads, or retained in mesh bags or cages (Schippers et al., 2012).
One of the first attempts were done many years ago by Crawshay (1939), in which he used the so-called bath sponges. Specimens of wool sponges were cut in smaller pieces and placed in the sea of the Island of Abaco (Bahamas) on concrete discs. An initial weight loss was observed (neglectable), and intriguingly, when conditions became favorable, the volume of some samples tripled in just 12 months (Crawshay, 1939).
Several attempts were done to test different substrates to check which one was the best for the cultivation of Crambe crambe explants. Both substrates taken into account were travertine tiles and oyster shells, while the nylon thread was not used for the cultivation because, according to Padiglia et al. (2018), this kind of substrate is not suitable for an encrusting sponge. Not surprisingly, the best substrate since the sponge growth was the oyster shells because these are natural biogenic materials and the larval settlement takes place preferentially on calcareous substrates such as shells of oysters and other bivalves, crabs’ carapaces, and coralline algae (Padiglia et al., 2018).
Different volumes of Coscinoderma sp. were cultured with different methods (mesh, mesh line and threaded line) along Torres Strait (Australia) by Duckworth and Wolff (2007), demonstrating that mesh panels were the best support for the culture of this species combined with the explant size of 100 cm3. Corriero et al. (2004) tested explants of the sponge species Spongia (Spongia) officinalis (Linnaeus, 1759) (ex Spongia officinalis var. adriatica) and these did not show any significant difference between the two culture methods applied (culture on horizontal and vertical rearing structures), or on the initial explant dimensions as for other species (Palumbi, 1984; Duckworth, 2009). It was also demonstrated that this species (S. officinalis var. adriatica) does not have any preference on the orientation of the rearing structures (Corriero et al., 2004).
In a different study by Bergman et al. (2011) on samples of Diacarnus erythraenus (Kelly-Borges & Vacelet, 1995) planted on polyvinyl chloride (PVC) plates on different depths, a check of stability of the bacterial assemblage was carried out to understand if the sponge was in good health. Despite the difference in the cultivation depth (10 and 20 m) no significant change was noticed in growth rates or in seasonal growth. PVC plates were also used for the cultivation of specimens of Chondrosia reniformis (Nardo, 1847) on which sponges were attached and subsequently placed on a horizontal plate (Gökalp et al., 2019). This study also demonstrated how the sponges can grow up better in the proximity of fish aquaculture cages. Intriguingly, both the survival rates and growth were higher next to the aquaculture plans (86% and 170%, respectively) (Gökalp et al., 2019). Other experiments conducted on this species were devoted to optimize the culture protocol, demonstrating that the optimal growth temperature for Chondrosia reniformis was 25°C (Orel et al., 2021). Explants of Chondrosia reniformis were attached on polypropylene (PP) supports placed on horizontal lanterns in two sites: one under the influence of organic pollution (urban water discharge) and the other one on the pristine site. The results showed that growth rate was similar between the two sites (126% ± 99% and 218% ± 139%, respectively) (Gökalp et al., 2022). This might sound strange but previous studies have demonstrated that sponges can capture up to 70% of suspended bacteria and organic material (Pronzato et al., 1998) and it was specifically demonstrated that Chondrosia reniformis sponge can remove bacteria such as E. coli from the environment (Milanese et al., 2003).
Sponges of the species Dysidea avara were hung on horizontal ropes together with culture in cages and attachments of the pieces of the sponge to rigid frames with glue. This time, hanging from ropes was not as effective as when specimens were attached with the glue. Although this method led to more losses and death, it was effective in terms of growth rate and natural toxicity. The latter was measured using the Microtox® (Microbics, Carlsbad, CA, USA) method, which is based on the detection of the bioluminescence emitted by the bacterium Photobacterium phosporeum. When this species was placed in contact with the extract, the bioluminescence decreased (De Caralt et al., 2010). In another study in the same year, the same sponge species was cultivated using a method that has been named as the homonymous Turkish dish: “Shish Kebab”. Sponges were speared from side to side with metal wires just like a kebab, which were then attached to a horizontal support at different depths and in the proximity of a fish farm. Consistently with the distribution patterns of this sponge, the best survival and regeneration rates were obtained in the site in which the water was more turbid, this because light is an inhibiting factor (Osinga et al., 2010).
Differently from the methods tested by Schiefenhövel and Kunzmann (2012); Kiruba Sankar et al. (2016) cultivated specimens of Liosina paradoxa and Stylissa massa (Carter, 1887) in cages testing for the suitability of four different substrates: concrete blocks, coral rocks, tile, and rope. The results showed that there was no substantial preference from the sponge because it grew on all the four substrates, but the highest growth was recorded on coral rock substrate.
Reliant supports were also used by Baldacconi et al. (2010) to check for transplantation and restoration studies on the species Spongia officinalis, aiming to bring back this sponge in areas where it was present before. The transplantation experiments consisted of moving whole individuals or pieces of sponges. The results were promising because in the 12 months in which the experiment was carried out, a survival rate of 100% was recorded. In addition, larvae released from the transplanted individuals were not significantly different from the ones produced by the donor stock in the sampling site (Baldacconi et al., 2010).
Sponges belonging to the Negombata magnifica (Keller, 1889) species were cultured by Hadas et al. (2005) using two different approaches: nylon threads and plastic nets. Once again, the thread method revealed its efficiency also because the plastic nets underwent a process of fouling, in which other marine organisms competed with the cultivated sponge for space and feeding, but most importantly, the fouling process could reduce water circulation in the nets and interfere with the sponge filtration rate (Duckworth, 2009). This problem could be easily overcome with a cleaning of the nets every now and then, but this process would be expensive in terms of money and time (Hadas et al., 2005).
A study conducted some years later by Ruiz et al. (2013) on a different sponge [Discodermia dissoluta (Schmidt, 1880)] used soft mesh bags. The results showed a moderate volume growth of approximately 28%, but more interestingly, the bioactive molecule (+)-discodermolide synthesis was not only retained but the concentration of the molecule increased by 33% (Ruiz et al., 2013). Similar results were also obtained for the sponge Ircinia variabilis (Schmidt, 1862), for which an increase of 200% of the biomass was recorded after just 1 year of in situ mariculture (Van Treeck et al., 2003). In the same study, the suitability for the mesh method for two Axinella species was tested [Axinella verrucosa (Esper, 1794) and Axinella cannabina (Esper, 1794)] but significant losses and almost no growth was observed, indicating that the conditions in which the sponges were cultivated are not suitable and the mariculture method has to be changed (Van Treeck et al., 2003). The mesh method was also used for the culture of cuttings of Mycale (Carmia) hentscheli (Bergquist & Fromont, 1988) (ex Mycale hentscheli) where explants seemed to thrive, recording an increase in the wet weight up to 3,365% in 8 months. The growth was measured using both an underwater video camera (Hi-8 digital video camera) and increasing the wet weight at the beginning and at the end of the trial. In this study, it is also reported that only the cuttings that grew into the native site of the wild donors retained the biosynthetic activity of the active molecule, indicating that probably the synthesis of this compound might be environmentally controlled (Page et al., 2005).
Specimens of Neopetrosia sp. were cultivated using both grids oriented horizontally and suspending the cuttings on nylon threads (Schiefenhövel and Kunzmann, 2012). The best growth was obtained when the sponges were suspended on the nylon ropes recording a growth of 27%–35%. In the same study, the authors also tested the growth of Stylissa massa and concluded that there was no difference among the cuttings that grew on nylon threads and those on the grids (Schiefenhövel and Kunzmann, 2012). In conclusion, the various outcomes about sponge culture in meshes might be dependent, as it has been hypothesized by Duckworth et al. in 2004, by the sponge body structure. This means that sponges with relatively soft structure and low spicule density grew better through mesh holes, whereas sponges with a well-developed choanosome and more dense spicules could not grow much because their growth space was limited to the mesh pouch (Duckworth et al., 2004).
In the case of water temperature, several lines of evidence demonstrated that the transplant is more effective when the temperature is cooler, because the external layer (pinacoderm) healing after the cutting is stimulated whereas bacterial growth is delayed, leading to less frequent infections (Duckworth et al., 1997; Duckworth and Wolff, 2007; Duckworth, 2009). An experiment conducted in 2004 demonstrated this hypothesis. In fact, specimens of Latrunculia (Biannulata) wellingtonensis and Polymastia crocea had lower survival rates when moved in summer with higher water temperatures (Duckworth et al., 2004).
Also, specimens of Geodia cydonium (Linnaeus, 1767) did not show significant differences in the growth rate when cultivated in the proximity of a fish mussel farm or in the center of marine research basins with an open circulation system (Müller et al., 1999). The results obtained in this study demonstrated that, even though there were differences in the location, water composition, and environmental conditions, specimens of this sponge had no preference. Nonetheless, after 3 and 6 months, the fragments showed a significant increase in size (153% and 190%, respectively) in an independent manner from the culture site (Müller et al., 1999). However, this is not always true. In fact, Kelly et al. (2004) demonstrated that culturing site influenced the growth of specimens of Spongia (Heterofibria) manipulatus (Cook & Bergquist, 2001) collected in New Zealand. In particular, after 13 months, this sponge cultivated in lanterns in Marlborough Sounds was larger in volume than the one cultivated in Coromandel with the same system.
2.3 Cultivation for biotechnological applications
One of the main aims for the aquaculture of marine sponges is to obtain, in a sustainable way, large biomasses from which to extract bioactive compounds for biotechnological application, avoiding depleting natural wild stocks.
Preliminary attempts were performed in laboratory such as the case reported by Nickel and Brümmer (2003), in which lyophilized suspension of E. coli was also used for the cultivation of the marine sponge Chondrosia reniformis, where specimens were previously cut into small pieces and then transferred in six-well plates. After the cutting took place, an almost immediate cell proliferation was noticed even though it happened slowly, thus indicating long cell cycles and low proliferation rates. The fragment rearing lasted 19 months and cellular density decreased in time, whereas an increase in the collagen production was recorded. An enhancement of the collagen production was also noticed when explants of the sponge Chondrosia reniformis were exposed to quartz dust suspension. This dust in humans triggers an overproduction of collagen in the lungs (silicosis), but this sponge is capable of incorporating the silica with an increase of collagen production, which can be extracted and used for biotechnological purposes (Giovine et al., 2013; Pozzolini et al., 2017).
A study on the efficiency of the aquaculture of Mycale hentscheli trying to understand if this sponge, after several explantations, was still capable of producing the bioactive compound for which it was chosen (Peloruside A) gave positive feedback (Page et al., 2011). Encouraging results were obtained in a recent study by Santiago et al. (2019) conducted in the Philippines, in which they attempted to culture explants belonging to the sponge species Xestospongia sp. to assess if harvesting period and farming method were responsible for a change in the growth and the survival rates, but also at which extent these factors can influence the production of bioactive metabolites. At the end of the study, as recorded in previous ones, sponges’ death takes place because of wrong cultivation conditions, such as predation, piece loss, and water flow being too strong or too low. On the other hand, the synthesis of the bioactive molecule isolated from this sponge, the Renieramycin, was retained all along the cultivation experiment and was not influenced by multiple harvesting and wounding but was mainly influenced by seasonality and location. In fact, the anti-proliferative compound content was higher in the explants in the period from May to January; instead, the content was lower in the period from January to May (Santiago et al., 2019). The maintenance of the bioactivity was also observed in samples of Callyspongia subarmigera, which in situ recorded a daily growth of approximately 88.94 mg, even though at the end of the culture time the bioactivity was still present, but it was not as strong as at the beginning of the culture trial (Lipton and Sunith, 2009).
An important factor influencing sponge growth is the microbiome, playing a key role in sponge adaptation to various ecological conditions (Cerrano et al., 2022). This is mainly because the sponges host a great number of microorganisms, which can be simply associated with them or have a symbiotic relationship (Esposito et al., 2022b). This microbial population is strictly influenced by environmental factors, such as water temperature, light, and salinity.
There is a long-standing debate in understanding if the sponges are responsible for the synthesis of the bioactive compounds or the microbiota that are associated with them, or if the bioactive compounds are synthetized by the interaction between sponge cells and microorganisms; hence, it is of fundamental importance to understand if transplantation may cause a change in the microbial community and eventually the loss of the bioactivity. For this reason, the study of sponge microbiome is receiving great attention from the scientific community (Moitinho-Silva et al., 2017).
An experiment on the effect of the transplantation of the sponge Aplysina cavernicola (Vacelet, 1959) on its microbial community from a deep site to a shallower one revealed that, after an observation of the samples under the transmission electron microscope, the microbial community remained unchanged after a cultivation time of 3 months. Nonetheless, the metabolite pattern was unchanged, even though the sponges underwent a transplantation process (Thoms et al., 2003). Bergman et al. (2011) used the Denaturing Gradient Gel Electrophoresis (DGGE) profiling to check the bacterial community associated with wild and maricultured Dysdera erythraenus. These showed slight differences between the banding profiles of the associated bacterial community, but they were not significant. Samples taken from wild stocks and mariculture shared over 80% of similarity. Neither the depth nor sampling dates seemed to affect the bacterial community.
In contrast, in explants of Halichondria (Halichondria) melanodocia (de Laubenfels, 1936) (ex Halichondria melanodocia), a change in the associated organism’s assemblage was noticed after some samples were transplanted from a habitat to another, but according to what is reported from the authors, factors such as host morphology and short-term variation in the associated microorganism population are also determining factors (Ávila and Briceño-Vera, 2018; Orel et al., 2021). Interestingly, in a study conducted in 2016 on Ecionemia alata (Dendy, 1924), it was demonstrated that a change in the bacterial taxa was truly happening, but it is most likely abundance shifts instead of proper bacterial diversity losses (Meyer et al., 2016).
3 Bioremediation by sponges
Among sentinel organisms, sponges and bivalves represent the most efficient filter feeders in the marine environment, as demonstrated by Longo et al. (2016). In this study, the sponge Hymeniacidon perlevis (Montagu, 1814) showed greater efficiency in removing microorganisms from the aquatic environment, even pathogenic ones, compared to the mussel Mytilus galloprovincialis. In particular, the authors concluded that, due to evident bacterial accumulation by the sponge, the co-occurrence of Hymeniacidon perlevis with M. galloprovincialis was a powerful tool to reduce bacterial loads in shellfish farming areas, thus playing a role in mitigating health risks associated with the consumption of edible mussels (Longo et al., 2016). Sponges act as biofilters accumulating toxic substances up to higher levels of the food chain (Fasulo et al., 2015; Gokalp, 2021). In particular, they can absorb pollutants especially through food (Carmen Casado-Martinez et al., 2010), suggesting a potential role of these organisms in bioremediation processes. In recent years, interest in marine sponges has increased as demonstrated by European Project Remedia Life (Borghese et al., 2022) and Italian Project Systems Biology (Fasulo et al., 2015).
Remedia Life envisaged an Integrated Multitrophic Aquaculture (IMTA) system in the city of Taranto, with various organisms (polychaetes, porifera, mussels, and macro algae) (Borghese et al., 2022). Different anthropogenic pollutants, such as heavy metals, microplastics, and polychlorinated biphenyls, were tested. In particular, for Sarcotragus spinosulus (Schmidt, 1862) sponges, the effects of heavy metals were analyzed following exposure to lead, and after 1 year, the sponges showed higher values than those considered non-dangerous in the scientific literature.(Borghese et al., 2022). The System Biology project, funded by the Italian Ministry of Education, University and Research, provided experiments in mesocosms with sea sentinels and different remediation actions on the matrices collected from contaminated areas (Fasulo et al., 2015).
3.1 Heavy metals
Among the various contaminants produced by anthropic activities that reach the aquatic environment, heavy metals, which usually have density greater than 5 g/cm3, are the most worrying ones (Holleman and Widerg, 1985). The most frequent toxic metals, as reported by Environment Protection Agency, are mercury, arsenic, cadmium, lead, nickel, chromium, copper, and zinc. Despite being responsible for “less visible pollution”, they have the dangerous ability to bioaccumulate along the food chain and cause negative effects and alterations of ecosystem balance such as reduction of the growth of organisms and increase in mortality and anomalies in development (Khayatzadeh and Abbasi, 2010). It is also important to consider that they can have effects on organisms exposed to single metals or mixture of metals with consequent synergistic effects (Baby et al., 2011).
Dos Santon Esteves (2009) demonstrated that a marine sponge of the genus Cliona was able to accumulate nickel. In particular, the sponges accumulated heavy metals in its free ionic form and is associated with a molecular mass of less than 3,000 Da, in amounts of approximately 1,300 ppm. The bacterium Symbiodinium was probably involved in the bioaccumulation mechanism. Similarly, Santos-Gandelman et al. (2014) showed the ability of the bacteria associated with the sponges to detoxify mercury. Some bacteria were resistant to mercury (Bacillus sp., Enterococcus sp., Oceanobacillus iheyensis, Virgibacillus, Bacillus cereus, Halobacillus, Ruegeria atlantica, Pseudomonas sp., and Halomonas), while others were highly resistant because they were able to grow also when exposed to 100 μM HgCl2 (some strains of Bacillus sp., Virgibacillus sp., Halobacillus sp., Pseudomonas sp., Halomonas sp., Brachybacterium sp., Psychrobacter sp., and Kocuria sp.), which grew on plates with 100 microns of mercury). Other bacteria had the merA gene, which allows bacteria to reduce ionic mercury to its elemental form (some strains of Bacillus sp., O. iheyensis, B. cereus, Halobacillus, R. atlantica, Pseudomonas sp., Halomonas sp., Brachybacterium, and Kukuria sp.) and still others produced biosurfactant.
Keren et al. (2015) showed that the sponge Theonella swinhoei (Gray, 1868) was able to accumulate high concentrations of arsenic up to 8,500 mg/kg, probably thanks to the presence of bacteria associated with the sponge participating in the arsenic cycle, such as Actinobacteria, Gammaproteobacteria, and Alphaproteobacteria. This result was confirmed by the fact that the authors demonstrated separating bacteria from the sponge that later accumulate in the metal. In the same way, Bauvais et al. (2015) demonstrated that marine sponge S. officinalis resisted in an aquatic environment the presence of heavy metals such as zinc, nickel, lead, and copper, with high levels of concentrations of these metals (minimal inhibitory concentration values ranging from 0.10 to 25 mM). Such ability was given by the bacteria associated with this sponge (Proteobacteria 64%, with a few Firmicutes and Actinobacteria and Pseudovibrio 54%).
Orani et al. (2018) studied the efficiency of bioremediating the aquatic environment from some heavy metals, focusing on the fact that different sponges accumulate elements at different concentrations depending on the bacteria associated with various species of sponges. For example, Axinella damicornis (Cymbaxinella damicornis) (Esper, 1794) accumulated more arsenic and copper (between 56 and 61 and 55–85 mg kg−1, respectively) compared to other species of sponges, Chondrilla nucula (Schmidt, 1862) accumulated more nickel and molybdenum, Acanthella acuta accumulated more silver, and Hymeniacidon perlevis accumulated trace elements more effectively than Halichondria panicea (Orani et al., 2018).
An interesting study of Marzuki et al. (2021) focused on the role of sponges in the biomonitoring of heavy metals such as chromium, cadmium, polycyclic aromatic hydrocarbons (PAHs), and naphthalene. The authors conducted experiments, which allowed them to evaluate the biodegradation power of these pollutants by the symbiotic bacteria in the sponges. Bacillus pumilus, Niphate sp., and Pseudomonas stutzeri strains isolated from sponge Hyrtios erectus (Keller, 1889) exhibited the ability to remove naphthalene, chromium, and cadmium in mixture. Ravindran et al. (2020) demonstrated that Bacillus sp. bacterial strain isolated from the marine sponge Agelas clathrodes (Schmidt, 1870), collected in Palk Bay (India), exhibited the capability to produce biosurfactants able to bind heavy metals with high affinity, such as lead, cadmium, mercury, and manganese. Dechsakulwatana et al. (2022) studied bacteria associated to sponges Mycale (Mycale) grandis (Gray, 1867) (ex Mycale grandis), Haliclona sp., Gelliodes sp., and Cacospongia sp., collected in Thailand, which have the ability to degrade petroleum and show tolerance to heavy metals. Sphingobium naphthae and Bacillus aryabhatta strains are capable of producing biosurfactants, of tolerating heavy metals, and of having enzymes to degrade hydrocarbons (64% for crude oil, 63% for diesel oil, and 51% for fuel oil degradation efficiencies for 2,000 mg L−1 within 7 days).
Orani et al. (2022) in a study on the bioaccumulation of rare earth elements (REEs) compared mercury and methylmercury concentrations between sponges sampled in the Mediterranean Sea and Atlantic Sea. Among the species collected, the one that showed the highest concentrations of mercury (0.5 mg kKg-1) was the Mediterranean species Chondrilla nucula. This ability was probably related to the different endobiotic microorganisms of the sponges, involved in methylation and demethylation processes indicating that the species Chondrilla nucula might be a valid candidate for bioremediation. Finally, Shoham et al. (2021) showed that the strains Alteromonas macleodii and Pseudovibrio ascidisceicola associated with the sponge Theonella swinhoei were able to reduce arsenic from the form of arsenate to the form of arsenite (iAsV and iAsIII) in a water environment, showing tolerance to this toxic metal and therefore useful for bioremediation.
Rosado Rodríguez and Otero Morales (2020) investigated the ability of sponges, sampled in Puerto Rico, to bioaccumulate heavy metals such as arsenic, cadmium, and copper. This study focused on the fungal communities associated with sponge Tedania (Tedania) ignis (Duchassaing & Michelotti, 1864) (ex Tedania ignis) and demonstrated that the accumulation of heavy metals could be negatively influenced by the association of this fungi on sponges. Hansen et al. (1995) suggested the use of the sponge Halichondria panicea for biomonitoring of heavy metals—copper, zinc, and cadmium—given the large volumes of water that the sponge filters. During 6 days of expositions to a concentration of 300 μg L−1 of copper, zinc, and cadmium, the final body metal concentrations were 236, 956, and 271 μg g−1 dry wt day−1 respectively, while when the sponges were exposed to a concentration of 1,000 μg L−1 of copper, zinc, and cadmium, the final body metal concentrations were 818, 956, and 985 μg g−1 dry wt day−1, respectively.
Ferrante et al. (2018) studied the capacity of the sponge Chondrilla nucula to bio-cleanup the marine environment from cadmium, copper, and lead. In vivo experiments have shown that the sponge efficiently accumulated copper but had a lower tolerance for high concentrations for cadmium and lead (0.08 and 0.8 mg L−1, respectively). After 80 h of exposure to these concentrations, signs of cell necrosis and decreased absorption rates were observed by the presence of white patches on the surface. Gravina et al. (2022) explored the effects of cadmium and lead on the sponge Axinella damicornis. This sponge was exposed for 144 h to concentrations of 253.7 μg/g for lead and 42.7 μg/g for cadmium during exposure to individual metals. In the contemporary exposure to the two metals, lead had a concentration of 162.7 μg/g, while cadmium had a concentration of 18 μg/g. Furthermore, the synergy of two metals determined more signals of stress and cellular necrosis. As reported above, microorganisms, and mainly bacteria, are strongly associated with sponges.
For this reason, they are considered to be responsible for the bioremediation mediated by the sponges, thanks to the presence of specific enzymes involved in oxidation and reduction reaction, such as alkane monooxygenase or cytochrome P450 (Dechsakulwatana et al., 2022).
3.2 Microorganisms
The high microbial load is another problem of the marine areas usually removed by applying bioremediation. Several studies in literature demonstrated the ability of sponges to eliminate pathogenic bacteria from the aquatic environment thanks to their filtering non-selectively on organic particles with a size between 0.1 and 50 μm such as phytoplankton, heterotrophic bacteria, heterotrophic eukaryotes, and detritus (Osinga et al., 1999).
Several studies have reported the capacity of marine sponges to function as bioremediators of microorganisms. Milanese et al. (2003) demonstrated that Chondrilla nucula had excellent bioremediation capabilities for the E. coli bacterium. The study conducted in the laboratory has shown that 1-m2 patch of this sponge can filter up to 14 L/h of sea water retaining up to 7 × 1010 bacterial cells/h. Fu et al. (2006) tested the ability of the sponge Hymeniacidon perlevis to remove bacteria E. coli and Vibrio anguillarum. The results showed that in sterilized natural seawater, the sponge can remove an average of 96% of E. coli within 10.5 h at a filter rate of ca. (7.53–8.03) × 107 cells/h × gram of fresh sponge. Furthermore, the bioremediation for V. anguillarum showed that 1.5 g of fresh sponges can control the growth of bacteria at an initial density of 3.6 × 104 cells/mL of 200 mL water volume. The species of sponge Hymeniacidon perlevis was used in the experiments of Zhang et al. (2010) in Dalian Heshengfeng Mariculture Farm (China). In addition, this sponge showed efficiency in removing different pathogenic bacteria in the intensive aquaculture water system of Scophthalmus maximus. The highest efficiency occurred at a temperature of 10–20°C, with maximal removal approximately 71.4%–78.8% of fecal coliform and approximately 73.9–98.7 of pathogenic Vibrio.
Also, the study by Maldonado et al. (2010) confirmed the ability of Hymeniacidon perlevis to be a bioremediatory for E. coli and V. anguillarum. In particular, it was demonstrated that this sponge had preferential ingestion for E. coli, probably due to the presence of the flagellum in V. anguillarum that makes its digestion more complex.
As reported by Stabili et al. (2006) also the sponges belonging to Spongia (Spongia) officinalis demonstrated bioremediation capabilities of microorganisms. In fact, the sponges decreased the concentration of bacteria, reducing in 1 h the maximum clearance rate to 210 mL h−1 g−1 of dry weight. Moreover, sponges removed mainly medium and large bacteria from the environment, suggesting that the size of the bacteria influenced the absorption rate of the sponges. Instead, Ledda et al. (2014) conducted field experiments on the Ligurian and Sardinian coasts with sponges Ircina variabilis and Agelas oroides (Schmidt, 1864). The sponges demonstrated filtration and clearance capability in all sites, especially those contaminated by suspended organic matter and pathogenic microorganisms, which had been divided according to their size into small size, medium size, and large size (<0.065 μg3, 0.065–0.320 μg3, and 0.320–0.780 μg3, respectively). After 4 months, the sponges deleted up to 80% of the bacterial charge from the water.
3.3 Microplastics
Interest and concern for microplastics (MPs) increased in the last years because of their ubiquity and persistence (Ryan et al., 2009). The tiny plastic debris was found in the oceans of the world, including the Antarctic Ocean (Zarfl and Matthies, 2010), and the water is the primary medium by which these MPs are transported (Alimi et al., 2018). Several studies showed that, after the treatment, residual plastics were discharged into rivers and oceans, entering the environment (Fu et al., 2019; Galafassi et al., 2019). There is still little information on the microbial degradation mechanisms of emerging contaminants, such as microplastics. It seems that microorganisms able to degrade microplastics colonize the surface and secrete their extracellular enzymes, which, in turn, degrade the polymers. A further step was the bio-assimilation of low-molecular-weight compounds by microorganisms and mineralization, obtaining CO2, H3O, and inorganic compounds (Tokiwa et al., 2009). To date, there are few studies on bioremediation of microplastics by marine sponges because of the size of the particles, which they manage to incorporate. In fact, Turon et al. (1997) studied the clearance rate between the sponge Crambe crambe and Dysidea avara, demonstrating that the most retained particles had a size of 1 micron and highlighting that sponges had a preference for filtering particles with small size and fibers (Karlsson et al., 2017).
Few studies are present in literature on bioremediation of MPs by sponges, considering that the sponges prefer to filter small particles of about <70 microns and the difficulty of growing sponges in laboratory (Fallon and Freeman, 2021). Bosker et al. (2018) evaluated the presence of MPs in Caribbean waters and found on average 261 ± 6 MPs/kg of dry sand, mostly represented by fibers. In the same way, Modica et al. (2020) explored the presence of MPs in marine sponges coming from Cantabrian Sea Maritime Museum and dating back more 20 years ago. Indeed, the presence of plastic was highlighted in more than half of the samples from the Atlantic Ocean, analyzing the shape of the body and demonstrating that this contaminant has been interacting with the sponges for more than two decades.
Soares et al. (2022) conducted an interesting study to determine the amount of MPs present in Cinachyrella alloclada (Uliczka, 1929) species, using sponges sampled in the Museum of Zoology of the Federal University of Bahia, dated 1981 and sponges sampled in 2017. The results showed that in sponges of 2017, 80% of the samples had MPs unlike those of 1981, of which only 10% had plastics. Furthermore, Celis-Hernández et al. (2021) investigated the presence of MPs in the water, on sediment, and in the marine sponges Halichondria (Halichondria) melanadocia (de Laubenfels, 1936) (ex Halichondra melanodocia), Haliclona (Reniera) implexiformis (Hechtel, 1965) (ex Haliclona implexiformis), and Amorphinopis atlantica (Carvalho, Hajdu, Mothes & van Soest, 2004). The sponges showed average MPs from 1,861 to 3,456 items kg−1 of dry weight, confirming their bioaccumulation capacity of these contaminants. Saliu et al. (2022) used new methods to determine the presence of MPs in Haliclona’s tissue.
One of the latest articles by Krikech et al. (2023) proposes a theory by which the abundance of microplastics present in the sponge samples is not linked to the sponge species but to the pollution of the sampling site. In fact, in this work, the sponges took into account different species: Chondrosia reniformis, Ircinia variabilis, Petrosia ficiformis, and Sarcotragus spinosulus. The MPs were found in all the samples and their abundance ranged from 3.95 × 105 to 1.05 × 106 particles per each gram of sponge tissue (dry weight) (Krikech et al., 2023).
The analysis showed that 70% of the samples presented the MPs in a concentration of approximately 1.2 g of tissue and polyolefin was the most present polymer. Almeida et al. (2019) demonstrated for the first time that some microorganisms were capable of synthesizing enzyme that hydrolyzes polyethylene terephthalate (PET). The bacterial strain Streptomyces associated with marine sponges Lissodendoryx (Extyodoryx) diversichela (Lundbeck, 1905) (ex Lyssodendoryx diversichela) and Inflatella pellicula (Schmidt, 1875) produced enzymes with similar function to hydrolase for the polymer PET contributing to its bioremediation.
Giametti and Finelli (2022) evaluated the presence of compounds associated with plastics (phthalates, phthalate metabolite, bisphenol A, and a brominated flame retardant) in marine sponge tissues. The sponges examined were Xestospongia muta (Schmidt, 1870) and Niphates digitalis (Lamarck, 1814) and the experiments demonstrated the presence of di(2-ethylhexyl) phthalate in all samples of sponges. Baird (2016) exposed the sponges Tethya bergquistae (Hooper in Hooper & Wiedenmayer, 1994) and Crella incrustans (Carter, 1885) collected from New Zealand to plastic particles with different sizes 1 μm and 6 μm at two different concentrations 200,000/mL and 400,000/mL. The aim of the experiments was to evaluate how the absorption of the plastic affects the respiration rate of the sponges (oxygen concentration, mg/L). It was observed that Tethya berguistae showed an alteration in respiration rate by calculating oxygen consumption through an oxygen probe. De Marchi et al. (2022) demonstrated that that the absorption of MPs can reduce the filtration and respiration rates on marine sponges. After 72 h, the adsorption increased (approximately 30% of the particles in MPs after 72 h) while the filtration rate decreased.
Loredana et al. (2017) studied the potential effects of bacteria associated to Hymeniacidon perlevis in the removal of the pesticide lindane (POP) in the marine environment. In general, pesticides are classified according to their mechanisms of action and lindane is an organochlorine pesticide capable of destroying the functionality of the nervous system of organisms (DeLorenzo et al., 2001; Grung et al., 2015). Not all bacterial species were characterized, but those characterized (Mameliella phaeodactyli, Pseudovibrio ascidiaceicola, Oceanicaulis stylophorae, and R. atlantica) were able to use lindane as a carbon source, exhibiting a decrease in the concentration of the pesticide in 12 days.
Gupta and Kiran (2017) isolated a lipopeptide from the marine sponge F. cavernosa (MMD32 strain), which was able to degrade PAHs, forming a micellar system that solubilizes the hydrophobic substrate and reduces surface tension. Phenanthrene and pyrene at a concentration of 100 mg/L were degraded in 7 days, and in 5 days the pyrene was reduced to a concentration of 8.425 mg. Marzuki et al. (2021) explored the ability of marine sponges Niphate sp., Hyrtios erectus, and Clathria to resist the presence of PAHs. These authors demonstrated that B. pumilus, P. stutzeri, and Acinetobacter calcoaceticus associated to all sponges examined degraded anthracene and pyrene. In particular, the pyrene has been degraded less, because it is more toxic.
Oakland (2013) demonstrated that marine sponges are useful to clear the turbidity level and phosphate concentrations from an average of 3.93 μg/L to 1.33 within 36 h. In particular, they used four sponges: the “gray”, “green”, “red branching”, and “black” species were sampled in Tanzania in 15 L of contaminated water. Orani et al. (2022) explored the ability of sponges Haliclona (Halichoclona) fulva (Topsent, 1893), Acanthella acuta (Schmidt, 1862), Chondrilla nucula, Chondrosia reniformis, Axinella verrucosa, Aplysina cavernicola, and Axinella polypoides (Schmidt, 1862) collected in Villefranche-sur-Mer (Mediterranean Sea) to bioaccumulate rare earth elements, defined as emergent contaminants (Castor and Hedrick, 2005). The results showed that Aplysina cavernicola accumulated the highest quantity of rare earth elements (particularly a concentration of lanthanum of 8.3 ± 2.1 mg kg−1 after 96 h was observed). It was hypothesized that this ability was related first of all to the microbial community that lives in association with the sponges and then to other physiological characteristics of the different species. Narayan et al. (2023) studied the capacity of sponge Cinachyrella cavernosa (Lamarck, 1815) (Tethya cavernosa) to bioremediation of azo dyes. The bacterial strain Yangia pacifica was isolated from sponge and decolorized the dyes at 50 mg L−1 concentration reaching 96% effect in 1 week.
4 Role of sponges in integrated multitrophic aquaculture
Integrated Multitrophic Aquaculture (IMTA) is an aquaculture system that enables supplying food to the population, but in a more responsible and sustainable way (Khanjani et al., 2022). As suggested by the name of this practice, the “multi-trophic” approach refers to the presence in the aquaculture plan of organisms belonging to different trophic positions (Barrington et al., 2009) and the food niche of one species uses the waste produced by another species (Nissar et al., 2023).
Despite the ecological role of the sponges in IMTA systems and their efficiency in bioremediation, these marine organisms are still little used (Aguilo-Arce et al., 2023).
Pronzato et al. (1998) were the first to include sponges in the IMTA system as an extractive component. Gökalp et al. (2019) demonstrated that the sponges Chondrosia reniformis achieved 86% survival and 170% growth rates growing on PVC sheets suspended near a fish farm, compared to the lowest percentages of controls grown in pristine conditions. Gokalp 2021; Gökalp 2021) proposed a theoretical design for an IMTA combining seaweed Gracilaria sp., Caribbean sponge Halisarca caerulea (Vacelet & Donadey, 1987), and sea cucumbers Apostichopus japonica. In this system, sponges played a role in converting dissolved organic matter (DOC) from algae in particulate organic matter (POM) used for sea cucumbers feed. One of the first attempts of the IMTA system in Taranto for organisms of the Mediterranean Sea was organized by Giangrande et al. (2020). They reported the results on the first year of co-culture among sponges (Sarcotragus spinosulus), polychaetes (Sabella spellanzanii), and macroalgae (Chaetomorpha linum and Gracilarua bursa-pastoris). In particular, for sponges Sarcotragus spinosulus, it was observed that despite signals of stress, these benthic organisms showed a great capacity for regeneration after an initial period of adaptation, and in 1 year, their volume has doubled. Trani et al. (2021) used an IMTA system to increase the biomass of the sponge Sarcotragus spinosulus, the alga C. linum, and the sea bass Dicentrarchus labrax. In the experiment, it was observed that ammonium was the most abundant inorganic compound (with a higher concentration of 1 mg/L), while phosphates and nitrates decreased compared to the control tanks. However, sponges, unlike fish and algae, did not show a significant increase in biomass after 96 h of experiments.
5 Discussion
The use of sponge dates back thousands of years, and interest in these invertebrates is growing as new applications are discovered. One of the first uses of marine sponges concerns marine aquarium hobbyists, where they are used as ornamental species, due to a variety of their coloration and shape (Kieattisak et al., 2017) (see Figure 4). In the last decades, the interest versus sponges prompted scientists to explore them as sources of bioactive compounds with a wide range of biotechnological applications in pharmaceutical, nutraceutical, and cosmeceutical fields. Additionally, thanks to the circulation chambers of the aquiferous system, where particulate materials are trapped, sponges can also be useful in bioremediation of the marine environment from xenobiotics of human origin and natural toxins.
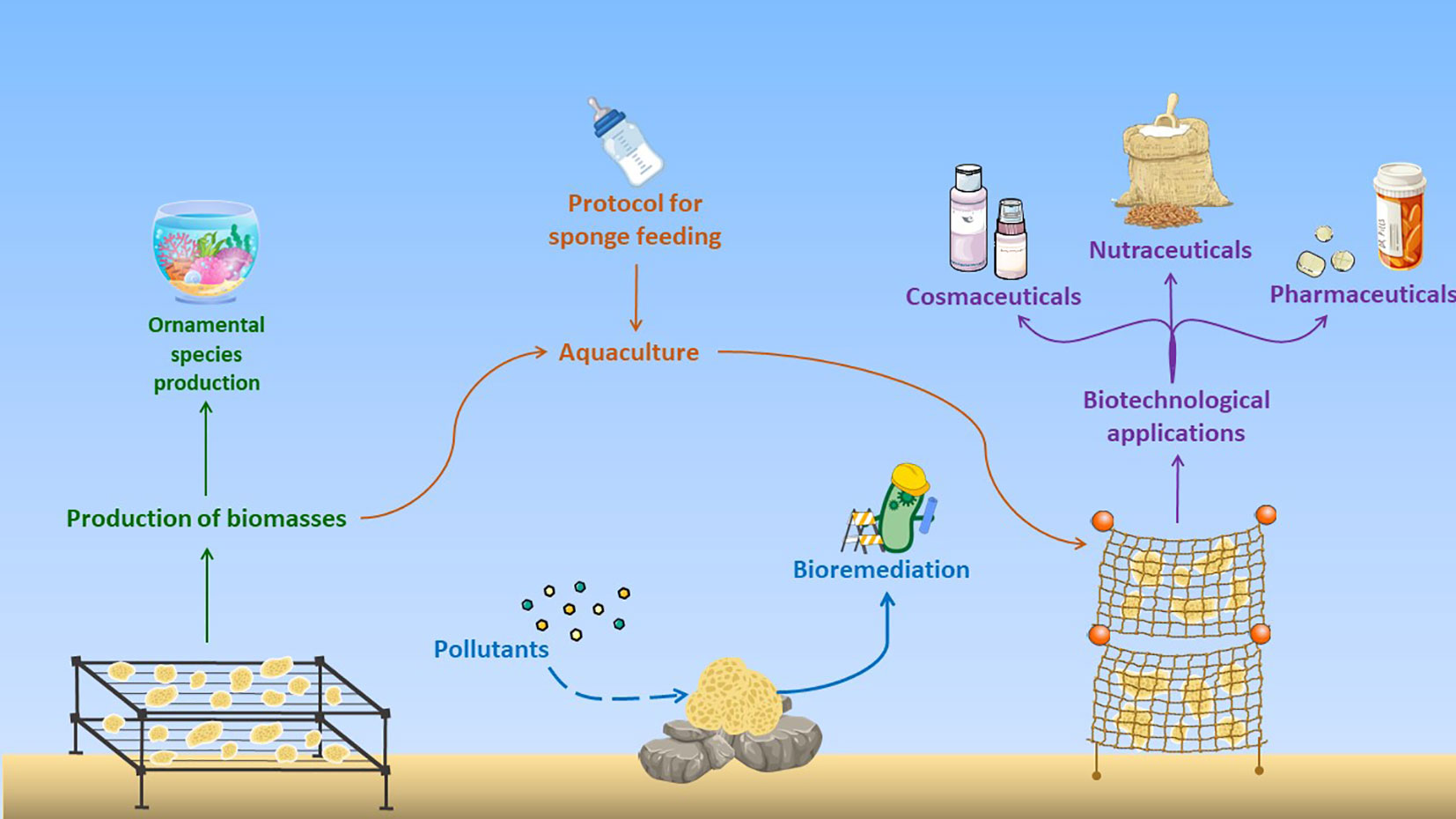
Figure 4 Different uses of sponges in aquariology as ornamental species, and in aquaculture for the production of biomass for biotechnological applications in cosmeceutical, nutraceutical, and pharmacological fields, and for bioremediation of marine environment (modified from Aguilo-Arce et al., 2023).
The increasing global awareness of the negative impact of the natural marine sponge collection led researchers to evaluate a sustainable solution for the supply of raw sponge material for the pharmaceutical industry. In fact, secondary metabolites isolated from sponges were present in a small amount and are difficult to synthetize, pushing the scientists to optimize aquaculture methods to increase marine sponge biomass, promoting the production of bioactive metabolites. Although most sponge cultures currently take place in situ with various approaches as reported above, such as the attachment of sponge specimens or their explants to rocks, travertine tiles, oyster shells, carapaces of crabs, coralline algae, wooden boxes speared with bamboo sticks in continuous movement, suspended on nylon threads, or retained in mesh or cages, bags, PVC, or PP supports, aquaculture in captivity could be a game changer. In fact, in a closed system, different parameters, such as salinity, temperature, water flow, light, nutrients, rapid spreading of microbes, and eventual diseases, can be kept under control, also changing one parameter at a time, and therefore increasing biomass production.
As demonstrated in this review, the ideal farming structure may vary depending on the sponge species, but generally involves threading sponges on rope or placing them inside a mesh. Another important aspect in the use of marine sponges extensively underlined in this review concerns their use to bioremediate the marine environment. In lieu of this, the IMTA practice seems to be the most challenging alternative in combining the cultivation of sponges, which showed a good biotechnological potential, with their ability of bioremediation, allowing better water quality and promising high economic return and social suitability.
Porifera seems to be one of the most promising marine organisms, although they are still underexploited. For this reason, we suggest that ad hoc experimental mesocosms, purposely contaminated with plastics, can be set up in laboratory for different marine sponges (with biotechnological potential) to reconstruct marine habitat for their rearing suitability and performance, for producing sponge biomass by in situ aquaculture. After plastic exposure and increase in sponge volume, the biomass thus obtained can be used for chemical extraction and bioassay-guided fractionation for the identification of bioactive compounds for biotechnological applications.
Among the most complicated aspects in ex situ sponge cultures are the inability of sponges to keep their natural composition of associated microorganisms and understanding their dietary requirements. These limits could be overcome using synthetic biology and genome mining techniques (transcriptomic, proteomic, genomic, meta-transcriptomic, and meta-genomics) to understand the best culture conditions for each species of sponge. In the future, two main methods can be applied for ex situ cultures, namely, using choanocytes as stem cells that can differentiate other cell types and making them a good starting material, or transfection of seawater juvenile sponges for sponge cell immortalization (Schippers et al., 2012).
Author contributions
All authors contributed to the study conception and design of the review. AA, RE, and SF performed data collection and analysis of literature and wrote the first draft of the manuscript; MP, MGi, VZ, and MC supervised the work; MP, MGi, MB, MG, LM, and GL critically revised the text. All authors contributed to the article and approved the submitted version.
Funding
AA was supported by a PhD fellowship (PhD in Biology, University of Naples Federico II) funded by the project “Biomonitoraggio di micro e nanoplastiche biodegradabili: dall’ambiente all’uomo in una prospettiva one health” (BioPlast4Safe), Piano Nazionale per gli Investimenti Complementari (PNC) E.1 Salute, Ambiente, Biodiversità e Clima. SF was supported by a PhD fellowship co-funded by the Stazione Zoologica Anton Dohrn (Naples, Italy) and the University of Genova.
Acknowledgments
This work was performed in the framework of the PRIN 2022 project “Mediterranean marine organisms as sources of new anti- inflammatory and pro-resolving compounds”. It also is performed in the framework of the call “Progetti @CNR” for the project “Sea cosmetics and nutraceutics: from fishery and aquaculture byproducts” (SCANFISH).
Conflict of interest
The authors declare that the research was conducted in the absence of any commercial or financial relationships that could be construed as a potential conflict of interest.
Publisher’s note
All claims expressed in this article are solely those of the authors and do not necessarily represent those of their affiliated organizations, or those of the publisher, the editors and the reviewers. Any product that may be evaluated in this article, or claim that may be made by its manufacturer, is not guaranteed or endorsed by the publisher.
References
Aguilo-Arce J., Ferriol P., Trani R., Puthod P., Pierri C., Longo C. (2023). Sponges as emerging by-product of integrated multitrophic aquaculture (IMTA). J. Mar. Sci. Eng. 11, 80. doi: 10.3390/jmse11010080
Alimi O. S., Farner Budarz J., Hernandez L. M., Tufenkji N. (2018). Microplastics and nanoplastics in aquatic environments: aggregation, deposition, and enhanced contaminant transport. Environ. Sci. Technol. 52, 1704–1724. doi: 10.1021/acs.est.7b05559
Almeida E. L., Rincón A. F. C., Jackson S. A., Dobson A. D. W. (2019). In silico screening and heterologous expression of a polyethylene terephthalate hydrolase (PETase)-like enzyme (SM14est) with polycaprolactone (PCL)-degrading activity, from the marine sponge-derived strain Streptomyces sp. SM14. Front. Microbiol. 10. doi: 10.3389/fmicb.2019.02187
Amelia T. S. M., Suaberon F. A. C., Vad J., Fahmi A. D. M., Saludes J. P., Bhubalan K. (2022). Recent advances of marine sponge-associated microorganisms as a source of commercially viable natural products. Mar. Biotechnol. 24, 492–512. doi: 10.1007/s10126-022-10130-2
Andrade P., Willoughby R., Pomponi S. A., Kerr R. (1999). Biosynthetic studies of the alkaloid, stevensine, in a cell culture of the marine sponge Teichaxinella morchella. Tetrahedron 40, 4775–4778. doi: 10.1016/S0040-4039(99)00881-3
Ávila E., Briceño-Vera A. E. (2018). A reciprocal inter-habitat transplant reveals changes in the assemblage structure of macroinvertebrates associated with the Sponge Halichondria melanadocia. Estuar. Coasts. 41, 1397–1409. doi: 10.1007/s12237-017-0359-2
Baby J., Raj J., Biby E., Sankarganesh P., Jeevitha M., Ajisha S., et al. (2011). Toxic effect of heavy metals on aquatic environment. Int. J. Biol. Chem. Sci. 4, 939–952. doi: 10.4314/ijbcs.v4i4.62976
Baldacconi R., Cardone F., Longo C., Mercurio M., Marzano C. N., Gaino E., et al. (2010). Transplantation of Spongia officinalis L. (Porifera, Demospongiae): A technical approach for restocking this endangered species. Mar. Ecol. 31, 309–317. doi: 10.1111/j.1439-0485.2009.00299.x
Bano R., Kuddus K., Zaheer M. R., Zia M. (2017). Microbial enxymatic degradation of biodegradable plastics. Curr. Pharm. Biotechnol. 18, 429–440. doi: 10.2174/1389201018666170523165742
Barrington K., Chopin T., Robinson S. (2009). Integrated mariculture - a global review. FAO Fish. Aquac. J. 529, 7–46. doi: 10.2174/1389201018666170523165742
Barthel D., Theede H. (1986). A new method for the culture of marine sponges and its application for experimental studies. Ophelia 25, 75–82. doi: 10.1080/00785326.1986.10429715
Bauvais C., Zirah S., Piette L., Chaspoul F., Domart-Coulon I., Chapon V., et al. (2015). Sponging up metals: bacteria associated with the marine sponge Spongia officinalis. Mar. Environ. Res. 104, 20–30. doi: 10.1016/j.marenvres.2014.12.005
Becerro M. A. (1997). Multiple functions for secondary metabolites in encrusting marine invertebrates. J. Chem. Ecol. 23, 1527–1547. doi: 10.1023/B:JOEC.0000006420.04002.2e
Belarbi E. H., Ramírez Domínguez M., Cerón García M. C., Contreras Gómez A., García Camacho F., Molina Grima E. (2003). Cultivation of explants of the marine sponge Crambe crambe in closed systems. Biomol. Eng. 20, 333–337. doi: 10.1016/S1389-0344(03)00043-1
Bergman O., Haber M., Mayzel B., Anderson M. A., Shpigel M., Hill R. T., et al. (2011). Marine-based cultivation of Diacarnus sponges and the bacterial community composition of wild and maricultured sponges and their larvae. Mar. Biotechnol. 13, 1169–1182. doi: 10.1007/s10126-011-9391-6
Bierwirth J., Mantas T. P., Villechanoux J., Cerrano C. (2022). Restoration of marine sponges—What can we learn from over a century of experimental cultivation? Water 14 (7), 1055. doi: 10.3390/w14071055
Borghese J., Arduini D., Calabrese C., Licciano M., Giangrande A., Migoni D., et al. (2022). “Monitoring biomass quality of by-rpoducts from an IMTA system: evaluation of heavy metals,” in Aquaculture Europe 2022: Innovative solutions in a changing world (European Aquaculture Society). doi: 10.13140/RG.2.2.34547.20001
Bosker T., Guaita L., Behrens P. (2018). Microplastic pollution on Caribbean beaches in the Lesser Antilles. Mar. pollut. Bull. 133, 442–447. doi: 10.1016/j.marpolbul.2018.05.060
Cappello E., Nieri P. (2021). From life in the sea to the clinic: the marine drugs approved and under clinical trial. Life 11 (12), 1390. doi: 10.3390/life11121390
Carballo J. L., Yañez B., Zubía E., Ortega M. J., Vega C. (2010). Culture of explants from the sponge Mycale cecilia to obtain bioactive mycalazal-type metabolites. Mar. Biotechnol. 12, 516–525. doi: 10.1007/s10126-009-9235-9
Carmen Casado-Martinez M., Smith B. D., Luoma S. N., Rainbow P. S. (2010). Metal toxicity in a sediment-dwelling polychaete: threshold body concentrations or overwhelming accumulation rates? Environ. pollut. 158, 3071–3076. doi: 10.1016/j.envpol.2010.06.026
Castor S. B., Hedrick J. B. (2005). “Rare earth elements,” in Industrial minerals and rocks, 769–792. doi: 10.1002/9781119951438.eibd0664
Celis-Hernández O., Ávila E., Ward R. D., Rodríguez-Santiago M. A., Aguirre-Téllez J. A. (2021). Microplastic distribution in urban vs pristine mangroves: using marine sponges as bioindicators of environmental pollution. Environ. pollut. 284, 117391. doi: 10.1016/j.envpol.2021.117391
Cerrano C., Giovine M., Steindler L. (2022). Petrosia ficiformis (Poiret 1789): an excellent model for holobiont and biotechnological studies. Curr. Opin. Biotechnol. 74, 61–65. doi: 10.1016/j.copbio.2021.10.022
Chu Y., Zhang C., Chen X., Li X., Ren N., Ho S. H. (2023). Multistage defense response of microalgae exposed to pharmaceuticals in wastewater. Chin. Chem. Lett. 34, 107727. doi: 10.1016/j.cclet.2022.08.007
Corriero G., Longo C., Mercurio M., Nonnis Marzano C., Lembo G., Spedicato M. T. (2004). Rearing performance of Spongia officinalis on suspended ropes off the southern italian coast (central Mediterranean sea). Aquaculture 238, 195–205. doi: 10.1016/j.aquaculture.2004.04.030
Crawshay B. L. R. (1939). Studies in the market sponges I.Growth from the planted cutting. J. Mar. Biol. Assoc. United. Kingdom. 23, 553–574. doi: 10.1017/S0025315400014077
De Caralt S., Agell G., Uriz M. J. (2003). Long-term culture of sponge explants: conditions enhancing survival and growth, and assessment of bioactivity. Biomol. Eng. 20, 339–347. doi: 10.1016/S1389-0344(03)00045-5
De Caralt S., Sánchez-Fontenla J., Uriz M. J., Wijffels R. H. (2010). In situ aquaculture methods for Dysidea avara (Demospongiae, Porifera) in the Northwestern Mediterranean. Mar. Drugs 8, 1731–1742. doi: 10.3390/md8061731
Dechsakulwatana C., Rungsihiranrut A., Muangchinda C., Ningthoujam R., Klankeo P., Pinyakong O. (2022). Biodegradation of petroleum oil using a constructed nonpathogenic and heavy metal-tolerant bacterial consortium isolated from marine sponges. J. Environ. Chem. Eng. 10, 108752. doi: 10.1016/j.jece.2022.108752
DeLorenzo M. E., Scott G. I., Ross P. E. (2001). Toxicity of pesticides to aquatic microorganisms: A review. Environ. Toxicol. Chem. 20, 84–98. doi: 10.1002/etc.5620200108
De Marchi L., Renzi M., Anselmi S., Pretti C., Guazzelli E., Martinelli E., et al. (2022). Polyethylene microplastics reduce filtration and respiration rates in the Mediterranean sponge Petrosia ficiformis. Environ. Res. 211, 113094. doi: 10.1016/j.envres.2022.113094
Di Bari G., Gentile E., Latronico T., Corriero G., Fasano A., Marzano C. N., et al. (2014). Comparative analysis of protein profiles of aqueous extracts from marine sponges and assessment of cytotoxicity on different mammalian cell types. Environ. Toxicol. Pharmacol. 38, 1007–1015. doi: 10.1016/j.etap.2014.10.021
Dos Santon Esteves A. I. (2009). Esponjas marinhas: potenciais aplicações biotecnlógicas (Lisbona (PT: Universidade de Lisboa).
Duckworth A. (2009). Farming sponges to supply bioactive metabolites and bath sponges: a review. Mar. Biotechnol. 11, 669–679. doi: 10.1007/s10126-009-9213-2
Duckworth A. R., Battershill C. N., Bergquist P. R. (1997). Influence of explant procedures and environmental factors on culture success of three sponges. Aquaculture 156, 251–267. doi: 10.1016/S0044-8486(97)00131-2
Duckworth A. R., Battershill C. N., Schiel D. R. (2004). Effects of depth and water flow on growth, survival and bioactivity of two temperate sponges cultured in different seasons. Aquaculture 242, 237–250. doi: 10.1016/j.aquaculture.2004.08.046
Duckworth A. R., Wolff C. (2007). Bath sponge aquaculture in Torres Strait, Australia: effect of explant size, farming method and the environment on culture success. Aquaculture 271, 188–195. doi: 10.1016/j.aquaculture.2007.06.037
Dumdei E. J., Blunt J. W., Munro M. H. G., Battershill C. N., Page M. J. (1998). “The whys and whats of sponge chemistry: why chemists extract sponges and what problems does this cause?,” in Sponge science; multidisciplinary perspectives (Springer).
Ereskovsky A. V. (2003). Problems of coloniality, modularity, and individuality in sponges and special features of their morphogeneses during growth and asexual reproduction. Russ. J. Mar. Biol. 29, 46–56. doi: 10.1023/B:RUMB.0000011716.90730.ac
Esposito R., Federico S., Bertolino M., Zupo V., Costantini M. (2022a). Marine Demospongiae: a challenging treasure of bioactive compounds. Mar. Drugs 20, 1–22. doi: 10.3390/md20040244
Esposito R., Federico S., Glaviano F., Somma E., Zupo V., Costantini M. (2022b). Bioactive compounds from marine sponges and algae: effects on cancer cell metabolome and chemical structures. Int. J. Mol. Sci. 23, 10680. doi: 10.3390/ijms231810680
Esposito R., Ruocco N., Viel T., Federico S., Zupo V., Costantini M. (2021). Sponges and their symbionts as a source of valuable compounds in cosmeceutical field. Mar. Drugs 19, 444. doi: 10.3390/MD19080444
Fallon B. R., Freeman C. J. (2021). Plastics in Porifera: The occurrence of potential microplastics in marine sponges and seawater from Bocas del Toro, Panamá. PeerJ 9, e11638. doi: 10.7717/peerj.11638
Fasulo S., Guerriero G., Cappello S., Colasanti M., Schettino T., Leonzio C., et al. (2015). The “SYSTEMS BIOLOGY” in the study of xenobiotic effects on marine organisms for evaluation of the environmental health status: biotechnological applications for potential recovery strategies. Rev. Environ. Sci. Biotechnol. 14, 339–345. doi: 10.1007/s11157-015-9373-7
Faulkner D. J. (2000). Marine pharmacology. Int. J. Gen. Mol. Microbiol. 77, 135–145. doi: 10.1023/A:1002405815493
Ferrante M., Vassallo M., Mazzola A., Brundo M. V., Pecoraro R., Grasso A., et al. (2018). In vivo exposure of the marine sponge Chondrilla nucula Schmidt 1862 to cadmium (Cd), copper (Cu) and lead (Pb) and its potential use for bioremediation purposes. Chemosphere 193, 1049–1057. doi: 10.1016/j.chemosphere.2017.11.144
Fu J., Liu N., Peng Y., Wang G., Wang X., Wang Q., et al. (2023). An ultra-light sustainable sponge for elimination of microplastics and nanoplastics. J. Hazard. Mater. 456, 131685. doi: 10.1016/j.jhazmat.2023.131685
Fu W., Sun L., Zhang X., Zhang W. (2006). Potential of the marine sponge Hymeniacidon perleve as a bioremediator of pathogenic bacteria in integrated aquaculture ecosystems. Biotechnol. Bioeng. 93, 1112–1122. doi: 10.1002/bit
Fu W., Wu Y., Sun L., Zhang W. (2007). Efficient bioremediation of total organic carbon (TOC) in integrated aquaculture system by marine sponge Hymeniacidon perleve. Biotechnol. Bioeng. 97, 1387–1397. doi: 10.1002/bit
Fu D., Zhang Q., Fan Z., Qi H., Wang Z., Peng L. (2019). Aged microplastics polyvinyl chloride interact with copper and cause oxidative stress towards microalgae Chlorella vulgaris. Aquat. Toxicol. 216, 105319. doi: 10.1016/j.aquatox.2019.105319
Galafassi S., Nizzetto L., Volta P. (2019). Plastic sources: a survey across scientific and grey literature for their inventory and relative contribution to microplastics pollution in natural environments, with an emphasis on surface water. Sci. Total. Environ. 693, 133499. doi: 10.1016/j.scitotenv.2019.07.305
Giametti S. D., Finelli C. M. (2022). Detection of plastic-associated compounds in marine sponges. Mar. pollut. Bull. 175, 113141. doi: 10.1016/j.marpolbul.2021.113141
Giangrande A., Pierri C., Arduini D., Borghese J., Licciano M., Trani R., et al. (2020). An innovative IMTA system: polychaetes, sponges and macroalgae co-cultured in a southern italian in-shore mariculture plant (Ionian Sea). J. Mar. Sci. Eng. 8, 1–24. doi: 10.3390/JMSE8100733
Giovine M., Scarfì S., Pozzolini M., Penna A., Cerrano C. (2013). “Cell reactivity to different silica,” in Biomedical inorganic polymers. Progress in molecular and subcellular biology, vol. 54 . Eds. Müller W., Wang X., Schröder H. (Berlin, Heidelberg: Springer). doi: 10.1007/978-3-642-41004-8_6
Gokalp M. M. (2021). “The Multifunctional role of marine sponges in multi-trophic mariculture systems,” (Wageningen (NL): Wageningen University).
Gökalp M., Mes D., Nederlof M., Zhao H., Merijn de Goeij J., Osinga R. (2021). The potential roles of sponges in integrated mariculture. Rev. Aquac. 13 (3), 1159–1171. doi: 10.1111/raq.12516
Gökalp M., Wijgerde T., Murk A., Osinga R. (2022). Design for large-scale maricultures of the Mediterranean demosponge Chondrosia reniformis Nardo 1847 for collagen production. Aquaculture 548, 737702. doi: 10.1016/j.aquaculture.2021.737702
Gökalp M., Wijgerde T., Sarà A., De Goeij J. M., Osinga R. (2019). Development of an integrated mariculture for the collagen-rich sponge Chondrosia reniformis. Mar. Drugs 17, 1–15. doi: 10.3390/md17010029
Gravina M. F., Longo C., Puthod P., Rosati M., Colozza N., Scarselli M. (2022). Heavy metal accumulation capacity of Axinella damicornis (Esper 1794) (Porifera, Demospongiae): a tool for bioremediation of polluted seawaters. Mediterr. Mar. Sci. 23, 125–133. doi: 10.12681/mms.27792
Grung M., Lin Y., Zhang H., Steen A. O., Huang J., Zhang G., et al. (2015). Pesticide levels and environmental risk in aquatic environments in China - a review. Environ. Int. 81, 87–97. doi: 10.1016/j.envint.2015.04.013
Gupta A., Kiran G. S. (2017). Effect of lipopeptide from marine sponge in biofilm degradation, bioremediation and emulsification. Chem. Sci. Rev. Lett. 6, 2127–2132.
Hadas E., Shpigel M., Ilan M. (2005). Sea ranching of the marine sponge Negombata magnifica (Demospongiae, Latrunculiidae) as a first step for latrunculin B mass production. Aquaculture 244, 159–169. doi: 10.1016/j.aquaculture.2004.11.052
Hansen I. V., Weekst J. M., Depledge M. H. (1995). Accumulation of copper, zinc, cadmium and chromium by the marine sponge Halichondria panicea Pallas and the implications for biomonitoring. Mar. pollut. Bull. 31, 133–138. doi: 10.1016/0025-326X(94)00228-2
Hesp K., van der Heijden J. M., Munroe S., Sipkema D., Martens D. E., Wijffels R. H., et al. (2023). First continuous marine sponge cell line established. Sci. Rep. 13 (1), 5766. doi: 10.1038/s41598-023-32394-x
Hogg M. M., Tendal O. S., Conway K. W., Pomponi S. A., Van Soest R. W. M., Gutt J., et al. (2010). Deep-sea sponge grounds: reservoirs of biodiversity (Cambridge: UNEP World Conservation Monitoring Centre).
Holleman A. S., Widerg E. (1985). Lehrbuch der anorganischen Chemie Walter de Gruyter. Walter de Gruyter GmbH & Co KG.
Karlsson T. M., Vethaak A. D., Almroth B. C., Ariese F., van Velzen M., Hassellöv M., et al. (2017). Screening for microplastics in sediment, water, marine invertebrates and fish: method development and microplastic accumulation. Mar. pollut. Bull. 122, 403–408. doi: 10.1016/j.marpolbul.2017.06.081
Kelly M., Handley S., Page M., Butterfield P., Hartill B., Kelly S. (2004). Aquaculture trials of the New Zealand bath-sponge Spongia (Heterofibria) manipulates using lanterns. New Zeal. J. Mar. Freshw. Res. 38, 231–241. doi: 10.1080/00288330.2004.9517233
Keren R., Lavy A., Mayzel B., Ilan M. (2015). Culturable associated-bacteria of the sponge Theonella swinhoei show tolerance to high arsenic concentrations. Front. Microbiol. 6. doi: 10.3389/fmicb.2015.00154
Khanjani M. H., Zahedi S., Mohammadi A. (2022). Integrated multitrophic aquaculture (IMTA) as an environmentally friendly system for sustainable aquaculture: functionality, species, and application of biofloc technology (BFT). Environ. Sci. pollut. Res. 29, 67513–67531. doi: 10.1007/s11356-022-22371-8
Khayatzadeh J., Abbasi E. (2010). “The effects of heavy metals on aquatic animals,” in The 1st international applied geological congress, department of geology, islamic azad university–mashad branch, Iran, 26–28.
Kieattisak Y., Udomsak D., Wattana W., Patchara P. (2017). Cultivation of blue marine sponge, Xestospongia sp. (CF Neopetrosia sp.) in hatchery as an ornamental species. Agric. Res. Technol. 12, 1–4. doi: 10.19080/ARTOAJ.2017.12.555838
Kiruba Sankar R., Chadha N. K., Dam Roy S., Banerjee P., Saharan N., Krishnan P. (2016). Growth and survival of marine sponges, Stylissa massa (Carter 1887) and liosina paradoxa (Thiele 1899) in sea and land based culture systems. Indian J. Fish. 63, 55–60. doi: 10.21077/ijf.2016.63.4.43906-09
Koopmans M., Martens D., Wijffels R. H. (2009). Towards commercial production of sponge medicines. Mar. Drugs 7 (4), 787–802. doi: 10.3390/md7040787
Krikech I., Conti G. O., Pulvirenti E., Rapisarda P., Castrogiovanni M., Maisano M., et al. (2023). Microplastics (≤ 10 μm) bioaccumulation in marine sponges along the Moroccan Mediterranean coast: Insights into species-specific distribution and potential bioindication. Environ. Res. 235, 116608. doi: 10.1016/j.envres.2023.116608
Langenbruch P. F. (1983). Untersuchungen zum Körperbau von Meeresschwämmen. II. Das Wasserleitungssystem vonHalichondria panicea. Helgoländer. Meeresun. 36, 337–346. doi: 10.1007/BF01983636
Ledda F. D., Pronzato R., Manconi R. (2014). Mariculture for bacterial and organic waste removal: a field study of sponge filtering activity in experimental farming. Aquac. Res. 45, 1389–1401. doi: 10.1111/are.12084
Li P., Lu H., Zhang Y., Zhang X., Liu L., Wang M., et al. (2023). The natural products discovered in marine sponge-associated microorganisms: structures, activities, and mining strategy. Front. Mar. Sci. 10. doi: 10.3389/fmars.2023.1191858
Lindequist U. (2016). Marine-derived pharmaceuticals–challenges and opportunities. Biomol. Ther. 24 (6), 561. doi: 10.4062/biomolther.2016.181
Lipton A., Sunith S. (2009). Mariculture of marine sponges for drug development: bioactivity potentials of cultured sponges, Callyspongia subarmigera (Ridley) and Echinodictyum gorgonoides (Dendy). Mar. Fish. Inf. Serv. T&.E. Ser. 200, 7–10.
Longo C., Cardone F., Corriero G., Licciano M., Pierri C., Stabili L. (2016). The co-occurrence of the demosponge Hymeniacidon perlevis and the edible mussel Mytilus galloprovincialis as a new tool for bacterial load mitigation in aquaculture. Environ. Sci. pollut. Res. 23, 3736–3746. doi: 10.1007/s11356-015-5587-z
Loredana S., Graziano P., Antonio M., Carlotta N. M., Caterina L., Maria A. A., et al. (2017). Lindane bioremediation capability of bacteria associated with the demosponge Hymeniacidon perlevis. Mar. Drugs 15, 1–15. doi: 10.3390/md15040108
Maldonado M., Zhang X., Cao X., Xue L., Cao H., Zhang W. (2010). Selective feeding by sponges on pathogenic microbes: a reassessment of potential for abatement of microbial pollution. Mar. Ecol. Prog. Ser. 403, 75–89. doi: 10.3354/meps08411
Marzuki I., Asaf R., Paena M., Athirah A., Nisaa K., Ahmad R., et al. (2021). Anthracene and pyrene biodegradation performance of marine sponge symbiont bacteria consortium. Molecules 26, 6851. doi: 10.3390/molecules26226851
Marzuki I., Daris L., Nisaa K., Emelda A. (2020). The power of biodegradation and bio-adsorption of bacteria symbiont sponges sea on waste contaminated of polycyclic aromatic hydrocarbons and heavy metals. IOP. Conf. Ser.: Earth Environ. Sci. 584, 012013. doi: 10.1088/1755-1315/584/1/012013
Marzuki I., Rosmiati R., Mustafa A., Sahabuddin S., Tarunamulia T., Susianingsih E., et al. (2023). Potential utilization of bacterial consortium of symbionts marine sponges in removing polyaromatic hydrocarbons and heavy metals, review. Biology 12 (1), 86. doi: 10.3390/biology12010086
Maslin M., Gaertner-Mazouni N., Debitus C., de Voogd N. J., Ho R. (2021). Marine sponge aquaculture towards drug development: an ongoing history of technical, ecological, chemical considerations and challenges. Aquac. Rep. 21, 100813. doi: 10.1016/j.aqrep.2021.100813
Mendola D. (2003). Aquaculture of three phyla of marine invertebrates to yield bioactive metabolites: process developments and economics. Biomol. Eng. 20, 441–458. doi: 10.1016/S1389-0344(03)00075-3
Mendola D., De Caralt S., Uriz M. J., Van Den End F., Van Leeuwen J. L., Wijffels R. H. (2008). Environmental flow regimes for Dysidea avara sponges. Mar. Biotechnol. 10, 622–630. doi: 10.1007/s10126-008-9102-0
Meyer K. M., Deines P., Schupp P. J., Taylor M. W. (2016). Impact of explantation techniques on the microbiota of the marine sponge Ecionemia alata. J. Exp. Mar. Bio. Ecol. 484, 11–15. doi: 10.1016/j.jembe.2016.08.003
Milanese M., Chelossi E., Manconi R., Sarà A., Sidri M., Pronzato R. (2003). The marine sponge Chondrilla nucula Schmidt 1862 as an elective candidate for bioremediation in integrated aquaculture. Biomol. Eng. 20, 363–368. doi: 10.1016/S1389-0344(03)00052-2
Modica L., Lanuza P., García-Castrillo G. (2020). Surrounded by microplastic, since when? Testing the feasibility of exploring past levels of plastic microfibre pollution using natural history museum collections. Mar. pollut. Bull. 151, 110846. doi: 10.1016/j.marpolbul.2019.110846
Moitinho-Silva L., Nielsen S., Amir A., Gonzalez A., Ackermann G. L., Cerrano C., et al. (2017). The sponge microbiome project. Gigascience 6, 1–7. doi: 10.1093/gigascience/gix077
Müller W. E. G., Schröder H. C., Wiens M., Perovic-Ottstadt S., Batel R., Müller I. M. (2004). Traditional and modern biomedical prospecting: part II—the benefits. Evidence-Based Complement. Altern. Med. 1, 133–144. doi: 10.1093/ecam/neh030
Müller W. E. G., Wimmer W., Schatton W., Böhm M., Batel R., Filic Z. (1999). Initiation of an aquaculture of sponges for the sustainable production of bioactive metabolites in open systems: example, Geodia cydonium. Mar. Biotechnol. 1, 569–579. doi: 10.1007/PL00011812
Munro M. H. G., Blunt J. W., Dumdei E. J., Hickford S. J. H., Lill R. E., Li S., et al. (1999). The discovery and development of marine compounds with pharmaceutical potential. J. Biotechnol. 70, 15–25. doi: 10.1016/s0168-1656(99)00052-8
Narayan S., Nair S., Gonsalves M. J. (2023). Sponge-associated bacterium, Yangia pacifica: a potential candidate for bioremediation of azo dyes. Res. Commun. 113, 965–969. doi: 10.18520/cs/v113/i05/965-969
Nickel M., Brümmer F. (2003). In vitro sponge fragment culture of Chondrosia reniformis (Nardo 1847). J. Biotechnol. 100, 147–159. doi: 10.1016/S0168-1656(02)00256-0
Nissar S., Bakhtiyar Y., Arafat M. Y., Andrabi S., Mir Z. A., Khan N. A., et al. (2023). The evolution of integrated multi-trophic aquaculture in context of its design and components paving way to valorization via optimization and diversification. Aquaculture 565, 739074. doi: 10.1016/j.aquaculture.2022.739074
Oakland H. C. (2013). Bioremediation mariculture in Zanzibar, Tanzania: a viability assessment of using bath sponge and pearl oyster farms to filter highly polluted waters in the Zanzibar channel. Independent Study Project (ISP) Collection. 1526. Oregon. Undergrad. Res. J. 5. doi: 10.5399/uo/ourj.5.1.3256
Orani A. M., Barats A., Vassileva E., Thomas O. P. (2018). Marine sponges as a powerful tool for trace elements biomonitoring studies in coastal environment. Mar. pollut. Bull. 131, 633–645. doi: 10.1016/j.marpolbul.2018.04.073
Orani A. M., Vassileva E., Thomas O. P. (2022). Marine sponges as coastal bioindicators of rare earth elements bioaccumulation in the French Mediterranean Sea. Environ. pollut. 304, 119172. doi: 10.1016/j.envpol.2022.119172
Orel B., Giovine M., Ilan M. (2021). On the path to thermostable collagen: culturing the versatile sponge Chondrosia reniformis. Mar. Drugs 19, 669. doi: 10.3390/md19120669
Osinga R., Sidri M., Cerig E., Gokalp S. Z., Gokalp M. (2010). Sponge aquaculture trials in the East-Mediterranean sea: new approaches to earlier ideas. Open Mar. Biol. J. 4, 74–81. doi: 10.2174/1874450801004010074
Osinga R., Tramper J., Wijffels R. H. (1999). Cultivation of marine sponges. Mar. Biotechnol. 1, 509–532. doi: 10.1007/PL00011807
Padiglia A., Ledda F. D., Padedda B. M., Pronzato R., Manconi R. (2018). Long-term experimental in situ farming of Crambe crambe (Demospongiae: Poecilosclerida). PeerJ 6, e4964. doi: 10.7717/peerj.4964
Page M. J., Handley S. J., Northcote P. T., Cairney D., Willan R. C. (2011). Successes and pitfalls of the aquaculture of the sponge Mycale hentscheli. Aquaculture 312, 52–61. doi: 10.1016/j.aquaculture.2010.12.006
Page M. J., Northcote P. T., Webb V. L., Mackey S., Handley S. J. (2005). Aquaculture trials for the production of biologically active metabolites in the New Zealand sponge Mycale hentscheli (Demospongiae: Poecilosclerida). Aquaculture 250, 256–269. doi: 10.1016/j.aquaculture.2005.04.069
Palumbi S. R. (1984). Tatics of acclimation: morphological changes of sponges in an unpredictable environment. Science 225, 1478–1480. doi: 10.1126/science.225.4669.1478
Poirrier M. A., Francis J. C., LaBiche R. A. (1981). A continuous-flow system for growing fresh-water sponges in the laboratory. Hydrobiologia 79, 255–259. doi: 10.1007/BF00006321
Pozzolini M., Scarfi S., Gallus L., Ferrando S., Cerrano C., Giovine M. (2017). Silica-induced fibrosis: an ancient response from the early metazoans. J. Exp. Biol. 220, 4007–4015. doi: 10.1242/jeb.166405
Proksch P. (1994). Defensive roles for secondary metabolites from marine sponges and sponge-feeding nudibranchs. Toxicon 32, 639–655. doi: 10.1016/0041-0101(94)90334-4
Pronzato R., Cerrano C., Cubeddu T., Lanza S., Magnino G., Manconi R., et al. (1998). Aquaculture and water: fish culture, sheellfish culture and water usage. Aquauclt. Europe., 12–17. doi: 10.13140/2.1.2461.3768
Ravindran A., Sajayan A., Priyadharshini G. B., Selvin J., Kiran G. S. (2020). Revealing the efficacy of thermostable biosurfactant in heavy metal bioremediation and surface treatment in vegetables. Front. Microbiol. 11. doi: 10.3389/fmicb.2020.00222
Rosado Rodríguez G., Otero Morales E. (2020). Assessment of heavy metal contamination at Tallaboa Bay (Puerto Rico) by marine sponges’ bioaccumulation and fungal community composition. Mar. pollut. Bull. 161, 111803. doi: 10.1016/j.marpolbul.2020.111803
Ruiz C., Valderrama K., Zea S., Castellanos L. (2013). Mariculture and natural production of the antitumoural (+)-discodermolide by the Caribbean marine sponge Discodermia dissoluta. Mar. Biotechnol. 15, 571–583. doi: 10.1007/s10126-013-9510-7
Ryan P. G., Moore C. J., Van Franeker J. A., Moloney C. L. (2009). Monitoring the abundance of plastic debris in the marine environment. Philos. Trans. R. Soc B. Biol. Sci. 364, 1999–2012. doi: 10.1098/rstb.2008.0207
Saliu F., Biale G., Raguso C., La Nasa J., Degano I., Seveso D., et al. (2022). Detection of plastic particles in marine sponges by a combined infrared micro-spectroscopy and pyrolysis-gas chromatography-mass spectrometry approach. Sci. Total. Environ. 819, 152965. doi: 10.1016/j.scitotenv.2022.152965
Santiago V. S., Manzano G. G., Clairecynth C. Y., Alino P. M., Salvador-Reyes L. A. (2019). Mariculture potential of renieramycin-producing Philippine blue sponge Xestospongia sp. (Porifera: Haposclerida). Aquaculture 502, 356–364. doi: 10.1016/j.aquaculture.2018.12.059
Santos-Gandelman J. F., Giambiagi-Demarval M., Muricy G., Barkay T., Laport M. S. (2014). Mercury and methylmercury detoxification potential by sponge-associated bacteria. Antonie van Leeuwenhoek. Int. J. Gen. Mol. Microbiol. 106, 585–590. doi: 10.1007/s10482-014-0224-2
Satyanarayana T., Johri B. N., Prakash A. (2012). Microorganisms in environmental management (New Delhi: Springer).
Schiefenhövel K., Kunzmann A. (2012). Sponge farming trials: survival, attachment, and growth of two Indo-Pacific sponges, Neopetrosia sp. and Stylissa massa. J. Mar. Biol. 11, 417360. doi: 10.1155/2012/417360
Schippers K. J., Sipkema D., Osinga R., Smidt H., Pomponi S. A., Martens D. E., et al. (2012). Cultivation of sponges, sponge cells and symbionts: achievements and future prospects. Adv. Mar. Biol. 62, 273–337. doi: 10.1016/B978-0-12-394283-8.00006-0
Shoham S., Weinberger A., Kaplan A., Avisar D., Ilan M. (2021). Arsenate reducing bacteria isolated from the marine sponge Theonella swinhoei: bioremediation potential. Ecotoxicol. Environ. Saf. 222, 112522. doi: 10.1016/j.ecoenv.2021.112522
Simpson T. (1963). The biology of the marine sponge Microciona prolifera (Ellis and Solander). I. A study of cellular function and differentiation. J. Exp. Zool. 154, 135–151. doi: 10.1002/jez.1401540109
Sipkema D., Franssen M. C. R., Osinga R., Tramper J., Wijffels R. H. (2005a). Marine sponges as pharmacy. Mar. Biotechnol. 7, 142–162. doi: 10.1007/s10126-004-0405-5
Sipkema D., Osinga R., Schatton W., Mendola D., Tramper J., Wijffels R. H. (2005b). Large-scale production of pharmaceuticals by marine sponges: sea, cell, or synthesis? Biotechnol. Bioeng. 90, 201–222. doi: 10.1002/bit.20404
Soares G. M., Barros F., Lanna E., da Silva M. V. S., Cavalcanti F. F. (2022). Sponges as libraries: increase in microplastics in Cinachyrella alloclada after 36 years. Mar. pollut. Bull. 185, 114339. doi: 10.1016/j.marpolbul.2022.114339
Stabili L., Licciano M., Giangrande A., Longo C., Mercurio M., Marzano C. N., et al. (2006). Filtering activity of Spongia officinalis var. adriatica (Schmidt) (Porifera, Demospongiae) on bacterioplankton: implications for bioremediation of polluted seawater. Water Res. 40, 3083–3090. doi: 10.1016/j.watres.2006.06.012
Steinert G., Stauffer C. H., Aas-Valleriani N., Borchert E., Bhushan A., Campbell A., et al. (2018). BluePharmTrain: biology and biotechnology of marine sponges. Grand Challenges in Marine Biotechnology (Cham: Springer).
Tanvi D. A., Pratam K. M., Lohit R. T., Vijayalakshmi B. K., Devaraja T. N., Vasudha M., et al. (2020). Biosorption of heavy metal arsenic from Industrial Sewage of Davangere District, Karnataka, India, using indigenous fungal isolates. SN. Appl. Sci. 2, 1–7. doi: 10.1007/s42452-020-03622-0
Thomassen S., Riisgard H. U. (1995). Growth and energetics of the sponge Halichondria panicea. Mar. Ecol. Prog. Ser. 128, 239–246. doi: 10.3354/meps128239
Thoms C., Horn M., Wagner M., Hentschel U., Proksch P. (2003). Monitoring microbial diversity and natural product profiles of the sponge Aplysina cavernicola following transplantation. Mar. Biol. 142, 685–692. doi: 10.1007/s00227-002-1000-9
Tokiwa Y., Calabia B. P., Ugwu C. U., Aiba S. (2009). Biodegradability of plastics. Int. J. Mol. Sci. 10, 3722–3742. doi: 10.3390/ijms10093722
Trani R., Corriero G., de Pinto M. C., Mercurio M., Pazzani C., Pierri C., et al. (2021). Filtering activity and nutrient release by the keratose sponge Sarcotragus spinosulus Schmidt 1862 (Porifera, demospongiae) at the laboratory scale. J. Mar. Sci. Eng. 9, 1–16. doi: 10.3390/jmse9020178
Turon X., Galera J., Uriz M. J. (1997). Clearance rates and aquiferous systems in two sponges with contrasting life-history strategies. J. Exp. Zool. 278, 22–36. doi: 10.1002/(SICI)1097-010X(19970501)278:1<22::AID-JEZ3>3.0.CO;2-8
Urban-Gedamke E., Conkling M., McCarthy P. J., Wills P. S., Pomponi S. A. (2021). 3-D culture of marine sponge cells for production of bioactive compounds. Mar. Drugs 19 (10), 569. doi: 10.3390/md19100569
Uriz M. J., Turon X., Galera J., Tur J. M. (1996). New light on the cell location of avarol within the sponge Dysidea avara (Dendroceratida). Cell Tissue Res. 285, 519–527. doi: 10.1007/s004410050668
Van Treeck P., Eisinger M., Müller J., Paster M., Schuhmacher H. (2003). Mariculture trials with Mediterranean sponge species: the exploitation of an old natural resource with sustainable and novel methods. Aquaculture 218, 439–455. doi: 10.1016/S0044-8486(03)00010-3
Webster N. S. (2007). Sponge disease: a global threat? Environ. Microb. 9 (6), 1363–1375. doi: 10.1111/j.1462-2920.2007.01303.x
Wijffels R. H. (2008). Potential of sponges and microalgae for marine biotechnology. Trends Biotechnol. 6 (1), 26–31. doi: 10.1016/j.tibtech.2007.10.002
Zarfl C., Matthies M. (2010). Are marine plastic particles transport vectors for organic pollutants to the Arctic? Mar. pollut. Bull. 60, 1810–1814. doi: 10.1016/j.marpolbul.2010.05.026
Keywords: bioremediation, marine biotechnology, sponge farming
Citation: Amato A, Esposito R, Federico S, Pozzolini M, Giovine M, Bertolino M, Guida M, Manfra L, Libralato G, Zupo V and Costantini M (2024) Marine sponges as promising candidates for integrated aquaculture combining biomass increase and bioremediation: an updated review. Front. Mar. Sci. 10:1234225. doi: 10.3389/fmars.2023.1234225
Received: 03 June 2023; Accepted: 26 December 2023;
Published: 31 January 2024.
Edited by:
Renato Crespo Pereira, Fluminense Federal University, BrazilReviewed by:
Guilherme Ramos Da Silva Muricy, Federal University of Rio de Janeiro, BrazilMarcelo Gonzalez, Chilean Antarctic Institute (INACH), Chile
Copyright © 2024 Amato, Esposito, Federico, Pozzolini, Giovine, Bertolino, Guida, Manfra, Libralato, Zupo and Costantini. This is an open-access article distributed under the terms of the Creative Commons Attribution License (CC BY). The use, distribution or reproduction in other forums is permitted, provided the original author(s) and the copyright owner(s) are credited and that the original publication in this journal is cited, in accordance with accepted academic practice. No use, distribution or reproduction is permitted which does not comply with these terms.
*Correspondence: Maria Costantini, bWFyaWEuY29zdGFudGluaUBzem4uaXQ=; dnp1cG9Ac3puLml0; Valerio Zupo, dnp1cG9Ac3puLml0
†These authors have contributed equally to this work and share first authorship