- 1Department of Aquaculture, Nofima, Tromsø, Norway
- 2Institute of Aquaculture, University of Stirling, Scotland, United Kingdom
- 3Laboratory of Neurophysiology and Pathology of Behavior Sechenov Institute of Evolutionary Physiology and Biochemistry, Saint Petersburg, Russia
- 4Department of Ecosystem Processes, Institute of Marine Research, Bergen, Norway
Aquaculture is one of the fastest growing food production sectors in the world and further expansion is expected throughout the 21st century. However, climate change is threatening the development of the sector and action is needed to prepare the industry for the coming challenges. Using downscaled temperature projections based on the Intergovernmental Panel on Climate Change (IPCC) climate projection (Shared Socioeconomic Pathway, SSP2-4.5), we analysed potential future temperatures at a selected Atlantic cod (Gadus morhua L.) farm site in Northern Norway. Results showed that the farming area may experience increased temperatures the next 10–15 years, including more days with temperatures above 17°C. Based on the predicted future conditions, we designed a study with Atlantic cod (Gadus morhua L.) to evaluate effects from high temperature alone and in combination with Fransicella noatunensis infection. Fish were kept at 12°C and 17°C for eight weeks and samples of skin and spleen collected at different timepoints were analysed with transcriptomics, histology, scanning electron microscopy and immunohistochemistry. Results showed that high temperature had a stronger effect on the barrier functions of skin than the infection. Increased temperature induced gene expression changes in skin and spleen, heat shock protein 47 and cold inducible RNA binding protein were identified as potential gene markers for thermal stress. The effect of bacterial challenge was small at 12°C. At high temperature, the development of severe pathology in spleen coincided with a significant decrease of immunoglobulins transcripts, which contrasted with the activation of multiple immune genes. In addition, we used an in vitro model of skin biopsies and scale explants exposed to hydrogen peroxide (H2O2) to assess the effects of thermal and oxidative stress. High temperature and H2O2 reduced proliferation and migration of keratocytes, and increased expression of stress markers, and compounding effects were observed with combined stressors. Results suggest that the projected increased seawater temperature will pose a significant threat to Norwegian cod farming, affecting various biological processes and making fish more vulnerable to stressors and pathogens. Cod farming needs high attention to temperature changes, and special precautions should be taken if the temperature increases beyond cods’ thermal optimum.
1 Introduction
Aquaculture is one of the fastest growing food production sectors in the world and further expansion is expected throughout the 21st century (FAO, 2022). However, climate change is already threatening the development of the sector and action is needed to prepare the industry for the coming challenges (Falconer et al., 2022). Climate stressors relevant for aquaculture include increased air and sea temperatures, rising sea level, more frequent extreme weather events, ocean acidification, altered salinity, and changes in rainfall (IPCC, 2014; IPCC, 2019). Continued emissions will result in further atmospheric and oceanic warming, with long-term changes to the climate system, which will exacerbate existing risks and create new challenges for life on earth (IPCC, 2014; IPCC, 2023). Since aquaculture includes a diversity of production systems, species, and farming environments (Metian et al., 2020), assessment of climate change is especially complex, and a holistic approach is needed to understand the impact and severity of future changes (Falconer et al., 2022).
Atlantic cod (Gadus morhua L.) is one of the most important species for commercial fisheries in the North Atlantic (Welch et al., 1992; Hop and Gjøsæter, 2013). In the last decades, spawning stock biomass and cod catches have declined, and traditional fisheries may become further reduced due to climate change (Misund et al., 2016). Reduced availability of wild cod has renewed the interest in cod farming (Henriksen et al., 2018; Puvanendran et al., 2022). The potential in Norwegian cod farming gradually declined from 2008 due to multiple factors, but new knowledge on biological performance, requirements, and production methods has in recent years initiated new investments in cod farming (Puvanendran et al., 2022). Novel methods and strategies that assess and predict cod health and welfare, as well as insight into how climate change will affect production, are important for successful further growth of the industry. For critical factors such as seawater temperature, targeted approaches are required to investigate the links between biological performance and projected future farm conditions. Seawater temperatures are increasing and there are more frequent marine heatwave events (Oliver et al., 2018; IPCC, 2019), thus farmed cod are likely to be subjected to higher temperatures in the future than currently experienced. Optimal seawater temperatures for mature cod are between 6 and 11°C (Holt and Jørgensen, 2015). Temperatures above 15°C enhance stress, impair swimming and haemoglobin oxygen affinity, and mortality increases when temperatures reach 20°C (Pörtner, 2001; Pérez-Casanova et al., 2008a; Norin et al., 2019; Zanuzzo et al., 2019). These thermal thresholds are worth a closer look, alongside projections of future temperatures in cod farming areas, to understand the limitations and possible strategies of adaptation, when planning to increase cod farming under climate change.
Increasing seawater temperatures, beyond thermal optimum, can make Atlantic cod more vulnerable to infections. The intracellular bacterium Furunculosis noatunensis, the causative agent of francisellosis (Mikalsen, 2008; Ottem et al., 2009), is considered one of the most important disease problems in Norwegian cod farming and was one of the reasons why the industry collapsed around 2010. Current expectations of increased production in combination with increasing sea water temperatures, could cause the disease to re-emerge as a challenge. Diseased cod lose appetite, the skin may appear darker and small ulcers may occur together with nodules (granulomas) in the skin, but usually there are no clear external signs of the disease. Internally, large numbers of yellow granulomas in the kidney, spleen and other organs can be found (Ottem et al., 2008). Bacteria cause cell and tissue damages (Nylund et al., 2006; Olsen et al., 2006), which can be aggravated by thermal stress. Water temperature above 15°C seems to play a significant role in development of francisellosis. The number of published reports on the effect of high seawater temperature on Atlantic cod immunity is limited. Expression of immune genes and respiratory burst were measured in leukocytes of cod exposed to gradual increase of temperature from 10°C to 19°C (Pérez-Casanova et al., 2008b). Transcriptome analysis in spleen of cod acclimated to 10°C and 16°C revealed thermal stimulation of immune responses to viral mimic polyinosinic:polycytidylic acid but not to formalin killed Aeromonas salmonicida (Hori et al., 2013). Immune stimulation in cod may coincide with increased mortality as shown in Atlantic cod infected with Brucella pinnipedialis (Larsen et al., 2018). Acclimation to high temperature increased immunoglobulin and innate antibody levels in cod serum (Magnadóttir et al., 1999), and have previously been shown to compromise immunity in other teleosts (Cabillon and Lazado, 2019; Scharsack and Franke, 2022). Reduced, or changed, immune functions may result in species being more vulnerable to challenges and secondary stressors and must be taken into consideration when evaluating the effects of climate change and warmer temperatures.
Using climate projections from the Intergovernmental Panel on Climate Change (IPCC) we have evaluated potential future temperatures at a commercial cod farm in Norway. Climate change scenarios represent the consequences of anthropogenic activities at global scale (Kriegler et al., 2012; O’Neill et al., 2014), but with the inclusion of dynamical downscaling and bias correction methods they can be used to evaluate how the environment will change at local scale (Falconer et al., 2020). Based on these results we designed a temperature study with cod, and further challenged the fish with F. noatunensis. Thermal effects alone and in combination with the infection, were studied using transcriptomics and several microscope imaging techniques. An in vitro skin model was used to further study how temperature affects migration capacity and response to a secondary stressor, i.e., oxidative stress. Overall, important mechanisms involved in cod health will be severely affected by the high temperatures predicted to occur in the near future.
2 Materials and methods
2.1 Climate scenarios
Prior to designing the fish trial, temperature data from a commercial cod farm in Dønna (Northern Norway, 66.17°N) was evaluated and climate projection models used to look at possible future temperatures at that location. This farm was selected based on location and availability of farm data. Regional climate model projections of temperature were obtained from a 3D ocean circulation model NEMO-NAA10km (Hordoir et al., 2022) based on the NEMO ocean engine (Madec and The NEMO System Team, 2015) forced with atmospheric data from climate projections from the Norwegian Earth System Model (NorESM2) (Bentsen et al., 2013; Seland et al., 2020). Temperature data from the farm (daily average, 3m) was used to calibrate climate projections (IPCC, Shared Socioeconomic Pathway SSP2-4.5) according to the published bias correction method (BC1), (Falconer et al., 2020). The SSP2-4.5 climate scenario represents a “middle-of-the-road”, medium emissions scenario (Fricko et al., 2017). Seven individual years (2016 – 2022) of temperature data (farm measurements), and 7 individual years (2030 - 2036) of modelled temperatures (BC1 SSP2-4.5; 5-day averages, bias-corrected and interpolated to daily values) were analysed to evaluate the changes in temperature in the near-future, as this timeframe is relevant to present-day decision making.
2.2 Ethics statement
All fish handling and manipulations in the study adhered to the guidelines and protocols of European Union Directive 2010/63/EU and the trial was approved by the Norwegian Food Authorities (FOTS ID 23048).
2.3 Fish trial and sampling
Atlantic cod, produced at the Center of Marine Aquaculture (Nofima, Norway), were transferred to The Aquaculture Research Station (Tromsø, Norway). Fish were kept for acclimatization for 4 weeks in flow-through tanks at 8°C, and fed Amber Neptun (Skretting, Norway). Temperature increase was performed in two stages. First the fish were acclimated to 12°C for one week. Second, 120 fish were distributed in 4 tanks, 30 fish per tank, and temperature in two tanks increased to 17°C. Temperature in the two remaining tanks were kept at 12°C. After acclimatization, fish were moved to the challenge facility at the station where they were kept in 200 L tanks with similar conditions in two replicate tanks per temperature (Figure 1). Average weight and length were 123.4 grams (SD 39,50 grams) and 23.3 cm (SD 2,08 cm), respectively.
First day after transfer (T0), three fish from each tank were killed by an overdose of anaesthetics and sampled. Skin and spleen were transferred to RNA later (Life technologies, Carlsbad, CA, USA) and formalin (CellstoreTM, CellPath, Newtown, UK). RNALater samples were stored at –20°C and formalin samples on 4°C, according to the manufacturer’s protocols.
On the same day, a cohabitation trial with Francisella noatunensis (type strain NCIMB 14265) was started. The bacterium, recently re-isolated from infected Atlantic cod and kept frozen in glycerol, were thawed, and grown on CHAB plates for 4 days at 20°C. Multiple single colonies were resuspended in saline and OD600nm measured. To calculate CFU/ml a duplicate series of ten-fold dilutions of the bacterial suspension was titrated on CHAB plates and incubated at 20°C for 4 days before counting. At challenge, 6 fish per replicate tank at both 12 and 17°C were anesthetised and injected intraperitoneal with 0,1 ml F noatunensis suspension at a challenge dose of 108 cfu/fish, or with saline (uninfected control group). For each of the two temperature groups, 3 injected and 10 or 11 naïve (cohabitants), from each of the two replicate tanks were distributed into each of 4 new tanks as shown in Figure 1, in total 6 injected and 21 naïve fish per tank and with one infected and one uninfected (saline injected) tank per temperature. To be able to know which replicate tank the fish were transferred from, fish in one tank at 12°C and one tank at 17°C were tagged by VIE (visible implant elastomer, Northwest Marine Technologies Inc). The injected fish were tagged with an additional implant. At 3, 14 and 52 days post infection (dpi), 6 cohabitant fish per tank were sampled as described for the T0 sampling (Figure 1). To confirm infection by F. noatunensis, the bacterium was reisolated on CHAB plates from head kidney and spleen of all sampled fish and RTqPCR was run on selected spleen samples using F. noatunensis specific primers (Ottem et al., 2008).
2.4 Histology
Skin and spleen biopsies (N = 6 per treatment per timepoint) were examined in a stereo loupe (Leica Thunder) before embedding in paraffin. In brief, samples fixed in buffered 4% formalin, were carefully dissected, orientated, and placed in tissue embedding cassettes (Simport, Quebec, Canada). The samples were dehydrated through 100% alcohol and then in a clearent Xylene bath, using an automated tissue processor (TP1020, Leica Biosystems, Nussloch GmbH, Germany), before infiltrated in melted 60°C paraffin (Merck KGaA, Darmstadt, Germany). Paraffin-embedded tissue samples were cut in 2 µm sections using a Microtome (Leica RM 2165), mounted on polysin coated slides (VWR, Avantor, Pennsylvania, USA) and dried overnight at 37°C. The sections were deparaffinized and rehydrated and stained using automated special stainer (Autostainer XL Leica Biosystems, Nussloch GmbH, Germany). Paraffin sections of skin were stained with Alcian Blue Periodic Acid Schiff (AB/PAS, pH 2.5, Alcian Blue 8GX, Sigma Aldrich, Darmstadt, Germany), spleen was stained using Hematoxylin Eosin (Sigma Aldrich, St. Louis, MU, USA). Stained samples were scanned using a Leica light microscope slide scanner (Aperio CS2 fra Leica Biosystems, Wetzlar, Germany).
Skin was evaluated in Aperio Image Scope (Leica Microsystems, Wetzlar, Germany). Measurements were done in a region of ca. 1000 μm per section. In each region, epidermal mucous cells were counted and defined as either “outer” (those touching the outer border of the epidermis) or “total” (outer mucous cells including those distributed in the epidermis). Epidermal thickness was measured in 5 different locations of the selected region. The general appearance of the epidermis and the quality of the epithelial surface were characterised using a semi-quantitative 5-point scale skin health scoring system. Spleen was evaluated in QPath (Bankhead et al., 2017). Granulomas in the spleen were evaluated based on their morphology and categorised as either “early” or “developed”. These were counted, area was measured together with total area of the section, and percentage of affected spleen calculated. Statistics were done by ANOVA, followed by Tukey post hoc test showing significant differences (P < 0.05).
2.5 In vitro studies
Atlantic cod keratocytes were cultured from scale explants according to previously described protocol (Ytteborg et al., 2020), at weeks 1 and 8. Scales from fish reared at the two different temperatures were used for cultivation. To evaluate the impact of temperature in combination with secondary stressors other than infection, we exposed skin biopsies to oxidative stress in vitro according to previously described methods (Ytteborg et al., 2020). Skin biopsies were exposed to 1000 ppm hydrogen peroxide (H2O2, Merck, Rahway, NJ, USA) for 40 min, before scales were picked and further cultured. Control biopsies were kept 40 min in salt water. Samples from these biopsies were stored in formalin and RNALater, for histological evaluation and transcriptomics, respectively.
For keratocyte migration assays, 12 well tissue culture plates (Falcon Multiwell™ Becton Dickinson, NJ, USA) were used, 5 scales per well, 3 wells per fish, 5 fish per treatment. Each well contained 1ml L-15 supplemented with fetal bovine serum (FBS) 10%, 25μg amphotericin B, 10ml/L antibiotics, antimycotics and 0.01M HEPES (Sigma Aldrich). Plates were incubated at 12°C or 17°C, corresponding to the temperatures in respective fish tanks, in a cell incubator without CO2. After one, two, three and five days, wells were microscopically analyzed (Leica). Scales with migrating cells (defined as cells moving from the scales to the bottom of the well) were counted at every timepoint and percentage to the total number of attached scales was defined as an indicator of migratory potential. Morphology of migrating cells was documented, and wells were fixed for immunohistochemistry (IHC) using 4% formalin at the end of the experiment (day 7). Live cells were stained with EdU (Invitrogen, Waltham, MA, USA) according to the manufacturer’s protocols and visualized using a Zeiss Axio Observer Z1 equipped with an AxioCam MRc5 camera and AxioVision software (Carl Zeiss Microimaging GmbH, Göttingen, Germany).
2.6 Immunohistochemistry
Fixed cells were washed twice in FBS, incubated 10 min in 50 mM NH4Cl, washed twice in PBS, and permeabilized in 0,1% Triton X-100 for 10 min before blocking with 5% BSA for 30 min. Slides were incubated with primary rabbit anti-mouse iNOS from Affinity Bioreagents Inc. (Golden, CO, USA) diluted 1:2000 overnight at 4° and washed with PBS-T before secondary antibody (Goat anti-rabbit Alexa 488, A11008, Invitrogen) diluted 1:400 including Hoechst 1:1000 (H1399, Invitrogen) and incubated 1 h in room temperature. All dilutions were done with 0,5% BSA. Slides were washed 3x in PBS-T followed by PBS and microscopically examined using Zeiss Axio Observer Z1 (Carl Zeiss Microimaging GmbH).
2.7 Scanning electron microscopy
Skin samples for scanning electron microscopy (SEM) from the two temperature regimes (N = 3 per species per treatment) were dehydrated from PBS to 100% EtOH and dried using a Critical Point Dryer (CPD 030, Bal-tec AG, Schalksmühle, Germany) with liquid carbon dioxide as the transitional fluid. The samples were then mounted on stubs with carbon tape and coated with gold-palladium (Polaron Emitech SC7640 Sputter Coater, Quorum technologies, East Sussex, UK). Imaging was performed at the Imaging Centre, Faculty of Biosciences, Norwegian University of Life Sciences (Zeiss EVO-50–EP, Carl Zeiss SMT, Cambridge, UK).
2.8 RNA isolation
Total RNA was extracted from skin samples and spleen (N = 6 per organ, per treatment, per sampling point) and from skin from the in vitro skin biopsies (N = 6 per treatment). An automated Biomek 4000 (Beckman Coulter, Pasadena, CA, USA) including an on-column DNase treatment were used, according to the manufacturer’s protocol. 2100 Bioanalyzer and RNA Nano Chips (Agilent Technologies, Santa Clara, CA, USA) were used to verify the integrity of the RNA samples (RIN values > 7.5). RNA purity and concentration were measured using a NanoDrop ND-1000 Spectrophotometer (NanoDrop Technologies, Thermo Fisher Scientific). Total RNA samples were stored at −80 °C until prepared for multiple gene expression profiling.
2.9 cDNA and real time qPCR
Skin biopsies from the in vitro trial were analysed using real time quantitative PCR. cDNA was synthesised using Taqman Reverse Transcription Kit (Applied Biosystems, Waltham, MA, USA) in a 20 μL reaction mixture containing 9.6 μL 500 ng template RNA. Thermocycling was performed using a Veriti™ 96-Well Thermal Cycler (Applied Biosystems), with the parameters 25 °C for 10 min, 37 °C for 30 min and 95 °C for 5 min. Transcript levels of selected genes were quantified by real-time quantitative polymerase chain reaction (RT-qPCR) in QuantStudio™ 5 (Applied Biosystems). Each assay consisted of 5 μl of PowerUp™ SYBR™ Green Master Mix (Applied Biosystems), 0.5 μl 10 μM of each forward/reverse primer (Invitrogen) and 4 μl of 1:10 cDNA, with the parameters: pre-incubation at 95 °C for 20 s, 40x amplification cycles at 95 °C for 1 s and 60 °C for 20 s, and a dissociation stage of 95 °C for 1 s, 60 °C for 20 s and 95 °C for 1 s. A five-step standard curve of 2-fold dilution series was prepared from pooled cDNA to determine the amplification efficiencies. Primers are listed in Table 1. Results were analysed using REST (Pfaffl, 2001; Pfaffl et al., 2004).
2.10 Microarray
Transcriptome analyses were performed using Nofima’s 44k genome-wide oligonucleotide Atlantic cod microarray. Analyses included skin and spleen of intact and infected fish, two temperatures and two time-points (one week at different temperatures and seven weeks after challenge with Francisella), totally 59 samples were analysed. Microarrays were manufactured by Agilent Technologies, and all reagents and equipment were purchased from the same provider. RNA amplification and labelling were performed with a One-Color Quick Amp Labelling Kit, and a Gene Expression Hybridization kit was used for fragmentation of labelled RNA. After overnight hybridization in an oven (17 hours, 65°C, rotation speed 0.01 g), arrays were washed (Gene Expression Wash Buffers 1 and 2) and scanned. Subsequent data analysis was performed with Nofima’s bioinformatic package STARS (Krasnov et al., 2011). Global normalization was performed by equalizing the mean intensities of all microarrays. The individual values for each feature were divided by the mean value of all samples producing expression ratios (ER). The log2-ER were calculated and normalized with the locally weighted non-linear regression (Lowess). Differentially expressed genes (DEG) were selected by criteria: 1.75-fold and p<0.05. STARS annotations were used for comparisons of functional groups of genes, difference was assessed with t test (p < 0.05).
3 Results
3.1 Future seawater temperatures
Looking at the temperature data from the farm at Dønna, two years from 2016 to 2022 had average daily temperatures above 15°C, with a maximum temperature of 16°C (Figure 2). At this site, the BC1 model projections suggest higher temperatures in future years (2030 – 2036); six years had days when the temperatures were above 16°C, and more than half of the years had temperatures above 17°C, with a maximum temperature of 19.5°C.
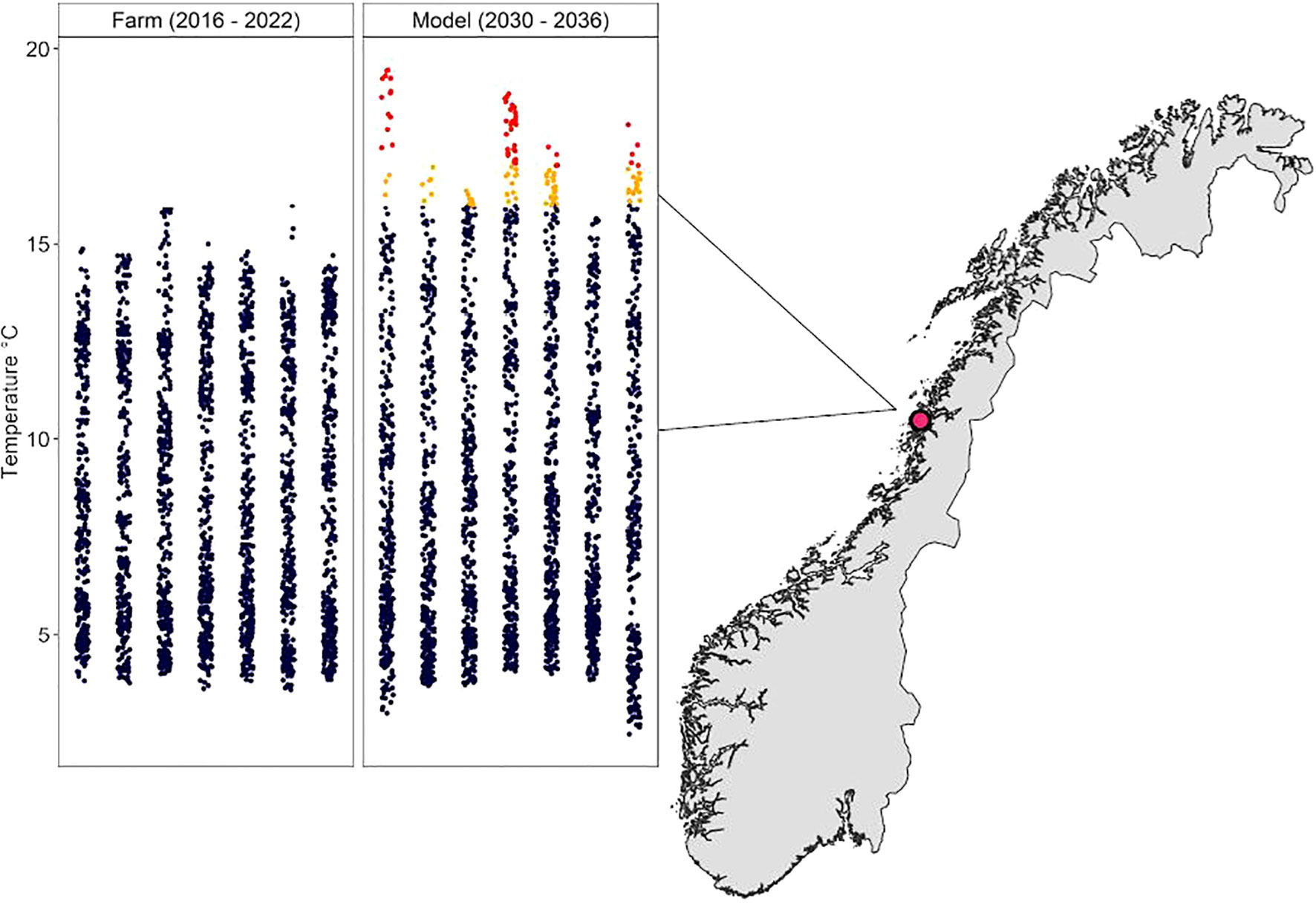
Figure 2 Map showing Dønnas´ location in Norway and the temperatures at the farm 2016 – 2022 and the model projection temperatures 2030 - 2036, temperatures below 16°C in blue, 16-17°C in orange, and above 17°C in red.
3.2 Fish trial
No significant differences in growth were observed between any of the groups during the trial period, but at the end of experiment the tendency was a higher growth in fish groups kept at 12°C compared to the 17°C groups (data not shown). No mortality was registered during the trial. While uninfected control fish at both temperatures had very few internal macroscopic changes throughout the trial, increasing macroscopic changes were observed on internal organs in infected fish, specifically granulomas in one or several of the organs; head kidney, spleen, liver and skin (fins), and swollen spleen and head kidney (results not shown). These observations were done on 2/6 sampled fish 14 dpi and on 4/5 sampled fish 52 dpi in the 17°C group, but only on 1/6 fish 52 dpi in the 12°C group. F. noatunensis was reisolated from head kidney and spleen in sampled fish from the infected groups at 52 dpi, while all of the sampled fish from the control groups were negative. The infection was also confirmed by qPCR in 2 out of 6 spleens at 14 dpi and in 3 out of 4 spleens 52 dpiin the 17°C group (results not shown).
3.3 Development of granulomas in the spleen is temperature dependent
A significantly higher number of granulomas were detected in the spleen from the 17°C group (Figure 3). This temperature-dependent profile was further reproduced in both the number of early and developed granulomas, as well as the area of affected tissue (measured as % of spleen affected).
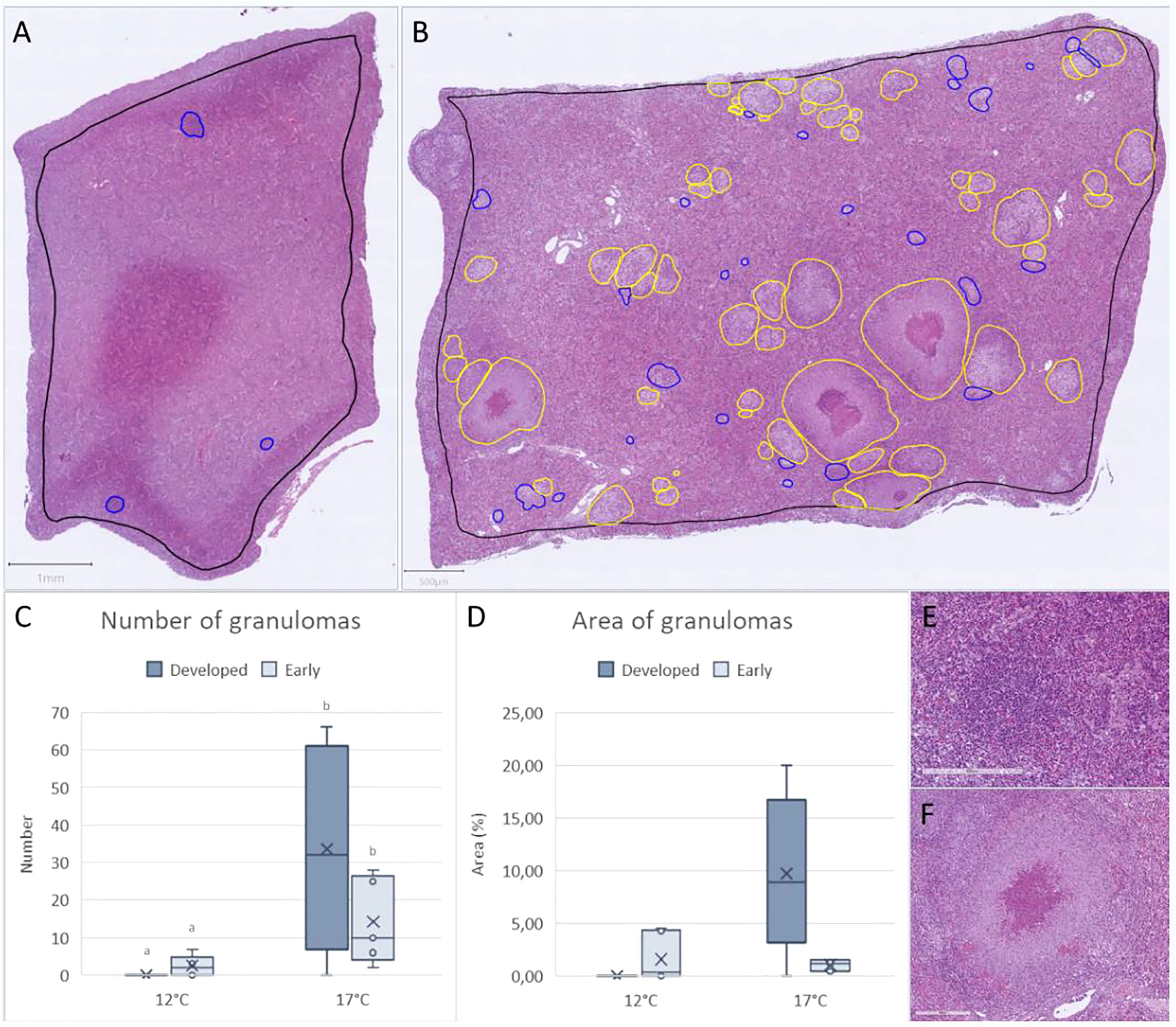
Figure 3 The number and area of early (blue) and developed (yellow) granulomas in spleen of (F) noatunensis-infected Atlantic cod. (A) Example from early developing granulomas (scale bar 800µm). (B) Example from a highly affected spleen with several developed and early granulomas (scale bar 500µm). Histological scoring of granulomas in spleen showing (C) Number of granulomas and (D) area of granulomas in spleen from 12°C (n=6) and 17°C (n=5). (E) Example of early and (F) developed granuloma in spleen (scale bar 200 µm).
3.4 Temperature and francisellosis have compounding effects on skin
Fish from the 17°C group had a lighter skin color and weaker spots compared to fish from the 12°C group (Figure 4). This could be clearly observed in the whole fish, and the difference became more apparent when evaluating skin biopsies under the stereo loupe. Melanocytes in skin from the 12°C group were darker and denser, while melanocytes from the 17°C group had spindle shaped appearance and weaker color. There were no obvious differences between non-infected and infected fish from the 12°C group, but infected fish from 17°C had darker skin color and higher frequency of melanocytes. Melanocytes in this group were darker and denser compared to non-infected fish from the same temperature regime.
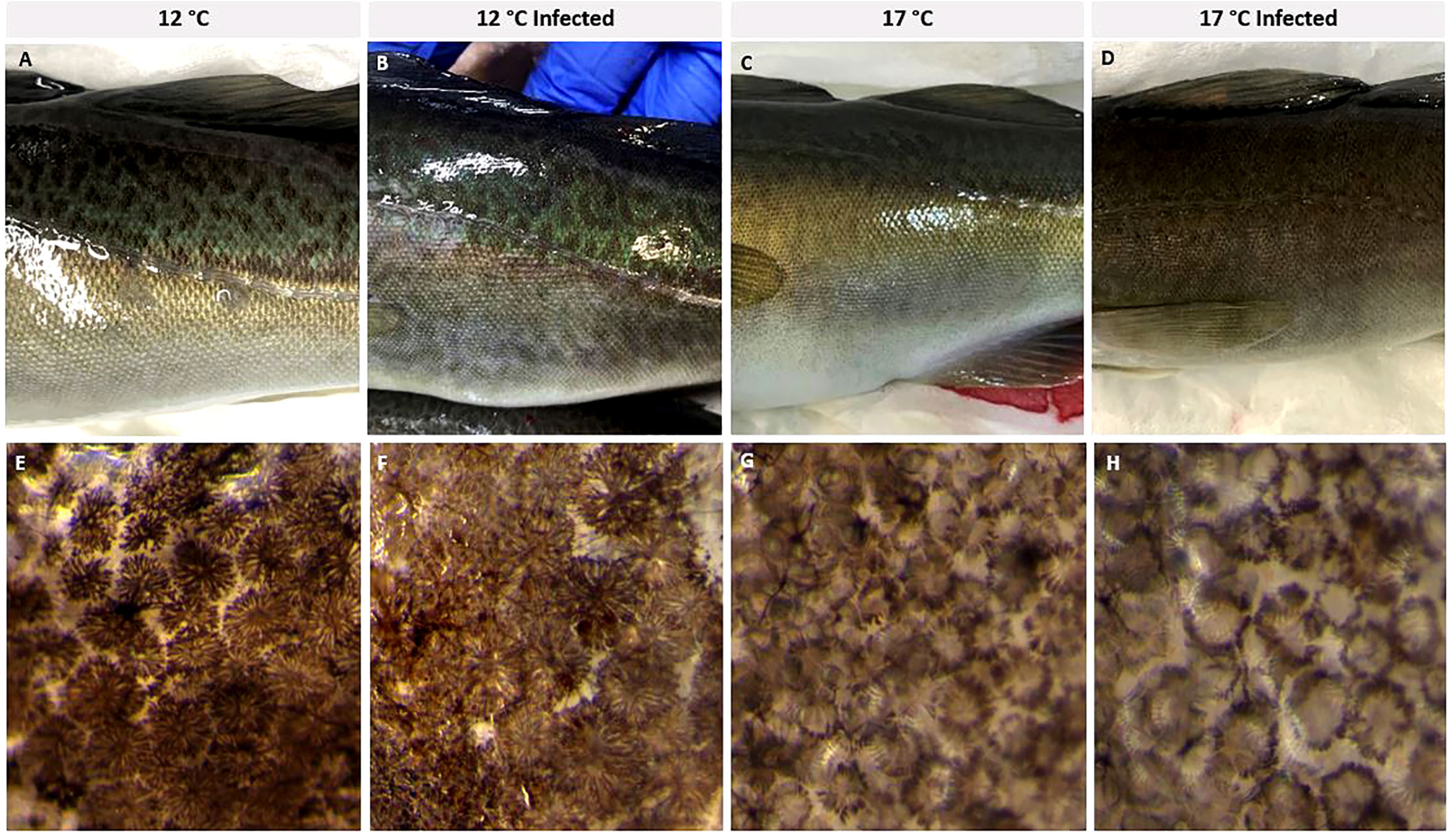
Figure 4 Skin appearance of representative specimens from the non-infected and infected fish from 12°C (A, B) and 17°C (C, D) group. (E-H) Loupe images of skin from the same groups showing the different morphology of the melanocytes. N = 6 per treatment.
Histological measures (Figure 5) showed increased epidermal thickness in skin from fish from the 12°C uninfected group after 5 weeks compared to fish from 17°C, both in uninfected and infected fish and to infected fish from the 12°C group. Eight weeks into the trial, the 12°C group still had thicker epidermis, and infected fish from the 17°C group had significantly thinner epidermis compared to 17°C control fish. Numbers of sensory cells were higher in the 17°C group 2 weeks into the trial, but this was changed at week 5, when the 12°C fish had higher numbers of these cells compared to the 17°C group. Sensory cells did not change in the infected fish, but these fish had higher numbers of club cells. Infected fish from the 12°C group showed this pattern already from week 3, infected fish from both temperature regimes had increased club cell number at week 5 and 8. Position of the club cells in the epidermis did not differ between the groups. Histological scoring further showed that the epidermis in fish from the high temperature regime was more damaged compared to the low temperature regime, for both non-infected and infected fish. Spacing of the epidermis was more pronounced in fish from 17°C, and more melanin was observed in infected fish from the same temperature (Figures 5H–J).
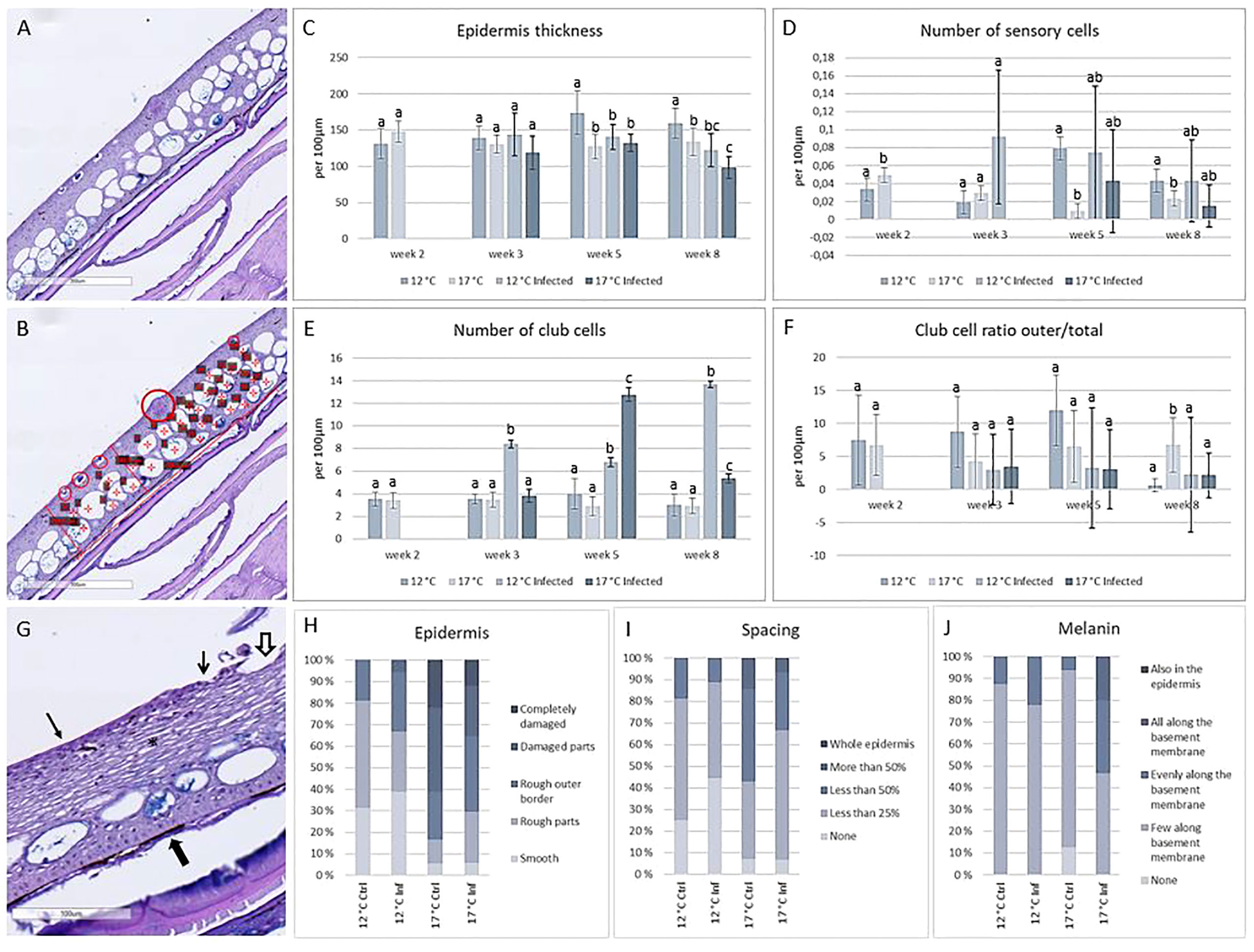
Figure 5 Histological measurements and scoring of skin. (A) Skin sample from cod, 12°C non-infected. (B) Same sample with measures; large circle: sensory cell, small circles: outer club cells, asterix: club cells, line: epidermal thickness. (C) Thickness of epidermis. (D) Number of sensory cells per 100µm of skin. (E) Number of club cells per 100µm of skin. (F) Club cell ratio outer/total. (G) Histological evaluation of smooth epidermis (arrow), rough epidermis (open arrow), damaged epidermis (hollow arrow), melanin (filled arrow) and spacing (asterix). (H) Epidermis, (I) Spacing and (J) Melanin scoring in the four treatment groups. N = 6 per treatment per timepoint, significant differences (P < 0.05) marked with different letters.
3.5 High temperature increases the impact of oxidative stress in skin
Skin samples from week 8 exposed to H2O2 showed damages like rougher outermost border of the epidermis, increased spacing of the epidermis, and detachment of the epidermis to the basement membrane. Differences between the two temperature regimes were minor, but more severe cases of damage could be found in the high temperature group (Figure 6).
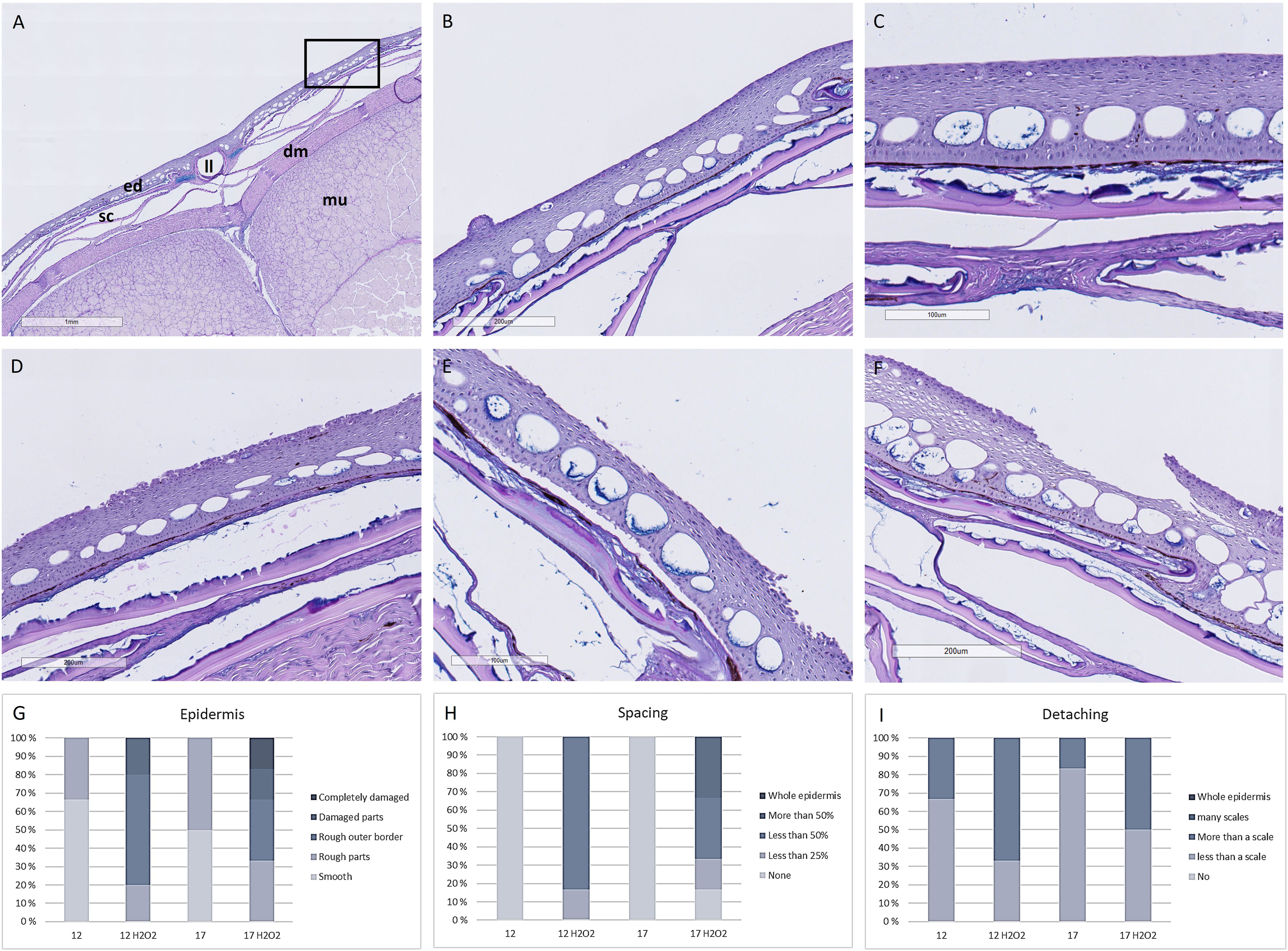
Figure 6 Hisotological measures of skin biopsies. (A) Overview of skin, (B) Higher magnification of box in A showing smooth outer border of epidermis with a sensory cell (arrow) and (C) No spacing between keratocytes in the epidermis in skin samples from fish from the 12°C group. Representative images of skin with (D) Rough outer border (arrows), (E) Detatching epidermis from the basal membrane (arrows) and damaged epidermis and (F) Damaged epidermis with spacing (arrow). Scoring of N=6 samples from each treatment of (G) Epidermis, (H) Spacing and (I) Detachment from the basal membrane. mu, muscle; sc, scales; dm, dermis; ed, epidermis; ll, lateral line. N = 6 per treatment.
Analysing skin biopsies with SEM mirrored the results of histology (Figure 7). More severe damages to the outermost keratocytes, including breaches in the cell layer, dead cells and loss of epidermis were found in the high temperature group and in both groups exposed to H2O2, with pronounced damages in the high temperature group. In skin samples from the high temperature group, areas were found with severe loss of keratocytes, exposing the deeper layers of the epidermis. Damages in the keratocytes lining the sensory cells were also found in exposed groups. With increasing temperature and H2O2 exposure, epidermis got more fragmented, keratocytes lost definition of microridges and detached from the continuous outermost epidermal layer, leaving breaches and exposed areas.
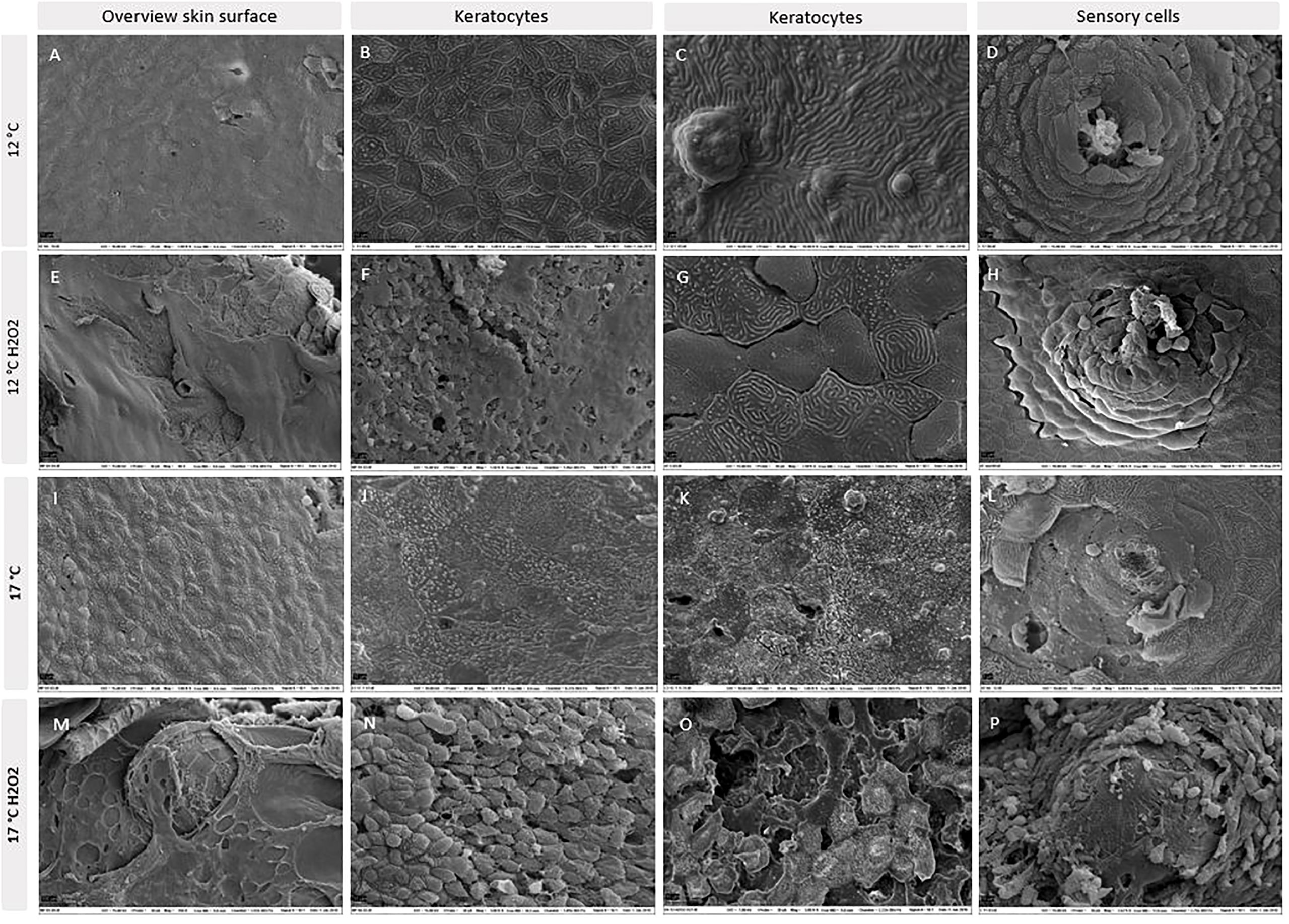
Figure 7 SEM images of skin biopsies from 12°C (A–D), 12°C exposed to H2O2 (E–H), 17°C (I–L) and 17°C exposed to H2O2 (M–P). N = 6 per treatment.
3.6 Keratocytes are affected by temperature and oxidative stress
The migration capacity of the keratocytes from scale explants was analysed at week 1 and 8. Differences between the temperature regimes and between the exposed and non-exposed explants were clear. Keratocytes from scales picked from fish at 17°C had higher migration capacity compared to keratocytes from the 12°C group (Figure 8) at days one and three (only at week 8, Figure 8F), but decreased migration at days six and seven, when more scales from the 12°C group had migrating cells. Scales from 12°C showed 55-65% migration compared to 25-30% at 17°C after a week in culture. Scales exposed to H2O2, had lower migration rates compared to the unexposed controls, with the 17°C group having migration from less than 10% of the scales after seven days. In comparison, scales from the 12°C group reached almost 70%. The proliferation marker EdU, used to assess the proliferation capacity, showed more positive cells identified in cell sheets from the 12°C group. Immunohistochemistry using antibodies against iNOS (stress marker) showed less activity in scale explants from the 12°C group compared to all other groups.
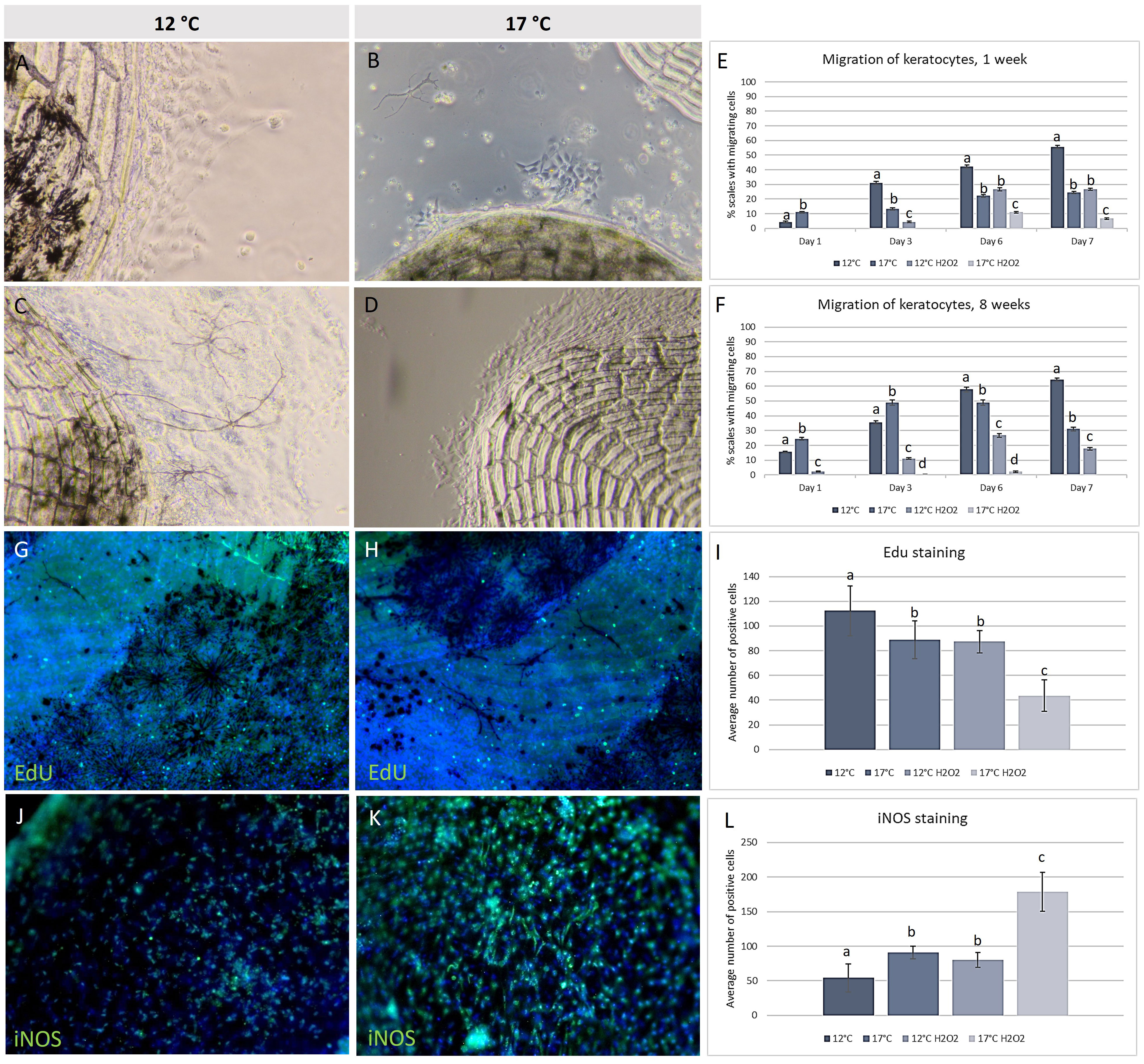
Figure 8 In vitro keratocyte cell culture. Migrating keratocytes from scales at (A) 12°C, (B) 17°C and same temperature after H2O2 exposure (C, D). Migration capacity showing % of scales with migrating cells, at (E) week 1 and (F) week 8. Cells were stained with EdU and showed higher proliferations rate at (G) 12°C compared to (H) 17°C, as measured (I). IHC with iNOS showed weaker staining at (J) 12°C compared to (K) 17°C, as measured (L). Different letters indicate significance (P ≤ 0,05. Scale bar = 200 µm. Green color = positive EdU/iNOS, blue color = Hoechst. N = 5 scales per well, 3 wells per fish, 5 fish per treatment, significant differences (P < 0.05) marked with different letters.
Observations on scales from the two different temperature regimes were in concordance with the overall skin appearance: Melanocytes in scales from the 12°C group were denser and darker compared to melanocytes in the skin from the 17°C group. After H2O2 exposure, melanocytes from both temperature regimes changed. In the 12°C group, melanocytes appeared spindle-shaped with a weaker colour, while melanocytes from the 17°C group were fragmented and nearly disappeared from the scale explants (Figure 9).
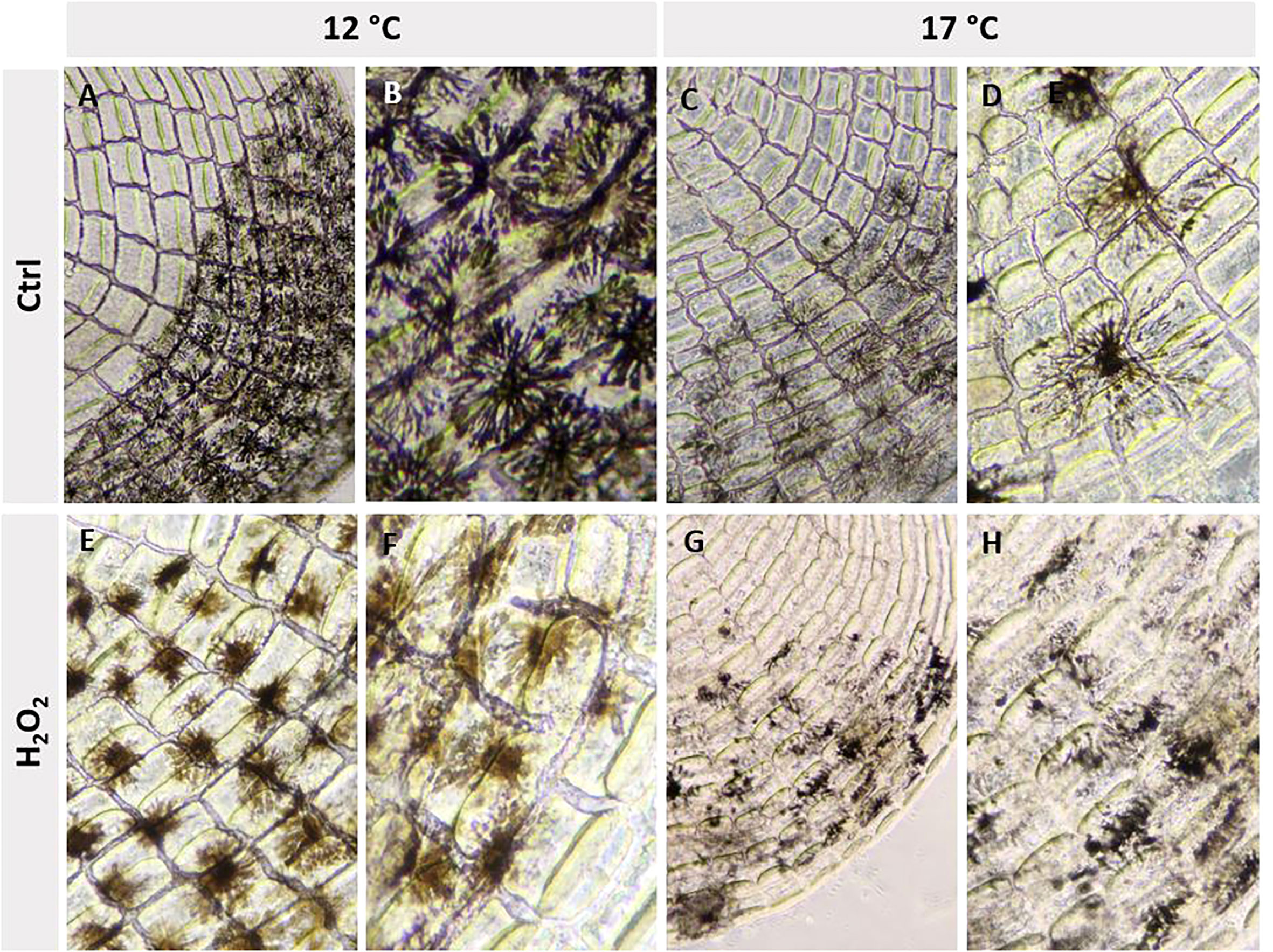
Figure 9 The melanocytes had different morphology at 12°C (A, B) and 17°C (C, D), and after exposure to H2O2 (E–H).
The expression of selected genes in skin biopsies, which were further exposed to H2O2, was determined in the two temperature groups at weeks 1 and 8. RT qPCR analysis (Figure 10) included proinflammatory cytokines (tnfα, il1β and il8) and antibacterial agent lysozyme, gene markers of responses to protein damage (hsp70, hsp90 and sip1) and oxidative stress (sod, catalase and gsh). Caspase 6 and fortilin are involved in variety of cellular processes including apoptosis and inflammation. At week 1, il1β and il8 were stimulated with the increase temperature, exposure to H2O2 and their combination. Tnfα and lysozyme were suppressed by joint treatment as well as caspase 6 and fortilin. The markers of protein damage showed a tendency to upregulation though significant difference from 12°C was found only in 17°C group. The expression profiles of enzymes involved in oxidative stress response were different. Catalase and gsh (not significant) were downregulated in respectively 17°C and H2O2 17°C groups, while sod expression was stimulated with H2O2 at both temperatures. Analysis performed at week 8 indicated reduced activation of several genes. Four genes did not show any difference between the groups (hsp90, sip1, sod and catalase). High temperature stimulated, tctp, il1β and il8, while tnfα was downregulated. H2O2 exposure led to increased transcription of hsp70 and gsh (only at 12°C) and reduced transcription of caspase 6 and lysozyme at both temperatures, and tnfα and il1β at 17°C.
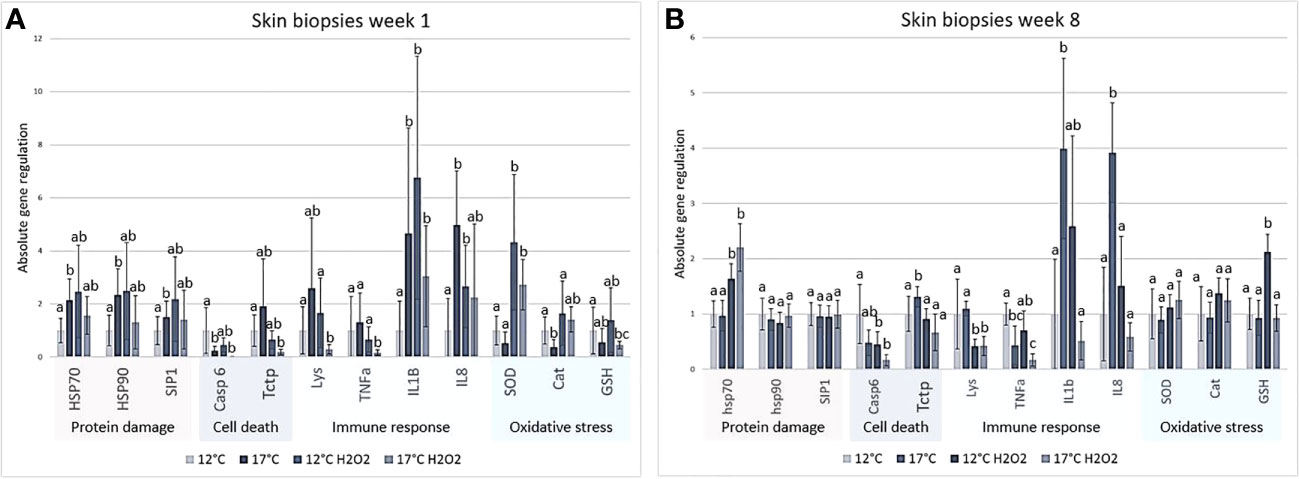
Figure 10 Real time qPCR of skin biopsies exposed to H2O2 from (A) 1 week and (B) 8 weeks. Relative gene expression is shown as mean ± std, different letters indicate significance (P ≤ 0,05), N = 6 per treatment per timepoint. Results were analysed using REST (Pfaffl, 2001; Pfaffl et al., 2004), significant differences (P < 0.05) marked with different letters.
3.7 Microarrays
Microarray analyses (GEO Accession viewer (nih.gov)) were performed on skin and spleen samples from the non-infected and infected fish at two temperatures, at weeks one and eight (Figures 11A–C). Judging by the number of DEG, effect of temperature was equal at both time-points in skin and increased with time in the spleen. The gene expression differences between the temperature regimes markedly decreased for both tissues at high temperature. Responses to challenge with Francisella were markedly greater at 17°C in both tissues. At week 1, the effect of high temperature was apparently larger in the spleen as shown by the substantially higher number of DEGs compared with the skin. Several functional groups of genes showed co-ordinated expression changes (Figure 11D). Genes involved in cell cycle were downregulated in both tissues. Their number was twice higher in skin that also showed decreased expression of genes encoding chromosomal proteins.
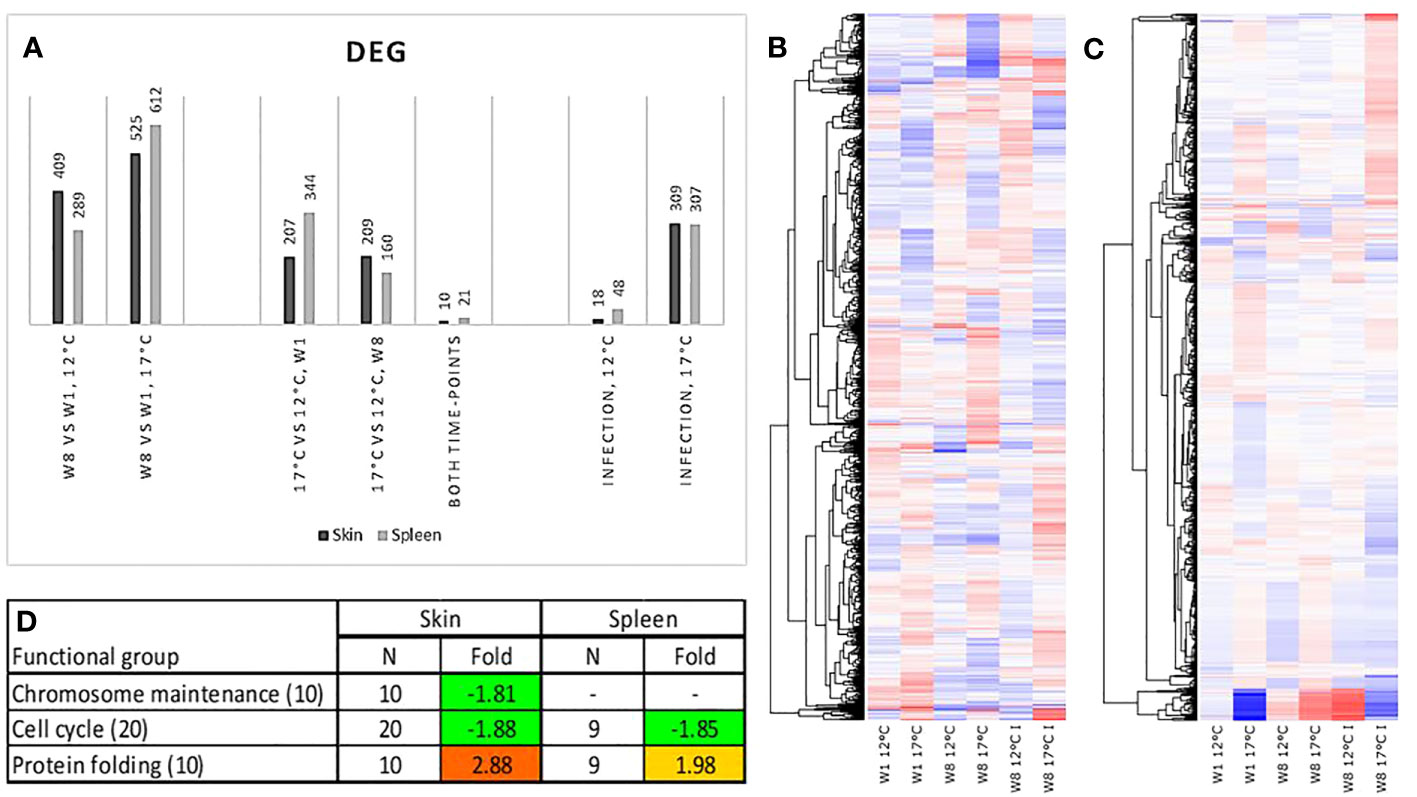
Figure 11 (A) Number of differentially expressed genes (DEGs). Heat maps of gene expression in (B) skin and (C) spleen. Genes with expression differences between the time-points, temperatures or infected and control fish were clustered with K means. Data are log2 – expression ratios to the means of all samples (D) Functional groups with co-ordinated expression changes at week 1, differences from 12°C are significant, data are folds and differential expression is highlighted with underlined bold italics.
Effects of temperature alone and the interaction of temperature and infection status at individual gene levels was further evaluated. Eleven genes responded to temperature in both tissues including heat shock protein 47, heat shock protein 90, cold inducible RNA binding protein, and metallothionein (Figure 12). Heat shock proteins and metallothionein coding genes were upregulated while the other genes were downregulated. Protein stress was indicated by upregulation of eight chaperones in addition to two chaperones presented in Figure 12A. Putative suppression of cell proliferation in the skin could be to some extent compensated by stimulation of genes that control the development of tissue, such as genes encoding connective tissue growth factor, enzymes modifying extracellular components and regulators of differentiation (Figure 12B). High temperature also affected genes associated with histogenesis of the spleen (Figure 12C). In contrast with the skin, downregulation prevailed, and most DEG encoded the components of extracellular matrix including the proteins of cartilage and bone.
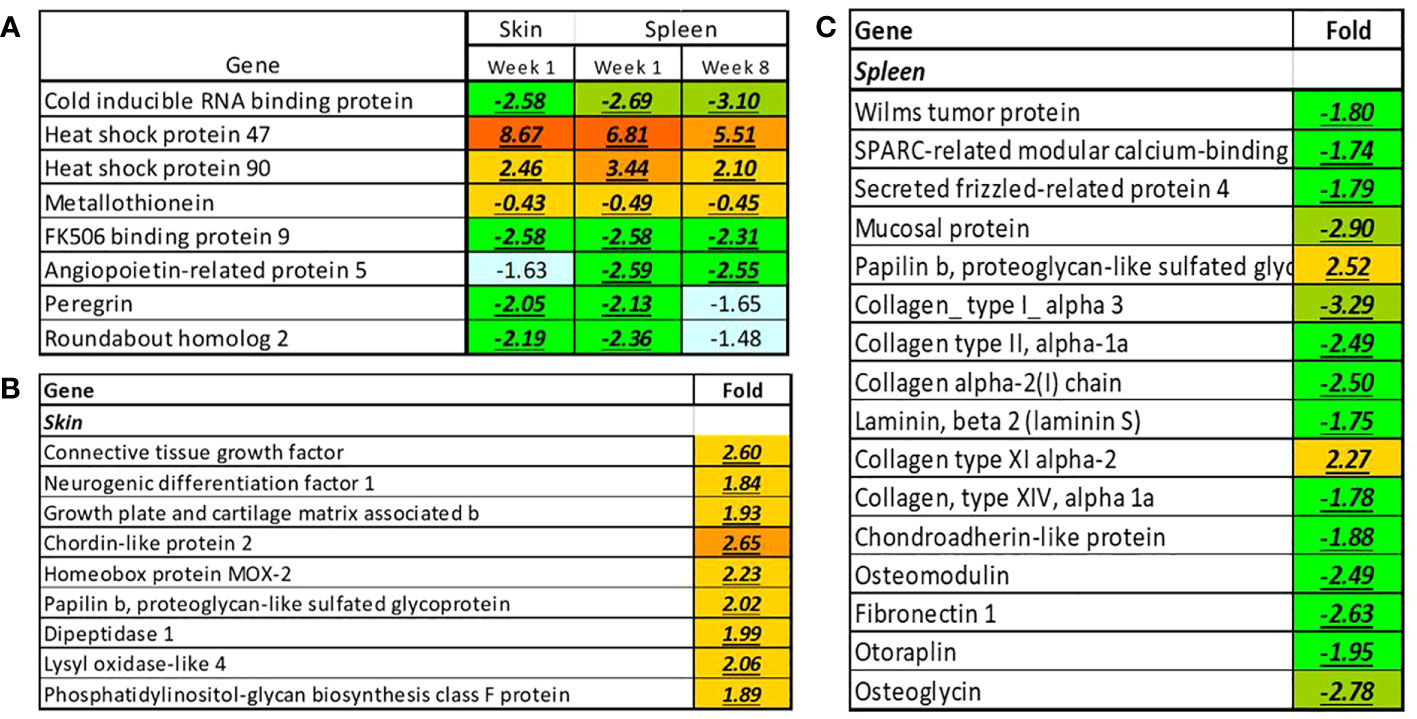
Figure 12 Genes responsive to temperature changes in the skin and spleen of Atlantic cod. (A) Genes responsive to elevated temperature in both organs, (B, C) Individual genes with tissue-specific expression changes, week 1. Data are folds and differential expression is highlighted with underlined bold italics.
Elevated water temperature could affect the full range of immune functions including pathogen detection, signalling via chemokines, cytokines and lipid mediators, antigen presentation, humoral and cellular effectors, lectins, lymphocyte-specific genes, and regulators of various pathways; these effects were seen at both time-points (Figure 13). Of interest are toll receptor 8 and nilt1 that also can be involved in the detection of pathogens (Stet et al., 2005). The greatest 76.6-fold increase was seen in il-2 receptor in spleen at week 1. The expression of several immunoglobulins transcripts (ig, highlighted with bold blue in Figure 13) showed a decrease in both tissues at week 1 and accompanied by a massive drop in the spleen at week 8: 21 features with average 2.65-fold decrease.
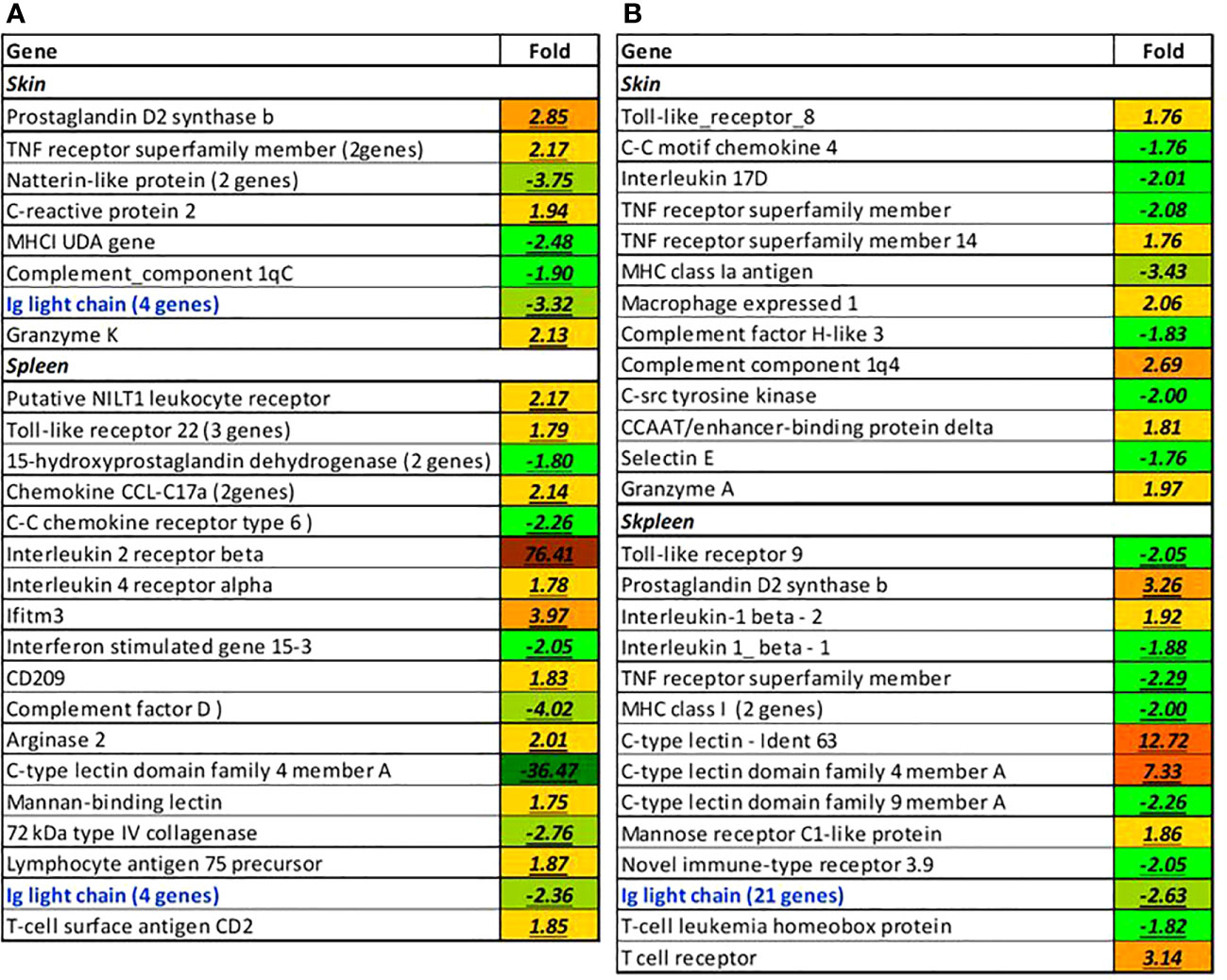
Figure 13 Selected immune-related genes affected by elevated temperature at week 1 (A) and 8 (B), folds to control. If more than one paralog was affected (blue), the number of genes is indicated in parentheses. All differences from control are significant.
We then evaluated the effects of F. noatunensis infection at two different temperatures. Six weeks following challenge with Francisella gene expression changes were minor at 12°C, especially in the skin (data not shown). Thereby we focused the assessment on the groups reared at 17 °C. Stimulation of blood circulation indicated by the 8.1-fold increase of eight hemoglobin transcripts in the skin and chemokine signaling in the spleen was in parallel with the downregulation of cell cycle proteins and collagens in these tissues (Figure 14A). A small number of immune genes was downregulated including tlr9, a lectin, extracellular proteases (chymase and three granzyme-like genes), and a protease inhibitor (tnfa-induced protein 8) in the spleen (Figure 14B), four genes encoding arachidonate 12-lipoxygenases, the enzymes of eicosanoid metabolism, protease htra1b and two lectins in the skin (Figure 14C). However, immune stimulation prevailed in both tissues. In the spleen, the upregulated pathogen recognition receptors were represented with nilt1, tlr8 and tlr21. Signalling was mediated with a suite of chemokines and il1b known as a highly active proinflammatory cytokine. Activation of antigen presentation was suggested by several mhci components, and the antigen transporter tap. The effector arm included complement factors, multiple lectins that may bind to pathogens and promote leukocyte traffic, and b-245, a component of oxidative burst; arginase ii is characterized with strong responses to bacterial infections in teleosts (Krasnov et al., 2021). Lectins, complement factors and proteases were activated in the skin. Francisella also induced a panel of immune regulators including imap gtpases. Their role is unknown, but salmon homologs show very high immune activity. Increased expression was observed in pu.1, skap2 and samsn1a, which may control the development and activity of B cells. The immune responses were supplemented with stimulation of apoptosis, autophagy, and detoxification. A panel of caspases and dram1, a gene marker of autophagy was upregulated in the spleen. Moreover, xenobiotic metabolism was represented with three enzymes upregulated in skin. Stimulation of metallothionein points to possible sequestration of zinc that could reduce its availability for the pathogen.
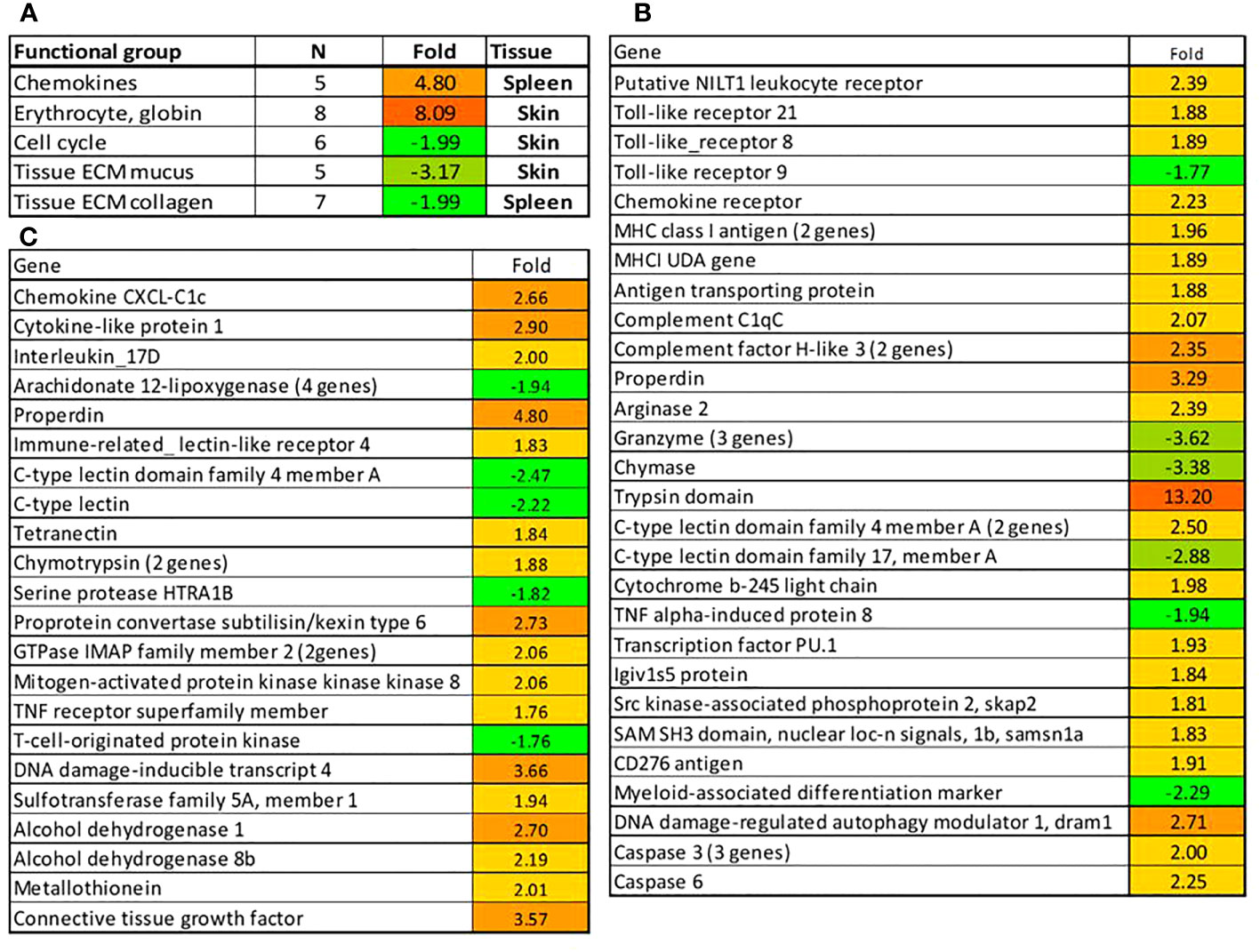
Figure 14 Responses in the spleen and gills to challenge with F noatunensis at 17°C. Samples were collected 6 weeks after experimental infection. (A) Functional groups of genes with co-ordinated expression changes. (B, C) Genes with differential expression in spleen (B) and skin (C), folds to uninfected control. All differences from control are significant.
4 Discussion
There is an urgent need to understand how climate change will impact aquaculture farming conditions so that the industry can develop appropriate responses and protective measures in advance. To study realistic scenarios and assess risks, local farm conditions should be considered, especially when high temperatures will act in combination with secondary stressors and pathogens. Given the complexity of the interactions, multifaceted studies are required. We report a step in this direction that included profiling of climate projections at a Norwegian cod farm site, in vivo and in vitro trials, and powerful analytic methods to understand how high environmental temperatures and secondary stress affect the performance of Atlantic cod. We included in vivo F. noatunensis infection to evaluate the impact of temperature stress in combination with a pathogen, and in vitro exposure using H2O2 as an oxidative stressor, to simulate production related stress. The analytical approach focused at finding biological significance of increasing temperatures combined with secondary stressors, including general performance, skin barrier responses, pathological changes in the spleen and transcriptomics, to understand risk factors associated with future temperatures.
4.1 Near future temperatures will increase beyond cods preferred temperatures
The future scenario from the IPCC (SSP2-4.5, intermediate emission scenario) downscaled to local conditions, showed that temperatures potentially will increase from maximum temperatures around 15°C in 2016 – 2022, to above 17°C in 2030- 2036. As 15°C are considered high for cod, reducing the health and welfare of the fish (Hori et al., 2013; Larsen et al., 2018), these temperatures will challenge productive farming. The site we included in this work was located in North Norway. Most of the commercial cod farming takes place further North, but new locations further South (in the Bergen area) were recently suggested by the Norwegian Directorate of Fisheries. According to The Special Report on the Ocean and Cryosphere in a Changing Climate (IPCC, 2019), there is high confidence in the IPCC ocean climate projections and that climate-induced stressors on aquatic ecosystems present risks for commercial fisheries and aquaculture, with implications for regional economies, cultures, and the global supply of fish. From the modelling of future temperatures at the cod farm site, 17°C is far from an unrealistic scenario, although we are aware that applying a multiple of climate change scenarios with a global multi-model ensemble and several dynamical and statistical down-scaling to farm site level would increase the confidence of the projected temperature ranges. Also, this study used climate model data that had been averaged to 5-days intervals, which may mask more extreme temperatures and days with high temperatures. Challenges with temperature have also been reported in other countries that have tried to farm cod, such as Canada. In Newfoundland waters, cod can be exposed to temperatures of up to 20°C (6 m) and short-term (daily/weekly) temperature fluctuations of as much as 10°C during the summer months (Gollock et al., 2006). These large temperature fluctuations and high summer temperatures, as well as health challenges due to sudden temperature changes and long periods of temperature outwith optimal conditions, were some of the technical challenges that ended Canadian attempts at commercial farming of cod (Nardi et al., 2021). From our study it is obvious that temperatures at 17°C are too high for cod, as also previously suggested by other studies (Pérez-Casanova et al., 2008a; Larsen et al., 2018). This study focused on conditions in the near-future, and were from an intermediate emission scenario, but even higher temperatures may be expected in the longer term, or with higher emissions scenarios such as SSP5-8.5 (IPCC, 2019/ IPCC, 2023). Other cod aquaculture locations will also be affected by increasing temperatures, thus, precautions and adaptation measures are needed to sustain current farming practice in the future, as well as increased efforts to reduce the sectors own emissions. Mean ocean temperature increase may lead to further decline, and in worst case to a collapse, of several coastal cod stocks (Drinkwater, 2005), thus adaptation of aquaculture to climate change may be of even greater importance.
4.2 Cod farmed at high temperature exhibit distinct stress response profiles
Atlantic cod has a low tolerance to temperature change (Lannig et al., 2004), and like other ectotherms, its physiology is directly influenced by the seawater temperature (Mccormick, 2011). During our 8 weeks in vivo infection trial, significant differences in growth were not detected between groups. However, the individual variance in weight was relatively large at start of the trial and the weight and length data were only registered from a small number of fish (N=6) in each group at each of the sampling points. Nevertheless, there was a tendency that fish in both of the 17°C groups had a lower wight gain from start till end of the trial compared to the 15°C groups Growth rate of Atlantic cod was not affected by disease nor temperature after infection with B. pinnipedialis at 15°C either, and findings were explained by multiple energy demanding processes taking place at the same time (Larsen et al., 2018). Studies including multiple stressors, e.g., high temperature and infection, may highlight cumulative stress responses and are especially important when studying biological impact of climate related stressors. Simultaneous stressors may lower the cod’s thermal tolerance, as previously shown for other species, and several studies have linked increasing seawater temperatures to increased outbreaks of marine diseases (reviewed in Burge et al., 2014). We did not observe any mortality in any of the groups, but our results clearly showed that fish are more vulnerable to both oxidative stress and francisellosis at high temperature. The latter is in line with observations from the field and other experimental studies of francisellosis in cod, showing faster and more comprehensive disease development with increasing temperatures (reviewed in Puvanendran et al., 2022)
Obvious differences in skin coloration were observed between fish from the two temperature regimes, with the characteristic spotted cod skin pattern more or less lost at 17°C. In both Atlantic salmon (Salmo salar) and Atlantic mackerel (Scomber scombrus), changed skin color has been observed after exposure to stress (Thorsen, 2019; Tveit et al., 2022). In this trial, melanocytes in high temperature exposed skin had spindle shaped appearance and appeared weaker, probably causing the overall weaker impression of color in these fish. Further evaluating skin from the different temperature regimes showed reduced thickness of the epidermis, reduced number of sensory cells and increased number of club cells in the outer border of the epidermis of fish exposed to high temperature. Similar alterations in skin have previously been connected with increased stress response in Atlantic cod (Ytteborg et al., 2020), as well as in lumpfish (Cyclopterus lumpus) and salmon (Karlsen et al., 2021; Ytteborg et al., 2023). Interestingly, severe morphological changes in skin were not observed until the end of the trial, at week 8, indicating long term effects of 17°C and highlighting the importance of running temperature exposure trials for longer periods. Based on these findings, a sudden reduction in the coloration of the skin and weaker spots may be considered as an indicator of temperature related stress in farmed cod, and farmers should pay special attention to these changes when entering warmer periods.
There were clear signs that the fish were under stress at elevated temperatures as shown by key stress-related genes in the core gene set responsive to temperature in both skin and spleen. Heat shock proteins (hsps), also known as stress proteins and extrinsic chaperones, are a family of highly conserved proteins of varying molecular weight (ca. 16-100 kDa) produced in all cellular organisms when exposed to a stress condition, thus often considered classic markers of heat stress in fish (Roberts et al., 2010). They function as key regulatory molecules for refolding of misfolded proteins, thereby contributing to the maintenance of cellular homeostasis (Shan et al., 2020). Here we identified hsp47 and hsp90 as highly responsive to changes in temperature in both organs. The results corroborated an earlier study in Atlantic cod exposed to a heat challenge where the expression of these genes was shown to be upregulated in liver, head kidney and skeletal muscle (Hori et al., 2010). Hsp47 (serpin h1), which is essential for collagen processing in endoplasmic reticulum under normal condition, prevents secretion of damaged procollagens with abnormal conformation from stressed cells and its induction by heat stress is regulated by a heat shock element in its promoter region (Nagata, 1996; Ishida and Nagata, 2011). On the other hand, Hsp90 and its co-chaperones orchestrate crucial physiological processes such as cell survival, cell cycle control, hormone signaling, and apoptosis, and utilizes its ATPase activity to drive conformational changes to facilitate client protein binding, stabilization, and activation (Hoter et al., 2018; Chen et al., 2020). Regulations of these hsps and several other chaperone protein coding genes identified allow Atlantic cod to master adaptive responses to the physiological demands of the heat stress. Moreover, responsiveness of these genes to temperature in several tissues indicates their ubiquitous role during heat stress, and perhaps, they can be considered as key biomarkers for climate-related heat stress response in cod. This function has been reported in different fish species and thus can be considered a cross-species marker for climate-related stress (Lele et al., 1997; Stefanovic et al., 2016; Wang et al., 2016). We also identified another potential biomarker, cold-inducible RNA-binding protein (cirp), an intracellular stress-response protein that affects expression of many genes by regulating mRNA stability in response to various stressors (reviewed in (Zhong et al., 2021)). CIRBP encodes an 18-kD protein consisting of an N-terminal RNA recognition motif (RRM) and a C-terminal arginine-rich region and initially associated with cold-shock, but recent evidence demonstrates hypoxia, UV radiation, glucose deprivation, and heat stress (Zhong and Huang, 2017). Though the functional role of this has not yet been elucidated in cod, upregulation of CIRP protein was observed in skin mucus of Atlantic cod infected with Vibrio anguillarum indicating its role mucosal immunity (Rajan et al., 2013). The identification of molecular biomarkers will facilitate a better understanding of how Atlantic cod responds and behaves to heat stress and the current study have identified potential candidates that can be further verified, especially their reproducibility under different thermal scenarios.
4.3 High temperature alters the immune response to francisellosis
The interaction of immune system and temperature has long been identified, but discussion in fish has recently received significant attention in light of the threats from elevated temperatures associated with climate change. It is known that when temperatures increase and reach the upper permissive range, teleost immunity is compromised thereby making them vulnerable to challenges and stressors (Cabillon and Lazado, 2019; Scharsack and Franke, 2022). The spleen is regarded as the primordial secondary lymphoid organ while the presence of mucosa-associated lymphoid tissue in the skin highlights its immunological features in fish (Bjørgen and Koppang, 2021). Both of these organs mount immune responses to various environmental stressors, including temperature (Hori et al., 2013; Scharsack and Franke, 2022). Here we showed that elevated temperature affected the immunological functions of both organs as shown by the changes in an array of immune-related genes and the responses were both acute (week 1) and persistent (week 8), though the magnitude of responses were more pronounced in the spleen. Because immune genes showed both up and downregulation, it is difficult to judge the character and consequences of high temperature for Atlantic cod immunity. Nonetheless, some of the genes provide insights into the immunological impacts of elevated temperature in cod. Lectins mediate pathogen recognition via the carbohydrate recognition domain that specifically and reversibly binds to a carbohydrate which is widely distributed in bacteria, fungi and viruses, thereby activating the innate immune system (Elumalai et al., 2019). The present study identified that splenic lectins were heavily influenced by elevated temperature, particularly, c-type lectin domain family 4 member A. There was a dramatic downregulation at week 1 and upregulation was observed at week 8. In trout, c-type lectin domain family 4 is expressed in monocytes/macrophages (Johansson et al., 2016). It remains to be functionally verified the relevance of this striking trend, but downregulation at week 1 might indicate suppressed macrophage-mediated activity but likely compensated by other immune molecules that have been activated. Immunoglobulins (Igs) are highly specialized glycoproteins that recognize a multitude of antigens from bacteria, viruses, and other disease-causing organisms and recruit other cells and molecules to destroy these pathogens (Salinas et al., 2011). Decreased abundance of immunoglobulins transcripts at 17°C was observed already after one week and turned into a massive drop at the eighth week. Serum antibodies were not analyzed in this study, however, Magnadóttir and coauthors (Magnadóttir et al., 1999) did not observe decrease of antibodies in cod acclimated to high water temperatures. Apparent downregulation of immunoglobulins can be due to massive evasion of B cells from the lymphatic organs, especially in an infected fish. It is likely that recruitment of lymphocyte left spleen unprotected. The duration of the trial was sufficient for the formation of acquired immunity. Consequently, specific antibodies can participate in protection along with innate heterologous antibodies, which are always abundant in fish, including Atlantic cod.
As in previously published studies, high temperature caused complex changes in expression of immune genes without a trend towards overall stimulation or suppression. Francisella infection induced significant changes in gene expression at 17°C with a predominance of upregulation, while responses were minor at lower temperature. This result would be difficult, if possible, to interpret without knowledge of the pathology. A stronger immune response can be either evidence of active defense or a consequence of a more severe course of disease. Given the greater number and area of splenic granulomas at high temperature, it is evident that transcriptome changes, which were likely linked to the immune responses, did not protect cod. In challenge trials, there is usually stronger stimulation of immune genes in fish with higher pathogen levels. More serious damage could develop as a consequence of active proliferation of bacteria at a higher temperature. Increased mortality despite stronger immune response was observed in in Atlantic cod infected with B. pinnipedialis (Larsen et al., 2018). The authors attributed this finding to the trade-off between rapid growth at high temperature which in theory could divert resources from immune defense. Some of the striking transcriptomic changes in the skin and spleen, despite of lesser magnitude as expected for an infection challenge, mirrored previous observations in F. noatunensis infected cod where the overall classic innate immune response was demonstrated such as upregulation of genes related to inflammation, acute-phase proteins and cell recruitment (Solbakken et al., 2019). Inflammation was activated through an array of pro-inflammatory chemokines and cytokines.
4.4 The skins barrier function is reduced at high temperature
Unlike the salmon industry, which uses H2O2 to treat against salmon lice, this agent is not common in cod farming. However, H2O2 was applied in this trial as a chemical inducer of oxidative stress in the same way as in similar in vitro exposure studies (Karlsen et al., 2021; Ytteborg et al., 2023). In vitro migration potential of keratocytes offers insight into the skins wound healing capacity, as migration of keratocytes is the first step in the healing process of skin damages (Sveen et al., 2020). From scale explants, a reduced migration capacity was observed at 17°C, with lowest migration found in scales exposed to H2O2 from this temperature regime. It is interesting that changes were induced already after 1 week at high temperature, and that the changes seemed to worsen throughout the fish trial. Skin samples from the 17°C temperature regime further had thinner epidermis and fewer sensory cells compared to samples from 12°C. SEM analyses showed superficial alterations like detaching keratocytes and breaches in the continuous keratocytes layer at increased temperature, a morphology that expanded after exposure to H2O2. With increasing temperature and H2O2 exposure, epidermis got more fragmented, keratocytes lost their defined structures of microridges, and detached from the continuous outermost epidermal layer, leaving breaches and exposed areas. Similar changes have already been shown after exposure to oxidative stress at low temperatures (Ytteborg et al., 2020), but it is of interest that elevated temperature worsened the effect of oxidative stress. The microridges have several functions in fish skin, i.e. enlarge the cell surface for gas exchange, serve as support to hold mucous coating, provide mechanical strength and support sensory cells (Henrikson and Matolsky, 1968; Olson and Fromm, 1973; Fishelson, 1984), thus damaged microridges may negatively affect important skin functions. In addition, elevated temperature and H2O2 exposure further altered the morphology and colour of the melanocytes in skin. Fragmented and spindle shaped melanocytes were observed at both treatments, and more severe when temperature and H2O2 exposure were combined. Similar observations were seen in lumpfish after exposure to stress (Ytteborg et al., 2023) and in salmon after the operational procedures crowding and netting (Thorsen, 2019). Melanocytes in skin may function as antioxidants (Hardeland, 2005; Galano et al., 2013), and reduced function caused by stress may thus reduce the robustness of skin as a protective barrier in fish. Analysing gene transcription from non-exposed and H2O2 exposed biopsies from both temperatures showed different transcription of proinflammatory cytokines (tnfα, il1β and il8), antibacterial agent lysozyme, gene markers of stress and responses to protein damage (hsp70, hsp90 and sip1) and oxidative stress (sod, catalase and gsh). The stress markers were markedly increased at week one in the high temperature biopsies, but only significant for the infected groups after eight weeks. The use of several markers for chaperone genes, oxidative stress, and protein folding have been suggested and already used to detect thermal stress in fish (Akbarzadeh et al., 2018). Increased expression of il1β and il8 were also found in skin biopsies from the high temperature regime at both timepoints. Elevated transcription of both il1β and il8 has previously been reported in blood plasma from cod (Pérez-Casanova et al., 2008a) and in salmon liver (Beemelmanns et al., 2021) after exposure to high temperature. Combined, the approach of using in vitro systems with skin and scale explants, provided valuable insight into how different temperatures affect skin. Overall, high temperatures seem to reduce the skins potential to heal quickly and alter its response to secondary stressors, two important skin functions vital for the fish health and welfare.
5 Conclusion
Overall, our results imply biological alterations caused by high temperature that may reduce the cod’s robustness to diseases and production stress. Understanding how multiple stressors affect the biological performance of farmed species is essential when planning sustainable production. Fish in production systems are exposed to changing environmental conditions, pathogens, and operational procedures, that may expose the animals to stress either individually or in combination. High temperature was shown to reduce skin integrity and barrier functions, leaving it more vulnerable to wounds and secondary infections. Although transcriptome analysis did not reveal global immune suppression, challenge with Francicella caused severe damage in spleen at 17°C, which could be due to impaired B cell responses. A weakened skin barrier combined with reduced resistance to pathogens at high temperatures poses a threat to the fish health and welfare. Taken together, our results provide valuable information on how secondary stressors combined with increased temperature may affect farmed cod, and the industry should pay high attention to environmental changes. As shown here, climate change projections show markedly increased temperature at an existing farm site close to the Arctic Circle, and future sites for cod production should be selected taking into account temperature projections and assessment of local environmental conditions.
Data availability statement
The datasets presented in this study can be found in online repositories. The names of the repository/repositories and accession number(s) can be found in the article/supplementary material.
Ethics statement
The animal study was approved by Norwegian Food Safety Authority. The study was conducted in accordance with the local legislation and institutional requirements.
Author contributions
EY, LF, L-HJ and CL conceptualized and designed the study. EY, LF, CL, GJ, SH and ØH collected and sampled the data. EY, LF, L-HJ, CL, AK, SA, VH, GJ and GT analysed the data. EY, CL, AK and GT performed the statistical analysis. EY, LF, L-HJ, CL and AK drafted the manuscript. All authors contributed to the article and approved the submitted version.
Funding
This work was supported by Norwegian Troms and Finnmark County (TFFK2021-179, SecureCod), the Norwegian Research Council (No. 194050, Insight), the UK Research and Innovation Future Leaders Fellowship (MR/V021613/1), the Bjerknes Centre for Climate Research through the project SKD-LOES and by the Bjerknes Climate Prediction Unit (BCPU) in Bergen through the Trond Mohn Foundation (Project number: BFS2018TMT01).
Acknowledgments
The authors are grateful to Miroslava Hansen at the Veterinary Institute (Harstad, Norway) for preparation of histological sections, and to the personnel at the Center of Marine Aquaculture and The Aquaculture Research Station (Tromsø, Norway) for excellent handling of the fish. The climate simulations for this article were made on the supercomputer Fram, which is part of the Norwegian Infrastructure for Research and Education (Sigma2). We thank Dr. Robinson Hordoir, Institute of Marine Research for performing the downscaled climate simulations.
Conflict of interest
The authors declare that the research was conducted in the absence of any commercial or financial relationships that could be construed as a potential conflict of interest.
Publisher’s note
All claims expressed in this article are solely those of the authors and do not necessarily represent those of their affiliated organizations, or those of the publisher, the editors and the reviewers. Any product that may be evaluated in this article, or claim that may be made by its manufacturer, is not guaranteed or endorsed by the publisher.
References
Akbarzadeh A., Günther O. P., Houde A. L., Li S., Ming T. J., Jeffries K. M., et al. (2018). Developing specific molecular biomarkers for thermal stress in salmonids. BMC Genomics 19, 749. doi: 10.1186/s12864-018-5108-9
Bankhead P., Loughrey M. B., Fernández J. A., Dombrowski Y., Mcart D. G., Dunne P. D., et al. (2017). QuPath: Open source software for digital pathology image analysis. Sci. Rep. 7, 16878. doi: 10.1038/s41598-017-17204-5
Beemelmanns A., Zanuzzo F. S., Sandrelli R. M., Rise M. L., Gamperl A. K. (2021). The Atlantic salmon’s stress- and immune-related transcriptional responses to moderate hypoxia, an incremental temperature increase, and these challenges combined. G3 (Bethesda) 11 (7), jkab102. doi: 10.1093/g3journal/jkab102
Bentsen M., Bethke I., Debernard J. B., Iversen T., Kirkevåg A., Seland Ø., et al. (2013). The Norwegian Earth System Model, NorESM1-M – Part 1: Description and basic evaluation of the physical climate. Geosci. Model. Dev. 6, 687–720. doi: 10.5194/gmd-6-687-2013
Bjørgen H., Koppang E. O. (2021). Anatomy of teleost fish immune structures and organs. Immunogenetics 73, 53–63. doi: 10.1007/s00251-020-01196-0
Burge C. A., Eakin C. M., Friedman C. S., Froelich B., Hershberger P. K., Hofmann E. E., et al. (2014). Climate change influences on marine infectious diseases: implications for management and society. Annu. Rev. Mar. Sci. 6, 249–277. doi: 10.1146/annurev-marine-010213-135029
Cabillon N. A. R., Lazado C. C. (2019). Mucosal barrier functions of fish under changing environmental conditions. Fishes 4, 2. doi: 10.3390/fishes4010002
Chen B., Yang B., Zhu J., Wu J., Sha J., Sun J., et al. (2020). Hsp90 relieves heat stress-induced damage in mouse kidneys: involvement of antiapoptotic PKM2-AKT and autophagic HIF-1α Signaling. Int. J. Mol. Sci. 21, 1646. doi: 10.3390/ijms21051646
Drinkwater K. F. (2005). The response of Atlantic cod (Gadus morhua) to future climate change. ICES J. Mar. Sci. 62, 1327–1337. doi: 10.1016/j.icesjms.2005.05.015
Elumalai P., Rubeena A. S., Arockiaraj J., Wongpanya R., Cammarata M., Ringø E., et al. (2019). The role of lectins in finfish: A review. Rev. Fish. Sci. Aquacult. 27, 152–169. doi: 10.1080/23308249.2018.1520191
Falconer L., Hjøllo S. S., Telfer T. C., Mcadam B. J., Hermansen Ø., Ytteborg E. (2020). The importance of calibrating climate change projections to local conditions at aquaculture sites. Aquaculture 514, 734487. doi: 10.1016/j.aquaculture.2019.734487
Falconer L., Telfer T. C., Garrett A., Hermansen Ø., Mikkelsen E., Hjøllo S. S., et al. (2022). Insight into real-world complexities is required to enable effective response from the aquaculture sector to climate change. PloS Climate 1, e0000017. doi: 10.1371/journal.pclm.0000017
FAO (2022). The State of World Fisheries and Aquaculture: Towards Blue Transformation (Rome: FAO). 266pp.
Fishelson L. (1984). A comparative study of ridge-mazes on surface epithelial cell-membranes of fish scales (Pisces, Teleostei). Zoomorphology 104, 231–238. doi: 10.1007/BF00312036
Fricko O., Havlik P., Rogelj J., Klimont Z., Gusti M., Johnson N., et al. (2017). The marker quantification of the Shared Socioeconomic Pathway 2: A middle-of-the-road scenario for the 21st century. Global Environ. Change 42, 251–267. doi: 10.1016/j.gloenvcha.2016.06.004
Galano A., Tan D. X., Reiter R. J. (2013). On the free radical scavenging activities of melatonin’s metabolites, AFMK and AMK. J. Pineal Res. 54, 245–257. doi: 10.1111/jpi.12010
Gollock M. J., Currie S., Petersen L. H., Gamperl A. K. (2006). Cardiovascular and haematological responses of Atlantic cod (Gadus morhua) to acute temperature increase. J. Exp. Biol. 209, 2961–2970. doi: 10.1242/jeb.02319
Hardeland R. (2005). Atioxidative protection by melatonin. Endocrine 27, 119–130. doi: 10.1385/ENDO:27:2:119
Henriksen E., Morten H., Hansen ØyvindJ., Mortensen A. (2018). Kunnskaps- og erfaringsgrunnlag for torskeoppdrett. Research report/Knowledge and experience basis for cod farming (Nofima).
Henrikson R. C., Matolsky A. G. (1968). The fine structure of the teleost epidermis. I. Introduction and filament containing cells. J. Ultrastruct Res. 21, 194–212. doi: 10.1016/S0022-5320(67)80091-1
Holt R. E., Jørgensen C. (2015). Climate change in fish: effects of respiratory constraints on optimal life history and behaviour. Biol. Lett. 11, 20141032. doi: 10.1098/rsbl.2014.1032
Hop H., Gjøsæter H. (2013). Polar cod (Boreogadus saida) and capelin (Mallotus villosus) as key species in marine food webs of the Arctic and the Barents Sea. Mar. Biol. Res. 9, 878–894. doi: 10.1080/17451000.2013.775458
Hordoir R., Skagseth Ø., Ingvaldsen R. B., Sandø A. B., Löptien U., Dietze H., et al. (2022). Changes in arctic stratification and mixed layer depth cycle: A modeling analysis. J. Geophys. Res.: Oceans 127, e2021JC017270. doi: 10.1029/2021JC017270
Hori T. S., Gamperl A. K., Afonso L. O., Johnson S. C., Hubert S., Kimball J., et al. (2010). Heat-shock responsive genes identified and validated in Atlantic cod (Gadus morhua) liver, head kidney and skeletal muscle using genomic techniques. BMC Genomics 11, 72. doi: 10.1186/1471-2164-11-72
Hori T. S., Gamperl A. K., Nash G., Booman M., Barat A., Rise M. L. (2013). The impact of a moderate chronic temperature increase on spleen immune-relevant gene transcription depends on whether Atlantic cod (Gadus morhua) are stimulated with bacterial versus viral antigens. Genome 56, 567–576. doi: 10.1139/gen-2013-0090
Hoter A., El-Sabban M. E., Naim H. Y. (2018). The HSP90 family: structure, regulation, function, and implications in health and disease. Int. J. Mol. Sci. 19, 2560. doi: 10.3390/ijms19092560
IPCC (2014). Climate Change 2014: Synthesis Report. Contribution of Working Groups I, II and III to the Fifth Assessment Report of the Intergovernmental Panel on Climate Change [Core Wring Team, Pachauri R. K., Meyer L. A.r (eds.)]. (Geneva, Switzerland: IPCC) 151.
IPCC (2019). IPCC Special Report on the Ocean and Cryosphere in a Changing Climate [Pörtner H. -O., Roberts D. C., Masson-Delmote V., Zhai P., Tignor E., Poloczanska K., et al. (eds.)]. (Cambridge, UK and New York, NY, USA: Cambridge University Press) 755. doi: 10.1017/9781009157964
IPCC, Core Writing Team (2023). “Climate Change 2023: Synthesis Report. A Report of the Intergovernmental Panel on Climate Change,” in Contribution of Working Groups I, II and III to the Sixth Assessment Report of the Intergovernmental Panel on Climate Change. Eds. Lee H., Romero J. (Geneva, Switzerland: IPCC).
Ishida Y., Nagata K. (2011). Hsp47 as a collagen-specific molecular chaperone. Methods Enzymol. 499, 167–182. doi: 10.1016/B978-0-12-386471-0.00009-2
Johansson P., Wang T., Collet B., Corripio-Miyar Y., Monte M. M., Secombes C. J., et al. (2016). Identification and expression modulation of a C-type lectin domain family 4 homologue that is highly expressed in monocytes/macrophages in rainbow trout (Oncorhynchus mykiss). Dev. Comp. Immunol. 54, 55–65. doi: 10.1016/j.dci.2015.08.005
Karlsen C., Bogevik A. S., Krasnov A., Ytteborg E. (2021). In vivo and in vitro assessment of Atlantic salmon skin exposed to hydrogen peroxide. Aquaculture 540, 736660. doi: 10.1016/j.aquaculture.2021.736660
Krasnov A., Johansen L. H., Karlsen C., Sveen L., Ytteborg E., Timmerhaus G., et al. (2021). Transcriptome responses of atlantic salmon (Salmo salar L.) to viral and bacterial pathogens, inflammation, and stress. Front. Immunol. 12, 705601. doi: 10.3389/fimmu.2021.705601
Krasnov A., Timmerhaus G., Afanasyev S., Jorgensen S. M. (2011). Development and assessment of oligonucleotide microarrays for Atlantic salmon (Salmo salar L.). Comp. Biochem. Physiol. Part D Genomics Proteomics 6 (1), 31–38. doi: 10.1016/j.cbd.2010.04.006
Kriegler E., O’neill B. C., Hallegatte S., Kram T., Lempert R. J., Moss R. H., et al. (2012). The need for and use of socio-economic scenarios for climate change analysis: A new approach based on shared socio-economic pathways. Global Environ. Change 22, 807–822. doi: 10.1016/j.gloenvcha.2012.05.005
Lannig G., Bock C., Sartoris F. J., Pörtner H. O. (2004). Oxygen limitation of thermal tolerance in cod, Gadus morhua L., studied by magnetic resonance imaging and on-line venous oxygen monitoring. Am. J. Physiology-Regul. Integr. Comp. Physiol. 287 (4), R902–R910. doi: 10.1152/ajpregu.00700.2003
Larsen A. K., Nymo I. H., Sørensen K. K., Seppola M., Rødven R., Jiménez De Bagüés M. P., et al. (2018). Concomitant temperature stress and immune activation may increase mortality despite efficient clearance of an intracellular bacterial infection in Atlantic cod. Front. Microbiol. 9, 2963. doi: 10.3389/fmicb.2018.02963
Lele Z., Engel S., Krone P. H. (1997). hsp47 and hsp70 gene expression is differentially regulated in a stress- and tissue-specific manner in zebrafish embryos. Dev. Genet. 21, 123–133. doi: 10.1002/(SICI)1520-6408(1997)21:2<123::AID-DVG2>3.0.CO;2-9
Madec G., The NEMO System Team. (2015). Nemo ocean engine, version 3.6 Stable (Tech. Rep.). IPSL. Available at: htp://www.nemo-ocean.eu/.
Magnadóttir B., Jónsdóttir H., Helgason S., Björnsson B., Jørgensen T. O., Pilström L. (1999). Humoral immune parameters in Atlantic cod (Gadus morhua L.) I. The effects of environmental temperature. Comp. Biochem. Physiol. B Biochem. Mol. Biol. 122, 173–180. doi: 10.1016/S0305-0491(98)10156-6
Mccormick S. D. (2011). “Hormonal control of metabolism and ionic regulation,” in The Hormonal Control of Osmoregulation in Teleost Fish. Encyclopedia of Fish Physiology: From Genome to environment, (San Diego: Academic Press) 2, 1466–1473.
Metian M., Troell M., Christensen V., Steenbeek J., Pouil S. (2020). Mapping diversity of species in global aquaculture. Rev. Aquacult. 12, 1090–1100. doi: 10.1111/raq.12374
Mikalsen J. (2008). Diagnosis and Charcterisation of Intra-cellular Gram-negative Pathogens of Marine and Salmonid Fish (Unipub: Norwegian School of Veterinary Science).
Misund O. A., Heggland K., Skogseth R., Falck E., Gjøsæter H., Sundet J., et al. (2016). Norwegian fisheries in the Svalbard zone since 1980. Regulations, profitability and warming waters affect landings. Polar Sci. 10, 312–322. doi: 10.1016/j.polar.2016.02.001
Nagata K. (1996). Hsp47: a collagen-specific molecular chaperone. Trends Biochem. Sci. 21, 22–26. doi: 10.1016/S0968-0004(06)80023-X
Nardi G., Prickett R., Van Der Meeren T., Boyce D., Moir J. (2021). Atlantic cod aquaculture: Boom, bust, and rebirth? J. World Aquacult. Soc. 52, 672–690. doi: 10.1111/jwas.12811
Norin T., Canada P., Bailey J. A., Gamperl A. K. (2019). Thermal biology and swimming performance of Atlantic cod (Gadus morhua) and haddock (Melanogrammus aeglefinus). PeerJ 7, e7784–e7784. doi: 10.7717/peerj.7784
Nylund A., Ottem K. F., Watanabe K., Karlsbakk E., Krossøy B. (2006). Francisella sp. (Family Francisellaceae) causing mortality in Norwegian cod (Gadus morhua) farming. Arch. Microbiol. 185, 383–392. doi: 10.1007/s00203-006-0109-5
Oliver E. C. J., Donat M. G., Burrows M. T., Moore P. J., Smale D. A., Alexander L. V., et al. (2018). Longer and more frequent marine heatwaves over the past century. Nat. Commun. 9, 1324. doi: 10.1038/s41467-018-03732-9
Olsen A. B., Mikalsen J., Rode M., Alfjorden A., Hoel E., Straum-Lie K., et al. (2006). A novel systemic granulomatous inflammatory disease in farmed Atlantic cod, Gadus morhua L., associated with a bacterium belonging to the genus Francisella. J. Fish Dis. 29, 307–311. doi: 10.1111/j.1365-2761.2006.00714.x
Olson K. R., Fromm P. O. (1973). A scanning electromicroscopic study of secondary lamellae and chloride cells of rainbow trout (Salrno gairdneri). Z Zellforsch Mikrosk Anat 143, 439449. doi: 10.1007/BF00306764
O’Neill B. C., Kriegler E., Riahi K., Ebi K. L., Hallegatte S., Carter T. R., et al. (2014). A new scenario framework for climate change research: the concept of shared socioeconomic pathways. Climatic Change 122, 387–400. doi: 10.1007/s10584-013-0905-2
Ottem K. F., Nylund A., Isaksen T. E., Karlsbakk E., Bergh Ø. (2008). Occurrence of Francisella piscicida in farmed and wild Atlantic cod, Gadus morhua L., in Norway. J. Fish Dis. 31, 525–534. doi: 10.1111/j.1365-2761.2008.00930.x
Ottem K. F., Nylund A., Karlsbakk E., Friis-Møller A., Kamaishi T. (2009). Elevation of Francisella philomiragia subsp. noatunensisMikalsen et al (2007) to Francisella noatunensis comb. nov. [syn. Francisella piscicida Ottem et al (2008) syn. nov.] and characterization of Francisella noatunensis subsp. orientalis subsp. nov., two important fish pathogens. J. Appl. Microbiol. 106, 1231–1243. doi: 10.1111/j.1365-2672.2008.04092.x
Pérez-Casanova J. C., Afonso L. O. B., Johnson S. C., Currie S., Gamperl A. K. (2008a). The stress and metabolic responses of juvenile Atlantic cod Gadus morhua L. to an acute thermal challenge. J. Fish Biol. 72, 899–916. doi: 10.1111/j.1095-8649.2007.01763.x
Pérez-Casanova J. C., Rise M. L., Dixon B., Afonso L. O., Hall J. R., Johnson S. C., et al. (2008b). The immune and stress responses of Atlantic cod to long-term increases in water temperature. Fish Shellfish Immunol. 24, 600–609. doi: 10.1016/j.fsi.2008.01.012
Pfaffl M. W. (2001). A new mathematical model for relative quantification in real-time RT-PCR. Nucleic Acids Res. 29 (9), e45. doi: 10.1093/nar/29.9.e45
Pfaffl M. W., Tichopad A., Prgomet C., Neuvians T. P. (2004). Determination of stable housekeeping genes, differently regulated target genes and sample integrity: BestKeeper – Excel-based tool using pair-wise correlations. Biotechnol. Lett. 26, 509–515. doi: 10.1023/B:BILE.0000019559.84305.47
Pörtner H. (2001). Climate change and temperature-dependent biogeography: oxygen limitation of thermal tolerance in animals. Naturwissenschaften 88, 137–146. doi: 10.1007/s001140100216
Puvanendran V., Mortensen A., Johansen L.-H., Kettunen A., Hansen Ø.J., Henriksen E., et al. (2022). Development of cod farming in Norway: Past and current biological and market status and future prospects and directions. Rev. Aquacult. 14, 308–342. doi: 10.1111/raq.12599
Rajan B., Lokesh J., Kiron V., Brinchmann M. F. (2013). Differentially expressed proteins in the skin mucus of Atlantic cod (Gadus morhua) upon natural infection with Vibrio Anguillarum. BMC Vet. Res. 9, 103. doi: 10.1186/1746-6148-9-103
Roberts R. J., Agius C., Saliba C., Bossier P., Sung Y. Y. (2010). Heat shock proteins (chaperones) in fish and shellfish and their potential role in relation to fish health: a review. J. Fish Dis. 33, 789–801. doi: 10.1111/j.1365-2761.2010.01183.x
Salinas I., Zhang Y.-A., Sunyer J. O. (2011). Mucosal immunoglobulins and B cells of teleost fish. Dev. Comp. Immunol. 35, 1346–1365. doi: 10.1016/j.dci.2011.11.009
Scharsack J. P., Franke F. (2022). Temperature effects on teleost immunity in the light of climate change. J. Fish Biol. 101, 780–796. doi: 10.1111/jfb.15163
Seland Ø., Bentsen M., Olivié D., Toniazzo T., Gjermundsen A., Graff L. S., et al. (2020). Overview of the Norwegian Earth System Model (NorESM2) and key climate response of CMIP6 DECK, historical, and scenario simulations. Geosci. Model. Dev. 13, 6165–6200. doi: 10.5194/gmd-13-6165-2020
Shan Q., Ma F., Wei J., Li H., Ma H., Sun P. (2020). Physiological functions of heat shock proteins. Curr. Protein Pept. Sci. 21, 751–760. doi: 10.2174/1389203720666191111113726
Solbakken M. H., Jentoft S., Reitan T., Mikkelsen H., Gregers T. F., Bakke O., et al. (2019). Disentangling the immune response and host-pathogen interactions in Francisella noatunensis infected Atlantic cod. Comp. Biochem. Physiol. Part D: Genomics Proteomics 30, 333–346. doi: 10.1016/j.cbd.2019.04.004
Stefanovic D. I., Manzon L. A., Mcdougall C. S., Boreham D. R., Somers C. M., Wilson J. Y., et al. (2016). Thermal stress and the heat shock response in embryonic and young of the year juvenile lake whitefish. Comp. Biochem. Physiol. A Mol. Integr. Physiol. 193, 1–10. doi: 10.1016/j.cbpa.2015.12.001
Stet R. J., Hermsen T., Westphal A. H., Jukes J., Engelsma M., Lidy Verburg-Van Kemenade B. M., et al. (2005). Novel immunoglobulin-like transcripts in teleost fish encode polymorphic receptors with cytoplasmic ITAM or ITIM and a new structural Ig domain similar to the natural cytotoxicity receptor NKp44. Immunogenetics 57, 77–89. doi: 10.1007/s00251-005-0771-9
Sveen L., Karlsen C., Ytteborg E. (2020). Mechanical induced wounds in fish – a review on models and healing mechanisms. Rev. Aquacult. 12, 2446–2465. doi: 10.1111/raq.12443
Thorsen D. K. (2019). Melanin-based skin pigmentation and stress in Atlantic salmon (Salmon salar). Master’s theses (BioVit), Norwegian university of Life Sciences.
Tveit G. M., Anders N., Bondø M. S., Mathiassen J. R., Breen M. (2022). Atlantic mackerel (Scomber scombrus) change skin colour in response to crowding stress. J. Fish Biol. 100, 738–747. doi: 10.1111/jfb.14987
Wang Y., Liu Z., Li Z., Shi H., Kang Y., Wang J., et al. (2016). Effects of heat stress on respiratory burst, oxidative damage and SERPINH1 (HSP47) mRNA expression in rainbow trout Oncorhynchus mykiss. Fish Physiol. Biochem. 42, 701–710. doi: 10.1007/s10695-015-0170-6
Welch H. E., Bergmann M. A., Siferd T. D., Martin K. A., Curtis M. F., Crawford R. E., et al. (1992). Energy flow through the marine ecosystem of the lancaster sound region, Arctic Canada. Arctic 45, 343–357. doi: 10.14430/arctic1413
Ytteborg E., Hansen Ø.J., Høst V., Afanasyev S., Vieweg I., Nahrgang J., et al. (2020). Morphology, transcriptomics and in vitro model of skin from polar cod (Boreogadus saida) and Atlantic cod (Gadus morhua). Fishes 5, 34. doi: 10.3390/fishes5040034
Ytteborg E., Lazado C. C., Noble C., Hansen R. I., Johansen L.-H. (2023). The skin mucosal barrier of lumpfish (Cyclopterus lumpus L.) is weakened by exposure to potential aquaculture production related stressors. J. Fish Biol. 19. doi: 10.1111/jfb.15352
Zanuzzo F. S., Bailey J. A., Garber A. F., Gamperl A. K. (2019). The acute and incremental thermal tolerance of Atlantic cod (Gadus morhua) families under normoxia and mild hypoxia. Comp. Biochem. Physiol. Part A: Mol. Integr. Physiol. 233, 30–38. doi: 10.1016/j.cbpa.2019.03.020
Zhong P., Huang H. (2017). Recent progress in the research of cold-inducible RNA-binding protein. Future Sci. OA 3, Fso246. doi: 10.4155/fsoa-2017-0077
Keywords: Atlantic cod, aquaculture, climate change, fransicella, IPCC, temperature
Citation: Ytteborg E, Falconer L, Krasnov A, Johansen L-H, Timmerhaus G, Johansson GS, Afanasyev S, Høst V, Hjøllo SS, Hansen ØJ and Lazado CC (2023) Climate change with increasing seawater temperature will challenge the health of farmed Atlantic Cod (Gadus morhua L.). Front. Mar. Sci. 10:1232580. doi: 10.3389/fmars.2023.1232580
Received: 31 May 2023; Accepted: 04 September 2023;
Published: 20 September 2023.
Edited by:
Tomaso Fortibuoni, Istituto Superiore per la Protezione e la Ricerca Ambientale (ISPRA), ItalyReviewed by:
Pedro Miguel Guerreiro, University of Algarve, PortugalM. Virginia Martín, Spanish Institute of Oceanography (IEO), Spain
Copyright © 2023 Ytteborg, Falconer, Krasnov, Johansen, Timmerhaus, Johansson, Afanasyev, Høst, Hjøllo, Hansen and Lazado. This is an open-access article distributed under the terms of the Creative Commons Attribution License (CC BY). The use, distribution or reproduction in other forums is permitted, provided the original author(s) and the copyright owner(s) are credited and that the original publication in this journal is cited, in accordance with accepted academic practice. No use, distribution or reproduction is permitted which does not comply with these terms.
*Correspondence: Elisabeth Ytteborg, ZWxpc2FiZXRoLnl0dGVib3JnQG5vZmltYS5ubw==