- Leigh Marine Laboratory, Institute of Marine Science, University of Auckland, Leigh, New Zealand
Marine protected areas have long been proposed as a key tool to restore lost food web interactions and increase the resilience of ecosystems to climate change impacts. However, a changing climate can result in the arrival of new species or differentially affect native species, which can alter ecosystem dynamics and make it difficult to predict how ecosystems will respond to protection. The long-spined sea urchin Centrostephanus rodgersii is a well-known range extender with large impacts on kelp forest ecosystems, yet its response to warming and long-term marine protection has not been examined within its native range. We examine long-term trends in C. rodgersii and the endemic sea urchin Evechinus chloroticus following no-take protection within the Poor Knights Islands Marine Reserve, in northeastern Aotearoa New Zealand, from 1999-2022, and compare with population trends at an unprotected island group. Within the marine reserve, E. chloroticus decreased in density, became more cryptic, and urchin barrens associated with this species largely disappeared, whereas in fished areas, populations and extent of barrens remained stable. This differing response between the reserve and fished location is consistent with a top-down effect and greater abundance of predatory fish in the reserve. In contrast, the subtropical sea urchin C. rodgersii increased in abundance by 9.3 times in the Poor Knights Island Marine Reserve and 4.3 times at the fished location, with areas of urchin barrens associated with this species developing at both locations. This increase coincides with substantial warming over the monitoring period (0.25°C decade-1) and low numbers of key predators (rock lobster) at both reserve and fished locations. This highlights the emerging threat of C. rodgersii to rocky reefs in the region and how marine protection alone may not increase resilience to this threat. This suggests multifaceted management approaches are needed to mitigate the impacts of emerging pest species and increase the resilience of temperate reef ecosystems in a warming climate.
Introduction
The loss of top predators has cascading effects and has led to long-term shifts in the structure and function of many ecosystems worldwide (Estes et al., 2011; Ripple et al., 2014). Consequently, the protection and restoration of predator populations is considered an important management action to restore trophic interactions, increase ecosystem stability and resistance to climate change impacts (Ripple and Beschta, 2012; Ripple et al., 2014; Sala and Giakoumi, 2018; Peleg, et al., 2023). However, climate change can lead to the arrival of new species through range extensions (Ling et al., 2009a; Poloczanska et al., 2013; Pratchett et al., 2014; Vergés et al., 2014; Pecl et al., 2017) and irruptions (large increase) of native species (Carey et al., 2012; Greenville et al., 2012; Sambaraju et al., 2019) that can alter ecosystem dynamics and lead to novel trophic pathways (Edwards and Richardson, 2004; Miranda et al., 2019). These irruptions are generally facilitated by interactive stressors that reduce overall ecosystem resilience, making them more prone to stress and concurrent outbreaks (Breitburg and Riedel, 2005; Purcell, 2012; Ban et al., 2014; Ling et al., 2015). While the protection of predators is expected to limit irruptions of both native and introduced species (Ling et al., 2009b; Wallach et al., 2015), predator populations and their recovery following protection can also be influenced by climate change (Eisenlord et al., 2016; Harvell et al., 2019) and the extent of historic population depletion (Hutchings and Reynolds, 2004; Neubauer et al., 2013). Thus, in a changing environment, it is becoming increasingly difficult to predict how food webs and ecosystems will respond to protection (Wallach et al., 2015; Rilov et al., 2020; Hammerschlag et al., 2022).
In temperate marine environments, the overharvesting of predators on rocky reefs has long been attributed to the loss of kelp forests in many regions worldwide (Steneck et al., 2002; Filbee-Dexter and Scheibling, 2014; Ling et al., 2015). The loss of predators often triggers a trophic release of herbivorous sea urchins, leading to the deforestation of kelp forests, leaving large areas of reef devoid of kelp (termed “urchin barrens”) with reduced productivity, biodiversity and function (Jackson et al., 2001; Steneck et al., 2002; Filbee-Dexter and Scheibling, 2014). These urchin barrens represent alternative stable states, maintained by strong feedback mechanisms that can persist for decades and be extremely difficult to reverse (Filbee-Dexter and Scheibling, 2014; Ling et al., 2015). Nevertheless, in many cases the protection of predators and a concomitant population increase has resulted in a reversal of this pattern with the recovery of kelp forests (Estes and Palmisano, 1974; Babcock et al., 1999; Shears and Babcock, 2002; Shears and Babcock, 2003; Sangil et al., 2012). This was observed in Alaska where the protection and subsequent recolonization of sea otters (Enhydra lutris) resulted in significant declines of sea urchins and a reforestation of kelp (Estes and Duggins, 1995). Similarly, within no-take marine protected areas (MPAs), the recovery of large predatory fishes and rock lobster has resulted in the reverse shift from urchin barrens to kelp forest following decades of protection (Babcock et al., 1999; Behrens and Lafferty, 2004; Edgar et al., 2009; Eger and Baum, 2020; Peleg et al., 2023). Consequently, the protection of predators in no-take MPAs is widely considered one of the most effective management tools for restoring trophic interactions and reversing the impacts of fishing on kelp forest ecosystems (Sala and Giakoumi, 2018).
The protection of predators within MPAs is expected to increase the resilience of kelp forests and mitigate the impacts of climate change (Ling and Johnson, 2012; Gallardo et al., 2017). However, climate change poses a number of novel threats making it difficult to predict how these ecosystems will respond to marine protection. Warming oceans can have direct impacts on kelps via heat stress (Wernberg et al., 2016; Filbee-Dexter et al., 2020) and can also lead to the arrival of new organisms or the expansion of native species that alter ecosystem dynamics and often result in the loss of habitat forming kelps (Ling, 2008; Vergés et al., 2014). For example, the subtropical sea urchin Centrostephanus rodgersii (commonly known as the “long-spined sea urchin”) has extended its range into eastern Tasmania resulting in the extensive loss of kelp forests with flow-on impacts to biodiversity, ecosystem services and local fisheries (Johnson et al., 2005; Ling, 2008; Ling et al., 2009a; Perkins et al., 2015; Ling and Keane, 2018). While this range extension is related to long-term oceanic warming and the poleward expansion of the East Australian Current, it has also been facilitated by the overfishing of the spiny lobster Jasus edwardsii, which is considered the main predator of C. rodgersii in the region (Ling et al., 2009b; Johnson et al., 2011). The protection of predators, primarily J. edwardsii, in Tasmanian marine reserves has been shown to increase resilience to the initial formation of C. rodgersii barrens, but in new reserves where extensive barrens already occur, there was no evidence for the recovery of kelp forests (Perkins et al., 2020; Ling and Keane, 2021). This highlights how the abundance and impacts of range extenders can be exacerbated by the removal of predators through overfishing.
C. rodgersii also occurs along the northeastern coast of Aotearoa New Zealand where it is widely expected to increase in abundance and range in response to oceanic warming (Pecorino et al., 2013a; Thomas et al., 2021). Given the extent of habitat degradation seen in Tasmania (Johnson et al., 2005; Ling, 2008; Ling and Keane, 2018), there is concern over how a population increase would impact kelp forest communities in New Zealand. However, there is currently no information on the long-term population trends of C. rodgersii in this region and how it may respond to warming, overfishing of predators, or marine protection. While C. rodgersii has previously been described as having extended its range to northeastern New Zealand (Pecorino et al., 2012; Byrne and Andrew, 2020), it was first recorded in New Zealand in 1897 and is considered to be a native species (Fell, 1949). It is most common in areas that are strongly influenced by the warm East Auckland current, specifically the northern-most part of the country and at offshore islands along the northeast coast (Ayling, 1974; Riddell, 1980; Choat and Schiel, 1982; Cole et al., 1992; Pecorino et al., 2013a; Byrne and Andrew, 2020; Thomas et al., 2021).
Unlike in eastern Australia, C. rodgersii has historically only occurred in low numbers and is not known to form urchin barrens in New Zealand (Choat and Schiel, 1982; Schiel, 1984; Jones and Andrew, 1990; Shears and Babcock, 2004; Byrne and Andrew, 2020). Instead, the endemic sea urchin Evechinus chloroticus or “kina” is the dominant sea urchin species and forms extensive areas of urchin barrens on many rocky reefs in the region (Choat and Schiel, 1982; Andrew, 1988; Shears and Babcock, 2004). An increase in E. chloroticus abundance and prevalence of associated urchin barrens in northeastern New Zealand has been linked to overfishing of its major predators, snapper (Chrysophrys auratus) and spiny lobster (J. edwardsii), however within long-established marine reserves (30 years +), this pattern has been reversed with the recovery of predators and subsequent trophic interactions (Babcock et al., 1999; Shears and Babcock, 2002; Shears and Babcock, 2003). It is currently unknown whether these predators can play a similar role in controlling C. rodgersii populations within MPAs, and how these interactions may be influenced by climate change. While the rate of long-term ocean warming in northeastern New Zealand is lower than that in Tasmania (Shears and Bowen, 2017; Sutton and Bowen, 2019), the region has experienced large marine heatwaves in recent years (Salinger et al., 2019; Bell et al., 2022), and a more recent analysis of satellite-derived SST since 1982 suggests higher rates of warming in northeastern New Zealand than previously reported (Sutton and Bowen, 2019). Consequently, recent warming trends in the region may have promoted similar increases in C. rodgersii with wider consequences for rocky reef communities.
In this study, we examine long-term trends in both the endemic sea urchin E. chloroticus and the subtropical sea urchin C. rodgersii, and the extent of urchin barrens associated with both species, at a protected and unprotected offshore archipelago in northeastern, New Zealand: The Poor Knights Island Marine Reserve (PKI) and the Mokohinau Islands. The PKI have been fully protected from all forms of fishing since 1998, whereas the Mokohinau Is are heavily fished by recreational and commercial fishers. Predatory fish numbers, primarily C. auratus, have increased dramatically following protection at PKI (Denny et al., 2004; Schiel et al., 2018), but unlike mainland marine reserves there has been no evidence for the recovery of lobster (J. edwardsii and Sagmariasus verreauxi; Taylor et al., 2011). Shallow reefs at both locations were surveyed in 1999, 8 months after the PKI reserve was established, and found that while both sea urchin species were common, E. chloroticus was considerably more abundant and dominated the urchin barren habitat (Shears and Babcock, 2004; Shears et al., 2008). Ongoing monitoring of these sites from 1999-2022 provides a unique opportunity to examine how these two sea urchin species have responded to marine protection over a period of ocean warming. Based on long-term changes observed in coastal reserves, we expected the recovery of predators at PKI to result in a long-term decline in density of E. chloroticus and associated urchin barren habitat. In contrast, there is little understanding on how C. rodgersii may be impacted by marine protection or conversely how this species has responded to recent warming trends (Sutton and Bowen, 2019). Based on trends seen in Tasmania, we predicted a long-term increase in C. rodgersii in relation to warming, but this would be less evident in the reserve if predators were effective at controlling C. rodgersii densities and increasing resilience to barren formation. To better understand the potential drivers of changes over this period, we also provide an updated analysis of trends in satellite-derived SST data and quantified lobster populations at both locations in 2022 as there was limited available data on the abundance of lobster and their response to protection. Therefore, by comparing long-term trends in the two sea urchin species between a marine reserve and a fished location, we aimed to (1) gain a better understanding of long-term trends in C. rodgersii populations in northern New Zealand, and (2) assess the role of marine reserves as a management option for mitigating their impacts.
Methods
Study locations
Tawhiti Rahi and Aorangi Island's, commonly known as the Poor Knights Islands, are located 22 km off the northeastern coast of Aotearoa New Zealand (Figure 1A; Supplementary Figure 1). The islands were fully protected from fishing in a no-take MPA in October 1998, which encompasses the whole island chain to 800 m offshore (1890 ha; Sim-Smith and Kelly, 2009). The islands are an extremely popular dive location with ~15,000 divers visiting annually and are known for the pristine habitat, high fish abundance and clear water (Sim-Smith and Kelly, 2009). The Mokohinau Islands are located 55 km to the southeast and provide a fished control for PKI due to their similar hydrological and topographic features, and biological communities (Denny et al., 2004; Shears et al., 2008; Edgar et al., 2017). Both locations have high wave-exposure, are remote from land-based impacts and are influenced by the East Auckland Current that flows south east along the eastern side of the North Island, leading to temperatures roughly 1°C warmer than the mainland coast (Shears and Babcock, 2004). This has a strong influence on the species assemblages and there is a rich diversity of both subtropical and temperate species (Choat and Ayling, 1987; Choat et al., 1988; Schiel et al., 2018). The dominant reef fish species are the same at both island groups and reef fish communities are very similar, but the PKI generally has a higher diversity and abundance of subtropical species (Brook, 2002; Allard, 2020).
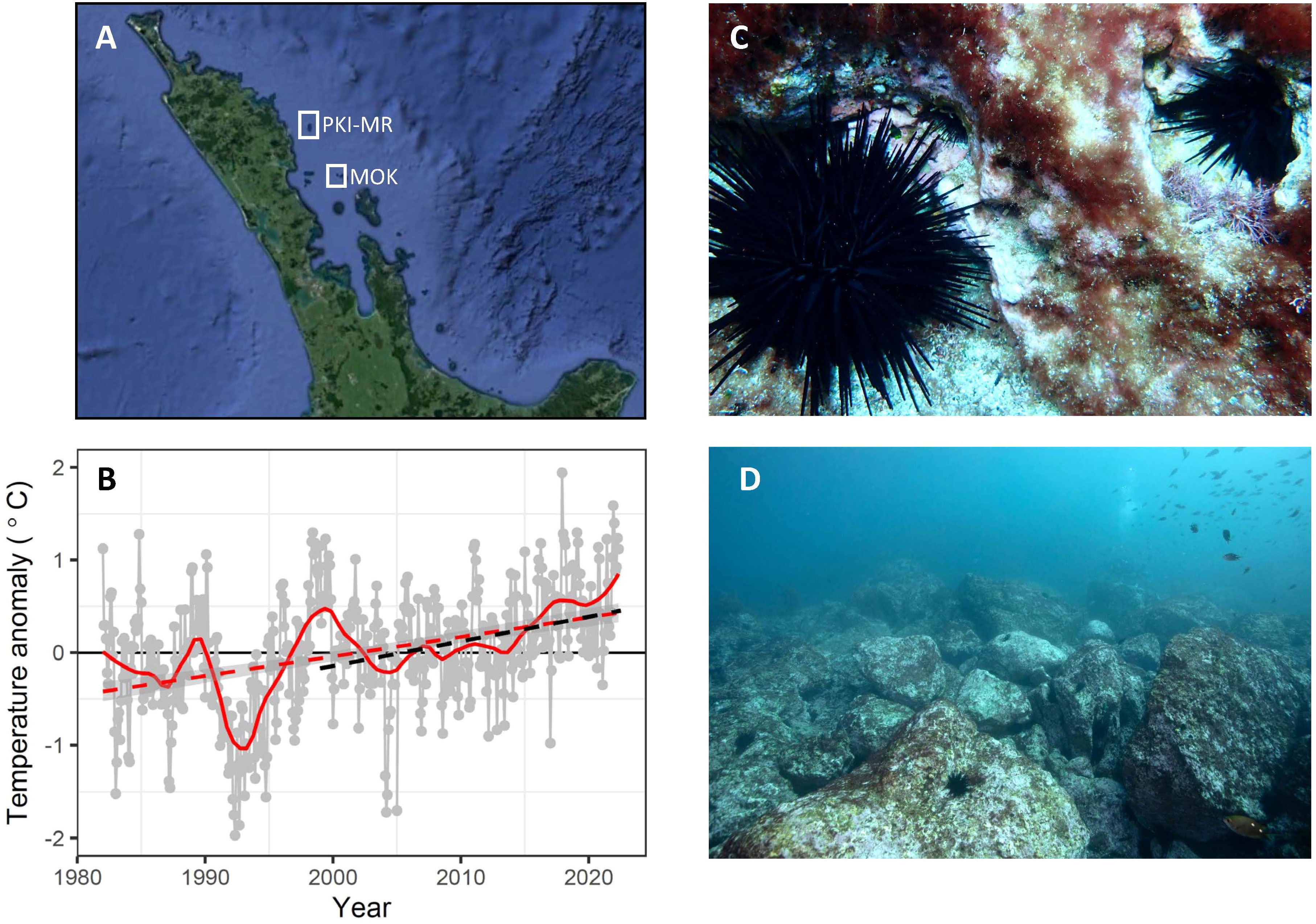
Figure 1 Location of the Poor Knights Islands Marine Reserve and the Mokohinau Islands (MOK - fished) in northeastern Aotearoa New Zealand (A). Warming trends at the Poor Knights Islands based on satellite derived sea surface temperature (SST) (B). The subtropical sea urchin Centrostephanus rodgersii at the Poor Knights Islands note there are three C. rodgersii evident in (C), with approximate sizes of 20, 50 and 100 mm test diameter. (C) and areas of C rodgersii barrens at the Poor Knights Islands that have developed over the course of this study (D) (1999-2022). Long-term SST anomaly from 1982-2022 were based on daily OISST v.2.1 extracted for the closest grid point to the Poor Knights Islands (174.625°E, 35.3750°S). Loess smoother (red solid line) and linear regression (red dashed line; Slope: 0.20 ± 0.06°C decade-1, t = 3.71, p<0.001). Dashed black line shows linear regression over monitoring period (1999-2022, Slope: 0.25 ± 0.1°C decade-1, t = 2.47, p < 0.001). Photos: N. Shears (C) and P. Caiger (D).
Shallow subtidal reef communities at both island groups are typical of temperate reef ecosystems being dominated by large brown macroalgae (Laminariales and Fucales) with sea urchins as the dominant grazers (Choat and Schiel, 1982; Shears and Babcock, 2004). Large macroalgae typically have a bimodal depth distribution with mixed assemblages of fucoids (predominantly Carpophyllum spp.) and kelps (Ecklonia radiata and Lessonia variegata) dominating the shallow water (<5 m depth) and dense forests of E. radiata at depths below 10 m (Choat and Schiel, 1982; Schiel, 1984; Shears et al., 2004). The sea urchin E. chloroticus typically dominates at intermediate depths (5-10 m) and can form urchin barrens, but turfing and foliose algae may also be common in this zone (Shears and Babcock, 2004). The depth limits of these broad zones and species composition also vary around the islands in relation to wave exposure (Shears and Babcock, 2004; Shears et al., 2008). While environmental conditions and reef communities are similar between the two locations from a regional perspective, the density of exposed E. chloroticus and extent of associated urchin barrens were lower at the PKI at the start of this study (1999), indicating some inherent differences prior to protection. C. rodgersii has historically been common at both island chains, but densities were generally low with no reported impacts on kelp forest communities (Riddell, 1980; Choat and Schiel, 1982; Schiel, 1984; Jones and Andrew, 1990; Shears and Babcock, 2004). Three other species of urchins also occur in low numbers on the reefs at the study locations (Diadema palmeri, Diadema savignyi and Heilocidaris tuberculata). Both Diadema species were not recorded in the quadrat surveys, and only eight H. tuberculata have been recorded in quadrats over the course of the study (1029 quadrats in total).
Trends in sea surface temperature
Remote-sensed daily SST measurements from 1st January 1982 to 30th May 2022 were obtained for both locations from NOAAs Optimum Interpolation Sea Surface Temperature v2.1 (NOAA OI SST; Huang et al., 2021). The daily SST anomaly fields are calculated relative to a 1971-2000 climatology. These data are available in ¼° grid format and were extracted for the nearest available points to the PKI (174.625°E, 35.575°S) and the Mokohinau Is (175.125°E, 35.875°S). Monthly SST anomalies were calculated over the time series and trends were analysed using linear regression separately for the entire time series (Jan 1982-May 2022) and across the monitoring period (Jan 1999-May 2022). Generalized Least Squares regression was used to account for auto-correlation by including an autoregressive process (AR1) for the residuals. A model comparison approach (AIC) was used for each analysis to determine whether it was necessary to include the autoregressive process in the linear model. Monthly anomalies from the two grid points were highly correlated (Pearson correlation coefficient = 0.96) and regression slopes were indistinguishable, so only data from the PKI grid point are presented. To examine seasonal variation in long-term trends, the monthly SST anomaly was averaged by season for each year (Austral seasons: Summer: Dec-Feb, Autumn: Mar-May, Winter: Jun-Aug, Spring: Sept-Nov). Long-term trends in SST were analysed separately for each season using the same approach as above.
Monitoring site locations and survey methodology
Shallow reef monitoring sites were initially surveyed at the PKI and Mokohinau Is in June 1999, 8 months after the PKI became a full no-take marine reserve (Figure 1A; Supplementary Figure 1; Shears and Babcock, 2004; Shears et al., 2008). Nine sites were initially surveyed in each location spanning a mix of gradual to steep sloping reefs down to a depth of at least 12 m, providing representative examples of rocky reef habitat around each island group (Shears et al., 2008). GPS coordinates were taken at each site, along with a photograph of the intertidal rock formations, to allow exact relocation of sites during subsequent surveys. The site monitoring at each of the island groups have been sporadically surveyed since 1999, with eight of the sites in each location sampled in 2019 and 2022.
At each site, a line transect was sampled down the reef to estimate the overall extent of urchin barrens and other major habitat types. The transect (a leaded rope marked with 5 m increments) was run perpendicular to the shore on a fixed compass bearing from mean low water (MLW) out to the reef edge or to a maximum of 15 m depth (below MLW), whichever occurred first. The habitat type, depth and rock type were recorded every 5m along each transect, with habitat types and protocol described in Shears and Babcock (2004). The total length of each transect differed among sites due to differing topography and slope of the reef; ranging from 15 to 110 m (mean: 69.4 m) at the PKI and 20 to 100 m (mean: 60.6 m) at the Mokohinau Is. The type of urchin barren was also classified according to which urchin species were present; “E. chloroticus barrens” were characterised as when only exposed E. chloroticus were present, “C. rodgersii barrens” are when C. rodgersii were present and no exposed E. chloroticus occurred, or “Mixed barrens” if both C. rodgersii and exposed E. chloroticus were present. Note that small cryptic E. chloroticus may be present in C. rodgersii barrens, but these would not be openly grazing the reef and C. rodgersii were considered to have the main grazing impact.
The abundance and size structure of macroalgae and mobile macroinvertebrates was quantified at each site in three depth ranges (4-6, 7-9 and 10-12 m) with 1 m2 quadrats. For the purpose of this study only data on sea urchin size and abundance is presented and analysed. All sea urchins were counted in five 1m2 quadrats that were haphazardly sampled in each depth range, within 5 m either side of the transect line. The test diameter (TD) of all urchins was measured and the behaviour of E. chloroticus was recorded as either “cryptic” when hidden or in a crevice or “exposed” when the urchin was out in the open. This behavioural information was not recorded for C. rodgersii in the original surveys as this species typically exhibits cryptic behaviour in New Zealand, even at large sizes. In the 2022 surveys, 95% of C. rodgersii recorded were classified as being cryptic and 5% exposed.
Changes in sea urchin abundance and extent of urchin barrens
The statistical analysis was restricted to 1999, 2019 and 2022, the years when all eight sites were sampled in both locations. Counts of C. rodgersii and exposed and cryptic E. chloroticus were analysed with a general linear mixed model (GLMM) using the lme4 package (Bates et al., 2015) in R (v. 4.1717, R Core Team, 2021). A Poisson distribution was used to analyse count data for each species testing the effects of fixed factors Location (PKI or Mokohinau Is), Year (1999, 2019, 2022) and Depth (DR2: 4-6 m, DR3: 7-9 m, DR4: 10-12 m), with Site as a random effect variable. A model selection approach was used based on the Akaike’s Information Criterion (AIC), using the AICcmodavg package (Mazerolle, 2023). For each test, the model with the lowest AIC value or the model with the fewest explanatory variables with a △AIC <2 (△AIC = AICmodel – AIClowest AIC from set of models) was selected (Mazerolle, 2023). Diagnostic plots of model residuals were visualized and checked using the DHARMa package to ensure that the model assumptions were fulfilled (Hartig, 2022). Post hoc Tukey tests were run on each GLMM to compare which levels of each factor was significant along with an ANOVA to test the overall significance of the model effects. To assess the magnitude of change in C. rodgersii populations between 1999 and 2022, a separate GLMM was run using the count data from the PKI and Mokohinau Is separately with Year (1999 & 2022) as a fixed factor.
The overall coverage of urchin barrens habitat at each site was estimated as a percentage of total length of the line transect at each site. The presence or absence of urchin barrens at each 5 m interval along the transect was analysed using a GLMM with binomial distribution, with the fixed factors Location (PKI or Mokohinau Is), Year (1999, 2019, 2022) and Depth (continuous), and Site as a random effect. The best model was selected using AIC and diagnostic plots were run to ensure all model assumptions were fulfilled (as in above analyses). ANOVA was used to test the overall significance of model effects and post hoc Tukey tests were run on the GLMM to compare which levels of each factor was significant. Estimated marginal means of linear trends for the continuous predictor variable Depth at each location was compared using the emtrends function in the emmeans package (Lenth et al., 2021).
Rock lobster abundance and size
The only known predators of C. rodgersii in New Zealand are the spiny lobster (J. edwardsii) and packhorse rock lobster (S. verreauxi; Ling et al., 2009b; Ling and Johnson, 2012; Day et al., 2021; Smith et al., 2022). To characterise population abundance and size structure of these two species, transect surveys were undertaken at 9 sites at both PKI and Mokohinau Is between February-April 2022. Surveys were carried out at this time of year as this is when both male and female J. edwardsii are concentrated on shallow reefs for mating (MacDiarmid, 1991; Kelly and MacDiarmid, 2003; Hanns et al., 2022).
Methods were consistent to those used for long-term rock lobster monitoring in other northern New Zealand marine reserves (MacDiarmid, 1991; LaScala-Gruenewald et al., 2021). At each site, two to three 50 x 10 m transects were surveyed on contiguous reef in two depth zones (<10 m and 10-25 m). Along each transect, all available habitat was searched, and any lobsters found were counted, identified to species, sexed and their carapace length estimated visually with the aid of a ruler. Due to very low counts of lobster recorded on transects, and a predominance of zero counts, statistical analysis was not possible and unnecessary.
Results
Trends in sea surface temperature
Significant warming was evident at the PKI and Mokohinau Is (Figure 1B), with an increase of 0.20 ± 0.06°C per decade over the satellite record from January 1982 to May 2022 (Supplementary Table 1) and a rate of warming of 0.25 ± 0.10°C decade-1 over the period of this study (1999-2022: Supplementary Table 1). There was a very warm period in 1999 coinciding with the beginning of the monitoring period and SST has been consistently above average since 2015. There was also strong seasonality in the rate of warming over the satellite area, with greatest rates of warming in summer (0.27 ± 0.08°C decade-1) and autumn (0.30 ± 0.07°C decade-1) and no significant warming trend in winter and spring (Supplementary Table 1; Supplementary Figure 2). Similar seasonal patterns were evident over the monitoring period (1999-2022), again with high rates of warming in summer (0.42 ± 0.17°C decade-1) and autumn (0.35 ± 0.14°C decade-1), but no evidence of warming in winter and spring (Supplementary Table 1).
Sea urchin density and size structure
The long-term trend in the density of exposed E. chloroticus varied between the two locations and with depth, as indicated by a significant interaction between Year, Location and Depth (Figure 2; Supplementary Table 2). Exposed E. chloroticus density was generally highest in the 4-6 m depth stratum and lower in the deeper strata (7-9 and 10-12 m; Figure 2; Supplementary Table 3). The density of exposed E. chloroticus gradually declined in the PKI marine reserve from 1999-2022 across all depths, and very few exposed individuals were recorded in the most recent surveys (Figure 2). At the Mokohinau Is, exposed E. chloroticus densities remained relatively stable, except for a sharp decline between 2019 and 2022 at 4-6 m and 7-9 m.
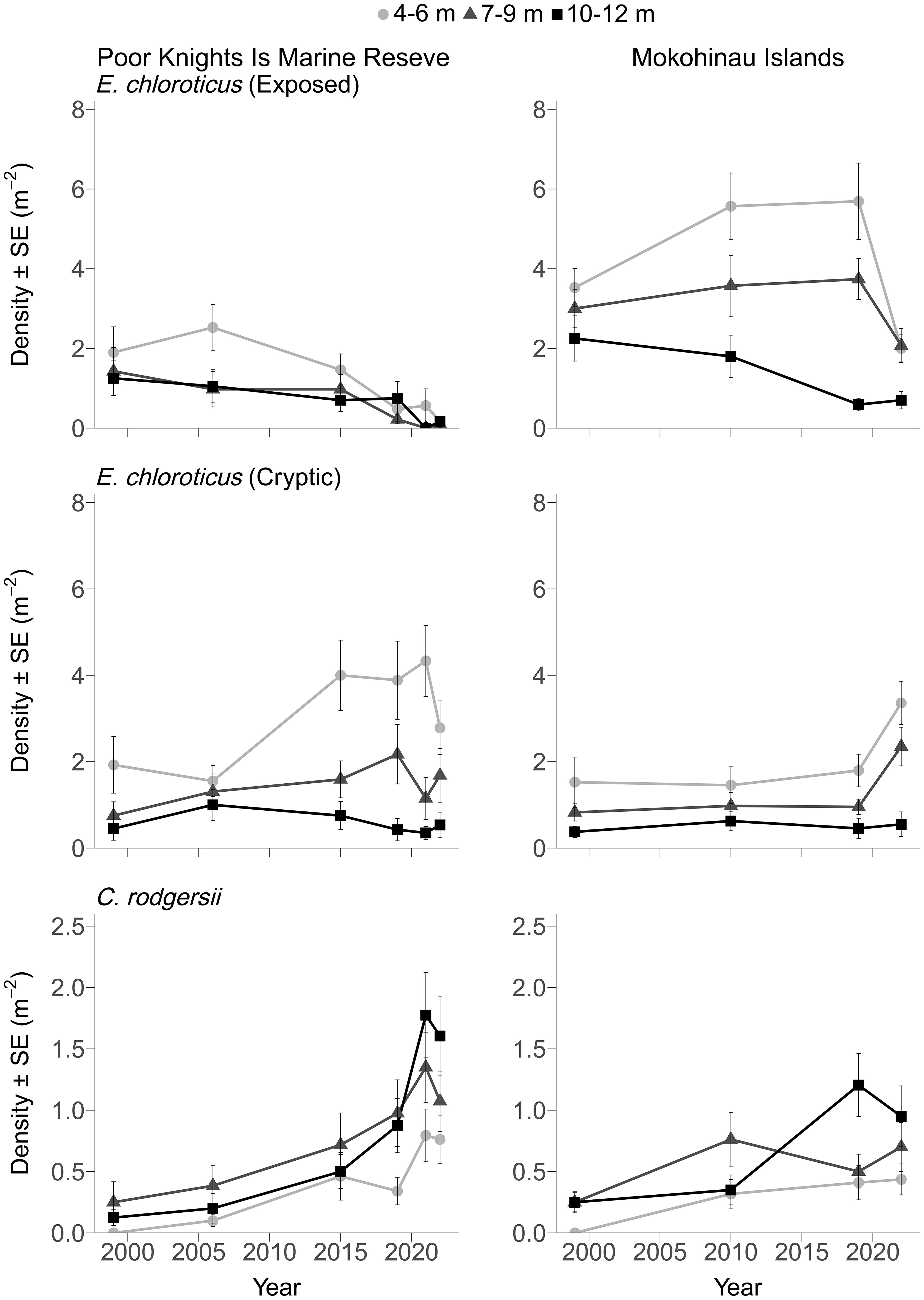
Figure 2 Mean density of Exposed and Cryptic E. chloroticus and C. rodgersii at the fished Mokohinau Is (Fished) and in the Poor Knights Islands Marine Reserve from 1999 to 2022 across three depth ranges (4-6m, 7-9m, 10-12m). The years used for statistical analysis were 1999, 2019 & 2022.
The density of cryptic E. chloroticus did not differ between the two locations but there was a significant effect of Year and with Depth (Figure 2; Supplementary Table 2). Cryptic E. chloroticus were most abundant in the shallow stratum (4-6 m) and declined with depth (Figure 2; Supplementary Table 3). At the PKI, the density of cryptic E. chloroticus exhibited a gradual increase over time. In contrast, cryptic E. chloroticus at the Mokohinau Is were generally stable over time, except for the sharp increase in 2022 at 4-6 and 7-9 m, which coincided with the decline in exposed individuals at these depths (Figure 2).
The size structure of E. chloroticus has remained relatively stable over time at the PKI (Supplementary Figure 3). In 1999, the majority of large urchins (>70 mm TD) were exposed whereas these large size classes have become increasingly cryptic over time (Supplementary Figure 3). At the Mokohinau Is, the population has been consistently dominated by large, exposed urchins (>70 mm), but an increased proportion of these large individuals exhibited a cryptic behaviour in 2022 (Supplementary Figure 3). The size-structure data also highlights the shift in behaviour of E. chloroticus at PKI, where the proportion of exposed individuals has declined over time with the majority of individuals (95%) being cryptic and hidden in crevices in 2022 (Figure 2; Supplementary Figure 3). In contrast, at the Mokohinau Is, most sea urchins have remained exposed over time, except in 2022 when a higher percentage (56%) were cryptic than in previous years (~23%).
The density of C. rodgersii increased over the 23-year study period at both locations (Figure 2), but this increase was greatest at the PKI as reflected by a significant interaction between Location x Year (Supplementary Table 2). The increase in density was evident across all depths but densities were generally highest in the two deepest stratum. The greatest relative increase occurred in the 4-6 m depth range as C. rodgersii was not recorded in this depth range in 1999 and was indicated in the significant Year x Depth interaction (Supplementary Tables 2, 3). Overall, the density of C. rodgersii increased by 9.3 times (CL95: 5.5-15.8, 1999-2022: p < 0.001) between 1999 and 2022 at the PKI and 4.3 times (CL95: 2.6-6.9, 1999-2022: p < 0.001) at the Mokohinau Is (Figure 2). In 1999, the size structure of C. rodgersii at the PKI and Mokohinau Is was dominated by low numbers of large individuals (≥80 mm TD; Supplementary Figure 4), whereas subsequent surveys recorded a greater range of sizes with a higher proportion of smaller urchins, including individuals <50 mm in the most recent surveys (Figure 1C; Supplementary Figure 4).
Extent and type of urchin barrens
The Mokohinau Is generally had higher total barren cover than the PKI (Figure 3) with the respective long-term averages of 31.1% and 10.28% of reef <15 m deep (Supplementary Tables 4A–C). The cover of urchin barrens did not differ at the two locations at the start and end of the time series (1999 vs. 2022), but the two locations had differing patterns over time (Figure 3; Year x Location: p = 0.0058, Supplementary Table 4B). Urchin barren cover at the Mokohinau Is, was significantly higher in 2019, whereas at the PKI, there was no significant difference between 1999, 2019 and 2022 (Figure 3; Supplementary Table 4C). There was a general downward trend at PKI between 2006 and 2021, and a subsequent increase in 2022 (Figure 3). There was also a significant Location x Depth interaction (Supplementary Table 2, p = 0.004) and post hoc tests revealed the presence of barrens increased with depth at the PKI (Supplementary Table 4D), but there was no effect of depth at the Mokohinau Is (Supplementary Table 4D).
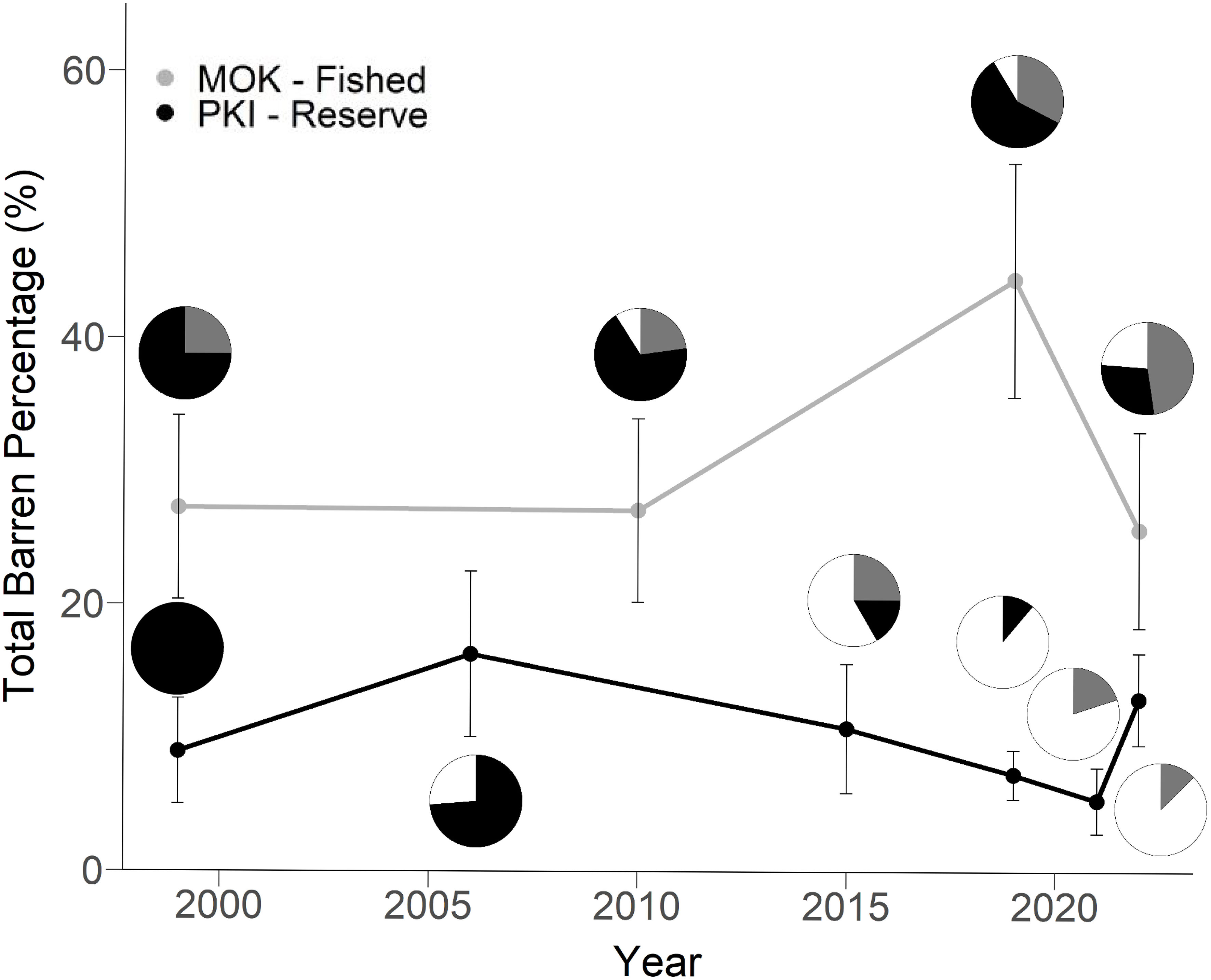
Figure 3 Mean extent of urchin barrens on shallow reefs (0-15 m depth) at the fished Mokohinau Is (MOK – Fished, grey line) and in the Poor Knights Is Marine Reserve (PKI – Reserve, black solid line) from 1999 to 2022. Pie charts indicate relative proportion of different urchin barren types during each survey: E. chloroticus barren (black), C. rodgersii barren (white) and mixed barren, i.e. both species present (grey). The years used for statistical analysis were 1999, 2019 & 2022.
While the overall extent of urchin barrens at both locations did not differ between 1999 and 2022, there was a large change in the type of urchin barrens that dominated each location. In 1999, E. chloroticus barrens were dominant at both locations and C. rodgersii barrens were not recorded (Figure 3). At the PKI, there has been a general decline in the proportion of E. chloroticus barrens, with none recorded in 2022 and an increase in the predominance of C. rodgersii barrens (Figure 3). C. rodgersii barrens were first recorded at PKI in 2006 at two sites (Frasers and Cleanerfish), occurring at depths of 10-12 m in areas that were previously classified as kelp forest. The establishment of C. rodgersii barrens has primarily occurred at depths ≥10 m, whereas E. chloroticus barrens were historically more common on shallower reef, as evident in the density data (Figure 2). At the Mokohinau Is, E. chloroticus barrens have remained the dominant barren type in shallow water (>10m), but C. rodgersii barrens have also established in deeper water (≥10 m) and the relative contribution of C. rodgersii barrens has increased over time (Figures 2, 3). The increase in C. rodgersii barrens contributed to the high overall barren cover in 2019 and the subsequent decrease in barren cover in 2022 was due to a decline in E. chloroticus barrens at some of the sites (Figure 3).
Rock lobster size and abundance
The density of lobster was very low at both locations (Figure 4), with lobster only present on 3 out of 24 transects (12.5%) at Mokohinau Is and 4 out of 27 transects (15%) at PKI. For J. edwardsii, only one individual was recorded at the Mokohinau Islands (Carapace length [CL]: 110 mm) and four at the PKI (26 transects, CL: 110, 110, 125, 130 mm). S. verreauxi were slightly more common, with four recorded at the Mokohinau Is (CL: 110-145 mm) and eight recorded at the PKI (CL: 90-160 mm).
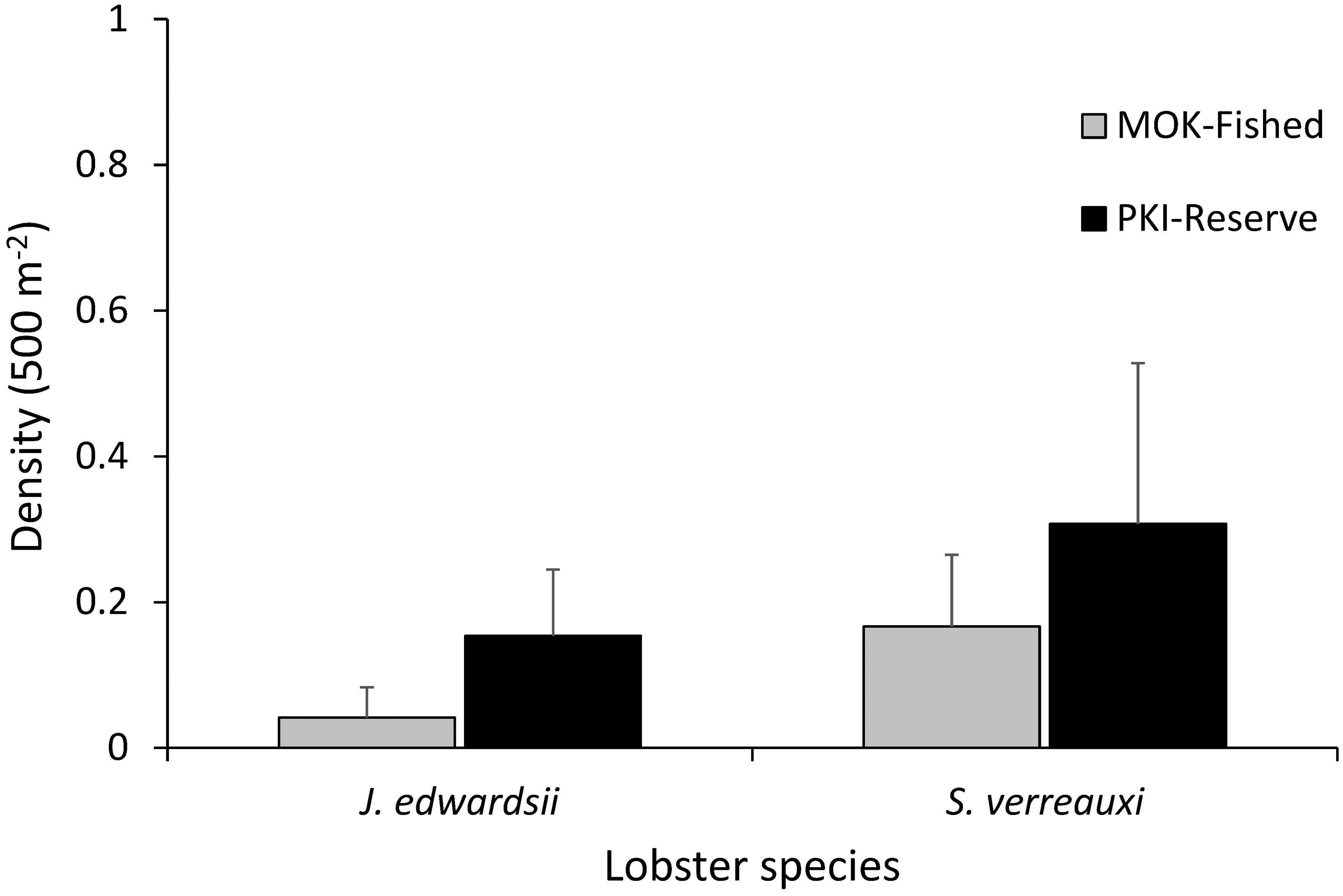
Figure 4 Mean density of rock lobster species J. edwardsii and S. verreauxi at the fished Mokohinau Islands (MOK - Fished) and in the Poor Knights Islands Marine Reserve (PKI - Reserve).
Discussion
The densities and population structure of the two sea urchins, E. chloroticus and C. rodgersii, exhibited markedly different responses in relation marine reserve protection over the 23-year period. Densities of exposed E. chloroticus declined in the Poor Knights Islands Marine Reserve and urchin barrens associated with this species disappeared over the 23-year period. In contrast, densities of exposed E. chloroticus remained relatively stable at the fished location, with no long-term change in the extent of associated barrens. These results are consistent with a top-down effect of the very high abundance of predatory fish in the reserve (Denny et al., 2004; Schiel et al., 2018; Allard, 2020) and demonstrate how urchin barrens associated with this species can be reversed following marine protection. In contrast, the densities of the subtropical sea urchin C. rodgersii increased significantly at both reserve and non-reserve sites, with a concomitant emergence and increase in the extent of urchin barrens associated with this species (Figure 1D). The increase in this species coincides with significant ocean warming in northeastern New Zealand over the study period and the increase in the marine reserve suggests a lack of predators capable of preventing this outbreak. Despite full protection from fishing at the PKI, our surveys found very low numbers of rock lobster (J. edwardsii and S. verreauxi), which are likely the most important predators of C. rodgersii in northern New Zealand. Our results demonstrate the emerging threat C. rodgersii poses to rocky reefs in the region and how marine protection alone may not increase resilience to this threat.
Long-term population trends of the common sea urchin E. chloroticus followed similar patterns to those seen inside and outside other marine reserves in northeastern New Zealand, with long-term declines in exposed urchins and associated barrens within marine reserves due to increased predation (Babcock et al., 1999; Shears and Babcock, 2003; Edgar et al., 2017). The long-term decline in E. chloroticus and a shift to predominantly cryptic behaviour within the PKI marine reserve is most likely attributable to the large increase in abundance and size of C. auratus (Denny et al., 2004; Schiel et al., 2018; Allard, 2020), which are important predators of E. chloroticus (Shears and Babcock, 2002; Spyksma et al., 2017). Despite the rapid increase in C. auratus populations in the PKI marine reserve (Denny et al., 2004; Schiel et al., 2018; Allard, 2020), the declines in E. chloroticus numbers and associated barrens were gradual, taking ~20 years for a complete decline in E. chloroticus barren habitat to be seen. This is consistent with other marine reserves globally, where trophic interactions on non-fished species take on average 13 years to manifest, while fished species generally recover much faster (~5 years; Babcock et al., 2010). At the Mokohinau Is, where C. auratus are heavily targeted and remain at low levels (Denny et al., 2004; Allard, 2020), E. chloroticus densities and the cover of urchin barrens have generally remained stable. However, there was a sharp decrease in exposed urchins and concurrent increase in cryptic urchins between 2019 and 2022 at the Mokohinau Islands. This likely reflects a temporary shift in behaviour from exposed to cryptic caused by an extended bloom of the tropical dinoflagellate Ostreopsis siamensis that occurred at the Mokohinau Islands at the time of the 2022 survey (December 2021-May 2022; authors pers. obs.). O. siamensis has a number of impacts on E. chloroticus, including causing urchins to seek shelter and temporarily exhibit cryptic behaviour (Shears and Ross, 2009). The decline in the extent of E. chloroticus barrens at the Mokohinau Is between 2019 and 2022 may be also related to O. siamensis blooms as well as commercial harvest of E. chloroticus from some of the sites (~80 tonnes of E. chloroticus were harvested from the Mokohinau Is between 2017-2022, P. Herbert – Sea Urchin New Zealand, pers. comm.). Nevertheless, the general contrasting long-term patterns and trajectories between the marine reserve and fished locations provide further evidence on how long-term protection of predators can exert a top-down control on some sea urchin species and decrease the prevalence of urchin barrens.
In contrast, there was no apparent effect of marine reserve protection on C. rodgersii, with the population increasing steadily between 1999 and 2022 and the subsequent development of C. rodgersii barrens at both reserve and fished locations. This demonstrates that while the high population abundance of C. auratus at the PKI has been sufficient to control E. chloroticus populations, it has not had a regulatory effect on C. rodgersii populations. While C. auratus are known to be important predators of E. chloroticus, they are not known to predate on C. rodgersii which have considerably longer spines and are predominantly cryptic during the day (Ling et al., 2009b; Byrne and Andrew, 2020). Large rock lobster (J. edwardsii and S. verreauxi), on the other hand, can effectively predate on C. rodgersii (Ling et al., 2009b; Ling and Johnson, 2012; Provost et al., 2017; Day et al., 2021) and healthy populations of lobster have been demonstrated to regulate sea urchin populations and prevent the initial formation of C. rodgersii barrens in Tasmania (Ling et al., 2009b; Ling and Johnson, 2012). Rock lobster were very rare at both locations in this study, which is congruous with J. edwardsii being considered ecologically extinct in the wider region (MacDiarmid et al., 2013). The low densities (<1 lobster 500 m2) and lack of recovery of lobster following marine reserve protection is consistent with previous studies at PKI (MacDiarmid and Breen, 1992; Taylor et al., 2011). This strongly contrasts mainland marine reserves in New Zealand where lobster have increased following protection and occur at much greater abundances (>10 m2) than seen in the Poor Knights Islands marine reserve (Freeman et al., 2012). The reasons for the lack of recovery following protection from fishing at the PKI are generally unknown, but may include limited larval supply associated with the small and isolated islands, limited shallow reef habitat for settlement, and a low regional pool of larvae associated with a severely depleted spawning population (MacDiarmid and Breen, 1992; MacDiarmid et al., 2016; Hanns, 2021). Despite full no-take protection at the PKI, an absence of key predators and lack of trophic redundancy in both locations has meant that with warmer conditions more suitable for C. rodgersii, the populations have been able to increase unchecked.
This study provides the first documentation of an increase in C. rodgersii, and emergence of urchin barren habitat associated with this species in New Zealand. Only E. chloroticus has previously been reported as forming urchin barrens in the region (Choat and Schiel, 1982; Shears and Babcock, 2004) and earlier research at these study locations only report low densities of C. rodgersii with limited grazing impacts (Riddell, 1980; Schiel, 1984; Battershill, 1986; Berben et al., 1988; Cole, 1993). In our study, E. chloroticus barrens were common at both locations in 1999, and C. rodgersii was only present in a low number of quadrats (10%) and did not form barrens. Now, 23 years later, C. rodgersii are commonly recorded in quadrats (40%) and barrens associated with this species are a common feature of reef ecosystems at both the PKI and Mokohinau Islands. While quantitative data is lacking from other locations, there are anecdotal reports of similar increases in C. rodgersii over the last decade at other offshore islands in northeastern New Zealand and in areas of the mainland coast north of the PKI (authors pers. obs.). At both locations examined in the current study, C. rodgersii and associated barrens were generally found deeper than E. chloroticus barrens (>10 m), often with an area of overlap (“mixed barrens”) at intermediate depths (~7-10 m). The presence of barrens did not vary across the depths sampled at the Mokohinau Islands, but there was an increasing likelihood of barrens with depth at the PKI which likely related to the decline in E. chloroticus barrens in shallow and emergence of deeper C. rodgersii barrens. C. rodgersii were most abundant in the deepest depth substratum (10-12 m) at both locations, but also extended well beyond the depths monitored and has formed extensive barrens to depths of ~25 m at some monitoring sites (authors, unpublished. data). This deeper distribution has been observed in Tasmania with C. rodgersii forming barrens to depths of ~ 58 m (Perkins et al., 2015). Consequently, we have likely underestimated the overall extent of C. rodgersii barrens in each location. The greater depth range of C. rodgersii poses a threat to deeper kelp forests and other reef habitats that are not typically impacted by E. chloroticus grazing, suggesting it may pose a greater threat to reef biodiversity than E. chloroticus. The range expansion of C. rodgersii into Tasmania has been described as the “single largest biologically mediated threat to the integrity of important shallow water rocky reef communities” (Johnson et al., 2011), with extensive economic implications to environment, tourism and fisheries (Johnson et al., 2005; Ling and Keane, 2018). While these effects in New Zealand are likely in an earlier stage than Tasmania and their extent largely undocumented, C. rodgersii likely presents an increasing and serious threat to rocky reef ecosystems.
The increase in C. rodgersii observed in this study coincides with a period of warming ocean temperatures, which is consistent with trends observed in Tasmania (Johnson et al., 2005; Ling et al., 2008; Ling et al., 2009a) and earlier predictions for this species (Pecorino et al., 2013a; Byrne and Andrew, 2020). Recent evidence suggests local recruitment is playing an increasingly important role for New Zealand populations (Thomas et al., 2021) and our results clearly demonstrate a widening size structure at both locations over time. In 1999, only large individuals (≥80 mm TD) were recorded, which is consistent with earlier studies that noted an absence of juvenile C. rodgersii on northeastern New Zealand reefs (Cole, 1993). The large increase in density at PKI in 2022 coincided with higher numbers of small urchins, which may indicate higher recruitment associated with recent warm years. In eastern Tasmania, warmer ocean temperatures were a major contributing factor to the population expansion of C. rodgersii as this provided more favourable developmental conditions for larvae (Ling, 2008; Ling et al., 2009a). Previous research from northeastern New Zealand has shown that winter temperatures when C. rodgersii spawn were above the threshold for successful reproduction and recruitment albeit at the lower end of the temperature limit (Pecorino et al., 2013a; Pecorino et al., 2013b). Despite a warming trend during summer and autumn, there was no evidence of long-term warming in winter and spring which would include the time of spawning and larval dispersal. Nevertheless, recent marine heatwaves and consistently warmer than average conditions since 2015 across all months may have provided more favourable conditions for reproduction and larval development which is reflected in the emergence of juvenile C. rodgersii. While the exact mechanisms linking warming and population increases in northern New Zealand are unknown, it is likely that C. rodgersii populations will continue to increase in density with continued warming and increasingly self-sustaining populations (Thomas et al., 2021).
MPAs are traditionally considered an effective tool for rebuilding predator populations, and subsequently restoring trophic interactions and increasing ecosystem resilience (Roberts et al., 2017; Sala and Giakoumi, 2018; Eger and Baum, 2020; Peleg et al., 2023). However, as seen in this study, marine protection alone may not always restore the full complement of predators required to prevent irruptions of native species. While there is some contention over the role of lobster in controlling C. rodgersii populations and associated barren habitat (Glasby and Gibson, 2020; Smith et al., 2022; Kingsford and Byrne, 2023), rock lobster are likely the most important predator of C. rodgersii in New Zealand waters and they have not recovered in the marine reserve examined. In both Tasmania and now New Zealand, the increase in C. rodgersii coincides with ocean warming, but also follows a long history of exploitation of J. edwardsii, which has provided an opportunity for this species to proliferate, unconstrained from predatory control (Ling et al., 2009b; Ling et al., 2015). While MPAs with healthy lobster populations may be more resilient to future outbreaks of C. rodgersii (Ling et al., 2009b; Perkins et al., 2020; Ling and Keane, 2021), recovery of predators in MPAs may be limited by both local and regional factors. Furthermore, once extensive urchin barrens have established, these areas are extremely difficult to reverse and may take decades to recover, even with full marine protection (Shears and Babcock, 2003; Babcock et al., 2010; Ling et al., 2015; Perkins et al., 2020; Ling and Keane, 2021).
Consequently, there is an urgent need for an active and multi-faceted approach to controlling C. rodgersii in northern New Zealand in order to reduce their potential socio-economic and ecological impacts and build ecosystem resilience to future warming. While more research into the role of key predators such as rock lobster in controlling C. rodgersii populations is needed, rebuilding populations of key predators at large spatial scales is crucial to increase resilience and can be achieved by increasing the area of MPAs combined with large-scale reductions in fisheries catch. In areas where C. rodgersii have already established, active restoration through culling or harvest, or the introduction of lobsters, may also be needed to promote recovery (Tracey et al., 2015; Ling and Keane, 2021; Miller et al., 2022).
Global research into the effects of climate change on marine ecosystems has largely focused on how range extending species can lead to large-scale changes in ecosystems (Pecl et al., 2017). Our study demonstrates an irruption of a native species with the scope to have comparable ecosystem level consequences to that of range extending species. As seen in Tasmania, it is likely that this increase has been facilitated by a warming climate and the historic and current overharvesting of the only known predator in the region. Increases in native species can pose additional challenges to conservation and management (Carey et al., 2012), and in this study, marine protection did not provide the expected resilience to this irruption. Consequently, the absence of large predators from many marine ecosystems ultimately reduces ecosystem resilience and provides unabated opportunities for new species to take hold under changing environmental conditions. The rate and scale of the emergence and outbreaks of these species is therefore likely to intensify with climate change unless urgent and radical changes are made to how we manage marine ecosystems and fisheries (Lotze et al., 2011; Duarte et al., 2020).
Data availability statement
The raw data supporting the conclusions of this article will be made available by the authors, without undue reservation.
Author contributions
NS designed and led the monitoring and data collection. CB performed the data analysis and wrote the manuscript with input from NS. CB and NS contributed to the manuscript revisions and approved the final version. All authors contributed to the article and approved the submitted version.
Funding
Funding for monitoring has been provided by Department of Conservation and Auckland Council (Mokohinau Is). Additional funding to NS was provided through a Royal Society of New Zealand Rutherford Discovery Fellowship, and to CB and NS through National Science Challenge: Sustainable Seas (1.1 Ecological responses to cumulative effects).
Acknowledgments
We would like to acknowledge Te Whanau a Rangiwhakaahu Hapu, Ngāti Manuhiri and other hapu of Ngātiwai who whakapapa to Tawhiti Rahi and Aorangi Island’s (the Poor Knights Is) and the Mokohinau Islands. Thanks to the many divers who have helped with surveys over the years including Caitlin Blain, Charles Bedford, Ohad Peleg, Kelsey Miller, Benn Hanns, Paul Caiger, Tim Haggitt, Rob Russell, Debbie Freeman, Don Neale, Alan Fleming, Jarrod Walker, Paul Buisson, Richie Hughes, Kaitlin Lawrence and Kirsten Rodgers. Much appreciation also to Brady Doak for skippering RV Proteus and RV Hawere, and sharing his immense knowledge of the Poor Knights. Kelsey Miller has also provided invaluable comments on the manuscript. Thank you also to Benn Hanns for assisting with the statistics and Robert Smith for assistance with extracting temperature data. We would also like to thank the two reviewers for their thorough and constructive review of the manuscript.
Conflict of interest
The authors declare that the research was conducted in the absence of any commercial or financial relationships that could be construed as a potential conflict of interest.
Publisher’s note
All claims expressed in this article are solely those of the authors and do not necessarily represent those of their affiliated organizations, or those of the publisher, the editors and the reviewers. Any product that may be evaluated in this article, or claim that may be made by its manufacturer, is not guaranteed or endorsed by the publisher.
Supplementary material
The Supplementary Material for this article can be found online at: https://www.frontiersin.org/articles/10.3389/fmars.2023.1224067/full#supplementary-material
References
Allard H. (2020). The direct and indirect effects of marine reserve protection on reef fish assemblages (Auckland (NZ: University of Auckland).
Andrew N. L. (1988). Ecological aspects of the common sea urchin, Evechinus chloroticus, in northern New Zealand: a review. N. Z. J. Mar. Freshwat. Res. 22, 415–426. doi: 10.1080/00288330.1988.9516313
Babcock R. C., Kelly S., Shears N. T., Walker J. W., Willis T. J. (1999). Changes in community structure in temperate marine reserves. Mar. Ecol. Prog. Ser. 189, 125–134. doi: 10.3354/meps189125
Babcock R. C., Shears N. T., Alcala A. C., Barrett N. S., Edgar G. J., Lafferty K., et al. (2010). Decadal trends in marine reserves reveal differential rates of change in direct and indirect effects. Proc. Natl. Acad. Sci. U.S.A. 107, 18256–18261. doi: 10.1073/pnas.0908012107
Ban S. S., Graham N. A., Connolly S. R. (2014). Evidence for multiple stressor interactions and effects on coral reefs. Global Change Biol. 20, 681–697. doi: 10.1111/gcb.12453
Bates D., Mächler M., Bolker B., Walker S. (2015). Fitting linear mixed-effects models using lme4. J. Stat. Software 67, 1–48. doi: 10.18637/jss.v067.i01
Battershill C. (1986). Marine benthos of caves, archways, and vertical reef walls of the Poor Knights Islands, A Poor Knights Islands Marine Reserve study. (Auckland, New Zealand: University of Auckland).
Behrens M. D., Lafferty K. D. (2004). Effects of marine reserves and urchin disease on southern Californian rocky reef communities. Mar. Ecol. Prog. Ser. 279, 129–139. doi: 10.3354/meps279129
Bell J. J., Smith R. O., Micaroni V., Strano F., Balemi C. A., Caiger P. E., et al. (2022). Marine heat waves drive bleaching and necrosis of temperate sponges. Curr. Biol. 33, 158–163. doi: 10.1016/j.cub.2022.11.013
Berben P., McCrone A., Creese R., Ballantine W. (1988). “The Mokohinau Islands: a marine survey,” in Leigh Laboratory Bulletin, vol. 21. (Auckland, New Zealand: University of Auckland) 21, 1–9.
Breitburg D. L., Riedel G. F. (2005). “Multiple stressors in marine systems,” in Marine conservation biology: the science of maintaining the sea's biodiversity. Eds. Norse E. A., Crowder L. B. (Washington DC: Island Press).
Brook F. (2002). Biogeography of near-shore reef fishes in northern New Zealand. J. R. Soc N. Z. 32 (2), 243–274. doi: 10.1080/03014223.2002.9517694
Byrne M., Andrew N. L. (2020). “Centrostephanus rodgersii and Centrostephanus tenuispinus,” in Developments in Aquaculture and Fisheries Science. Ed. Lawrence J. M. (London: Elsevier), 379–396. doi: 10.1016/B978-0-12-819570-3.00022-6
Carey M. P., Sanderson B. L., Barnas K. A., Olden J. D. (2012). Native invaders–challenges for science, management, policy, and society. Front. Ecol. Environ. 10, 373–381. doi: 10.1890/110060
Choat J., Ayling A. (1987). The relationship between habitat structure and fish faunas on New Zealand reefs. J. Exp. Mar. Biol. Ecol. 110, 257–284. doi: 10.1016/0022-0981(87)90005-0
Choat J., Ayling A., Schiel D. (1988). Temporal and spatial variation in an island fish fauna. J. Exp. Mar. Biol. Ecol. 121, 91–111. doi: 10.1016/0022-0981(88)90249-3
Choat J., Schiel D. R. (1982). Patterns of distribution and abundance of large brown algae and invertebrate herbivores in subtidal regions of northern New Zealand. J. Exp. Mar. Biol. Ecol. 60, 129–162. doi: 10.1016/0022-0981(82)90155-1
Cole R. G. (1993). Distributional relationships among subtidal algae, sea urchins and reef fish in northeastern New Zealand (Auckland (NZ: University of Auckland).
Cole R. G., Creese R. G., Grace R. V., Irving P., Jackson B. R. (1992). Abundance patterns of subtidal benthic invertebrates and fishes at the Kermadec Islands. Mar. Ecol. Prog. Ser. 82, 207–218. doi: 10.3354/meps082207
Day J. K., Knott N. A., Swadling D. S., Ayre D. J. (2021). Dietary analysis and mesocosm feeding trials confirm the eastern rock lobster (Sagmariasus verreauxi) as a generalist predator that can avoid ingesting urchin spines during feeding. Mar. Freshwat. Res. 72, 1220–1232. doi: 10.1071/MF20287
Denny C. M., Willis T. J., Babcock R. C. (2004). Rapid recolonisation of snapper Pagrus auratus: Sparidae within an offshore island marine reserve after implementation of no-take status. Mar. Ecol. Prog. Ser. 272, 183–190. doi: 10.3354/meps272183
Duarte C. M., Agusti S., Barbier E., Britten G. L., Castilla J. C., Gattuso J.-P., et al. (2020). Rebuilding marine life. Nature 580, 39–51. doi: 10.1038/s41586-020-2146-7
Edgar G. J., Barrett N. S., Stuart-Smith R. D. (2009). Exploited reefs protected from fishing transform over decades into conservation features otherwise absent from seascapes. Ecol. Appl. 19, 1967–1974. doi: 10.1890/09-0610.1
Edgar G. J., Stuart-Smith R. D., Thomson R. J., Freeman D. J. (2017). Consistent multi-level trophic effects of marine reserve protection across northern New Zealand. PloS One 12, e0177216. doi: 10.1371/journal.pone.0177216
Edwards M., Richardson A. J. (2004). Impact of climate change on marine pelagic phenology and trophic mismatch. Nature 430, 881–884. doi: 10.1038/nature02808
Eger A. M., Baum J. K. (2020). Trophic cascades and connectivity in coastal benthic marine ecosystems: a meta-analysis of experimental and observational research. Mar. Ecol. Prog. Ser. 656, 139–152. doi: 10.3354/meps13430
Eisenlord M., Groner M., Yoshioka R., Elliott J., Maynard J., Fradkin S., et al. (2016). Ochre star mortality during the 2014 wasting disease epizootic: role of population size structure and temperature. Philos. Trans. R. Soc. B. Biol. 371 (20150212). doi: 10.1098/rstb.2015.0212
Estes J. A., Duggins D. O. (1995). Sea otters and kelp forests in Alaska: generality and variation in a community ecological paradigm. Ecol. Monogr. 65, 75–100. doi: 10.2307/2937159
Estes J. A., Palmisano J. F. (1974). Sea otters: their role in structuring nearshore communities. Science 185, 1058–1060. doi: 10.1126/science.185.4156.1058
Estes J. A., Terborgh J., Brashares J. S., Power M. E., Berger J., Bond W. J., et al. (2011). Trophic downgrading of planet Earth. Science 333, 301–306. doi: 10.1126/science.1205106
Fell H. B. (1949). "The occurrence of Australian echinoids in New Zealand waters", in. Records Auckland Institute Museum 6, 343–346. Available at: https://www.jstor.org/stable/42906025.
Filbee-Dexter K., Scheibling R. E. (2014). Sea urchin barrens as alternative stable states of collapsed kelp ecosystems. Mar. Ecol. Prog. Ser. 495, 1–25. doi: 10.3354/meps10573
Filbee-Dexter K., Wernberg T., Grace S., Thormar J., Fredriksen S., Narvaez C., et al. (2020). Marine heatwaves and the collapse of marginal North Atlantic kelp forests. Sci. Rep. 10, 1–11. doi: 10.1038/s41598-020-70273-x
Freeman D. J., Macdiarmid A. B., Taylor R. B., Davidson R. J., Grace R. V., Haggitt T. R., et al. (2012). Trajectories of spiny lobster Jasus edwardsii recovery in New Zealand marine reserves: is settlement a driver? Environ. Conserv. 39, 295–304. doi: 10.1017/S037689291200015X
Gallardo B., Aldridge D. C., González-Moreno P., Pergl J., Pizarro M., Pyšek P., et al. (2017). Protected areas offer refuge from invasive species spreading under climate change. Global Change Biol. 23, 5331–5343. doi: 10.1111/gcb.13798
Glasby T. M., Gibson P. T. (2020). Decadal dynamics of subtidal barrens habitat. Mar. Environ. Res. 154, 104869. doi: 10.1016/j.marenvres.2019.104869
Greenville A. C., Wardle G. M., Dickman C. R. (2012). Extreme climatic events drive mammal irruptions: regression analysis of 100-year trends in desert rainfall and temperature. Ecol. Evol. 2, 2645–2658. doi: 10.1002/ece3.377
Hammerschlag N., McDonnell L. H., Rider M. J., Street G. M., Hazen E. L., Natanson L. J., et al. (2022). Ocean warming alters the distributional range, migratory timing, and spatial protections of an apex predator, the tiger shark (Galeocerdo cuvier). Global Change Biol. 28, 1990–2005. doi: 10.1111/gcb.16045
Hanns B. J. (2021). Marine reserves, Fisheries, and the spiny lobster Jasus edwardsii (Auckland (NZ: University of Auckland).
Hanns B. J., Haggitt T., Shears N. T. (2022). Marine protected areas provide unfished reference information to empirically assess fishery status. Biol. Conserv. 276, 109775. doi: 10.1016/j.biocon.2022.109775
Hartig F. (2022). DHARMa: Residual diagnostics for hierarchical (Multi-level/mixed) regression models. Available at: https://CRAN.R-project.org/package=DHARMa.
Harvell C., Montecino-Latorre D., Caldwell J., Burt J., Bosley K., Keller A., et al. (2019). Disease epidemic and a marine heat wave are associated with the continental-scale collapse of a pivotal predator (Pycnopodia helianthoides). Sci. Adv. 5, eaau7042. doi: 10.1126/sciadv.aau7042
Huang B., Liu C., Banzon V., Freeman E., Graham G., Hankins B., et al. (2021). Improvements of the daily optimum interpolation sea surface temperature (DOISST) version 2.1. J. Clim. 34, 2923–2939. doi: 10.1175/jcli-d-20-0166.1
Hutchings J. A., Reynolds J. D. (2004). Marine fish population collapses: consequences for recovery and extinction risk. Bioscience 54, 297–309. doi: 10.1641/0006-3568(2004)054[0297:MFPCCF]2.0.CO;2
Jackson J. B., Kirby M. X., Berger W. H., Bjorndal K. A., Botsford L. W., Bourque B. J., et al. (2001). Historical overfishing and the recent collapse of coastal ecosystems. Science 293, 629–637. doi: 10.1126/science.1059199
Johnson C. R., Banks S. C., Barrett N. S., Cazassus F., Dunstan P. K., Edgar G. J., et al. (2011). Climate change cascades: Shifts in oceanography, species' ranges and subtidal marine community dynamics in eastern Tasmania. J. Exp. Mar. Biol. Ecol. 400, 17–32. doi: 10.1016/j.jembe.2011.02.032
Johnson C. R., Ling S. D., Ross D. J., Shepherd S., Miller K. J. (2005). Establishment of the long-spined sea urchin (Centrostephanus rodgersii) in Tasmania: first assessment of potential threats to fisheries (Hobart, Tasmania: Institute of Marine and Antarctic Studies, Hobart: University of Tasmania).
Jones G., Andrew N. (1990). Herbivory and patch dynamics on rocky reefs in temperate Australasia: the roles of fish and sea urchins. Aust. J. Ecol. 15, 505–520. doi: 10.1111/j.1442-9993.1990.tb01474.x
Kelly S., MacDiarmid A. (2003). Movement patterns of mature spiny lobsters, Jasus edwardsii, from a marine reserve. N. Z. J. Mar. Freshwat. Res. 37, 149–158. doi: 10.1080/00288330.2003.9517153
Kingsford M., Byrne M. (2023). New South Wales rocky reefs are under threat. Mar. Freshw. Res. 74, 95–98. doi: 10.1071/MF22220
LaScala-Gruenewald D. E., Grace R. V., Haggitt T. R., Hanns B. J., Kelly S., MacDiarmid A., et al. (2021). Small marine reserves do not provide a safeguard against overfishing. Conserv. Sci. Pract. 3, e362. doi: 10.1111/csp2.362
Lenth R., Buerkner P., Herve M., Love J., Riebl H., Singmann H. (2021). Package “emmeans.". doi: 10.1080/00031305.1980.1048303
Ling S. (2008). Range expansion of a habitat-modifying species leads to loss of taxonomic diversity: a new and impoverished reef state. Oecologia 156, 883–894. doi: 10.1007/s00442-008-1043-9
Ling S., Johnson C. (2012). Marine reserves reduce risk of climate-driven phase shift by reinstating size-and habitat-specific trophic interactions. Ecol. Appl. 22, 1232–1245. doi: 10.2307/23213957
Ling S. D., Johnson C., Frusher S., King C. (2008). Reproductive potential of a marine ecosystem engineer at the edge of a newly expanded range. Global Change Biol. 14, 907–915. doi: 10.1111/j.1365-2486.2008.01543.x
Ling S., Johnson C., Frusher S., Ridgway K. (2009b). Overfishing reduces resilience of kelp beds to climate-driven catastrophic phase shift. Proc. Natl. Acad. Sci. U.S.A. 106, 22341–22345. doi: 10.1073pnas/0907529106
Ling S., Johnson C. R., Ridgway K., Hobday A. J., Haddon M. (2009a). Climate-driven range extension of a sea urchin: inferring future trends by analysis of recent population dynamics. Global Change Biol. 15, 719–731. doi: 10.1111/j.1365-2486.2008.01734.x
Ling S. D., Keane J. P. (2018). “Resurvey of the longspined sea urchin (Centrostephanus rodgersii) and associated barren reef in Tasmania,” (Hobart Tasmania: Institute of Marine and Antarctic Studies). doi: 10.13140/RG.2.2.16363.80162
Ling S., Keane J. (2021). Decadal resurvey of long-term lobster experimental sites to inform Centrostephanus control (Institute of Marine and Antarctic Studies, Hobart: University of Tasmania).
Ling S., Scheibling R., Rassweiler A., Johnson C., Shears N., Connell S., et al. (2015). Global regime shift dynamics of catastrophic sea urchin overgrazing. Philos. Trans. R. Soc Ser. B Biol. Sci. 370, 20130269. doi: 10.1098/rstb.2013.0269
Lotze H. K., Coll M., Magera A. M., Ward-Paige C., Airoldi L. (2011). Recovery of marine animal populations and ecosystems. Trends Ecol. Evol. 26, 595–605. doi: 10.1016/j.tree.2011.07.008
MacDiarmid A. (1991). Seasonal changes in depth distribution, sex ratio and size frequency of spiny lobster Jasus edwardsii on a coastal reef in northern New Zealand. Mar. Ecol. Prog. Ser. 70, 129–141. doi: 10.3354/meps070129
MacDiarmid A., Abraham E., Baker C., Carroll E., Chagué-Goff C., Cleaver P., et al. (2016). “Taking Stock–the changes to New Zealand marine ecosystems since first human settlement: synthesis of major findings, and policy and management implications,” in New Zealand Aquatic Environment and Biodiversity Report no. 170 (Wellington: Ministry of Primary Industries).
MacDiarmid A., Breen P. (1992). “Spiny lobster population change in a marine reserve,” in Proceedings of the Second International Temperate Reef Symposium, Auckland. (Auckland, New Zealand : NIWA, Wellington).
MacDiarmid A., Freeman D., Kelly S. (2013). Rock lobster biology and ecology: contributions to understanding through the Leigh Marine Laboratory 1962–2012. N. Z. J. Mar. Freshwat. Res. 47, 313–333. doi: 10.1080/00288330.2013.810651
Miller K. I., Blain C. O., Shears N. T. (2022). Sea urchin removal as a tool for macroalgal restoration: A review on removing “the spiny enemies”. Front. Mar. Sci. 9. doi: 10.3389/fmars.2022.831001
Miranda R. J., Coleman M. A., Tagliafico A., Rangel M. S., Mamo L. T., Barros F., et al. (2019). Invasion-mediated effects on marine trophic interactions in a changing climate: positive feedbacks favour kelp persistence. Proc. R. Soc B: Biol. Sci. 286, 20182866. doi: 10.1098/rspb.2018.2866
Neubauer P., Jensen O. P., Hutchings J. A., Baum J. K. (2013). Resilience and recovery of overexploited marine populations. Science 340, 347–349. doi: 10.1126/science.1230441
Pecl G. T., Araújo M. B., Bell J. D., Blanchard J., Bonebrake T. C., Chen I.-C., et al. (2017). Biodiversity redistribution under climate change: Impacts on ecosystems and human well-being. Science 355, eaai9214. doi: 10.1126/science.aai9214
Pecorino D., Lamare M. D., Barker M. F. (2012). Growth, morphometrics and size structure of the Diadematidae sea urchin Centrostephanus rodgersii in northern New Zealand. Mar. Freshw. Res. 63, 624–634. doi: 10.1071/MF12040
Pecorino D., Lamare M. D., Barker M. F. (2013b). Reproduction of the Diadematidae sea urchin Centrostephanus rodgersii in a recently colonized area of northern New Zealand. Mar. Biol. Res. 9, 157–168. doi: 10.1080/17451000.2012.708046
Pecorino D., Lamare M. D., Barker M. F., Byrne M. (2013a). How does embryonic and larval thermal tolerance contribute to the distribution of the sea urchin Centrostephanus rodgersii (Diadematidae) in New Zealand? J. Exp. Mar. Biol. Ecol. 445, 120–128. doi: 10.1016/j.jembe.2013.04.013
Peleg O., Blain C. O., Shears N. T. (2023). Long-term marine protection enhances kelp forest ecosystem stability. Ecol. Appl. e2895. doi: 10.1002/eap.2895
Perkins N. R., Hill N. A., Foster S. D., Barrett N. S. (2015). Altered niche of an ecologically significant urchin species, Centrostephanus rodgersii, in its extended range revealed using an Autonomous Underwater Vehicle. Estuar. Coast. Shelf Sci. 155, 56–65. doi: 10.1016/j.ecss.2015.01.014
Perkins N. R., Hosack G. R., Foster S. D., Monk J., Barrett N. S. (2020). Monitoring the resilience of a no-take marine reserve to a range extending species using benthic imagery. PloS One 15, e0237257. doi: 10.1371/journal.pone.0237257
Poloczanska E. S., Brown C. J., Sydeman W. J., Kiessling W., Schoeman D. S., Moore P. J., et al. (2013). Global imprint of climate change on marine life. Nat. Clim. Chan. 3, 919–925. doi: 10.1038/NCLIMATE1958
Pratchett M. S., Caballes C. F., Rivera-Posada J. A., Sweatman H. P. A. (2014). “Limits to understanding and managing outbreaks of crown-of-thorns starfish (Acanthaster spp.),” in Oceanography and Marine Biology: An Annual Review, vol. 52 . Eds. Hughes R. N., Hughes D. J., Smith I. P. (Boca Raton, FL: Crc Press-Taylor & Francis Group), 133–199. doi: 10.1201/b17143-4
Provost E. J., Kelaher B. P., Dworjanyn S. A., Russell B. D., Connell S. D., Ghedini G., et al. (2017). Climate-driven disparities among ecological interactions threaten kelp forest persistence. Global Change Biol. 23, 353–361. doi: 10.1111/gcb.13414
Purcell J. E. (2012). Jellyfish and ctenophore blooms coincide with human proliferations and environmental perturbations. Ann. Rev. Mar. Sci. 4, 209–235. doi: 10.1146/annurev-marine-120709-142751
R Core Team (2021). R: A Language and Environment for statistical computing (Vienna: Foundation for Statistical Computing. R Foundation for Statistical Computing).
Riddell D. J. (1980). Benthic communities of hard substrates at the Mokohinau Islands. (Auckland New Zealand) Tane. 26, 91–98.
Rilov G., Fraschetti S., Gissi E., Pipitone C., Badalamenti F., Tamburello L., et al. (2020). A fast-moving target: achieving marine conservation goals under shifting climate and policies. Ecol. Appl. 30, e02009. doi: 10.1002/eap.2009
Ripple W. J., Beschta R. L. (2012). Trophic cascades in Yellowstone: the first 15 years after wolf reintroduction. Biol. Conserv. 145, 205–213. doi: 10.1016/j.biocon.2011.11.005
Ripple W. J., Estes J. A., Beschta R. L., Wilmers C. C., Ritchie E. G., Hebblewhite M., et al. (2014). Status and ecological effects of the world’s largest carnivores. Science 343, 1241484. doi: 10.1126/science.1241484
Roberts C. M., O’Leary B. C., McCauley D. J., Cury P. M., Duarte C. M., Lubchenco J., et al. (2017). Marine reserves can mitigate and promote adaptation to climate change. Proc. Natl. Acad. Sci. U.S.A. 114, 6167–6175. doi: 10.1073/pnas.1701262114
Sala E., Giakoumi S. (2018). No-take marine reserves are the most effective protected areas in the ocean. ICES J. Mar. Sci. 75, 1166–1168. doi: 10.1093/icesjms/fsx059
Salinger M. J., Renwick J., Behrens E., Mullan A. B., Diamond H. J., Sirguey P., et al. (2019). The unprecedented coupled ocean-atmosphere summer heatwave in the New Zealand region 2017/18: drivers, mechanisms and impacts. Environ. Res. Lett. 14, 044023. doi: 10.1088/1748-9326/ab012a
Sambaraju K. R., Carroll A. L., Aukema B. H. (2019). Multiyear weather anomalies associated with range shifts by the mountain pine beetle preceding large epidemics. For. Ecol. Manage. 438, 86–95. doi: 10.1016/j.foreco.2019.02.011
Sangil C., Clemente S., Martín-García L., Hernández J. C. (2012). No-take areas as an effective tool to restore urchin barrens on subtropical rocky reefs. Estuar. Coast. Shelf Sci. 112, 207–215. doi: 10.1016/j.ecss.2012.07.025
Schiel D. R. (1984). Poor Knights Islands marine reserve survey. Leigh Laboratory Bulletin. (Auckland, New Zealand: University of Auckland) 15, 1–97.
Schiel D. R., Ayling T., Kingsford M. J., Battershill C. N., Choat J. H., Andrew N. L., et al. (2018). Change in the rocky reef fish fauna of the iconic Poor Knights Islands Marine Reserve in north-eastern New Zealand over 4 decades. Mar. Freshw. Res. 69, 1496–1507. doi: 10.1071/MF18037
Shears N. T., Babcock R. C. (2002). Marine reserves demonstrate top-down control of community structure on temperate reefs. Oecologia 132, 131–142. doi: 10.1007/s00442-002-0920-x
Shears N. T., Babcock R. C. (2003). Continuing trophic cascade effects after 25 years of no-take marine reserve protection. Mar. Ecol. Prog. Ser. 246, 1–16. doi: 10.3354/meps246001
Shears N. T., Babcock R. C. (2004). Community composition and structure of shallow subtidal reefs in northeastern New Zealand (Wellington: Department of Conservation).
Shears N. T., Babcock R. C., Duffy C. A. J., Walker J. W. (2004). Validation of qualitative habitat descriptors commonly used to classify subtidal reef assemblages in north-eastern New Zealand. N. Z. J. Mar. Freshwat. Res. 38, 743–752. doi: 10.1080/00288330.2004.9517273
Shears N. T., Babcock R. C., Salomon A. K. (2008). Context-dependent effects of fishing: Variation in trophic cascades across environmental gradients. Ecol. Appl. 18, 1860–1873. doi: 10.1890/07-1776.1
Shears N. T., Bowen M. M. (2017). Half a century of coastal temperature records reveal complex warming trends in western boundary currents. Sci. Rep. 7, 1–9. doi: 10.1038/s41598-017-14944-2
Shears N. T., Ross P. M. (2009). Blooms of benthic dinoflagellates of the genus Ostreopsis; an increasing and ecologically important phenomenon on temperate reefs in New Zealand and worldwide. Harmful Algae 8, 916–925. doi: 10.1016/j.hal.2009.05.003
Sim-Smith C., Kelly M. (2009). A literature review on the Poor Knights Islands Marine Reserve. NIWA Client Report (Whangarei: Department of Conservation).
Smith J. E., Keane J., Mundy C., Gardner C., Oellermann M. (2022). Spiny lobsters prefer native prey over range-extending invasive urchins. ICES J. Mar. Sci. 79, 1353–1362. doi: 10.1093/icesjms/fsac058
Spyksma A. J., Taylor R. B., Shears N. T. (2017). Predation cues rather than resource availability promote cryptic behaviour in a habitat-forming sea urchin. Oecologia 183, 821–829. doi: 10.1007/s00442-017-3809-4
Steneck R. S., Graham M. H., Bourque B. J., Corbett D., Erlandson J. M., Estes J. A., et al. (2002). Kelp forest ecosystems: biodiversity, stability, resilience and future. Environ. Conserv. 29, 436–459. doi: 10.1017/S0376892902000322
Sutton P. J., Bowen M. (2019). Ocean temperature change around New Zealand over the last 36 years. N. Z. J. Mar. Freshwat. Res. 53, 305–326. doi: 10.1080/00288330.2018.1562945
Taylor R. B., Morrison M. A., Shears N. T. (2011). Establishing baselines for recovery in a marine reserve (Poor Knights Islands, New Zealand) using local ecological knowledge. Biol. Conserv. 144, 3038–3046. doi: 10.1016/j.biocon.2011.09.009
Thomas L., Liggins L., Banks S., Beheregaray L., Liddy M., McCulloch G., et al. (2021). The population genetic structure of the urchin Centrostephanus rodgersii in New Zealand with links to Australia. Mar. Biol. 168, 1–11. doi: 10.1007/s00227-021-03946-4
Tracey S. R., Baulch T., Hartmann K., Ling S. D., Lucieer V., Marzloff M. P., et al. (2015). Systematic culling controls a climate driven, habitat modifying invader. Biol. Invasions. 17, 1885–1896. doi: 10.1007/s10530-015-0845-z
Vergés A., Steinberg P. D., Hay M. E., Poore A. G., Campbell A. H., Ballesteros E., et al. (2014). The tropicalization of temperate marine ecosystems: climate-mediated changes in herbivory and community phase shifts. Proc. R. Soc Ser. B. Biol. Sci. 281, 20140846. doi: 10.1098/rspb.2014.0846
Wallach A. D., Ripple W. J., Carroll S. P. (2015). Novel trophic cascades: apex predators enable coexistence. Trends Ecol. Evol. 30, 146–153. doi: 10.1016/j.tree.2015.01.003
Keywords: native invader, urchin barrens, ocean warming, predator loss, marine reserves, marine protected areas, temperate reefs
Citation: Balemi CA and Shears NT (2023) Emergence of the subtropical sea urchin Centrostephanus rodgersii as a threat to kelp forest ecosystems in northern New Zealand. Front. Mar. Sci. 10:1224067. doi: 10.3389/fmars.2023.1224067
Received: 17 May 2023; Accepted: 14 July 2023;
Published: 03 August 2023.
Edited by:
Gretchen E Hofmann, University of California, Santa Barbara, United StatesReviewed by:
Neville Scott Barrett, University of Tasmania, AustraliaTin Yan Hui, Lingnan University, China
Copyright © 2023 Balemi and Shears. This is an open-access article distributed under the terms of the Creative Commons Attribution License (CC BY). The use, distribution or reproduction in other forums is permitted, provided the original author(s) and the copyright owner(s) are credited and that the original publication in this journal is cited, in accordance with accepted academic practice. No use, distribution or reproduction is permitted which does not comply with these terms.
*Correspondence: Celia A. Balemi, Y2JhbDQwOUBhdWNrbGFuZHVuaS5hYy5ueg==