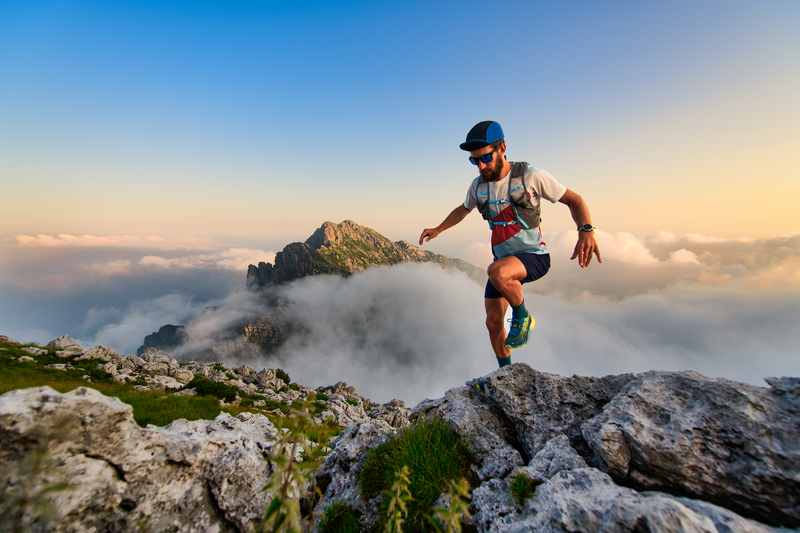
95% of researchers rate our articles as excellent or good
Learn more about the work of our research integrity team to safeguard the quality of each article we publish.
Find out more
ORIGINAL RESEARCH article
Front. Mar. Sci. , 28 December 2023
Sec. Marine Pollution
Volume 10 - 2023 | https://doi.org/10.3389/fmars.2023.1223766
This article is part of the Research Topic Marine Biofilms on Natural and Artificial Surfaces : Impacts and Novel Solutions View all 4 articles
The increasing need for renewable energy has led to the transition of renewable energy devices to the marine environment. Currently, mainly offshore wind farms have been completely developed and are operational in the North Sea. The solar energy sector is also rapidly evolving and floating photovoltaics are continuously created and deployed. In this study, we investigated the colonisation patterns and community changes with time of fouling fauna on the first floating photovoltaics in the coastal Dutch North Sea. Samples were collected by divers from the underwater side of 4 floaters, coated with different anti-fouling techniques (Intersleek, GreenPowerNano PPDura, Finsulate and Pato) at two different moments, shortly after the deployment of the floaters and approximately a year later. In total, 72 fouling taxa were identified on the floaters, from which ca. 11% are known to be non-indigenous species for the region. The anti-fouling coating Intersleek seemed to work the most efficiently against fouling colonisation, since the fouling community sampled from this floater contained the least taxa. However, the small number of samples collected from the different floaters did not allow for a direct comparison between the anti-fouling coatings. The communities evolved with time, with young communities accommodating a larger number of individuals and old communities having less individuals but higher biomass, indicating that the organisms become bigger in size and compete for the available space. Nevertheless, the communities had not reached a stable climax yet, while this process might take multiple years due to the dynamic environment in which floating photovoltaics are deployed. Monitoring the fouling communities occurring on floating photovoltaics in the North Sea for a long-term is necessary to understand the effects of these new man-made structures on the marine environment, especially since floating photovoltaics are moving to offshore locations and will be possibly co-located with offshore wind farms in the future.
Renewable energy plays a major role in decarbonizing the global energy supply. In offshore areas, wind energy is the fastest growing industry with a capacity of 56 GW on a global scale in 2021, and a total of 370 GW expected to be installed by 2031 (GWEC, 2022). However, solar energy is also emerging, with the use of floating photovoltaics (‘floatovoltaics’ or FPV) (Oliveira-Pinto et al., 2020; Hooper et al., 2021), reaching a capacity of 5.2 GW in 2022 in inshore waters (SERIS, 2019). Marine demonstrations of floatovoltaics have been established among others at an offshore site in the North Sea (Netherlands), in deep fjords (Norway), and tropical lagoons (Maldives) (Hooper et al., 2021; Vlaswinkel et al., 2023), while there is a continuously increasing demand for and construction of floatovoltaics all around the world (Sahu et al., 2016; Pimentel Da Silva and Branco, 2018).
Effects of floatovoltaics on the environment are currently mainly known for inshore locations such as pontoons, basins, lakes and lagoons in the UK (Exley et al., 2021), Brazil (Pimentel Da Silva and Branco, 2018), Spain (Redón Santafé et al., 2014), Australia (Rosa-Clot et al., 2017), and other countries. Studies at these locations mainly include effects of floatovoltaics on evaporation rates and water quality (Redón Santafé et al., 2014; Sahu et al., 2016; Pimentel Da Silva and Branco, 2018). On the contrary, our knowledge on the effects of offshore floatovoltaics on the environment remain scarce (Vlaswinkel et al., 2023). Possible offshore effects of floatovoltaics include impacts on substrates due to anchors and cables, and disturbances during installation, such as sediment resuspension, creation of electromagnetic fields and obstructing light penetration (Sahu et al., 2016; Pimentel Da Silva and Branco, 2018; Hooper et al., 2021). For offshore locations in the North Sea, a water column modelling study has predicted a small reduction of primary production when the platform coverage exceeds 20% of the model surface (Karpouzoglou et al., 2020), which translates to very extensive (tens to hundreds of kilometres of) coverage of the water surface, which is considered unrealistic for future large-scale offshore solar farms. The water column model also suggested a reduction of sediment suspension due to less turbulence under the floatovoltaics (Karpouzoglou et al., 2020), which could eventually result in clearer waters.
Offshore floating installations provide new artificial habitat that can get rapidly colonised by fouling organisms. This habitat is considered novel for fouling organisms (Holloway and Connell, 2002) and its introduction increases the local biodiversity. Fouling communities may contain up to 115 taxa per floating installation (Nall et al., 2017). The composition of such communities is different compared to that of fixed structures (Holloway and Connell, 2002), such as oil and gas platforms and offshore wind turbine foundations. For example, floating structures may contain ~10 times higher presence of non-indigenous species compared to fixed installations (Leclerc et al., 2020), while community functionality varies between floating and non-floating structures (Figueroa et al., 2021). These differences could be explained by the variation of the physical properties of the installations (e.g. complexity, materials, roughness, etc.) but also by the variability of environmental conditions (e.g. depth, water motion, disturbance regimes, etc.) (Holloway and Connell, 2002; Giachetti et al., 2020).
Fouling organisms increase the weight of floating structures. For example, fouling fauna occupying the shallow subtidal parts (0-0.25 m) of floating structures in Scotland, increase the total structure weight, by a mean of approx. 2.5 kg m-2 wet weight (Nall et al., 2017). From fixed structures in the German North Sea, wet weight biomasses of up to 67 kg m-2 have been reported at 1 meter depth (Krone et al., 2013). Fouling organisms may roughen the structures, elevate corrosion and corrosion fatigue and increase maintenance costs (Edyvean, 1987; Yang et al., 2017). On fixed structures in the North Sea, a thickness of up to 40 cm of mussels have been reported (Krone et al., 2013). Moreover, fouling organisms could also spread into the splash zone and potentially cover the photovoltaic surfaces, reducing the light absorption by the panels (Harris et al., 2013). Therefore, the effectiveness of anti-fouling technologies or other environmentally friendly alternatives have been and continue being investigated (Gittens et al., 2013) to be applied on many offshore structures, including floatovoltaics.
In the North Sea, Oceans of Energy (OOE) is currently developing the first offshore solar system in high waves that will cover an area of ~ 6400 m2, equivalent to a capacity of 1MW (https://oceansofenergy.blue/north-sea-2/). From 2020 until 2021, OOE conducted a pilot study (50 kW - https://oceansofenergy.blue/north-sea-1/) at a near-shore location (Brouwersdam, Figure 1) to investigate the functionality of their high wave offshore solar design. Various environmental observations were carried out, such as biodiversity and abundance of birds on the platforms, monitoring of light and water quality parameters underneath the floating structures compared to a reference location, and sampling of the sediment underneath and around the floating structures (Vlaswinkel et al., 2023). Moreover, several different anti-fouling solutions were applied on the submerged parts of the floaters to examine whether there would be differences in fouling community composition (thickness, fouling community composition, etc.). The aim of this study is to describe the fouling communities on the first deployed floaters of floatovoltaics in the North Sea. The fouling community composition was analysed and compared between the different anti-fouling coatings.
Sampling was conducted at 4 floaters of floatovoltaics (NS1-3, NS1-4, NS1-5 and NS1-6) that were placed coastally at approximately 500-1000 meters from the Brouwersdam shore, in a water depth of 4-7 meters in June 2020 and March 2021 (Figure 1; 51.77N and 3.85E). All floatovoltaics had the same floater design (Figure 2), while they had different anti-fouling coatings and installation dates (Table 1).
Figure 2 Image of two floaters placed at the Brouwersdam location in the province of Zeeland, The Netherlands (source OOE).
Fouling organisms were collected by SCUBA divers, who used a putty knife, a quadrat and a sampling net (mesh size 0.5 mm) to scrape off and collect samples with a surface of 0.05 m2 from the underwater part of the floatovoltaics (Figure 3). In total, 3 scrape samples were randomly collected from every floatovoltaic per sampling event. Each scrape sample was placed in a separate numbered net by the divers. All fouling organisms collected were carefully flushed with seawater from the sampling nets into plastic containers (2.5 – 5 L) containing ethanol (99.9%). The ethanol was renewed 24 h later to assure the preservation of the fouling organisms until further analysis. The fouling communities sampled had different ages (17 - 428 d), depending on the day of installation of the floaters (Table 1).
Figure 3 Images of the sampling method used. (A) shows the placement of the quadrat that was used to collect samples from a known surface area and (B) is a sampling net, containing a sample that is numbered as number 6 (source: Oscar Bos/Wageningen Marine Research).
In the laboratory, the samples from the containers were sieved through a 212 µm sieve and sorted into large taxonomic groups. Taxa were then identified by taxonomists to the lowest taxonomic level possible under a stereomicroscope. The World Register of Marine Species (https://www.marinespecies.org/) was used to extract the accepted scientific names of the taxa sampled. Species were considered indigenous or non-indigenous based on the Dutch North Sea species list (Bos et al., 2017). For taxa that were not identified to the species level, it was not possible to be defined as indigenous or non-indigenous. All countable individuals belonging to the same taxon were counted. On the contrary, the total colony surface area of colonial species was registered per sample, to the nearest cm2. For colonies that expand vertically below the floatovoltaics, this means that their surface area could expand the 10,000 cm2 m-2. Finally, the biomass of the different taxa found in every sample was measured (wet weight - WW in g).
Data deriving from this study were stored in a single dataset. Colonial taxa were included in the analysis as cm2 coverage instead of number of individuals m-2. When individuals were identified to a taxonomic level above the species-level in a single sample, they were combined with the species that belongs to the same taxon, when only one species from that taxon occurred in that same sample. When more than one species belonging to this taxon were present in the same sample, the abundance of the higher taxon was proportionally split among the identified taxa. When no species of the same taxon were collected in the same sample, then organisms identified to a higher level remained at the higher level in the dataset. Differences in species richness, numbers of individuals m-2 and biomass (WW in g) m-2 between the different floatovoltaics were analysed with a PERMANOVA (9999 permutations, Bray-Curtis similarity index – Bray and Curtis, 1957). The PERMANOVA was performed on the untransformed data using the adonis2 function from the vegan package (Oksanen et al., 2022). Differences in community composition between the four sampled floatovoltaics coated with different anti-fouling were visualized using non-metric multi-dimensional scaling (MDS, using Bray-Curtis similarity index and 2 dimensions) by applying the metaMDS function (vegan package).
A Generalized Additive model (GAM) was used to model species richness using the date of sampling as a smoothed variable to identify potential succession of the community composition. GAMs are non-parametric generalizations of multiple linear regressions and have less limitations regarding the underlying data distribution (Hastie and Tibshirani, 1990). The GAM model was created using the gam function of the mgcv R-package (Wood, 2011). The model used the Poisson distribution with log link, included coating type as a factor variable and the number of knots for the smoother age was set to 5. The residuals of each model were plotted against the fitted values and inspected to confirm assumptions of homogeneity of variance and normality were met. Model fit was assessed by plotting residuals versus all variables in the model as well as fitted values. All data analyses were performed using R version 4.2.1 (R Core Team, 2022) and Rstudio version 2022.07.0 (Posit Team, 2023).
Most floatovoltaics were fully colonised by fouling fauna (Figures 4, 5; Table S1). Algae, hydrozoans, amphipods and bryozoans were the first colonisers, while more species occupied the available habitat with time. In total, 72 taxa were recorded on all the floatovoltaics, with coating A (Intersleek) being colonised by the lowest number of taxa (26) and coating C (Finsulate) by the highest number of taxa (43- Table 2). B (GreenPowerNano PPDura) and D (Pato) coated floaters were colonised by 38 and 39 different taxa, respectively. Ten taxa occurred at every floatovoltaic floater: the New Zealand barnacle Austrominius modestus, the Japanese skeleton shrimp Caprella mutica, the encrusting bryozoan species Conopeum reticulum and hairy sea-mat Electra pilosa, the amphipods Jassa marmorata and Monocorophium acherusicum, the blue mussel Mytilus edulis, the doubletoothed hydroid Obelia bidentata, the polychaete worm Platynereis dumerilii and organisms belonging to the infraorder Brachyura. Obelia bidentata covered the largest surface area of the floater coated with B (Finsulate) (17,427 cm2), while M. edulis was the second most abundant species on the B coated floater (13,390 ind. m-2) and the most abundant on the D (Pato) (13,940 ind. m-2) and B (GreenPowerNano PPDura) (7,573 ind. m-2) coated floaters (Table 2). Six plant taxa were also recorded on the floatovoltaics, while they were mainly occurring at the edges of the floaters, where more light was available.
Figure 4 Image of the underwater part of the floatovoltaics completely colonised by fouling fauna, and especially mussels of the species Mytilus edulis (source: Oscar Bos/Wageningen Marine Research).
Figure 5 Fouling communities as they were found and sampled during the first sampling event from the different floaters: (A) Intersleek – 17 days after installation; (B) GreenPowerNano PPDura – 78 days after installation; (C) Finsulate – 144 days after installation; (D) Pato – 144 days after installation.
Table 2 Mean numbers (or mean surface area covered by colonial species) per taxon m-2 ± standard error (shown as NA for single sample observations) rounded to zero decimals collected from the floatovoltaics with different anti-fouling coatings/techniques: A (In: Intersleek), B (GPN: GreenPowerNano PPDura), C (Fi: Finsulate) and D (Pa: Pato).
Eight taxa were found to be non-indigenous for the North Sea (Table 2), four of which (A. modestus, C. mutica, J. marmorata, and M. acherusicum) occurred in all the floatovoltaics under study.
The number of individuals m-2 (and, respectively, for colonial species the surface area of their bodies per m2 – Figure 6A), WW m-2 (Figure 6B) and species richness (Figure 6C) per sample varied between the floatovoltaics with different anti-fouling coatings, but these differences were not significant (p values >0.05). The MDS analysis revealed that the fouling communities are divided into a younger (up to 144 days after installation) and an older (from 299 up to 426 days after deployment) community (Figure 7). Furthermore, the fouling communities on coating A remarkably differed from those on the other floaters (coatings B, C and D).
Figure 6 Differences between samples collected from the four floatovoltaics with the different anti-fouling coatings on: (A) the number of individuals per m2 (or body surface area for colonial species), (B) wet weight (WW) per m2 and (C) species richness. The boxes represent 50% of the data, the horizontal lines in the boxes are the median values, while the lower and upper lines (whiskers) represent the 95th percentile, which contain 95% of all the data. The dots show data outliers.
Figure 7 Multidimensional scaling analysis, with which it is visible that the fouling communities on the floatovoltaics can be divided into young (up to 144 days after installation) and old (from 299 up to 426 days after deployment).
The GAM on richness explained 77.8% of the variation in the response variables, the wet weight GAM explained 89% and the abundance GAM explained 72%. The GAM output indicated that species richness per sample varies with time (p<0.05), with a peak at around 180 days after deployment, while it seemed to increase again 400 days after the introduction (Figure 8A). However, variation in richness was high and, in particular around the peak, the prediction errors were very large. Wet weight increased with time since deployment (p<0.05 – Figure 8B), while the number of individuals decreased with time (p<0.05 – Figure 8C), indicating an increase in the weight of the average individual with time.
Figure 8 Generalised additive model output on: (A) species richness per sample with time, (B) wet weight (g) per sample with time and (C) number of individuals per sample with time.
This study explored for the first time the community composition of fouling fauna colonising floaters of floatovoltaics in the coastal Dutch North Sea. The communities developed through time, with younger assemblages (up to 144 days after installation) differing from the older ones, while species richness, wet weight and number of individuals per sample significantly varied with time. Therefore, we are certain that the communities had not reached their final successional stage at the time of the last sampling event. This has been observed with fouling communities on artificial habitats in the southern North Sea, which may not reach a permanent stable climax even 11 years after the installation of the artificial hard substrates (Zupan et al., 2023). The simultaneous decrease of number of individuals per sample and increase of wet weight per sample indicates a general increase in the weight of the average individual with time. As individuals become larger in size, they need more space, outcompeting other attached individuals or dominating against potentially new colonisers. This could be the first step towards a stable fouling community. However, whether this could be developed on floatovoltaics, which are installed in highly dynamic offshore environments and are prone to bad weather conditions, is yet to be examined. Finally, the anti-fouling coating A (Intersleek) seemed to function effectively against the colonisation of fouling fauna, while the coating C (Finsulate) was considered the most inefficient to prevent fouling fauna from attaching to the surface. However, no significant differences in species richness, number of individuals m-2 and WW m-2 were observed between the different types of antifouling coatings, likely due to the low number of samples per floater/anti-fouling technique.
The first colonisers included algae, hydrozoans, bryozoans and amphipods, while at a later stage the hydroid Obelia bidentata and the blue mussel Mytilus edulis were the most abundant species on all the floaters. Mytilus edulis has also been observed to dominate floating structures in the Swedish west coast (Langhamer et al., 2009), in Orkney waters and the Pentland Firth (Want et al., 2017). Similarly, hydroids are also considered dominant (in surface coverage degree) epibenthic organisms on floating structures (Langhamer et al., 2009). On the contrary, sea squirts (Ascidiaceae) and calcareous serpulid worms (Spirobranchus triqueter) were not dominantly present in our samples, while they were found in great abundances in other floating structures (Moate, 1985; Langhamer et al., 2009). The abundant presence of S. triqueter might also contribute to the colonisation of new hard substrates by fouling fauna (Moate, 1985), but this has not been observed in our study, since this species occurred only on two floaters and it was not abundantly present.
Early colonisers occupy most of the available habitats and might prevent other species from establishing on the newly introduced structures by predating on their larvae, a process named inhibitory response (Coolen et al., 2022). On the contrary, other early colonisers might facilitate the attachment of other fouling species (see below). The season of larval release in combination with the time of installation of artificial structures are crucial for the establishment of organisms on the available habitats (Kerckhof et al., 2010). The A coated floater was deployed in late spring 2020, while the other three floaters were installed earlier in the year. Therefore, the planktonic larvae of species that reproduce earlier in the year had likely already disappeared from the water column when the A coated floater was deployed. On the contrary, species that breed and settle later in the year may have benefitted from the lack of competition for the available space and quickly colonised this floater. For example, the hydrozoan Obelia bidentata produces medusae that can be found in May and June in the North Sea (Cornelius, 1995). This species can attach to a wide variety of substrates but it shows preference for areas with water movement and low light conditions (Gili and Hughes, 1995), which suggests that the floaters of floatovoltaics are the ideal settlement habitat for this species. The late installation of the A coated floater also explains why this was the only floater that was sampled in June 2020 that was not colonised by the blue mussel Mytilus edulis. Mytilus edulis larvae primarily settle on filamentous red and green algae and/or hydrozoans (Bayne, 1964; Dobretsov and Wahl, 2001). Thus, the relatively bare habitat of the Intersleek-coated floater was not ideal for mussel colonisation during the first few weeks after installation. However, during the second sampling event, mussel larvae have had the time and the appropriate habitat (provided by the initial colonisation of the floater by O. bidentata) to colonised the A coated floater, as well. Individuals of M. edulis were observed to create grape-vine clumps hanging from the hydroids, exploiting the entire surface area of these organisms as available habitat for colonisation. Therefore, it is suggested that O. bidentata could potentially provide secondary habitat for colonisation.
Another potential reason for the low colonisation observed on the A coated floater could be the initial absence of M. edulis from its surface. The shells of M. edulis create secondary hard substrates that increase habitat heterogeneity and, subsequently, lead to increased species richness (Krone et al., 2013; Coolen et al., 2020). Indeed, the presence of M. edulis has been mainly linked to high species diversity (Norling and Kautsky, 2007). However, in some cases, this species might monopolise the habitats, contributing to a high biomass of fouling assemblages on offshore artificial structures, a phenomenon known as “Mytilusation” (Krone et al., 2013). The shell drop-off from mussels attached on renewable energy devices or suspended mussel aquacultures also creates secondary habitat on the seafloor and attracts multiple epifauna species (Krone et al., 2013; Wilding and Nickell, 2013). This suggests that the seafloor underneath floatovoltaic farms could also be enriched with shells. First sedimentary observations underneath the same floatovoltaic systems did not reveal an organic matter enrichment (Vlaswinkel et al., 2023), but possibly this could happen after longer periods of time, underneath larger farms, or at other, less dynamic locations. A potentially increased habitat availability due to mussel shells could eventually lead to enhanced local benthic biodiversity, since a variety of species would find the ideal conditions (habitat- and food-wise) to inhabit.
Numerous studies have indicated that marine sprawl (including marine renewable energy devices) supports the settlement (and potentially also the reproduction) of non-indigenous species, acting as stepping stones promoting their success (Vaselli et al., 2008; Airoldi et al., 2015; De Mesel et al., 2015; Leclerc et al., 2020; Taormina et al., 2020). In this study, approx. 11% of the identified taxa occurring on the floaters of floatovoltaics were characterised as non-indigenous for the southern North Sea. This percentage is higher than the one found on a 3-year-old floating wave energy device in Scotland (< 5%) (Nall et al., 2017), but much lower than that found on buoys on Belgian coastal waters, where 9 out of the 11 identified species were considered non-indigenous (Kerckhof and Cattrijsse, 2001). These differences could be explained by the variation of environmental conditions, differences in the structure materials and/or the period of deployment and sample collection. The wave energy device was placed in the Northern North Sea, a location which experiences high waves from both the north-west and the south-east directions and are able to propagate in the area (Lawrence et al., 2009). This could have implications on the success of settlement of many species, including the non-indigenous ones. The high percentage of non-indigenous species on the Belgian buoys could be explained by the difference time of sampling after installation, since these buoys were sampled between 4 and 24 months after deployment (Kerckhof and Cattrijsse, 2001). Communities evolve with time, a process that may last more than a decade (Zupan et al., 2023). It is, thus, possible that the floaters of the floatovoltaics could also sustain a larger amount of (non-indigenous) species multiple months/years after installation.
Some of the main non-indigenous species (i.e. Caprella mutica and Austrominius modestus) that occurred on the floaters have also been observed on navigational buoys in UK waters (Macleod, 2013). Furthermore, we observed similarities on the composition of non-indigenous species between the floaters and Belgian offshore wind turbine foundations (e.g. the amphipod Jassa marmorata, the splash midge Telmatogeton japonicus and the New Zealand barnacle Austrominius modestus) (De Mesel et al., 2015). Surprisingly, none of the non-indigenous species occurring on the floaters were found on the buoys sampled from the Belgian coastal waters, where mainly species of the genera Balanus and Megabalanus colonised the floating structures (Kerckhof and Cattrijsse, 2001). The non-indigenous species J. marmorata, T. japonicus and A. modestus occurred on the floaters within the first year after the installation, while some of them were the most abundant species on these newly introduced habitats. It is, therefore, possible that offshore solar energy installations could function as stepping stones for the increased distribution of these species. Finally, some coastal non-indigenous species were present on the floaters, e.g. Caprella mutica, indicating that the location of the introduced artificial structures plays a significant role on the community composition and on the species settlement and may thrive on these structures (Coolen et al., 2016).
To conclude, this study analysed the community composition of fouling fauna on floaters of the first floatovoltaics in the coastal Dutch North Sea. It was indicated that the fouling organisms comprised of a typical coastal community, with known non-invasive species for the region. The variability of richness, number of individuals and wet weight per sample with time indicated that the fouling communities present on the underside of the floaters are still developing. At the same time, individuals were gradually becoming larger in size, since the number of individuals per sample decreased, while the wet weight increased. The floatovoltaics have already been transferred to offshore locations in the Dutch North Sea and, in the future, they might be located within offshore wind farm areas. Therefore, long-term monitoring of fouling fauna on floatovoltaics is necessary as it could facilitate the design of floating systems (i.e. relating with the weight of the fauna to colonise the new surfaces) and provide us with significant information as to how the deployment of floatovoltaics, their co-location with offshore wind farms and the subsequent colonisation of these man-made surfaces by fouling organisms could affect the wider marine environment.
The original contributions presented in the study are included in the article/Supplementary Material. Further inquiries can be directed to the corresponding authors.
The manuscript presents research on animals that do not require ethical approval for their study.
NM wrote the first version of the manuscript and created the final submitted version. OGB, BV and PR wrote sections of the manuscript and OGB performed part of the sampling. JC wrote sections of the manuscript, performed the data analysis, conducted the sampling and got the funding for this research. OB, WG, BW performed the laboratory work. All authors read and approved the submitted version.
The author(s) declare financial support was received for the research, authorship, and/or publication of this article. This research project was carried out by Wageningen Marine Research and partly subsidized by the ministry of Agriculture, Nature and Food Quality for the purposes of Knowledge Base Research Theme: Project ‘Solar at sea’ (project no. KB36-003-034). The researchers were further supported by an RVO grant (Rijksdienst voor Ondernemend Nederland) under the project title ‘North Sea Two Offshore Solar Project’.
We would like to acknowledge Oceans of Energy and the North Sea 1 project for the collaboration and for allowing us to collect samples from their installations. We would also like to thank the three reviewers who provided insightful comments that improved the manuscript considerably.
The authors declare that the research was conducted in the absence of any commercial or financial relationships that could be construed as a potential conflict of interest.
All claims expressed in this article are solely those of the authors and do not necessarily represent those of their affiliated organizations, or those of the publisher, the editors and the reviewers. Any product that may be evaluated in this article, or claim that may be made by its manufacturer, is not guaranteed or endorsed by the publisher.
The Supplementary Material for this article can be found online at: https://www.frontiersin.org/articles/10.3389/fmars.2023.1223766/full#supplementary-material
Airoldi L., Turon X., Perkol-Finkel S., Rius M. (2015). Corridors for aliens but not for natives: Effects of marine urban sprawl at a regional scale. Divers. Distrib 21, 755–768. doi: 10.1111/ddi.12301
Bayne B. L. (1964) Primary and Secondary Settlement in Mytilus edulis L. (Mollusca). Available at: https://www.jstor.org/stable/2569?seq=1&cid=pdf-reference#references_tab_contents.
Bos O. G., Gittenberger A., De Boois I. J., de, van Asch J. T., van der Wal J., Cremer B. H., et al. (2017). Soortenlijst Nederlandse Noordzee (Den Helder: Wageningen University & Research Rapport C125/16A), 100.
Bray J. R., Curtis J. T. (1957). An ordination of the upland forest communities of southern Wisconsin. Ecol. Monogr. 27, 326–349. doi: 10.2307/1942268
Coolen J. W. P., Lengkeek W., Degraer S., Kerckhof F., Kirkwood R. J., Lindeboom H. J. (2016). Distribution of the invasive Caprella mutica Schurin 1935 and native Caprella linearis (Linnaeus 1767) on artificial hard substrates in the North Sea: Separation by habitat. Aquat Invasions 11, 437–449. doi: 10.3391/ai.2016.11.4.08
Coolen J. W. P., Vanaverbeke J., Dannheim J., Garcia C., Birchenough S., Krone R., et al. (2022). Generalized changes of benthic communities after construction of wind farms in the southern North Sea. J. Environ. Manage 315, 115173. doi: 10.1016/j.jenvman.2022.115173
Coolen J. W. P., van der Weide B., Cuperus J., Blomberg M., van Moorsel G. W. N. M., Faasse M. A., et al. (2020). Benthic biodiversity on old platforms, young wind farms, and rocky reefs. ICES J. Mar. Sci. 77, 1250–1265. doi: 10.1093/icesjms/fsy092
Cornelius P. F. S. (1995). North-west European thecate hydroids and their medusae. Parts 1 and 2. Synopses of the British Fauna, New Series 50: 1-347 and 1-386.
De Mesel I., Kerckhof F., Norro A., Rumes B., Degraer S. (2015). Succession and seasonal dynamics of the epifauna community on offshore wind farm foundations and their role as stepping stones for non-indigenous species. Hydrobiologia 756, 37–50. doi: 10.1007/s10750-014-2157-1
Dobretsov S., Wahl M. (2001). Recruitment preferences of blue mussel spat (Mytilus edulis) for different substrata and microhabitats in the White Sea (Russia). Hydrobiologia 445, 27–35. doi: 10.1023/A:1017502126707
Edyvean R. G. J. (1987). Biodeterioration problems of North Sea oil and gas production - A review. Int. Biodeterior 23, 199–231. doi: 10.1016/0265-3036(87)90002-9
Exley G., Armstrong A., Page T., Jones I. D. (2021). Floating photovoltaics could mitigate climate change impacts on water body temperature and stratification. Solar Energy 219, 24–33. doi: 10.1016/j.solener.2021.01.076
Figueroa N. N., Brante A., Viard F., Leclerc J. C. (2021). Greater functional similarity in mobile compared to sessile assemblages colonizing artificial coastal habitats. Mar. Pollut. Bull. 172, 112844. doi: 10.1016/j.marpolbul.2021.112844
Giachetti C. B., Battini N., Castro K. L., Schwindt E. (2020). Invasive ascidians: How predators reduce their dominance in artificial structures in cold temperate areas. J. Exp. Mar. Biol. Ecol. 533, 151459. doi: 10.1016/j.jembe.2020.151459
Gili J. M., Hughes R. G. (1995). The ecology of marine benthic hydroids. Oceanography and marine biology: an annual review. Oceanograph. Literat. Rev. 12, 1250.
Gittens J. E., Smith T. J., Suleiman R., Akid R. (2013). Current and emerging environmentally-friendly systems for fouling control in the marine environment. Biotechnol. Adv. 31, 1738–1753. doi: 10.1016/j.bioteChadv.2013.09.002
GWEC. (2022). “Global Wind Energy Council” report. (Brussels, Belgium: Rue de Commerce 31), pp. 154.
Harris L., Tozzi S., Wiley P., Young C., Richardson T. M. J., Clark K., et al. (2013). Potential impact of biofouling on the photobioreactors of the Offshore Membrane Enclosures for Growing Algae (OMEGA) system. Bioresour Technol. 144, 420–428. doi: 10.1016/j.biortech.2013.06.125
Holloway M. G., Connell S. D. (2002). Why do floating structures create novel habitats for subtidal epibiota? Mar. Ecol. Prog. Ser. 235, 43–52. doi: 10.3354/meps235043
Hooper T., Armstrong A., Vlaswinkel B. (2021). Environmental impacts and benefits of marine floating solar. Solar Energy 219, 11–14. doi: 10.1016/j.solener.2020.10.010
Karpouzoglou T., Vlaswinkel B., van der Molen J. (2020). Effects of large-scale floating (solar photovoltaic) platforms on hydrodynamics and primary production in a coastal sea from a water column model. Ocean Sci. 16, 195–208. doi: 10.5194/os-16-195-2020
Kerckhof F., Cattrijsse A. (2001). Exotic Cirripedia (Balanomorpha) from buoys off the Belgian coast. Senckenbergiana maritima 31, 245–254. doi: 10.1007/BF03043033
Kerckhof F., Rumes B., Jacques T., Degraer S., Norro A. (2010). Early development of the subtidal marine biofouling on a concrete offshore windmill foundation on the Thornton Bank (southern North Sea): First monitoring results. Underwater Technol. 29, 137–149. doi: 10.3723/ut.29.137
Krone R., Gutow L., Joschko T. J., Schröder A. (2013). Epifauna dynamics at an offshore foundation - Implications of future wind power farming in the North Sea. Mar. Environ. Res. 85, 1–12. doi: 10.1016/j.marenvres.2012.12.004
Langhamer O., Wilhelmsson D., Engström J. (2009). Artificial effect and fouling impacts on offshore wave power foundations and buoys – a pilot study. Estuar. Coast. Shelf Sci. 82, 426–432. doi: 10.1016/j.ecss.2009.02.009
Lawrence J., Kofoed-Hansen H., Chevalier C. (2009). “High-resolution metocean modelling at EMEC’s (UK) marine energy test sites,” in Proceedings of the 8th European Wave and Tidal Energy Conference. (Upsala, Sweden).
Leclerc J. C., Viard F., González Sepúlveda E., Díaz C., Neira Hinojosa J., Pérez Araneda K., et al. (2020). Habitat type drives the distribution of non-indigenous species in fouling communities regardless of associated maritime traffic. Divers. Distrib 26, 62–75. doi: 10.1111/ddi.12997
Macleod A. K. (2013). The role of marine renewable energy structures and biofouling communities in promoting self-sustaining populations of non-native species (Aberdeen University: Doctoral dissertation).
Moate R. (1985). Offshore fouling communities and settlement and early growth in Tubularia larynx (Ellis and Solander) and Pomatoceros triqueter (L.).
Nall C. R., Schläppy M. L., Guerin A. J. (2017). Characterisation of the biofouling community on a floating wave energy device. Biofouling 33, 379–396. doi: 10.1080/08927014.2017.1317755
Norling P., Kautsky N. (2007). Structural and functional effects of Mytilus edulis on diversity of associated species and ecosystem functioning. Mar. Ecol. Prog. Ser. 351, 163–175. doi: 10.3354/meps07033
Oksanen J., Simpson G. L., Blanchet F. G., Kindt R., Legendre P., Minchin P. M., et al. (2022). _vegan: Community Ecology Package_. R package version 2.6-2. Available at: https://CRAN.R-project.org/package=vegan.
Oliveira-Pinto S., Rosa-Santos P., Taveira-Pinto F. (2020). Assessment of the potential of combining wave and solar energy resources to power supply worldwide offshore oil and gas platforms. Energy Convers Manag 223, 113299. doi: 10.1016/j.enconman.2020.113299
Pimentel Da Silva G. D., Branco D. A. C. (2018). Is floating photovoltaic better than conventional photovoltaic? Assessing environmental impacts. Impact Assess. Proj Apprais 36, 390–400. doi: 10.1080/14615517.2018.1477498
Posit Team. (2023). RStudio: Integrated Development Environment for R. (Boston, MA: Posit Software, PBC). Available at: http://www.posit.co/.
R Core Team. (2022). R: A language and environment for statistical computing (Vienna, Austria: R Foundation for Statistical Computing). Available at: https://www.R-project.org.
Redón Santafé M., Torregrosa Soler J. B., Sánchez Romero F. J., Ferrer Gisbert P. S., Ferrán Gozálvez J. J., Ferrer Gisbert C. M. (2014). Theoretical and experimental analysis of a floating photovoltaic cover for water irrigation reservoirs. Energy 67, 246–255. doi: 10.1016/j.energy.2014.01.083
Rosa-Clot M., Tina G. M., Nizetic S. (2017). “Floating photovoltaic plants and wastewater basins: An Australian project,” in Energy Procedia (Elsevier Ltd) 134, 664–674. doi: 10.1016/j.egypro.2017.09.585
Sahu A., Yadav N., Sudhakar K. (2016). Floating photovoltaic power plant: A review. Renewable Sustain. Energy Rev. 66, 815–824. doi: 10.1016/j.rser.2016.08.051
SERIS (2019). Where Sun meets Water. Floating Solar Market Report. Prepared by the Solar Energy Research Institute of Singapore (SERIS) at the National University of Singapore (NUS), under contract from the World Bank (Washington DC, USA: The World Bank). Available at: https://openknowledge.worldbank.org/handle/10986/31880.
Taormina B., Percheron A., Marzloff M. P., Caisey X., Quillien N., Lejart M., et al. (2020). Succession in epibenhtic communities on artificial reefs associated with marine renewable energy facilities within a tide-swept environment. ICES J. Mar. Sci. 77, 2656–2668. doi: 10.1093/icesjms/fsaa129
Vaselli S., Bulleri F., Benedetti-Cecchi L. (2008). Hard coastal-defence structures as habitats for native and exotic rocky-bottom species. Mar. Environ. Res. 66, 395–403. doi: 10.1016/j.marenvres.2008.06.002
Vlaswinkel B., Roos P., Nelissen M. (2023). Environmental observations at the first offshore solar farm in the North Sea. Sustainability 15, 6533. doi: 10.3390/su15086533
Want A., Crawford R., Kakkonen J., Kiddie G., Miller S., Harris R. E., et al. (2017). Biodiversity characterisation and hydrodynamic consequences of marine fouling communities on marine renewable energy infrastructure in the Orkney Islands Archipelago, Scotland, UK. Biofouling 33, 567–579. doi: 10.1080/08927014.2017.1336229
Wilding T. A., Nickell T. D. (2013). Changes in benthos associated with mussel (Mytilus edulis L.) farms on the West-Coast of Scotland. PloS One 8, e68313. doi: 10.1371/journal.pone.0068313
Wood S. N. (2011). Fast stable restricted maximum likelihood and marginal likelihood estimation of semiparametric generalized linear models. J. R Stat. Soc. Ser. B 73, 3–36. doi: 10.1111/j.1467-9868.2010.00749.x
Yang S. H., Ringsberg J. W., Johnson E., Hu Z. (2017). Biofouling on mooring lines and power cables used in wave energy converter systems—Analysis of fatigue life and energy performance. Appl. Ocean Res. 65, 166–177. doi: 10.1016/j.apor.2017.04.002
Keywords: renewable energy, floating photovoltaics, biofouling, community composition, species richness, anti-fouling coating
Citation: Mavraki N, Bos OG, Vlaswinkel BM, Roos P, de Groot W, van der Weide B, Bittner O and Coolen JWP (2023) Fouling community composition on a pilot floating solar-energy installation in the coastal Dutch North Sea. Front. Mar. Sci. 10:1223766. doi: 10.3389/fmars.2023.1223766
Received: 01 June 2023; Accepted: 11 December 2023;
Published: 28 December 2023.
Edited by:
Laurent Urios, Université de Pau et des Pays de l’Adour, FranceReviewed by:
Bob Rumes, Royal Belgian Institute of Natural Sciences, BelgiumCopyright © 2023 Mavraki, Bos, Vlaswinkel, Roos, de Groot, van der Weide, Bittner and Coolen. This is an open-access article distributed under the terms of the Creative Commons Attribution License (CC BY). The use, distribution or reproduction in other forums is permitted, provided the original author(s) and the copyright owner(s) are credited and that the original publication in this journal is cited, in accordance with accepted academic practice. No use, distribution or reproduction is permitted which does not comply with these terms.
*Correspondence: Ninon Mavraki, bmlub24ubWF2cmFraUB3dXIubmw=; Joop W. P. Coolen, am9vcC5jb29sZW5Ad3VyLm5s
†Present address: Pauline Roos, Eneco, Rotterdam, Netherlands
Disclaimer: All claims expressed in this article are solely those of the authors and do not necessarily represent those of their affiliated organizations, or those of the publisher, the editors and the reviewers. Any product that may be evaluated in this article or claim that may be made by its manufacturer is not guaranteed or endorsed by the publisher.
Research integrity at Frontiers
Learn more about the work of our research integrity team to safeguard the quality of each article we publish.