- 1Marine Chemistry and Biochemistry, The Institute of Oceanology of the Polish Academy of Sciences, Sopot, Poland
- 2Geochemistry and Isotope Biogeochemistry, Leibniz Institute for Baltic Sea Research, Rostock, Germany
- 3Marine Geochemistry, University of Greifswald, Greifswald, Germany
- 4Interdisciplinary Faculty, University of Rostock, Rostock, Germany
- 5Physical Oceanography, The Institute of Oceanology of the Polish Academy of Sciences, Sopot, Poland
Submarine groundwater discharge (SGD) can be a significant source of dissolved nutrients, inorganic and organic carbon, and trace metals in the ocean and therefore can be a driver for the benthic-pelagic coupling. However, the influence of hypoxic or anoxic SGD on the carbonate system of coastal seawater is still poorly understood. In the present study, the production of dissolved inorganic carbon (DIC) and alkalinity (AT) in coastal sediments has been investigated under the impact of oxygen-deficient SGD and was estimated based on the offset between the measured data and the conservative mixing of the end members. Production of AT and DIC was primarily caused by denitrification and sulphate reduction. The AT and DIC concentrations in SGD decreased by approximately 32% and 37% mainly due to mixing with seawater counterbalanced by reoxidation and CO2 release into the atmosphere. Total SGD-AT and SGD-DIC fluxes ranged from 0.1 to 0.2mol m-2 d-1 and from 0.2 to 0.3mol m-2 d-1, respectively. These fluxes are probably the reason why the seawater in the Bay of Puck is enriched in AT and DIC compared to the open waters of the Baltic Sea. Additionally, SGD had low pH and was undersaturated with respect to the forms of the aragonite and calcite minerals of CaCO3. The seawater of the Bay of Puck also turned out to be undersaturated in summer (Inner Bay) and fall (Outer Bay). We hypothesize that SGD can potentially contribute to ocean acidification and affect the functioning of the calcifying invertebrates.
1 Introduction
SGD is defined as any flow of water from the seabed to the ocean, regardless of the composition of the fluid or the driving force (Burnett et al., 2003; Taniguchi et al., 2019). It occurs in all permeable coastal aquifers; both unconfined and confined (Johannes, 1980). SGD includes meteoric and recirculated seawater; therefore, it contains water of any salinity (Taniguchi et al., 2019). Usually, the fresh component of SGD is significantly smaller than that of saline (Santos et al., 2009a; Luijendijk et al., 2020). Coastal SGD is mainly affected by waves, tides, currents and bioirrigation, while deeper SGD is often influenced by hydraulic gradients, convection, and tidal pumping (Santos et al., 2009a; Santos et al., 2009b; Arévalo-Martínez et al., 2022). Although global SGD rate assessments are difficult to establish, it is estimated that the magnitude of fresh SGD in the ocean is between 1 and 10% of annual river discharge (Burnett et al., 2003; Taniguchi et al., 2019). Moore (1996) determined that SGD, mainly saline SGD, to the ocean shelf must be about 40% of the river flux to the same area. In the Baltic Sea, fresh SGD represents ~4% of river runoff (Peltonen, 2002). However, total (fresh and saline - driven by seawater recirculation on varying timescales) SGD can be significantly higher. Kłostowska et al. (2020) indicated that the total SGD to the Bay of Puck, southern Baltic Sea, is two orders of magnitude higher than fresh SGD. Additionally, SGD ranged from 5 to 25 times more than river discharge in the area (Kłostowska et al., 2020).
Although SGD has been identified and quantified in many regions of the world, it is still necessary to improve our understanding of the proportion of the saline component of SGD; its spatial and temporal variability (Taniguchi et al., 2019; Santos et al., 2021); and the impact of biogeochemical conditions occurring in the subterranean estuary (STE - zone where groundwater mixes with seawater; Moore, 1999) on SGD composition (Taniguchi et al., 2019; Moosdorf et al., 2021). Groundwater and seawater mixing zones are strongly influenced by groundwater flow rates and the redox gradient between SGD and seawater (Slomp and Van Cappellen, 2004). Additionally, the steep biogeochemical gradient at the water-sediment interface where anoxic SGD meets oxic seawater can significantly modulate the composition of the discharged solution (Charette et al., 2001; Charette and Sholkovitz, 2002; Testa et al., 2002; Slomp and Van Cappellen, 2004; Donis et al., 2017).
In many parts of the world, SGD contributes to a significant loading of nutrients on coastlines (Valiela et al., 2002; Wang et al., 2015; Zhang et al., 2017; Taniguchi et al., 2019). For example, Cho et al. (2018) determined that SGD and the accompanying flux of dissolved inorganic nitrogen, phosphorus, and silica (DSi) are comparable to river inputs on a global scale. Rahman et al. (2019) projected that the load of DSi via saline SGD accounts for an increase of 25-30% in global estimates of net DSi to the ocean. The high concentration of nutrients in SGD is generally caused by the leakage of nitrogen and phosphorus to aquifers from agriculture or sewage plants (Bishop et al., 2007) and the decomposition of organic matter (OM) (Cai et al., 2003; Liu et al., 2012; Liu et al., 2014; Wang et al., 2018). As groundwater is in contact with sediments for a long period, during this time, the particulate organic matter (POM) in the sediments is decomposed (during recharge and in STE), releasing nutrients, DIC, and dissolved organic carbon (DOC) (Cai et al., 2003; Liu et al., 2012; Liu et al., 2014; Wang et al., 2018). Further degradation of DOC causes an additional increase in DIC and CO2. Consequently, SGD could make the receiving coastal seawater a source of CO2 for the atmosphere. On the other hand, the supply of nutrients derived from SGD can enhance primary productivity in coastal waters, which would reduce surface water pCO2 (Wang et al., 2018). Therefore, SGD can potentially have wide-reaching consequences for the coastal marine environment, such as the development of coastal eutrophication; hypoxia/anoxia of bottom waters; the enhancement or buffering of ocean acidification (OA); positive or negative influence on primary productivity and the composition, activity and maintenance of the benthic community (Zeebe and Wolf-Gladrow, 2001; Slomp and Van Cappellen, 2004; Zipperle and Reise, 2005; Knee and Paytan, 2011; Kotwicki et al., 2014; Wang et al., 2014; Winde et al., 2014; Liu et al., 2017; Middelburg et al., 2020; Moosdorf et al., 2021).
Several studies indicated a large input of AT and DIC through SGD into the ocean (Moore et al., 2011; Liu et al., 2012; Liu et al., 2014; Santos et al., 2015; Sadat-Noori et al., 2018), however, the production of AT and DIC coupled to anaerobic biogeochemical processes is still an unresolved field of study that requires further research. In addition to poor knowledge of the impact of SGD on DIC and AT generation in sediments, there is generally limited understanding of quantitative benthic AT and DIC fluxes from shallow marine environments and the potential importance of coastal sediments for the global carbon cycle and climate (Krumins et al., 2013; Łukawska-Matuszewska and Graca, 2018). In addition, AT generated through anaerobic processes and its release to the water column may be especially important for basins with low buffer capacity.
The Baltic Sea is an example of the sea, which has a low buffer capacity due to low salinity (S) and is believed to be especially vulnerable to CO2 ‐ induced acidification. Recent studies in the Baltic show that surface water AT has increased since the early twentieth century due to potential changes in precipitation patterns, continental weathering, agricultural liming, and internal AT sources (Müller et al., 2016). The composition of Baltic Sea water is strongly affected by the freshwater component, which is well reflected in the brackish character of the basin. This results in a high number of ion anomalies observed here, including those directly influencing the structure of the marine CO2 system and the distribution of AT and DIC (Bełdowski et al., 2010; Kuliński et al., 2018; Kuliński et al., 2022). The regional impact depends on the lithology of the catchment area and the degree of management of the estuaries (e.g., Humborg et al., 2002; Ehlert von Ahn et al., 2023). Gustafsson et al. (2019) indicated assumed that known AT sources do not explain AT concentration in the Baltic Sea. Some of this missing AT input can be attributed to the release from hypoxic and anoxic sediments, or coastal erosion of carbonate-bearing rocks. For example, Łukawska-Matuszewska (2016) calculated that the average diffusive flux of AT from the fine-grained sediments in the southern Baltic Sea was 1397 ± 511μmol m−2d−1. Silberberger et al. (2022) indicated that benthic DIC fluxes in the Bay of Puck (ranged from −2.1 to 110.6mmol C m−2d−1) were partially decoupled from oxygen fluxes and could be a consequence of carbonate dissolution, anaerobic respiration and SGD. The potential influence of SGD on the marine CO2 system in the Baltic Sea and marine benthic calcifying organisms has not been evaluated to date.
Here, we provide data showing that SGD is a significant source of AT and DIC in the Bay of Puck, southern Baltic Sea. We prove that anaerobic OM mineralization causes the production of AT and DIC in STE. We show that groundwater and SGD are enriched in CO2 and hypothesise that SGD-derived CO2 flux results in CO2 release into the atmosphere. Furthermore, we present that SGD has a low pH and is undersaturated with respect to the forms of aragonite and calcite minerals of CaCO3 that can affect the maintenance of the calcifying invertebrates.
2 Materials and methods
2.1 Study area
The Bay of Puck is located in the western part of the Gulf of Gdańsk, the southern Baltic Sea (Figure 1), and is separated from the open Baltic Sea by the Hel Peninsula. It consists of two parts: the Inner and Outer Bay of Puck. The natural sand barrier called Rybitwia Mielizna separates both parts, which differ in terms of depth and morphology. The westernmost end is only about 2 m deep (Inner Bay of Puck), while the entrance to the bay (Outer Bay of Puck) reaches 50 m depth (Nowacki, 1993). The hydrology of the outer part is mainly influenced by the inflow of water from the Gulf of Gdańsk (Nowacki, 1993). The water circulation in the inner part depends on the direction of the wind and the limited exchange of water with the eastern outer part (Nowacki, 1993). Additionally, the inner part receives input from numerous freshwater sources, such as rivers and springs, including the Reda, Płutnica, Gizdepka, and Zagórska Struga rivers, of which the Reda River has the highest runoff (the average runoff equals approximately 5m3 s-1; Szymczak and Piekarek-Jankowska, 2007). Fine-grained sands dominate the sediments of the inner part while in the outer part of the bay; coarse-grained sands to a depth of about 20m predominate, while fine-aurantite sands are the majority in the deepest parts (Piekarek-Jankowska and Łęczyński, 1993).
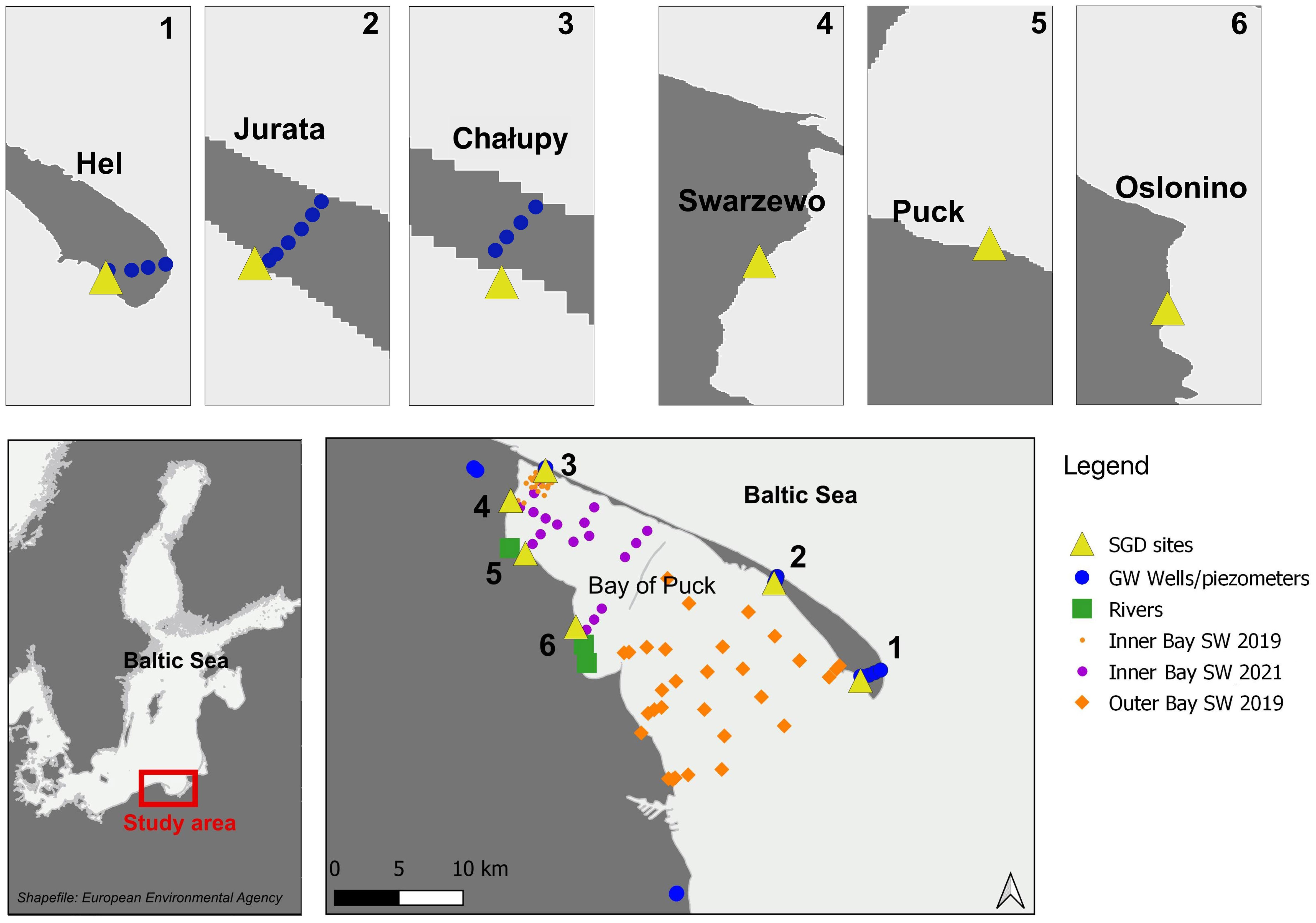
Figure 1 Diagram illustrating the study area. Yellow triangles correspond to sites where SGD has been identified such as Hel, Jurata, Chałupy, Swarzewo, Osłonino and Puck. The investigated area at each site was approximately 50m2. Green squares represent the sites where the rivers were sampled and blue dots represent the sites where groundwater was collected from piezometers and groundwater wells. Purple dots and orange dots correspond to the Inner Bay of Puck stations, while orange rhombus resembles to the Outer Bay of Puck stations, respectively, where surface seawater was collected.
The Bay of Puck is surrounded by a young glacial landscape and consists of isolated fragments of a moraine plateau separated from each other with deeply cut ice marginal valleys (Kramarska et al., 1995; Szymkiewicz et al., 2020). The Bay of Puck region is covered with Quaternary deposits consisting of glacial moraine with layers of sand and gravel and glacial fluvial or river sand and silty sand in the valleys. The dominant soil types are sandy loam (glacial till), sandy loam covered with loamy sand (weathered glacial till), sand (of glacifluvial origin), and peat (in larger river valleys) (Kramarska et al., 1995; Szymkiewicz et al., 2020).
The bay has a complex hydrogeological system that consists of Cretaceous, Tertiary, and Quaternary aquifers (Piekarek-Jankowska, 1994; Piekarek-Jankowska, 1996; Szymkiewicz et al., 2020). Shallow groundwater up to 10 m below the ground level occurs in the form of small-perched aquifers and sand lenses enclosed in moraine deposits. The deeper Quaternary aquifers were shaped in glacial deposits (sand and gravel) separated with a coat of moraine till. Some of the aquifers are hydraulically connected; however, the majority are confined (piezometric head reaches 45m above mean sea level, Szymkiewicz et al., 2020), except for areas in the valleys where the till cover was eroded and the upper aquifer is exposed, unconfined, and indirectly connected with surface waters. Shallow groundwater is recharged by the infiltration of rainwater. Deeper aquifers receive seepage water from the layers above (Piekarek-Jankowska, 1994; Szymkiewicz et al., 2020).
The Bay of Puck was described in several studies as an active groundwater discharging area (Piekarek-Jankowska, 1994; Piekarek-Jankowska, 1996; Szymczycha et al., 2012; Kotwicki et al., 2014; Matciak et al., 2015; Donis et al., 2017; Kłostowska et al., 2020; Szymczycha et al., 2020a; Szymczycha et al., 2020b; Ehlert von Ahn et al., 2023). Groundwater models estimate that fresh groundwater discharge to the Bay of Puck from all aquifers is about 1.1 10-12 L cm-2 s-1 (Piekarek-Jankowska, 1994; Piekarek-Jankowska, 1996). Kłostowska et al. (2020) estimated that the total SGD (fresh and saline) in the Bay of Puck is significantly higher and ranges from 1.8 10-7 to 2.8 10-7L cm-2 s-1. High variability of SGD flux is due to short-time-scale factors (wind direction and monthly precipitation) and long-time-scale factors (total precipitation and large-scale sea-level variations).
Previous studies indicated that fresh anoxic groundwater seepage can influence the coastal marine environment of the Bay of Puck by the discharge of methane, nutrients, and dissolved organic and inorganic carbon, including pharmaceuticals and caffeine (Szymczycha et al., 2012; Kotwicki et al., 2014; Donis et al., 2017; Szymczycha et al., 2020a; Szymczycha et al., 2020b). It should be noted that the methane loads delivered by SGD to the Bay of Puck are believed to be only slightly oxidised within the sediments and have the potential to reach the atmosphere at a maximum rate of 3mmol CH4 m- 2 d- 1 (Kotwicki et al., 2014; Donis et al., 2017).
2.2 Sampling strategy
Six coastal surveys were conducted seasonally from autumn 2017 to summer 2018 in the Bay of Puck, southern Baltic Sea (Hel, Jastarnia, Chałupy, Swarzewo, Puck and Osłonino (Figure 1). Three pore water salinity surveys were repeated in October 2019 (Hel, Chałupy and Swarzewo) and June 2021 (Hel, Chałupy, Swarzewo) (Figure 1). In all coastal surveys, salinity was used as a SGD tracer and the investigated area at each site equaled approximately 50m2. Additionally, two surface seawater surveys were also conducted: on r/v Oceania in the Outer Bay of Puck in October 2019 and with s/y Świrek in the Inner Bay of Puck in June 2021.
Surface seawater from the Outer Bay was collected with the pumping system installed on r/v Oceania and having the water inlet at the 2.5m depth, while in the Inner Bay of Puck, the water samples were taken using a Niskin bottle from a depth of about 0.5m.
Porewater samples were collected at several depths (5, 10 and 15cm) or at a depth of 10cm depending on the sediment type in transects every 1m up to 5m offshore using stainless steel lances and syringes (Szymczycha et al., 2012). The details regarding the sampling strategy including the spatial scale, coverage of groundwater endmember collection, and representativeness of the endmembers are given in Kłostowska et al. (2020).
Water samples from Reda, Zagórska Struga, and Płutnica rivers (R, n = 32) were also collected using the beaker during several campaigns (summer 2017-2019 and 2021).
Groundwater samples were collected from piezometers and coastal groundwater wells located in Chałupy, Jastarnia, and Hel on the Hel Peninsula and Władysławowo, Swarzewo and Gdynia on the mainland. Groundwater samples were collected during the standard method via a peristaltic pump using Teflon tubing connected to nylon tubing to prevent samples from degassing.
2.3 Chemical and statistical analyses
Salinity, pH, temperature, and oxidation-reduction potential (Eh) measurements of surface water from inner bay sediment pore water, groundwater and river water were performed with a WTW Multi 3400i Multi-Parameter Field Metre that yielded an accuracy of 0.02, 0.1, 0.1°C, respectively. Oxygen (O2) and Eh were measured in coastal sampling campaigns from autumn 2017 to summer 2018 with an accuracy of 0.1mg L-1 and 0.1mV, respectively (e.g. Szymczycha et al., 2020a).
Unfiltered samples for DIC and AT were collected in 250ml glass bottles, preserved with 100μL of a saturated HgCl2 solution, tightly sealed and stored in the refrigerator until analysis. The DIC was measured in a TOC-L analyser (Shimadzu Corp., Japan). The measurement method is based on sample acidification and detection of evolving CO2 in a nondispersive infrared (NDIR) detector. AT was analysed using an automated open-cell potentiometric titration system developed by A. Dickson (California, San Diego; Dickson et al., 2007). The quality check for both parameters was ensured using certified reference materials (CRMs, Marine Physical Laboratory at the Scripps Institution of Oceanography, University of California, San Diego). The DIC and AT measurement precisions, defined as the standard deviation, were equal to 7μmol L-1 and 4μmol kg-1, respectively.
Analyses of DOC were carried out using a ‘HyPerTOC’ analyser (Thermo Electron Corp., The Netherlands), using the UV/persulfate oxidation method and non-dispersive infrared (NDIR) detection. The quality control of the DOC analysis was performed using seawater (supplied by the Hansell Laboratory, University of Miami) as the accuracy tracer for each series of samples (average recovery was equal to 96 ± 3%). The precision, described as the relative standard deviation of the analysis in triplicate, was not less than 3%. More details can be found in Szymczycha et al. (2014; 2020a).
During the cruises, a Seabird 9/11 + CTD sensor was used to measure conductivity, temperature, and density in the surface water of Outer Puck Bay. The accuracies of the CTD were: C=0.0003S cm-1, T=0.001°C, and D=0.015%. Statistical analyses were performed using Statistica software (Statsoft). The partial pressure of carbon dioxide (pCO2) was calculated based on T, S, pH, and DIC using the CO2SYS programme (Pierrot et al., 2006). Knowing that the Baltic Sea characterizes is characterized by ion anomalies e.g. calcium (Millero, 1978; Anderson and Dyrssen, 1980); the saturation states of aragonite (ΩAr) and calcite (ΩCa) were calculated using two approaches: (A) based on T, S, pH, and DIC using the CO2SYS programme (Pierrot et al., 2006) and (B) following Mucci (1983) including calcium concentrations derived from salinity based on Anderson and Dyrssen (1980) and carbonate ion concentrations based on CO2SYS programme. Figure S2 presents ΩAr and ΩCa calculated using both approaches. Including calcium and carbonate concentration anomaly in the calculations (B) of ΩAr and ΩCa mostly influenced results for river water (ΩAr and ΩCa increase higher than in A) and seawater (ΩAr and ΩCa decrease lower than in A). Therefore, in further discussion, we used ΩAr and ΩCa obtained within approach (A) only, which is further justified as calcium concentrations derived based on Anderson and Dyrssen (1980) do not relate reflect the groundwater calcium concentrations found by Kłostowska et al. (2020).
3 Results
Groundwater samples (n=41) collected from wells and piezometers had low salinity (0.2 ± 0.2), and low pH (7.1 ± 0.5) (Figure 2, Table S1). The salinity of the rivers flowing into the Bay of Puck was comparable to groundwater (Figure 2, Table S2, p>0.05). However, the pH of river water was significantly higher (7.8 ± 0.3) than that of groundwater. The average salinity and pH of the SGD samples (n=84) equalled 3.3 ± 2.4 and 6.8 ± 0.5, respectively. The average salinity in the Inner Bay of Puck (6.6 ± 0.2) was slightly lower than in the Outer Bay of Puck (6.8 ± 0.3), while an average opposite trend was observed for pH (7.9 ± 0.2 and 7.8 ± 0.3, respectively). The concentrations of AT and DIC in groundwater had a wide range, with mean concentrations equal to 4223 ± 1800µmol kg-1 and 4404 ± 1748µmol L-1, respectively. The concentrations of AT and DIC in the rivers were similar to those of groundwater; however, with a lower range (Figure 2, Table S2, p>0.05). In SGD AT and DIC were similar to groundwater samples and characterised by a wide range of concentrations with mean values equal to 2954 ± 1263µmol kg-1 and 3217 ± 1434µmol L-1, respectively. The surface waters of the Inner and Outer Bay of Puck showed AT values of 1853 ± 61µmol kg-1 and 1779 ± 86µmol kg-1, respectively, and DIC values of 1833 ± 68µmol L-1 and 1714 ± 84µmol L-1, respectively (Figure 2). Although the salinity, AT, and DIC of the rivers that flow into the Inner Bay of Puck were comparable to groundwater, they indicated a lower range of minimum and maximum concentrations (Figure 2, Table S2, p>0.05). Ar was the lowest in groundwater and SGD with averages equal to 0.3 and 0.1, respectively (Figure 2). At the same time in these samples, pCO2 reached the highest level equal to 59881 and 67009µatm, respectively. ΩAr and pCO2 in rivers ranged from 0.05 to 1.1 and from 622 to 12003µatm, respectively. In the Inner and Outer Bay of Puck, the mean pCO2 was equal to 937 and 1578µatm while ΩAr was in the range of 0.2 to 0.7 and 0.0 to 0.9, respectively. Furthermore, we observed that SGD has a high average pCO2 compared to the average of local surface seawater (Figure 2). Generally, ΩCa followed the ΩAr distribution in all sample types.
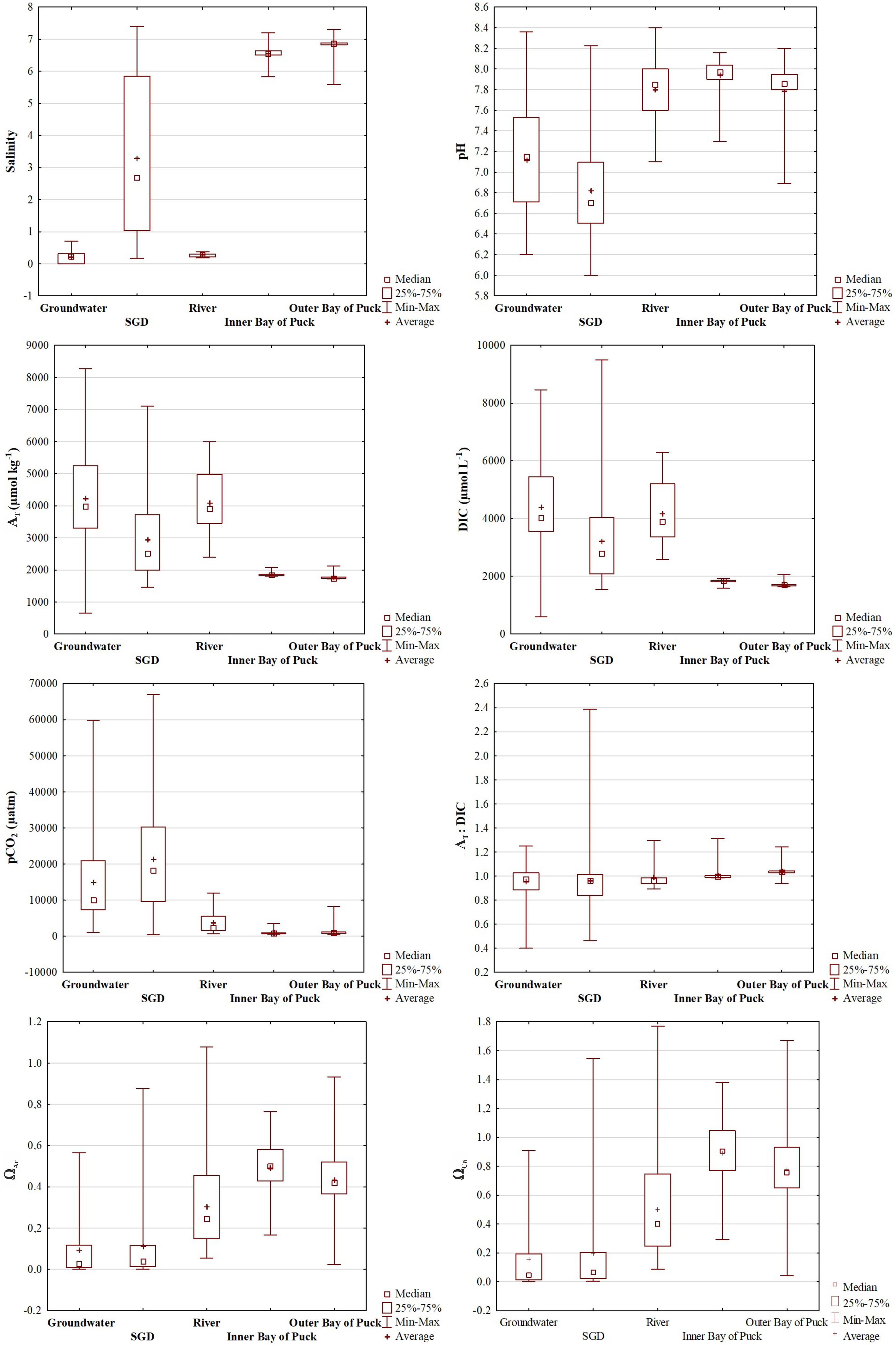
Figure 2 Box plot of salinity, pH, AT, DIC, pCO2, AT: DIC, ΩAr and ΩCa in different types of collected water samples.
Eh, O2, and DOC were not measured during all sampling campaigns, only from autumn 2017 to summer 2018. The results are published in Szymczycha et al. (2020a) and presented in Supplementary Figure 1. Within groundwater samples, Eh ranged from −225.5 to 94.2mV, and O2 concentrations ranged from 0.08 to 9.0mg L-1 and DOC from 343.3 to 3318.3 µmol L-1. Eh, O2 and DOC had scattered concentrations in SGD that ranged from -246.0 to 142mV, from 0 to 7.6mg L- 1 and from 278 to 2654µmol L-1, respectively. In seawater Eh, O2, and DOC were equal to 27mV, 9.0mg L- 1 and 201µmol L-1, respectively. Generally, fresh SGD was anoxic, had very low Eh and increased DOC compared to seawater.
4 Discussion
4.1 Carbonate system of SGD in the Bay of Puck
The AT, DIC, pH, AT: DIC, pCO2, ΩAr and ΩCa in groundwater, rivers, SGD, and surface water of the Inner and Outer Bay of Puck have been plotted against salinity, additionally pCO2, ΩAr and ΩCa also against pH (Figure 3). Distributions of DIC, AT, and pCO2 generally followed salinity, with higher concentrations of DIC, AT and pCO2 associating with lower salinities.
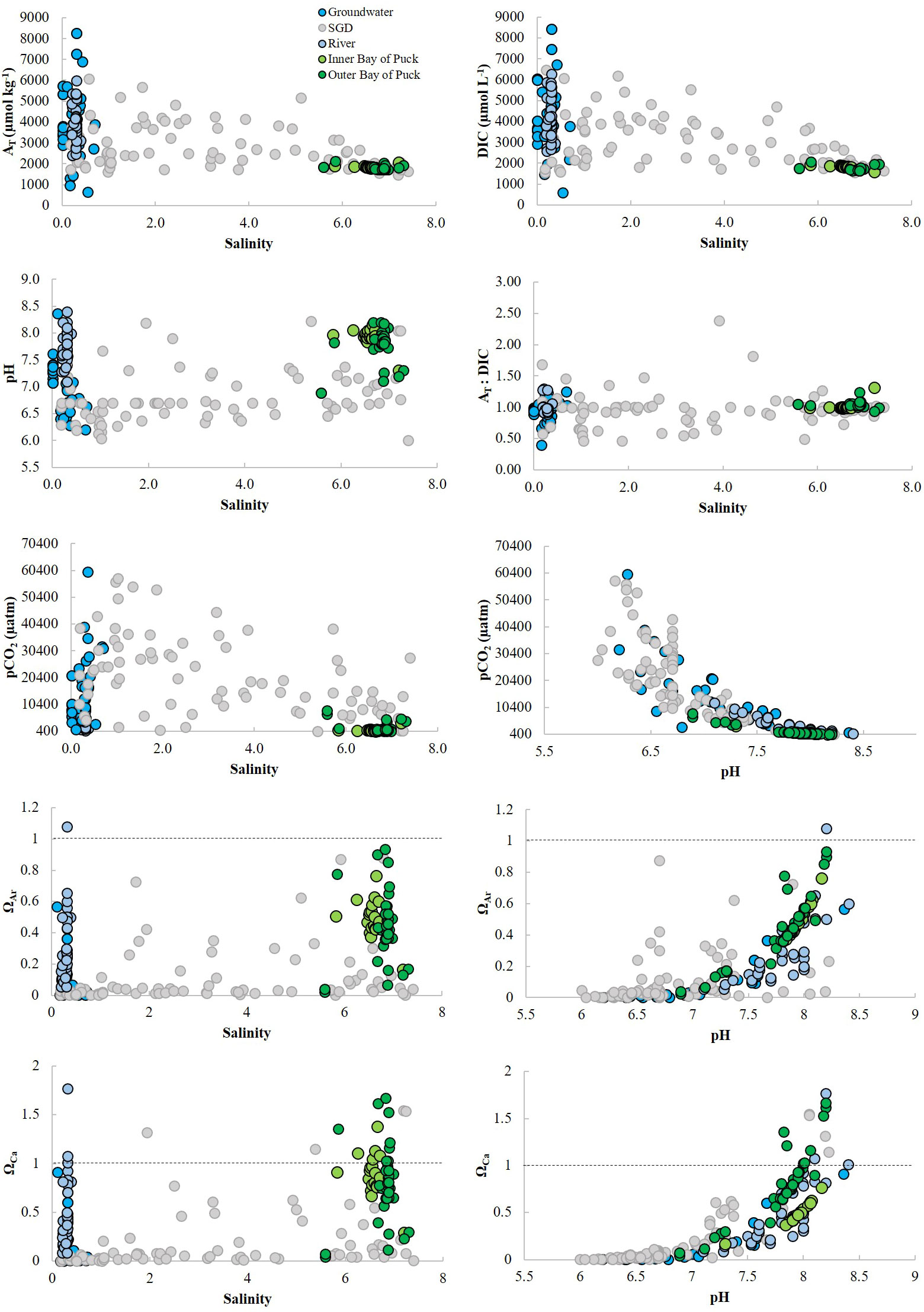
Figure 3 Relationship between AT, DIC, pH, AT: DIC, pCO2, ΩAr, ΩCa with salinity and pCO2, ΩAr and ΩCa with pH in collected samples.
The average concentrations of AT and DIC in groundwater, rivers and fresh SGD were 2.4 and 2.6, 1.7 and 1.9, 2.3 and 2.4 times higher than in surface waters, respectively, comprising a source of AT and DIC to the bay. What is more the concentration of AT in SGD was comparable to the Vistula River, the second-largest river entering the Baltic Sea (Hjalmarsson et al., 2008; Stokowski et al., 2021),
It should be noted that in the Inner Bay of Puck, both AT and DIC were slightly higher than in the outer bay, which may suggest that the discharge of AT and DIC through SGD and rivers has a more significant effect on this part of the Bay of Puck. The Outer Bay of Puck has direct contact with the open Baltic Sea waters; therefore, the impact of SGD - derived AT and DIC can be smaller in comparison with the sheltered Inner Bay of Puck.
Both rivers and groundwater samples had a very variable pH ranging from 6.2 to about 8.5. In SGD, high pH variability also occurred, with an increase in pH along with a salinity up to values observed in surface water (Figure 3). The low pH of groundwater and SGD suggests a possible dissolution of CaCO3 resulting in high values of values of DIC (Figure 3) (Deines et al., 1974; Winde et al., 2014; Wang et al., 2018; Liu et al., 2021). Freshwater end members that are enriched in AT and DIC, are a common phenomenon observed in limestone-rich catchments such as the Puck Bay catchment (Giani et al., 2023). For example, samples collected in Moreton Bay (Australia) showed average groundwater concentrations of AT and DIC 1.5 and 1.7 times higher than in the surface waters of Moreton Bay, respectively, which implies also that SGD may be a source of these solutes in surface waters (Stewart et al., 2015). Another example is from a limestone catchment in Taiwan where the average AT and DIC were significantly higher than those found in local surface seawater in the coastal zone (Wang et al., 2018).
Although the AT: DIC ratio oscillated around 1, relatively high variability of about ±0.5 have been observed for groundwater, rivers, and SGD. In this study, the AT: DIC <<1 suggests the presence of high pCO2 which is in good agreement with the generally low pH and ΩCa observed especially for groundwater and SGD (Figure 3). On the other hand, AT: DIC >> 1 may indicate a high share of noncarbonate components in alkalinity and/or low pCO2, however, the latter was probably not the case considering the cooccurrence of a relatively low pH (Figure 3).
Groundwater generally has a long residual time and, consequently, accumulates more products of OM decomposition, chemical weathering, and human activities, such as potential contamination by sewage or fertilisers (Knee and Paytan, 2011; Wang et al., 2018; Chen et al., 2019; Liu et al., 2021; Santos et al., 2021). In addition, it can flow through coastal aquifers that are organized in a complex matrix of confined, semi-confined and unconfined systems, influencing its composition (Moosdorf et al., 2021). Moreover, both AT and DIC concentrations can be modified in different ways by various diagenetic reactions within the STE due to the different importance of electron acceptors and donors (e.g., Froelich et al., 1979; Moosdorf et al., 2021).
To determine the behaviour of AT and DIC in the STE, a two-endmember mixing model was created using salinity as a conservative parameter to qualitatively depict sources and sinks of AT and DIC in the STE following Liu et al. (2021). The distribution of the average concentrations of AT and DIC (1 PSU step) in all SGD samples shows great variability (Figure 4). It must be noted that fresh SGD end members were already enriched in AT and DIC most likely coming from CaCO3 dissolution, and diagenetic processes occurring already in the aquifers, therefore the calculated production of AT and DIC in SGD (the anomaly from the conservative mixing) already included the AT and DIC signal derived from groundwater. The substantial increase in the mean values of AT and DIC identified in the mixing zone indicates the potential importance of the processes that occur in STE that release both AT and DIC. The conservative behaviour of AT was also observed when the results of different study sites were evaluated separately, e.g. in Jurata (Figure 4). However, in most sites, the AT concentration was higher than the conservative mixing line, indicating the production of AT. It must be underlined that the decomposition of OM generally lowers pH in the aerobic environment. In this study, the average pH of the SGD was significantly lower than that of the local surface seawater (Figures 2, 3). For AT and DIC, their concentration rises with the decomposition of organic matter (Liu et al., 2021). The calculated production of AT and DIC was in the range of 31 to 4505µmol kg-1 and to 6274µmol L-1, respectively. Modulations of AT and DIC in the mixing zone are usually the result of the dissolution or precipitation of carbonates, sulphur minerals and different biogeochemical pathways such as aerobic respiration and the spectrum of anaerobic redox reactions as previously described by Cai et al. (2003); Santos et al. (2015), and Liu et al. (2021).
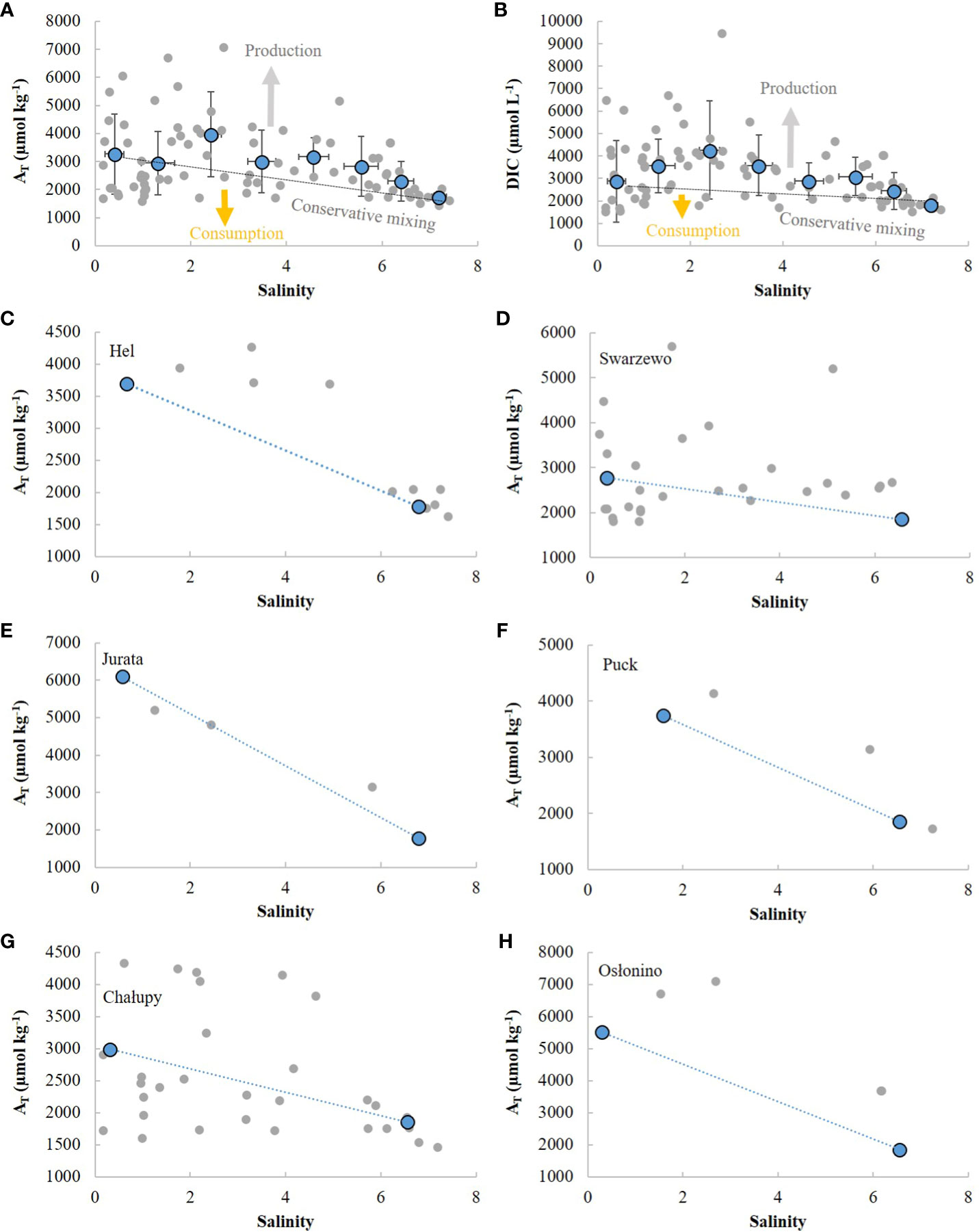
Figure 4 Relationship between (A) AT and (B) DIC with salinity in the freshwater-seawater mixing zone where the blue dots represent the averaged AT and DIC concentrations for every 1PSU with standard deviations denoted as error bars. (C-H) presents the relationship between AT and salinity in SGD (grey dots) divided into locations from where the samples were collected. The conservative mixing line shows the mixing between the fresh SGD endmember and saline endmember (seawater) (blue dots). The saline endmembers were calculated separately for Hel and Jurata (Outer Bay of Puck) and Chałupy, Puck, Swarzewo, and Osłonino (Inner Bay of Puck).
To illustrate the importance of different biogeochemical pathways the ΔAT has been plotted against ΔDIC with division into the study area and type of SGD (Figure 5). ΔAT and ΔDIC were calculated according to the offset between the measured data and the conservative mixing (Figure 4). The presented method provides information only on the cumulative effect of processes generating or consuming AT and DIC, therefore the influence of different processes can be masked in such a diagram. However, the possible production and depletion pathways have been added to Figure 5. The stoichiometric ratios were taken from Liu et al. (2021). The positive ΔAT and ΔDIC in most of the SGD collected in Swarzewo, Puck and Osłonino were located close to the denitrification and sulfate reduction pathway (ΔAT: ΔDIC=0.9 and 1, respectively) Samples collected in Hel were located a bit below the denitrification and sulfate reduction pathway. Part of the samples from Swarzewo and Chałupy followed the aerobic respiration pathway (ΔAT:ΔDIC=-0.2). The negative ΔAT and ΔDIC in Swarzewo and Chałupy were located close to the FeS precipitation pathway (ΔAT: ΔDIC=-1). CaCO3 precipitation pathway was not considered as all SGD samples were characterized with low ΩCa. In all SGD samples (saline, mixed and fresh) denitrification pathway and sulfate reduction pathway were responsible primarily for the production of AT and DIC (Figure 5). The depletion of ΔAT and ΔDIC was observed only in some fresh and mixed SGD samples and can be attributed to the FeS precipitation pathway. Our results suggest that the production of AT and DIC in STE was mainly due to denitrification and sulphate reduction. This is consistent with previous studies showing depletion or absence of nitrate and nitrite (Szymczycha et al., 2012; Szymczycha et al., 2020b), absence of oxygen and occurrence of sulphides (Donis et al., 2017).
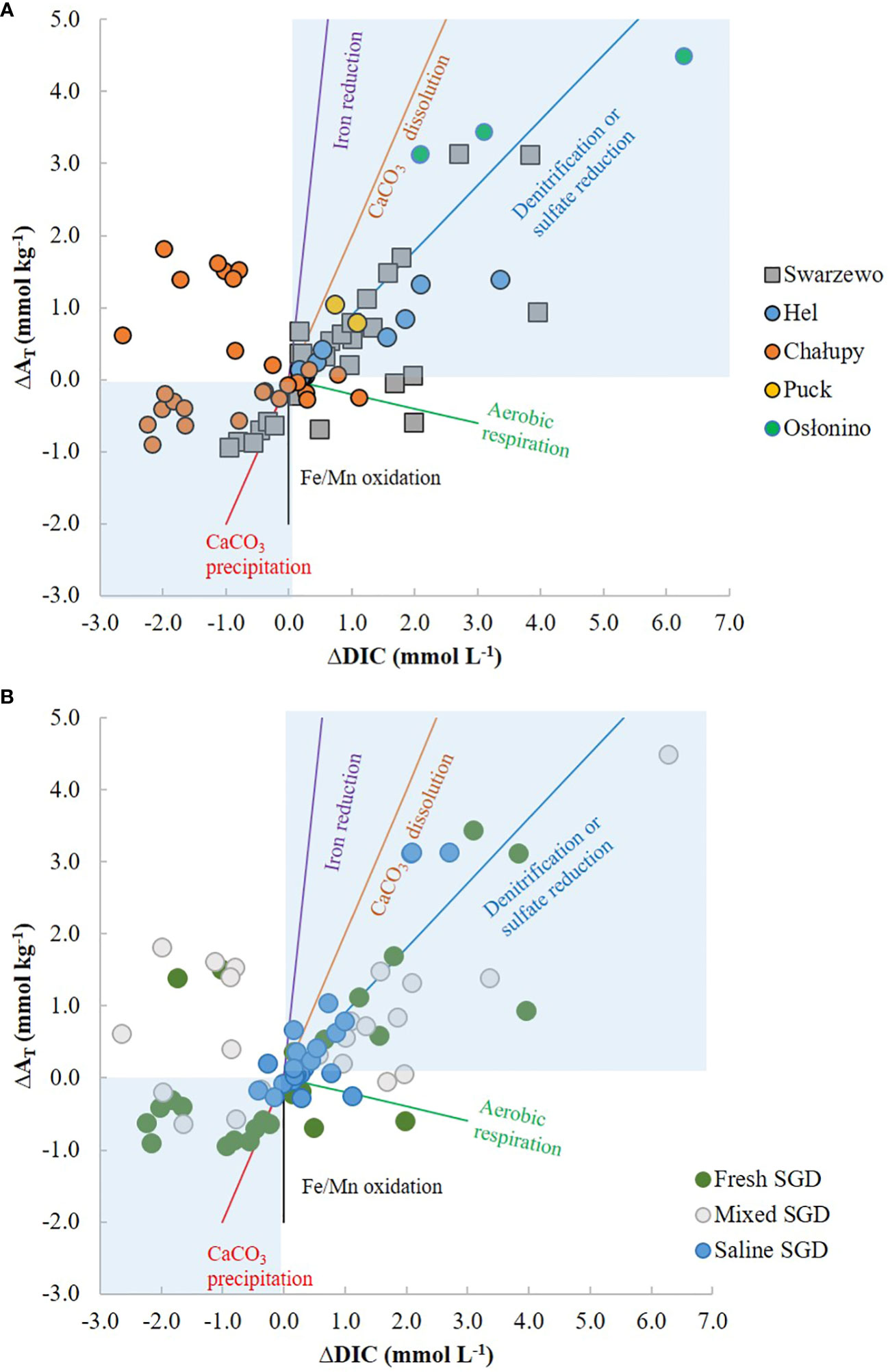
Figure 5 Relationship between ΔAT and ΔDIC to identify the biogeochemical pathways of carbonates in the freshwater-seawater mixing zone (A) with division into the study site, (B) with division into SGD type.
However, it should be noted that once hypoxic/anoxic SGD reaches oxic conditions (Figure 3) decrease in AT and DIC can be observed. Part of the depletion can be attributed to the mixing of AT and DIC enriched SGD with AT and DIC decreased seawater. AT depletion can also be attributed to nitrification, iron oxidation, and manganese oxidation. The decrease in AT and DIC can be further explained by the precipitation of FeS and CaCO3 while DIC can drop due to the CO2 outgassing (Liu et al., 2021).
The concentration of nutrients in SGD at the same time of sampling and in the same area was lower (NO3-: 26.4 ± 38.9µmol L-1, NH4+: 110.9 ± 25.6µmol L-1, PO43-: 10.8 ± 3.6µmol L-1, Szymczycha et al., 2020b) than the average excess DIC supported by SGD (DIC: from 1354µmol L-1 to 6274µmol L-1). Therefore, the primary production supported by the input of nutrients from SGD is insufficient to compensate for the high DIC and pCO2 supplied by SGD. As a result, the SGD in the Puck of Bay leads to a CO2 outgassing to the atmosphere. To calculate the loss of AT and DIC in SGD, we used the regressions for AT and DIC and calculated the differences for fresh ad saline end-members. The loss of AT and DIC reached 32% and 37%, respectively. The uncertainty is considered to be less than 3% based on the standard deviations for AT and DIC measurements.
4.2 AT and DIC fluxes via SGD to the Bay of Puck
To estimate the AT and DIC fluxes via SGD, the average AT and DIC concentrations in SGD samples were multiplied by the minimal and maximal SGD fluxes calculated at the same study sites which were equal to 1.8 10-7L cm-2 s-1 and 2.8 10-7 L cm-2 s-1, respectively (Kłostowska et al., 2020). The obtained AT fluxes ranged from 0.5 mol m-2 d-1 to 0.7 mol m-2 d-1 while the DIC fluxes ranged from 0.5 mol m-2 d-1 to 0.8mol m-2 d-1. As the change from anoxic to oxic conditions led to a decrease in AT and DIC by 32 and 37%, respectively, we included this loss in calculations of the SGD-AT and SGD-DIC fluxes. The final AT and DIC fluxes through SGD ranged from 0.1 to 0.2mol m-2 d-1 and from 0.2 to 0.3mol m-2 d-1, respectively. The benthic flux of AT in the Gdańsk Deep calculated as the sum of fluxes of particular AT components and assuming complete oxidation of released sulfide, equalled 0.001263 ± 0.000518mol m−2 d−1 (Łukawska-Matuszewska and Graca, 2018). The DIC benthic fluxes characteristic for the coastal zone of the southern Baltic Sea ranged from −0.0021mol m-2 d-1 to 0.1106mol m-2 d-1 (Silberberger et al., 2022). AT and DIC fluxes calculated in this study were significantly higher than the benthic fluxes characteristic of the southern Baltic Sea.
In the surface ocean, the distribution of AT is often strongly interlinked with salinity, as it is mainly controlled by freshwater addition (such as a river, groundwater discharge, and ice melting) or removal (e.g. ice formation) (Millero et al., 1998). We compared AT results found in the surface waters of the Bay of Puck (this study) with those calculated based on the dependences of AT-S available for the Central Baltic–Belt Sea–Kattegat region: AT=25.3*S+1470μmol kg−1 (Bełdowski et al., 2010) and for the Vistula River plume region (southern Gulf of Gdansk): AT=-184*S + 3379μmol kg−1 (Stokowski et al., 2021) taking into account the typical salinity for surface waters in the Bay of Puck, of about 7 (Figure 6). The average AT concentration in the Bay of Puck based on this study is equal to 1941 ± 126μmol kg−1 and was significantly higher than the estimated average AT concentration(AT=1653 ± 1.4μmol kg−1) based on AT-S dependence from Bełdowski et al. (2010) and slightly lower than that calculated based on AT-S dependence from Stokowski et al. (2021) (S=7, AT=2049 ± 10.6μmol kg−1). Knowing that the Bay of Puck is under the limited influence of the Vistula River, as westerly winds are predominant in that region, and the river outflow is typically directed east along the coast and slowly entrained into the Baltic anticyclonic current (Matciak and Nowacki, 1995; Wielgat-Rychert et al., 2013) we propose that the increased surface seawater concentration of AT in comparison to the Central Baltic–Belt Sea–Kattegat region may be mostly a result of SGD derived AT fluxes.
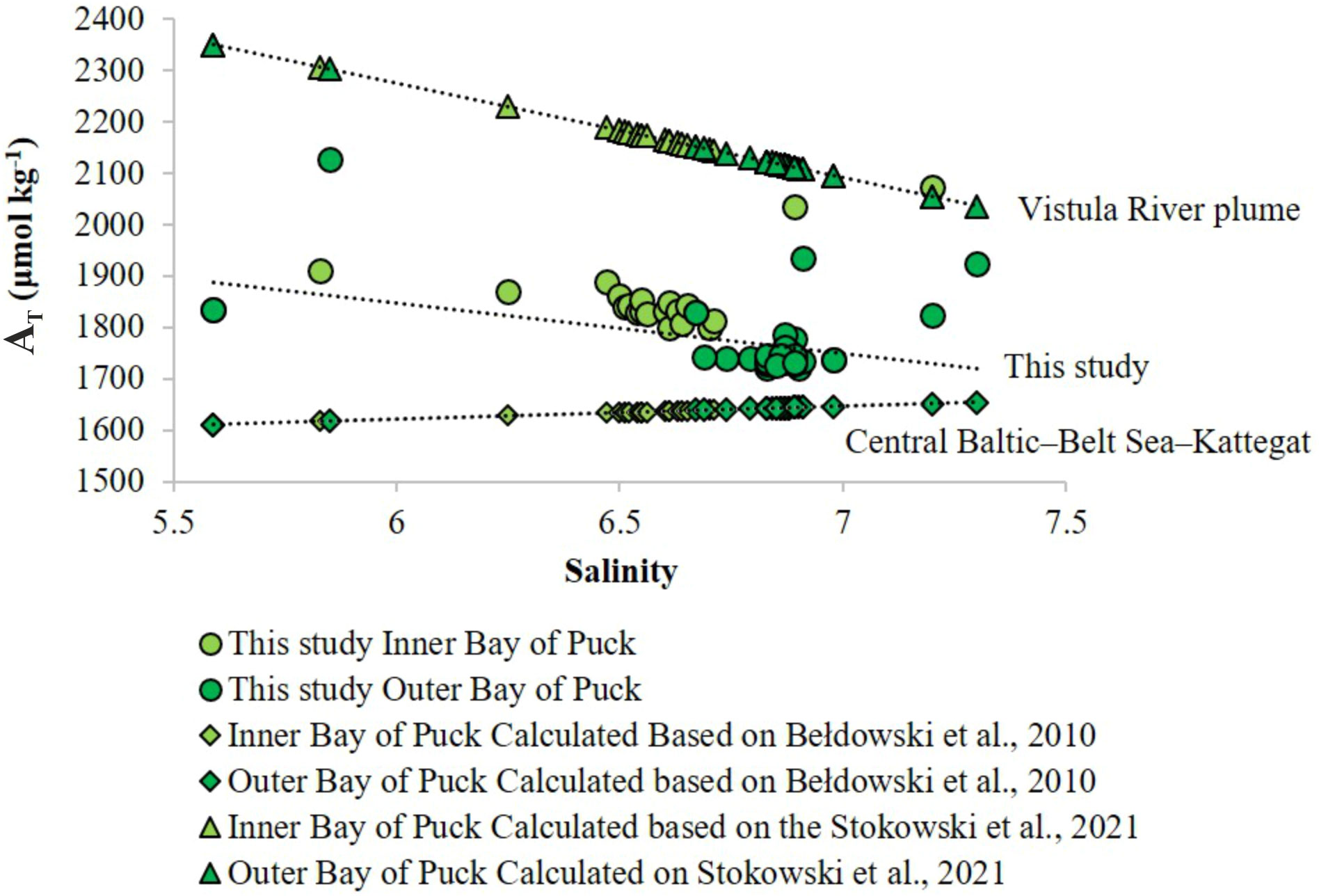
Figure 6 Measured salinity and AT concentrations in seawater (this study), and the calculated AT based on the AT-S functions from Bełdowski et al. (2010) and Stokowski et al. (2021). The dotted lines represent the regression lines.
What is worth underlining, Bay of Puck surface seawater concentrations of both AT and DIC in the Bay of Puck are higher than those obtained in the reported for other areas of the Baltic Sea. AT concentrations in the surface seawater of the Baltic Sea (including Baltic Proper, Gulf of Bothnia, Gulf of Riga and Gulf of Finland) were described in Bełdowski et al. (2010). Figure 7 presents the averaged AT concentrations in the rivers, groundwater, SGD and seawater in the Bay of Puck in comparison to the surface waters of all areas of the Baltic Sea apart from the Gulf of Riga (Bełdowski et al., 2010). In addition, DIC concentrations in surface seawater obtained in this study (from 1714 ± 84µmol L-1 to 1833 ± 68µmol L-1) were higher than those obtained reported for the Baltic Sea (surface water in the Arkona Deep) that ranged from 1506µmol L-1 to 1584µmol L- 1 (Kuliński et al., 2011). Therefore, it seems that SGD is the most important factor contributing to the AT pool in the Bay of Puck. Along the Polish coast, SGD has a similar DIC concentration (Szymczycha et al., 2014) therefore, it can be speculated that AT concentrations are also comparable to those in the Bay of Puck. Consequently, the AT input through SGD along the Polish coast can be an important mechanism explaining AT concentration in the southern Baltic Sea. However, this assumption needs to be confirmed by further SGD studies along the Baltic Sea coast.
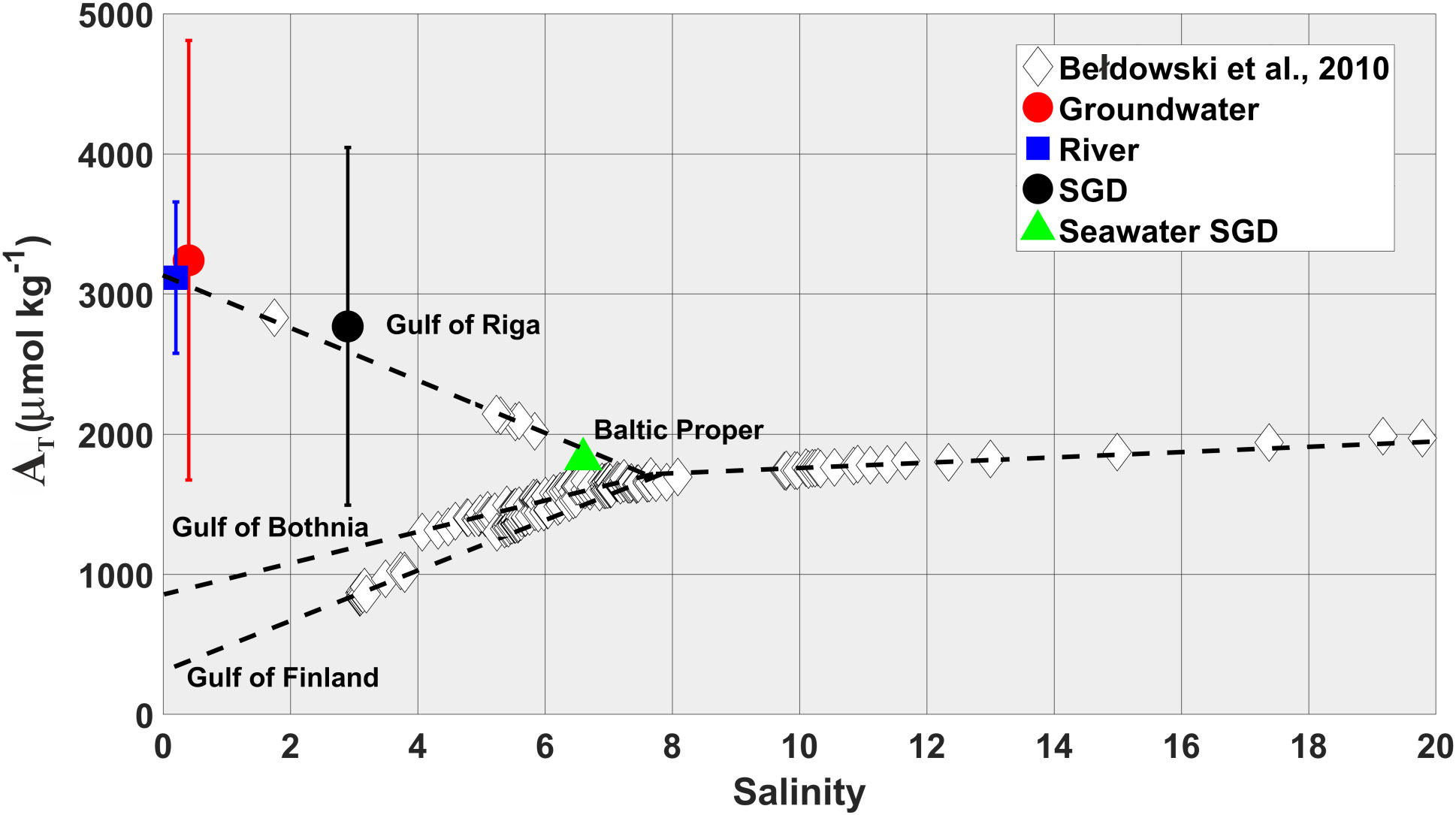
Figure 7 Surface AT concentrations in the Baltic Sea (modified after Bełdowski et al., 2010).
4.3 The influence of SGD on marine calcifying invertebrates
Marine calcifying organisms are threatened by global stressors, such as increasing sea surface temperatures and ocean acidification, and by local stressors such as land-based sources of pollution and/or SGD that can magnify the effects of OA (Ni et al., 2018; Prouty et al., 2022).
In shallow regions of the Gulf of Gdańsk (Figure 1), invertebrates that can form calcium carbonate skeletons are commonly found. In a study by Piwoni-Piórewicz et al. (2021) aragonitic bivalves Cerastoderma glaucum, Limecola balthica, and Mya arenaria were detected in the Bay of Puck; while at the border of the Puck Bay and Gulf of Gdańsk occur bimineralic bivalve Mytilus trossulus and calcite arthropod Amphibalanus improvises were detected. The Mg-calcite-forming Spirorbis spirorbis has been found in the Baltic Sea (Kiel Bight, Ni et al., 2018) attached to the brown macroalgae Fucus.
Generally, inorganic precipitation of calcium carbonate is likely to occur substantially above saturation degrees of 1 (e.g., Michaelis et al., 1985), whereas carbonate dissolution occurs with Ω decreasing further below 1. Tyrrell et al. (2008) in a seasonal study proved that the Gotland Sea, Gulf of Riga, and Bothnian Bay (Baltic Sea) become undersaturated in winter. They claimed that the absence of the coccolithophore Emiliania huxleyi could therefore potentially be explained by the dissolution of their coccoliths in winter, suggesting that minimum annual (wintertime) saturation states could be most important in determining future ocean acidification impacts. In a mesocosm study using coastal Baltic Sea water (Wahl et al., 2015), CO2 enrichment led to the co-occurrence of S. spirorbis growth and corrosion (Ni et al., 2018). In addition, Stokowski et al. (2021) observed an undersaturation (Ω<1) with aragonite in the mouth of the Vistula River (Gdańsk Gulf) in wintertime. In a study by Thomsen et al. (2010) Mytilus edulis, a dominant macrobenthic species in the Baltic Sea, was found to maintain shell and somatic growth rates at ca.1400 µatm CO2 (pH around 7.6). Their unusual ability to maintain physiological function despite substantial levels of acidification was due in part to an abundance of food, which provided the energy needed to offset the physiological costs of maintenance and growth at such low ambient pH (Melzner et al., 2011). However, more sensitive early stages of the Skagerrak Mytilus edulis life history have been shown to respond differently to OA: fertilisation success increased at reduced pH (induced by high pCO2) whereas subsequent larval shell growth was negatively affected.
In our study, low ΩAr and ΩCa together with low pH were found in SGD. In addition, low ΩAr and ΩCa were also found in surface seawater. The average ΩAr and ΩCa in the surface seawater of the Inner Bay of Puck (summer) was equal to 0.5 ± 0.1 and 0.9 ± 0.2, respectively (Figure 2), while in the Outer Bay of Puck (beginning of autumn) the average ΩAr and ΩCa equalled 0.4 ± 0.2 and 0.7 ± 0.4, respectively. The conditions in SGD-impacted sediments and, generally, seawater of the Bay of Puck (undersaturation with respect to the forms of the aragonite and calcite minerals of CaCO3 and low pH) are, most probably, negatively influencing the growth and functioning of calcifying invertebrates. We think further studies are needed to understand how SGD affects the Bay of Puck ecosystem.
5 Conclusions
The concentrations of AT, DIC, and pCO2 and pH for groundwater, rivers, SGD, and surface water in the Bay of Puck have been presented. AT and DIC production in the STE has been observed. The nutrients supplied by SGD are, most probably, insufficient to maintain production high enough to compensate for the high DIC release, and therefore SGD in the Bay of Puck can be a source of CO2 for the atmosphere. Although SGD and accompanying AT fluxes enhance the buffer capacity of the Bay of Puck against ocean acidification, still low pH caused by high CO2 release, and thus low ΩAr and ΩCa can negatively influence the marine benthic calcifying organisms.
Data availability statement
The original contributions presented in the study are included in the article/Supplementary Material. Further inquiries can be directed to the corresponding author.
Author contributions
BS was responsible for the preparation of the concept of research, sampling, participation in the analysis of environmental samples, preparation of figures and tables, interpretation of results, and writing and editing of the manuscript. CA participated in the sampling campaigns and the conventionalization of the study. MB, MD, KK-M, KK, and CA contributed to data discussion in the writing and editing of the manuscript. MD and AW were responsible for AT and DIC analyses. The PM was involved in sampling, figures preparation and manuscript editing. The authors declare that they did not use artificial intelligence-based software to produce any parts of the text in this study. All authors contributed to the article and approved the submitted version.
Funding
The reported results were obtained within the framework of the statutory activities of the Institute of Oceanology of the Polish Academy of Sciences and the following research projects: WaterPUCK BIOSTRATEG3/343927/3/NCBR/2017 financed by the National Centre for Research and Development (NCBiR) within the BIOSTRATEG III program; PharmSeepage 2016/21/B/ST10/01213 and IDEAL 2019/34/E/ST10/00217 funded by the Polish National Science Centre. MB and CA acknowledge support from a stipend for CA from DAAD, and support to MB within the ASSEMBLE+ and CARBOSTORE projects.
Acknowledgments
The publication is CARBOSTORE publication No. 007. The paper further contributes to the science plan of the Surface Ocean-Lower Atmosphere Study (SOLAS), which is supported by the U.S. National Science Foundation via the Scientific Committee on Oceanic Research (SCOR). MEB and CVA acknowledge support from a stipend for CVA from DAAD. The research leading to these results received partial funding from the European Union's Horizon 2020 research and innovation programme under grant agreement No. 730987, Assemble Plus project. MEB dedicates this study to the memory of the coastal ecologist and biochemist Thomas Höpner, who recently passed away. BSz would like to thank Piotr Prusiński and Laura Bromboszcz for their support in the manuscript editing. BSz would like to acknowledge Marta Borecka, Żaneta Kłostowska, Monika Lengier, Leszek Łęczyński and Marcin Stokowski for their help in sampling campaigns. BSz is grateful to Jacek Bełdowski for managing the sampling campaign on board s/y Świrek, and to the captain and crew of the r/v Oceania.
Conflict of interest
The authors declare that the research was conducted in the absence of any commercial or financial relationships that could be construed as a potential conflict of interest.
Publisher’s note
All claims expressed in this article are solely those of the authors and do not necessarily represent those of their affiliated organizations, or those of the publisher, the editors and the reviewers. Any product that may be evaluated in this article, or claim that may be made by its manufacturer, is not guaranteed or endorsed by the publisher.
Supplementary material
The Supplementary Material for this article can be found online at: https://www.frontiersin.org/articles/10.3389/fmars.2023.1218245/full#supplementary-material
References
Anderson L., Dyrssen O. (1980) . Excess calcium and alkalinity in the baltic and southern kattegatt. Available at: https://archimer.ifremer.fr/doc/00121/23215/.
Arévalo-Martínez D. L., Haroon A., Bange H. W., Erkul E., Jegen M., Moosdorf N., et al. (2022). Ideas and perspectives: Land-ocean connectivity through groundwater. Biogeosciences disc. BG2022-148, 1–27. doi: 10.5194/bg-2022-148
Bełdowski J., Löffler A., Schneider B., Joensuu L. (2010). Distribution and biogeochemical control of total CO2 and total alkalinity in the Baltic Sea. J. Mar. Syst. 81, 252–259. doi: 10.1016/j.jmarsys.2009.12.020
Bishop J. M., Glenn C. R., Amato D. W., Dulai H. (2007). Effect of land use and groundwater flow path on submarine groundwater discharge nutrient flux. J. Hydrol.: Reg. Stud. 11, 194–218. doi: 10.1016/j.ejrh.2015.10.008
Burnett W. C., Bokuniewicz H., Huettel M., Moore W. S., Taniguchi M. (2003). Groundwater and pore water inputs to the coastal zone. Biogeochemistry 66 (1-2), 3–33. doi: 10.1023/B:BIOG.0000006066.21240.53
Cai W. J., Wang Y. C., Krest J., Moore W. S. (2003). The geochemistry of dissolved inorganic carbon in a surficial groundwater aquifer in North Inlet, South Carolina, and the carbon fluxes to the coastal ocean. Geochimica Cosmochimica Acta 67 (4), 631–639. doi: 10.1016/S0016-7037(02)01167-5
Charette M. A., Buesseler K. O., Andrews J. E. (2001). Utility of radium isotopes for evaluating the input and transport of groundwater-derived nitrogen to a Cape Cod estuary. Limnology Oceanography 46, 465–470. doi: 10.4319/lo.2001.46.2.0465
Charette M. A., Sholkovitz E. R. (2002). The formation of an iron curtain in the subterranean estuary of a coastal bay. Geophysical Res. Lett. 29, 2001GL014512.
Chen C.-T. A., Huang T.-H., Lui H.-K., Zhang J. (2019). Submarine groundwater discharge. Oceanogr Fish Open Access J. 10 (5), 555797. doi: 10.19080/ofoaj.2019.10.555797
Cho H. M., Kim G., Kwon E. Y., Moosdorf N., Garcia-Orellana J., Santos I. R. (2018). Radium tracing nutrient inputs through submarine groundwater discharge in the global ocean. Sci. Rep. 8. doi: 10.1038/s41598-018-20806-2
Deines P., Langmuir D., Harmon R. S. (1974). Stable carbon isotope ratios and the existence of a gas phase in the evolution of carbonate ground waters. Geochim. Cosmochim. Acta 38 (7), 1147–1164. doi: 10.1016/0016-7037(74)90010-6
A. G. Dickson, C. L., Sabine, J. R. Christian (2007). Guide to best practices for ocean CO2 measurement (Sidney, British Columbia: North Pacific Marine Science Organization), 191. doi: 10.25607/OBP-1342
Donis D., Janssen F., Liu B., Wenzhofer F., Dellwig O., Escher P., et al. (2017). Biogeochemical impact of submarine ground water discharge on coastal surface sands of the southern Baltic Sea. Estuar. Coast. Shelf Sci. 189, 131–142. doi: 10.1016/j.ecss.2017.03.003
Ehlert von Ahn C. M., Böttcher M. E., Malik C., Westphal J., Rach B., Nantke C., et al. (2023). Spatial and temporal variations in the isotope hydrobiogeochemistry of a managed river draining towards the southern Baltic Sea. Geochemistry, 125979. doi: 10.1016/j.chemer.2023.125979
Ehlert von Ahn C. M., Scholten J., Malik C., Feldens P., Liu B., Dellwig O., et al. (2021). A multi-tracer study of fresh submarine and surface water sources for a temperate urbanized coastal bay. Front. Environm. Sci. 9, 642346. doi: 10.3389/fenvs.2021.642346
Froelich P. N., Klinkhammer G. P., Bender M. L., Luedtke N. A., Heath G. R., Cullen D., et al. (1979). Early oxidation of organic matter in pelagic sediments of the eastern equatorial Atlantic: suboxic diagenesis. Geochimica Cosmochimica Acta 43, 1075–1090. doi: 10.1016/0016-7037(79)90095-4
Giani M., Ogrinc N., Tamše S., Cozzi S. (2023). Elevated river inputs of the total alkalinity and dissolved inorganic carbon in the Northern Adriatic Sea. Water 15, 894. doi: 10.3390/w15050894
Gustafsson E., Hagens M., Sun X., Reed D. C., Humborg C., Slomp C. P., et al. (2019). Sedimentary alkalinity generation and long-term alkalinity development in the Baltic Sea. Biogeosciences 16, 437–456. doi: 10.5194/bg-16-437-2019
Hjalmarsson S., Wesslander K., Anderson L. G., Omstedt A., Perttilä M., Mintrop M. (2008). Distribution, long-term development and mass balance calculation of total alkalinity in the Baltic Sea. Continental Shelf Res. 28, 593–601. doi: 10.1016/j.csr.2007.11.010
Humborg C., Blomqvist S., Avsan E., Bergensund Y., Smedberg E., Brink J., et al. (2002). Hydrological alterations with river damming in northern Sweden: Implications for weathering and river biogeochemistry. Global Biogeochem. Cyc. 1. doi: 10.1029/2000GB001369
Johannes R. E. (1980). The ecological significance of the submarine discharge of groundwater. Mar. Ecol. Prog. Ser. 3 (4), 365–373. doi: 10.3354/MEPS003365
Kłostowska Z., Szymczycha B., Lengier M., Zarzeczanska D., Dzierzbicka-Glowacka L. (2020). Hydrogeochemistry and magnitude of SGD in the Bay of Puck, southern Baltic Sea. Oceanologia 62 (1), 1–11. doi: 10.1016/j.oceano.2019.09.001
Knee K. L., Paytan A. (2011). “Submarine groundwater discharge: A source of nutrients, metals, and pollutants to the coastal ocean,” in Treatise on estuarine and coastal science, vol. 4 . Eds. Wolanski E., McLusky D. S. (Waltham: Academic Press), 205–233. doi: 10.1016/B978-0-12-374711-2.00410-1
Kotwicki L., Grzelak K., Czub M., Dellwig O., Gentz T., Szymczycha B., et al. (2014). Submarine groundwater discharge to the Baltic coastal zone: Impacts on the meiofaunal community. J. Mar. Sys. 129, 118–126. doi: 10.1016/j.jmarsys.2013.06.009
Kramarska R., Uścinowicz S., Zachowicz J., Kawińska M. (1995). Origin and evolution of the puck lagoon. J. Coast. Res. 22, 187–191.
Krumins V., Gehlen M., Arndt S., Van Cappellen P., Regnier P. (2013). Dissolved inorganic carbon and alkalinity fluxes from coastal marine sediments: model estimates for different shelf environments and sensitivity to global change. Biogeosciences 10, 1, 371–1, 398. doi: 10.5194/bg-10-371-2013
Kuliński K., Rehder G., Asmala E., Bartosova A., Carstensen J., Gustafsson B., et al. (2022). Biogeochemical functioning of the Baltic Sea, earth syst. Dynam. 13, 633–685. doi: 10.5194/esd-13-633-2022
Kuliński K., She J., Pempkowiak J. (2011). Short and medium term dynamics of the carbon exchange between the Baltic Sea and the North Sea. Continental Shelf Res. 31, 1611–1619. doi: 10.1016/j.csr.2011.07.001
Kuliński K., Szymczycha B., Koziorowska K., Hammer K., Schneider B. (2018). Anomaly of total boron concentration in the brackish waters of the Baltic Sea and its consequence for the CO2 system calculations. Mar. Chem. 204, 11–19. doi: 10.1016/j.marchem.2018.05.007
Liu Q., Charette M. A., Breier C. F., Henderson P. B., McCorkle D. C., Martin W., et al. (2017). Carbonate system biogeochemistry in a subterranean estuary – Waquoit Bay, USA. Geochim. Cosmochim. Acta 203, 422–439. doi: 10.1016/j.gca.2017.01.041
Liu Q., Charette M. A., Henderson P. B., McCorkle D. C., Martin W., Dai M. H. (2014). Effect of submarine groundwater discharge on the coastal ocean inorganic carbon cycle. Limnology Oceanography 59 (5), 1529–1554. doi: 10.4319/lo.2014.59.5.1529
Liu Q., Dai M., Chen W., Huh C. A., Wang G., Li Q., et al. (2012). How significant is submarine groundwater discharge and its associated dissolved inorganic carbon in a river-dominated shelf system? Biogeosciences 9 (5), 1777–1795. doi: 10.5194/bg-9-1777-2012
Liu Y., Jiao J. J., Liang W., Santos I. R., Kuang X., Robinson C. R. (2021). Inorganic carbon and alkalinity biogeochemistry and fluxes in an intertidal beach aquifer: Implications for ocean acidification. J. Hydrol. 595. doi: 10.1016/j.jhydrol.2021.126036
Luijendijk E., Gleeson T., Moosdorf N. (2020). Fresh groundwater discharge insignificant for the world’s oceans but important for coastal ecosystems. Nat. Commun. 11, 1–12. doi: 10.1038/s41467-020-15064-8
Łukawska-Matuszewska K. (2016). Contribution of non-carbonate inorganic and organic alkalinity to total measured alkalinity in pore waters in marine sediments (Gulf of Gdansk, S-E Baltic Sea). Mar. Chem. 186, 211–220. doi: 10.1016/j.marchem.2016.10.002
Łukawska-Matuszewska K., Graca B. (2018). Pore water alkalinity below the permanent halocline in the Gdańsk Deep (Baltic Sea) - Concentration variability and benthic fluxes. Mar. Chem. 204, 49–61. doi: 10.1016/j.marchem.2018.05.011
Matciak M., Bieleninik S., Botur A., Podgorski M., Trzcinska K., Draganska K., et al. (2015). Observations of presumable groundwater seepage occurrence in Puck Bay (the Baltic Sea). Oceanological Hydrobiological Stud. 44 (2), 267–272. doi: 10.1515/ohs-2015-0025
Matciak M., Nowacki J. (1995). The Vistula river discharge front-surface observations Plume front Surface water properties River Vistula. Oceanologia 37, 75–75.
Melzner F., Stange P., Trübenbach K., Thomsen J., Casties I., Panknin U., et al. (2011). Food Supply and Seawater pCO2 Impact Calcification and Internal Shell Dissolution in the Blue Mussel Mytilus edulis. PloS One 6. doi: 10.1371/journal.pone.0024223
Michaelis J., Usdowski E., Menschel G. (1985). Partitioning of 13C and 12C on the degassing of CO2 and the precipitation of calcite - Rayleigh-type fractionation and a kinetic model. Am. J. Sci. 285, 318–327. doi: 10.2475/ajs.285.4.318
Middelburg J. J., Soetaert K., Hagens M. (2020). Ocean alkalinity, buffering and biogeochemical processes. Rev. Geophysics 58, e2019RG000681. doi: 10.1029/2019RG000681
Millero F. J., Lee K., Roche M. (1998). Distribution of alkalinity in the surface waters of the major oceans. Mar. Chem. 60, 111–130. doi: 10.1016/S0304-4203(97)00084-4
Moore W. S. (1996). Large groundwater inputs to coastal waters revealed by 226Ra enrichments. Nature 380 (6575), 612–614. doi: 10.1038/380612a0
Moore W. S. (1999). The subterranean estuary: a reaction zone of ground water and sea water. Mar. Chem. 65 (1-2), 111–125. doi: 10.1016/S0304-4203(99)00014-6
Moore W. S., Beck M., Riedel T., van der Rutgers L., Dellwig M., Shaw O., et al. (2011). Radium-based pore water fluxes of silica, alkalinity, manganese, DOC, and uranium: A decade of studies in the German Wadden Sea. Geochim. Cosmochim. Acta 75 (21), 6535–6555. doi: 10.1016/j.gca.2011.08.037
Moosdorf N., Böttcher M. E., Adyasari D., Erkul E., Gilfedder B., Greskowiak J., et al. (2021). A state-of-the-art perspective on the characterization of subterranean estuaries at the regional scale. Front. Earth Sci. 9. doi: 10.3389/feart.2021.601293
Mucci A. (1983). The solubility of calcite and aragonite in seawater at various salinities, temperatures, and one atmosphere total pressure. Am. J. Sci. 283, 780–799. doi: 10.2475/ajs.283.7.780
Müller J. D., Schneider B., Rehder G. (2016). Long-term alkalinity trends in the Baltic Sea and their implications for CO2-induced acidification. Limnol. Oceanogr. 61, 1984–2002. doi: 10.1002/lno.10349
Ni S., Taubner I., Böhm F., Winde V., Böttcher M. E. (2018). Effect of temperature rise and ocean acidification on growth of calcifying tubeworm shells (Spirorbis spirorbis): An in-situ benthocosm approach. Biogeosciences 15, 1425–1445. doi: 10.5194/bg-15-1425-2018
Nowacki J. (1993). Gulf morphometry. Eds. Bay P., Korzeniewski K. (Gdańsk: Fund. Rozw. Uniw. Gdańs), 71–78.
Peltonen K. (2002). Direct groundwater flow to the Baltic Sea (Copenhagen: Nordic Council of Ministers, TemaNord Environment).
Piekarek-Jankowska H. (1994). Zatoka Pucka jako obszar drenażu wód podziemnych (Gdańsk: Rozprawy i Monografie nr 204, Wydawnictwo Uniwersytetu Gdańskiego), 31–32,
Piekarek-Jankowska H. (1996). Hydrochemical effects of submarine groundwater discharge to the Puck Bay (southern Baltic Sea, Poland). Geographica Polonica 67, 103–119.
Piekarek-Jankowska H., Łęczyński L. (1993). Morfologia dna. Ed. Korzeniewski K. (Gdańsk: Zatoka Pucka. Fundacja Rozwoju UG), 222–281.
Pierrot D., Lewis E., Wallace D. W. R. (2006). MS excel program developed for CO2 system calculations. doi: 10.3334/CDIAC/otg.CO2SYS_XLS_CDIAC105a
Piwoni-Piórewicz A., Strekopytov S., Humphreys-Williams E., Kukliński P. (2021). The patterns of elemental concentration (Ca, Na Sr, Mg, Mn, Ba, Cu, Pb, V, Y, U and Cd) in shells of invertebrates representing different CaCO3 polymorphs: a case study from the brackish Gulf of Gdańsk (the Baltic Sea). Biogeosciences 18, 707–728. doi: 10.5194/bg-18-707-2021
Prouty N. G., Wall M., Fietzke J., Cheriton O. M., Anagnostou E., Phillips B. L., et al. (2022). The role of pH up-regulation in response to nutrient-enriched, low-pH groundwater discharge. Mar. Chem. 243. doi: 10.1016/j.marchem.2022.104134
Rahman S., Tamborski J. J., Charette M. A., Cochran J. K. (2019). Dissolved silica in the subterranean estuary and the impact of submarine groundwater discharge on the global marine silica budget. Mar. Chem. 208, 29–42. doi: 10.1016/j.marchem.2018.11.006
Sadat-Noori M., Tait D. R., Maher D. T., Holloway C., Santos I. R. (2018). Greenhouse gases and submarine groundwater discharge in a Sydney Harbour embayment (Australia). Estuarine Coast. Shelf Sci. 207, 499–509s. doi: 10.1016/j.ecss.2017.05.020
Santos I. R., Beck M., Brumsack H.-J., Maher D. T., Dittmar T., Waska H., et al. (2015). Porewater exchange as a driver of carbon dynamics across a terrestrial-marine transect: insights from coupled 222Rn and pCO2 observations in the German Wadden Sea. Mar. Chem. 171, 10–20. doi: 10.1016/j.marchem.2015.02.005
Santos I. R., Burnett W. C., Chanton J., Dimova N., Peterson R. N. (2009a). Land or ocean?: Assessing the driving forces of submarine groundwater discharge at a coastal site in the Gulf of Mexico. J. Geophys. Res. 114 (C4), C04012. doi: 10.1029/2008JC005038
Santos I. R., Burnett W. C., Dittmar T., Suryaputra I. G. N. A., Chanton J. (2009b). Tidal pumping drives nutrient and dissolved organic matter dynamics in a Gulf of Mexico subterranean estuary. Geochim. Cosmochim. Acta 73 (5), 1325–1339. doi: 10.1016/j.gca.2008.11.029
Santos I. R., Chen X., Lecher A. L., Sawyer A. H., Moosdorf N., Rodellas V., et al. (2021). Submarine groundwater discharge impacts on coastal nutrient biogeochemistry. Nat. Rev. Earth Environ. 2 (5), 307–323. doi: 10.1038/s43017-021-00152-0
Silberberger M. J., Koziorowska-Makuch K., Borawska Z., Szczepanek M., Kędra M. (2022). Disentangling the drivers of benthic oxygen and dissolved carbon fluxes in the coastal zone of the Southern Baltic Sea. Estuaries Coasts 45, 2450–2471. doi: 10.1007/s12237-022-01074-w
Slomp C. P., Van Cappellen P. (2004). Nutrient inputs to the coastal ocean through submarine groundwater discharge: controls and potential impact. J. Hydrol. 295 (1–4), 64–86. doi: 10.1016/j.jhydrol.2004.02.018
Stewart B. T., Santos I. R., R. Tait D., Macklin P. A., Maher D. T. (2015). Submarine groundwater discharge and associated fluxes of alkalinity and dissolved carbon into Moreton Bay (Australia) estimated via radium isotopes. Mar. Chem. 174, 1–12. doi: 10.1016/j.marchem.2015.03.019
Stokowski M., Winogradow A., Szymczycha B., Carstensen J., Kuliński K. (2021). The CO2 system dynamics in the vicinity of the Vistula River mouth (the southern Baltic Sea): A baseline investigation. Estuarine Coast. Shelf Sci. 258. doi: 10.1016/j.ecss.2021.107444
Szymczycha B., Maciejewska A., Winogradow A., Pempkowiak J. (2014). Could submarine groundwater discharge be a significant carbon source to the southern baltic sea? Oceanologia 56, 327–347. doi: 10.5697/oc.56-2.327
Szymczak E., Piekarek-Jankowska H. (2007). The transport and distribution of the river load from the Reda River into the Puck Lagoon (Southern Baltic Sea, Poland). Oceanological Hydrobiological Stud. 36, 103–124. doi: 10.2478/v10009-007-0012-7
Szymczycha B., Borecka M., Białk-Bielińska A., Siedlewicz G., Pazdro K. (2020a). Submarine groundwater discharge as a source of pharmaceutical and caffeine residues in coastal ecosystem: Bay of Puck, southern Baltic Sea case study. Sci. Total Environ. 713, 136522. doi: 10.1016/j.scitotenv.2020.136522
Szymczycha B., Klostowska Ż., Lengier M., Dzierzbicka-Głowacka L. (2020b). Significance of nutrient fluxes via submarine groundwater discharge in the Bay of Puck, southern Baltic Sea. Oceanologia 117–125. doi: 10.1016/j.oceano.2019.12.004
Szymczycha B., Maciejewska A., Winogradow A., Pempkowiak J. (2014). Could submarine groundwater discharge be a significant carbon source to the southern Baltic Sea? Oceanologia 56, 327–347. doi: 10.5697/oc.56-2.327
Szymczycha B., Vogler S., Pempkowiak J. (2012). Nutrient fluxes via submarine groundwater discharge to the Bay of Puck, southern Baltic Sea. Sci. Total Environ. 438, 86–93. doi: 10.1016/j.scitotenv.2012.08.058
Szymkiewicz A., Potrykus D., Jaworska-Szulc B., Gumuła-Kawęcka A., Pruszkowska-Caceres M., Dzierzbicka-Głowacka L. (2020). Evaluation of the influence of farming practices and land use on groundwater resources in a coastal multi-aquifer system in puck region (Northern Poland). Water 12, 1042. doi: 10.3390/w12041042
Taniguchi M., Dulai H., Burnett K. M., Santos I. R., Sugimoto R., Stieglitz, et al. (2019). Submarine groundwater discharge: updates on its measurement techniques, geophysical drivers, magnitudes, and effects. Front. Environ. Sci. 7 (141). doi: 10.3389/fenvs.2019.00141
Testa J. M., Charette M. A., Sholkovitz E. R., Allen M. C., Rago A., Herbold C. W. (2002). Dissolved iron cycling in the subterranean estuary of a coastal bay: Waquoit Bay, Massachusetts. Biol. Bull. 203, 255–256. doi: 10.2307/1543427
Thomsen J., Gutowska M. A., Saphörster J., Heinemann A., Trübenbach K., Fietzke J., et al. (2010). Calcifying invertebrates succeed in a naturally CO2-rich coastal habitat but are threatened by high levels of future acidification. Biogeosciences 7, 3879–3891. doi: 10.5194/bg-7-3879-2010
Tyrrell T., Schneider B., Charalampopoulou A., Riebesell U. (2008). Coccolithophores and calcite saturation state in the Baltic and Black Seas. Biogeosciences 5, 485–494. doi: 10.5194/bg-5-485-2008
Valiela I., Bowen J. L., Kroeger K. D. (2002). Assessment of models for estimation of land-derived nitrogen loads to shallow estuaries. Appl. Geochemistry 17 (7), 935–953. doi: 10.1016/S0883-2927(02)00073-2
Wahl M., Buchholz B., Winde V., Golomb D., Guy-Haim T., Müller J., et al. (2015). A mesocosm concept for the simulation of near-natural shallow underwater climates: The Kiel Outdoor Benthocosms (KOB). Limnol. Oceanogr.-Meth 13, 651–663. doi: 10.1002/lom3.10055
Wang S.-L., Chen C.-T. A., Huang T.-H., Tseng H.-C., Lui H.-K., Peng T.-R., et al. (2018). Submarine groundwater discharge helps making nearshore waters heterotrophic. Sci. Rep. 8 (1), 11650. doi: 10.1038/s41598-018-30056-x
Wang G., Jing W., Wang S., Xu Y.I., Wang Z., Zhang Z., et al. (2014). Coastal acidification induced by tidal-driven submarine groundwater discharge in a coastal coral reef system. Environ. Sci. Technol. 48 (22), 13069–13075. doi: 10.1021/es5026867
Wang X., Li H., Jiao J. J., Barry D. A., Li L., Luo X., et al. (2015). Submarine fresh groundwater discharge into Laizhou Bay comparable to the Yellow River flux. Sci. Rep. 5, 8814. doi: 10.1038/srep08814
Wielgat-Rychert M., Ameryk A., Jarosiewicz A., Kownacka J., Rychert K., Szymanek L., et al. (2013). Impact of the inflow of Vistula river waters on the pelagic zone in the Gulf of Gda´nsk. Oceanologia 55, 859–886. doi: 10.5697/oc.55-4.859
Winde V., Böttcher M. E., Escher P., Böning P., Beck M., Liebezeit G., et al. (2014). Tidal and spatial variations of DI13C and aquatic chemistry in a temperate tidal basin during winter time. J. Mar. Sys. 129, 394–402. doi: 10.1016/j.jmarsys.2013.08.005
Zeebe R. E., Wolf-Gladrow D. (2001). CO2 in seawater: equilibrium, kinetics, isotopes (Elsevier Oceanography Series), ISBN: ISBN: 9780444509468.
Zhang Y., Li H., Xiao K., Wang X. J., Lu X. T., Zhang M., et al. (2017). Improving estimation of submarine groundwater discharge using radium and radon tracers: application in Jiaozhou Bay, China. J. Geophysical Research-Oceans 122 (10), 8263–8277. doi: 10.1002/2017JC013237
Keywords: SGD, submarine groundwater discharge, CO2 partial pressure, Baltic Sea, ocean acidification
Citation: Szymczycha B, Böttcher ME, Diak M, Koziorowska-Makuch K, Kuliński K, Makuch P, von Ahn CME and Winogradow A (2023) The benthic-pelagic coupling affects the surface water carbonate system above groundwater-charged coastal sediments. Front. Mar. Sci. 10:1218245. doi: 10.3389/fmars.2023.1218245
Received: 06 May 2023; Accepted: 24 August 2023;
Published: 14 September 2023.
Edited by:
Margarita Fernández Tejedor, Institute of Agrifood Research and Technology (IRTA), SpainReviewed by:
Wei-dong Zhai, Southern Marine Science and Engineering Guangdong Laboratory (Zhuhai), ChinaJames Sippo, Southern Cross University, Australia
Copyright © 2023 Szymczycha, Böttcher, Diak, Koziorowska-Makuch, Kuliński, Makuch, von Ahn and Winogradow. This is an open-access article distributed under the terms of the Creative Commons Attribution License (CC BY). The use, distribution or reproduction in other forums is permitted, provided the original author(s) and the copyright owner(s) are credited and that the original publication in this journal is cited, in accordance with accepted academic practice. No use, distribution or reproduction is permitted which does not comply with these terms.
*Correspondence: Beata Szymczycha, YmVhdC5zekBpb3Bhbi5nZGEucGw=