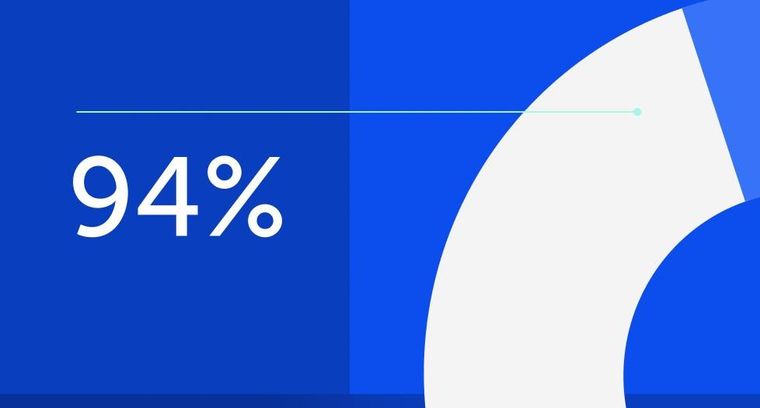
94% of researchers rate our articles as excellent or good
Learn more about the work of our research integrity team to safeguard the quality of each article we publish.
Find out more
ORIGINAL RESEARCH article
Front. Mar. Sci., 12 July 2023
Sec. Marine Fisheries, Aquaculture and Living Resources
Volume 10 - 2023 | https://doi.org/10.3389/fmars.2023.1217792
This article is part of the Research TopicDesign Change to Fishery Independent Surveys: When to Adjust and How to Account For ItView all 14 articles
Fisheries-independent surveys that reliably sample a broad size range of exploited and ecologically important species provide valuable data in support of fisheries management and ecosystem science. The operational consistency of surveys over time and space is fundamental to the interpretation of data in the contexts of population dynamics processes, community interactions, policy impacts, and environmental forcing. However, the need to maintain historic sampling protocols over extended time periods limits the utilization of new technologies that could lead to improved data collection. Survey vessel replacements also become inevitable as the maturity of sampling programs becomes multidecadal. This case study describes the motivational origin, initial design, and redesign of a bottom trawl survey operating in Chesapeake Bay, the largest estuary in the United States. Regional aspirations to consider ecosystem principles in fisheries management aided initial development of the survey, and the need to collect specific data types to support that endeavor impacted several early design elements. Following the beginning years of full-scale survey operations, a consistently evolving awareness of potential areas of improvement for the survey grew from formal efforts to engage with scientific and industry partners on trawl gear design, leverage the program for additional survey opportunities, utilize gear testing technology, and analyze extant data. When the delivery of a new, state-of-the-art research vessel forced the transfer of survey operations to a new platform, all potential changes were incorporated simultaneously. A subsequent paired-tow experiment was conducted to build a calibration database that successfully provided estimates of relative selectivity for routinely sampled taxa. This experience yielded several lessons learned that are intended to aid investigators faced with adopting structural changes to fisheries-independent surveys in the future.
Fish and aquatic invertebrate populations are routinely surveyed using a variety of sampling gears such as trawls, dredges, gillnets, longlines, traps, seines, hydroacoustics, and video photography (Kimura and Somerton, 2006). Regardless of the gear type used, the primary purpose of a fisheries-independent survey is to obtain representative data that allow estimation of key population quantities. In the context of stock assessment, survey data are typically analyzed to estimate indices of relative abundance over a defined spatiotemporal scale. Depending on the survey sampling design, indices can be estimated using design-based methods based on classic sampling theory (e.g., stratified random sampling, Cochran, 1977; Thompson, 2012) or model-based procedures (e.g., generalized linear models and their extensions, Maunder and Punt, 2004; Venables and Dichmont, 2004). These indices are then used as inputs to a stock assessment model under the assumption that the temporal pattern in the indices reflects that of the overall population. In this respect, survey data can be considered a central component of any fisheries management system (Hilborn and Walters, 1992). However, when survey gear effectively samples species that are not exploited, assessed, or managed, the resulting data can form the basis of valuable biological, ecological, and community analyses. These analyses are usually model-based since they often relate survey catches to synoptically measured abiotic and biotic covariates under the broader theme of ecosystem science. These studies may also be structured to help inform policies that fall along the ecosystem-approaches to ecosystem-based fisheries management gradient (Link, 2010; Link and Marshak, 2022).
Irrespective of the objectives motivating analyses of survey data, an assumption typically required for inference is that survey catches are proportional to total population abundance. This concept is formalized as , where is survey catch, E is survey effort, N is total population abundance, and is the catchability coefficient defined as the fraction of the population captured with one unit of effort (Ricker, 1975). Re-arranging yields , which illustrates the proportional linkage between catch-per-unit-effort () and population abundance. Explicit to the expression for is the notion that remains constant over the spatiotemporal domain of sampling. To minimize variability in , a high emphasis is placed on maintaining consistency in field protocols across the life of a survey. Gear configuration parameters, deployment procedures, survey vessel in the case of towed gear, and calendar dates of survey expeditions are intentionally held constant over time to avoid influencing . However, despite the most well executed efforts to ensure operational consistency, it is recognized that can vary temporally due to anthropogenic, environmental, biological, and management processes (Wilberg et al., 2010), or spatially because of heterogeneity in bottom substrate within the sampling domain where towed demersal gear is deployed (Thorson et al., 2013). Examining the constant assumption should therefore be a continual process throughout the life of a fisheries-independent survey, with information coming from analyses of extant data combined with specific process-oriented field studies designed to investigate factors hypothesized to affect catchability.
The operational consistency of a survey to meet the constant assumption can be both an asset and a liability. Data collected in the same manner over a defined sampling frame and in accordance with a valid statistical sampling design is arguably the most valuable aspect of a fisheries-independent survey. The accumulated data streams can provide insight into the synergist effects of population dynamics processes, community interactions, fisheries management impacts (for exploited resources), and environmental forcing over short, medium, and long time periods. However, as a survey matures and its longevity becomes multidecadal, the need to maintain historic sampling protocols limits the utilization of new technologies that could lead to improved data collection. Moreover, for surveys with towed gear, vessel refits and eventual replacements can present challenges to maintaining the integrity of data streams since towed gear performance is often tied to specific design and mechanical characteristics of the survey vessel.
The Chesapeake Bay Multispecies Monitoring and Assessment Program (ChesMMAP) is a relatively long-term (2002 – present) bottom trawl survey designed to provide species-specific, community-level, and trophic interactions data for late juvenile/adult fishes and shellfish in Chesapeake Bay (Latour et al., 2003). Recently, the institution responsible for conducting the ChesMMAP survey took delivery of a newly constructed, state-of-the-art research vessel that possesses significantly more capabilities for conducting fisheries-independent surveys when compared to the original vessel. To take advantage of this modern research platform, the ChesMMAP survey was fully revamped, including modifications to the sampling design, gear package, field deployment protocols, and operations were shifted to the new research vessel. This case study describes the inspiration for ChesMMAP, the rationale and process by which the survey was redesigned, field and analytical efforts to maintain interpretability of data streams given the new sampling platform and gear, and lessons learned along the way.
The Chesapeake Bay is a partially mixed coastal plain estuary located on the U.S. east coast. The bay’s watershed covers an expansive area (164,200 km2) and mean depth is relatively shallow (6.5 m, Kemp et al., 2005). Estuarine circulation is driven by freshwater inputs mainly from northern and western tributaries combined with landward-flowing sea water from the Atlantic. Water temperatures in the bay are dynamic intra-annually and can range from as low as 1-4˚C in winter (Dec-Mar) to as high as 28-30˚C in summer (Jun-Sep). As a result, the bay serves as an important foraging and refuge area for diverse assemblages of both resident taxa and seasonally occurring boreal, temperate, and subtropical fishes (Murdy et al., 1997). Many of those species support economically valuable commercial and recreational fisheries, as well as an array of non-market ecosystem services (Kirkley et al., 2005; Lellis-Dibble et al., 2008; National Marine Fisheries Service [NMFS], 2020).
While the bay remains a highly productive ecosystem, it has experienced significant anthropogenic change since the late 19th century. Eutrophication resulting from increased nutrient inputs has affected water quality, the distribution and density of submerged aquatic vegetation (Orth et al., 2010), hypoxic events (Hagy et al., 2004), and the relative roles of benthic and planktonic processes underlying ecosystem functioning (Kemp et al., 2005). Fishing activities have also had major effects on both resident and seasonally available natural resources in the bay, including cases of stock collapse (Richards and Rago, 1999; Wilberg et al., 2011). Climate change has impacted the bay ecosystem through warming (Ding and Elmore, 2015; Hinson et al., 2022), altered timing of spring phenological events (Thomas et al., 2017), spatiotemporal extent of hypoxic volume (Irby et al., 2018; Tian et al., 2022), and relative habitat utilization among the bay and coastal areas by several taxa (Schonfeld et al., 2022). Additional climate change related effects on the physical, chemical, and biological processes of the bay are expected in the future (Najjar et al., 2010).
Management of fisheries resources important to the Chesapeake Bay region is achieved through a complex jurisdictional framework. State agencies in Pennsylvania, Maryland, and Virginia along with their counterpart in the District of Columbia, and the Potomac River Fisheries Commission (a Maryland-Virginia bi-state agency) each have regulatory authority over fisheries targeting year-round resident species within their respective boundaries. Coastal species that are seasonal bay residents but also inhabit nearshore areas in the Atlantic extending across state boundaries (0-3 nm offshore) are managed by the Atlantic States Marine Fisheries Commission (ASMFC). The home ranges of some ASMFC managed species encompass parts of the exclusive economic zone (EEZ; 3-200 nm offshore) and are co-managed with regional fishery management councils that have federal authority through the Magnuson-Stevens Fishery Conservation and Management Act (Methot et al., 2014). Stock assessments are conducted by various academic and governmental agencies, but regardless of those responsible for the analyses, most assessments incorporate fisheries-independent survey data from the bay.
Surveys of fisheries resources in the Chesapeake Bay have been operating for many decades. The earliest began in 1939 and targeted the eastern oyster (Crassostrea virginica; Wilberg et al., 2011). Finfish surveys were initiated in the 1950s and designed to sample juvenile fishes given the importance of the bay as a nursery area for many mid-Atlantic species. Over time, several additional surveys targeting juvenile and adult life stages of diadromous fishes, bivalves, and crustaceans were developed largely in response to emerging management needs. While many of these surveys are longstanding and provide valuable information, the jurisdictional boundary between Maryland and Virginia has historically hindered efforts to develop comprehensive, bay-wide sampling programs.
During the late 1990s and early 2000s, significant attention was focused on considering ecosystem principles in U.S. fisheries management both federally and across many local sectors. At the national level, the NMFS Ecosystem Principles Advisory Panel (NMFS Panel) produced a report outlining recommendations for implementing ecosystem philosophies, goals, and policies in U.S. fisheries conservation, management, and research (National Marine Fisheries Service [NMFS], 1998). Within the Chesapeake Bay region, similar technical documents were developed that summarized perspectives and rationale for incorporating multispecies and ecosystem considerations into fisheries management (Miller et al., 1996; Fernandez and Leach, 1998). In response to key recommendations from the NMFS Panel, a comprehensive prototype fisheries ecosystem plan (FEP) was developed to provide strategic guidance for ecosystem-based fisheries management in Chesapeake Bay and information on the function and structure of the bay ecosystem (National Oceanic and Atmospheric Administration Chesapeake Bay Fisheries Ecosystem Advisory Panel [NOAA CBFEAP], 2006).
Scientific products that support ecosystem principles require additional data types when compared to those needed for traditional stock assessments. The supporting technical documents and FEP highlighted key data gaps, despite the region having several long-term surveys and a rich understanding of the physical, chemical, and biological processes of the bay. Most notable were data types necessary to develop multispecies and ecosystem models, namely bay-wide information on species abundances, age/size composition, growth and mortality rates, and trophic interactions. The need for these data types along with the regional interest in ecosystem management inspired the design and implementation of ChesMMAP.
Conceptualization of ChesMMAP began in 2001 with a review of several existing fisheries-independent sampling programs, including fish trawl surveys conducted by ICES in the North and Baltic Seas, the Northeast Fisheries Science Center (NEFSC) in the northwest Atlantic, and the Alaska Fisheries Science Center in the Gulf of Alaska and Bering Sea (Latour et al., 2003). General consideration was given to vessel and trawl gear specifications, temporal and spatial sampling frequency (acknowledging that Chesapeake Bay is geographically much smaller), data types collected, and onboard data collection processes. The intent behind gathering this information was to build familiarity with other successful programs with similar scientific objectives, and to begin shaping design elements for ChesMMAP.
Initial tactical decisions focused on five key areas: identifying a survey vessel, choosing the trawl gear package, sampling design, onboard catch processing logistics, and staffing. All these areas were evaluated with respect to the financial resources available for operations, and choices often reflected tradeoffs among what was considered ideal versus what was practical. After reviewing the specifications of several vessels in the Chesapeake Bay region with research vessel (R/V) designations (length overall, operational parameters, propulsion, electrical service, working deck space, wet and dry lab space, and berthing), the R/V Bay Eagle was selected as the survey platform. None of the available research vessels were ideal platforms for conducting trawl operations aimed at sampling larger, more mobile fishes and invertebrates, however, among those in the area, the R/V Bay Eagle was the only one that satisfied the very minimum specifications necessary for the survey. Owned and operated by William & Mary’s Virginia Institute of Marine Science (VIMS), this vessel is a 19.8 m crewboat with a 400 nm range and 3-4 day endurance that was retrofitted to conduct scientific research. Beam size and available aft deck space did create an upper limit on trawl net size and required a single towing warp deployment with a bridle.
To guide selection of the gear package, information was gathered on the combinations of vessel sizes and gear specifications used by other trawl surveys operating in the region, as well as from those used by the commercial shrimp fishery in the U.S. southeast. Following consideration of several options, the chosen gear package included a 13.7 m four seam bottom trawl with 15.2 cm stretch body mesh and 7.6 cm stretch cod end mesh constructed from twisted nylon twine. The net was equipped with a looped chain sweep for simplicity and to aid adherence to the bottom, and hydroacoustic wing and headrope sensors to provide data for area/volume swept estimation. Accompanying the net was a pair of standard 1 m2 steel vee-doors designed to achieve spreading primarily through ground sheer. The larger body and cod end stretch meshes were intended to mitigate the pressure wave created at faster tow speeds thereby increasing the capture probability of larger, more mobile animals.
The need for a bay-wide survey led to defining the sampling frame for ChesMMAP as the bay mainstem in both Maryland and Virginia (3900 km2 survey area). Although the bay’s major tributaries represent important habitat for fishes and invertebrates, cost analyses associated with sampling a spatial area larger than the mainstem against those of increased frequency of cruises per year favored the latter, particularly because of the seasonally dynamic nature of the Chesapeake Bay fish community and the goal to collect information on as many species as possible. Accordingly, survey cruises occurred bimonthly from March to November, and sampling followed a random stratified design with stratification based on region (five 30-minute latitudinal strata) and depth (three strata: 3.0 – 9.1, 9.1 – 15.2, and >15.2 m; Figure 1A). Allocation of sampling effort was proportional to stratum surface area, and 20-min tows were made with the current (initial gear testing revealed the net frequently lost bottom contact when towing against the current, and vessel speed was adjusted when towing with the current to maintain optimal gear geometry based on net mensuration measurements). Wingspread and headrope height were combined with the vessel GPS track to calculate swept area/volume. During the first few years of the survey, a full cruise consisted of 90 sampling sites, however, that target was reduced to 80 based on analyses that indicated such a reduction did not lead to significant losses in precision of estimated relative abundances.
Figure 1 Sampling frames and stratification schemes for the Chesapeake Bay Multispecies Monitoring and Assessment Program (ChesMMAP) bottom trawl survey (A) historically, 2002-2018 and (B) currently, 2019-present. Numbers denote regional strata separated by horizontal lines and the shaded bathymetry shows depth zones.
A central philosophy of ChesMMAP is to maximize the data collected at each sampling site. Therefore, each trawl catch is sorted and measured for aggregate weight, count, and individual specimen lengths by species or size-class if distinct classes within a particular species are evident. A subsample of each fish species (excluding bay anchovy, Anchoa mitchilli, and striped anchovy, A. hepsetus) or size-class is further processed for weight, sex, macroscopic maturity stage, and material for aging and diet composition analysis is preserved and returned to the laboratory for processing. For a few species, additional material is preserved for disease and reproductive biology analysis. More recently, sampling of the benthos and zooplankton community has been added to expand the dimensions of the bay ecosystem for which information is collected. Opportunistic sampling, in the form of additional trawl hauls, biological sample acquisition, and data collection, has been conducted as needed throughout the survey history to support studies conducted by researchers, and particularly students, both within and external to VIMS.
As noted above, while institutions typically make every effort to minimize spatiotemporal variation in survey catchability through standardization of sampling protocols, there are times when large changes to survey procedures are unavoidable. The Northeast Fisheries Science Center (NEFSC) Bottom Trawl Survey took delivery of a new survey vessel in 2007 and used the opportunity to implement several new technologies meant to enhance sampling consistency, including improved survey trawl gear (Miller, 2013). Specifically, the NEFSC convened a panel of commercial fishers, trawl manufacturers, and fisheries scientists in 2003 to develop a fishing system designed to maintain a more consistent trawl geometry (i.e., headline height and wingspread), sample a variety of fishes and invertebrates across a broad size range, and be of an appropriate scale for the new research vessel (Johnson and McCay, 2012). The final design was a three-bridle, four-seam bottom trawl that measured 23.3 m along the headline with a 48 m circumference fishing circle. The body of the net was comprised of both 6 cm and 4 cm stretch-mesh polyethylene webbing with a 2.54 cm knotless nylon lined codend and a sweep made of 40.6 cm rubber disks.
At the same time, the ASMFC had partnered with VIMS and several state agencies to develop the Northeast Area Monitoring and Assessment Program (NEAMAP) Inshore Trawl Survey, which was intended to sample the coastal ocean of the Mid-Atlantic Bight given that the new NEFSC vessel would no longer be able to conduct operations in these shallow environments. NEAMAP chose to adopt this three-bridle, four-seam bottom trawl to maintain consistency with the redesigned NEFSC survey, although the sweep was comprised of smaller, 7.6 cm rubber disks since the seafloor in the NEAMAP sampling frame has very few naturally occurring obstructions.
VIMS began sampling with this trawl on NEAMAP in 2007 (Gartland et al., 2023), while the NEFSC Bottom Trawl Survey formally transitioned to this fishing system in 2009 (Miller, 2013). Given the remarkable consistency of the trawl geometry and the diversity and quantity of resulting catch recorded by both surveys, in 2009 ChesMMAP personnel contacted the aforementioned trawl manufacturers and a subset of the commercial fishers referenced above to inquire as to whether a smaller version of this fishing system could be developed for sampling in Chesapeake Bay. The result was a trawl that was identical in design to those used by NEAMAP and the NEFSC, but that measured 11.2 m along the headline with a 24 m circumference fishing circle and a sweep made of 3.8 cm rubber disks, and thus was effectively half of the size.
Prior to conducting field trials with this new trawl net, VIMS commissioned the construction of 1:6 scale models of both the original ChesMMAP trawl and the new trawl, and these model nets were subjected to flume tank trials at Memorial University in St. John’s, NL (Figures 2A, B). Given the high costs typically associated with vessel time, flume testing of survey trawls represents a cost-effective approach to evaluating candidate gears prior to conducting sea-trails (Winger et al., 2006). Relative to the model of the original ChesMMAP trawl, the new net model maintained a more stable geometry and higher headline height over a wider range of simulated current speeds, and it also experienced more consistent water pressures across the net body (taught webbing throughout). When combined with the smaller mesh size, these results implied catchability should be higher and more consistent (Winger et al., 2010).
Figure 2 Photos of 1:6 scale models of the (A) original ChesMMAP survey trawl net (13.7 m four seam bottom trawl with 15.2 cm stretch body mesh and 7.6 cm stretch cod end mesh) and (B) the new ChesMMAP survey trawl (three-bridle, four-seam bottom trawl that measures 11.2 m along the headline with a 24 m circumference fishing circle and a 3.8 cm flat sweep) in the flume tank Memorial University in St. John’s, NL. December 2009.
The new net was coupled with a set of 0.88 m2 high-efficiency, cambered trawl doors and limited field-trials were conducted during 2010 and 2011 on the R/V Bay Eagle. Members of the commercial fishing industry were instrumental in the execution of these early trials, as they provided valuable advice on the appropriate rigging and deployment of this more complex trawl, and assisted survey personnel with identifying a trawl door configuration that would consistently yield optimal net geometry. Measurements from net mensuration gear during these sea-trials confirmed that headline height and wingspread values were half of those observed for the net used by NEAMAP and the NEFSC, and this new trawl appeared to collect a greater diversity of taxa, a broader size range of animals, and a much larger quantity of catch relative to the original ChesMMAP trawl net (unpubl. data). Although this new trawl appeared to yield a more consistent and robust sampling of the ecological community inhabiting Chesapeake Bay, transitioning the survey to this new fishing system on the R/V Bay Eagle would have incurred relatively large costs and posed several logistical challenges. The preferred deployment method for this trawl calls for a dual-warp design, which would have required the acquisition and installation of an additional winch on the vessel, as well as extensive structural modifications to the sampling platform. Given the expected increase in the diversity and quantity of catch, it would also have been necessary to construct and install at least two additional data collection workstations on the aft deck. Taken together, these two modifications would have been very difficult to accommodate, given the already limited deck space. Further, efforts to generate calibration coefficients between the original and new trawls would necessarily have needed to follow a single-vessel, paired-tow design, and the R/V Bay Eagle simply did not have the capacity to accommodate both fishing systems onboard simultaneously, let alone to rapidly switch between the two as would be required during a calibration experiment. Fortunately, VIMS acquired funds necessary to construct a new, state-of-the-art research vessel shortly thereafter, and thus implementation of this new trawl was suspended until vessel delivery so that all major changes to the survey could be adopted concurrently.
The new VIMS vessel, the R/V Virginia, was delivered in October 2018 and is a 28.3 m ship that has a 1500 nm range, 10 day endurance, and was designed and equipped to support a myriad of research activities in Chesapeake Bay and along the U.S. east coast. ChesMMAP sampling operations were transferred to this platform along with the new dual-warp fishing system as soon as it became available for charter in the early summer of 2019. While some sampling programs have decided to defer the implementation of survey changes until after paired-tow experiments were completed and calibration coefficients for key species were estimated (e.g., Miller, 2013), it was decided to make all ChesMMAP changes immediately while concurrently initiating a paired-tow calibration experiment. By implementing changes in this way, the benefits of the improved trawl net (i.e., increases in faunal diversity and size ranges of catch) were able to be realized as soon as was practicable. A drawback, however, was that unanticipated but extended setbacks in completing the calibration experiment (see Section 4.1) prevented the release of data for stock assessments given the need for calibration coefficients to link the time-series collected pre- and post-2019.
Since 2019, trawl sampling in the mainstem of Chesapeake Bay (ChesMMAP) and across the continental shelf in the Mid-Atlantic and New England (NEAMAP, NEFSC) has occurred using a consistent net design. The duration of a standard trawl haul and the target vessel speed over ground during a ChesMMAP tow remained unchanged from the original survey design. The safe operating depth of the R/V Virginia is approximately 6 m, however, meaning that it was no longer possible to sample the shallower areas of the original ChesMMAP sampling frame. In response, this logistical challenge was used as an opportunity to redefine and subsequently re-stratify the ChesMMAP survey area. Specifically, analyses (design- and model-based estimation of relative abundance indices and associated uncertainties) of post-stratified extant catch data were conducted to identify four new latitudinal strata that are each subdivided into two depth strata (6.0 – 12.2, > 12.2 m; Figure 1B).
Given the increased daily charter costs associated with the R/V Virginia, maintaining the original sampling frequency was not possible. Therefore, analyses of extant data were conducted to examine the spatiotemporal distribution of migratory taxa, and results were compared against available financial resources to evaluate tradeoffs in the annual allocation of sampling effort seasonally and spatially. Efforts were also directed at understanding potential impacts of sampling effort changes on the data streams routinely supplied to stock assessments. Four ChesMMAP survey cruises now occur annually during the months of March, June, September, and November. Sampling during June and September is conducted throughout the mainstem of the bay. March cruises occur in the two northernmost latitudinal strata to sample key anadromous fishes during their spring spawning migrations, while November cruises are limited to the two southernmost strata, as various taxa congregate in the lower bay prior to their migration to overwintering habitats on the continental shelf.
Data used to support the estimation of calibration coefficients for fishes and invertebrates routinely sampled by ChesMMAP were collected through a series of 15 research cruises (hereafter, calibration cruises) conducted from June 2019 – November 2022. Each calibration cruise occurred after the completion of a survey sampling event, and the sites sampled were those associated with the most recent survey. Thus, the data used to estimate calibration coefficients for the various taxa were collected following a stratified random sampling design. Approximately five to seven days elapsed between the sampling of a site during a survey and a subsequent calibration cruise to minimize any disturbance effects (e.g., Lewy et al., 2004). While a more-costly approach, this temporal separation of survey and calibration cruises ensured that the survey data would not be impacted by the presence of a second vessel sampling in close proximity (Brown et al., 2007).
At a given sampling site, both the R/V Virginia and R/V Bay Eagle conducted a trawl haul concurrently (i.e., a paired-tow). Both vessels towed in the same direction and were separated by approximately 350 m. Sampling on the R/V Virginia occurred using the new fishing system while the original gear was deployed from the R/V Bay Eagle. Side-by-side positions of the vessels were randomized and a total of 516 paired-tows were completed. Date and time of sampling was denoted at the outset of each paired-tow, and position (latitude and longitude) was recorded by each vessel throughout the tow. The headline height and wingspread of each trawl were recorded during each tow, and wingspread data were coupled with tow distance to calculate the area swept by each trawl at a given site. For each vessel, resulting catches were sorted by species, and aggregate weight, count, and individual length measurements were recorded for each. Over the course of this field experiment, a total of 97 fishes and 20 invertebrate taxa were collected, where 24 fishes and six invertebrate species were unique to the R/V Virginia utilizing the new fishing system and seven fishes and zero invertebrates were unique to the R/V Bay Eagle. Three notable events delayed the completion of the field sampling for this calibration experiment by almost a year; two separate, major mechanical failures on the R/V Bay Eagle resulted in the loss of approximately seven months of sampling, and the COVID-19 pandemic led to the suspension of field operations for nearly four months.
Intercalibration of the two vessel-trawl combinations was based on applying log-Gaussian Cox processes to the paired-tow data (following Thygesen et al., 2019). This approach models the size distribution of the population at each sampling site and the size-structured clustering of animals at small temporal and spatial scales to estimate selectivity ratios across the domain of observed size classes. By utilizing a Poisson probability distribution for the catch numbers conditional on latent log-Gaussian variables, the method allows for overdispersion and correlation between catch counts in neighboring size classes. The model structure is as follows:
where for site , gear , and size class , is the number of individuals captured, is the area swept, is the relative size selectivity (on log scale) such that , is the log-density that characterizes the population size distribution encountered by both gears, and is the variability in the size composition at small temporal and spatial scales (independent components unique to each gear). The quantities , , and are random variables such that and are modeled as random walks over size classes and is the sum of a white noise (WN) process and a zero-mean first-order autoregressive (AR) process, (see Thygesen et al., 2019 for details).
The model estimates the fixed effect parameters , , , , and (correlation coefficient associated with the AR process) along with a large number of random effects: , , and have , , and parameters, respectively. Models were fitted using the R package gearcalib (available at github.com/Uffe-H-Thygesen/Intercalibration). This package applies the Laplace approximation to integrate out the unobserved random effects , , and thus yielding a likelihood function defined by the fixed effect parameters. After the likelihood is maximized and the fixed effects are estimated, the posterior modes of the random effects , , and are reported, where those associated with are of primary interest. Optimization of the likelihood and use of the Laplace approximation was accomplished with the Template Model Builder (TMB) package (Kristensen et al., 2016).
For illustrative purposes, the log-Gaussian Cox processes model was applied to the paired-tow data of four species: Atlantic croaker (Micropogonias undulatus), striped bass (Morone saxatilis), summer flounder (Paralichthys dentatus), and female adult blue crab (Callinectes sapidus). These species were chosen because they support valuable fisheries, differ morphologically, and have contrasting habitat characteristics. Prior to modeling, the paired-tow data were filtered to remove samples collected from months and strata that consistently yielded near zero catches by both vessels owing to the notion that not all species are available for sampling during all months of the year or abundantly distributed in all strata. Summaries of the data analyzed indicated that the R/V Virginia captured considerably more total animals (except for female adult blue crab likely due to differing trawl sweep configurations between the gears), wider size ranges (except for striped bass due to a few very large animals collected by the R/V Bay Eagle), and animals more frequently as evidenced by consistently higher proportion positive tows (probability at least one animal is sampled; Table 1; Figures 3A–H).
Table 1 Sampling and catch summaries of the paired-tow data for the four selected species. Italicized subscripts denote the R/V Bay Eagle (BE) and R/V Virginia (VA).
Figure 3 Vessel-specific size composition (first column) and proportion positive in relation to size (second column) data summaries from the paired-tow calibration experiment for (A, B) Atlantic croaker, (C, D) striped bass, (E, F) summer flounder, and (G, H) adult female blue crab.
Application of the log-Gaussian Cox processes models was generally successful with the caveat that the white noise component of the residuals for all species could not be identified, and as a result, estimates of approached zero ( order of magnitude). This situation was frequently encountered in a simulation study conducted to verify the model (Thygesen et al., 2019) and is indicative of the broader challenge of estimating variance components in hierarchical models (Auger-Méthé et al., 2016). Within the modeling framework, the random variable is intended to represent small-scale fluctuations in local abundance. Since the paired tows occur at slightly different locations, it is possible for one gear to encounter or miss an aggregation of animals within the overall sampling space of the two gears. Depending on the information content of the paired-tow data, could also represent random fluctuations in gear selectivity, which creates a situation where the two effects are confounded. In this case, a high catch in one gear could be the result of encountering an aggregation or because it performed better than average at the sampling site (Thygesen et al., 2019).
This confounding appears to be present in the paired-tow data analyzed herein. On a tow-by-tow basis for the species considered (and several others analyzed but not presented), the gear on the R/V Virginia consistently met or outperformed that of the R/V Bay Eagle in terms of encounter rates and total catch (Table 1). Thus, fluctuations in local abundance associated with encountering or missing animal aggregations were not distinguishable from the comparative superiority of the R/V Virginia fishing system, and consequently was not estimable. To further explore this concept, a small simulation study was conducted where the species-specific catches of the two vessels were randomly interchanged and then analyzed with the fully saturated log-Gaussian Cox processes model. For over half of the randomly modified data sets, the parameter was estimated well. Therefore, only reduced models that excluded the fixed effect were considered for analysis, and all fixed effects parameters associated with the reduced models were generally well estimated (Table 2).
Table 2 Parameter and standard error estimates associated with the log-Gaussian Cox processes models fitted to the paired-tow data for the four species.
The resultant estimated relative size selectivity curves confirmed trends in the raw data in that most of the estimates exceeded 1.0 for each of the four species (note, the magnitude of the estimates was quite large for Atlantic croaker and summer flounder, Figures 4A–D). While the calibration experiment and data analyses appear to be successful, questions remain about how best to treat the ChesMMAP survey data moving forward, particularly for stock assessments. Developing time-series of indices that span the vessel changes could be accomplished by converting R/V Bay Eagle survey data into R/V Virginia units using the above relative size selectivity estimates, however, this approach is not without drawbacks. First, since conversion of survey data requires multiplying the size-specific catches by the relative size selectivity estimates, the issue of how to convert the historic R/V Bay Eagle zero observations emerges. Due to the superiority of the new trawl net, numerous paired-tows resulted in zero R/V Bay Eagle catches and nonzero R/V Virginia catches for many species (and vice versa but to a much lesser extent). From the paired-tow data where the R/V Bay Eagle catches were zero, a model-based analysis of the associated R/V Virginia catches (e.g., generalized additive models) could potentially guide which historic zero catches should remain unchanged or be converted. Second, applying the relative size selectivity estimates (and potentially results from the analyses of zeros) implies the associated estimates of uncertainty need to be incorporated into analyses that yield indices of relative abundance. The confidence intervals around the above relative size selectivity curves were quite wide for some size classes, and when this uncertainty is combined with natural survey observation error (perhaps Monte Carlo or bootstrapping), the overall uncertainty estimates of the relative abundance indices could be quite high.
Figure 4 Relative selectivity (blue lines) of the trawl gear on the R/V Virginia (new fishing system) with that on the R/V Bay Eagle (original fishing system) for (A) Atlantic croaker, (B) striped bass, (C) summer flounder, and (D) adult female blue crab. For the new fishing system, values above the horizonal lines indicate higher selectivity, values below indicate lower selectivity, and values at the horizonal lines denote no selectivity differences. Shaded areas are 95% confidence intervals.
Other model-based approaches could potentially be utilized to estimate indices of relative abundance that span the full time-period, provided there is sufficient temporal overlap among the vessel-specific data sets. As noted previously, the ChesMMAP calibration sites were selected according to the underlying stratified sampling design, and because the calibration experiment transpired over several years, it is possible to view the paired-tow data as an extension of the R/V Bay Eagle data and supplemental to the ongoing R/V Virginia time-series. This temporal overlap facilitates application of models that can structurally accommodate distinct catchability and vessel effects (e.g., vector autoregressive spatio-temporal (VAST), Thorson, 2019), or the estimation of vessel-specific indices that could then be reconciled with a time-series approach (e.g., dynamic factor analysis, Peterson et al., 2021). However, both options require a background in advanced statistical modeling and computer coding as the details and implementation of these approaches are not trivial.
Lastly, there are options to bridge vessel changes at the level of the stock assessment model. Time-series of relative abundance could be estimated separately for the two vessels, which could then be inputs to an assessment model with different selectivity patterns and likelihood components (this becomes more practical once the R/V Virginia data gain longevity). Or perhaps the stock assessment model could be configured to accommodate the survey data from both vessels and the paired-tow data to internally estimate relative selectivity values along with other assessment parameters (an area of future research). In practice, however, choosing among the analytical options will likely depend on the specific goals and methodological approaches of future applications of the ChesMMAP survey data.
The ChesMMAP survey is now in its fifth year of sampling using the new trawl package, vessel, and survey design, and calibration coefficients needed to link the contemporary catch data to those collected prior to the 2019 conversion are available for most of the key species sampled. The new trawl has yielded much larger catches relative to the original fishing system, but more importantly it has sampled a greater diversity of taxa and a broader size range of animals for most species. The R/V Virginia has proven to be an ideal platform to accommodate this survey and associated larger catches, while adjustments to the spatiotemporal extent of sampling effort have improved operational efficiency. Indeed, implementing these changes to ChesMMAP incurred some significant costs, however, the data generated now provide a more robust and likely more consistent characterization of the living marine resources in Chesapeake Bay. Further, during the process of redesigning ChesMMAP, our successes, missteps, and reflections on the original survey design revealed five important lessons that could be useful in future situations when it becomes either necessary or desirable to alter survey procedures. Several of these lessons are also applicable when developing a new survey.
Network development and open, frequent communication with external partners provided the avenue for the initial discovery and successful implementation of the new trawl gear package adopted by ChesMMAP. While the design, size, and performance of several trawl gears used by successful fisheries-independent surveys and the shrimp fishery in the U.S. southeast were researched when originally designing ChesMMAP, this review was based solely on existing documentation and available expertise within VIMS. Involvement with the NEFSC Trawl Survey Advisory Panel (TSAP) during the early 2000s provided an opportunity for direct engagement with commercial fishers, trawl gear manufacturers, and scientific colleagues conducting fisheries-independent surveys. During these meetings, researchers outlined the desirable characteristics of a scientific survey trawl and the industry members (i.e., fishers and gear manufacturers) designed a gear package to match those criteria as closely as possible. The breadth and depth of industry expertise yielded a trawl net that maintained a very consistent trawl geometry over a broad range of depths and seafloor conditions, and in turn yielded catches that reflected a greater diversity of taxa and a broader size range of animals. Exposure to this gear development process in terms of the relationships established with the industry members and other survey scientists, and the successful implementation of the TSAP trawl on the NEFSC Bottom Trawl Survey and NEAMAP led to inquiries about designing a smaller version of this trawl for ChesMMAP. Without the development of these networks and cultivation of relationships with industry and peers in the scientific community, replacing the original trawl gear with the more efficient fishing system now used by ChesMMAP would have been unlikely.
Including key industry members in the new trawl design resulted in appreciable ‘buy-in’ by fishers on the successful implementation of this gear by all three trawl surveys. Prior to the initial field trials of the new gear package on the R/V Bay Eagle, two local fishers donated their time to assist survey personnel with the proper configuration of the wires that connect the net to the trawl doors, given that the more-complicated three-bridle design uses eight wires in total to make these connections. They also provided advice on appropriate setting and hauling procedures, including valuable tips on how to prevent these wires from becoming entangled during these processes. Based on catch rates observed by the NEFSC and NEAMAP with this trawl design, catches with the new ChesMMAP net were expected to be larger than those typically observed with the original gear. The industry members shared approaches that they use to retrieve very large catches and outfitted the ChesMMAP trawl with all rigging materials needed to perform these more-complicated retrievals at no cost. During the first day of field trials, it was clear that the trawl doors were not performing as intended, because wingspread measurements were much lower than expected and examination of the wear patterns on the doors revealed that they were lying flat on the bottom for at least part of each tow. An industry member volunteered to advise operations during the second day of these field trials, and by the third haul had identified the proper adjustments to the trawl warp connections and door backstrap chains to achieve the optimal geometry of the survey trawl. Thus, when selecting and implementing a new sampling gear for a fisheries-independent survey, either as part of a survey transition or development of a new sampling program, partnering with the fishing industry and other survey scientists to benefit from the experience and expertise of both groups is strongly recommended.
Prior to conducting the initial field trials with the new ChesMMAP trawl on the R/V Bay Eagle, scale models of both the new and original nets were constructed, and their performance evaluated in a flume tank located at Memorial University in St. John’s, NL. Three survey personnel traveled to this facility in early December 2009, and the performance of the model trawls was documented over a two-day period. Specifically, the geometry of the trawls and the variability in both net wingspread and headline height were measured over a range of simulated towing speeds, rigging configurations, trawl door designs, and codend fullness (representing varying catch sizes). These data clearly demonstrated the superiority of the new net with respect to maintaining a consistent trawl geometry while achieving a greater headline height across the full range of conditions tested. Visual evaluation of the trawl in the flume also revealed that the new net maintained a consistent shape throughout the trials, while the original net was somewhat disfigured at the center of the headline and between the wings of the net and the footrope. Further, the sweep of the new net remained in contact with the bottom across the full range of towing speeds (1.3 – 1.7 m s-1), while the original gear would rise off bottom at higher speeds.
The encouraging flume data of the scale model of the new net prompted purchase of two 11.2 m three-bridle, four-seam trawls for field testing in fall 2010. The total expenses associated with the flume trials, including construction of the model trawls, travel, flume rental, and personnel time, were approximately equal to one day of vessel costs. When adding procurement costs of the new trawl package, associated sampling supplies, and personnel compensation, the daily cost of field testing far exceeded the total for the flume trials. Had the new trawl performed poorly, the flume trials would have yielded appreciable cost savings since field testing would have been unnecessary. Moreover, visual evaluation of both net models in the flume provided insight on their overall shape and ability to maintain bottom contact that would have been extremely difficult to acquire through field trials. Given the valuable information gained from the flume, it is recommended that flume testing be conducted on all currently used trawls for performance data and any candidate trawls prior to field operations.
Results from the flume trials and initial field testing provided strong support for adopting the new gear package on ChesMMAP. As noted above, transitioning to this gear on the R/V Bay Eagle would have incurred significant costs and presented substantial logistical challenges, but doing so was not entirely unachievable. However, shortly after flume testing, VIMS acquired funds to design and build the R/V Virginia, and accommodating the new ChesMMAP fishing system influenced many design elements. Given the pending arrival of this vessel and plans to remove the R/V Bay Eagle from the VIMS fleet shortly thereafter, it was decided to delay incorporating the new trawl into ChesMMAP until operations could be shifted to the new sampling platform. This conclusion emerged following careful evaluation of both the financial costs associated with a transition and impacts on the time-series of survey data. Had changes been made to ChesMMAP in two steps, first implementing the new trawl on the R/V Bay Eagle and then moving survey operations to the R/V Virginia once available, two calibration experiments (one at each step) would have been necessary, and financial resources required to maintain linkages across the full time-series would have been approximately doubled. Further, two considerable sources of uncertainty would have been introduced into the time-series, one from each of the respective calibration efforts, which could have unnecessarily diminished the utility of the survey dataset.
Once the decision was made to delay implementation of the new survey trawl until operations transitioned to the new research vessel, all aspects of the ChesMMAP sampling design were more formally evaluated for possible improvements. Although draft restrictions of the R/V Virginia forced abandonment of the shallowest sampling locations within the original sampling frame, analyses of extant data supported adjusting the boundaries of the latitudinal regions and depth strata (modest changes in relative abundance patterns and associated uncertainties). Further, the increased daily rate of the new vessel prompted evaluation of the spatiotemporal patterns of the extant catch data to identify redundancies and sampling season and region combinations that historically yielded scant abundance, life history, and trophic information. The resulting stratification changes and associated reallocation of sampling effort were implemented concurrently with the transition of the survey to the new vessel and trawl gear package, and thus the impacts of all adjustments were captured by the calibration coefficients generated from a single paired-tow experiment. Thus, it is recommended to view periods of survey transition as unique opportunities to evaluate and improve as many aspects of the sampling operation as possible, and subsequently implement all changes simultaneously so that the cumulative impacts of these new procedures are reflected in one comprehensive calibration experiment.
Whether changes to sampling procedures are implemented by choice or out of necessity, it is critical that all costs, both financial and non-monetary, be considered. Total costs can be substantial, and if available financial and human resources are not sufficient to meet expected costs, it will be necessary to critically evaluate trade-offs among the proposed survey adjustments and identify those that are both achievable and that provide the greatest benefit to the sampling program. Among the largest costs incurred during a survey transition are those associated with the calibration experiments. In the case where the original vessel is retained but a new trawl package is utilized, data to generate calibration coefficients are collected through a single-vessel, paired-tow design where the original and new gears are hauled in succession at several sampling locations. Because the net that is towed first usually alternates among sampling sites, it is necessary to conduct this experiment separately from routine survey operations because disturbances from towing the alternate trawl first could negatively impact the time-series of survey data. This separation of survey and calibration activities typically requires substantial financial resources to support the additional ship and personnel time.
When both the vessel and survey trawl are to be replaced, the two platforms can conduct simultaneous paired-tows to generate data needed calibration information. Costs associated with this approach can be reduced by coupling the paired-tow experiment with survey operations such that one vessel is conducting the survey while the other samples concurrently and in close proximity at either all or a subset of sites. However, the presence of the second vessel could create disturbance effects that impact survey catches, so whenever possible separating these paired-tow experiments from the survey operations is recommended. Regardless of the approach, personnel costs are approximately double those associated with routine survey operations since it is necessary to employ a science team to process catches on two vessels. At an institutional-level, fleet maintenance costs will increase (at least temporarily) between the time the new vessel is delivered and the previous vessel is retired, as it is imperative that both remain fully operational so that the paired-tow experiment can be completed as quickly as possible. Further, when the decision is made to transition the sampling procedures immediately and conduct paired-tows concurrently, which was the approach adopted herein, it is important to recognize that there can be delays in providing updated indices of relative abundance to stock assessment activities. This information gap represents a cost to the assessment management processes.
Many times, when there is a change in sampling platform, the replacement vessel is often newer, larger, requires additional vessel crew, and therefore is more expensive to operate. For ChesMMAP, funds available to the survey remained relatively constant, which required reexamination of the spatiotemporal distribution of sampling effort. Further, when the vessel is coupled with a new survey trawl package, there are obvious start-up costs associated with the acquisition of the net, trawl doors, and associated rigging materials. If the new trawl is much more efficient than the original gear and average catches increase appreciably, the program will realize increased long-term costs often in one of two ways. Either additional catch processing time will be needed at each sampling site which will lengthen cruises and increase vessel costs, or additional scientific personnel will be needed to process the larger catch volumes. Following an evaluation of the trade-offs between vessel and personnel costs, increasing the scientific crew from four on the R/V Bay Eagle to six on the R/V Virginia was necessary. While ChesMMAP now generates a more robust, consistent sampling of the Chesapeake Bay ecosystem, the immediate and longer-term costs associated with the transition were quite large, and it is recommended that researchers think broadly and estimate all associated costs prior to initiation changes to survey sampling procedures.
The decision to explore implementation of the 11.2 m three-bridle, four-seam bottom trawl on ChesMMAP was motivated in large part by reports of the gear performance and catch composition generated by the larger version of this gear used by the NEFSC and NEAMAP. Likewise, following successful flume and initial field trials with the new ChesMMAP net, data, results, and experiences rigging, deploying, and retrieving this gear were shared through presentations to regional management councils and commissions, annual progress reports to funding agencies, and informally to colleagues. The ChesMMAP net design has since been adopted by a United States Geological Survey bottom trawl survey in Lake Erie and is under consideration by two additional surveys, one operating in the southeast U.S. Atlantic waters and the other in Southern New England. Using a standardized trawl design on multiple surveys that yields robust, consistent sampling of ecological communities facilities important cross-system comparisons of programmatic datasets, and so as surveys are developed or undergo transitions, broad communication of experiences and results to promote coordination and standardization of sampling procedures where possible is recommended.
The raw data supporting the conclusions of this article will be made available by the authors, without undue reservation.
The animal study was reviewed and approved by William & Mary Institutional Animal Care and Use Committee.
All authors contributed to the design of the original ChesMMAP survey, the subsequent redesign following gear and vessel changes, and the design of the paired sampling experiment. CB and JG organized the paired-tow database and RL performed the statistical analyses with input from JG. RL and JG co-wrote the first draft of the manuscript and incorporated comments provided by CB. All authors contributed to the article and approved the submitted version.
Financial support over the duration of the ChesMMAP survey was provided by the NOAA Chesapeake Bay Office, the Virginia Environmental Endowment, the U.S. Fish and Wildlife Service, and the Virginia Marine Resources Commission.
The authors wish to acknowledge the field and laboratory efforts of past and present staff and students associated with the ChesMMAP survey at the Virginia Institute of Marine Science. Former and current crews of the R/V Bay Eagle and R/V Virginia deserve many thanks for their contributions to vessel maintenance and operations. Comments provided by two reviewers and the Associate Editor reviewers helped improve this manuscript.
The authors declare that the research was conducted in the absence of any commercial or financial relationships that could be construed as a potential conflict of interest.
All claims expressed in this article are solely those of the authors and do not necessarily represent those of their affiliated organizations, or those of the publisher, the editors and the reviewers. Any product that may be evaluated in this article, or claim that may be made by its manufacturer, is not guaranteed or endorsed by the publisher.
Auger-Méthé M., Field C., Albertsen C. M., Derocher A. E., Lewis M. A., Jonsen I. D., et al. (2016). State-space models’ dirty little secrets: even simple linear Gaussian models can have estimation problems. Sci. Rep. 6, 26677. doi: 10.1038/srep26677
Brown R. W., Fogarty M., Legault C., Miller T., Nordahl V., Politis P., et al. (2007). Survey transition and calibration of bottom trawl surveys along the northeastern continental shelf of the united states (International Council for the Exploration of the Seas). CM 2007/Q:20, Copenhagen.
Ding H., Elmore A. J. (2015). Spatio-temporal patterns in water surface temperature from landsat time series data in the Chesapeake bay, U.S.A. Remote Sens. Environ. 168, 335–348. doi: 10.1016/j.rse.2015.07.009
Fernandez L., Leach S. (1998). Prospects for multispecies fisheries management in Chesapeake bay Vol. 79 (Edgewater, MD: Chesapeake Bay Scientific and Technical Advisory Committee [STAC] Publication 98-002).
Gartland J., Gaichas S. K., Latour R. J. (2023). Spatiotemporal patterns in the ecological community of the nearshore mid-Atlantic bight. Mar. Ecol. Prog. Ser. 704, 15–33. doi: 10.3354/meps14235
Hagy J. D., Boynton W. R., Keefe C. W., Wood K. V. (2004). Hypoxia in Chesapeake Bay,1950-2001: long-term change in relation to nutrient loading and river flow. Estuaries 27, 634–658. doi: 10.1007/BF02907650
Hilborn R., Walters C. J. (1992). Quantitative fisheries stock assessment (New York: Chapman & Hall).
Hinson K. E., Friedrichs M. A. M., St-Laurent P., Da F., Najjar R. G. (2022). Extent andcauses of Chesapeake bay warming. J. Am. Water Resour. Assoc. 58, 805–825. doi: 10.1111/1752-1688.12916
Irby I. D., Friedrichs M. A. M., Da F., Hinson K. E. (2018). The competing impacts of climate change and nutrient reductions on dissolved oxygen in Chesapeake bay. Biogeosciences 15, 2649–2668. doi: 10.5194/bg-15-2649-2018
Johnson T. R., McCay B. J. (2012). Trading expertise: the rise and demise of an industry/government committee on survey trawl design. Marit. Stud. 11, 14. doi: 10.1186/2212-9790-11-14
Kemp W. M., Boynton W. R., Adolf J. E., Boesch D. F., Boicourt W. C., Brush G., Cornwell G.J. C., et al. (2005). Eutrophication of Chesapeake bay: historical trends and ecological interactions. Mar. Ecol. Prog. Ser. 303, 1–29. doi: 10.3354/meps30301
Kimura D. K., Somerton D. A. (2006). Review of statistical aspects of survey sampling for marine fisheries. Rev. Fish. Sci. 14, 245–283. doi: 10.1080/10641260600621761
Kirkley J. E., Murray T. J., Duberg J. (2005). Economic contributions of virginia’s commercial seafood and recreational fishing industries: a user’s manual for assessing economic impacts (Gloucester Point, VA: Virginia Institute of Marine Science, Marine Resource Report), 2005–2009. doi: 10.21220/m2-q76v-kq84
Kristensen K., Nielsen A., Berg C. W., Skaug H., Bell B. M. (2016). TMB: automatic differentiation and Laplace approximation. J. Stat. Software 70, 1–21. doi: 10.18637/jss.v070.i05
Latour R. J., Brush M. J., Bonzek C. F. (2003). Toward ecosystem-based fisheries management: strategies for multispecies modeling and associated data requirements. Fisheries 28, 10–22. doi: 10.1577/1548-8446(2003)28[10:TEFM]2.0.CO;2
Lellis-Dibble K. A., McGlynn K. E., Bigford T. E. (2008). Estuarine fish and shellfish species in U.S. commercial and recreational fisheries: economic value as an incentive to protect and restore estuarine habitat. U.S. Dep. Commerce, NOAA technical memorandum NMFS-F/SPO-90 Vol. 102 (Silver Spring, MD: National Oceanic and Atmospheric Administration).
Lewy P., Nielsen J. R., Hovgård H. (2004). Survey gear calibration independent of spatial fish distribution. Can. J. Fish. Aquat. Sci. 61, 636–647. doi: 10.1139/F04-034
Link J. S. (2010). Ecosystem-based fisheries management: confronting tradeoffs (New York: Cambridge University Press).
Link J. S., Marshak A. R. (2022). Ecosystem-based fisheries management: progress, importance, and impacts in the united states (New York: Oxford University Press).
Maunder M. N., Punt A. E. (2004). Standardizing catch and effort data: a review of recent approaches. Fish. Res. 70, 141–159. doi: 10.1016/j.fishres.2004.08.002
Methot J. R.D., Tromble G. R., Lambert D. M., Greene K. E. (2014). Implementing a science-based system for preventing overfishing and guiding sustainable fisheries in the united states. ICES J. Mar. Sci. 71, 183–194. doi: 10.1093/icesjms/fst119
Miller T. J. (2013). A comparison of hierarchical models for relative catch efficiency based on paired-gear data for US Northwest Atlantic fish stocks. Can. J. Fish. Aquat. Sci. 70, 1306–1316. doi: 10.1139/cjfas-2013-0136
Miller T. J., Houde E. D., Watkins E. J. (1996). Chesapeake Bay fisheries: prospects for multispecies management and sustainability Vol. 73 (Edgewater, MD: Chesapeake Research Consortium [CRC] Publication 154B).
Murdy E. O., Birdson R. S., Musick J. A. (1997). Fishes of Chesapeake bay (Washington, DC: Smithsonian).
Najjar R. G., Pyke C. R., Adams M. B., Breitburg D., Hershner C., Kemp M., et al. (2010). Potential climate change impacts on the Chesapeake Bay. Estuar. Coast. Shelf Sci. 86, 1–20. doi: 10.1016/j.ecss.2009.09.026
National Marine Fisheries Service [NMFS] (1998). Ecosystem-based fisheries management: a report to congress by the ecosystem principles advisory panel Vol. 62 (Silver Spring, MD: U.S. Department of Commerce, NOAA). Available at: https://repository.library.noaa.gov/view/noaa/23730.
National Marine Fisheries Service [NMFS] (2020). Fisheries economics of the United States 2020. U.S. Dep. Commerce, NOAA tech. memo. NMFS-F/SPO-236. (Silver Spring, MD: National Oceanic and Atmospheric Administration).
National Oceanic and Atmospheric Administration Chesapeake Bay Fisheries Ecosystem Advisory Panel [NOAA CBFEAP] (2006). Fisheries ecosystem planning for Chesapeake bay Vol. 462 (Bethesda, MD: American Fisheries Society).
Orth R. J., Marion S. R., Moore K. A., Wilcox D. J. (2010). Eelgrass (Zostera marina l.) in the Chesapeake bay region of mid-Atlantic coast of the USA: challenges in conservation and restoration. Estuar. Coasts. 33, 139–150. doi: 10.1007/s12237-009-9234-0
Peterson C. D., Courtney D. L., Cortés E., Latour R. J. (2021). Reconciling conflicting survey indices of abundance prior to stock assessment. ICES J. Mar. Sci. 78, 3101–3120. doi: 10.1093/icesjms/fsab179
Richards R. A., Rago P. J. (1999). A case history of effective fishery management: Chesapeake bay striped bass. N. Am. J. Fish. Manage. 19, 356–375. doi: 10.1577/1548-8675(1999)019<0356:ACHOEF>2.0.CO;2
Ricker W. E. (1975). Computation and interpretation of biological statistics of fish populations. Bull. Fish. Res. Board Can. 191, 1–382.
Schonfeld A. J., Gartland J., Latour R. J. (2022). Spatial differences in estuarine utilization by seasonally resident species in mid-Atlantic bight, USA. Fish. Oceanogr. 31, 615–628. doi: 10.1111/fog.12611
Thomas A. C., Pershing A. J., Friedland K. D., Nye J. A., Mills K. E., Alexander M. A., et al. (2017). Seasonal trends and phenology shifts in sea surface temperature on the north American northeastern continental shelf. Elem. Sci. Anth. 5, 48. doi: 10.1525/elementa.240
Thorson J. T. (2019). Guidance for decision using vector autoregressive spatio-temporal (VAST) package in stock, ecosystem, habitat, and climate assessments. Fish. Res. 210, 143–161. doi: 10.1016/j.fishres.2018.10.013
Thorson J. T., Clarke M. E., Stewart I. J., Punt A. E. (2013). The implications of spatially varying catchability on bottom trawl surveys of fish abundance: a proposed solution involving underwater vehicles. Can. J. Fish. Aquat. Sci. 70, 294–306. doi: 10.1139/cjfas-2013-0330
Thygesen U. H., Kristensen K., Jansen T., Beyer J. E. (2019). Intercalibration of survey methods using paired fishing operations and log-Gaussian cox processes. ICES J. Mar. Sci. 76, 1189–1199. doi: 10.1093/icesjms/fsy191
Tian R., Cerco C. F., Bhatt G., Linker L. C., Shenk G. W. (2022). Mechanisms controlling climate warming impact on the occurrence of hypoxia in Chesapeake bay. J. Am. Water Resour. Assoc. 58, 855–875. doi: 10.1111/1752-1688.12907
Venables W. N., Dichmont C. M. (2004). GLMs, GAMs and GLMMs: an overview of theory for applications in fisheries research. Fish. Res. 70, 319–337. doi: 10.1016/j.fishres.2004.08.011
Wilberg M. J., Thorson J. T., Linton B. C., Berkson J. (2010). Incorporating time-varying catchability into population dynamic stock assessment models. Rev. Fish. Sci. 18, 7–24. doi: 10.1080/10641260903294647
Wilberg M. J., Livings M. E., Barkman J. S., Morris B. T., Robinson J.M. (2011).Overfishing, disease, habitat loss, and potential extirpation of oysters in upper Chesapeake bay.Mar. Ecol. Prog. Ser. 704, 15–33. doi: 10.3354/meps09161
Winger P. D., DeLouche H., Legge G. (2006). Designing and testing new fishing gears: the value of a flume tank. Mar. Technol. Soc J. 40, 44–49. doi: 10.4031/002533206787353240
Keywords: trawl survey, Chesapeake Bay, intercalibration, log-Gaussian Cox process, lessons learned
Citation: Latour RJ, Gartland J and Bonzek CF (2023) Design and redesign of a bottom trawl survey in Chesapeake Bay, USA. Front. Mar. Sci. 10:1217792. doi: 10.3389/fmars.2023.1217792
Received: 05 May 2023; Accepted: 28 June 2023;
Published: 12 July 2023.
Edited by:
Kyle Shertzer, NOAA Southeast Fisheries Science Center, United StatesReviewed by:
Gary Nelson, Massachusetts Division of Marine Fisheries, United StatesCopyright © 2023 Latour, Gartland and Bonzek. This is an open-access article distributed under the terms of the Creative Commons Attribution License (CC BY). The use, distribution or reproduction in other forums is permitted, provided the original author(s) and the copyright owner(s) are credited and that the original publication in this journal is cited, in accordance with accepted academic practice. No use, distribution or reproduction is permitted which does not comply with these terms.
*Correspondence: Robert J. Latour, bGF0b3VyQHZpbXMuZWR1
Disclaimer: All claims expressed in this article are solely those of the authors and do not necessarily represent those of their affiliated organizations, or those of the publisher, the editors and the reviewers. Any product that may be evaluated in this article or claim that may be made by its manufacturer is not guaranteed or endorsed by the publisher.
Research integrity at Frontiers
Learn more about the work of our research integrity team to safeguard the quality of each article we publish.