- 1Independent International Consultant, Pasadena, CA, United States
- 2Environmental Defense Fund, Boston, MA, United States
- 3Environmental Defense Fund, San Francisco, CA, United States
Climate change influences marine environmental conditions and is projected to increase future environmental variability. In the North Atlantic, such changes will affect the behavior and spatiotemporal distributions of large pelagic fish species (i.e., tunas, billfishes, and sharks). Generally, studies on these species have focused on specific climate-induced changes in abiotic factors separately (e.g., water temperature) and on the projection of shifts in species abundance and distribution based on these changes. In this review, we consider the latest research on spatiotemporal effects of climate-induced environmental changes to HMS’ life history, ecology, physiology, distribution, and habitat selection, and describe how the complex interplay between climate-induced changes in biotic and abiotic factors, including fishing, drives changes in species productivity and distribution in the Northwest Atlantic. This information is used to provide a baseline for investigating implications for management of pelagic longline fisheries and to identify knowledge gaps in this region. Warmer, less oxygenated waters may result in higher post-release mortality in bycatch species. Changes in climate variability will likely continue to alter the dynamics of oceanographic processes regulating species behavior and distribution, as well as fishery dynamics, creating challenges for fishery management. Stock assessments need to account for climate-induced changes in species abundance through the integration of species-specific responses to climate variability. Climate-induced changes will likely result in misalignment between current spatial and temporal management measures and the spatiotemporal distribution of these species. Finally, changes in species interactions with fisheries will require focused research to develop best practices for adaptive fisheries management and species recovery.
1 Introduction
Rising anthropogenic greenhouse gas emissions are causing dramatic changes in physicochemical properties and conditions of the ocean, including increased temperature, altered stratification of water masses, decreased pH and levels of dissolved oxygen in the water column, and changes to ocean circulation patterns that are projected to continue and intensify in the future (Solomon et al., 2007; Stocker et al., 2013; Hoegh-Guldberg et al., 2018; Kwiatkowski et al., 2020). In turn, these changes are significantly impacting the world’s coastal and marine ecosystems and the fisheries they support (Walther et al., 2002; Doney et al., 2012; Free et al., 2019).
Rates of ocean warming in the Northwest Atlantic, which we define here to be located between latitudes 10°N and 50°N and to include the Gulf of Mexico (GOM) and Caribbean Sea (CS), are among the highest in the world (Saba et al., 2016), and species’ responses to climate change are expected to scale accordingly (Kleisner et al., 2017; McHenry et al., 2019). Given the intensity of changes in this region, it is imperative to account for climate drivers in fisheries management. However, the ability to predict how species will respond to climate change is challenging due to inherent system complexities. Primarily, ocean productivity and fisheries yields are linked through complex non-linear relationships (Friedland et al., 2012; Stock et al., 2017), and are co-influenced by climate-induced changes in oceanic water conditions through effects on primary and secondary production, food web dynamics, and species-specific life history and distribution of target and bycatch species (Brander, 2007; Blanchard et al., 2012). Additionally, because these effects may be cumulative, synergistic, or antagonistic, the inherent complexity of climate-driven impacts on marine ecosystems and their fisheries make it challenging to understand and predict species responses at various spatiotemporal scales (Woodward et al., 2010; Simpson et al., 2011; Brose et al., 2012).
Environmental variability, also shaped by climate change, has known global effects on the spatial distributions, migratory phenology, and population dynamics of pelagic highly migratory fish species (HMS), including tunas, swordfish, istiophorid billfishes, and sharks (Lehodey et al., 1997; Ravier and Fromentin, 2004; Chang et al., 2013; Skubel et al., 2018). Arguably, the significant rates of ocean warming and concomitant changes in ocean circulation in the Northwest Atlantic will affect HMS species in this region more intensely than in other parts of the world. Additionally, the Northwest Atlantic Ocean has been characterized by several climate-driven changes in regional environmental conditions over recent decades, including warmer sea surface temperature (SST) (Karnauskas et al., 2013; Loder and Wang, 2015) and bottom water temperature (Brickman et al., 2018), increased summertime stratification of shelf waters (Li et al., 2015), changes in dissolved oxygen concentration (DO) levels (Stendardo and Gruber, 2012) and acidification (Cai et al., 2011), and altered oceanographic processes (Karnauskas et al., 2015). In light of these changes, there are a number of considerations that make the projection of HMS distribution and productivity in this region challenging, including ontogenetic shifts that result in changes in species distribution.
Commonly, HMS undergo ontogenetic shifts in distribution - expansions of movement and habitat niches through maturity - with some species traversing entire ocean basins over a few months as adults (Block et al., 2005; Bonfil et al., 2005; Queiroz et al., 2016). Accordingly, fishery management schemes for HMS must progressively cope with larger spatial contexts as young fish mature into adults, from a regional-based focus for larvae and juveniles to, in many cases, the entire ocean basins across which adult HMS migrate, reproduce, and feed, potentially spanning dozens of exclusive economic zones (EEZs) plus the high seas.
Pelagic longline (PLL) fisheries constitute the most significant fleets targeting many HMS in the Northwest Atlantic. The main target of the U.S. Atlantic PLL fishery includes swordfish (Xiphias gladius, SWF), yellowfin tuna (Thunnus albacares, YFT), and bigeye tuna (Thunnus obesus, BET), with bycatch of other less commercial HMS, and in particular sharks (Mandelman et al., 2008; NMFS, 2019a). The dynamics of the PLL fishing fleets are also shaped by variations in environmental conditions that change the catchability of HMS (Ward and Hindmarsh, 2007; Crespo et al., 2018). Hence, HMS abundance and distribution are influenced by a combination of habitat characteristics, species-specific environmental niches, and spatial exposure to fishing practices (Lynch et al., 2018). Understanding the many facets and intersections between climate change, climate variability, and fishing pressure will be key for advancing sustainable fishery management for HMS (Fuentes et al., 2016), which are a high-priority for international management and conservation efforts (Field et al., 2009; Collette et al., 2011; NMFS, 2020).
The full span of knowledge encompassing HMS science is relatively wide-ranging. For the most part, research has been derived from fishery-dependent data (Nicol et al., 2013) and, more recently, electronic tags and satellite-based data (Nielsen et al., 2009; Block et al., 2011). However, current knowledge is fragmented across HMS, as scientific studies on these climate-induced effects often focus on single species, primarily those representing the main targets of PLL fisheries (Lehodey et al., 2013; Erauskin-Extramiana et al., 2019; Erauskin-Extramiana et al., 2020), or single aspects related to climate change, such as warming waters (Tanaka et al., 2021), and changes in ocean acidification (Pistevos et al., 2015) and DO levels (Stramma et al., 2012). Although this information has clearly contributed to analysis and interpretation of projected changes of several HMS distributions to climate change (Chang et al., 2013; Hazen et al., 2013), as well as integrated climate vulnerability risk assessments for some species and regions (Chin et al., 2010; Hare et al., 2016), there has been little work distilling scientific information on the effects of multiple climate stressors to HMS across both target and bycatch species impacted by PLL fisheries (Dell’Apa et al., 2018; Bell et al., 2021; Nicol et al., 2022).
Here, we review the potential effects of climate change and climate variability1 on Northwest Atlantic HMS, for both target and bycatch species (Table 1, and see Supplementary Material), and across life cycles (i.e., adults, juveniles, eggs and larvae), and species responses to these changes. We discuss impacts within categories, and also consider interactions between abiotic, biotic, and fishery drivers across these categories. This synthesis reveals the status of knowledge on climate-driven impacts on HMS in this region as well as key scientific gaps. Within this information, we identify several pathways to develop and implement climate-robust management practices for North Atlantic PLL fisheries.
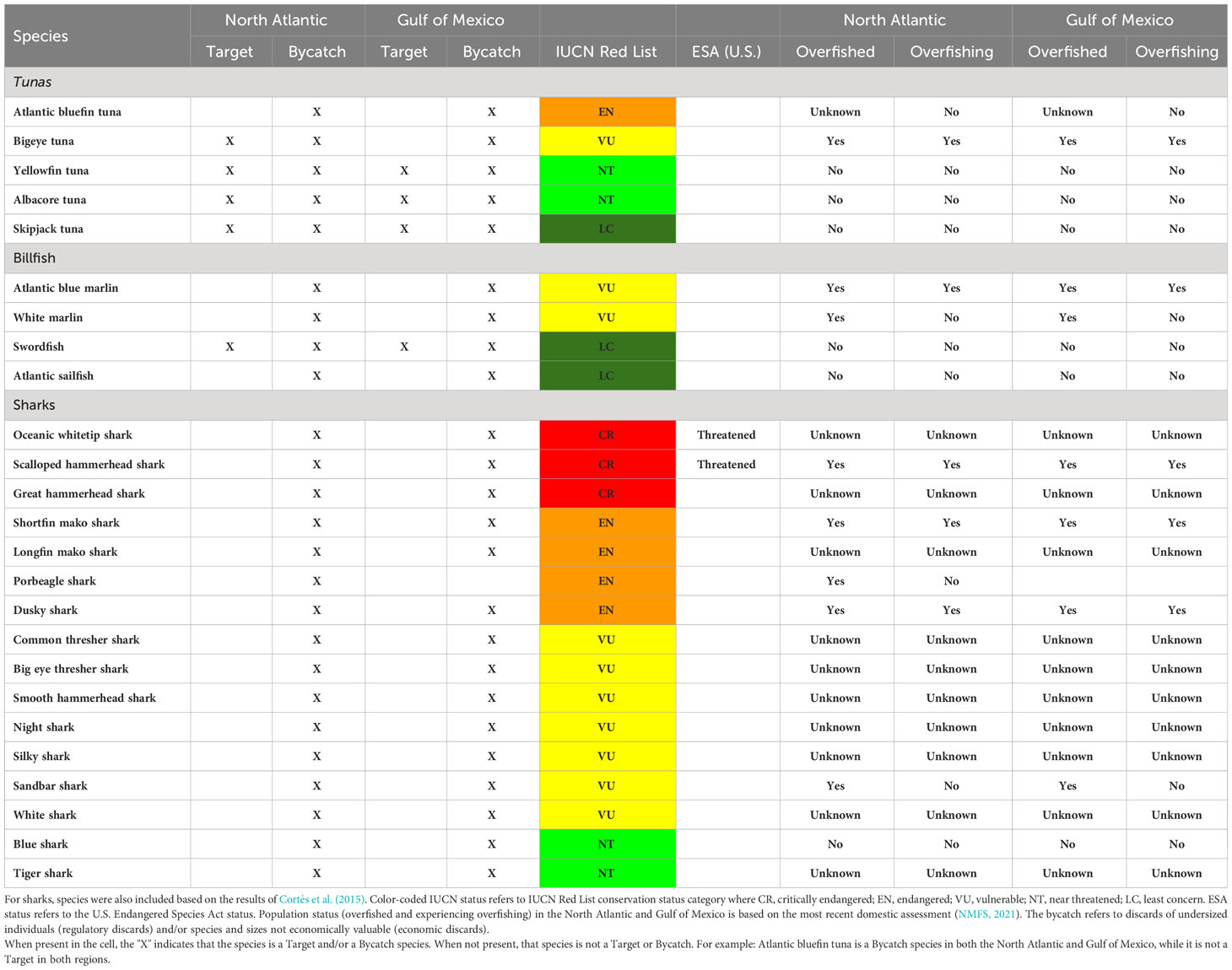
Table 1 List of large pelagic fish species commonly caught in the U.S. Atlantic pelagic longline fishery, including the Caribbean Sea, and considered as the focus of this study.
2 Climate-driven changes and associated impacts to HMS and species responses
Overall, HMS responses to the effects of climate change and variable fishing pressures will be species-specific (i.e., tropical vs. temperate species) and life stage-specific (i.e., adults vs. juveniles). Across many HMS, a common response is that individual species are influenced by regional impacts of climate change that, by the end of 2100, will translate into changes in species physiology, phenology (e.g., migration timing), behavior, distribution, survival, and reproductive success. The major climate-driven changes that will have an influence on HMS are: climate variability, ocean warming, changes in DO levels, and changes in oceanographic processes. Accordingly, in the following sections we summarize the information in the context of these climate-driven impacts on key target and bycatch HMS groups for PLL fisheries in the Northwest Atlantic region, specific to life history stage where possible. We also discuss interactions between abiotic, biotic, and fishery drivers and how they might impact the distribution and abundance of HMS species (Table 2).
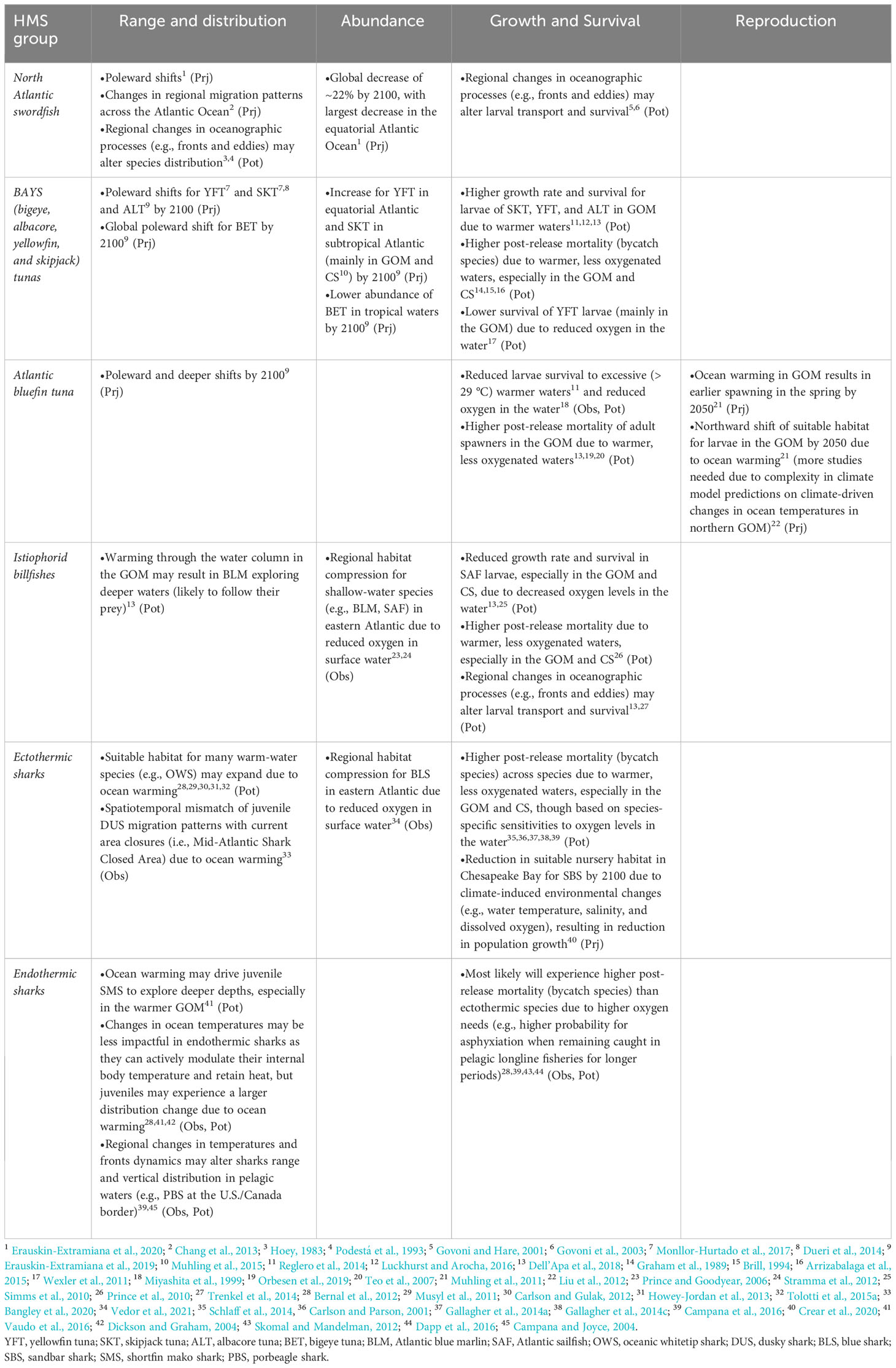
Table 2 Summary of projected (Prj), observed (Obs), and potential (Pot – based upon experimental hypothesis, theoretical reasoning, and/or professional judgement) climate impacts to key target and bycatch species of pelagic longline fisheries in the North Atlantic, Gulf of Mexico (GOM), and Caribbean Sea (CS).
2.1 Impacts to HMS due to changes in climate variability
In the North Atlantic, the Atlantic Multidecadal Oscillation (AMO) is a common index of decadal climate variability and is connected to variations in the Atlantic Meridional Overturning Circulation (AMOC) through changes in the North Atlantic Oscillation (NAO) (Wills et al., 2019)2 Recently, abundance and distribution of the Atlantic bluefin tuna (Thunnus thynnus, BFT) were linked to environmental variations expressed by both AMO and NAO (i.e., the relative influence of AMO to historical BFT abundance was 47.1%, while that of NAO was 19.5%), with higher abundance of BFT (eastern Atlantic population) during positive (i.e., warm) AMO phases and lower abundance during negative (i.e., cold) phases (Failletaz et al., 2019).
Similarly, long-term climate variability and associated changes in marine environmental conditions can influence global abundance and distribution of YFT, with decadal and multidecadal climate variations having oscillating transoceanic effects (~8-16 years periodicity) for this species (Wu et al., 2020). In the Atlantic, interdecadal relative abundance for YFT was significantly negatively correlated with the AMO (Wu et al., 2020)3. Due to the AMO’s influence on change in primary production in the Atlantic Ocean (Nye et al., 2014; Morse et al., 2017), changes in the marine environment can lead to bottom-up alterations of energy and nutrient pathways propagating upward through the food web to higher-level consumers (Blanchard et al., 2012). Hence, these alterations across trophic levels may affect recruitment, growth rates, and abundance of YFT (Wu et al., 2020).
Marine environmental changes due to large-scale climatological variability, which is also influenced by climate change, can shape SWF habitat suitability in the equatorial Atlantic Ocean (Chang et al., 2013). Specifically, climate variability alters regionally based (i.e., east vs. west basin) mixed layer depth (MLD) and sea surface height anomaly (SSHA), which in turn influence SWF habitat suitability and distribution. These results, based on modeled changes in habitat suitability, indicate that SWF migration in the equatorial Atlantic Ocean most likely proceeds southeasterly during summer (June through August) and northwesterly thereafter. These changes in geographical movements were interpreted as a direct consequence of seasonal shifts in environmental conditions, primarily SST, which further implies that SWF distribution can be influenced by interannual and interdecadal environmental variations (Chang et al., 2013). However, the mechanistic influence of climate variability on the Atlantic SWF population is ultimately linked to a combination of direct and indirect responses to changes in oceanographic factors and the overall change in habitat quality. In fact, the results of Chang et al. (2013) highlighted the existence of complex nonlinear relationships between SWF relative abundance (i.e., nominal catch per unit of effort, CPUE) and various environmental factors that may have direct influence on presence and catchability of the species (eg., SST, MLD) or can be proxies of more complex mechanisms and variables difficult to observe, e.g., large scale and mesoscale abundance of prey (SSHA, and chlorophyll a – CHL).
2.2 Impacts to HMS due to ocean warming
Results from the aforementioned studies also highlight the link between multidecadal natural climate variability4 and ocean warming, and the potential impacts to HMS physiology, phenology, behavior, reproductive success, and survival (Chen et al., 2011; Laffoley and Baxter, 2016; Poloczanska et al., 2016; Monllor-Hurtado et al., 2017; Dell’Apa et al., 2018). In the North Atlantic, and depending on the climate scenario used, SST is projected to increase ∼2°C by the end of 2100, with a concurring 25-33% reduction in the strength of the AMOC (Schmittner et al., 2005; Drijfhout and Hazeleger, 2006; Kwiatkowski et al., 2020; Liu et al., 2020). Combined, these two environmental changes are expected to lead to strong alterations of key ecological processes in both the Atlantic and GOM marine ecosystems, including a decrease in primary productivity due to reduced upwelling of deep, colder water rich in nutrients and the resulting gradual decrease of these nutrients in the upper water column (Schmittner et al., 2005; Liu et al., 2012). Warmer ocean surface waters will lead to marked changes in HMS behavioral responses and spatial distribution, also due to changes in regional trophic and predator-prey interactions, with, likely, potential negative impacts to species survival and reproductive success. However, these impacts will differ by species and life stage (i.e., adults, juveniles, eggs and larvae) due to species-specific (Boyce et al., 2008; O’Brien et al., 2013) and age-specific (Pörtner and Farrel, 2008; Moyano et al., 2017) thermal tolerances and physiological traits.
2.2.1 Species sensitivity to ocean temperature
2.2.1.1 Tunas and swordfish (i.e., the target of PLL fisheries), and istiophorid billfishes
Among adult tunas in the Northwest Atlantic, BFT and BET have wider ranges of tolerance for ambient water temperature (Tw) compared to more tropical or less temperate species, such as YFT, skipjack tuna (Katsuwonus pelamis, SKT), and albacore tuna (Thunnus alalunga, ALT) (Boyce et al., 2008)5. Similarly, among adult billfishes, SWF has a much wider range of Tw tolerance compared to istiophorid billfishes, including Atlantic blue marlin (Makaira nigricans, BLM), white marlin (Tetrapturus albidus, WHM), and Atlantic sailfish (Istiophorus albicans, SAF). Also, when considering mean temperature preferences, BFT, SWF, BET, and ALT spend most of their time in colder waters compared to other tunas and istiophorid billfishes (Boyce et al., 2008). With the exclusion of ALT, which has an epipelagic distribution (0-450 m) depending on size, Tw, and diurnal cycle (Goñi et al., 2009; Trenkel et al., 2014), these species can be found at deeper depths as they have specialized physiological and morphological traits allowing them to withstand colder Tw.
Specifically, these species are endothermic (see Supplementary Material). As a result of their endothermic capacity, BFT can dive to colder, deeper waters, down to depths of 1,000 m (Block et al., 2001), and occasionally undertake trans-Atlantic migrations through areas with a wide range of Tw (Block et al., 2005). Similarly, BET can dive to water depths of ~1,200 m (Lam et al., 2014), and can generally remain in these colder, hypoxic waters longer than other tunas before having to return to shallower (warmer) depths (Schaefer et al., 2009; Schaefer and Fuller, 2010). BET exhibits diurnal vertical migrations, spending nights in surface waters to thermoregulate (Holland et al., 1992; Brill, 1994; Arrizabalaga et al., 2008; Schaefer and Fuller, 2010) and moving to deeper waters during the day6 to feed on vertically migrating prey associated with the deep scattering layer (Dagorn et al., 2000; Matsumoto et al., 2013). In addition, BET is adapted to spend more time at colder, deeper depths due to enhanced cardiac performance (Galli et al., 2009).
In contrast, tropical tunas (e.g., YFT and SKT) spend most of their time above the thermocline due to their reduced tolerance to low Tw (Graham and Dickson, 2004; Boyce et al., 2008; Arrizabalaga et al., 2015). Accordingly, YFT and SKT show a preference for higher Tw (~21-26°C for YFT, and ~19-26°C for SKT) than BFT (~15-23°C), BET (~17-22°C), and ALT (~15-19°C) (Boyce et al., 2008). Moreover, YFT are likely restricted from excessively warmer Tw (> 30°C), as it can limit their cardiac capacity (Blank et al., 2002). Similarly, BFT, though having one of the largest thermal tolerances, becomes physiologically stressed at Tw > 26-28°C (Block et al., 2005; Boyce et al., 2008) as the result of overheating (Sharp and Vlymen, 1978) and metabolic stress (Block and Stevens, 2001; Teo et al., 2007).
Generally, istiophorid billfishes prefer water depths above the thermocline and can occasionally dive to waters as deep as 200 m (Block et al., 1992). Conversely, SWF is considered a deep-dwelling species, as they are mostly found below the thermocline during the day (Dewar et al., 2011). SWF can also swim to deeper waters (300-600 m) to feed on mesopelagic prey (Takahashi et al., 2003; Dewar et al., 2011; Abecassis et al., 2012), due to a higher level of physiological thermoregulation (see Supplementary Material) and specialized cardiac capacity allowing them to tolerate colder Tw for prolonged periods (Fritsches et al., 2005; Galli et al., 2009; Stoehr et al., 2018). Modeling results suggest a low probability of SWF presence at Tw < 15°C, and highest abundance at 20-25°C (Erauskin-Extramiana et al., 2020).
Conversely, BLM, WHM, and SAF are considered epipelagic, remaining within the upper portion of the water column, or surface mixed layer (SML) (Boyce et al., 2008; Hoolihan et al., 2009). In the GOM, BLM spend most of their time in warmer surface waters (Kraus and Rooker, 2007). Moreover, there seems to be a link between the percentage of time that BLM spend at deeper waters and the temperature change related to the ocean surface (Saito et al., 2004), with most of the time spent at temperatures within 3°C of surface Tw (Kraus and Rooker, 2007). Despite the primary role of Tw in the species spatial distribution, light penetration through the water column and reduced DO levels in deeper waters appear to be key limiting factors for the vertical distribution of BLM and other billfishes (Prince and Goodyear, 2006; Kraus and Rooker, 2007). Consequently, these species-specific differences in habitat thermal conditions are reflected in the range of ambient water preferences in those species, with SWF characterized by a wider range of thermal preference (~15-23°C) than those of other istiophorid billfishes (e.g., ~25-30°C for BLM; ~25-27°C for WHM; and ~26-28°C for SAF) (Boyce et al., 2008).
2.2.1.2 Ectothermic sharks
For large pelagic sharks, Tw is a key environmental factor controlling physiological and metabolic processes (Carlson et al., 2004; Schlaff et al., 2014), particularly for ectothermic sharks (see Supplementary Material)7.
However, the thermal sensitivity range is species-specific across sharks, and changes in Tw can influence species behavior and spatial distribution as a response to differing thermal habitat requirements (Bernal et al., 2012). Thus, projected global ocean warming will influence temperature-mediated spatial patterns and diurnal and seasonal movements (both geographically and vertically) in ectothermic sharks (Bernal et al., 2012; Schlaff et al., 2014)8.
Many ectothermic sharks are capable of behavioral thermoregulation9. For blue sharks (Prionace glauca, BLS), behavioral thermoregulation is most likely the result of a feeding strategy. Results by Campana et al. (2011) of immature BLS tagged-and-released with pop-up satellite archival transmitting tags (PSATs) suggest that the Northwest Atlantic population may use warm waters in the Gulf Stream and part of the Sargasso Sea as overwintering grounds. When entering these warmer waters, immature sharks exhibit a diel vertical migration, remaining at the surface (average depth of 74 m) during nighttime and diving to deeper (average depth of 412 m), colder waters during daytime, most likely as a strategy to forage on their vertically migrating prey (e.g., squid). These results suggest that this feeding-driven thermoregulatory behavior provides a metabolic advantage to BLS even when considering the presence of any reduced foraging success associated with remaining in deeper, colder waters (Campana et al., 2011). Similar results based on conventional tags were reported for adult BLS in the Northwest Atlantic, showing that BLS swim to warmer surface waters after prolonged periods at deeper, cooler depths of ~250 m, and experience changes in Tw between 7-9°C (Carey and Scharold, 1990).
Behavioral thermoregulation is also exhibited by the oceanic whitetip shark (Carcharhinus longimanus, OWS) (Howey-Jordan et al., 2013). This epipelagic species has a preference for warmer waters (20-26°C) and is primarily found in waters above ~100-125 m depth (Musyl et al., 2011; Carlson and Gulak, 2012; Howey-Jordan et al., 2013; Tolotti et al., 2015a; Andrzejaczek et al., 2018)10. Results from PSATs data of OWS in the tropical Northwest Atlantic indicated the presence of seasonal changes in vertical movement patterns in this species, with a negative relationship between the percentage of time that OWS spent in the upper 50 m and SST. Chiefly, OWS showed a preference for deeper waters during the summer, which is interpreted as a thermoregulatory strategy to avoid prolonged exposure to SST warmer than ~28°C (Andrzejaczek et al., 2018).
Within the Northwest Atlantic, the dusky shark (Carcharhinus obscurus, DUS) is a coastal-pelagic species that can be found from temperate waters in Georges Bank to warmer waters in the northern GOM (Castro, 2011). Results from PSAT data of tagged-and-released individuals (mostly adult females) in the northern GOM suggested that DUS have a preference for warmer (24-26°C) and shallower (20-75 m) waters, with no evidence of daily changes in depth or temperature ranges (Hoffmayer et al., 2014)11. An acoustic tags study in the Northwest Atlantic indicated a higher juvenile presence probability between 17 and 26°C Tw (Bangley et al., 2020). Based on information from a climate vulnerability assessment, DUS has a high potential to exhibit a shift in their distribution in the Northwest Atlantic due to climate change (Hare et al., 2016). Evidence indicates that juvenile DUS are migrating into and outside the Mid-Atlantic Shark Closed Area (MASCA),12 respectively, two months earlier than expected, suggesting that the existing area closure may not be timed optimally to protect these juvenile sharks (Bangley et al., 2020).
The sandbar shark (Carcharhinus plumbeus, SBS) is a common coastal-pelagic species in the Northwest Atlantic, using many bays and estuaries along the U.S. Atlantic coast as nursery areas (Castro, 2011). Recent modeling results by Crear et al. (2020) projected suitable habitat for juvenile SBS in the Chesapeake Bay, the most important nursery habitat for the species in the Northwest Atlantic, to significantly decline due to climate-driven sea surface warming. Projected changes in Tw, salinity, and DO by 2100 will cause juvenile SBS to shift behavioral strategies to avoid unsuitable environmental conditions, which may impact their feeding ecology or predator avoidance strategies (Crear et al., 2020). Similarly, experimental results indicate that an increase in Tw will likely result in higher metabolic rates in juvenile SBS and increased oxygen demand, leading to higher physiological stress (substantial decline in overall performance at Tw = 32°C), or changes in swimming behavior (Crear et al., 2019).
Several Sphyrnidae (hammerheads) can be found in the Northwest Atlantic Ocean. The most common hammerheads in this region are the scalloped hammerhead (Sphyrna lewini, SHS), the smooth hammerhead (Sphyrna zygaena, SMH), and the great hammerhead (Sphyrna mokarran, GHS)13. Less information is available for biological data, movement patterns, and habitat requirements (including thermal tolerance) of hammerheads compared to other coastal and pelagic sharks, despite the common capture of Sphyrnidae in PLL fisheries in the Atlantic Ocean (NMFS, 2011). Among these three species, SHS is the most abundant, and inhabits warm temperate and tropical coastal areas and offshore waters at Tw > 22°C (Schulze-Haugen and Kohler, 2003; Castro, 2011). It is generally found in waters, including bays and estuaries, with depths ranging from the surface to ~500 m depth (Compagno, 1984; Klimley, 1993), with occasional deeper dives (~1,000 m depth) (Jorgensen et al., 2009). However, one PSAT-tagged female in the northern GOM exhibited daily vertical migrations, which was interpreted as possible behavioral thermoregulation14. Conversely, diel vertical migration was not reported in PSAT-tagged juvenile and adult SHS in the eastern equatorial and tropical Atlantic, with both life stages remaining closer to the surface, spending the majority of time in warmer (Tw > 23°C) and relatively shallow (0-50 m depths) waters (Santos and Coelho, 2018). Uniquely, juveniles spent nights at significantly deeper and colder waters than adults (Santos and Coelho, 2018).
2.2.1.3 Endothermic sharks
Although endothermic sharks have higher metabolic rates than ectothermic sharks (Carlson et al., 2004; Bernal et al., 2012), they are less susceptible to changes in Tw as they can modulate their internal body temperature and retain heat (see Supplementary Material) (Dickson and Graham, 2004; Bernal et al., 2012). Thus, we expect lower impacts of warming waters for endothermic species, though their horizontal (i.e., geographic) and vertical (i.e., depth) distributions might fluctuate in response to habitat changes in their prey (mainly those living in the upper-ocean layers) as a result of warmer water conditions and predator-prey synchronizations (Sergeant et al., 2014).
Preliminary results from PSATs data on juvenile shortfin mako sharks (Isurus oxyrinchus, SMS) in the Northwest Atlantic and GOM showed that vertical movements of tagged sharks were significantly influenced by Tw, with a clear preference for warmer waters (22-27°C) indicating that endothermy might not be an adaption for thermal niche expansion in this species (Vaudo et al., 2016). Also, juvenile SMS exhibit diel shifts in vertical habitat use patterns, spending the majority of the day at deeper (colder) depths than night, when these same sharks were spending most of their time closer to surface (10-50 m depth) and mainly in colder bodies of water. Thus, when comparing vertical distributions between the warmer GOM (SST routinely in excess of 28°C) and colder North Atlantic, juveniles in the former region were diving to deeper depths and avoiding warmer surface Tw (Vaudo et al., 2016). Consequently, climate-driven ocean warming could potentially impact movements and depth distributions of juvenile SMS, especially in the Northwest Atlantic, through an expansion to deeper depths. As for adults, results of potential implications of climate change to SMS are available only for the eastern North Pacific, where projections indicate a decrease in core SMS habitat of ~25% by 2100 (Hazen et al., 2013)15. However, for the Northwest Atlantic population, SMS is known to undergo seasonal migrations that are influenced by changes in Tw16.
Being the largest among endothermic sharks, the white shark, (Carcharodon carcharias, WHS) is the species with the highest thermal inertia due to a lower surface area:volume ratio, on average, compared to other species17. Consequently, this species may be least physiologically impacted by projected ocean warming among endothermic sharks, although more studies are needed to determine the impacts of climate change to WHS populations (Huveneers et al., 2018). Interestingly, results of species distribution modeling by Hazen et al. (2013), based on output from a global climate model, predicted a ~10% increase in core WHS habitat in the eastern North Pacific by 2100. To our knowledge, there is a lack of similar predictive studies in the Northwest Atlantic. However, along the U.S. Atlantic region, the population is characterized by north-south seasonal migrations that appear to be influenced by Tw and prey availability (Curtis et al., 2014; Skomal et al., 2017). WHS in the U.S. Atlantic exhibit a wide thermal tolerance (4-32°C), although occupy a preferred range of Tw (13-25°C) (Curtis et al., 2014; Skomal et al., 2017), with juveniles selecting a narrower range (18-20°C) than adults (Shaw et al., 2021). As for their vertical distribution, juveniles, which are more coastal, are mostly distributed in shallow waters (< 40-50 m depth, and with avoidance for waters < 10 m – Shaw et al., 2021), while subadults and adults, which are more pelagic, exhibit a bimodal depth distribution with a shift between shallower waters (< 25 m depth) and the mesopelagic zone (200-600 m, and maximum recorded depth of 1,128 m). This dichotomy is interpreted as a foraging strategy (Skomal et al., 2017). The geographical and vertical niche expansion by adults into offshore waters is most likely a consequence of ontogenetic shifts in thermoregulatory abilities in this species (Skomal et al., 2017).
Among endothermic sharks, the common thresher shark (Alopias vulpinus, THR) can be globally considered a warm-water oceanic species (Cheung et al., 2015). THR has a preference for Tw between 16 and 21°C (based on archival tag results off the Southern California Bight - Cartamil et al., 2011), and in the Northwest Atlantic is captured in waters with SST between 4 and 31°C and primarily between 12 and 18°C (based on results of fishery-dependent surveys - Kneebone et al., 2020). Captures of young of the year THR occur in waters with a narrower SST range (6-26°C) than adults, which suggest the presence of ontogenetic shifts in thermoregulatory capacity in this species, similar to WHS (Skomal et al., 2017), with small individuals (i.e., higher surface:area volume than adults) having a reduced ability to retain heat through endothermy (Bernal and Sepulveda, 2005; Kneebone et al., 2020). There is a dearth of information on THR spatiotemporal distribution and habitat use in the Northwest Atlantic,18 where the species distribution appears to be characterized by ontogenetic shifts and seasonality.19 Catch-based distribution patterns in the Northwest Atlantic show extensive seasonal migrations in THR which may depend on the species thermal preferences, although more research is needed to clarify if they might also be triggered by thermal preferences in their prey or regional oceanographic processes (Cartamil et al., 2010; Kneebone et al., 2020)20.
Finally, the porbeagle shark (Lamna nasus, PBS) is the endothermic shark with the narrowest thermal preference range in the Northwest Atlantic (Carey et al., 1985),21 where the population is also characterized by north-south seasonal migrations22. This species is primarily caught (based on SST rather than Tw at fishing depth) in colder waters (5-10°C) (Campana and Joyce, 2004) and seldom found south of 30°N, although juveniles spend most of their time in waters between 6 and 20°C (Skomal et al., 2021). Additionally, PBS exhibit an association with colder frontal fronts in the spring, which was anecdotally used by Canadian fishers as a strategy to increase PBS catch rates by selectively fishing where frontal zones occur. However, the presence of PBS in cold fronts appears to be most likely due to higher presence of prey rather than thermal preference by the species (Campana and Joyce, 2004). Interestingly, although adult males and juveniles of both sexes are found exclusively in colder waters at northern latitudes, results from PSAT tags data indicate that adult females may routinely migrate to the warmer waters of the Sargasso Sea, using this area as a possible pupping ground (Campana et al., 2010). Also, during this southern migration, and due to this species being more sensitive to higher Tw, female PBS tend to dive to deeper, colder waters below the Gulf Stream, with a maximum recorded depth of 1,360 m. During the route, females exhibit diel vertical distributions with deeper dives at nighttime versus daytime (Campana et al., 2010). These diel depth changes are also typical for the remaining PBS population in the North Atlantic (Campana and Joyce, 2004; Pade et al., 2009; Saunders et al., 2011). However, recent results from PSAT tags indicated that this wintertime diving behavior in the Gulf Stream also exists in juveniles23.
2.2.2 Projected responses of HMS to global warming
In response to climate-driven ocean warming, tropical tunas like YFT and SKT will likely be able to migrate toward northern latitudes in sub-tropical regions within the North Atlantic basin, as these species are more adapted to warmer Tw compared to more temperate (i.e., BFT, ALT) and intermediate (i.e., BET) species (Monllor-Hurtado et al., 2017). Accordingly, modeling results under RCP8.5-based climate change scenarios24 projected significant shifts in habitat distribution and abundance in the North Atlantic for ALT (i.e., poleward shift), BET (i.e., north-west shift), BFT (i.e., northward shift in the West Atlantic, but no significant shift in the East Atlantic), and SKT (i.e., north and east shift in the West Atlantic, but no significant shift in the East Atlantic) by the end of 2100. Also, all stocks were projected to undergo an eastward shift in distribution by the end of 2100 in the North Atlantic (Erauskin-Extramiana et al., 2019)25.
Similarly, results of models integrating population dynamics, life history, metabolic rates, and behavioral responses under the combined effects of environmental conditions and fishery exploitations showed significant changes in the projected spatial distribution of habitat suitability for SKT by 2100 (Dueri et al., 2014). Globally, results showed a reduction in suitable habitat for SKT in the majority of tropical waters and an increase in habitat at higher latitudes, with the primary driver for these spatial changes being ocean warming and, secondarily, changes in food density. Similar projections suggest a positive response of SKT to increasing ocean surface temperatures in the northern GOM portion of the tropical West Atlantic basin (Muhling et al., 2015).
In Dueri et al. (2014), specific results for the Atlantic Ocean indicated that, compared to other regions, the most suitable habitat for SKT has been in shallow water (< 50 m) in the eastern basin. Concurrently, basin-wide changes in food density are projected to lead to habitat reduction for this species throughout the upper 100 m. Changes in DO levels and light penetration will be the main drivers for reduced habitat conditions at water depths between 50 and 150 m (Dueri et al., 2014), similar to what was reported for BLM (Prince and Goodyear, 2006; Kraus and Rooker, 2007). In the GOM, warmer SST may contribute to changes in BLM spatial distribution, with the species possibly moving into deeper waters to follow deepening vertical migrations of their prey as warmer water masses will extend deeper in the water column (Dell’Apa et al., 2018).
In the case of SWF, global projections based on habitat suitability (under the RCP8.5 climate change scenario) suggest an overall decrease in stock relative abundance (based on CPUEs) of ~22% by the end of 2100 (Erauskin-Extramiana et al., 2020). In detail, projections showed an increase in relative abundance in both the northern and southern boundaries of the species range (mainly in the western portions of both the North Atlantic and Mediterranean Sea), with a decrease in equatorial areas across all oceans and the highest decrease in abundance that was projected in the equatorial Atlantic Ocean (Erauskin-Extramiana et al., 2020). However, as suggested by the authors, these projections should be considered only as general trends due to the high uncertainty in fisheries scenarios and the assumption of constant fishing dynamics over time. Also, they did not account for possible life history and physiological evolutionary responses of SWF that might allow populations to adapt to global climate changes (Erauskin-Extramiana et al., 2020).
Climate warming has also been suggested as a key driver for changes in the timing of feeding migrations of BFT and ALT in the Northeast Atlantic, with a gradually earlier arrival of adult spawners in the Bay of Biscay over the period analyzed for both species (Dufour et al., 2010)26. Also, the results of Dufour et al. (2010) support the hypothesis that climate-driven changes in primary productivity and associated shifts in trophic dynamics in the North Atlantic will likely result in negative impacts on phenology (e.g., feeding migration) and spatial distribution of BFT and ALT, with the former being a key bycatch species of PLL fisheries across the entire Atlantic basin.
In turn, and due to the high specificity of its spawning areas, these impacts may have potential implications for BFT reproductive success during both breeding in the North Atlantic and spawning in the GOM and Mediterranean Sea (Domingues et al., 2016)27. Contrary to other Atlantic tropical tunas, such as YFT that can spawn in different areas in both the eastern (in the Gulf of Guinea) and western (in the GOM between May and August, and in the southeastern CS between July and September) tropical Atlantic (Arocha et al., 2001), and SKT that can spawn almost throughout the year over large areas (Matsumoto et al., 1984; Muhling et al., 2015), BFT spawning is much more spatiotemporally restricted. The western Atlantic BFT population spawn exclusively in the northern GOM between March and June (Mather III et al., 1995; Teo and Block, 2010; Muhling et al., 2013; Knapp et al., 2014), while the eastern population spawn only in the Mediterranean Sea between June and August (Teo and Block, 2010)28.
In the GOM, the mean offshore (> 200 m depth) SST has been warming over the last three decades (Karnauskas et al., 2013), with a projected SST increase of 2-3°C across the basin by 2100 (Biasutti et al., 2012). Inferences from climate modeling results (based on the A1B emissions scenario) suggest that these warmer SST will result in changes to BFT phenology, with earlier spawning in the spring (Muhling et al., 2011). This is also supported by analyses of preferred environmental conditions for BFT larvae in the GOM (Domingues et al., 2016). These results further support the notion that BFT spawning behavior is triggered by SST thresholds, with a higher probability of occurrence for BFT larvae in areas where SST is > 26°C (Rooker et al., 2007). Therefore, ocean surface warming in the GOM may result in reduced reproductive success for the Western BFT population due to potential habitat losses for larvae, as suggested by recent results of modeled probability of larval presence indicating a reduction in larvae presence in water warmer than ~28°C in the GOM (Muhling et al., 2015). Similarly, Muhling et al. (2011) projected a decrease of 39-61% in areas of high probability of BFT larvae occurrence in late spring (May-June), and an increase (62%) in suitable early spring (March) habitat by 2050. Additionally, by 2050, suitable habitat for BFT larvae will likely shift northward due to warmer SST. In general, these results are in line with a reported Tw tolerance between ~25-28°C for BFT larvae, with a ~29°C threshold limit of survival (Reglero et al., 2014).
In contrast, warmer surface ocean conditions may favor survival and growth of larvae for other tropical tunas in the GOM, mainly SKT larvae and, to a minor extent, YFT and ALT larvae (Reglero et al., 2014; Luckhurst and Arocha, 2016; Dell’Apa et al., 2018). In fact, the results of Muhling et al. (2015) indicated that SKT larvae, similar to adults, will be more likely to occur in warmer waters in the GOM, with higher abundances associated with Tw > 29°C. Furthermore, they projected that ocean warming in the GOM will result in a general habitat expansion of adult SKT by 2090 and, consequently, in increased probability of larval occurrence across the GOM (Muhling et al., 2015). These results are in partial agreement with those of Dueri et al. (2014) for climate-driven latitudinal changes in SKT distribution, showing a higher projected population biomass at 10° latitude in both hemispheres by 2050, although, contrary to Muhling et al. (2015), by 2095 the biomass peak is projected to return to the Equator. As reported by Muhling et al. (2015) some result discrepancies between these two studies are likely due to the use of different rates of projected warming among different regions (i.e., use of downscaled vs. global climate models) and different values for upper thermal tolerance limits for SKT.
2.3 Impacts to HMS due to changes in dissolved oxygen concentrations through the water column
Global ocean warming will contribute to modify DO levels in the upper ocean layers due to decreased oxygen solubility at higher water temperatures, thermal stratification through the water column, and higher oxygen consumption rates by the biotic compartment within it as temperature increases (Keeling et al., 2010). This will likely have strong impacts on many HMS species because they are obligate ram-ventilators. Typically, obligate ram-ventilating species need to swim continuously in order to force water flowing through their open mouth and over the gills, allowing adequate oxygen uptake from the water. This specific respiratory mode is associated with high energetic demands in these more active species, which is also reflected in their higher metabolic and oxygen consumption rates compared to other sedentary or less active fish species (Bernal et al., 2012). Moreover, environmental conditions play a key controlling factor in regulating oxygen concentrations in ambient water (e.g., as Tw increases, DO levels decrease), resulting in many physiological and behavioral responses in fish species as oxygen in the water decreases. In the case of ram-ventilating HMS, generally, the behavioral response to less oxygenated or hypoxic water conditions is to swim faster, so to augment oxygen uptake through the gills, and to increase metabolism (Carlson and Parson, 2001), or simply leave the area to search for more suitable oxygen conditions (Graham et al., 1990; Bushnell and Brill, 1991; Bernal et al., 2012). Typically, warmer sea surface waters favor a faster transition to depleted DO or hypoxic (DO < 2 mg/l) conditions that may not support the high-performance physiology and metabolic rates of HMS (Brill, 1996; Idrisi et al., 2003; Bernal et al., 2009).
The upper portion of the ocean, or SML, is essential for circulating nutrients and controlling biological processes such as microbial decomposition (Sieburth, 1983). Aerobic decomposition processes can locally deplete oxygen concentrations below species-specific limit thresholds if mixing does not take place. Changes in the SML depth via an upward vertical expansion of the oxygen minimum zone (OMZ), also known as shoaling, reduces the volume of oxygenated habitat suitable for HMS (Prince and Goodyear, 2006; Prince et al., 2010). By the end of 2100, average DO levels between 200 and 700 m depth (i.e., the main depth layers where most HMS live and forage), are projected to decrease globally throughout the mid- and high-latitudes, and in the North Atlantic primarily in more coastal regions along the eastern tropical basin (Leung et al., 2019). Thus, along with Tw, DO levels in the water affect key biological and metabolic processes that co-limit the spatial distribution of tunas (Brill, 1994), billfishes (Stramma et al., 2012), and sharks (Schlaff et al., 2014), with potential negative implications for growth, maturation, recruitment, and survival (Dell’Apa et al., 2018).
2.3.1 Tunas, swordfish, and istiophorid billfishes
Species-specific oxygen tolerance limits will result in different changes in species habitat suitability, including habitat reduction for some species, due to climate-driven water column deoxygenation. For example, ALT have a low tolerance for reduced DO (lower DO tolerance limit is ~3.7 ml/l – Graham et al., 1989 – and lethal conditions for DO < 1.23 ml/l – Sharp and Vlymen, 1978), as well as SKT (lower lethal conditions for DO < 2.4-2.8 mg/l – Brill, 1994), and YFT (lower lethal conditions for DO < 1.5-2.3 mg/l – Brill, 1994). BET have a higher tolerance for reduced DO levels (lower lethal conditions for DO < 0.5-0.7 mg/l – Brill, 1994) due to specific blood-oxygen binding characteristics compared to other tunas (Lowe et al., 2000).
Results by Arrizabalaga et al. (2015) suggest that reduced DO levels in the water will have the strongest negative effects on temperate compared to tropical species. Specifically, SKT have a much narrower minimum oxygen range than YFT, while temperate tunas, like ALT, are very intolerant of low DO levels. Similarly, experimental results by Bushnell et al. (1990) indicated that cardiorespiratory responses of SKT to highly reduced DO levels in water make this species more sensitive to oxygen reductions than YFT and BET. These results are in accordance with the established epipelagic distribution of SKT, and modeling results projecting its poleward shift in response to ocean warming (Dueri et al., 2014). In the Atlantic, changes in DO levels will affect habitat conditions for SKT particularly between 50 m and 150 m depth (Dueri et al., 2014).
Mislan et al. (2017) provided compelling modeling results for the projected implications of climate change on the vertical distribution of tunas based on their species-specific value for P5029. These results, based upon the RCP8.5 emissions scenario, projected a shoaling of P50 for many species and especially in the Pacific region; an indication of vertical habitat compression for Pacific tunas (Mislan et al., 2017).
Similarly, istiophorid billfishes, and to a lesser extent SWF, are particularly sensitive to DO levels in the water column, and changes in DO may reduce the vertical depth of suitable habitat for these species due to shoaling of the OMZ (Prince and Goodyear, 2006; Stramma et al., 2012). The lower habitat hypoxic threshold for these species is considered at DO ≤ 3.5 ml/l (Brill, 1994; Brill, 1996; Prince and Goodyear, 2006). Stramma et al. (2012) constructed maps of historical changes (1960-2010) in vertical depths of lower DO threshold (i.e., OMZ) for billfishes in the tropical Atlantic, and compared tagging data on vertical movements of BLM in both the western North Atlantic and eastern tropical Atlantic to validate mapping outcomes in regard to changes in species habitat use associated with OMZ vertical expansion. They found that suitable oxygen habitat in the upper surface layer of the tropical East Atlantic declined 15% over the period analyzed, suggesting that a shallowing of this mixed layer vertically compresses the suitable oxygen habitat for billfishes. No reductions in vertical movements of tagged BLM were found in the western North Atlantic because this region is not characterized by OMZ shoaling (i.e., absence of OMZ). Conversely, maximum depths for BLM tagged in the eastern tropical Atlantic decreased moving eastward, indicating that fish might be restricted to the more oxygenated water near the surface (Stramma et al., 2012). Similar results were found by Prince et al. (2010) based on data from tagged BLM and SAF, suggesting the presence of oxygen habitat compression and OMZ shoaling in the eastern Atlantic but not in the western North Atlantic.
To our knowledge, specific habitat compressions due to changes in DO levels have not been reported in tunas. However, given the similarities in physiology and oxygen demands between tunas and billfishes, this process was hypothesized to occur in tunas (Green, 1967; Barkely et al., 1978; Prince and Goodyear, 2007), as also suggested by climate-based projections of their P50 depth shoaling (mainly in the Pacific Ocean) (Mislan et al., 2017).
2.3.2 Pelagic sharks and other bycatch species of PLL fisheries
Less oxygenated or hypoxic waters, in conjunction with warmer waters, are more conducive to higher metabolic stress and fishing mortalities in bycatch HMS, such as pelagic sharks (Schlaff et al., 2014), billfishes (Prince et al., 2010), and some tunas (Brill, 1994). Pelagic sharks may exhibit behavioral responses to oxygen habitat compression and OMZ shoaling in the eastern Atlantic, similar to the scenario reported in billfishes (Stramma et al., 2012), as suggested by PSAT tagging results for BLS showing that this species swim in significantly shallower water when transiting above the OMZ compared to adjacent normoxic areas in the North Atlantic (Vedor et al., 2021). Arguably, any form of interference with the need for a constant uptake of oxygen, including reduced swimming activity due to entanglement in fishing gears, can lead to increased metabolic stress and mortality in HMS. Hence, fishery interactions and characteristics (e.g., the type of fishing gear and operations, soaking times, depths, handling times and practices), coupled with environmental conditions (e.g., warmer Tw and associated lower DO levels) can contribute to amplify fishing mortalities of HMS that are unintentionally caught and discarded as bycatch (Davis, 2002; Gallagher et al., 2014a; Huang et al., 2016)30. In particular, PLL fisheries targeting tunas and SWF can incidentally capture other HMS, with magnitudes, at times, exceeding those of target species. Such a dynamic is particularly of concern for large pelagic sharks (Molina and Cooke, 2012; Oliver et al., 2015).
In the Northwest Atlantic, BFT are often incidentally caught as bycatch and, because the western Atlantic stock is depleted (although not subject to overfishing and with an “unknown” overfished status), they must be released alive in the water31. In the GOM, at-vessel mortality for BFT caught in PLL fisheries is estimated to be between 30% (Block et al., 2005) and 54% (Orbesen et al., 2019), depending on the data used for the analysis (short experimental longline sets by Block et al., 2005, and the NOAA Southeast Fisheries Science Center’s Pelagic Observer Program by Orbesen et al., 2019). Also, results of PSATs data for adult BFT in the GOM estimated a post-release mortality rate of 12%-29% for tagged and released BFT, which combined with their estimated total at-vessel mortality led to an estimated total mortality rate of 59% (Orbesen et al., 2019). Block et al. (2005) hypothesized that warmer waters in the GOM, although being more favorable for eggs and larvae growth and development, are more conducive to metabolic stress in adult spawners. Also, the joint effect of warmer, less oxygenated waters and interactions with PLL fisheries could result in a combination of higher thermal and hypoxic stresses, capture and fighting related traumas, and eventual asphyxiation due to the inability to effectively ram ventilate while remaining on the line (Block et al., 2005; Orbesen et al., 2019). According to the scenario postulated by Block et al. (2005) and the physiology of BFT, it seems likely to assume that projected water warming in the GOM will favor increasingly hypoxic conditions in the water column (Biasutti et al., 2012; Karnauskas et al., 2013). In turn, these water conditions may exacerbate post-release mortality in BFT during their spawning season (Medina et al., 2002; Teo et al., 2007; Dell’Apa et al., 2018).
Climate-driven reductions in pelagic DO levels in the GOM may increase post-release mortalities for other bycaught HMS. Many billfishes and large pelagic sharks are commonly captured and released in PLL and recreational fisheries in the GOM and CS (Diaz, 2008; Mohan et al., 2020), with DO levels below limit thresholds that can result in higher metabolic stress in these species (Carlson and Parson, 2001; Prince and Goodyear, 2006; Prince et al., 2010). Encouragingly, in regard to istiophorid billfishes, recent results of a global meta-analysis suggest that these species may quickly recover from physiological and metabolic stresses associated with fishing captures, as approximately 86% survive (i.e., ~14% post-release mortality) after being released from recreational, longline, and harpoon fishing gears (Musyl et al., 2015). Similarly, istiophorid billfishes recreationally caught and released, after having been tagged with PSATs, exhibited post-release mortality rates of 17-26% (Domeier et al., 2003; Horodysky and Graves, 2005).
In contrast, discard mortalities are generally higher in sharks, and particularly in obligate ram-ventilating species (e.g., Carcharhinidae and Lamnidae), due to the fact that their respiratory mode is compromised during capture (Ellis et al., 2017). Additionally, coastal and pelagic sharks are caught by multiple commercial (including PLL fisheries) and recreational fishing gears32. In the Northwest Atlantic, the most common shark species caught by PLL fisheries are BLS, PBS, SMS, and THR, although the bycatch rate of BLS is far larger than other species (Skomal, 2007; Oliver et al., 2015). However, a study by Campana et al. (2016) estimated lower at-vessel mortality (14.7%) and post-release mortality (9.8%) in BLS compared to the two lamniformes PBS (mean at-vessel mortality at 43.8%, and post-release mortality at 27.2%) and SMS (mean at-vessel mortality at 26.2%, and post-release mortality at 31.3%). These results are in line with the expectation for obligate ram-ventilating and endothermic lamnid sharks, PBS and SMS, to be more impacted by PLL fisheries interactions due to their higher metabolic rates and oxygen requirements than the ectothermic BLS (though also a ram-ventilating shark). Consequently, while hooked on the line, PBS and SMS have a reduced ability for ram ventilation and, thus, higher probabilities to asphyxiate (Bernal et al., 2012; Campana et al., 2016). Furthermore, because physical, thermal, and hypoxia-related trauma can contribute to higher stress and either at-vessel and post-release mortalities, obligate ram-ventilating sharks will be more likely impacted species among the list of bycaught sharks in relation to decreased DO levels (Bernal et al., 2012; Skomal and Mandelman, 2012; Campana et al., 2016; Dapp et al., 2016).
In general, shark survival in PLL fisheries significantly decreases as Tw increases, DO levels decrease, and soaking time increases, which favor asphyxiation and increased induced metabolic stress in captured sharks (Skomal and Bernal, 2010; Gallagher et al., 2014a; Reinhardt et al., 2018). However, responses and mortality rates differ depending on the species. In this regard, a study by Gallagher et al. (2014a) used historical (1995-2012) bycatch data from the U.S. Atlantic PLL fishery to assess the survival of 12 shark species (BLS; SMS; PBS; OWS; DUS; SBS; SHS; BES; longfin mako shark, Isurus paucus – LMS; night shark, Carcharhinus signatus - NIS; silky shark, Carcharhinus falciformis - SIS; and tiger shark, Galeocerdo cuvier - TIS) in relation to fishery target (SWF vs. tuna), operational, environmental, and biological variables. This study found that survival significantly decreases with increasing soaking time for BLS, PBS, and SIS; and with increasing Tw for SIS, BLS, DUS, and NIS. Additionally, species mortalities differed by target species, with the lowest survival rates in sets targeting SWF for NIS (22.2%), SHS (42.3%), BES (44.9%), and SIS (44.9%), and lowest survival in tuna sets for NIS (43.8%) and LMS (37.4%) compared to other species. In both SWF and tuna sets, TIS and BLS had the highest survival rates (97.1% in SWF sets and 96.5% in tuna sets for TIS, and 82.7% in SWF sets and 87% in tuna sets for BLS).
Morphological, behavioral, and ecological specializations result in hammerheads being more vulnerable to fishery interactions (Gallagher et al., 2014b). Results from Gallagher et al. (2014c), which assessed, among other study objectives, post-release mortality associated with five species of sharks typically captured in the GOM and CS (GHS; TIS; blacktip shark, Carcharhinus limbatus; bull shark, Carcharhinus leucas; and lemon shark, Negaprion brevirostris) indicated that GHS was the most vulnerable to capture stress and fishing mortality among the species analyzed, as suggested by high levels of lactate in blood33. In general, higher levels of blood lactate in sharks captured on a longline translate to higher at-vessel mortality rates (Marshall et al., 2012; Gallagher et al., 2014c). Arguably, decreased DO levels due to climate warming can contribute to higher lactate in white muscle cells due to anaerobic respiration, which increases species post-release mortality.
Based on survival results by Gallagher et al. (2014a), combining SWF and tuna sets and taking into consideration age at maturity and fecundity, the most vulnerable species to the combined effect of PLL fishery interactions and climate-driven environmental changes will likely be LMS, BES, and DUS, followed by SHS, SBS, and SMS. Interestingly, survival for many species, and particularly for BLS, SHS, and NIS, appears to be higher at deeper hooking depths, which was interpreted as a consequence of higher DO levels at deeper, cooler waters favoring the maintenance of efficient respiratory mode and oxygen uptake while captured on the line (Gallagher et al., 2014a). However, despite the existence of a clear relationship between Tw and DO levels in the water, results from other studies, both in the Atlantic (Sulikowski et al., 2020) and GOM (Marshall et al., 2015), suggest that at-vessel mortality in DUS is not associated with Tw but rather is positively associated with longer soaking times. In addition, discrepancies in results for total mortality rates (at-vessel mortality + post-release mortality) between these two studies, with a much higher combined total mortality by Marshall et al. (2015) in the GOM (~97%) versus comparative methodological results by Sulikowski et al. (2020) in the western North Atlantic (~5%, with also no at-vessel mortality observed), were interpreted as a consequence of differences in gear types and environmental factors (Sulikowski et al., 2020)34.
2.3.3 Early life history and juveniles
Less oxygenated or hypoxic water conditions resulting from global climate warming may also affect early life stages in HMS. For example, reduced DO levels are known to decrease survival, growth rate, and development of YFT larvae (Wexler et al., 2011) and BFT larvae (Miyashita et al., 1999), and can also delay hatching time in YFT (Wexler et al., 2011). Additionally, daily instantaneous growth rate of SAF larvae in the northern GOM was found, at times, to be lower in areas within anticyclones, which also had the lowest DO levels compared to other sampled areas (Simms et al., 2010). However, warmer waters within anticyclonic eddies can also lead to higher larval densities than water outside of them (Tidwell et al., 2007). Similarly, juveniles can have lower tolerance to reduced or hypoxic DO levels than adults. For example, juvenile SBS, which are more coastal than adults, appear to have low hypoxia tolerance (critical DO level: 3.5 mg/l - Crear et al., 2019).
Juvenile stages for many large pelagic sharks are primarily concentrated in nursery areas in bays, estuaries, and coastal waters, which are more prone to changes in environmental conditions due to shallower depths and increased primary productivity, vertical stratification, and reduced DO levels (Froeschke et al., 2010; Ward-Paige et al., 2015; Oh et al., 2017). Consequently, as warmer hypoxic waters are projected to increase in estuarine and coastal areas, available shark nursery habitat areas might be reduced in the future, with negative implications for the population, as it was suggested for SBS in the western Atlantic due to climate-driven reductions in nursery habitat within Chesapeake Bay (Crear et al., 2019). Modeling results from climate projections of this basin, paired with fishery-independent data, indicated that juvenile SBS will likely be more abundant in waters with DO levels between 3.5 and 5.4 mg/l - those along the edge of hypoxic areas. The authors hypothesized that juvenile SBS may have a preference for these areas as a strategy to avoid larger sharks, or possibly because these areas have a higher abundance of prey and, therefore, juveniles can take advantage of brief forays into the hypoxic zone to prey on species that are more tolerant to hypoxic waters (Crear et al., 2020).
2.4 Impacts to HMS due to changes in other oceanographic processes
Climate-drive changes in oceanographic processes in the Northwest Atlantic, including current circulation patterns, will have strong impacts on coastal and marine ecosystems (Bryden et al., 2005; Lehrter et al., 2017; Claret et al., 2018), resulting in corresponding shifts in suitable habitat, productivity, and prey abundance that will affect marine species (Parsons and Lear, 2001; Scavia et al., 2002; Grose et al., 2020). For HMS that move through large expanses to complete their life histories, such changes and impacts are commonly predicted through analyses based upon global climate models, whose resulting predictions can at times be conflicting.
The spatial resolution of global climate models can introduce sources of error in the prediction of spatiotemporal responses of species distribution (Stock et al., 2011; Muhling et al., 2015; Nazzaro et al., 2021). Specifically, some climate models are based on numerical ocean circulations that may be biased due to their large spatial resolution (typically 1°) (Bryan et al., 2007; Stock et al., 2011; Saba et al., 2016). This resolution is too coarse for an effective integration of important, smaller scale oceanographic features such as mesoscale (10-100 km) eddies and regional ocean current systems. This is particularly true for the GOM, where these features are key drivers of thermal characteristics of the upper ocean across the basin (Liu et al., 2012), and also for the U.S. Northeast Continental Shelf, where the use of global climate models may have led to underestimating projected ocean surface warming (Saba et al., 2016; Kleisner et al., 2017).
Conversely, projections based on downscaled high-resolution climate models were able to capture a large reduction of the Loop Current (LC) transport by 2050 (Liu et al., 2012) and a significant decline of up to 25% in the LC strength by 2100 (Liu et al., 2015; Muhling et al., 2015). The LC transport warms the GOM basin through episodic formations of warm-core anticyclonic (clockwise) eddies that pinch-off the LC, advancing in a westward direction. These offshore eddies extend through the water column to depths of several hundred meters, and as they reach the continental slope their spinning movement can force exchanges of water masses across the continental shelf (Dietrich and Lin, 1994; Morey et al., 2003; Mendoza-Alfaro and Alvarex-Torres, 2012). Consequently, projected reductions in the LC and the associated decrease in the formation of warm-core eddies are expected to result in lower rates of warming across the GOM, and especially in the northern region, which will impact basin-wide ecosystem processes and linked species responses to Tw changes.
The projected weakening of the LC strength by 2100 will affect spatial pathways for eggs and larvae transport, mortality, and most likely the overall species reproductive success and future population sizes for tunas (e.g., BFT, YFT, and BET) and istiophorid billfishes (Trenkel et al., 2014; Dell’Apa et al., 2018; Cornic and Rooker, 2021). The northern GOM and Florida Strait are considered primary spawning and nursery areas for SWF and istiophorid billfishes, especially BLM and SAF (Richardson et al., 2009; Simms et al., 2010; Rooker et al., 2012). In the GOM, BFT larvae are primarily found along the northwestern continental slope region (Nishida et al., 1998; Teo et al., 2007), but, along with billfish larvae, are also common in waters characterized by highly dynamic oceanographic processes favoring primary productivity, such as within the LC frontal zone in the central GOM (Richards et al., 1989; Teo et al., 2007; Rooker et al., 2012; Domingues et al., 2016) and the boundaries of anticyclonic (warm-core) eddies in the western GOM (Lindo-Atichati et al., 2012). Correspondingly, breeding BFT entering into the GOM in the spring (mainly March and April) have a preference for deeper waters of the continental shelf that are highly influenced by the water transport of cyclonic (cold-core) and anticyclonic (warm-core) eddies (Bakun, 2013), while YFT are distributed throughout the GOM and in shallower waters than BFT (Weng et al., 2009; Teo and Block, 2010). Arguably, a decrease in the strength and influence of the LC, and particularly via a reduction in warm water transport through eddy formation and westward propagation, may negatively impact the western Atlantic BFT population, as breeding BFT have a preference for offshore areas in the western GOM with a presence of mesoscale eddies (Teo et al., 2007; Teo and Block, 2010; Dell’Apa et al., 2018). Also, decreased transport of warmer surface waters by anticyclonic eddies originating from the LC may result in less optimal Tw conditions for tunas and billfish larvae (Teo et al., 2007; Tidwell et al., 2007; Lindo-Atichati et al., 2012; Rooker et al., 2012; Cornic and Rooker, 2021).
Similar to the GOM scenario, different life stages of many HMS in the Northwest Atlantic are associated with characteristic oceanographic features within the region, such as eddies, thermal frontal zones, and positions of major currents (e.g., the Gulf Stream). For example, among pelagic sharks, PBS are commonly caught by Canadian PLL fisheries along colder frontal zones in the spring, most likely because these areas have higher prey abundance (Campana and Joyce, 2004). Both SWF catches and BLM recreational catches appear to be higher in areas associated with oceanic frontal systems, including eddies and ring edges, and also those in close proximity to surface thermal zones, most likely as a feeding strategy like the one theorized for PBS (Hoey, 1983; Podestá et al., 1993; Seki et al., 2002). Similarly, the distribution of BFT schools in the Northwest Atlantic is influenced by the presence of thermal frontal zones, as the result of this species using these oceanographic features as an environmental cue of the distribution and abundance of prey (e.g., clupeidae) that is driven by primary and secondary production concentrated along thermal frontal zones (Humston et al., 2000; Schick et al., 2004; Golet et al., 2013). Therefore, potential climate-driven changes in oceanographic dynamics along the Northwest Atlantic may result in associated changes in HMS spatial distribution to either adjust to thermal changes in the water column or shifts in prey distributions. For instance, latitudinal changes in the Gulf Stream may contribute to thermal changes in the Northwest Atlantic (Saba et al., 2016). Similarly, Gawarkiewicz et al. (2018) reported an increasing influence of warm core rings, originating from the Gulf Stream, in the southern New England continental shelf. Arguably, thermal characteristics of these rings could influence the effects of ocean warming at a more localized scale, further complicating predictions for potential associated shifts in HMS distribution in response to these ocean surface thermal alterations, although more research is needed to test this hypothesis.
3 Discussion
Climate variability, as well as long-term climate-driven changes in ocean conditions and fisheries activities, will affect physiology, phenology, behavior, spatiotemporal distribution, abundance, survival, and reproductive success of HMS in the Northwest Atlantic. Evidence from the Atlantic also suggests that climate change will impact larval survival and recruitment potential, with overall negative implications to stock recruitment and productivity (e.g., Muhling et al., 2015, though more advanced modeling projections have been used to predict the presence of tuna larvae in the Pacific Ocean – see Lehodey et al., 2013; Lehodey et al., 2015). HMS in the Northwest Atlantic will be affected by climate variability operating on multidecadal scales and longer-term, climate-driven changes in marine environmental factors (i.e., Tw, DO levels, and oceanographic processes) and ecosystem processes (e.g., primary and secondary productivities, species interactions). These changes will occur simultaneously and influence each other, resulting in a range of HMS responses. PLL fisheries will also affect HMS stock distribution and abundance as fleets adapt to these climate-induced shifts. This review considers what is known about the multiple effects of climate change and variability to HMS and PLL fisheries in the Northwest Atlantic, but also calls attention to the many scientific gaps that still preclude a comprehensive understanding of these effects. In this regard, several questions remain to be addressed that will help foster climate resilient management for HMS and PLL fisheries in this region. In the section below, we start by describing possible approaches to help answer some of these questions and fill existing gaps to advance stock assessment frameworks in the face of climate change impacts to HMS and PLL fisheries. We then provide a description of implications for management of HMS in the Northwest Atlantic and available pathways that can help improve climate-robust management practices for PLL fisheries in the Northwest Atlantic based on the current status of knowledge identified through the information gathered in this synthesis.
3.1 Improving observation of environmental drivers and ecosystem dynamics on HMS life histories: applications for improving stock assessments and other applications
3.1.1 Improve estimates of key life history parameters
Key environmental drivers of habitat use and ecological dynamics in HMS include Tw, DO levels, and CHL (as a proxy for primary productivity) (Pecoraro et al., 2017), which are influenced by climate change. In particular, and due to their inverse relationship leading to increased metabolic rate as Tw increases, changes in Tw and DO levels co-influence fish species abundance, distribution, and reproduction, as well as physiological stress, thus also resulting in changes in natural mortality rate (M; i.e., mortality rate in absence of fishing mortality) and survival across life stages (Ficke et al., 2007; Pollock et al., 2007; Pörtner and Peck, 2010; Baudron et al., 2014). Ocean warming will directly affect M, which is one of the most important demographic parameters in fish stock assessment models to help estimate population dynamics and stock productivity (Kenchington, 2014). However, it is often difficult to estimate the true level of M due to the challenges of accounting for multiple intrinsic (e.g., growth rate, length and size at maturity, age) and extrinsic (e.g., environmental variables such as Tw and DO) factors that influence the estimation process and that may have data limitations (Punt et al., 2021; Hoyle et al., 2023; Pereira Campos et al., 2023). Also, the effect of ocean warming will depend on species’ positions along the continuum of r-selected (faster growing, smaller body size, early age-at-maturity, long spawning duration, shorter life span) vs. k-selected (slower growing, larger body size, low M, long life span) species’ life histories. In marine fish, it seems that climate-driven changes in environmental conditions can result in greater increases in M in r-selected species compared to k-selected species (Levangie et al., 2021). Ocean warming is likely to be less advantageous for fish populations with more r-selected life history traits, as increased Tw can accelerate some of these traits, such as rapid growth rate, higher M, and earlier maturation (Wang et al., 2020a). For HMS in the Northwest Atlantic, temperate species (e.g., BFT, BET, SWF), and even in larger measure pelagic sharks, have slower life history traits typical of k-selected species, while tropical species (e.g., YFT, SKT, ALT) display faster life history traits more in line with characteristics of r-selected species (Fromentin and Fonteneau, 2001). Arguably, we may expect larger climate-driven increases in M for r-selected compared to k-selected HMS and populations in the Northwest Atlantic, although more empirical studies are needed to confirm this hypothesis.
3.1.2 Improve our understanding of population dynamics and trophic structure
Climate-driven changes in Tw and DO levels in marine ecosystems can alter fish population dynamics and trophic structure at different levels of the food web (Pörtner and Peck, 2010; Roman et al., 2019). However, in the Northwest Atlantic there is still a cursory understanding of predator-prey interactions for HMS and their prey species that contributes to uncertain implications for the impact of changes in trophic structure to HMS. Overall, HMS have a key trophic role as apex predators (e.g., large size species, such as BFT, SWF, and sharks) and mesopredators (smaller to medium size species, such as YFT and ALT), and large pelagic predators are known to regulate the productivity and abundance of their prey populations (Ritchie and Johnson, 2009). At the same time, the selective removal of large pelagic predators by fishing activities has altered the trophic structure of marine food webs (Ward and Myers, 2005; Polovina et al., 2009). It also adds to this complexity the possibility for intra-guild predation across HMS, with larger apex predators such as sharks and billfish regulating the populations of mesopredators such as tropical tunas (Hunsicker et al., 2012), although specific studies are needed in the North Atlantic. Within this complex scenario, it is difficult to predict the complete suite of trophic changes due to climate change that will translate into a shift in population dynamics and abundance across HMS and their prey species. To our knowledge, there are few studies that analyzed climate-driven population level changes across HMS in the Northwest Atlantic based on observed changes in species-specific parameters like growth, maturity, and reproduction, which are important intrinsic factors for M estimation (Kenchington, 2014). In turn, there is a crucial gap in the understanding of species responses to climate-driven changes across life stages that can support fishery management planning. Similarly, there is a paucity of studies that investigated the direct link between changes in primary and secondary production and the resulting effects to HMS populations as high trophic level predators.
Overall, the combined effects of fishing and consumption of apex marine predators can result in large changes in the trophic structure of small pelagic communities, with potential cascading effects throughout the entire local community (Britten et al., 2014; Mariani et al., 2017). Hence, there is a need to improve our understanding of HMS spatial dynamics due to the changing climate and the associated implications to potential changes in local trophic structures. For example, migrations of BFT have been reported east of Greenland, which was not previously observed as an area used by this species (Jansen et al., 2016). A similar habitat expansion was observed for SWF in Norwegian pelagic waters (Sundby et al., 2013). In both cases, these species habitat expansions have been explained by ocean warming and improved habitat conditions (Sundby et al., 2013; Jansen et al., 2016). Overall, the implication of these observed climate-driven change in HMS migratory behavior to local fish communities is uncertain, also in terms of potential cascading effects on these communities, with implications for fishery management that would need to be considered under future climate change scenarios (Jansen et al., 2016; Mariani et al., 2017). Generally, habitat use by top marine predators can change due to a combination of prey patch characteristics (e.g., depth, local density) and physical habitat, which can influence predator patterns (Benoit-Bird et al., 2013). More studies are needed to fill gaps in our understanding of the direct relationships between changes in ocean productivity, shifts in marine biodiversity (Beaugrand et al., 2015), and responses to HMS predator-prey relationships, energy, growth, behavior, and reproduction (Briscoe et al., 2017; Lorrain et al., 2020).
The majority of HMS are also influenced by changes in ocean currents that affect prey concentrations, in particular secondary producers (prey of many HMS larvae) and micronekton (prey of many juvenile and adult HMS) (Lehodey et al., 2013). Also, spawning, reproduction, and feeding grounds of HMS will likely be influenced by climate-driven changes in ocean circulation and mesoscale activity, which will influence vertical stratification and prey distribution (Lehodey et al., 2011a; Lehodey et al., 2011b). As the result of interactions between changes in the marine environment and species-specific physiology and behavior, HMS in the Northwest Atlantic will respond in complex ways to the various climate-driven environmental changes that will occur in this region. Thus, climate-driven changes in primary productivity that will propagate through upper trophic level in the food web will have an impact on HMS in the Northwest Atlantic, which need further attention for research, conservation, and management.
3.1.3 Improve spatially-explicit modeling of HMS
There is an urgent need to expand the comprehensive investigation of the potential impact of climate-driven environmental changes to the North Atlantic marine ecosystem through the use of modeling frameworks that can describe more specifically the spatial dynamics of HMS while also accounting for both the fishing impact to these species and environmental variability. This type of effort has been conducted successfully in the Pacific Ocean through the use of innovative management tools, such as the Spatial Ecosystem and Population Dynamics Model (SEAPODYM) modelling framework, which have contributed to enhance our understanding of climate-driven impacts to tunas and their fisheries in the region and that helped advance management efforts (see results for various tunas in Lehodey et al., 2008; Lehodey et al., 2013; Lehodey et al., 2015). It is promising that researchers have started to conduct initial studies using SEAPODYM to enhance our understanding of the past and present spatial dynamics of ALT population in the North Atlantic (Dragon et al., 2015), with this effort that can hopefully be extended to other HMS in the region.
Importantly, many study results for the links between changes in environmental conditions and HMS behavior and movements are based on correlative analyses, which cannot be unequivocally considered as evidence of a direct association of climate-driven changes in ocean conditions with species responses, or rather due to other indirect causes (e.g., changes in prey distribution and availability), or some combination of these factors (Schlaff et al., 2014). Also, HMS responses will be species-specific (e.g., tropical vs. temperate species), life stage-specific (i.e., adults vs. juveniles), and in many cases region-specific, which can further complicate the prediction of cumulative effects of climate change on HMS (Hobday et al., 2015; Dell’Apa et al., 2018).
3.1.4 Additional needs
There are other climate-driven changes in ocean water conditions and oceanographic processes that have not been included in our review due to a lack of sufficient studies focusing on HMS, but that may be important for understanding the impact of climate change on these species and their fisheries, necessitating more research. For example, although studies have reported changes in olfactory behavior and potential reduction in predatory success in smaller sharks due to ocean acidification (Dixson et al., 2010; Dixson et al., 2015; see also a criticism of these studies by Clark et al., 2020), to our knowledge similar studies are lacking for other large pelagic sharks and also for tunas and billfishes. Additionally, increased ocean acidification may affect the distribution, survival, and growth of tuna eggs and larvae, which requires further investigation (Bromhead et al., 2015). Similarly, global climate projections indicate an increase in the intensity of stronger tropical storms (Knutson et al., 2010), including in the GOM (Biasutti et al., 2012), and some studies suggest that hurricanes and tropical storms can alter the behavior and distribution of both adult and juvenile pelagic sharks (Heupel et al., 2003; Udyawer et al., 2013; Wang et al., 2020b; Gutowsky et al., 2021). However, no direct evidence has been collected for other HMS, although changes in storm patterns may have an influence on tuna and billfish eggs and larvae survival (Dell’Apa et al., 2018). Given the importance of these climate-driven changes on behavioral responses of some HMS, it will be important for future studies to consider a wider array of climatic changes on HMS life stages across the North Atlantic.
3.2 Incorporating impacts of multidecadal climate variability into management advice for HMS
3.2.1 Improve quantitative estimates of environmental data in stock assessments
Multidecadal climate variability, which is also influenced by climate change, can have a significant effect on the distribution of tunas and billfishes in the North Atlantic basin (Chang et al., 2013; Failletaz et al., 2019; Wu et al., 2020), similar to what has been reported for other large pelagic species in the Pacific Ocean (Lehodey, 2001; Su et al., 2011). Accordingly, this highlights the importance of integrating (quantitatively rather than qualitatively) species-specific responses to climate variability, as well as species responses to changes in other environmental factors influenced by this variability, into HMS’ stock assessment analyses and management plans in the Atlantic Ocean, which can help managers plan for near-term (i.e., within the next year to 10 years) changes and to understand how responsive management interventions need to be. Such analyses should be inclusive of shark populations as they represent a considerable amount of bycatch in PLL fisheries and large pelagic sharks can be impacted by climate variability (Skubel et al., 2018). The incorporation of environmental covariates into stock assessment models is becoming more common (Keyl and Wolff, 2008). For example, a recent model illustrated an improved model fit when the AMO was integrated as a driver of catchability of BFT (Walter et al., 2018; Hansell et al., 2020). Similar approaches could improve stock assessment models for other HMS whose distribution and abundance is influenced by climate variability (Chang et al., 2013; Failletaz et al., 2019; Hansell et al., 2020; Wu et al., 2020). Additionally, most HMS stock assessments are single-species, precluding evaluations of interactions between species (Stock et al., 2011). Therefore, multispecies and ecosystem models that include measures of interannual and interdecadal variability could yield important insights for HMS management.
3.2.2 Improve species-specific habitat suitability modeling
There is also a need to increase the number of habitat suitability models across HMS species, and especially for bycatch species, that integrate key environmental factors (e.g., SST, MLD, SSHA, and CHL), which are primarily driven by climate variability, to better predict species distribution in relation to environmental changes in the Atlantic Ocean (Chang et al., 2013; Druon et al., 2016; Erauskin-Extramiana et al., 2019). In this regard, comprehensive analyses of species habitat models, based on animal movement data collected through advanced remote sensing and tagging technologies, and integrated with environmental data and climate projections that are responsive to interannual and decadal scale variability can help predict species habitat shifts as part of a dynamic and adaptive ocean management strategy (Hazen et al., 2013; Hobday et al., 2013; Hazen et al., 2018). Accordingly, there is a need to expand tagging efforts for bycatch species in the North Atlantic to support the development of habitat models, similar to efforts conducted in the Pacific Ocean that helped advance the understanding of animal movement in relation to ocean temperatures and other environmental processes (Block et al., 2011). This will further advance management efforts within the ecosystem-based fisheries management framework (Link, 2010), consistent with the long-term management strategy for U.S. federal fisheries resources (NMFS, 2016a; NMFS, 2016b). Although predicting the full suite of HMS responses to climate changes and variability is a challenging task, also due to the broad spatial scales used to complete their life histories, scientific efforts to that end are currently underway in the North Atlantic (see https://fisheriesclimatetoolkit.sdsu.edu/). Better information will help the U.S. Atlantic PLL fishery and management to be more adaptive and enhance climate resilience.
3.3 Incorporating long-term climate change impacts (i.e., changes in ocean circulation, ocean warming, and reduced DO levels in the water column) into management advice for HMS
3.3.1 Improve projections of oceanographic conditions and impacts on species abundance and distribution and the use of this information in management
Analyses of multidecadal to centennial climate-driven changes in marine environmental conditions and species distributions may be significantly limited by the use of global climate models that, compared to downscaled high-resolution climate models, may not resolve finer-scale features and processes that are important to marine species (Liu et al., 2012; Saba et al., 2016; Kleisner et al., 2017). Although computationally intensive, high-resolution climate models can help provide a better understanding of climate change impacts to ocean ecosystems (Saba et al., 2016; Kleisner et al., 2017), and when used to develop species habitat suitability models, can generate modeling results that more adequately represent the reality of localized systems (Stock et al., 2011). More accurate estimates for regional changes in ocean circulation, including mesoscale features such as eddies, rings, and frontal zones, can be generated by using downscaled high-resolution climate models and complemented with in situ oceanographic monitoring, with appropriate understanding of a given model’s ability to project future conditions (Czaja et al., 2019). Overall, potential changes in distribution and reproductive functioning need to be integrated into projections of species abundance to better evaluate the impacts of PLL fishery interactions in the North Atlantic.
Climate-induced oceanographic changes will be important considerations in the management of both target and bycatch HMS, particularly large pelagic sharks. In general, sharks select specific thermal conditions, with a preference for frontal boundary habitats distinguished by steep SST and productivity gradients, including frontal zones (Campana and Joyce, 2004; Queiroz et al., 2019). Oceanic frontal regions of the Gulf Stream and the North Atlantic Current/Labrador Current convergence zone in the Northwest Atlantic, which are characterized by high productivities, represent key hotspots of overlap between large pelagic sharks and PLL fisheries in the North Atlantic, with increased shark susceptibility to bycatch and overfishing (Queiroz et al., 2019). Potential changes in the Gulf Stream position and strength will also affect the distribution of target species (i.e., SWF and tunas) for the PLL fishery and, consequently, the geographic distribution of fishing fleets targeting those species. For example, a key area for SWF along the Northwest Atlantic, which is periodically influenced by the presence of eddies and rings developing from the Gulf Stream, is the Charleston Gyres region (Sedberry and Loefer, 2001), which is an important fishing ground for the PLL fishery targeting SWF (Cramer, 1996; Cramer, 2001). Thermal fronts created by the Charleston Bump are areas where SWF larvae are readily found (Govoni and Hare, 2001), and large numbers of juvenile SWF are discarded by the PLL fishery operating in the vicinity of the Charleston Gyre (Cramer, 2001), further suggesting that these waters might serve as a nursery for SWF (Govoni et al., 2003). Therefore, improved predictions of climate-induced changes in the dynamics of frontal zone and ocean current edges (e.g., the Gulf Stream edge – Saba et al., 2016) in these areas can provide more realistic information on potential shifts in both target species and PLL fleet distributions that, in turn, will affect the vulnerability of pelagic sharks and other bycatch HMS to the PLL fishery.
Climate change will also affect HMS’ horizontal and vertical distributions, and these changes in species distribution will have implications for PLL fisheries in regard to shifts in fleet distributions and species catchability that will need to be carefully considered when addressing challenges for fisheries that have developed their operations, supply chains, and management regimes around the current distributions of HMS. Changes in species catchability due to global ocean warming and changes in other environmental factors should be accounted for in estimates of stock abundances (e.g., maximum deviation of Tw from SST, and minimum Tw fished in each set; for details see Lynch et al., 2018), both when statistically standardizing indices of abundance and in the overall stock assessment.
For a more comprehensive evaluation of the impacts of climate change and altered PLL fishing pressures to pelagic sharks, we must recognize the role of ontogenetic shifts in distribution in many shark species. This is particularly true for coastal-pelagic sharks for which early life stages rely on shallow estuarine and coastal habitat as nurseries areas (Heupel et al., 2007) and may be more affected than adults by warmer and less oxygenated waters. Such appears to be the case for SBS in the Northwest Atlantic (Crear et al., 2019; Crear et al., 2020). In other words, management measures introduced to reduce adult bycatch, while allowing PLL fisheries to cope with climate change, will likely be less effective for the success of population recovery or conservation if climate-driven changes to juveniles habitat, distribution, and mortality are not also taken into consideration.
3.3.2 Adopt more dynamic spatial management approaches that build on more comprehensive data
Changes in distribution and behavior due to climate warming will generally be more pronounced in ectothermic than endothermic species, and in juveniles compared to adults. For pelagic sharks, the negative effects of climate warming would most likely depend on animal size and life stage, with smaller bodied species and juveniles experiencing a larger change in vertical habitat distribution due to their lower thermal inertia capacity (i.e., higher surface area:volume ratio) compared to larger sharks and adults (Schmidt Nielsen, 1984; Skubel et al., 2018), though more research is needed to support this hypothesis.
With respect to DUS, this species is of great conservation concern in the Northwest Atlantic,35 which emphasizes the need to account for the species responses to climate-driven environmental changes and the implications for fisheries management. Climate warming will most likely lead to a geographic shift in the Northwest Atlantic population of DUS (Hare et al., 2016), which is already resulting in a mismatch between juvenile DUS migration patterns and the current location and timing of the MASCA (Bangley et al., 2020). Hence, more dynamic and responsive spatiotemporal management will be key for addressing distribution shifts in DUS, and arguably also in other HMS (Hammerschlag et al., 2022). Additionally, more dynamic spatial management approaches can help address species distribution shifts and be less impactful economically to the fishing community. In particular, research by Hazen et al. (2018) conducted in the U.S. Pacific Ocean suggested that dynamic closures could be two to 10 times smaller than existing static closures while still providing adequate protection to bycatch species.
The development and implementation of dynamic area closures will also need to be framed within the context of the impacts of other fisheries in the North Atlantic, both commercial and recreational, to bycatch HMS. For example, many of the prohibited sharks, including DUS, are caught in other fisheries including bottom longline, gillnet, (NMFS, 2019b) and recreational fisheries (Ajemian et al., 2016). Preliminary results, characterized by high levels of variability, estimated that between 2005 and 2015 more than 66 million sharks across 35 different species were caught by recreational anglers along the U.S. eastern Atlantic coast alone, including more than 1.2 million sharks from prohibited species (Kilfoil et al., 2017). Many of these sharks may not survive after being released due to a considerable amount of physiological and metabolic stresses experienced during capture (Kneebone et al., 2013), thus representing a significant source of fishing mortality.
3.3.3 Modify gear usage to ameliorate climate effects and ensure sensitive life histories are protected
In the Atlantic Ocean, among the list of species of highest concern due to overfishing and associated historical decline in abundance are OWS and DUS (Pacoureau et al., 2021), which are also among the key species most impacted by climate change. OWS is an ectothermic shark that may expand its horizontal distribution, and will likely shift its vertical distribution to deeper waters as a thermoregulatory strategy to maintain optimal physiological performance in Tw colder than 28°C (Andrzejaczek et al., 2018). OWS is a common bycatch in PLL fisheries in tropical waters and has experienced a severe decline in global abundance trends due to excessive fishing pressure, including shark finning (Clarke et al., 2012; Tolotti et al., 2015b). Consequently, gear modifications to use deeper sets and avoid areas that are considered potential or effective mating and pupping grounds (i.e., northern and central Bahamas - Howey-Jordan et al., 2013), could help mitigate the potential impacts of climate change to OWS by reducing its catchability on vulnerable life history stages. In this regard, OWS catch rates significantly increase as hook fishing depth decreases in PLL fisheries both in the tropical Atlantic (Tolotti et al., 2013) and the Pacific Ocean (Nakano et al., 1997). However, such gear changes should be evaluated as to the impacts on the catchability of other target and bycatch species (Tolotti et al., 2015a).
A potential increase in the minimum allowable depth of nighttime fishing gears might be an effective management measure to reduce species catchability and bycatch mortality for certain species, although it has yet to be tested and implications for target species are unknown (Cartamil et al., 2010). For example, given the diurnal changes in THR depth distribution, with adults being closer to the sea surface at night (Cao et al., 2011; Cartamil et al., 2011), this species may be more susceptible to fisheries interactions occurring at night in surface waters (e.g., certain PLL fisheries targeting SWF). Similarly, juvenile SHS occupying deeper depths than adults in the upper water column (0-50 m) at night have important implications for fishery management. Specifically, this behavior was theorized to increase the susceptibility of juvenile SHS to fishing pressures from PLL fisheries operating at night in deeper waters (Santos and Coelho, 2018). Theorized increases in juvenile SHS catchability in the PLL fishery operating at nighttime could be influenced by climate-driven changes in water conditions, including Tw, changing vertical habitat use across life stages and altering species vulnerability to the fishery. Catches would reduce population recovery by disproportionately impacting survival in juveniles.
Globally, ocean warming will create deoxygenated conditions in the water that will reduce habitat suitability for many HMS, although mainly in relation to depth and based on species-specific sensitivities to DO levels, and co-dictate their region-specific distributions. In particular, reduced DO levels, including hypoxic conditions, are already a permanent water column feature in the eastern tropical Atlantic, where a large OMZ is expected to further expand vertically and thus reduce habitat suitability for tropical HMS (Stramma et al., 2012). This is especially true for epipelagic species such as BLM and SAF (Stramma et al., 2012), but also BLS (Vedor et al., 2021), and possibly other pelagic shark species (Sims, 2019) and tunas (Leung et al., 2019). This habitat compression may increase the susceptibility of bycatch species to surface fishing gear (e.g., PLL fisheries) operating across the region above the OMZ (Prince et al., 2010; Stramma et al., 2012). However, habitat compression in the eastern tropical Atlantic may result in more foraging opportunities for HMS due to the fact that their prey (e.g., scombrids, clupeidae, cephalopods) are concurrently affected by similar surface-compressed habitat constraints (Prince and Goodyear, 2006; Prince and Goodyear, 2007; Stramma et al., 2012; Sims, 2019)36. It is worth noting that the majority of adult HMS are considered opportunistic feeders, and so these species may be able to adapt to forage on other prey. However, deoxygenated waters can influence critical life processes other than feeding, such as spawning, intra- or interspecific interactions and competition, and migrations, as a behavior response to seek more favorable environmental conditions. Moreover, various life stages may be affected by DO changes differently due to distinctive oxygen needs. Hence, climate-driven changes in DO levels will potentially lead to desynchronization of key processes in HMS, resulting in negative effects at the population level (Thackeray et al., 2016; Erauskin-Extramiana et al., 2020).
Changes in species behavior and vertical distribution have direct implications for the fishery management of HMS in a changing climate. Increased concentrations of fish near the ocean surface due to habitat compression in the eastern tropical Atlantic may result in higher catches (both of HMS and their preferred prey) inside versus outside compressed shoaling areas (the “shooting fish in a barrel” scenario – Sims, 2019). This scenario may result in hyperstability (Hillborn and Walters, 1992), as higher CPUEs in the compressed area are not necessarily related to true species abundance but due to differences in species density. Hence, environmental influences (e.g., DO levels and Tw at depth of the fishing gear) on species distribution need to be effectively integrated into stock assessments to avoid incorrect stock status perceptions between the eastern and western regions in the North Atlantic (Bigelow and Maunder, 2007; Prince et al., 2010). In this regard, more HMS studies using temperature-DO-depth recording tags rather than modelled data are needed to provide detailed information on changes in species behavior and vertical distributions (Coffey and Holland, 2015), which will help our understanding of how HMS may respond to climate-induced environmental changes. Additionally, management planning and implementation of PLL fisheries would benefit from the integration of information on climate-driven projected changes in species vertical distributions as responses to ocean warming and associated changes in the depth of the oxycline. Higher capture rates in more deoxygenated waters may increase fishing mortalities (i.e., immediate mortality plus post-release mortality) of bycatch species, such as sharks, billfishes, BFT (and especially in the warmer GOM during the spawning season), and other undersized HMS. These results are especially concerning for pelagic sharks given their dramatic decrease in abundance and associated increase in global extinction risks across species in recent years caused by overfishing, including for bycatch species in the North Atlantic, resulting in a global abundance decline of 71% (overall abundance decline of 46% in the North Atlantic) since 1970 due to an 18-fold increase in relative fishing pressure (Pacoureau et al., 2021).
From a fishing practice perspective, a key recommendation to facilitate higher bycatch survival of large pelagic sharks, and especially for obligate ram-ventilating species in warmer waters like the GOM and CS, would be to use longer gangions that can allow these sharks to reach deeper, more oxygenated waters while hooked at the line, in combination with shorter soaking times. This recommendation goes along with the best management practice of using circle hooks rather than J hooks in PLL and recreational fisheries, as much as possible (already mandatory in the U.S. Atlantic PLL fishery and when targeting sharks recreationally in U.S. federal waters) (Cooke and Suski, 2004; Serafy et al., 2009; Musyl et al., 2015; Reinhardt et al., 2018; Rudershausen, 2019).
4 Conclusions
Over the coming decades, major climate-driven changes in marine environments are projected to occur globally. The pace and intensity of such changes will be particularly strong in the Northwest Atlantic and will affect the biology, ecology, distribution, abundance, survival, reproductive success, and management of HMS in this region, although effects, both in magnitude and directionality, will differ based on species and life stages. Some climate-driven changes appear to be more urgent than others in terms of their impacts to HMS. In particular, ocean warming and associated water deoxygenation have most likely already altered species behavior and distribution, and will continue to contribute to these changes further triggering species-specific responses. Many temperate species (e.g., SWF) are already shifting poleward, a trend that is likely to continue, while more tropical species (e.g., YFT, SKT) will likely expand their ranges toward northern latitudes and into deeper waters as ocean warming continues. Warmer, less oxygenated waters may result in higher post-release mortality in some HMS (e.g., large pelagic sharks). Conversely, other projected climate-driven changes in the ocean environment, and their impacts to HMS, will be more evident in years to come. Chiefly, climate change and climate-driven changes in interdecadal climate variability will alter the dynamics of large-scale (e.g., global and regional ocean currents) and mesoscale (e.g., frontal zones, eddies, gyres) oceanographic processes that regulate HMS behavior and distribution, as well as fishery dynamics since these processes provide important cues to HMS presence and abundance (e.g., SWF presence in relation to frontal zones).
Because fisheries are social-ecological systems, effects of climate change and climate variability on HMS need to be viewed through the lens of feedbacks between changes in fisheries behavior, oceanographic dynamics, and associated responses in the species they exert pressure on. For some species (e.g., DUS, BFT), changes in their geographic range, migration timing, and reproductive phenology will result in misalignment between their spatiotemporal distribution and current spatial and seasonal fishery restrictions intended to protect these species. To address this misalignment, some of the existing spatiotemporal restrictions would need to be revised to account for climate-driven shifts in species distribution and seasonal presence. Also, HMS stock assessments should strive to account for impacts of climate-induced changes in environmental conditions to species growth, survival, distribution, and recruitment, and associated changes in species abundance through the integration of species-specific responses to climate change and variability. Species interactions with fisheries, including species-specific vulnerability to fishing gears and survival, will likely change as well and will require focused research aimed at developing adaptive best practices (e.g., changes in soaking times, allowable fishing depths, and gangions length) that can aid in species recovery, conservation, and protection.
Increasing our understanding of the most likely responses of HMS and their habitats to changes in the marine environment (e.g., expanding tagging efforts to less studied species, developing more habitat suitability models, employing higher-resolution climate models to project changes in ocean conditions and dynamics) is a necessary step to help build more resilient PLL fisheries in the face of climate change. In this regard, the current scientific knowledge on climate change impacts to HMS is fragmented, especially for bycatch species. Filling these knowledge gaps and supporting more adaptive management frameworks that can account for species shifts in geographic and vertical distributions are needed to help conserve target and bycatch HMS. By gaining a more holistic understanding of climate change impacts and increasing the adaptability of current management approaches, fisheries stakeholders can build more resilient and sustainable PLL fisheries in the North Atlantic Ocean.
Author contributions
Authorship was arranged, in descending order, by the level of contribution of each author to different aspects of the manuscript. AD, RB, and KK conceived the ideas and framework for the review. AD and KK designed the methodology. AD and RB reviewed the literature and compiled the information to be included in the review. AD and KK created, reviewed, and edited the tables. AD wrote the manuscript with RB. RF, and KK contributing critically to the drafts, and all authors reviewed and edited all versions of the manuscript. AD coordinated efforts to complete the manuscript and submit to journal. All authors contributed to the article and approved the submitted version.
Funding
This work was supported by Builders Initiative.
Acknowledgments
We are grateful to Katie Westfall, Enric Cortés, Tobey Curtis, and Jeremy Vaudo for providing helpful and constructive comments on the manuscript. We also thank three reviewers for their thoughtful and thorough comments which helped to improve the paper.
Conflict of interest
The author(s) declared that they were an editorial board member of Frontiers, at the time of submission. This had no impact on the peer review process and the final decision.
Publisher’s note
All claims expressed in this article are solely those of the authors and do not necessarily represent those of their affiliated organizations, or those of the publisher, the editors and the reviewers. Any product that may be evaluated in this article, or claim that may be made by its manufacturer, is not guaranteed or endorsed by the publisher.
Supplementary material
The Supplementary Material for this article can be found online at: https://www.frontiersin.org/articles/10.3389/fmars.2023.1206911/full#supplementary-material
Footnotes
- ^ Throughout the manuscript, and based on the IPCC (2012) definitions, we consider “climate variability” as interannual (multi-year) or interdecadal (occurring over more than one decade) fluctuations in temperature and precipitation, while “climate change” as the more persistent (occurring over centuries) deviations from long-term averages.
- ^ The complete mechanistic process among these three indexes of climate variability (i.e., AMO, AMOC, and NAO) is not yet fully understood (Delworth and Mann, 2000; Knight et al., 2006; Börgel et al., 2020). Regardless, the AMO, which is based on SST fluctuations and expressed over timescales between 60-80 years (Kushnir, 1994; Moore et al., 2017), is a major driver of changes in marine processes and ecosystem state across the North Atlantic and the GOM (Liu et al., 2012; Karnasuskas et al., 2015), including changes in primary productivity and species distribution (Nye et al., 2014).
- ^ This analysis used standardized catch per unit of effort, CPUE, of longline fisheries from 1971 to 2010; for details see Wu et al., 2020.
- ^ Multidecadal natural climate variability is also affected by the by-product of anthropogenic greenhouse gas emissions that have dramatically amplified recent changes in other physicochemical characteristics of ocean waters (Brander, 2007).
- ^ However, Brill et al. (2005) recommended avoiding a classification into “tropical” and “temperate” tunas because these terms usually refer to sea surface conditions, despite the fact that these species do not live solely at the sea surface.
- ^ Between 300-500 m in the Atlantic (Matsumoto et al., 2005), and between 100-400 m in the tropical Atlantic (Yang et al., 2020).
- ^ Generally, the metabolic rate (also considered as oxygen consumption rate) in ectothermic sharks increases two to three-fold for every 10°C rise (known as Q10 - Schmidt-Nielsen, 1983) in Tw (Bernal et al., 2012).
- ^ The bigeye thresher shark (Alopias superciliosus, BES) may be the only exception to this scenario among ectothermic sharks, as the species exhibits a puzzling thermal behavior and physiological traits that makes it a potential candidate for the presence of regional endothermy (see Supplementary Material). Specifically, BES exhibit a preference for warmer surface waters (20-26°C, and 10-100 m) at nighttime, while diving to colder and deeper waters (6-12°C, and 300-600 m) at daytime (Weng and Block, 2004; Smith et al., 2008; Stevens et al., 2010). This greater thermal tolerance is also expressed by the fact that BES is able to remain at this colder water depth (at times even down to 4°C) during the day for a prolonged time (between 6 and 8 or more hours) (Nakano et al., 2003; Weng and Block, 2004).
- ^ i.e., the ability of an animal to selectively move between different thermal environments to maintain constant body temperature, or as a response to optimize energetic benefits, including for growth, feeding, digestion, reproduction, and gestation time (Carey and Scharold, 1990; Economakis and Lobel, 1998; Bernal et al., 2012; Speed et al., 2012; Howey-Jordan et al., 2013; Thums et al., 2013; Papastamatiou et al., 2015).
- ^ Occasional brief dives (average of ~13 minutes) to deeper waters (maximum recorded depth of 1,082 m) have also been reported for this species in the Northwest Atlantic and interpreted as a foraging strategy (Howey-Jordan et al., 2013).
- ^ DUS occasionally dive to deeper waters outside the shelf edge (maximum recorded depth at 573 m - Hoffmayer et al., 2014).
- ^ The MASCA was established in 2005 to close seasonally (from January 1 through July 31) a coastal area off North Carolina to bottom longline fisheries to protect overwintering habitat for juvenile sharks (Bangley et al., 2020).
- ^ GHS is considered more pelagic than SHS and SMH and is occasionally found as bycatch in PLL fisheries in oceanic tropical waters (Castro, 2011), while SMH is also found in pelagic waters in the eastern Atlantic (Santos and Coelho, 2018).
- ^ This adult female SHS remained at the surface (0-60 m) for the majority of the daily time, while spending ~40% of the nightly time at depth below the MLD with multiple dives to depths of ~1,000 m. During the day the shark experienced Tw between ~13 and ~30°C and spent ~87% of the time at Tw between 23 and 28°C, while nighttime Tw ranged from ~6 to ~30°C with the majority of the time spent at Tw between 26 and 28°C (Hoffmayer et al., 2013).
- ^ It is unclear if this decrease is due to projected rising SST, projected changes in primary productivity, or a combination of these two factors. In fact, seasonal movements of SMS in the eastern North Pacific (Block et al., 2011; Hazen et al., 2013) and GOM (Vaudo et al., 2017) are correlated with changes in CHL (a common proxy for ocean productivity).
- ^ Casey and Kohler (1992) theorized the presence of seasonal migrations for SMS along the Northwest Atlantic. Based on this hypothesis (i.e., the “Sargasso Sea” hypothesis), SMS are primarily distributed off the continental shelf of the U.S. mid-Atlantic region in the spring. They migrate northward along the shelf to the Gulf of Maine and offshore the Georges Bank region between U.S. and Canada during the summer and early fall, and then move offshore toward overwintering grounds in the warmer Gulf Stream and further south to the Sargasso Sea. Recently, these movement patterns, which are clearly linked to changes in Tw, were partially confirmed by satellite telemetry data of tagged-and-released SMS (Vaudo et al., 2017). Although this recent study confirmed the importance of the U.S. Mid-Atlantic Bight as a core region for SMS distribution, it also suggested that the Sargasso Sea during summer might be simply a migration corridor to more productive waters further south, as tagged sharks did not linger in the region, most likely due to lower productivity and, potentially, low prey abundance (Vaudo et al., 2017). These results further suggest that adult SMS may be less impacted by climate-driven changes in ocean Tw compared to corresponding fluctuations in primary productivity and prey distribution and habitat use, which requires further investigation.
- ^ Higher body mass generally corresponds with lower Q10 in elasmobranchs, indicating lower sensitivity to Tw for larger-bodied versus smaller-bodied species (Du Preez et al., 1988).
- ^ Preliminary results of catch records from fishery-dependent data in this region indicate that, across all life stages and almost exclusively for young of the year individuals, THR are mainly distributed in waters within the continental shelf (< 200 m), with a less frequent occurrence in offshore pelagic waters throughout the Northwest Atlantic (Kneebone et al., 2020).
- ^ Young of the year THR are found primarily in coastal waters between the Mid-Atlantic and southern New England during the summer, with higher catches in shallower waters (< 50 m) off North Carolina during the winter. As they grow through maturity, juvenile and adult THR move into deeper waters (Kneebone et al., 2020), a behavior that was also reported in the Northeast Pacific (Kinney et al., 2020). The results of Kneebone et al. (2020) also suggest that THR undertake north-south seasonal migrations along the Northwest Atlantic, similar to what is anecdotally reported in the Northeast Pacific for this species (based on preliminary results of PSAT tags data off California - Smith and Aseltine-Neilson, 2001).
- ^ Global results from PSATs data indicated the presence of diel vertical migrations in THR (although this behavior has not been observed in the Northwest Atlantic), with a preference for shallower depth in the warmer mixed layer (0-200 m) at nighttime, likely for foraging, and most of the daytime spent at deeper (maximum recorded depth at 640 m in Australia - Stevens et al., 2010) and cooler depths below the thermocline (> 200 m) (Cao et al., 2011; Cartamil et al., 2011).
- ^ PBS is characterized by the presence of two separate stocks between the eastern and western North Atlantic (Campana et al., 1999; Francis et al., 2008; Skomal et al., 2021).
- ^ PBS exhibits a north-south seasonal migration between the Gulf of Maine and Newfoundland, Canada, with sharks captured primarily in northeastern waters along the continental shelf in the spring and in more southwestern inshore areas in the summer and fall (Campana et al., 1999; 2002; 2003). Similar seasonal changes in distribution have been recently confirmed for PSAT-tagged juveniles, showing their presence off shelf waters in the Gulf of Maine and George’s Bank during the fall, followed by their transition into offshore waters in the Northwest Atlantic during the winter. Also, during this wintertime residence, some juveniles moved into deeper (colder) mesopelagic (200–1000 m) waters in the Gulf Stream. The tagged juveniles moved to warmer shelf waters in the spring before returning to the Gulf of Maine and Scotian Shelf in the summertime (Skomal et al., 2021).
- ^ Most likely, this represents a feeding strategy and further questions the Gulf Stream parturition hypothesis advanced by Campana et al. (2010) (Skomal et al., 2021).
- ^ This is the highest greenhouse gas emissions scenario that is commonly used to assess the consequences of anthropogenic climate change.
- ^ This analysis used nominal CPUE from the Japanese pelagic longline fishery between 1958 and 2004; for details see Erauskin-Extramiana et al. (2019).
- ^ From 1981 to 2005 for BFT, and from 1967 to 2005 for ALT; for details see Dufour et al. (2010).
- ^ Results by Rooker et al. (2008) suggested that as many as 60% of sampled juvenile BFT in the West Atlantic foraging areas were likely born in the Mediterranean Sea.
- ^ A recent study by Richardson et al. (2016) reported spawning of likely smaller BFT in slope waters in the Northwest Atlantic, between the Gulf Stream and continental shelf, although these results were based on a limited number of larvae collected in the area sampled and, thus, did not clarify if this potential spawning area can effectively contribute to the western Atlantic BFT population.
- ^ i.e., the depth at which the oxygen partial pressure - pO2 - of ambient water is equal to the species blood P50 values, where P50, a measure of blood oxygen affinity, is considered the pO2 at which blood is 50% saturated and that is commonly used as a proxy for a species tolerance to low ambient oxygen; for details see Mislan et al. (2017).
- ^ Bycatch HMS can also incur in fishing mortality prior to retention and discard (i.e., immediate mortality or at-vessel mortality). Hence, the total fishing mortality in bycatch species (i.e., discard mortality) is a measure of the combined result of species survival at retrieval and their survival after being discarded (i.e., total discard mortality = immediate mortality + post-release mortality) (Dapp et al., 2016).
- ^ However, all legal-sized BFT found dead at haul-back must be retained and counted against an individual quota allocation.
- ^ Critically, recreational catch-and-release fishing for sharks has been increasing in popularity in the Northwest Atlantic (Dell’Apa et al., 2015; Kilfoil et al., 2017) and GOM (Shiffman and Hammerschlag, 2014; Ajemian et al., 2016).
- ^ Lactate is the main by-product of anaerobic metabolism in white muscles that is produced, for example, during strenuous exercise, under which the cellular demand rate of oxygen is higher than the supply rate by the cardiovascular system; for details see Gallagher et al. (2014c).
- ^ Specifically, higher mortalities in Marshall et al. (2015) were likely due to the use of bottom longline fisheries (i.e., this fishery uses shorter branch lines, or gangions, of ~2 m length that can effectively limit movement and ram-ventilation in sharks captured on the line) during summer (i.e., a time for, in general, more hypoxic conditions in the northern GOM) to capture sharks. In contrast, in Sulikowski et al. (2020) sharks were captured using commercial PLL fishery gear (i.e., longer gangions length of ~20 m, allowing sharks to swim while hooked on the line and access deeper and more oxygenated waters) in a more temperate region during the month of May (i.e., lower Tw compared to those in the northern GOM during summer).
- ^ The most recent stock assessment for DUS indicated that the population is overfished and still experiencing overfishing due to fishery bycatch, including PLL fisheries, and highly k-selected life history characteristics in the species (Romine et al., 2009; SEDAR, 2016).
- ^ Interpreted as the result of enhanced predator-prey interactions, certain studies showed larger average sizes for SAF landed in the eastern tropical Atlantic and eastern tropical Pacific compared to fish caught in western, non-compression areas (Prince and Goodyear, 2006; Prince et al., 2010).
References
Abecassis M., Dewar H., Hawn D., Polovina J. (2012). Modeling swordfish daytime vertical habitat in the North Pacific Ocean from pop-up archival tags. Mar. Ecol. Progr. Ser. 452, 219–236. doi: 10.3354/meps09583
Ajemian M. J., Jose P. D., Froeschke J. T., Wildhaber M. L., Stunz G. W. (2016). Was everything bigger in Texas? Characterization and trends of a landbased recreational shark fishery. Mar. Coast. Fish. 8, 553–566. doi: 10.1080/19425120.2016.1227404
Andrzejaczek S., Gleiss A. C., Jordan L. K. B., Pattiaratchi C. B., Howey L. A., Brooks E. J., et al. (2018). Temperature and the vertical movements of oceanic whitetip sharks, Carcharhinus longimanus. Sci. Rep. 8, 8351. doi: 10.1038/s41598-018-26485-3
Arocha F., Lee D. W., Marcano L. A., Marcano J. S. (2001). Update information on the spawning of Yellowfin tuna, Thunnus albacares, in the Western Central Atlantic. ICCAT Collect. Vol. Sci. Pap. 52, 167–176.
Arrizabalaga H., Dufour F., Kell L., Kell L., Merino G., Ibaibarriaga L., et al. (2015). Global habitat preferences of commercially valuable tuna. Deep Sea Res. 2 Top. Stud. Oceanogr. 113, 102–112. doi: 10.1016/j.dsr2.2014.07.001
Arrizabalaga H., Pereira J. G., Royer F., Galuardi B., Goni N., Artetxe I., et al. (2008). Bigeye tuna (Thunnus obesus) vertical movements in the Azores Islands determined with pop-up satellite archival tags. Fish. Oceanogr. 17, 74–83. doi: 10.1111/j.1365-2419.2008.00464.x
Bakun A. (2013). Ocean eddies, predator pits and Bluefin tuna: implications of inferred “low risk-limited payoff”. Reprod. scheme (former) archetypical Top. predator. Fish Fish. 14, 424–438. doi: 10.1111/faf.12002
Bangley C. W., Curtis T. H., Secor D. H., Latour R. J., Ogburn M. B. (2020). Identifying important juvenile dusky shark habitat in the Northwest Atlantic Ocean using acoustic telemetry and spatial modeling. Mar. Coast. Fish. 12, 348–363. doi: 10.1002/mcf2.10120
Barkely R. A., Neill W. H., Gooding R. M. (1978). Skipjack tuna, Katsuwonus pelamis, habitat based on temperature and oxygen requirements. Fish. Bull. 76, 653–662.
Baudron A. R., Needle C., Rijnsdorp A. D., Marshall C. T. (2014). Warming temperatures and smaller body sizes: synchronous changes in growth of North Sea fishes. Glob. Change Biol. 20, 1023–1031. doi: 10.1111/gcb.12514
Beaugrand G., Edwards M., Raybaud V., Goberville E., Kirby R. R. (2015). Future vulnerability of marine biodiversity compared with contemporary and past changes. Nat. Clim. Change 5, 695–701. doi: 10.1038/nclimate2650
Bell J. D., Senina I., Adams T., Aumont O., Calmettes B., Clark S., et al. (2021). Pathways to sustaining tuna-dependent Pacific Island economies during climate change. Nat. Sustain. 4, 900–910. doi: 10.1038/s41893-021-00745-z
Benoit-Bird K. J., Battaile B. C., Heppell S. A., Hoover B., Irons D., Jones N., et al. (2013). Prey patch patterns predict habitat use by top marine predators with diverse foraging strategies. PloS One 8, e53348. doi: 10.1371/journal.pone.0053348
Bernal D., Carlson J. K., Goldman K. J., Lowe C. G. (2012). “Energetics, metabolism, and endothermy in sharks and rays,” in Biology of sharks and their relatives, 2nd edn. Eds. Carrier J. C., Musick J. A., Heithaus M. R. (Boca Raton: CRC Press), 211–237.
Bernal D., Sepulveda C. A. (2005). Evidence for temperature elevation in the aerobic swimming musculature of the common thresher shark, Alopias vulpinus. Copeia 2005, 146–151. doi: 10.1643/CP-04-180R1
Bernal D., Sepulveda C., Musyl M., Brill R. (2009). “The eco-physiology of swimming and movement patterns of tunas, billfishes and large pelagic sharks,” in Fish locomotion — an etho-ecological approach. Eds. Domenici P., Kapoor B. G. (Enfield, NH, USA: Scientific Publishers), 437–483.
Biasutti M., Sobel A. H., Camargo S. J., Creyts T. T. (2012). Projected changes in the physical climate of the Gulf coast and caribbean. Clim. Change 112, 819–845. doi: 10.1007/s10584-011-0254-y
Bigelow K. A., Maunder M. N. (2007). Does habitat or depth influence the catch rates of pelagic fishes? Can. J. Fish. Aquat. Sci. 64, 1581–1594. doi: 10.1139/f07-115
Blanchard J. L., Jennings S., Holmes R., Harle J., Merino G., Allen J. I., et al. (2012). Potential consequences of climate change for primary production and fish production in large marine ecosystems. Philos. Trans. R. Soc Lond. B Biol. Sci. 367, 2979–2989. doi: 10.1098/rstb.2012.0231
Blank J. M., Morrissette J. M., Davie P. S., Block B. A. (2002). Effects of temperature, epinephrine and Ca2+ on the hearts of yellowfin tuna (Thunnus albacares). J. Exp. Biol. 205, 1881–1888. doi: 10.1242/jeb.205.13.1881
Block B. A., Booth D. T., Carey F. G. (1992). Depth and temperature of the blue marlin, Makaira nigricans, observed by acoustic telemetry. Mar. Biol. 114, 175–183. doi: 10.1007/BF00349517
Block B. A., Dewar H., Blackwell S. B., Williams T. D., Prince E. D., Farwell C. J., et al. (2001). Migratory movements, depth preferences, and thermal biology of Atlantic bluefin tuna. Science 293, 1310–1314. doi: 10.1126/science.1061197
Block B. A., Jonsen I. D., Jorgensen S. J., Winship A. J., Schaffer S. A., Bograd S. J., et al. (2011). Tracking apex marine predator movements in a dynamic ocean. Nature 475, 86–90. doi: 10.1038/nature10082
Block B. A., Stevens E. D. (2001). Tuna: Physiology, Ecology, and Evolution (San Diego: Academic Press).
Block B. A., Teo S. L. H., Walli A., Boustany A., Stokesbury M. (2005). Electronic tagging and population structure of Atlantic bluefin tuna. Nature 434, 1121–1127. doi: 10.1038/nature03463
Bonfil R., Meyer M., Scholl M. C., Johnson R., O’Brien S., Oosthuizen H., et al. (2005). Transoceanic migration, spatial dynamics, and population linkages of white sharks. Science 310, 100–103. doi: 10.1126/science.1114898
Börgel F., Frauen C., Neumann T., Meier H. E. M. (2020). The Atlantic Multidecadal Oscillation controls the impact of the North Atlantic Oscillation on North European climate. Environ. Res. Lett. 15, 104025. doi: 10.1088/1748-9326/aba925
Boyce D. G., Tittensor D. P., Worm B. (2008). Effects of temperature on global patterns of tuna and billfish richness. Mar. Ecol. Prog. Ser. 355, 267–276. doi: 10.3354/meps07237
Brander K. M. (2007). Global fish production and climate change. Proc. Natl. Acad. Sci. U.S.A. 10450, 19709–19714. doi: 10.1073/pnas.0702059104
Brickman D., Hebert D., Wang Z. (2018). Mechanism for the recent ocean warming events on the Scotian Shelf of eastern Canada. Cont. Shelf Res. 156, 11–22. doi: 10.1016/j.csr.2018.01.001
Brill R. W. (1994). A review of temperature and oxygen tolerance studies of tunas pertinent to fisheries oceanography, movement models and stock assessments. Fish. Oceanogr. 3, 204–216. doi: 10.1111/j.1365-2419.1994.tb00098.x
Brill R. W. (1996). Selective advantages conferred by the high performance physiology of tunas, billfish, and dolphinfish. Comp. Biochem. Physiol. A Mol. Integr. Physiol. 113, 3–15. doi: 10.1016/0300-9629(95)02064-0
Brill R. W., Bigelow K. A., Musyl M. K., Fritsches K. A., Warrant E. J. (2005). Bigeye tuna (Thunnus obesus) behavior and physiology and their relevance to stock assessments and fishery biology. ICCAT Collect. Vol. Sci. Pap. 57, 142–161.
Briscoe D. K., Hobday A. J., Carlisle A., Scales K., Eveson J. P., Arrizabalaga H., et al. (2017). Ecological bridges and barriers in pelagic ecosystems. Deep Sea Res. 2 Top. Stud. Oceanogr. 140, 182–192. doi: 10.1016/j.dsr2.2016.11.004
Britten G. L., Dowd M., Minto C., Ferretti F., Boero F., Lotze H. K. (2014). Predator decline leads to decreased stability in a coastal fish community. Ecol. Lett. 17, 1518–1525. doi: 10.1111/ele.12354
Bromhead D., Scholey V., Nicol S., Margulies D., Wexler J., Stein M., et al. (2015). The potential impact of ocean acidification upon eggs and larvae of yellowfin tuna (Thunnus albacares). Deep Sea Res. 2 Top. Stud. Oceanogr. 113, 268–279. doi: 10.1016/j.dsr2.2014.03.019
Brose U., Dunne J. A., Montoya J. M., Petchey O. L., Schneider F. D., Jacob U. (2012). Climate change in size-structured ecosystems. Philos. Trans. R Soc Lond. B Biol. Sci. 367, 2903–2912. doi: 10.1098/rstb.2012.0232
Bryan F. O., Hecht M. W., Smith R. D. (2007). Resolution convergence and sensitivity studies with North Atlantic circulation models. Part I: The western boundary current system. Ocean Modell. 16, 141–159. doi: 10.1016/j.ocemod.2006.08.005
Bryden H. L., Longworth H. R., Cunningham S. A. (2005). Slowing of the Atlantic meridional overturning circulation at 25° N. Nature 438, 655–657. doi: 10.1038/nature04385
Bushnell P. G., Brill R. W. (1991). Responses of swimming skipjack (Katsuwonus pelamis) and yellowfin tuna (Thunnus albacares) tunas to acute hypoxia, and a model of their cardio-respiratory function. Physiol. Zool. 64, 787–811. doi: 10.1086/physzool.64.3.30158207
Bushnell P. G., Brill R. W., Bourke R. E. (1990). Cardiorespiratory responses of skipjack tuna (Katsuwonurpelamis), yellowfin tuna (Thunnus albacores), and bigeye tuna (Thunnus obesus) to acute reductions of ambient oxygen. Can. J. Zool. 68, 1857–1865. doi: 10.1139/z90-265
Cai W.-J., Hu X., Huang W.-J., Murrell M. C., Lehrter J. C., Lohrenz S. E., et al. (2011). Acidification of subsurface coastal waters enhanced by eutrophication. Nat. Geosci. 4, 766–770. doi: 10.1038/ngeo1297
Campana S. E., Dorey A., Fowler M., Joyce W., Wang Z., Wright D., et al. (2011). Migration pathways, behavioural thermoregulation and overwintering grounds of blue sharks in the Northwest Atlantic. PloS One 6, e16854. doi: 10.1371/journal.pone.0016854
Campana S. E., Joyce W. N. (2004). Temperature and depth associations of porbeagle shark (Lamna nasus) in the northwest Atlantic. Fish. Oceanogr. 13, 52–64. doi: 10.1111/j.1365-2419.2004.00236.x
Campana S. E., Joyce W., Fowler M. (2010). Subtropical pupping ground for a cold-water shark. Can. J. Fish. Aquat. Sci. 67, 769–773. doi: 10.1139/F10-020
Campana S. E., Joyce W., Fowler M., Showell M. (2016). Discards, hooking, and post-release mortality of porbeagle (Lamna nasus), shortfin mako (Isurus oxyrinchus), and blue shark (Prionace glauca) in the Canadian pelagic longline fishery. ICES J. Mar. Sci. 73, 520–528. doi: 10.1093/icesjms/fsv234
Campana S. E., Joyce W., Marks L. (2003). Status of the Porbeagle Shark (Lamna nasus) Population in the Northwest Atlantic in the Context of Species at Risk. Can. Stock Assess. Secretariate Res. Document 2003/007.
Campana S. E., Joyce W., Marks L., Natanson L. J., Kohler N. E., Jensen C. F., et al. (2002). Population dynamics of the porbeagle in the Northwest Atlantic Ocean. N. Am. J. Fish. Manage. 22, 106–121. doi: 10.1577/1548-8675(2002)022<0106:PDOTPI>2.0.CO;2
Campana S. E., Marks L., Joyce W., Hurley P., Showell M., Kulka D. (1999). An analytical assessment of the porbeagle shark (Lamna nasus) population in the Northwest Atlantic. Can. Stock Assess. Secretariat Res. document 99/158, 57.
Cao D. M., Song L. M., Zhang Y., Lv K. K., Hu Z. X. (2011). Environmental preferences of Alopias superciliosus and Alopias vulpinus in waters near Marshall Islands. N. Z. J. Mar. Freshwater. Res. 45, 103–119. doi: 10.1080/00288330.2010.540017
Carey F. G., Casey J. G., Pratt H., Urquhart D., McCosker J. E. (1985). Temperature, heat production, and heat exchange in lamnid sharks. Mem. South Calif. Acad. Sci. 9, 92–108.
Carey F. G., Scharold J. V. (1990). Movements of blue sharks Prionace glauca in depth and course. Mar. Biol. 106, 329–342. doi: 10.1007/BF01344309
Carlson J. K., Goldman K. J., Lowe C. (2004). “Metabolism, energetic demand, and endothermy,” in The biology of sharks and their relatives. Eds. Carrier J., Musick J., Heithaus M. (Boca Raton: CRC Press), 203–224.
Carlson J. K., Gulak S. J. B. (2012). Habitat use and movements patterns of oceanic whitetip, bigeye thresher and dusky sharks based on archival satellite tags. ICCAT Collect. Vol. Sci. Pap. 68, 1922–1932.
Carlson J. K., Parson G. R. (2001). The effects of hypoxia on three sympatric shark species: physiological and behavioral responses. Environ. Biol. Fishes 61, 427–433. doi: 10.1023/A:1011641302048
Cartamil D. P., Sepulveda C. A., Wegner N. C., Aalbers S. A., Baquero A., Graham J. B. (2011). Archival tagging of subadult and adult common thresher sharks (Alopias vulpinus) off the coast of southern California. Mar. Biol. 158, 935–944. doi: 10.1007/s00227-010-1620-4
Cartamil D., Wegner N. C., Aalbers S., Sepulveda C. A., Baquero A., Graham J. B. (2010). Diel movement patterns and habitat preferences of the common thresher shark (Alopias vulpinus) in the Southern California Bight. Mar. Freshw. Res. 61, 596–604. doi: 10.1071/MF09153
Casey J. G., Kohler N. E. (1992). Tagging studies on the shortfin mako shark (Isurus oxyrinchus) in the western North Atlantic. Aust. J. Mar. Freshw. Res. 43, 45–60. doi: 10.1071/MF9920045
Chang Y.-L., Sun C.-L., Chen Y., Yeh S.-Z., DiNardo G., Su1 N.-J. (2013). Modelling the impacts of environmental variation on the habitat suitability of swordfish, Xiphias gladius, in the equatorial Atlantic Ocean. ICES J. Mar. Sci. 70, 1000–1012. doi: 10.1093/icesjms/fss190
Chen I. C., Hill J. K., Ohlemüller R., Roy D. B., Thomas C. D. (2011). Rapid range shifts of species associated with high levels of climate warming. Science 333, 1024–1026. doi: 10.1126/science.1206432
Cheung W. W. L., Brodeur R. D., Okey T. A., Pauly D. (2015). Projecting future changes in distributions of pelagic fish species of Northeast Pacific shelf seas. Prog. Oceanogr. 130, 19–31. doi: 10.1016/j.pocean.2014.09.003
Chin A., Kyne P. M., Walker T. I., McAuley R. B. (2010). An integrated risk assessment for climate change: analyzing the vulnerability of sharks and rays on Australia’s Great Barrier Reef. Glob. Change Biol. 16, 1936–1953. doi: 10.1111/j.1365-2486.2009.02128.x
Claret M., Galbraith E. D., Palter J. B., Bianchi D., Fennel K., Gilbert D., et al. (2018). Rapid coastal deoxygenation due to ocean circulation shift in the northwest Atlantic. Nat. Clim. Change 8, 868–872. doi: 10.1038/s41558-018-0263-1
Clark T. D., Raby G. D., Roche D. G., Binning S. A., Speers-Roesch B., Jutfelt F., et al. (2020). Ocean acidification does not impair the behavior of coral reef fishes. Nature 577, 370–375. doi: 10.1038/s41586-019-1903-y
Clarke S., Harley S. J., Hoyle S. D., Rice J. S. (2012). Population trends in Pacific oceanic sharks and the utility of regulations on shark finning. Conserv. Biol. 27, 197–209. doi: 10.1111/j.1523-1739.2012.01943.x
Coffey D., Holland K. (2015). First autonomous recording of in situ dissolved oxygen from free-ranging fish. Anim. Biotelemetry 3, e47. doi: 10.1186/s40317-015-0088-x
Collette B. B., Carpenter K. E., Polidoro B. A., Juan-Jorda M. J., Boustany A., Die D. J., et al. (2011). High value and long life-Double jeopardy for tunas and billfishes. Science 333, 291–292. doi: 10.1126/science.1208730
Compagno L. J. V. (1984). Sharks of the World. An annotated and illustrated catalogue of shark species known to date. Part II (Carcharhiniformes) Vol. 125 (Rome: FAO Fish. Synop).
Cooke S. J., Suski C. D. (2004). Are circle hooks an effective tool for conserving marine and freshwater recreational catch-and-release fisheries? Aquat. Conserv. 14, 299–326. doi: 10.1002/aqc.614
Cornic M., Rooker J. R. (2021). Temporal shifts in the abundance and preferred habitats of yellowfin and bigeye tuna larvae in the Gulf of Mexico. J. Mar. Syst. 217, 103524. doi: 10.1016/j.jmarsys.2021.103524
Cortés E., Domingo A., Miller P., Forselledo R., Mas F., Arocha F., et al. (2015). Expanded ecological risk assessment of pelagic sharks caught in Atlantic pelagic longline fisheries. ICCAT Collect. Vol. Sci. Pap. 71, 2637–2688.
Cramer J. (1996). Recent trends in the catch of undersized swordfish by the US pelagic longline fishery. Mar. Fish. Rev. 58, 24–32.
Cramer J. (2001). Geographic distribution of longline effort and swordfish discard rates in the Straits of Florida and oceanic waters of the continental shelf, slope, and Blake Plateau off Georgia and the Carolinas from 1991 to 1995”, in Island in the stream: the ecology and fisheries of the Charleston Bump. Am. Fish. Soc Symp, 97–103.
Crear D. P., Brill R. W., Bushnell P. G., Latour R., Schwieterman G. D., Steffen R. M., et al. (2019). The impacts of warming and hypoxia on the performance of an obligate ram ventilator. Conserv. Physiol. 7, coz026. doi: 10.1093/conphys/coz026
Crear D. P., Latour R. J., Friedrichs M. A. M., St-Laurent P., Weng K. C. (2020). Sensitivity of a shark nursery habitat to a changing climate. Mar. Ecol. Prog. Ser. 652, 123–136. doi: 10.3354/meps13483
Crespo G. O., Dunn D. C., Reygondeau G., Boerder K., Worm B., Cheung W., et al. (2018). The environmental niche of the global high seas pelagic longline fleet. Sci. Adv. 4, eaat3681. doi: 10.1126/sciadv.aat3681
Curtis T. H., McCandless C. T., Carlson J. K., Skomal G., Kohler N. E., Natanson L. J., et al. (2014). Seasonal distribution and historic trends in abundance of white sharks, Carcharodon carcharias, in the western North Atlantic ocean. PloS One 9, e99240. doi: 10.1371/journal.pone.0099240
Czaja A., Frankignoul C., Minobe S., Vannière B. (2019). Simulating the midlatitude atmospheric circulation: What might we gain from high-resolution modeling of air-sea interactions? Curr. Clim. Change Rep. 5, 390–406. doi: 10.1007/s40641-019-00148-5
Dagorn L., Bach P., Josse E. (2000). Movement patterns of large bigeye tuna (Thunnus obesus) in the open ocean, determined using ultrasonic telemetry. Mar. Biol. 136, 361–371. doi: 10.1007/s002270050694
Dapp D. R., Walker T. I., Huveneers C., Reina R. D. (2016). Respiratory mode and gear type are important determinants of elasmobranch immediate and post-release mortality. Fish Fish. 17, 507–524. doi: 10.1111/faf.12124
Davis M. W. (2002). Key principles for understanding fish bycatch discard mortality. Can. J. Fish. Aquat. Sci. 59, 1834–1843. doi: 10.1139/f02-139
Dell’Apa A., Carney K., Davenport T. M., Vernon Carle M. (2018). Potential medium-term impacts of climate change on tuna and billfish in the Gulf of Mexico: A qualitative framework for management and conservation. Mar. Environ. Res. 141, 1–11. doi: 10.1016/j.marenvres.2018.07.017
Dell’Apa A., Knight E., Overton A., Landry C., Dumas C., Whitehead J. C., et al. (2015). The North Carolina charter boat fishery changing with the times: A comparative analysis of the catch compositio and 2007–2008). Fisheries 40, 222–233. doi: 10.1080/03632415.2015.1026332
Delworth T. L., Mann M. E. (2000). Observed and simulated multidecadal variability in the Northern Hemisphere. Clim. Dyn. 16, 661–676. doi: 10.1007/s003820000075
Dewar H., Prince E. D., Musyl M. K., Brill R. W., Sepulveda C., Luo J., et al. (2011). Movements and behaviors of swordfish in the Atlantic and Pacific oceans examined using pop-up satellite archival tags. Fish. Oceanogr. 2, 219–241. doi: 10.1111/j.1365-2419.2011.00581.x
Diaz G. A. (2008). The effect of circle hooks and straight (J) hooks on the catch rates and numbers of white marlin and blue marlin released alive by the U.S. pelagic longline fleet in the Gulf of Mexico. N. Am. J. Fish. Manage. 28, 500–506. doi: 10.1577/M07-089.1
Dickson K. A., Graham J. B. (2004). Evolution and consequences of endothermy in fishes. Physiol. Biochem. Zool. 77, 998–1018. doi: 10.1086/423743
Dietrich D. E., Lin C. A. (1994). Numerical studies of eddy shedding in the Gulf of Mexico. J. Geophys. Res. Oceans 99, 7599–7615. doi: 10.1029/93JC03572
Dixson D. L., Jennings A. R., Atema J., Munday P. L. (2015). Odor tracking in sharks is reduced under future ocean acidification conditions. Glob. Change Biol. 21, 1454–1462. doi: 10.1111/gcb.12678
Dixson D. L., Munday P. L., Jones G. P. (2010). Ocean acidification disrupts the innate ability of fish to detect predator olfactory cues. Ecol. Lett. 13, 68–75. doi: 10.1111/j.1461-0248.2009.01400.x
Domeier M. L., Dewar H., Nasby-Lucas N. (2003). Mortality rate of striped marlin (Tetrapturus audax) caught with recreational tackle. Mar. Freshw. Res. 54, 435–445. doi: 10.1071/MF01270
Domingues R., Goni G., Bringas F., Muhling B., Lindo-Atichati D., Walter J. (2016). Variability of preferred environmental conditions for Atlantic bluefin tuna (Thunnus thynnus) larvae in the Gulf of Mexico during 1993-2011. Fish. Oceanogr. 25, 320–336. doi: 10.1111/fog.12152
Doney S. C., Ruckelshaus M., Emmett Duffy J., Barry J. P., Chan F., English C. A., et al. (2012). Climate change impacts on marine ecosystems. Ann. Rev. Mar. Sci. 4, 11–37. doi: 10.1146/annurev-marine-041911-111611
Dragon A. C., Senina I., Titaud O., Calmettes B., Conchon A., Arrizabalaga H., et al. (2015). An ecosystem-driven model for spatial dynamics and stock assessment of North Atlantic albacore. Can. J. Fish. Aquat. Sci. 72, 864–878. doi: 10.1139/cjfas-2014-0338
Drijfhout S. S., Hazeleger W. (2006). Changes in MOC and gyre induced Atlantic Ocean heat transport. Geophys. Res. Lett. 33, L07707. doi: 10.1029/2006GL025807
Druon J.-N., Fromentin J.-M., Hanke A. R., Arrizabalaga H., Damalas D., Ticina V., et al. (2016). Habitat suitability of the Atlantic bluefin tuna by size class: An ecological niche approach. Prog. Oceanogr. 142, 30–46. doi: 10.1016/j.pocean.2016.01.002
Dueri S., Bopp L., Maury O. (2014). Projecting the impacts of climate change on skipjack tuna abundance and spatial distribution. Glob. Change Biol. 20, 742–753. doi: 10.1111/gcb.12460
Dufour F., Arrizabalaga H., Irigoien X., Santiago J. (2010). Climate impacts on albacore and bluefin tunas migrations phenology and spatial distribution. Prog. Oceanogr. 86, 283–290. doi: 10.1016/j.pocean.2010.04.007
Du Preez H. H., Mclachlan A., Marais J. F. K. (1988). Oxygen consumption of two nearshore marine elasmobranchs, Rhino batos annulatus (Muller & Henl) and myliobatus aquila (Linnaeu). Comp. Biochem. Physiol. A Physiol. 89, 283–294. doi: 10.1016/0300-9629(88)91094-8
Economakis A., Lobel P. (1998). Aggregation behavior of the grey reef shark, Carcharhinus amblyrhynchos, at Johnston Atoll, Central Pacific Ocean. Environ. Biol. Fishes 51, 129–139. doi: 10.1023/A:1007416813214
Ellis J., McCully P., Poisson F. (2017). A review of capture and post-release mortality of elasmobranchs. J. Fish Biol. 90, 653–722. doi: 10.1111/jfb.13197
Erauskin-Extramiana M., Arrizabalaga H., Cabré A., Coelho R., Rosa D., Ibaibarriaga L., et al. (2020). Are shifts in species distribution triggered by climate change? A swordfish case study. Deep Sea Res. 2 Top. Stud. Oceanogr. 175, 104666. doi: 10.1016/j.dsr2.2019.104666
Erauskin-Extramiana M., Arrizabalaga H., Hobday A. J., Cabré A., Ibaibarriaga L., Arregui I., et al. (2019). Large-scale distribution of tuna species in a warming ocean. Glob. Change Biol. 25, 2043–2060. doi: 10.1111/gcb.14630
Failletaz R., Beaugrandl G., Goberville E., Kirby R. R. (2019). Atlantic Multidecadal Oscillations drive the basin-scale distribution of Atlantic bluefin tuna. Sci. Adv. 5, eaar6993. doi: 10.1126/sciadv.aar6993
Ficke A. D., Myrick C. A., Hansen L. J. (2007). Potential impacts of global climate change on freshwater fisheries. Rev. Fish. Biol. Fish. 17, 581–613. doi: 10.1007/s11160-007-9059-5
Field I. C., Meekan M. G., Buckworth R. C., Bradshaw C. J. A. (2009). Susceptibility of sharks, rays and chimaeras to global extinction. Adv. Mar. Biol. 56, 275–363. doi: 10.1016/S0065-2881(09)56004-X
Francis M. P., Natanson L. J., Campana S. E. (2008). ““ The biology and ecology of the porbeagle shark, Lamna nasus,” in Sharks of the open ocean: biology, fisheries and conservation. Eds. Camhi M. D., Pikitch E. K., Babcock E. A. (Oxford: Blackwell Publishing), 105–113.
Free C. M., Thorson J. T., Pinsky M. L., Oken K. L., Wiedenmann J., Jensen O. P. (2019). Impacts of historical warming on marine fisheries production. Science 363, 979–983. doi: 10.1126/science.aau1758
Friedland K. D., Stock C., Drinkwater K. F., Link J. S., Leaf R. T., Shank B. V., et al. (2012). Pathways between primary production and fisheries yields of large marine ecosystems. PloS One 10, e0133794. doi: 10.1371/journal.pone.0028945
Fritsches K. A., Brill R. W., Warrant E. J. (2005). Warm eyes provide superior vision in swordfishes. Curr. Biol. 15, 55–58. doi: 10.1016/j.cub.2004.12.064
Froeschke J., Stunz G. W., Wildhaber M. L. (2010). Environmental influences on the occurrence of coastal sharks in estuarine waters. Mar. Ecol. Progr. Ser. 407, 279–292. doi: 10.3354/meps08546
Fromentin J.-M., Fonteneau A. (2001). Fishing effects and life history traits: a case study comparing tropical versus temperate tunas. Fish. Res. 53, 133–150. doi: 10.1016/S0165-7836(00)00299-X
Fuentes M. M. P. B., Chambers L., Chin A., Dann P., Dobbs K., Marsh H., et al. (2016). Adaptive management of marine mega-fauna in a changing climate. Mitig. Adapt. Strateg. Glob. Change 21, 209–224. doi: 10.1007/s11027-014-9590-3
Gallagher A. J., Hammerschlag N., Shiffman D. S., Giery S. T. (2014b). Evolved for extinction: The cost and conservation implications of specialization in hammerhead sharks. BioScience 64, 619–624. doi: 10.1093/biosci/biu071
Gallagher A. J., Orbesen E. S., Hammerschlag N., Serafy J. E. (2014a). Vulnerability of oceanic sharks as pelagic longline bycatch. Glob. Ecol. Conserv. 1, 50–59. doi: 10.1016/j.gecco.2014.06.003
Gallagher A. J., Serafy J. E., Cooke S. J., Hammerschlag N. (2014c). Physiological stress response, reflex impairment, and survival of five sympatric shark species following experimental capture and release. Mar. Ecol. Progr. Ser. 496, 207–218. doi: 10.3354/meps10490
Galli G., Shiels H., Brill R. (2009). Cardiac temperature sensitivity in yellowfin tuna (Thunnus albacares), bigeye tuna (T. obesus), mahimahi (Coryphaena hippurus) and swordfish (Xiphias gladius). Physiol. Biochem. Zool. 82, 280–290. doi: 10.1086/597484
Gawarkiewicz G., Todd R. E., Zhang W., Partida J., Gangopadhyay A., Monim M.-U.-H., et al. (2018). The changing nature of shelf-break exchange revealed by the OOI Pioneer Array. Oceanogr 31, 60–70. doi: 10.5670/oceanog.2018.110
Golet W. J., Galuardi B., Cooper A. B., Lutcavage M. E. (2013). Changes in the distribution of Atlantic Bluefin Tuna (Thunnus thynnus) in the Gulf of Maine 1979-2005. PloS One 8, e75480. doi: 10.1371/journal.pone.0075480
Goñi N., Arregui I., Lezama A., Arrizabalaga H., Moreno G. (2009). “Small scale vertical behavior of juvenile albacore in relation to their biotic environment in the Bay of Biscay,” in Tagging and tracking of marine animals with electronic devices. Eds. Nielsen J., Arrizabalaga H., Fragoso N., Hobday A., Lutcavage M., Sibert J. (Netherlands: Springer), 51–73.
Govoni J. J., Hare J. A. (2001). The Charleston Gyre as spawning and larval nursery habitat for fishes”, in Island in the stream: the ecology and fisheries of the Charleston Bump. Am. Fish. Soc Symp, 123–136.
Govoni J. J., Laban E. H., Hare J. A. (2003). The early life history of swordfish (Xiphias gladius) in the western North Atlantic. Fish. Bull. 101, 778–789.
Graham J. B., Dewar H., Lai N. C., Lowell W. R., Arce S. M. (1990). Aspects of shark swimming performance determined using a large water tunnel. J. Exp. Biol. 151, 175–192. doi: 10.1242/jeb.151.1.175
Graham J. B., Dickson K. A. (2004). Tuna comparative physiology. J. Exp. Biol. 207, 4015–4024. doi: 10.1242/jeb.01267
Graham J. B., Lowell R. W., Lai N. C., Laurs R. M. (1989). O2 tension, swimming velocity, and thermal effects on the metabolic rate of the Pacific albacore, Thunnus alalunga. Exp. Biol. 48, 89–94.
Green R. E. (1967). Relationship of the thermocline to success of pursue seining for tuna. Trans. Am. Fish. Soc 96, 126–130. doi: 10.1577/1548-8659(1967)96[126:ROTTTS]2.0.CO;2
Grose S. O., Pendleton L., Leathers A., Cornish A., Waitai S. (2020). Climate change will re-draw the map for marine megafauna and the people who depend on them. Front. Mar. Sci. 7, 547. doi: 10.3389/fmars.2020.00547
Gutowsky L. F. G., James Rider M., Roemer R. P., Gallagher A. J., Heithaus M. R., Cooke S. J., et al. (2021). Large sharks exhibit varying behavioral responses to major hurricanes. Estuar. Coast. Shelf Sci. 256, 107373. doi: 10.1016/j.ecss.2021.107373
Hammerschlag N., McDonnell L. H., Rider M. J., Street G., Hazen E., Natanson L. J., et al. (2022). Ocean warming alters the distributional range, migratory timing, and spatial protections of an apex predator, the tiger shark (Galeocerdo cuvier). Glob. Change Biol. 28, 1990–2005. doi: 10.1111/gcb.16045
Hansell A., Walter J., Cadrin S., Golet W., Hanke A., Lauretta M., et al. (2020). Incorporating the Atlantic Mutidecadal Oscillation into the Western Atlantic bluefin tuna stock assessment. ICCAT Collect. Vol. Sci. Pap. 77, 376–388.
Hare J. A., Morrison W. E., Nelson M. W., Stachura M., Teeters E. J., Griffis R. B., et al. (2016). A vulnerability assessment of fish and invertebrates to climate change on the Northeast U.S. Continental Shelf. PloS One 11, e0146756. doi: 10.1371/journal.pone.0146756
Hazen E. L., Jorgensen S., Rykaczewski R. R., Bograd S. J., Foley D. G., Jonsen I. D., et al. (2013). Predicted habitat shifts of Pacific top predators in a changing climate. Nat. Clim. Change 3, 234–238. doi: 10.1038/nclimate1686
Hazen E. L., Scales K. L., Maxwell S. M., Briscoe D. K., Welch H., Bograd S. J., et al. (2018). A dynamic ocean management tool to reduce bycatch and support sustainable fisheries. Sci. Adv. 4, eaar3001. doi: 10.1126/sciadv.aar3001
Heupel M. R., Carlson J. K., Simpfendorfer C. A. (2007). Shark nursery areas: concepts, definition, characterization and assumptions. Mar. Ecol. Progr. Ser. 337, 287–297. doi: 10.3354/meps337287
Heupel M. R., Simpfendorfer C. A., Hueter R. E. (2003). Running before the storm: blacktip sharks respond to falling barometric pressure associated with Tropical Storm Gabrielle. J. Fish Biol. 63, 1357–1363. doi: 10.1046/j.1095-8649.2003.00250.x
Hillborn R., Walters C. J. (1992). Quantitative fisheries stock assessment: choice, dynamics and uncertainty (New York: Chapman and Hall).
Hobday A., Arrizabalaga H., Evans K., Nicol S., Young J., Weng K. (2015). Impacts of climate change on marine top predators: advances and future challenges. Deep Sea Res. 2 Top. Stud. Oceanogr. 113, 1–8. doi: 10.1016/j.dsr2.2015.01.013
Hobday A. J., Young J. W., Abe O., Costa D. P., Cowen R. K., Evans K., et al. (2013). Climate impacts and oceanic top predators: moving from impacts to adaptation in oceanic systems. Rev. Fish. Biol. Fish. 23, 537–546. doi: 10.1007/s11160-013-9311-0
Hoegh-Guldberg O., Jacob D., Taylor M., Bindi M., Brown S., Camilloni I., et al. (2018). “Impacts of 1.5°C global warming on natural and human systems,” in Global Warming of 1.5°C An IPCC special report on the impacts of global warming of 1.5°C above pre-industrial levels and related global greenhouse gas emission pathways, in the context of strengthening the global response to the threat of climate change. Eds. Masson-Delmotte P. Z. V., Zhai P., Pörtner H. O., Roberts D., Skea J., Shukla P. R., et al (Geneva: IPCC), 175–311.
Hoey J. J. (1983). Analysis of longline fishing effort for apex predators (swordfish, shark, and tuna) in the western North Atlantic and Gulf of Mexico (University of Rhode Island: Dissertation).
Hoffmayer E. R., Franks J. S., Driggers W. B., Howey P. W. (2013). Diel vertical movements of a scalloped hammerhead, Sphyrna lewini, in the northern Gulf of Mexico. Bull. Mar. Sci. 89, 551–557. doi: 10.5343/bms.2012.1048
Hoffmayer E. R., Franks J. S., Driggers W. B. III, McKinney J. A., Hendon J. M., Quattro J. M. (2014). Habitat, movements and environmental preferences of dusky sharks, Carcharhinus obscurus, in the northern Gulf of Mexico. Mar. Biol. 161, 911–924. doi: 10.1007/s00227-014-2391-0
Holland K. N., Brill R. W., Chang R. K. C., Sibert J. R., Fournier D. A. (1992). Physiological and behavioral thermoregulation in bigeye tuna (Thunnus obesus). Nature 358, 410–412. doi: 10.1038/358410a0
Hoolihan J. P., Luo J., Richardson D. E., Snodgrass D., Orbesen E. S., Prince E. D. (2009). Vertical movement rate estimates for Atlantic istiophorid billfishes derived from high-resolution pop-up satellite archival data. Bull. Mar. Sci. 84, 257–264.
Horodysky A. Z., Graves J. E. (2005). Application of pop-up satellite archival tag technology to estimate postrelease survival of white marlin (Tetrapturus albidus) caught on circle and straight-shank (“J”) hooks in the western North Atlantic recreational fishery. Fish. Bull. 103, 84–96.
Howey-Jordan L. A., Brooks E. J., Abercrombie D. L., Jordan L. K. B., Brooks A., Williams S., et al. (2013). Complex movements, philopatry and expanded depth range of a severely threatened pelagic shark, the oceanic whitetip (Carcharhinus longimanus) in the Western North Atlantic. PloS One 8, e56588. doi: 10.1371/journal.pone.0056588
Hoyle S. D., Williams A. J., Minte-Vera C. V., Maunder M. N. (2023). Approaches for estimating natural mortality in tuna stock assessments: Application to global yellowfin tuna stocks. Fish. Res. 257, 106498. doi: 10.1016/j.fishres.2022.106498
Huang H.-W., Swimmer Y., Bigelow K., Gutierrez A., Foster D. G. (2016). Influence of hook type on catch of commercial and bycatch species in an Atlantic tuna fishery. Mar. Policy 65, 68–75. doi: 10.1016/j.marpol.2015.12.016
Humston R., Ault J. S., Lutcavage M., Olson D. B. (2000). Schooling and migration of large pelagic fishes relative to environmental cues. Fish. Oceanogr. 9, 136–146. doi: 10.1046/j.1365-2419.2000.00132.x
Hunsicker M. E., Olson R. J., Essington T. E., Maunder M. N., Duffy L. M., Kitchell J. F. (2012). Potential for top-down control on tropical tunas based on size structure of predator-prey interactions. Mar. Ecol. Progr. Ser. 445, 263–277. doi: 10.3354/meps09494
Huveneers C., Apps K., Becerril-García E. E., Bruce B., Butcher P. A., Carlisle A. B., et al. (2018). Future research directions on the “elusive”. white shark. Front. Mar. Sci. 5, 455. doi: 10.3389/fmars.2018.00455
Idrisi N., Capo T. R., Luthy S., Serafy J. E. (2003). Behavior, oxygen consumption and survival of stressed juvenile sailfish (Istiophorus platypterus) in captivity. Mar. Freshw. Behav. Physiol. 36, 51–57. doi: 10.1080/1023624021000054299
IPCC. (2012). “Glossary of terms,” in Managing the risks of extreme events and disasters to advance climate change adaptation. Eds. Field C.B., Barros V., Stocker T. F., Qin D., Dokken D. J., Ebi K. L., et al. (Cambridge: Cambridge University Press), 555–564.
Jansen T., Post S., Kristiansen T., Oskarsson G. J., Boje J., MacKenzie B. R., et al. (2016). Ocean warming expands habitat of a rich natural resource and benefits a national economy. Ecol. Appl. 26, 2021–2032. doi: 10.1002/eap.1384
Jorgensen S. J., Klimley A. P., Muhlia-Melo A. F. (2009). Scalloped hammerhead shark Sphyrna lewini, utilizes deep-water, hypoxic zone in the Gulf of California. J. Fish Biol. 74, 1682–1687. doi: 10.1111/j.1095-8649.2009.02230.x
Karnasuskas M., Schirripa M. J., Craig J. K., Cook G. S., Kelble C. R., Agar J. J., et al. (2015). Evidence of climate-driven ecosystem reorganization in the Gulf of Mexico. Glob. Change Biol. 21, 2554–2568. doi: 10.1111/gcb.12894
Karnauskas M., Schirripa M. J., Kelble C. R., Cook G. S., Craig J. K. (2013). Ecosystem status report for the Gulf of Mexico. NMFS-SEFSC-653, 52.
Keeling R. F., Körtzinger A., Gruber N. (2010). Ocean deoxygenation in a warming world. Ann. Rev. Mar. Sci. 2, 199–229. doi: 10.1146/annurev.marine.010908.163855
Kenchington. T. J. (2014). Natural mortality estimators for information-limited fisheries. Fish Fish. 15, 533–562. doi: 10.1111/faf.12027
Keyl F., Wolff M. (2008). Environmental variability and fisheries: what can models do. Rev. Fish. Biol. Fish. 18, 273–299. doi: 10.1007/s11160-007-9075-5
Kilfoil J. P., Wetherbee B. M., Carlson J. K., Fox D. A. (2017). Targeted catch-and-release of prohibited sharks: sand tigers in coastal Delaware waters. Fisheries 42, 281–287. doi: 10.1080/03632415.2017.1306974
Kinney M. J., Kacev D., Sippel T., Dewar H., Eguchi T. (2020). Common thresher shark Alopias vulpinus movement: Bayesian inference on a data-limited species. Mar. Ecol. Progr. Ser. 639, 155–167. doi: 10.3354/meps13271
Kleisner K. M., Fogarty M. J., McGee S., Hare J. A., Moret S., Perretti C. T., et al. (2017). Marine species distribution shifts on the U.S. Northeast Continental Shelf under continued ocean warming. Prog. Oceanogr. 153, 24–36.
Klimley A. P. (1993). Highly directional swimming by scalloped hammerhead sharks, Sphyrna lewini, and subsurface irradiance, temperature, bathymetry, and geomagnetic field. Mar. Biol. 117, 1–22. doi: 10.1007/BF00346421
Knapp J. M., Aranda G., Medina A., Lutcavage M. (2014). Comparative assessment of the reproductive status of female Atlantic bluefin tuna from the Gulf of Mexico and the Mediterranean Sea. PloS One 9, e98233. doi: 10.1371/journal.pone.0098233
Kneebone J., Bowlby H., Mello J. J., McCandless C. T., Natanson L. J., Gervelis B., et al. (2020). Seasonal distribution and habitat use of the common thresher shark (Alopias vulpinus) in the western North Atlantic Ocean inferred from fishery-dependent data. Fish. Bull. 118, 399–412. doi: 10.7755/FB.118.4.8
Kneebone J., Chisholm J., Bernal D., Skomal G. (2013). The physiological effects of capture stress, recovery, and post-release survivorship of juvenile sand tigers (Carcharias taurus) caught on rod and reel. Fish. Res. 147, 103–114. doi: 10.1016/j.fishres.2013.04.009
Knight J. R., Folland C. K., Scaife A. A. (2006). Climate impacts of the atlantic multidecadal oscillation. Geophys. Res. Lett. 33, L17706. doi: 10.1029/2006GL026242
Knutson T. R., McBride J., Chan J., Emanuel K., Holland G., Landsea C., et al. (2010). Tropical cyclones and climate change. Nat. Geosci. 3, 157–163. doi: 10.1038/ngeo779
Kraus R. T., Rooker J. R. (2007). Patterns of vertical habitat use by Atlantic blue marlin (Makaira nigricans) in the Gulf of Mexico. Gulf Caribb. Res. 19, 89–97. doi: 10.18785/gcr.1902.11
Kushnir Y. (1994). Interdecadal variations in North-Atlantic sea-surface temperature and associated atmospheric conditions. J. Clim. 7, 141–157. doi: 10.1175/1520-0442(1994)007<0141:IVINAS>2.0.CO;2
Kwiatkowski L., Torres O., Bopp L., Aumont O., Chamberlain M., Christian J. R., et al. (2020). Twenty-first century ocean warming, acidification, deoxygenation, and upper ocean nutrient decline from CMIP6 model projections. Biogeosciences 17, 3439–3470. doi: 10.5194/bg-17-3439-2020
Laffoley D., Baxter J. M. (editors). (2016). Explaining Ocean Warming: Causes, Scale, Effects and Consequences. (Gland, Switzerland: IUCN), 456 pp. doi: 10.2305/IUCN.CH.2016.08.en
Lam C. H., Galuardi B., Lutcavage M. E. (2014). Movements and oceanographic associations of bigeye tuna (Thunnus obesus) in the Northwest Atlantic. Can. J. Fish. Aquat. Sci. 71, 1–15. doi: 10.1139/cjfas-2013-0511
Lehodey P. (2001). The pelagic ecosystem of the tropical Pacific Ocean: dynamic spatial modelling and biological consequences of ENSO. Prog. Oceanogr. 49, 439–468. doi: 10.1016/S0079-6611(01)00035-0
Lehodey P., Bertignac M., Hampton J., Lewis A., Picaut J. (1997). El Niño Southern Oscillation and tuna in the western Pacific. Nature 389, 715–717. doi: 10.1038/39575
Lehodey P., Hampton J., Brill R. W., Nicol S., Senina I., Calmettes B., et al. (2011a). “Vulnerability of oceanic fisheries in the tropical Pacific to climate change,” in Vulnerability of tropical pacific fisheries and aquaculture to climate change. Eds. Bell J., Johnson J. E., Hobday A. J. (Noumea: Secretariat of the Pacific Community), 447–506.
Lehodey P., Senina I., Calmettes B., Hampton J., Nicol S. (2013). Modelling the impact of climate change on Pacific skipjack tuna population and fisheries. Clim. Change 119, 95–109. doi: 10.1007/s10584-012-0595-1
Lehodey P., Senina I., Calmettes B., Hampton J., Nicol S., Williams P., et al. (2011b). “SEAPODYM working progress and applications to Pacific skipjack tuna population and fisheries,” in 7th regular session of the Scientific Steering Committee (Pohnpei: Federate States of Micronesia), WCPFC-SC7-2011/EB- WP 06), 8–17.
Lehodey P., Senina I., Murtugudde R. (2008). A spatial ecosystem and population dynamics model (SEAPODYM) – modelling of tuna and tuna-like populations. Prog. Oceanogr. 78, 304–318. doi: 10.1016/j.pocean.2008.06.004
Lehodey P., Senina I., Nicol S., Hampton J. (2015). Modelling the impact of climate change on South Pacific albacore tuna. Deep Sea Res. 2 Top. Stud. Oceanogr. 113, 246–259. doi: 10.1016/j.dsr2.2014.10.028
Lehrter J. C., Ko D. S., Lowe L. L., Penta B. (2017). “Predicted effects of climate change on northern Gulf of Mexico hypoxia,” in Modeling coastal hypoxia. Eds. Justic D., Rose K., Hetland R., Fennel K. (Cham: Springer), 173–214.
Leung S., Mislan K. A. S., Muhling B., Brill R. (2019). “The significance of ocean deoxygenation for open ocean tunas and billfishes,” in Ocean deoxygenation – everyone’s problem: causes, impacts, consequences and solutions. Eds. Laffoley D., Baxter J. M. (Gland, Switzerland: IUCN), 277–308.
Levangie P. E. L., Blanchfield P. J., Hutchings J. A. (2021). The influence of ocean warming on the natural mortality of marine fishes. Environ. Biol. Fish. 105, 1447–1461. doi: 10.1007/s10641-021-01161-0
Li Y., Frantantoni P. S., Chen C., Hare J. A., Sun Y., Beardsley R. C., et al. (2015). Spatio-temporal patterns of stratification on the Northwest Atlantic shelf. Prog. Oceanogr. 134, 123–137. doi: 10.1016/j.pocean.2015.01.003
Lindo-Atichati D., Bringas F., Goni G., Muhling B., Muller-Karger F. E., Habtes S. (2012). Varying mesoscale structures influence larval fish distribution in the northern Gulf of Mexico. Mar. Ecol. Progr. Ser. 463, 245–257. doi: 10.3354/meps09860
Link J. S. (2010). Ecosystem-based fisheries management: confronting tradeoffs (Cambridge: Cambridge University Press).
Liu W., Fedorov A. V., Xie S.-P., Hu S. (2020). Climate impacts of a weakened Atlantic Meridional Overturning Circulation in a warming climate. Sci. Adv. 6, eaaz4876. doi: 10.1126/sciadv.aaz4876
Liu Y., Lee S.-K., Enfield D. B., Muhling B. A., Lamkin J. T., Muller-Karger F. E., et al. (2015). Potential impact of climate change on the Intra-Americas Sea: Part-1. A dynamic downscaling of the CMIP5 model projections. J. Mar. Syst. 148, 56–69. doi: 10.1016/j.jmarsys.2015.01.007
Liu Y., Lee S.-K., Muhling B. A., Lamkin J. T., Enfield D. B. (2012). Significant reduction of the Loop current in the 21st century and its impact on the Gulf of Mexico. J. Geophys. Res. Oceans 117, C05039. doi: 10.1029/2011JC007555
Loder J. W., Wang Z. (2015). Trends and variability of sea surface temperature in the Northwest Atlantic from three historical gridded datasets. Atmos. Ocean 53, 510–528. doi: 10.1080/07055900.2015.1071237
Lorrain A., Pethybridge H., Cassar N., Receveur A., Allain V., Bodin N., et al. (2020). Trends in tuna carbon isotopes suggest global changes in pelagic phytoplankton communities. Glob. Change Bio. 26, 458–470. doi: 10.1111/gcb.14858
Lowe T. E., Brill R. W., Cousins K. L. (2000). Blood oxygen-binding characteristics of bigeye tuna (Thunnus obesus), a high-energy-demand teleost that is tolerant to low ambient oxygen. Mar. Biol. 136, 1087–1098. doi: 10.1007/s002270000255
Luckhurst B. E., Arocha F. (2016). Evidence of spawning in the Southern Sargasso Sea of fish species managed by ICCAT – albacore tuna, swordfish and white marlin. ICCAT Collect. Vol. Sci. Pap. 72, 1949–1969.
Lynch P. D., Shertzer K. W., Cortés E., Latour R. J. (2018). Abundance trends of highly migratory species in the Atlantic Ocean: accounting for water temperature profiles. ICES J. Mar. Sci. 75, 1427–1438. doi: 10.1093/icesjms/fsy008
Mandelman J. W., Cooper P. W., Werner T. B., Lagueux K. M. (2008). Shark bycatch and depredation in the U.S. Atlantic pelagic longline fishery. Rev. Fish. Biol. Fish. 18, 427–442. doi: 10.1007/s11160-008-9084-z
Mariani P., Andersen K. H., Lindegren M., MacKenzie B. R. (2017). Trophic impact of Atlantic bluefin tuna migrations in the North Sea. ICES J. Mar. Sci. 74, 1552–1560. doi: 10.1093/icesjms/fsx027
Marshall H., Field L., Afiadata A., Sepulveda C., Skomal G., Bernal D. (2012). Hematological indicators of stress in longline-captured sharks. Comp. Biochem. Physiol. A Mol. Integr. Physiol. 162, 121–129. doi: 10.1016/j.cbpa.2012.02.008
Marshall H., Skomal G., Ross P. G., Bernal D. (2015). At-vessel and post-release mortality of the dusky (Carcharhinus obscurus) and sandbar (C. plumbeus) sharks after longline capture. Fish. Res. 172, 373–384. doi: 10.1016/j.fishres.2015.07.011
Mather F. J. III, Mason J. M. Jr., Jones A. C. (1995). Historical document: Life history and fisheries of Atlantic Bluefin Tuna. NOAA Tech Memo NMFSSEFSC-370, 165.
Matsumoto T., Kitagawa T., Kimura S. (2013). Considerations on diving patterns of bigeye tuna Thunnus obesus based on archival tag data. Fish. Sci. 79, 39–46. doi: 10.1007/s12562-012-0571-8
Matsumoto T., Saito H., Miyabe N. (2005). Swimming behavior of adult bigeye tuna using pop-up tags in the central Atlantic Ocean. ICCAT Collect. Vol. Sci. Pap. 57, 151–170.
Matsumoto W. M., Skillman R. A., Dizon A. E. (1984). Synopsis of biological data on skipjack tuna, Katsuwonus pelamis. FAO Fisheries synopsis 136 NOAA Tech. Rep. NMFS Circ. 451, 92.
McHenry J., Welch H., Lester S. E., Saba V. (2019). Projecting marine species range shifts from only temperature can mask climate vulnerability. Glob. Change Biol. 25, 4208–4221. doi: 10.1111/gcb.14828
Medina A., Abascal F. J., Megina C., Garcia A. (2002). Stereological assessment of the reproductive status of female Atlantic northern bluefin tuna during migration to Mediterranean spawning grounds through the Strait of Gibraltar. J. Fish Biol. 60, 203–217. doi: 10.1111/j.1095-8649.2002.tb02398.x
Mendoza-Alfaro R., Alvarex-Torres P. (2012). “Gulf of Mexico large marine ecosystem: resources at risk from climate change,” in Frontline Observations on Climate Change and Sustainability of Large Marine Ecosystems. Eds. Sherman K., McGovern G. (New York: UNDP), 135–169.
Mislan K. A. S., Deutsch C. A., Brill R. W., Dunne J. P., Sarmiento J. L. (2017). Projections of climate-driven changes in tuna vertical habitat based on species-specific differences in blood oxygen affinity. Glob. Change Biol. 23, 4019–4028. doi: 10.1111/gcb.13799
Miyashita S., Hattori N., Sawada Y., Ishibashi Y., Nakatsukasa H., Okada T., et al. (1999). Ontogenetic change in oxygen consumption of bluefin tuna. Thunnus thynnus. Suisanzoshoku 47, 269–275.
Mohan J. A., Jones E. R., Hendon J. M., Falterman B., Boswell K. M., Hoffmayer E. R., et al. (2020). Capture stress and post-release mortality of blacktip sharks in recreational charter fisheries of the Gulf of Mexico. Conserv. Physiol. 8, coaa04. doi: 10.1093/conphys/coaa041
Molina J. M., Cooke S. J. (2012). Trends in shark bycatch research: current status and research needs. Rev. Fish. Biol. Fish. 22, 719–737. doi: 10.1007/s11160-012-9269-3
Monllor-Hurtado A., Pennino M. G., Sanchez-Lizaso J. L. (2017). Shift in tuna catches due to ocean warming. PloS One 12, e0178196. doi: 10.1371/journal.pone.0178196
Moore G. W. K., Halfar J., Majeed H., Adey W., Kronz A. (2017). Amplification of the Atlantic Multidecadal Oscillation associated with the onset of the industrial-era warming. Sci. Rep. 7, 40861. doi: 10.1038/srep40861
Morey S. L., Schroeder J. J., O’Brien J. J., Zavala-Hidalgo J. (2003). The annual cycle of riverine influence in the eastern Gulf of Mexico basin. Geophys. Res. Lett. 30, 1867–1870. doi: 10.1029/2003GL017348
Morse R. E., Friedland K. D., Tommasi D., Stock C., Nye J. (2017). Distinct zooplankton regime shift patterns across ecoregions of the US Northeast continental shelf Large Marine Ecosystem. J. Mar. Syst. 165, 77–91. doi: 10.1016/j.jmarsys.2016.09.011
Moyano M., Candebat C., Ruhbaum Y., Alvarez-Fernandez S., Claireaux G., Zambonino-Infante J.-L., et al. (2017). Effects of warming rate, acclimation temperature and ontogeny on the critical thermal maximum of temperate marine fish larvae. PloS One 12, e0179928. doi: 10.1371/journal.pone.0179928
Muhling B. A., Lee S.-K., Lamkin J. T., Liu Y. (2011). Predicting the effects of climate change on bluefin tuna (Thunnus thynnus) spawning habitat in the Gulf of Mexico. ICES J. Mar. Sci. 68, 1051–1062. doi: 10.1093/icesjms/fsr008
Muhling B. A., Liu J., Lee S.-K., Lamkin J. T., Roffer M. A., Muller-Karger F., et al. (2015). Potential impact of climate change on the Intra-Americas Sea: Part 2. Implications for Atlantic bluefin tuna and skipjack tuna adult and larval habitats. J. Mar. Syst. 148, 1–13. doi: 10.1016/j.jmarsys.2015.01.010
Muhling B. A., Reglero P., Ciannelli L., Alvarez-Berastegui D., Alemany F., Lamkin J. T., et al. (2013). Comparison between environmental characteristics of larval bluefin tuna Thunnus thynnus habitat in the Gulf of Mexico and western Mediterranean Sea. Mar. Ecol. Progr. Ser. 486, 257–276. doi: 10.3354/meps10397
Musyl M. K., Brill R. W., Curran D. S., Fragoso M. N., McNaughton L. M., Nielsen A., et al. (2011). Postrelease survival, vertical and horizontal movements, and thermal habitats of five species of pelagic sharks in the central Pacific Ocean. Fish. Bull. 109, 341–368.
Musyl M. K., Moyes C. D., Brill R. D., Mourato B., West A., McNaughton L. M., et al. (2015). Postrelease mortality in istiophorid billfish. Can. J. Fish. Aquat. Sci. 72, 1–19. doi: 10.1139/cjfas-2014-0323
Nakano H., Matsunaga H., Hiroaki M. (2003). Acoustic tracking of bigeye thresher shark, Alopias superciliosus, in the eastern Pacific Ocean. Mar. Ecol. Progr. Ser. 265, 255–261. doi: 10.3354/meps265255
Nakano H., Okazaki M., Okamoto H. (1997). Analysis of catch depth by species for tuna longline fishery based on catch by branch lines. Bull. Natl. Res. Inst. Fish. Eng. 34, 43–62.
Nazzaro L., Slesinger E., Kohut J., Saba G. K., Saba V. S. (2021). Sensitivity of marine fish thermal habitat models to fishery data sources. Ecol. Evol. 11, 13001–13013. doi: 10.1002/ece3.7817
Nicol S. J., Allain V., Pilling G. M., Polovina J., Coll M., Bell J., et al. (2013). An ocean observation system for monitoring the effects of climate change on the ecology and sustainability of pelagic fisheries in the Pacific Ocean. Clim. Change 119, 131–145. doi: 10.1007/s10584-012-0598-y
Nicol S., Lehodey P., Senina I., Bromhead D., Frommel A. Y., Hampton J., et al. (2022). Ocean futures for the world’s largest yellowfin tuna population under the combined effects of ocean warming and acidification. Front. Mar. Sci. 9, 816772. doi: 10.3389/fmars.2022.816772
Nielsen J. L., Arrizabalaga H., Frangoso N., Hobday A., Lutcavage M., Sibert J. (2009). Tagging and Tracking of Marine Animals with Electronic Devices (London: Springer).
Nishida T., Tsuji S., Segawa K. (1998). Spatial data analyses of Atlantic bluefin tuna larval surveys in the 1994 ICCAT BYP. ICCAT Collect. Vol. Sci. Pap. 48, 199–207.
NMFS (2011). 2011 Annual report of the United States to ICCAT. National Oceanic and Atmospheric Administration, National Marine Fisheries Service, Office of Sustainable Fisheries, Highly Migratory Species Management Division (Silver Spring, MD, United State: NMFS).
NMFS (2016a). Ecosystem-based fisheries management policy of the National Marine Fisheries Service (Silver Spring, MD, United State: NMFS).
NMFS (2016b). NOAA Fisheries ecosystem-based fisheries management road map (Silver Spring, MD, United State: NMFS).
NMFS (2019a). Atlantic Highly Migratory Species Stock Assessment and Fisheries Evaluation (SAFE) Report 2019 (Silver Spring, MD, United State: NMFS).
NMFS (2019b). Supplementary evaluation of dusky shark bycatch data Amendment 5b to the 2006 Consolidated Atlantic Highly Migratory Species Fishery Management Plan (Silver Spring, MD, United State: NMFS). Available at: https://media.fisheries.noaa.gov/dam-migration/supplementary-evaluation-of-dusky-shark-bycatch-data.pdf.
NMFS (2020). Atlantic Highly Migratory Species Management-Based Research Needs and Priorities (Silver Spring, MD, United State: NMFS).
NMFS (2021). “2020 Stock Assessment and Fishery Evaluation (SAFE) Report,” in Atlantic Highly Migratory Species (Silver Spring, MD, United State: NMFS).
Nye J. A., Baker M. R., Bell R., Kenny A., Halimeda Kilbourne K., Friedland K. D., et al. (2014). Ecosystem effects of the atlantic multidecadal oscillation. J. Mar. Syst. 133, 103–116. doi: 10.1016/j.jmarsys.2013.02.006
O’Brien S. M., Gallucci V. F., Hauser L. (2013). Effects of species biology on the historical demography of sharks and their implications for likely consequences of contemporary climate change. Conserv. Genet. 14, 125–144. doi: 10.1007/s10592-012-0437-8
Oh B. Z., Sequeira A. M., Meekan M. G., Ruppert J. L., Meeuwig J. J. (2017). Predicting occurrence of juvenile shark habitat to improve conservation planning. Conserv. Biol. 31, 635–645. doi: 10.1111/cobi.12868
Oliver S., Braccini M., Newman S. J., Harvey E. S. (2015). Global patterns in the bycatch of sharks and rays. Mar. Policy 54, 86–97. doi: 10.1016/j.marpol.2014.12.017
Orbesen E. S., Brown C. A., Snodgrass D., Serafy J. E., Walter J. F. III (2019). At-vessel and postrelease mortality rates of Bluefin tuna (Thunnus thynnus) associated with pelagic longline gear in the northern Gulf of Mexico. Fish. Bull. 117, 5–23.
Pacoureau N., Rigby C. L., Kyne P. M., Sherley R. B., Winker H., Carlson J. K., et al. (2021). Half a century of global decline in oceanic sharks and rays. Nature 589, 567–571. doi: 10.1038/s41586-020-03173-9
Pade N. G., Queiroz N., Humphries N. E., Witt M. J., Jones C. S., Noble L. R., et al. (2009). First results from satellite-linked archival tagging of porbeagle shark, Lamna nasus: area fidelity, wider-scale movements and plasticity in diel depth changes. J. Exp. Mar. Biol. Ecol. 370, 64–74. doi: 10.1016/j.jembe.2008.12.002
Papastamatiou Y. P., Watanabe Y. Y., Bradley D., Dee L. E., Weng K., Lowe C. G., et al. (2015). Drivers of daily routines in an ectothermic marine predator: Hunt warm, rest warmer? PloS One 10, e0127807. doi: 10.1371/journal.pone.0127807
Parsons L. S., Lear W. H. (2001). Climate variability and marine ecosystem impacts: a North Atlantic perspective. Prog. Oceanogr. 49, 167–188. doi: 10.1016/S0079-6611(01)00021-0
Pecoraro C., Zudaire I., Bodin N., Murua H., Taconet P., Díaz-Jaimes P., et al. (2017). Putting all the pieces together: integrating current knowledge of the biology, ecology, fisheries status, stock structure and management of yellowfin tuna (Thunnus albacares). Rev. Fish Biol. Fish. 27, 811–841. doi: 10.1007/s11160-016-9460-z
Pereira Campos C., Bitar S. D. B., Freitas C. (2023). Uncertainties regarding the natural mortality of fish can increase due global climate change. PeerJ 11, e14989. doi: 10.7717/peerj.14989
Pistevos J. C. A., Nagelkerken I., Rossi T., Olmos M., Connell S. D. (2015). Ocean acidification and global warming impair shark hunting behaviour and growth. Sci. Rep. 5, 16293. doi: 10.1038/srep16293
Podestá G. P., Browder J. A., Hoey J. J. (1993). Exploring the association between swordfish catch rates and thermal fronts on U.S. longline grounds in the western North Atlantic. Cont. Shelf Res. 13, 253–277. doi: 10.1016/0278-4343(93)90109-B
Pollock M. S., Clarke L. M. J., Dubé M. G. (2007). The effects of hypoxia on fishes: from ecological relevance to physiological effects. Env. Rev. 15, 1–14. doi: 10.1139/a06-006
Poloczanska E. S., Burrows M. T., Brown C. J., García Molinos J., Halpern B. S., Hoegh-Guldberg O., et al. (2016). Responses of marine organisms to climate change across oceans. Front. Mar. Sci. 3, 62. doi: 10.3389/fmars.2016.00062
Polovina J. J., Abecassis M., Howell E. A., Woodworth P. (2009). Increases in the relative abundance of mid-trophic level fishes concurrent with declines in apex predators in the subtropical North Pacifi–2006. Fish. Bull. 107, 523–531.
Pörtner H. O., Farrel A. P. (2008). Physiology and climate change. Science 322, 690–692. doi: 10.1126/science.1163156
Pörtner H. O., Peck M. A. (2010). Climate change effects on fishes and fisheries: towards a cause-and-effect understanding. J. Fish Biol. 77, 1745–1779. doi: 10.1111/j.1095-8649.2010.02783.x
Prince E. D., Goodyear C. P. (2006). Hypoxia-based habitat compression of tropical pelagic fishes. Fish. Oceanogr. 15, 451–464. doi: 10.1111/j.1365-2419.2005.00393.x
Prince E. D., Goodyear C. P. (2007). Consequences of ocean scale hypoxia constrained habitat for tropical pelagic fishes. Gulf Caribb. Res. 19, 17–20. doi: 10.18785/gcr.1902.04
Prince E. D., Luo J., Goodyear C. P., Hoolihan J. P., Snodgrass D., Orbesen E. S., et al. (2010). Ocean scale hypoxia-based habitat compression of Atlantic istiophorid billfishes. Fish. Oceanogr. 19, 448–462. doi: 10.1111/j.1365-2419.2010.00556.x
Punt A. E., Castillo-Jordán C., Hamel O. S., Cope J. M., Maunder M. N., Ianelli J. N. (2021). Consequences of error in natural mortality and its estimation in stock assessment models. Fish. Res. 233, 105759. doi: 10.1016/j.fishres.2020.105759
Queiroz N., Humphries N. E., Couto A., Vedor M., da Costa I., Sequeira A. M. M., et al. (2019). Global spatial risk assessment of sharks under the footprint of fisheries. Nature 572, 461–466. doi: 10.1038/s41586-019-1444-4
Queiroz N., Humphries N. E., Mucientes G., Hammerschlag N., Lima F. P., Scalese K. L. (2016). Ocean-wide tracking of pelagic sharks reveals extent of overlap with longline fishing hotspots. Proc. Natl. Acad. Sci. U.S.A. 6, 1582–1587. doi: 10.1073/pnas.1510090113
Ravier C., Fromentin J. M. (2004). Are the long-term fluctuations in Atlantic bluefin tuna (Thunnus thynnus) population related to environmental changes? Fish. Oceanogr. 13, 145–160. doi: 10.1111/j.1365-2419.2004.00284.x
Reglero P., Tittensor D. P., Alvarez-Berastegui D., Aparicio-Gonzalez A., Worm B. (2014). Worldwide distributions of tuna larvae: revisiting hypotheses on environmental requirements for spawning habitats. Mar. Ecol. Progr. Ser. 501, 207–224. doi: 10.3354/meps10666
Reinhardt J. F., Weaver J., Latham P. J., Dell’Apa A., Serafy J. E., Browder J. A., et al. (2018). Catch rate and at-vessel mortality of circle hooks versus J-hooks in pelagic longline fisheries: A global meta-analysis. Fish Fish. 19, 413–430. doi: 10.1111/faf.12260
Richards W. J., Leming T., McGowan M. F., Lamkin J. T., Kelley-Fraga S. (1989). Distribution of fish larvae in relation to hydrographic features of the Loop Current boundary in the Gulf of Mexico. ICES Mar Sci Symp. 191, 169–176.
Richardson D. E., Cowen R. K., Prince E. D., Sponaugle S. (2009). Importance of the Straits of Florida spawning ground to Atlantic sailfish (Istiophorus platypterus) and blue marlin (Makaira nigricans). Fish. Oceanogr. 18, 402–418. doi: 10.1111/j.1365-2419.2009.00520.x
Richardson D. E., Marancik K. E., Guyon J. R., Lutcavage M. E., Galuardi B., Hin Lam C., et al. (2016). Discovery of a spawning ground reveals diverse migration strategies in Atlantic bluefin tuna (Thunnus thynnus). Proc. Natl. Acad. Sci. U.S.A. 113, 3299–3304. doi: 10.1073/pnas.1525636113
Ritchie E. G., Jhonson C. N. (2009). Predator interactions, mesopredator release and biodiversity conservation. Ecol. Lett. 12, 982–998. doi: 10.1111/j.1461-0248.2009.01347.x
ROman M. R., Brandt S. B., Houde E. D. (2019). and Pierson, J Interactive effects of hypoxia and temperature on coastal pelagic zooplankton and fish. J Front. Mar. Sci. 6, 139. doi: 10.3389/fmars.2019.00139
Romine J. R., Musick J. A., Burgess G. H. (2009). Demographic analyses of the dusky shark, Carcharhinus obscurus, in the Northwest Atlantic incorporating hooking mortality estimates and revised reproductive parameters. Environ. Biol. Fish. 84, 277–289. doi: 10.1007/s10641-008-9435-6
Rooker J. R., Alvarado Bremer J. R., Block B. A., Dewar H., De Metrio G., Corriero A., et al. (2007). Life history and stock structure of Atlantic bluefin tuna (Thunnus thynnus). Rev. Fish. Sci. 15, 265–310. doi: 10.1080/10641260701484135
Rooker J. R., Secor D. H., De Metrio G., Schloesser R., Block B. A., Neilson J. D. (2008). Natal homing and connectivity in Atlantic bluefin tuna populations. Science 322, 742–744. doi: 10.1126/science.1161473
Rooker J. R., Simms J. R., Wells R. J. D., Holt S. A., Holt G. J., Graves J. E., et al. (2012). Distribution and habitat associations of billfish and swordfish larvae across mesoscale features in the Gulf of Mexico. PloS One 7, e34180. doi: 10.1371/journal.pone.0034180
Rudershausen P. J., Poland S. J., Merten W., Buckel J. A. (2019). Estimating discard mortality for dolphinfish in a recreational hook-and-line fishery. N. Am. J. Fish. Manage. 39, 1143–1154. doi: 10.1002/nafm.10348
Saba V. S., Griffies S. M., Anderson W. G., Winton M., Alexander M. A., Delworth T. L., et al. (2016). Enhanced warming of the Northwest Atlantic Ocean under climate change. J. Geophys. Res. Oceans 121, 118–132. doi: 10.1002/2015JC011346
Saito H., Takeuchi Y., Yokawa K. (2004). Vertical distribution of Atlantic blue marlin obtained from pop-up archival tags in the tropical Atlantic Ocean. SCRS/2003/037. ICCAT Collect. Vol. Sci. Pap. 56, 201–211.
Santos C. C., Coelho R. (2018). Migrations and habitat use of the smooth hammerhead shark (Sphyrna zygaena) in the Atlantic Ocean. PloS One 13, e0198664. doi: 10.1371/journal.pone.0198664
Saunders R. A., Royer F., Clarke M. W. (2011). Winter migration and diving behaviour of porbeagle shark, Lamna nasus, in the Northeast Atlantic. ICES J. Mar. Sci. 68, 166–174. doi: 10.1093/icesjms/fsq145
Scavia D., Field J. C., Boesch D. F., Buddemeier R. W., Burkett V., Cayan D. R., et al. (2002). Climate change impacts on U.S. coastal and marine ecosystems. Estuaries 25, 149–164. doi: 10.1007/BF02691304
Schaefer K. M., Fuller D. W. (2010). Vertical movements, behavior, and habitat of bigeye tuna (Thunnus obesus) in the equatorial eastern Pacific Ocean, ascertained from archival tag data. Mar. Biol. 157, 2625–2642. doi: 10.1007/s00227-010-1524-3
Schaefer K. M., Fuller D. W., Block B. A. (2009). “Vertical movements and habitat utilization of skipjack (Katsuwonus pelamis), yellowfin (Thunnus albacares), and bigeye (Thunnus obesus) tunas in the Equatorial Eastern Pacific Ocean, ascertained through archival tag data,” in Tagging and tracking of marine animals with electronic devices. Eds. Nielsen J. L., Arrizabalaga H., Fragoso N., Hobday A., Lutcavage M., Sibert J. (New York: Springer), 121–144.
Schick R. S., Goldstein J., Lutcavage M. E. (2004). Bluefin tuna (Thunnus thynnus) distribution in relation to sea surface temperature fronts in the Gulf of Main-96). Fish. Oceanogr. 13, 225–238. doi: 10.1111/j.1365-2419.2004.00290.x
Schlaff A. M., Heupel M. R., Simpfendorfer C. A. (2014). Influence of environmental factors on shark and ray movement, behaviour and habitat use: a review. Rev. Fish. Biol. Fish. 24, 1089–1103. doi: 10.1007/s11160-014-9364-8
Schmidt-Nielsen K. (1983). Animal physiology: Adaptation and environment (New York: Cambridge University Press).
Schmidt-Nielsen K. (1984). Scaling: why is animal size so important? (New York: Cambridge University Press).
Schmittner A., Latif M., Schneider B. (2005). Model projections of the North Atlantic thermohaline circulation for the 21st century assessed by observations. Geophys. Res. Lett. 32, L23710. doi: 10.1029/2005GL024368
Schulze-Haugen M., Kohler N. E. (2003). Guide to sharks, tunas, & billfishes of the U.S. Atlantic and Gulf of Mexico (Narragansett, RI, USA: Rhode Island Sea Grant & NOAA Fisheries).
SEDAR (2016). Update assessment to SEDAR 21: HMS Dusky Shark (North Charleston, South Carolina: SEDAR).
Sedberry G. R., Loefer J. K. (2001). Satellite telemetry tracking of swordfish, Xiphias gladius, off the eastern United States. Mar. Biol. 139, 355–360. doi: 10.1007/s002270100593
Seki M. P., Lumpkin R., Flament P. (2002). Hawaii cyclonic eddies and blue marlin catches: the case study of the 1995 Hawaiian International Billfish Tournament. J. Oceanogr. 58, 739–745. doi: 10.1023/A:1022854609312
Serafy J. E., Kerstetter D. W., Rice P. H. (2009). Can circle hook use benefit billfishes? Fish Fish. 10, 132–142. doi: 10.1111/j.1467-2979.2008.00298.x
Sergeant C. J., Armstrong J. B., Ward E. J. (2014). Predator–prey migration phenologies remain synchronized in a warming catchment. Freshw. Biol. 60, 724–732. doi: 10.1111/fwb.12524
Sharp G. D., Vlymen W. J. (1978). “The relation between heat generation, conservation, and the swimming energetics of tunas,” in The physiological ecology of tunas. Eds. Sharp G. D., Dizon A. E. (San Diego: Academic Press), 213–232.
Shaw R. L., Curtis T. H., Metzger G., McCallister M. P., Newton A., Fischer G. C., et al. (2021). Three-dimensional movements and habitat selection of young white sharks (Carcharodon carcharias) across a temperate continental shelf ecosystem. Front. Mar. Sci. 8, 643831. doi: 10.3389/fmars.2021.643831
Shiffman D. S., Hammerschlag N. (2014). An assessment of the scale, practices, and conservation implications of Florida’s charterboat-based recreational shark fishery. Fisheries 39, 395–407. doi: 10.1080/03632415.2014.941439
Sieburth J. M. (1983). “Microbiological and organic-chemical processes in the surface and mixed layers,” in Air-sea exchange of gases and particles, vol. 108 . Eds. Liss P. S., Slinn W. G. N. (Dordrecht: NATO ASI Series (Ser C: Mathem. Phys. Sci Springer).
Simms J. R., Rooker J. R., Holt S. A., Holt G. J., Bangma J. (2010). Distribution, growth, and mortality of sailfish (Istiophorus platypterus) larvae in the northern Gulf of Mexico. Fish. Bull. 108, 478–490.
Simpson S. D., Jennings S., Johnson M. P., Blanchard J. L., Schön P.-J., Sims D. W., et al. (2011). Continental shelf-wide response of a fish assemblage to rapid warming of the sea. Curr. Biol. 21, 1565–1570. doi: 10.1016/j.cub.2011.08.016
Sims D. W. (2019). “The significance of ocean deoxygenation for elasmobranchs,” in Ocean deoxygenation – everyone’s problem: causes, impacts, consequences and solutions. Eds. Laffoley D., Baxter J. M. (Gland, Switzerland: IUCN), 451–468.
Skomal G. B. (2007). Evaluating the physiological and physical consequences of capture on post-release survivorship in large pelagic fishes. Fish. Manage. Ecol. 14, 81–89. doi: 10.1111/j.1365-2400.2007.00528.x
Skomal G. B., Bernal D. (2010). “Physiological responses to stress in sharks,” in Sharks and their relatives II: Biodiversity, adaptive physiology, and conservation. Eds. Carrier J., Musick J., Heithaus M. (Boca Raton: CRC Press), 459–490.
Skomal G. B., Braun C. D., Chisholm J. H., Thorrold S. R. (2017). Movements of the white shark Carcharodon carcharias in the North Atlantic Ocean. Mar. Ecol. Progr. Ser. 580, 1–16. doi: 10.3354/meps12306
Skomal G. B., Mandelman J. W. (2012). The physiological response to anthropogenic stressors in marine elasmobranch fishes: a review with a focus on the secondary response. Comp. Biochem. Physiol. A Mol. Integr. Physiol. 162, 146–155. doi: 10.1016/j.cbpa.2011.10.002
Skomal G. B., Marshall H., Galuardi B., Natanson L., Braun C. D., Bernal D. (2021). Horizontal and vertical movement patterns and habitat use of juvenile porbeagles (Lamna nasus) in the Western North Atlantic. Front. Mar. Sci. 8, 624158. doi: 10.3389/fmars.2021.624158
Skubel R. A., Kirtman B. P., Fallows C., Hammerschlag N. (2018). Patterns of long-term climate variability and predation rates by a marine apex predator, the white shark. Carcharodon carcharias. Mar. Ecol. Progr. Ser. 587, 129–139. doi: 10.3354/meps12424
Smith S. E., Aseltine-Neilson D. (2001). “Thresher shark,” in California’s Living Marine Resources: A Status Report. Sea Grant Publication SG01-11. Eds. Leet W. S., Dewees C. M., Klingbeil R., Larson E. J. (Sacramento, CA, USA: California Department of Fish and Game/University of California Agriculture and Natural Resources), 339–341.
Smith S. E., Rasmussen R. C., Ramon D. A., Cailliet G. M. (2008). “The biology and ecology of thresher sharks (Alopiidae),” in Sharks of the Open Ocean. Eds. Camhi M. D., Pikitch E. K., Babcock E. A. (San Francisco: Wiley-Blackwell Publishing), 60–68.
Solomon S., Qin D., Manning M., Chen Z., Marquis M., Averyt K. B., et al (Eds.) (2007). Climate change 2007: The physical science basis, contribution of Working Group I to the Fourth Assessment Report of the Intergovernmental Panel on Climate Change (Cambridge and New York: Cambridge University Press).
Speed C. W., Meekan M. G., Field I. C., McMahon C. R., Bradshaw C. J. A. (2012). Heat-seeking sharks: support for behavioral thermoregulation in reef sharks. Mar. Ecol. Progr. Ser. 463, 231–244. doi: 10.3354/meps09864
Stendardo I., Gruber N. (2012). Oxygen trends over five decades in the North Atlantic. J. Geophys. Res. 117, C11004. doi: 10.1029/2012JC007909
Stevens J. D., Bradford R. W., West G. J. (2010). Satellite tagging of blue sharks (Prionace glauca) and other pelagic sharks off eastern Australia: depth behaviour, temperature experience and movements. Mar. Biol. 157, 575–591. doi: 10.1007/s00227-009-1343-6
Stock C. A., Alexander M. A., Bond N. A., Brander K. M., Cheung W. W. L., Curchitser E. N. (2011). On the use of IPCC-class models to assess the impact of climate on living marine resources. Prog. Oceanogr. 88, 1–27. doi: 10.1016/j.pocean.2010.09.001
Stock C. A., John J. G., Rykaczewski R. R., Asch R. G., Cheung W. W. L., Dunne J. P., et al. (2017). Reconciling fisheries catch and ocean productivity. Proc. Natl. Acad. Sci. U.S.A. 114, E1441–E1449. doi: 10.1073/pnas.1610238114
Stocker T. F., Qin D., Plattner G., Tignor M., Allen S. K., Boschung J., et al (Eds.) (2013). IPCC, 2013: Summary for policymakers in climate change 2013: The physical science basis, contribution of Working Group I to the Fifth Assessment Report of the Intergovernmental Panel on Climate Change (Cambridge: Cambridge University Press).
Stoehr A., Martin J. S., Aalbers S., Sepulveda C., Bernal D. (2018). Free-swimming swordfish, Xiphias gladius, alter the rate of whole body heat transfer: morphological and physiological specializations for thermoregulation. ICES J. Mar. Sci. 75, 858–870. doi: 10.1093/icesjms/fsx163
Stramma L., Prince E. D., Schmidtko S., Luo J., Hoolihan J. P., Visbeck M., et al. (2012). Expansion of oxygen minimum zones may reduce available habitat for tropical pelagic fishes. Nat. Clim. Change 2, 33–37. doi: 10.1038/nclimate1304
Su N.-J., Sun C.-L., Punt A. E., Yeh S.-Z., DiNardo G. (2011). Modelling the impacts of environmental variation on the distribution of blue marlin, Makaira nigricans, in the Pacific Ocean. ICES J. Mar. Sci. 68, 1072–1080. doi: 10.1093/icesjms/fsr028
Sulikowski J. A., Golet W., Hoffmayer E. R., Driggers W. B. III, Natanson L. J., Carlson A., et al. (2020). Observing post-release mortality for dusky sharks, Carcharhinus obscurus, captured in the U.S. pelagic longline fishery. Fish. Res. 221, 105341. doi: 10.1016/j.fishres.2019.105341
Sundby S., Nøttestad L., Myklevoll S., Tangen Ø. (2013). Swordfish towards the Arctic Atlantic in climate change. ICCAT Collect. Vol. Sci. Pap. 69, 1296–1303.
Takahashi M., Okamura H., Yokawa K., Okazaki M. (2003). Swimming behaviour and migration of swordfish recorded by an archival tag. Mar. Freshw. Res. 54, 527–534. doi: 10.1071/MF01245
Tanaka K. R., Van Houtan K. S., Mailander E., Dias B. S., Galginaitis C., O’Sullivan J., et al. (2021). North Pacific warming shifts the juvenile range of a marine apex predator. Sci. Rep. 113373. doi: 10.1038/s41598-021-82424-9
Teo S. L. H., Block B. A. (2010). Comparative influence of ocean conditions on yellowfin and Atlantic bluefin tuna catch from longlines in the Gulf of Mexico. PloS One 5, e10756. doi: 10.1371/journal.pone.0010756
Teo S. L. H., Boustany A. M., Block B. A. (2007). Oceanographic preferences of Atlantic bluefin tuna, Thunnus thynnus, on their Gulf of Mexico breeding grounds. Mar. Biol. 152, 1105–1119. doi: 10.1007/s00227-007-0758-1
Thackeray S. J., Henrys P. A., Hemming D., Bell J. R., Botham M. S., Burthe S., et al. (2016). Phenological sensitivity to climate across taxa and trophic levels. Nature 535, 241–245. doi: 10.1038/nature18608
Thums M., Meekan M., Stevens J., Wilson S., Polovina J. (2013). Evidence for behavioural thermoregulation by the world’s largest fish. J. R. Soc Interface 10, 20120477. doi: 10.1098/rsif.2012.0477
Tidwell M. T., Holt S., Rooker J. R., Holt G. J. (2007). The distribution and feeding ecology of larval billfish in the northern Gulf of Mexico. Proceed 60th Gulf Car Fish Institute. Punta Cana Dominican Republic, 379–384.
Tolotti M. T., Bach P., Hazin F., Travassos P., Dagorn L. (2015a). Vulnerability of the oceanic whitetip shark to pelagic longline fisheries. PloS One 10, e0141396. doi: 10.1371/journal.pone.0141396
Tolotti M. T., Filmalter J. D., Bacha P., Travassos P., Seret B., Dagorna L. (2015b). Banning is not enough: The complexities of oceanic shark management by tuna regional fisheries management organizations. Glob. Ecol. Conserv. 4, 1–7. doi: 10.1016/j.gecco.2015.05.003
Tolotti M. T., Travassos P., Frédou F. L., Wor C., Andrade H. A., Hazin F. (2013). Size, distribution and catch rates of the oceanic whitetip shark caught by the Brazilian tuna longline fleet. Fish. Res. 143, 136–142. doi: 10.1016/j.fishres.2013.01.014
Trenkel V. M., Huse G., MacKenzie B. R., Alvarez P., Arrizabalaga H., Castonguay M., et al. (2014). Comparative ecology of widely distributed pelagic fish species in the North Atlantic: Implications for modelling climate and fisheries impacts. Progr. Oceanogr. 129, 219–243. doi: 10.1016/j.pocean.2014.04.030
Udyawer V., Chin A., Knip D., Simpfendorfer C. A., Heupel M. R. (2013). Variable response of coastal sharks to severe tropical storms: environmental cues and changes in space use. Mar. Ecol. Progr. Ser. 480, 171–183. doi: 10.3354/meps10244
Vaudo J. J., Byrne M. E., Wetherbee B. M., Harvey G. M., Shivji M. S. (2017). Long-term satellite tracking reveals region-specific movements of a large pelagic predator, the shortfin mako shark, in the western North Atlantic Ocean. J. Appl. Ecol. 54, 1765–1775. doi: 10.1111/1365-2664.12852
Vaudo J. J., Wetherbee B. M., Wood A. D., Weng K., Howey-Jordan L. A., Harvey G. M., et al. (2016). Vertical movements of shortfin mako sharks Isurus oxyrinchus in the western North Atlantic Ocean are strongly influenced by temperature. Mar. Ecol. Progr. Ser. 547, 163–175. doi: 10.3354/meps11646
Vedor M., Queiroz N., Mucientes G., Couto A., da Costa I., dos Santos A., et al. (2021). Climate-driven deoxygenation elevates fishing vulnerability for the ocean’s widest ranging shark. eLife 10, e62508. doi: 10.7554/eLife.62508
Walter J., Sharma R., Ortiz M. (2018). Western Atlantic bluefin tuna stock assessment 1950-2015 using Stock Synthesis. ICCAT Collect. Vol. Sci. Pap. 74, 3305–3404.
Walther G. R., Post E., Convey P., Menzel A., Parmesan C., Beebee T. J. C., et al. (2002). Ecological responses to recent climate change. Nature 416, 389–395. doi: 10.1038/416389a
Wang Z., Horwitz R., Bowlby H. D., Ding F., Joyce W. N. (2020b). Changes in ocean conditions and hurricanes affect porbeagle Lamna nasus diving behavior. Mar. Ecol. Progr. Ser. 654, 219–224. doi: 10.3354/meps13503
Wang H.-Y., Shen S.-F., Chen Y.-S., Kiang Y.-K., Heino M. (2020a). Life histories determine divergent population trends for fishes under climate warming. Nat. Commun. 11, 4088. doi: 10.1038/s41467-020-17937-4
Ward P., Hindmarsh S. (2007). An overview of historical changes in the fishing gear and practices of pelagic longliners, with particular reference to Japan’s Pacific fleet. Rev. Fish. Biol. Fish. 17, 501–516. doi: 10.1007/s11160-007-9051-0
Ward P., Myers R. A. (2005). Shifts in open-ocean fish communities coinciding with the commercial fishing. Ecol 86, 835–847. doi: 10.1890/03-0746
Ward-Paige C. A., Britten G. L., Bethea D. M., Carlson J. K. (2015). Characterizing and predicting essential habitat features for juvenile coastal sharks. Mar. Ecol. 36, 419–431. doi: 10.1111/maec.12151
Weng K. C., Block B. A. (2004). Diel vertical migration of the bigeye thresher shark (Alopias superciliosus), a species possessing orbital retia mirabilia. Fish. Bull. 102, 221–229.
Weng K. C., Stokesbury M. J. W., Boustany A. M., Seitz A. C., Teo S. L. H., Miller S. K., et al. (2009). Habitat and behaviour of yellowfin tuna Thunnus albacares in the Gulf of Mexico determined using pop-up satellite archival tags. J. Fish. Biol. 74, 1434–1449. doi: 10.1111/j.1095-8649.2009.02209.x
Wexler J. B., Margulies D., Scholey V. P. (2011). Temperature and dissolved oxygen requirements for survival of yellowfin tuna, Thunnus albacares, larvae. J. Exp. Mar. Biol. Ecol. 404, 63–72. doi: 10.1016/j.jembe.2011.05.002
Wills R. C. J., Armour K. C., Battisti D. S., Hartmann D. L. (2019). Ocean–atmosphere dynamical coupling fundamental to the Atlantic multidecadal oscillation. J. Clim. 32, 251–272. doi: 10.1175/JCLI-D-18-0269.1
Woodward G., Benstead J. P., Beveridge O. S., Blanchard J., Brey T., Brown L. E., et al. (2010). Ecological networks in a changing climate. Adv. Ecol. Res. 42, 71–138. doi: 10.1016/B978-0-12-381363-3.00002-2
Wu Y.-L., Lan K.-W., Tian Y. J. (2020). Determining the effect of multiscale climate indices on the global yellowfin tuna (Thunnus albacares) population using a time series analysis. Deep Sea Res. 2 Top. Stud. Oceanogr. 175, 104808. doi: 10.1016/j.dsr2.2020.104808
Keywords: bycatch, climate impacts, Gulf of Mexico, highly migratory species, Northwest Atlantic, pelagic longline
Citation: Dell’Apa A, Boenish R, Fujita R and Kleisner K (2023) Effects of climate change and variability on large pelagic fish in the Northwest Atlantic Ocean: implications for improving climate resilient management for pelagic longline fisheries. Front. Mar. Sci. 10:1206911. doi: 10.3389/fmars.2023.1206911
Received: 16 April 2023; Accepted: 28 August 2023;
Published: 20 September 2023.
Edited by:
Mark Meekan, University of Western Australia, AustraliaReviewed by:
Geir Huse, Norwegian Institute of Marine Research (IMR), NorwayPeter J. Auster, Mystic Aquarium, United States
Patrick Lehodey, Mercator Ocean (France), France
Copyright © 2023 Dell’Apa, Boenish, Fujita and Kleisner. This is an open-access article distributed under the terms of the Creative Commons Attribution License (CC BY). The use, distribution or reproduction in other forums is permitted, provided the original author(s) and the copyright owner(s) are credited and that the original publication in this journal is cited, in accordance with accepted academic practice. No use, distribution or reproduction is permitted which does not comply with these terms.
*Correspondence: Andrea Dell’Apa, ZGVsbGFwYS5hbmRyZWFAZ21haWwuY29t
†ORCID: Andrea Dell’Apa, orcid.org/0000-0001-5939-8082
Robert Boenish, orcid.org/0000-0002-2662-8723
Kristin Kleisner, orcid.org/0000-0002-6918-1546