- 1Australian Antarctic Division, Kingston, TAS, Australia
- 2Australian Antarctic Program Partnership, University of Tasmania, Hobart, TAS, Australia
- 3Ocean Observations Group, National Institute for Water and Atmospheric Research, Wellington, New Zealand
- 4Department of Physics, University of Auckland, Auckland, New Zealand
- 5Department of Glacial Environment Research, Korea Polar Research Institute, Incheon, Republic of Korea
- 6Vice President’s Office, Korea Polar Research Institute, Incheon, Republic of Korea
- 7School of Earth and Environment, University of Canterbury, Christchurch, New Zealand
Our understanding of cross-disciplinary connections for Antarctica’s role in the Earth system remains incomplete, especially around its coastal margins. The focus here is on sea-ice, oceanic, and atmospheric drivers in the joint Ross Sea-far East Antarctic Region (RSfEAR)—one which spans a large longitudinal range and connects a number of ice shelves and polynyas promoting sea-ice growth and underpinning a diverse and rich ecosystem. Here, we present a minireview of recent case studies and how these inform the design for a future integrated ocean–sea ice–atmosphere observing system. The review is built around five themes: i) regional setting, ii) recent studies in the region and current strategies, iii) gap analysis, iv) future observing system design, and v) wider implications for stakeholders.
1 Introduction
Polar processes are critical in shaping our climate and overall Earth system. Large annual cycles, like that of sea ice, significantly impact aspects of the Earth’s climate system (Ayres et al., 2022) and ecosystems (Swadling et al., 2023) as well as the global radiation balance, air–sea heat exchange, and light penetration. Sea-ice formation and melt alter the buoyancy structure of the ocean, cloud properties, and consequently, the surface-radiation budget. Seawater freezing and the associated brine rejection result in salty, oxygenated water draining to the seafloor on a millennium-long global journey. Through advection, the almost salt-free sea ice drifts net-northward to eventually melt, stratifying the surface ocean elsewhere. The associated marine biogenic activity enhances trace-gas emissions into the atmosphere, modulating aerosols and thus cloud properties over the Southern Ocean (Mace et al., 2021; McFarquhar et al., 2021; Mace et al., 2023; Mallet et al., 2023).
Our understanding of cross-disciplinary connections for the polar system remains incomplete, especially around its coastal margins. This is largely due to low visitation, highly variable atmospheric drivers, ice–ocean interactions, varied coastal interactions, and the complex physical nature of various forms of ice and snow. The joint Ross Sea-far East Antarctic Region (RSfEAR) extends latitudinally from 85° to 65°S and spans a broad longitudinal range from 110°E to 155°W (Figure 1A, black dashed lines), connecting the wide Ross continental shelf to the narrower Far East continental shelf region around Cape Adare. RSfEAR is home to major ice shelves (Ross, Nansen), glacier tongues (Drygalski, Mertz, Dalton), and polynyas [Ross, McMurdo, Terra Nova Bay (TNB), Mertz, Ninnis, Dalton] (Figure 1A). The fate of sea ice here is linked to the integrity of these features and the ecosystems they support.
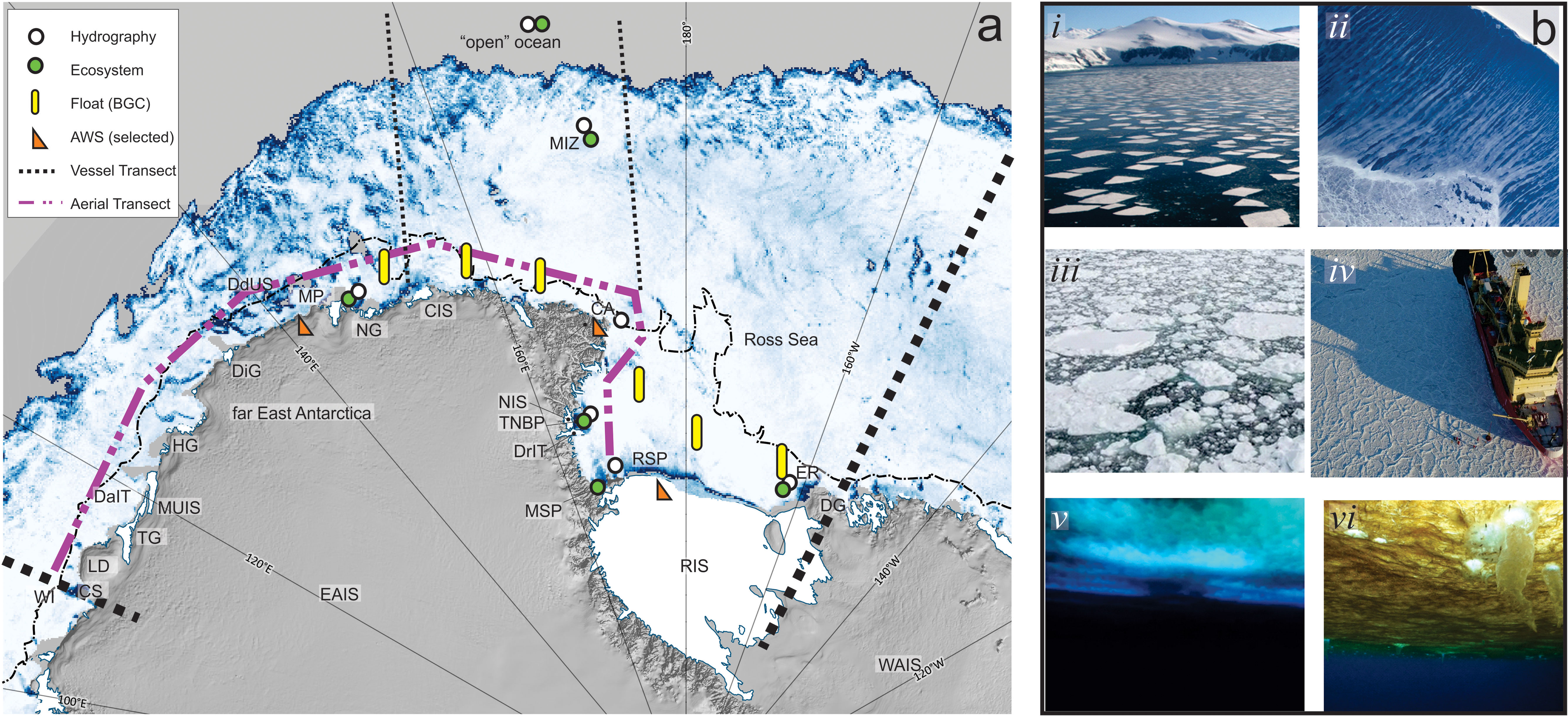
Figure 1 (A) The RSfEAR region of interest spanning between 110°E and 155°W (bold dashed lines) superimposed over sea-ice concentration (September 2022). EAIS and WAIS are East and West Antarctic Ice Sheet; CS, Casey Station; LD, Law Dome; CA, Cape Adare; ER, Eastern Ross; NIS, Nansen Ice Shelf; RIS, Ross Ice Shelf; TG, Totten Glacier; NG, Ninnis Glacier; MUIS, Moscow University Ice Shelf; DaIT, Dalton Iceberg Tongue; HG, Holmes Glacier; DiG, Dibble Glacier; DG, Dalton Glacier; DIT, Drygalski Ice Tongue; MP, Mertz Polynya; TNBP, Terra Nova Bay Polynya; MSP, McMurdo Ice Shelf Polynya; RSP, Ross Sea Polynya; WI, Windmill Islands; Dd’US, Dumont d’Urville Sea; MIZ, Marginal Ice Zone. The continental shelf-break is marked by a dash-dot line. The figure also shows potential locations for key elements of an observing system including hydrographic stations, ecosystem monitoring, nominal biogeochemical (BGC) float coverage, automatic weather stations (AWS), and ocean–sea ice–atmosphere transect information either from vessel or air. (B) Sea ice is highly varied: a mosaic of different sea-ice conditions including (i) sparse pack, (ii) active polynya, (iii) marginal ice zone, (iv) fast ice, (v) platelet underside, and (vi) significant under ice productivity.
This review uses recent studies in the RSfEAR as the basis for a conceptual design (Figure 1A) for an integrated regional ice–ocean observing system that spans the geographical domain while connecting basic properties to ecosystem response and societal usage and value (Figure 2A).
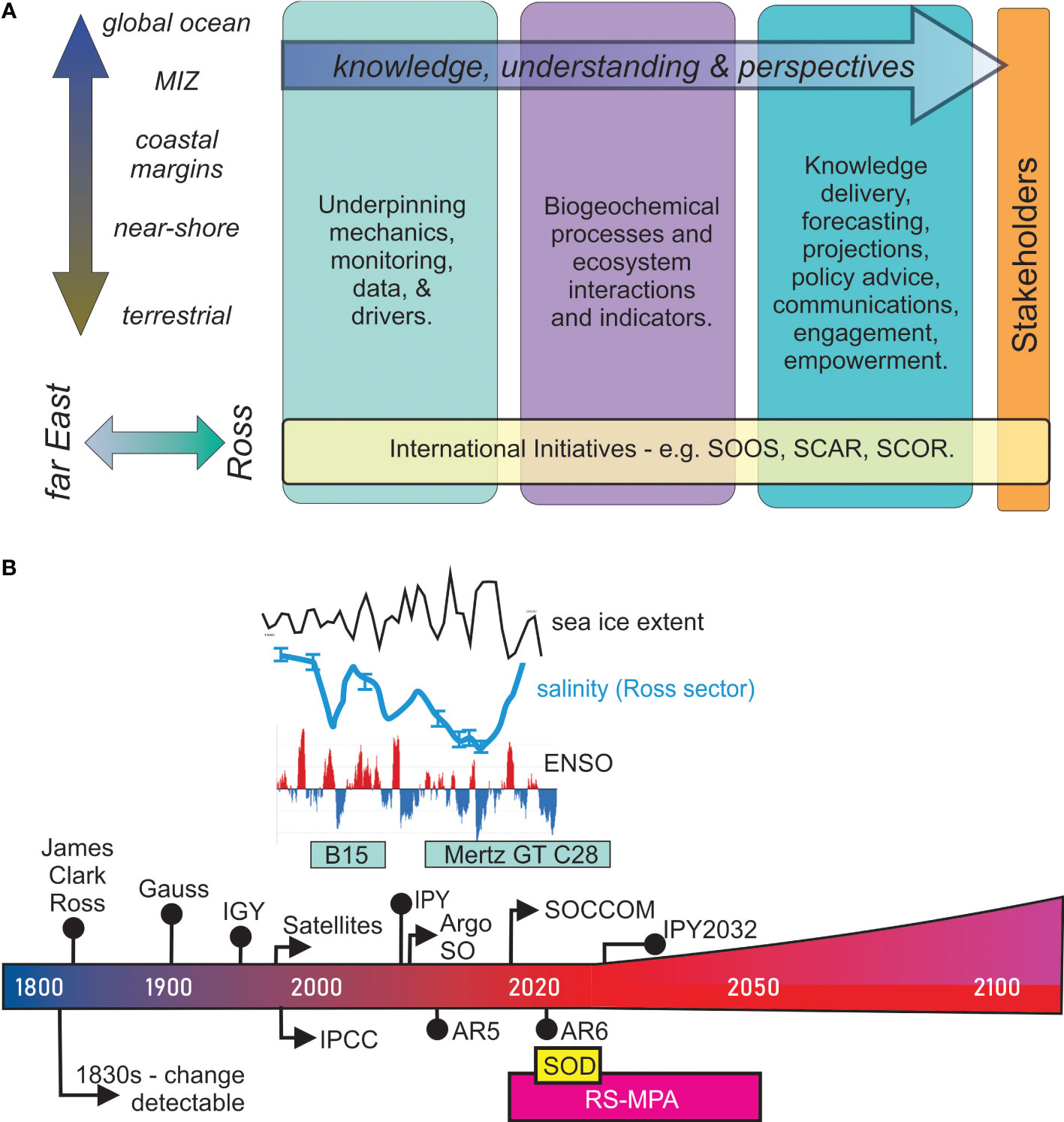
Figure 2 (A) Key integrated observing system components with a spatial element and then themes moving from underpinning through ecosystem to stakeholder focus underpinned by international coordinated efforts. (B) A timeline for the RSfEAR spanning three centuries (note the non-linear time dimension) including key observational initiatives from the early discovery voyages (James Clark Ross, Gauss, IGY) to modern technological approaches (Argo, satellite, SOCCOM). Modern era time series such as the sea-ice extent and Southern Oscillation strength and salinity of the region are included. Significant cryospheric events in the form of the calving of giant icebergs B15 and C28 are also included along with policy developments (MPA, IPCC AR5 and AR6, and Southern Ocean Decade—SOD).
2 Motivation
Recent extremes in Antarctic sea-ice extent (Stuecker et al., 2017; Raphael and Handcock, 2022; Turner et al., 2022), record cryospheric melt (Bell et al., 2017; Adusumilli et al., 2020), and reduction in bottom-water oxygen (Gunn et al., 2023) precipitated this work. Freshwater generated by the substantial melting in the West Antarctic is moving further west. This is superimposed on the long-term oceanic changes around Antarctica’s margins and accentuated by atmospheric changes (Jacobs et al., 2022).
Our contextual timeline for the region relates events to major milestones in exploration and stakeholder perspectives (Figure 2B). This reveals that, with the exception of southern McMurdo Sound (e.g., Langhorne et al., 2015; Lubin et al., 2020), TNB (e.g., Sansiviero et al., 2017; Bracci et al., 2022), the Windmill Islands (e.g., Clark et al., 2017), and the Dumont d’Urville Sea (e.g., Barbraud et al., 2015; Grazioli et al., 2017; Vignon et al., 2020), the RSfEAR is poorly observed. Hence, understanding these changes and their future trajectory requires renewed thinking in terms of information requirements.
2.1 Recent studies in the region
Several recent studies set the scene for identifying future initiatives. These include changes in sea-ice extent (Comiso et al., 2011), ice season length (Stammerjohn et al., 2012), and thickness (Rack et al., 2021) as well as subsurface ocean forcing (Zhang et al., 2022). Critical for the Antarctic are large ice shelves and the associated production of ice-shelf water and platelet-ice crystals (Hoppmann et al., 2020; Stevens et al., 2023). Declining summer and autumn sea-ice cover in the western Ross Sea and far eastern East Antarctic has increased the length of the coastline left unprotected at this time (Reid and Massom, 2022). With an evolving climate, the loss of sea ice and fast ice will increase the exposure to open ocean and waves (Voermans et al., 2021).
Polynyas, although overlooked in a recent horizon scan (Kennicutt et al., 2015), play a vital role in the Antarctic margin by interconnecting the atmosphere, ice, and ocean (Knuth and Cassano, 2014). They serve as an important nutrient source (Arrigo and van Dijken, 2003; Arrigo et al., 2015), fostering the production of sea ice (Tamura et al., 2008) and high-salinity shelf water (Tamura et al., 2016). Polynya activity and glacier-tongue and iceberg evolution are entwined (Barber and Massom, 2007; Yoon et al., 2020). For example, the collapse of the Mertz Glacier Tongue resulted in decades-long changes in near-coastal sea-ice formation (Tamura et al., 2012; Tamura et al., 2016).
Regional salinity trends show a long-term salinity reduction in deeper waters (Silvano et al., 2020; Bowen et al., 2021; Jacobs et al., 2022) and associated impacts on bottom-water formation (e.g., Gunn et al., 2023; Li et al., 2023). However, in recent years, this trend has reversed (Castagno et al., 2019). Tracking and projecting this trend is a major objective for the RSfEAR observing system design. Acquisition of upper ocean stratification and mixing data is operationally challenging. Furthermore, enhanced sea-ice production in coastal polynya increases Antarctic bottom-water formation. However, calving events and changing configurations of ice-shelf fronts, icebergs, and fast ice play a major role in reducing sea-ice production near the Ross Sea and Mertz glaciers (Tamura et al., 2016).
Ice shelves are a critical endemic component of the Antarctic margin. The large Ross Ice Shelf is essentially stable at present. However, the recent Conger Ice Shelf collapse to the west of the RSfEAR, as well as the Cook Ice Shelf collapse in the 1970s (Miles et al., 2018), suggests that stability is not sector-wide. The Cook glacier-shelf system, part of the Wilkes Basin drainage, provided evidence of subglacial flood events with dramatic velocity changes. However, “in terms of observations of subsurface ocean temperatures, bathymetry, and bed topography, it is one of the least studied” (Miles et al., 2018) and makes a central focal point for the RSfEAR observing system.
Sea-ice cover in the Ross Sea has increased over the satellite record, but trend reversals since 2014 have markedly reduced the statistical significance of this general increase (Parkinson, 2019). The seasonal cycle in the Ross Sea has a consistent minimum in February, but the winter maximum varies between July and November, consistent with the wider RSfEAR region (Parkinson, 2019). High winter maxima can be followed by either high or low February sea-ice extents, and it is clear that the surrounding atmospheric and oceanic conditions play a major role in shaping the decay. The El Niño Southern Oscillation and the Southern Annular Mode have a significant impact on the sea-ice trends across the RSfEAR (Gloersen, 1995; Simmonds and Jacka, 1995; Kwok and Comiso, 2002; Turner, 2004; Yuan, 2004; Simpkins et al., 2012; Matear et al., 2015; Hobbs et al., 2016). Recently, Zhang et al. (2022) showed that subsurface warming can destabilize the ocean from below and lead to years of low sea-ice cover. Analysis shows that anomalous southwesterly winds moved the Ross Sea sea ice offshore and northward, decreasing sea ice in the Ross Sea but increasing it to the north of the Ross Sea (Zhang and Li, 2023).
There is growing evidence that wave–ice interactions are important in the sea-ice evolution. However, wave dispersion and attenuation in sea ice, including the Marginal Ice Zone (MIZ), are poorly understood (Squire, 2022), let alone the importance of wave–ice interactions under future sea-ice states (Bennetts et al., 2022). Wave attenuation depends on local ice, wave, and wind conditions (Montiel et al., 2022), and year-round, high-quality observations for different ice types are needed. A lack of observations of air–sea ice–sea fluxes (Swart et al., 2019) constrains both understanding and forecasting.
2.2 Current strategies
Developments in sea-ice and ocean science in the RSfEAR are set within a number of strategies from national and international perspectives, and the proposed observing system design needs to work in this context:
● The United Nations’ Southern Ocean Decade (Janssen et al., 2022) identifies outcomes (e.g., clean, productive, predicted, safe oceans), all of which require science and data as central pillars.
● The Southern Ocean Observing System (SOOS) Implementation Plan (Newman et al., 2019) focuses on data access and future observational opportunities.
● The World Meteorological Organization’s Antarctic Regional Climate Center (Ma and Qin, 2018) is in pre-implementation planning to support agencies with regional products.
● The Fifth International Polar Year 2032/33 will fill the need for coordinated international research to address the biggest challenges of polar research, for both the polar regions themselves and for the Earth as a whole (World-Meteorological-Organization, 2022).
● National programs express interest in, and support for, Southern Ocean research and thus implicitly the RSfEAR including:
● New Zealand’s Foreign Affairs and Trade Ministry’s 2020-2030 Strategy (MFAT, 2021) provides a national perspective on Antarctica and the Southern Ocean regarding science, operations, and motivations with an emphasis on Scott Base and maritime operations.
● The Australian Department of Foreign Affairs and Trade’s Australian Antarctic Science Strategy (DCCEEW, 2022) updates a national perspective in the context of the Antarctic Treaty highlighting the large area of responsibility.
● The Australian Antarctic Science Program requires participants to deliver on key government policy and international obligations, science, and management outcomes, with the Australian Antarctic Science Decadal Plan (under development) to iterate on this.
● The Republic of Korea’s governmental Antarctic plan outlines the importance of understanding the impacts of glacial melt and collapse on the Southern Ocean and beyond, including for sea-level rise and storage of anthropogenic carbon; research funding has been made available for this.
● The Ross Sea Marine Protected Area (MPA), developed by the Commission for the Conservation of Antarctic Marine Living Resources, was designed for the ongoing protection of a subregion within the RSfEAR (Brooks et al., 2021). From 2027, the MPA will undergo formal decadal reviews of its efficacy toward meeting its specific objectives based on cross-disciplinary observations (Brooks et al., 2021).
These strategies are unanimous in identifying the lack of data as being the leading issue due to remoteness, harshness, and scale. Collectively, the major research gaps identified are consistent. Sea-ice and oceanic connections sit centrally in these gap analyses, with implications for sea-level rise, thermohaline evolution, ecosystem functions and survivability, and operational considerations.
3 Knowledge gaps
These motivations raise the following questions: a) Are we able to sufficiently describe the present sea-ice–ocean system in the RSfEAR? b) What are the connections between the Ross and far East Antarctic sectors? c) What are the key cross-disciplinary transfers connecting physics to biodiversity and ecosystem outcomes? d) What are the future projected trajectories for the critical components of the RSfEAR ocean–sea ice–atmosphere system?
3.1 Present-day ocean–sea ice–atmosphere system in the RSfEAR
There is a general lack of process knowledge for the RSfEAR other than the identified focal areas. Beyond process understanding, there is also a general paucity of sustained Southern Ocean/Antarctic observing especially in the RSfEAR, where few sustained sea-ice/ocean observing programs exist contrary to the West Antarctic. Antarctic sea ice is very challenging to observe. It spreads over a very large area with regional variability, yet it is annual and largely disappears every year. This is an impediment to any ocean observing technique. A lack of understanding of the role of snow in modulating ocean–sea ice–atmosphere processes further complicates the situation.
There are a number of environment categories that the proposed RSfEAR observing system needs to encapsulate, including polynya, fast ice, MIZ, and open ocean (Figure 1B). Furthermore, boundary conditions need to be identified for the Southern Ocean, ice-shelf margins, glacial ice tongues, and terrestrial boundaries. Stratification and mixing rate data, especially for the upper ocean, on the continental shelf (Porter et al., 2019) and near the coast (Yoon et al., 2020) are limited. Autonomous observing systems would ameliorate this although profiling floats are presently limited by their inability to surface through sea-ice cover (Porter et al., 2019; Oke et al., 2022). Enhanced designs and analysis improve these limitations where feasible. There is the potential to expand the acquisition of essential climate variables (Lavergne et al., 2022) in order to raise awareness of the breadth of climate and ecosystem processes and interactions that pertain to the RSfEAR.
Other observations rely on interdisciplinary field campaigns to fill fundamental knowledge gaps, i.e., of atmospheric processes and links to the ocean, sea ice, and biology (Mallet et al., 2023). Field-based research in the RSfEAR region will address these, including pathways and characteristics of aerosols, clouds, and precipitation near coastal Antarctica, which differ substantially from those in the warmer Southern Ocean to the north (Mace et al., 2021).
3.2 Connections between the Ross and far East Antarctic sectors
The RSfEAR geographical focus highlights the connection between regional change in West and East Antarctica. Recent overview sea-ice and ocean studies in the region include work on ocean salinity (Castagno et al., 2019), sea-ice deformation (Kousal et al., 2022), and ice–wave interaction (Horvat and Roach, 2022). However, there have been disconnects, both geographically and in terms of discipline. Geographically, there has been a separation between Ross Sea and far East Antarctic sectors due to the limited overlap in foci of the various national programs. Furthermore, as with many aspects of sea-ice and ocean science, disciplinary boundaries have constrained advances in what needs to be highly integrated research.
3.3 Key cross-disciplinary transfers
Bringing together a range of disciplines and scales is clearly required (Gutt et al., 2018). Evidence for the nature of tightly coupled ice–ocean biophysics is emerging through Argo float-based sampling with under-ice positioning methods as well as enhanced biogeochemical sensors (Hague and Vichi, 2021) developed through the Southern Ocean Carbon and Climate Observations and Modeling project. These initiatives improved the quantification of the timing and strength of phytoplankton blooms relative to local sea-ice structure (Figure 1B).
3.4 Future trajectories
There also needs to be an activity supporting the understanding of a future, largely ice-free RSfEAR. While this is likely primarily through modeling, observational approaches underpinning these projections will be affected by changing conditions that will largely be outside recent direct experience. Studies in the Arctic emphasize the regional variability in sea-ice coverage and wider impacts (Årthun et al., 2021). Gutt et al. (2018) also identify the need to understand potential future trajectories for the sea ice–ocean system. This requires continued effort around modeling tools and underpinning data streams. Notably, projections are typically at large scales relative to biophysical observations and processes, calling for down-/upscaling of both biophysical quantities and processes.
4 Future directions and implementation
Historical regional foci have resulted in a disconnect between the eastern and western RSfEAR sectors. While coincident case studies (e.g., SIPEX and SIMBA) sought to overcome this, only sustained distributed observatories can systematically address this. This review provides motivation for a system design rethink focused on the RSfEAR. Figure 1A shows the suggested locations for data-informed sentinel sites, an observing program, and key process studies, all in a time of great technological advances with remote sensing, robotics, and enhanced sensor capabilities. This review provides motivation for a system design rethink focused on the RSfEAR. A framework for observations and process studies (Figure 2A) should provide a consistent, sustainable time series of EOVs, as well as other regionally relevant quantities, from locations that provide the best sentinel conditions (Figure 1A).
The pathway to high-latitude observing system design is described in Newman et al. (2019) with options for components for optimal results. This SOOS program overview highlights the benefits of observing system design methods, the need and benefit for international coordination, and the potential for new technologies to revolutionize data pipelines from acquisition to delivery. The RSfEAR Integrated Observing System would directly deliver results to SOOS and downstream users and build on FAIR data principles (Wilkinson et al., 2016).
This review seeks to build on existing and ongoing activities to generate a sustained, resilient, adaptive RSfEAR observing system. Emerging in many stakeholder plans is a focus on the human dimension of the activity including justice, diversity, equity, and inclusivity both in terms of who is doing the work and to whom the benefits are aimed at (Meyer-Gutbrod et al., 2023). In conjunction with supporting the people enabling the processes, key observing system components should be built around the following:
4.1 Ocean
a. Hydrographic moorings and transects at key locations in RSfEAR (Figure 1A) to i) continue records and ii) provide a basis for sector connection (Bowen et al., 2021). Recommendation: Maintain a. (continue) key hydrographic time series stations at locations such as Ross Polynya, TNB, Cape Adare, and Mertz to maintain a continual presence, preferably augmented by annual transects for hydrographic and particle flux measurements as well as meteorological data.
b. Enhance the nascent BioGeoChemical (BGC) float network (Figure 1A). This presently encounters challenges, i.e., sea-ice cover and the relatively shallow continental shelf (Schallenberg et al., 2022).
4.2 Sea ice
a. Improved remotely sensed metrics including essential climate variables (Lavergne et al., 2022) and derived sea-ice drift. Recommendation: Obtain high-resolution satellite imagery to generate improved estimates of coastal ice drift and deformation (Farooq et al., 2020).
b. Improve technology for ice and snow freeboard detection as a proxy for thickness (Kacimi and Kwok, 2020; Tan et al., 2021). Recommendation: Deploy upward-looking sonars from drifting buoys and autonomous underwater vehicles (Williams et al., 2015) to derive ice draft and airborne transects 1a for ice and snow thickness and the sub-ice platelet layer (Rack et al., 2021; Langhorne et al., 2023).
c. Develop improved understanding and data streams of biological productivity and associated sea-ice, ocean, and atmosphere behaviors. Recommendation: Integrate biophysical sentinel sites 1a with ongoing sea-ice, upper ocean, and atmospheric observing and process studies (Cummings et al., 2019; Mallet et al., 2023).
4.3 Integration
a. Integration of observations with regional-scale coupled modeling (Malyarenko et al., 2022). Recommendation: Extend physical models to include biogeochemical processes and higher tropic level outcomes (Cavanagh et al., 2021) and to better elucidate connectivity across the RSfEAR.
b. Implementation of advanced data techniques, including the use of machine learning (Horvat and Roach, 2022) to project future sea-ice coverage in the RSfEAR. Recommendation: Produce and promote regular updates on ice–ocean metrics for RSfEAR, potentially as EOVs.
Clearly, there is a significant element of aspiration, and continuing effort will be required to develop and maintain these resources. However, as a counter, this work is overlaid by a sense of urgency from most stakeholder viewpoints around Antarctic sea ice in the coming decades. A range of studies, as well as the policy documents listed here, point to the next few years as critical for determining future conditions for one of the least understood regions of the Earth system (Holmes et al., 2022; Stokes et al., 2022).
Author contributions
PH, CS, and CE developed the concept and led the synthesis and writing. All the other authors contributed to the review, writing, and future emphases. All authors contributed to the article and approved the submitted version.
Funding
PH and SA received support through the Australian Antarctic Science Projects 4496, 4506, and 4625, and PH also received support through the International Space Science Institute (Bern, Switzerland) project #405. Aotearoa New Zealand contributions (CS and WR) are supported by the MBIE Antarctic Science Platform (ANTA1801). WL and CE were supported by a Korea Institute of Marine Science and Technology Promotion (KIMST) grant funded by the Ministry of Oceans and Fisheries (RS-2023-00256677; PM23020). HS was supported by KOPRI project PE23110 funded through Korea Polar Research Institute by the Korean Ministry of Oceans and Fisheries.
Acknowledgments
The ideas in this minireview are informed by a number of project teams as well as the Southern Ocean Observing System (SOOS), SCAR AntClimNow (Near-term Variability and Prediction of the Antarctic Climate System) Scientific Research Programme, and Global Cryosphere Watch’s CryoNet. The authors wish to thank two reviewers and also acknowledge Denise Fernandez, Natalie Robinson, and Sean Chua for contributions and discussions supporting this minireview. This work contributes to Projects 1 and 6 of the Australian Antarctic Program Partnership (ASCI000002) funded under the Australian Government’s Antarctic Science Collaboration Initiative program. Sea-ice concentrations for September 2022 have been obtained from https://seaice.unibremen.de/dataarchive/ (Spreen et al., 2008).
Conflict of interest
The authors declare that the research was conducted in the absence of any commercial or financial relationships that could be construed as a potential conflict of interest.
Publisher’s note
All claims expressed in this article are solely those of the authors and do not necessarily represent those of their affiliated organizations, or those of the publisher, the editors and the reviewers. Any product that may be evaluated in this article, or claim that may be made by its manufacturer, is not guaranteed or endorsed by the publisher.
References
Adusumilli S., Fricker H. A., Medley B., Padman L., Siegfried M. R. (2020). Interannual variations in meltwater input to the southern ocean from antarctic ice shelves. Nat. Geosci. 13, 616–620. doi: 10.1038/s41561-020-0616-z
Arrigo K. R., van Dijken G. L. (2003). Phytoplankton dynamics within 37 Antarctic coastal polynya systems. J. Geophys. Res. 108, 3271–3271. doi: 10.1029/2002JC001739
Arrigo K. R., Van Dijken G. L., Strong A. L. (2015). Environmental controls of marine productivity hot spots around Antarctica. J. Geophys. Res.: Oceans 120, 5545–5565. doi: 10.1002/2015JC010888
Årthun M., Onarheim I. H., Dörr J., Eldevik T. (2021). The seasonal and regional transition to an ice-free Arctic. Geophys. Res. Lett. 48, e2020GL090825. doi: 10.1029/2020GL090825
Ayres H. C., Screen J. A., Blockley E. W., Bracegirdle T. J. (2022). The coupled atmosphere–ocean response to antarctic sea ice loss. J. Climate 35, 4665–4685. doi: 10.1175/JCLI-D-21-0918.1
Barber D. G., Massom R. A. (2007). The role of sea ice in arctic and antarctic polynyas. Elsevier oceanogr. Ser. 74, 1–54. doi: 10.1016/S0422-9894(06)74001-6
Barbraud C., Delord K., Weimerskirch H. (2015). Extreme ecological response of a seabird community to unprecedented sea ice cover. R. Soc. Open Sci. 2, 140456. doi: 10.1098/rsos.140456
Bell R. E., Chu W., Kingslake J., Das I., Tedesco M., Tinto K. J., et al. (2017). Antarctic ice shelf potentially stabilized by export of meltwater in surface river. Nature 544, 344–348. doi: 10.1038/nature22048
Bennetts L. G., Bitz C. M., Feltham D. L., Kohout A. L., Meylan M. H. (2022). Marginal ice zone dynamics: Future research perspectives and pathways. Philos. Trans. R. Soc. A: Mathematical Phys. Eng. Sci. 380, 20210267. doi: 10.1098/rsta.2021.0267
Bowen M. M., Fernandez D., Forcen-Vazquez A., Gordon A. L., Huber B., Castagno P., et al. (2021). The role of tides in bottom water export from the western Ross Sea. Sci. Rep. 11, 1–11. doi: 10.1038/s41598-021-81793-5
Bracci A., Baldini L., Roberto N., Adirosi E., Montopoli M., Scarchilli C., et al. (2022). “Evidence of sublimation in the vertical profiles of radar reflectivity and its impact on snowfall estimation at the ground at mario zucchelli antarctic station,” in 2022 3rd URSI Atlantic and Asia Pacific Radio Science Meeting (AT-AP-RASC). 1–4. doi: 10.23919/AT-AP-RASC54737.2022.9814266
Brooks C. M., Bloom E., Kavanagh A., Nocito E. S., Watters G. M., Weller J. (2021). The ross sea, Antarctica: A highly protected mpa in international waters. Mar. Policy 134, 104795. doi: 10.1016/j.marpol.2021.104795
Castagno P., Capozzi V., DiTullio G. R., Falco P., Fusco G., Rintoul S. R., et al. (2019). Rebound of shelf water salinity in the ross sea. Nat. Commun. 10, 5441. doi: 10.1038/s41467-019-13083-8
Cavanagh R. D., Trathan P. N., Hill S. L., Melbourne-Thomas J., Meredith M. P., Hollyman P., et al. (2021). Utilising ipcc assessments to support the ecosystem approach to fisheries management within a warming southern ocean. Mar. Policy 131, 104589. doi: 10.1016/j.marpol.2021.104589
Clark G. F., Stark J. S., Palmer A. S., Riddle M. J., Johnston E. L. (2017). The roles of sea-ice, light and sedimentation in structuring shallow Antarctic benthic communities. PloS One 12, e0168391. doi: 10.1371/journal.pone.0168391
Comiso J. C., Kwok R., Martin S., Gordon A. L. (2011). Variability and trends in sea ice extent and ice production in the Ross Sea. J. Geophys. Res.: Oceans 116, C04021. doi: 10.1029/2010JC006391
Cummings V. J., Barr N. G., Budd R. G., Marriott P. M., Safi K. A., Lohrer A. M. (2019). In situ response of antarctic under-ice primary producers to experimentally altered ph. Sci. Rep. 9, 6069. doi: 10.1038/s41598-019-42329-0
DCCEEW (2022) Australian Antarctic strategy and 20 year action plan: Updated may 2022 (Accessed March 31, 2023).
Farooq U., Rack W., McDonald A., Howell S. (2020). Long-term analysis of sea ice drift in the western ross sea, Antarctica, at high and low spatial resolution. Remote Sens. 12, 1402. doi: 10.3390/rs12091402
Gloersen P. (1995). Modulation of hemispheric sea-ice cover by ENSO events. Nature 373, 503–506. doi: 10.1038/373503a0
Grazioli J., Madeleine J.-B., Gallee H., Forbes R. M., Genthon C., Krinner G., et al. (2017). Katabatic winds diminish precipitation contribution to the antarctic ice mass balance. Proc. Natl. Acad. Sci. 114, 10858–10863. doi: 10.1073/pnas.1707633114
Gunn K. L., Rintoul S. R., England M. H., Bowen M. M. (2023). Recent reduced abyssal overturning and ventilation in the Australian antarctic basin. Nat. Climate Change 13, 1–8. doi: 10.1038/s41558-023-01667-8
Gutt J., Isla E., Bertler A., Bodeker G. E., Bracegirdle T. J., Cavanagh R. D., et al. (2018). Crossdisciplinarity in the advance of antarctic ecosystem research. Mar. Genomics 37, 1–17. doi: 10.1016/j.margen.2017.09.006
Hague M., Vichi M. (2021). Southern Ocean biogeochemical Argo detect under-ice phytoplankton growth before sea ice retreat. Biogeosciences 18, 25–38. doi: 10.5194/bg-18-25-2021
Hobbs W. R., Massom R., Stammerjohn S., Reid P. P. P., Williams G. D., Meier W. (2016). A review of recent changes in Southern Ocean sea ice, their drivers and forcings. Global Planetary Change 143, 228–250. doi: 10.1016/j.gloplacha.2016.06.008
Holmes C., Bracegirdle T., Holland P. (2022). Antarctic sea ice projections constrained by historical ice cover and future global temperature change. Geophys. Res. Lett. 49, e2021GL097413. doi: 10.1029/2021GL097413
Hoppmann M., Richter M. E., Smith I. J., Jendersie S., Langhorne P. J., Thomas D. N., et al. (2020). Platelet ice, the Southern Ocean’s hidden ice: a review. Ann. Glaciol. 61, 341–368. doi: 10.1017/aog.2020.54
Horvat C., Roach L. A. (2022). Wiff1. 0: a hybrid machine-learning-based parameterization of wave-induced sea ice floe fracture. Geoscientific Model. Dev. 15, 803–814. doi: 10.5194/gmd-15-803-2022
Jacobs S., Giulivi C., Dutrieux P. (2022). Persistent Ross Sea freshening from imbalance West Antarctic ice shelf melting. J. Geophys. Res.: Oceans 127, e2021JC017808. doi: 10.1029/2021JC017808
Janssen A., Badhe R., Bransome N., Bricher P., Cavanagh R., De Bruin T., et al. (2022). Southern Ocean Action plan 2021-2030: In support of the United Nations Decade of Ocean Science for Sustainable Development. Available at: https://zenodo.org/record/6412191.
Kacimi S., Kwok R. (2020). The Antarctic sea ice cover from ICESat-2 and CryoSat-2: Freeboard, snow depth, and ice thickness. Cryosphere 14, 4453–4474. doi: 10.5194/tc-14-4453-2020
Kennicutt M. C., Chown S. L., Cassano J. J., Liggett D., Peck L. S., Massom R., et al. (2015). A roadmap for antarctic and southern ocean science for the next two decades and beyond. Antarctic Sci. 27, 3–18. doi: 10.1017/S0954102014000674
Knuth S. L., Cassano J. J. (2014). Estimating sensible and latent heat fluxes using the integral method from in situ aircraft measurements. J. Atmospheric Oceanic Technol. 31, 1964–1981. doi: 10.1175/JTECH-D-14-00008.1
Kousal J., Voermans J., Liu W., Heil P., Babanin A. V. (2022). A two-part model for wave-sea ice interaction: attenuation and break-up. J. Geophys. Res 127, e2022JC018571. doi: 10.1029/2022JC018571
Kwok R., Comiso J. C. (2002). Spatial patterns of variability in Antarctic surface temperature: Connections to the Southern Hemisphere Annular Mode and the Southern Oscillation. Geophys. Res. Lett. 29, 1705–1705. doi: 10.1029/2002GL015415
Langhorne P. J., Haas C., Price D., Rack W., Leonard G. H., Brett G. M., et al. (2023). Fast ice thickness distribution in the western ross sea in late spring. J. Geophys. Res.: Oceans 128. doi: 10.1029/2022JC019459
Langhorne P., Hughes K., Gough A., Smith I., Williams M., Robinson N., et al. (2015). Observed platelet ice distributions in Antarctic sea ice: An index for ocean-ice shelf heat flux. Geophys. Res. Lett. 42, 5442–5451. doi: 10.1002/2015GL064508
Lavergne T., Kern S., Aaboe S., Derby L., Dybkjaer G., Garric G., et al. (2022). A new structure for the sea ice essential climate variables of the global climate observing system. Bull. Am. Meteorol. Soc. 103, E1502–E1521. doi: 10.1175/BAMS-D-21-0227.1
Li Q., England M. H., Hogg A. M., Rintoul S. R., Morrison A. K. (2023). Abyssal ocean overturning slowdown and warming driven by antarctic meltwater. Nature 615, 841–847. doi: 10.1038/s41586-023-05762-w
Lubin D., Zhang D., Silber I., Scott R. C., Kalogeras P., Battaglia A., et al. (2020). AWARE: the atmospheric radiation measurement (ARM) west Antarctic radiation experiment. Bull. Am. Meteorol. Soc. 101, E1069–E1091. doi: 10.1175/BAMS-D-18-0278.1
Ma L., Qin D. (2018). “Cryospheric science needs to provide higher-quality services in the context of the new climate normals,” in Annual report on actions to address climate change, (2018) (Springer, Singapore), 239–249.
Mace G. G., Bensonand S R., Humphries R., Gombert P. M., Sterner E. (2023). Natural marine cloud brightening in the southern ocean. Atmos. Chem. Phys. 23, 1677–1685. doi: 10.5194/acp-23-1677-2023
Mace G. G., Protat A., Humphries R. S., Alexander S. P., McRobert I. M., Ward J., et al. (2021). Southern ocean cloud properties derived from capricorn and marcus data. J. Geophys. Res. Atmos. 126. doi: 10.1029/2020jd033368
Mallet M. D., Humphries R. S., Fiddes S. L., Alexander S. P., Altieri K., Angot H., et al. (2023). Untangling the influence of antarctic and southern ocean life on clouds. Elementa: Sci. Anthropocene 11. doi: 10.1525/elementa.2022.00130.00130
Malyarenko A., Gossart A., Sun R., Krapp M. (2023). Conservation of heat and mass in p-skrips version 1: the coupled atmosphere-ice-ocean model of the ross sea. Geosci. Model Dev. 16, 3355–3373. doi: 10.5194/gmd-16-3355-2023
Matear R. J., O’Kane T. J., Risbey J. S., Chamberlain M. (2015). Sources of heterogeneous variability and trends in Antarctic sea-ice. Nat. Commun. 6, 8656–8656. doi: 10.1038/ncomms9656
McFarquhar G. M., Bretherton C. S., Marchand R., Protat A., DeMott P. J., Alexander S. A., et al. (2021). Observations of clouds, aerosols, precipitation, and surface radiation over the southern ocean: An overview of capricorn, marcus, micre, and socrates. Bull. Am. Meteorol. Soc. 102, E894–E928. doi: 10.1175/BAMS-D-20-0132.1
Meyer-Gutbrod E. L., Pierson J. J., Behl M. (2023). Community perspectives on justice, equity, diversity, and inclusion in ocean sciences. Oceanography 36, 67–73. doi: 10.5670/oceanog.2023.106
MFAT (2021). Aotearoa New Zealand Antarctic and Southern Ocean research directions and priorities. Ministry for Foreign Affairs and Trade, 17. Available at: https://www.mfat.govt.nz/en/environment/antarctica-and-the-southern-ocean/aotearoa-new-zealand-antarctic-research-directions-and-priorities.
Miles B. W., Stokes C. R., Jamieson S. S. (2018). Velocity increases at cook glacier, east Antarctica, linked to ice shelf loss and a subglacial flood event. Cryosphere 12, 3123–3136. doi: 10.5194/tc-12-3123-2018
Montiel F., Kohout A. L., Roach L. A. (2022). Physical drivers of ocean wave attenuation in the marginal ice zone. J. Phys. Oceanogr. 52, 889–906. doi: 10.1175/JPO-D-21-0240.1
Newman L., Heil P., Trebilco R., Katsumata K., Constable A., Van Wijk E., et al. (2019). Delivering sustained, coordinated, and integrated observations of the Southern Ocean for global impact. Front. Mar. Sci. 6, 433. doi: 10.3389/fmars.2019.00433
Oke P. R., Rykova T., Pilo G. S., Lovell J. L. (2022). Estimating Argo float trajectories under ice. Earth Space Sci. 9, e2022EA002312. doi: 10.1029/2022EA002312
Parkinson C. L. (2019). A 40-y record reveals gradual Antarctic sea ice increases followed by decreases at rates far exceeding the rates seen in the Arctic. Proc. Natl. Acad. Sci. 116, 14414–14423. doi: 10.1073/pnas.1906556116
Porter D. F., Springer S. R., Padman L., Fricker H. A., Tinto K. J., Riser S. C., et al. (2019). Evolution of the seasonal surface mixed layer of the Ross Sea, Antarctica, observed with autonomous profiling floats. J. Geophys. Res.: Oceans 124, 4934–4953. doi: 10.1029/2018JC014683
Rack W., Price D., Haas C., Langhorne P. J., Leonard G. H. (2021). Sea ice thickness in the Western Ross Sea. Geophys. Res. Lett. 48, e2020GL090866. doi: 10.1029/2020GL090866
Raphael M. N., Handcock M. S. (2022). A new record minimum for antarctic sea ice. Nat. Rev. Earth Environ. 3, 215–216. doi: 10.1038/s43017-022-00281-0
Reid P. A., Massom R. A. (2022). Change and variability in Antarctic coastal exposure 1979–2020. Nat. Commun. 13, 1164. doi: 10.1038/s41467-022-28676-z
Sansiviero M., Maqueda M. M., Fusco G., Aulicino G., Flocco D., Budillon G. (2017). Modelling sea ice formation in the Terra Nova Bay polynya. J. Mar. Syst. 166, 4–25. doi: 10.1016/j.jmarsys.2016.06.013
Schallenberg C., Strzepek R. F., Bestley S., Wojtasiewicz B., Trull T. W. (2022). Iron limitation drives the globally extreme fluorescence/chlorophyll ratios of the southern ocean. Geophys. Res. Lett. 49, e2021GL097616. doi: 10.1029/2021GL097616
Silvano A., Foppert A., Rintoul S. R., Holland P. R., Tamura T., Kimura N., et al. (2020). Recent recovery of Antarctic bottom water formation in the Ross Sea driven by climate anomalies. Nat. Geosci. 13, 780–786. doi: 10.1038/s41561-020-00655-3
Simmonds I., Jacka T. H. (1995). Relationships between the interannual variability of antarctic sea ice and the Southern oscillation. Am. Meteorological Soc. 8, 637–647. doi: 10.1175/1520-0442(1995)008<0637:RBTIVO>2.0.CO;2
Simpkins G. R., Ciasto L. M., David. W. J., England M. H. (2012). Seasonal relationships between large-scale climate variability and antarctic sea ice concentration. J. Climate 25, 5451–5469. doi: 10.1175/JCLI-D-11-00367.1
Spreen G., Kaleschke L., Heygster G. (2008). Sea ice remote sensing using amsr-e 89 ghz channels. J. Geophys. Res. 113, C02503. doi: 10.1029/2005JC003384
Squire V. A. (2022). A prognosticative synopsis of contemporary marginal ice zone research. Philos. Trans. R. Soc. A: Mathematical Phys. Eng. Sci. 380, 20220094. doi: 10.1098/rsta.2022.0094
Stammerjohn S., Massom R., Rind D., Martinson D. (2012). Regions of rapid sea ice change: An inter-hemispheric seasonal comparison. Geophys. Res. Lett. 39, L06501–L06501. doi: 10.1029/2012GL050874
Stevens C., Robinson N., O’Connor G., Grant B. (2023). Ocean turbulent boundary-layer influence on ice crystal behaviour beneath fast ice in an Antarctic ice shelf water plume: The” dirty ice”. Front. Mar. Sci. 10. doi: 10.3389/fmars.2023.1103740
Stokes C. R., Abram N. J., Bentley M. J., Edwards T. L., England M. H., Foppert A., et al. (2022). Response of the east antarctic ice sheet to past and future climate change. Nature 608, 275–286. doi: 10.1038/s41586-022-04946-0
Stuecker M. F., Bitz C. M., Armour K. C. (2017). Conditions leading to the unprecedented low Antarctic sea ice extent during the 2016 austral spring season. Geophys. Res. Lett. 44, 9008–9019. doi: 10.1002/2017GL074691
Swadling K. M., Constable A. J., Fraser A. D., Massom R. A., Borup M. D., Ghigliotti L., et al. (2023). Biological responses to change in Antarctic sea ice habitats. Front. Ecol. Evol. 10. doi: 10.3389/fevo.2022.1073823
Swart S., Gille S. T., Delille B., Josey S., Mazloff M., Newman L., et al. (2019). Constraining southern ocean air-sea-ice fluxes through enhanced observations. Front. Mar. Sci. 6. doi: 10.3389/fmars.2019.00421
Tamura T., Ohshima K. I., Fraser A. D., Williams G. D. (2016). Sea ice production variability in Antarctic coastal polynyas. J. Geophys. Res.: Oceans 121, 2967–2979. doi: 10.1002/2015JC011537
Tamura T., Ohshima K. I., Nihashi S. (2008). Mapping of sea ice production for Antarctic coastal polynyas. Geophys. Res. Lett. 35, 1–5. doi: 10.1029/2007GL032903
Tamura T., Williams G., Fraser A., Ohshima K. (2012). Potential regime shift in decreased sea ice production after the Mertz Glacier calving. Nat. Commun. 3, 1–6. doi: 10.1038/ncomms1820
Tan A. E.-C., McCulloch J., Rack W., Platt I., Woodhead I. (2021). Radar measurements of snow depth over sea ice on an unmanned aerial vehicle. IEEE Trans. Geosci. Remote Sens. 59, 1868–1875. doi: 10.1109/TGRS.2020.3006182
Turner J. (2004). The el niño-southern oscillation and Antarctica. Int. J. Climatol. 24, 1–31. doi: 10.1002/joc.965
Turner J., Holmes C., Harrison T. C., Phillips T., Jena B., Reeves-Francois T., et al. (2022). Record low Antarctic sea ice cover in February 2022. Geophys. Res. Lett. 39, e2022GL098904. doi: 10.1029/2022GL098904
Vignon E., Picard G., Duran-Alarcon G., Alexander S. P., Gallee H., Berne A. (2020). Gravity wave excitation during the coastal transition of an extreme katabatic flow in Antarctica. J. Atmospheric Sci. 77, 1295–1312. doi: 10.1175/JAS-D-19-0264.1
Voermans J., Liu Q., Marchenko A., Rabault J., Filchuk K., Ryzhov I., et al. (2021). Wave dispersion and dissipation in landfast ice: comparison of observations against models. Cryosphere 15, 5557–5575. doi: 10.5194/tc-15-5557-2021
Wilkinson M. D., Dumontier M., Aalbersberg I. J., Appleton G., Axton M., Baak A., et al. (2016). The FAIR Guiding Principles for scientific data management and stewardship. Sci. Data 3, 373–388. doi: 10.1038/sdata.2016.18
Williams G., Maksym T., Wilkinson J., Kunz C., Murphy C., Kimball P., et al. (2015). Thick and deformed Antarctic sea ice mapped with autonomous underwater vehicles. Nat. Geosci. 8, 61–67. doi: 10.1038/ngeo2299
World-Meteorological-Organization (2022) 5th international polar year (ipy) 2032-33 (Accessed April 11, 2023).
Yoon S.-T., Lee W. S., Stevens C., Jendersie S., Nam S., Yun S., et al. (2020). Variability in high-salinity shelf water production in the Terra Nova Bay polynya, Antarctica. Ocean Sci. 16, 373–388. doi: 10.5194/os-16-373-2020
Yuan X. (2004). ENSO-related impacts on Antarctic sea ice: A synthesis of phenomenon and mechanisms. Antarctic Sci. 16, 415–425. doi: 10.1017/S0954102004002238
Zhang L., Delworth T. L., Yang X., Zeng F., Lu F., Morioka Y., et al. (2022). The relative role of the subsurface Southern Ocean in driving negative Antarctic sea ice extent anomalies in 2016–2021. Commun. Earth Environ. 3, 1–9. doi: 10.1038/s43247-022-00624-1
Keywords: Antarctic Earth-system science, Ross Sea-far East Antarctic Region, cross-disciplinary observing system, sea ice, hydrography, biogeochemistry, atmosphere, coastal processes
Citation: Heil P, Stevens C, Lee WS, Eayrs C, Shin HC, Alexander SP and Rack W (2023) Bridging the gap for ice–ocean–ecosystem processes: integrated observing system for the Ross Sea-far East Antarctic Region. Front. Mar. Sci. 10:1206119. doi: 10.3389/fmars.2023.1206119
Received: 14 April 2023; Accepted: 11 September 2023;
Published: 03 October 2023.
Edited by:
David Docquier, Royal Meteorological Institute of Belgium, BelgiumReviewed by:
Alice DuVivier, National Center for Atmospheric Research (UCAR), United StatesBianca Mezzina, Université Catholique de Louvain, Belgium
Copyright © 2023 Heil, Stevens, Lee, Eayrs, Shin, Alexander and Rack. This is an open-access article distributed under the terms of the Creative Commons Attribution License (CC BY). The use, distribution or reproduction in other forums is permitted, provided the original author(s) and the copyright owner(s) are credited and that the original publication in this journal is cited, in accordance with accepted academic practice. No use, distribution or reproduction is permitted which does not comply with these terms.
*Correspondence: Petra Heil, cGV0cmEuaGVpbEB1dGFzLmVkdS5hdQ==