- 1Key Laboratory of Marine Eco-Environmental Science and Technology, First Institute of Oceanography, Ministry of Natural Resources, Qingdao, China
- 2Laboratory of Marine Ecology and Environmental Science, National Laboratory for Marine Science and Technology, Qingdao, China
- 3CAS Key Laboratory of Marine Ecology and Environmental Sciences, Institute of Oceanology, Chinese Academy of Sciences, Qingdao, China
- 4Marine and Island Management Department, North China Sea Bureau of the Ministry of Natural Resources, Qingdao, China
Introduction: Sea cucumbers are cultivated mainly for their valuable body wall. Polybrominated diphenyl ethers are common persistent pollutants in sea waters with known impacts on aquatic animals nonetheless not yet studied for the body wall of sea cucumbers.
Methods: Using ltra-high performance liquid chromatography-quadrupole time-of-flight mass spectrometry (UPLC-Triple-TOF-MS), we investigated the metabolic impact of 2,2',4,4'-tetrabromodiphenyl ether (BDE-47) on the body wall of Apostichopus japonicus. etabolite changes and metabolic pathway alterations were assessed in response to three distinct concentrations of BDE-47: 0.1 µg/L, 1.0 µg/L, and 10.0 µg/L.
REsults: Exposure to BDE-47 led to notable alterations in the metabolic profiles of the body wall. A total of 95~102 metabolites in the 0.1 ~ 10.0 µg/L BDE-47 treated group were altered significantly, and various disrupted metabolic pathways were identified and characterized. These metabolites and metabolic pathways were mainly involved in lipid metabolism, energy metabolism, immunity, oxidative stress, inflammation, and neurotoxicity.
Discussion: The findings of our study shed light on the potential health risks that polybrominated diphenyl ethers present to sea cucumbers. This underscores the imperative for both researchers and policymakers to delve deeper into further investigations and studies. These results indicate the necessity for enhanced monitoring and management practices within the sea cucumber aquaculture industry to mitigate the impact of these persistent organic pollutants and protect the health and safety of this valuable resource.
Introduction
The sea cucumber Apostichopus japonicus (Selenka) is widely distributed along the coasts of the Pacific Northwest (Yang et al., 2015a) and is the primary commercial echinoderm species in China, Japan, Korea, and Russia (Sloan, 2020). Its body wall is soft and elastic, composed primarily of mutable collagenous tissue (Saito et al., 2002). The stiffness and extensibility of the tissue can be rapidly altered under nervous control (Wilkie, 2005; Mo et al., 2016). As a traditional tonic, the sea cucumber’s body wall is rich in collagen and mucopolysaccharides, exhibiting various bioactivities such as antioxidation, anti-inflammation, antimicrobial properties, gastric protection, anti-diabetes, and anticancer effects (Wang Y. et al., 2020; Xing et al., 2021). Due to its nutritional and medicinal value, the trade and consumption of A. japonicus have expanded globally (Duan et al., 2010), leading to a significant increase in aquaculture of this species over the past few decades (Sun et al., 2022). According to the recently published China Fishery Statistical Yearbook 2022, the total marine aquaculture area for sea cucumbers in China in 2021 was 247,419 hectares, with an output of 222,707 tons.
Polybrominated diphenyl ethers (PBDEs) are a class of brominated flame retardants (BFRs) known for their excellent thermostability, which has led to their use in reducing fire risks in appliances, building materials, and other applications (Jakobsson et al., 2002; Zhao et al., 2022). Generally characterized by long degradation cycles, strong lipid solubility, and bioaccumulation (Lorber, 2008), PBDEs have been detected extensively in both the environment and living organisms, raising concerns about contamination (Hayakawa et al., 2004; Herzke et al., 2005; Ramu et al., 2005). Among the 209 PBDE homologs, 2,2’,4,4’-tetrabromodiphenyl ether (BDE-47) is a new type of persistent organic pollutant with a higher detection rate in environmental and biological samples (Ikonomou et al., 2002; Lema et al., 2007; Byer et al., 2013). Previous studies reported that concentrations of BDE-47 in Jiaozhou Bay, China, reached up to 183.5 pg/L, with sediment and marine organism content as high as 5.50 ng/g and 29.75 ng/g, respectively (Jin et al., 2008; Zhou et al., 2010; Liu et al., 2014). In addition, Laizhou Bay and the mouth of the Yellow River are high accumulation zones for BDE-47. Laizhou Bay’s nearshore areas have a high density of brominated flame retardant production facilities, and sediment samples from Laizhou Bay have shown ranges of BDE-47 from 0.06 to 0.28 ng·g-1 dw (Jin et al., 2008). The potential exposure pathways of BDE-47 in sea cucumbers during aquaculture can include waterborne exposure through direct contact with contaminated water, uptake of contaminated sediment or food particles, and bioaccumulation through the food chain. Several studies have confirmed the widespread presence of BDE-47 in various types of benthic organisms from different regions in China, highlighting differences in concentrations among species, habitats, and locations (Zhou et al., 2008; Qiu et al., 2009; Zheng et al., 2011).
BDE-47 exhibits stronger toxicity and bioaccumulation than other homologs, which increases the potential risks to ecological security and biological health (Zhou et al., 2010; Geng et al., 2022). Although the impact of BDE-47 on mammals, fish, and crustaceans has been studied (Van de Merwe et al., 2011; Glazer et al., 2017; Rajput et al., 2021; Geng et al., 2022), our knowledge of its effect(s) on animal physiology remains insufficiently understood, particularly for marine-culture animals. Several studies have examined the effects of BDE-47 on marine animals, particularly benthic organisms. For example, research on benthic organisms such as mussels (Ji et al., 2013a; Messina et al., 2020) and earthworms (Ji et al., 2013c) has shown that BDE-47 exposure can lead to oxidative stress, endocrine disruption, immune system dysfunction, and alterations in metabolic pathways. Given the higher detection rate of BDE-47 in marine water samples from aquaculture zones (Zhou et al., 2010) and the fact that the body wall is the primary edible part of sea cucumbers, assessing the influence of BDE-47 on the body wall is an urgent necessity for ensuring the healthy development of the sea cucumber industry.
Metabolomics is an effective method for accurately detecting all small metabolites in animals, tissues, or cells subjected to specific biological perturbations (Shockcor and Holmes, 2002; Nicholson, 2006; Ding et al., 2021). This technology has been widely applied to identify promising biomarkers and potential mechanisms related to various environmental pollutants (He et al., 2020; Li et al., 2020a; Yang et al., 2018; Scoville et al., 2019). In this study, ultra-high performance liquid chromatography-quadrupole time-of-flight mass spectrometry (UPLC-Triple-TOF-MS) was employed to assess the changes in metabolites and metabolic pathways in the body wall of sea cucumbers exposed to three different concentrations (0.1 µg/L, 1.0 µg/L, and 10.0 µg/L) of BDE-47. Although the chosen exposure concentrations surpassing the typical levels in Laizhou Bay, China (Jin et al., 2008), they are still fall within an environmentally significant range. These concentrations were intentionally selected to emulate potential high pollution events, specifically those involving BDE-47, a typical brominated flame retardant. The initial objective of this study was to examine the metabolic changes in sea cucumbers exposed to BDE-47 and to identify potential biomarkers or metabolic pathways affected by the pollutant. Sea cucumbers are important ecological and economic organisms, and understanding the effects of BDE-47 on their metabolic profiles could contribute significantly to assessing the potential risks and impacts of such pollutants may pose to the sea cucumber aquaculture industry and marine ecosystems.
Materials and methods
Animals and maintenance
Sea cucumbers, A. japonicus, were collected from an outdoor pond at the Zhuwang port (37°15.656’N, 119°53.985’E) in Laizhou, China. They were then transferred to a laboratory in Laizhou and maintained in a 1500-liter cylindrical tank filled with sand-filtered and aerated seawater. The seawater was maintained at a salinity of 30‰, pH 8.0, temperature of 15 ± 0.5°C, and dissolved oxygen content of 8.5 ± 1.3 mg/L. All these water parameters were measured using a YSI EXO2 multi-parameter water quality sonde (YSI Inc., USA). During a two-week acclimation period, the sea cucumbers were fed a specially prepared diet consisting of 80% sea mud and 20% Sargassum powder at 8:00 am daily. To keep the tank clean, residual food and feces were removed using a siphon, and half of the seawater was replaced before each feeding.
BDE-47 treatment and sample collection
A total of 72 healthy sea cucumbers were selected from the maintenance tank and evenly and randomly divided into twelve 80-liter seawater tanks, forming four groups (n = 18 per group, distributed across three tanks each). One group served as the control (CT) group, which was exposed to sea water only with no addition of BDE-47, while the other three were designated as treatment groups (low, moderate, and high). The conditions of the seawater, feeding, and daily management in these tanks were maintained consistently with the initial setup. BDE-47 with a 95.00% purity, obtained from Shanghai Bide Pharmaceutical Technology Co., Ltd (Shanghai, China), was used in this study. The treatment groups were exposed to BDE-47 at different sublethal concentrations according to Ji et al. (2013a). The white powder of BDE-47 was first dissolved in dimethyl sulfoxide (DMSO), and then a specific amount was added to the seawater tanks to achieve the target concentrations. The working concentrations of BDE-47 were 0 µg/L in the control group, 0.1 µg/L in the low concentration group, 1.0 µg/L in the medium concentration group, and 10.0 µg/L in the high concentration group (Zhuo et al., 2022). The DMSO content was maintained at 0.001% in all groups (Jiang et al., 2017). Sea cucumbers in each seawater tank were exposed to their respective concentrations of BDE-47 for 20 days.
After 20 days of exposure, approximately 2 grams of body wall tissue were carefully removed from the body wall of each sea cucumber. These tissues were then washed with ultrapure water and dried with absorbent paper before being placed into a sterile tube. To minimize the influence of individual differences during detection, three random body wall specimens from each group were combined into one tube. Ultimately, 24 samples (tubes) were obtained in total, with six samples per group across the four groups. The six samples in the control group were labeled C_BW_1 to C_BW_6. Similarly, the tubes in the low concentration group were labeled L_BW_1 to L_BW_6, those in the moderate concentration group were labeled M_BW_1 to M_BW_6, and the ones in the high concentration group were labeled H_BW_1 to H_BW_6. All tissue-containing tubes were stored in liquid nitrogen.
Sample pretreatment for metabolomic detection
Approximately 50 mg of each sample stored in liquid nitrogen was added to a 2 mL Eppendorf tube containing a small steel ball (d = 6 mm) and 400 μL of extraction solution (methanol: water = 4:1) with 0.02 mg/L internal standard (L-2-chlorophenylalanine). Each sample was ground for 6 minutes (-10°C, 50 Hz) using a frozen tissue grinder. Subsequently, the sample was extracted via low-temperature ultrasound for 30 minutes (5°C, 40 kHz). After resting at -10°C for 30 minutes, the sample was centrifuged at 13,000 g for 15 minutes at 4°C. Then, 100 μL of supernatant from each sample was transferred to an injection vial with an internal tube for UPLC-Triple-TOF-MS analysis. In addition, 20 μL of supernatant from each test sample was combined to create quality control (QC) samples for quality assurance and control. All chemicals and solvents used in the above process were of analytical or HPLC grade.
UPLC-Triple-TOF-MS detection and data analysis
The LC-MS analysis was performed using an ultra-high performance liquid chromatography triple time-of-flight mass spectrometry system (AB SCIEX, USA). The chromatographic column employed was an ACQUITY UPLC HSS T3 (100 mm × 2.1 mm i.d., 1.8 µm; Waters, Milford, USA). Acetonitrile, water, and isopropanol containing 0.1% formic acid were used as mobile phase A (95% water + 5% acetonitrile) and B (47.5% acetonitrile + 47.5% isopropanol + 5% water), respectively. Metabolites were eluted with a gradient of 100% A for 0 minutes, 100% A for 0.5 minutes, 75% A for 2.5 minutes, 0% A for 9 minutes, and then maintained at 0% A for 13 minutes. The injection volume was 10 μL, with a flow rate of 0.4 mL/min and a column temperature of 40°C. Each sample was ionized by electrospray, and mass spectrum signals were collected using both positive and negative ion scanning modes. The specific parameters of mass spectrometry were set as follows:
The raw LC-MS data were imported into the metabolomics processing software Progenesis QI (Waters Corporation, Milford, USA) for baseline filtering, peak identification, integration, retention time correction, and peak alignment. A data matrix containing retention time, mass-to-charge ratio (m/z), and peak intensity was obtained. SIMCA-P 13.0 software (Umetrics AB, Umea, Sweden) was used to perform Multivariate Analysis (MVA) on the data. Additionally, partial least squares-discriminant analysis (PLS-DA), orthogonal projections to latent structures discriminant analysis (OPLS-DA), and the t-test were conducted on the acquired LC-MS data. Prior to analysis, Pareto scaling was used to preprocess all data (Yan et al., 2020). Variable importance of projection (VIP) values were also calculated. Potential metabolic biomarkers and key metabolites with significant changes were identified based on VIP values >1 and t-test p-values < 0.05. Finally, the identified metabolites were searched in the Kyoto Encyclopedia of Genes and Genomes (KEGG) biochemical database (Kanehisa et al., 2016) to annotate potential metabolic pathways.
Results
To assess the metabolic variations in the body walls of sea cucumbers exposed to BDE-47, both PLS-DA and OPLS-DA were employed in the supervised discriminant statistical analysis of metabolic data. Symbols in the plots represent samples from different groups. In the PLS-DA plot, the first (Component 1) and second (Component 2) principal components are represented by the abscissa and ordinate, respectively, and they explain the variance of the raw data (Figure 1). However, in the OPLS-DA plot, the predictive principal component and orthogonal principal component are the two principal components. Component 1 and orthogonal component 1 reflect the maximization of differences among groups (Figure S1). Both plots reveal that all three BDE-47 treated groups are clearly differentiated from the control group (Figure 1; Figure S1). Furthermore, Figure 2 displays heat maps of the top 30 differential metabolites from comparisons between the control group and other BDE-47 treated groups, suggesting that the abundance intensity of various metabolites in the control group differs from that of the three BDE-47 exposed groups.
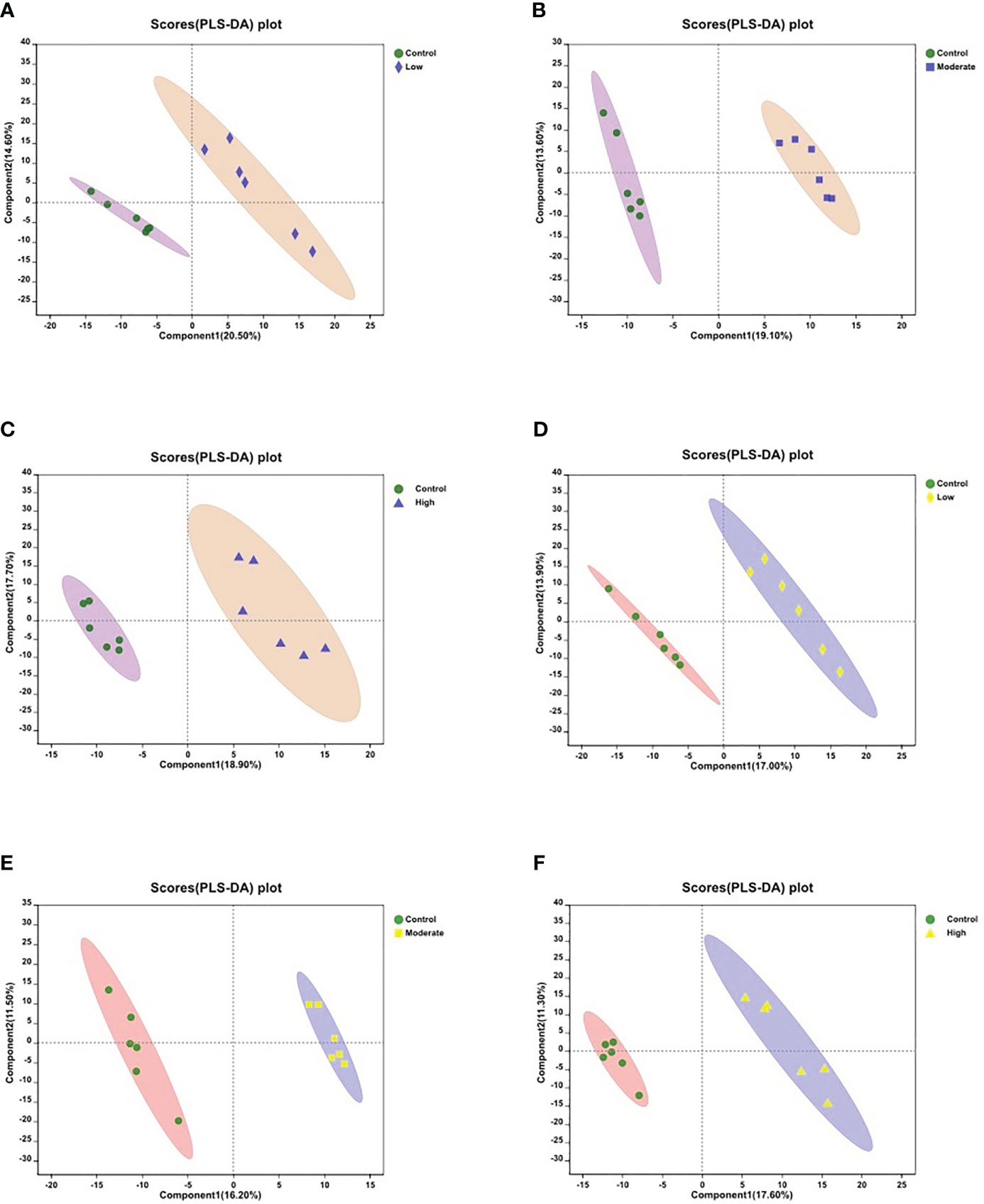
Figure 1 Partial Least Squares Discriminant Analysis (PLS-DA) score plots of metabolites in body walls from the control and three BDE-47 treated groups (A-C) for negative ions; (D-F) for positive ions). The x-axis and y-axis represent the first principal component (Component 1) and the second principal component (Component 2), respectively.
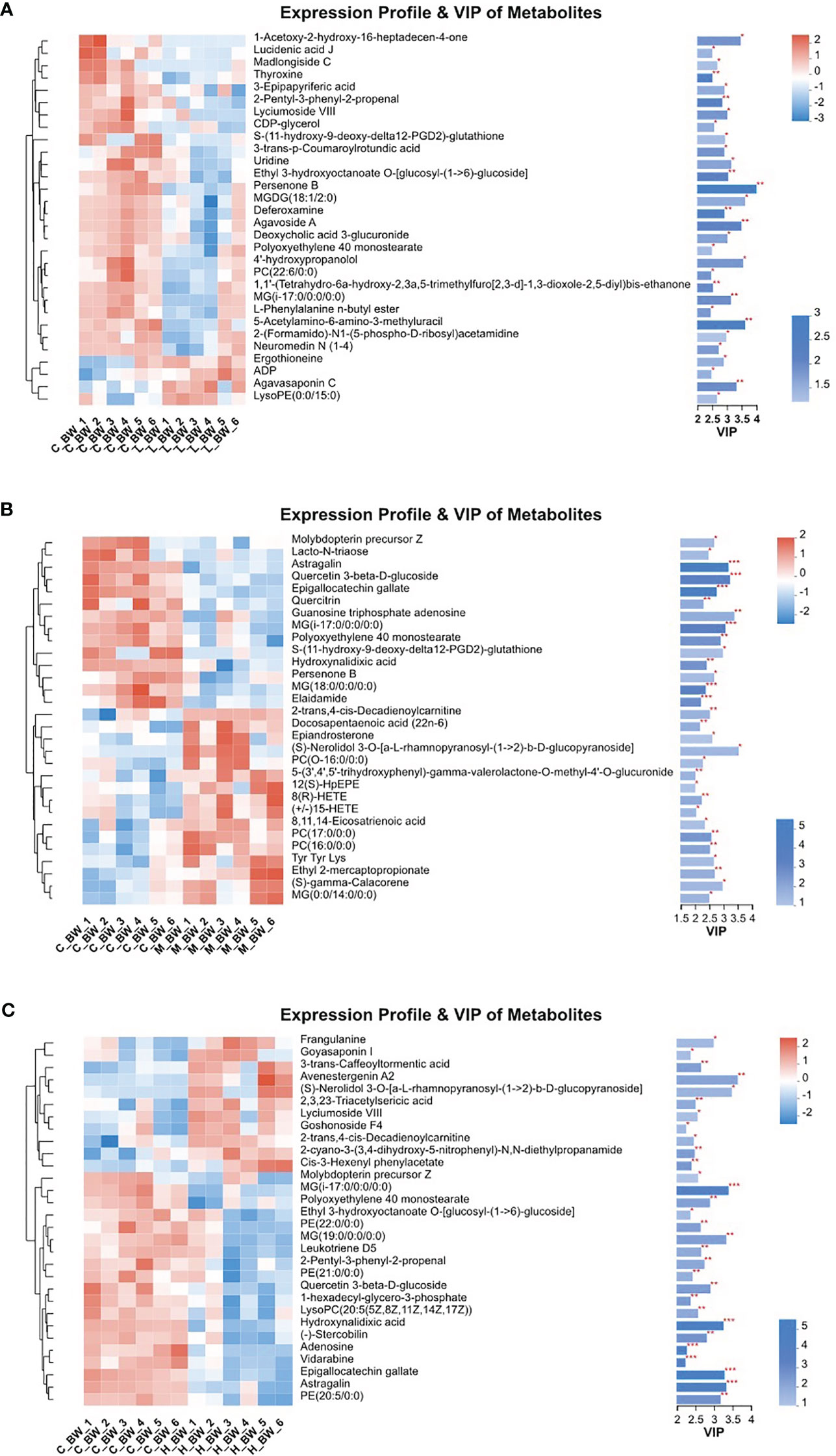
Figure 2 Heatmaps and VIP values of the top 30 differential metabolites from the control (C_BW) and BDE-47 treated (L_BW, M_BW, H_BW) groups (A: C_BW vs L_BW; B: C_BW vs M_BW; C: C_BW vs H_BW). The left side shows the metabolite cluster tree. Each line represents a differential metabolite, and each cross represents a body wall sample. Different colors indicate varying higher abundance intensities (mean value acquired from all detected samples of the same group). The right side displays the metabolite VIP bar chart. * denotes p < 0.05, ** denotes p < 0.01, *** denotes p < 0.001.
Figure 3 displays the number of differential metabolites in comparisons between the control group and the other three BDE-47 treated groups (low, moderate, and high concentrations) as well as a Venn diagram illustrating these relationships. A total of 102, 102, and 95 potential metabolites, filtered by the t-test (p < 0.05) and OPLS-DA model (VIP > 1.0), are identified and characterized from the positive and negative ion patterns, with significant changes in levels observed in the low, moderate, and high concentration BDE-47 treated groups, respectively (Figure 3).
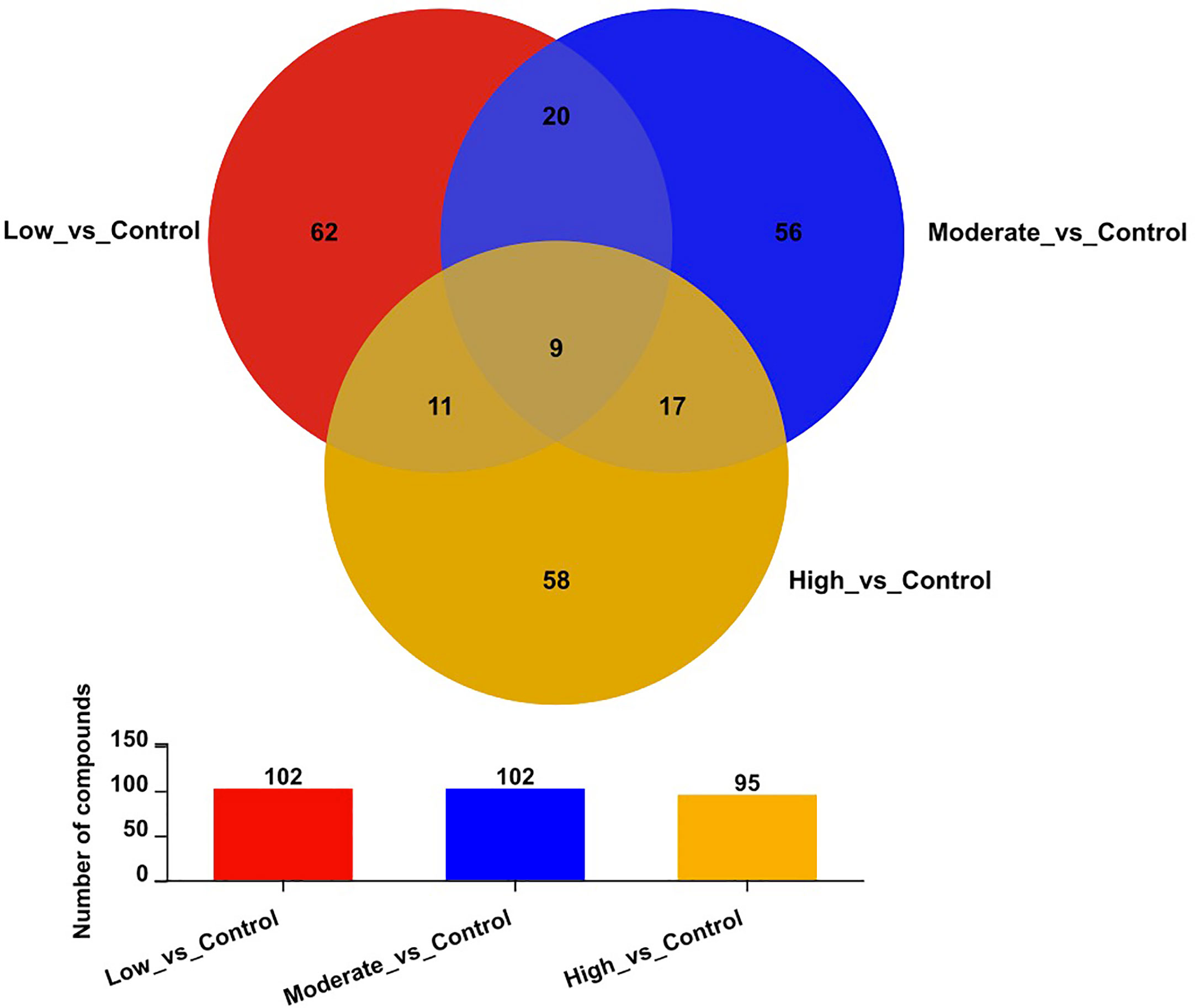
Figure 3 Venn diagram of differential metabolites in the control and three BDE-47 treated groups (low, moderate, and high concentrations).
Table 1 presents the differentiating metabolites between the control (C_BW) and low-concentration BDE-47 treatment (L_BW) groups (p < 0.01). Compared to the control group, 31 changed metabolites were detected in the low-concentration group, with nine up-regulated and 22 down-regulated metabolites. Up-regulated metabolites include L-3,5-Diiodotyrosine, ethyl 3-hydroxydodecanoate, C16 sphinganine, 6-hydroxyoctadecanoic acid, avocadene etc., while down-regulated metabolites consist of persenone B, palmyrolide A, astragalin, etc. These metabolites are involved in metabolic pathways such as purine metabolism, thyroid hormone synthesis, and biosynthesis of cofactors (Figure 4).
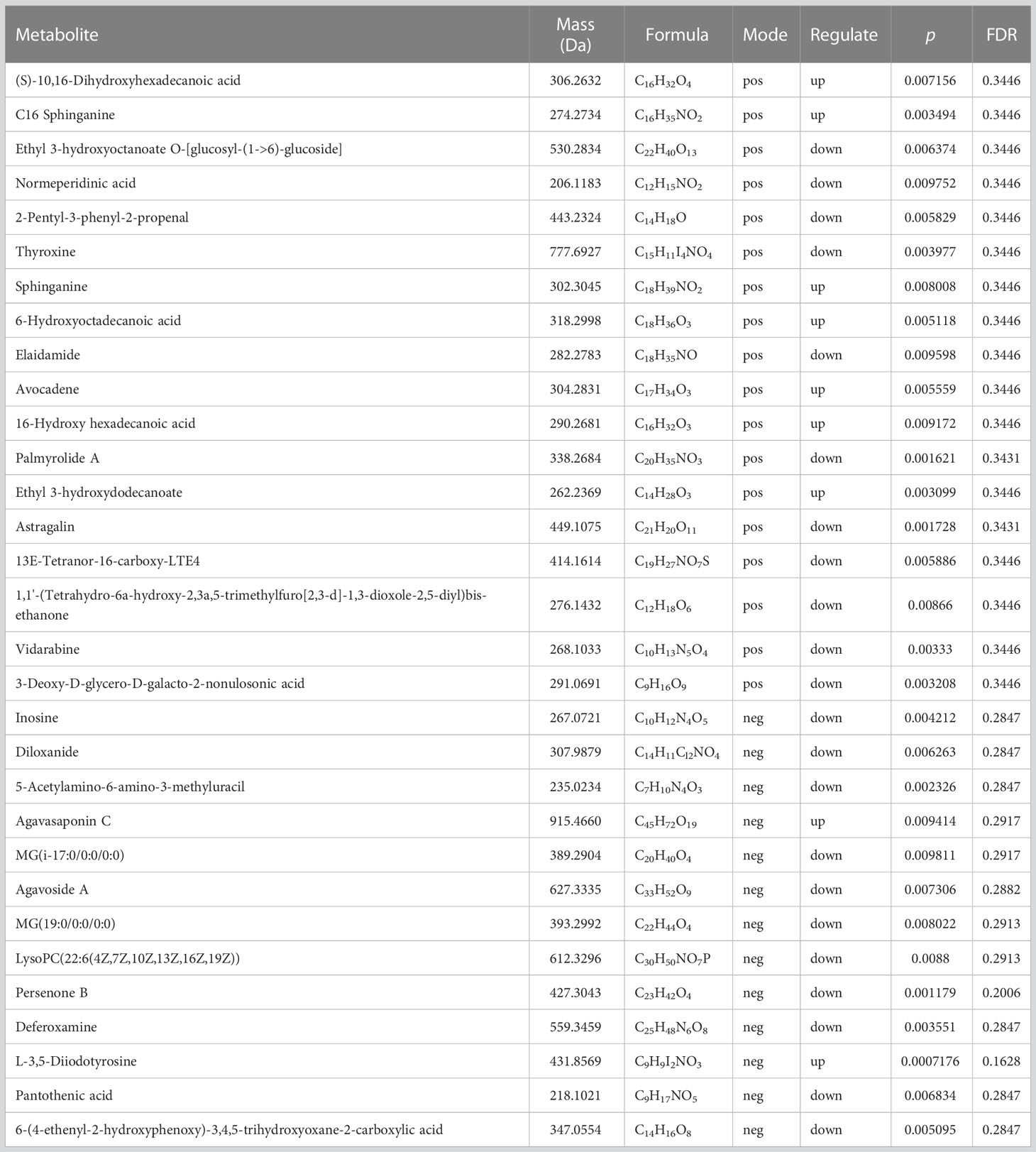
Table 1 Differentiating metabolites between the control (C_BW) and low-concentration BDE-47 treatment (L_BW) groups (p < 0.01).
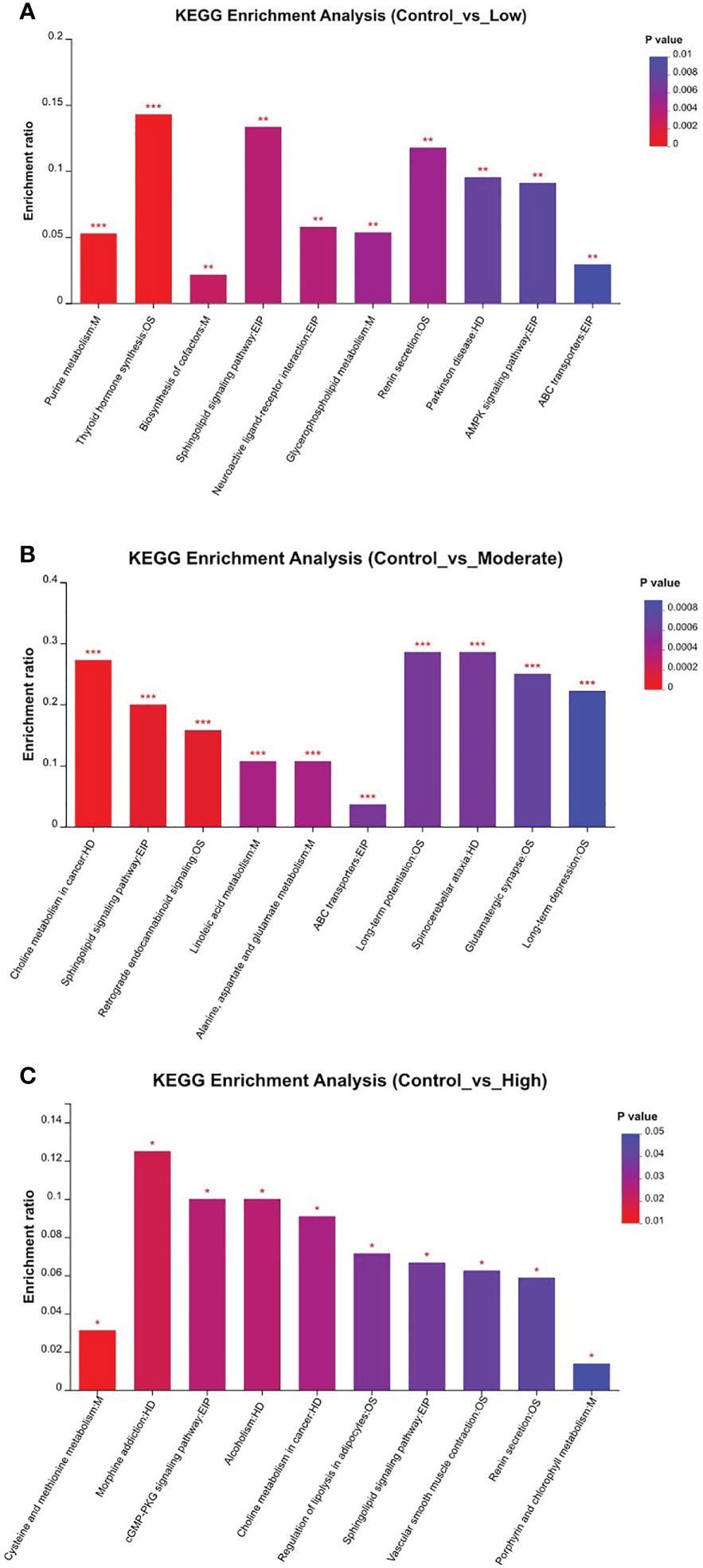
Figure 4 KEGG enrichment of relevant metabolic pathways that changed in the comparisons of the control and other three BDE-47 exposed groups (A: Control_vs_Low; B: Control_vs_Moderate; C: Control_vs_High). * denotes p < 0.05, ** denotes p < 0.01, *** denotes p < 0.001.
Additionally, Table S1 reveals the changed metabolites in the comparison between the control (C_BW) and moderate-concentration BDE-47 treatment (M_BW) groups (p < 0.01). In comparison to the control group, 42 changed metabolites were detected in the moderate-concentration group, with 23 up-regulated and 19 down-regulated metabolites. Up-regulated metabolites include aminocaproic acid, argininosuccinic acid, PC(17:0/0:0), LysoPC(0:0/16:0), inosine, etc., while down-regulated metabolites consist of astragalin, epigallocatechin gallate (EGCG), gallic acid, and quercetin 3-beta-D-glucoside, elaidamide, adenosine etc. These metabolites participate in metabolic pathways such as choline metabolism in cancer, sphingolipid signaling pathway, and retrograde endocannabinoid signaling (Figure 4).
Table S2 indicates the altered metabolites between the control (C_BW) and high-concentration BDE-47 treatment (H_BW) groups (p < 0.01). Compared to the control group, 44 changed metabolites were detected in the high-concentration group, with 14 up-regulated and 30 down-regulated metabolites. Up-regulated metabolites include cis-3-Hexenyl phenylacetate, asparagoside C, tragopogonsaponin A, osmundalacton, normeperidinic acid etc., while down-regulated metabolites consist of aphidicolin, epigallocatechin gallate, hydroxynalidixic acid, and astragalin, etc. These metabolites participate in metabolic pathways such as cysteine and methionine metabolism, morphine addiction, and cGMP-PKG signaling pathway (Figure 4).
Furthermore, nine metabolites, namely elaidamide, ethyl 3-hydroxyoctanoate O-[glucosyl-(1->6)-glucoside], polyoxyethylene 40 monostearate, MG(i-17:0/0:0/0:0), 6-(4-ethenyl-2-hydroxyphenoxy)-3,4,5-trihydroxyoxane-2-carboxylic acid, adenosine, epigallocatechin gallate, vidarabine, and astragalin were consistently down-regulated across all three BDE-47 treatment groups (p < 0.05) (Figure 3; Table 2). Except for these nine metabolites, among the differentiated metabolites identified in the low-concentration group, 20 and 11 metabolites were found to be shared in the moderate and high-concentration groups (Figure 3), and their fold changes and p values were shown in Table S3, 4, respectively. Moreover, 17 metabolites demonstrated consistent alterations in both the moderate and high concentration groups (Figure 3). The corresponding fold changes and p values for these metabolites are presented in Table S5. In the low-concentration, moderate-concentration, and high-concentration treatment groups, a total of 62, 56, and 58 metabolites, respectively, exhibited unique profiles exclusive to each group. These distinctive metabolites likely reflect specific biological responses linked to the corresponding concentration of BDE-47 exposure. The complete dataset of metabolomics in this study can been found in https://www.ebi.ac.uk/metabolights/MTBLS7730. (Haug et al., 2020).
Discussion
Several studies have focused on the effects of BDE-47 on the metabolic profiles of animals, including mice (Ji et al., 2019; Li et al., 2020b), mussels (Ji et al., 2013b), and earthworms (Ji et al., 2013c). However, there has been limited research on edible and marine cultured species, particularly economically valuable sea cucumbers, which are susceptible to BDE-47 contamination. Our metabolomic study revealed significant changes in the metabolic profiles of the body wall in sea cucumbers exposed to BDE-47. Using UPLC-Triple-TOF-MS, we identified numerous metabolites and metabolic pathways that were significantly altered and disrupted in the BDE-47 exposed groups.
Altered metabolites across all BDE-47 treatment groups
A total of 9 metabolites exhibited significant decreases in the sea cucumber body wall after exposure to all the concentrations of BDE-47 in this study (Table 2). Their alterations suggest the potential adverse effects of BDE-47 on the sea cucumber body wall, irrespective of the concentration. These metabolites are involved in multiple metabolic pathways and biological processes, such as lipid metabolism, carbohydrate metabolism, cellular membrane integrity, antioxidant defenses, and inflammatory responses in the sea cucumber body wall. Specifically, elaidamide, epigallocatechin gallate and adenosine are three crucial metabolites that may reflect the detrimental effects of BDE-47 on the sea cucumber body wall, potentially involving disruption of key biological processes.
Elaidamide, a derivative of the unsaturated fatty acid elaidic acid, is formed through an amide linkage (Leggett et al., 2016), and elaidic acid has been shown to play a role in lipid metabolism (Vendel Nielsen et al., 2013). It has been shown to have anti-inflammatory properties (Bradshaw et al., 2009) and can impact the energy storage capacity and structural integrity of tissues (Wang et al., 2015; Ronnenberg et al., 2018). However, research on elaidamide in sea cucumbers is scarce, warranting further investigation to understand its specific role in A. japonicus body wall. The decrease in elaidamide levels after BDE-47 exposure in all the treatment groups could suggest a disruption in lipid metabolism in the sea cucumber body wall, leading to alterations in tissue composition and quality. In addition, the disruption of lipid metabolism may further affect the energy storage capacity and structural integrity of the body wall (Liu et al., 2016), ultimately impacting the overall quality and nutritional value of the sea cucumber. Furthermore, the reduction in elaidamide levels might exacerbate oxidative damage caused by BDE-47 exposure, potentially leading to tissue damage and loss of nutritional value (Biswas, 2016).
EGCG is a bioactive polyphenol found in green tea and has been reported to exhibit antioxidant, anti-inflammatory, and anticancer activities (Singh et al., 2011). It has been found to exist in animal tissues following oral administration (Mehmood et al., 2022). In animal studies, particularly in rodents, researchers have observed that EGCG can be absorbed and distributed to various organs such as the liver, kidneys, and brain tissues after oral intake (Yang et al., 1998; Lambert and Yang, 2003). Numerous studies have demonstrated the bioactivity of EGCG in various animal models, including mammals, fish, insects, and other model organisms (Sachindra et al., 2010; Ferrari et al., 2022). For instance, in rodent models, EGCG has exhibited protective effects against cardiovascular diseases and tumor growth (Thangapazham et al., 2007; Singh et al., 2011). In fish models, EGCG has also displayed antioxidant and anti-inflammatory properties (Sachindra et al., 2010). These research findings suggest that EGCG possesses a wide range of bioactivities within diverse animal species. However, the research on EGCG is limited in sea cucumbers. EGCG is a known activator of the AMPK signaling pathway, which plays a crucial role in regulating energy metabolism and maintaining cellular energy homeostasis (Hardie, 2011). BDE-47 exposure could interfere with the AMPK signaling pathway by affecting EGCG levels in the sea cucumber body wall (Kim et al., 2022). As a result, the decrease in EGCG levels may disrupt energy metabolism and impair the tissue’s ability to cope with oxidative stress caused by BDE-47, ultimately affecting the quality of the sea cucumber body wall.
Adenosine is a nucleoside involved in various metabolic pathways, such as the cAMP signaling pathway, purine metabolism, and ABC transporters (Beavo and Brunton, 2002; Vasiliou et al., 2009). It has been reported to have multiple physiological roles, such as vasodilation, anti-inflammation, and neurotransmission (Fredholm et al., 2001). Despite the limited research on the specific functions of adenosine in sea cucumbers, studies on adenosine-binding aptamers and the role of adenosine receptors in regulating biological functions provide insights into its potential significance in sea cucumber body wall physiology (Van Calker et al., 2019; Jang et al., 2022). The decrease in adenosine levels after exposure to the environmental pollutant BDE-47 could disrupt the cAMP signaling pathway, which is crucial for processes such as cell growth, proliferation, and differentiation in the sea cucumber body wall (Beavo and Brunton, 2002; Antonioli et al., 2021). This disruption may lead to impaired tissue maintenance and regeneration, ultimately affecting the quality and nutritional value of the sea cucumber body wall. In addition, a study of BDE-47 induced metabolic changes indicated that adenosine was the most severely altered metabolites in mice (Ji et al., 2019). As a purine nucleoside, adenosine also plays an essential role in purine metabolism, and its decrease in all the BDE-47 treatment groups may suggest disturbances in energy metabolism and nucleotide synthesis in the sea cucumber body wall (Fredholm et al., 2001; Vasiliou et al., 2009).
Among the remaining metabolites, astragalin is a flavonoid compound with antioxidant and anti-inflammatory properties (Kim et al., 2011; Chen et al., 2020), and the decrease of it may indicate compromised antioxidant defenses and increased inflammatory response in the sea cucumber body wall in the presence of BDE-47. Vidarabine is an antiviral agent and is used clinically for antiviral therapy (Wang et al., 2021). Its decreased levels suggest potential impacts on antiviral defenses and viral replication processes in the sea cucumber body wall exposed to BDE-47. As for the other metabolites, including MG(i-17:0/0:0/0:0), polyoxyethylene 40 monostearate, 6-(4-ethenyl-2-hydroxyphenoxy)-3,4,5-trihydroxyoxane-2-carboxylic acid, and ethyl 3-hydroxyoctanoate O-[glucosyl-(1->6)-glucoside], we found no relevant report to clarify their specific functions. Therefore, we are unable to provide detailed insights into their biological significance or potential mechanisms. Further research efforts are warranted to elucidate their function and potential roles in the tissues that exposed to BDE-47.
Specifically altered metabolites in individual BDE-47 concentration groups
In total, 62, 56, and 58 metabolites were exclusively changed in low, moderate, and high concentration groups, respectively. The metabolites that were most affected by the exposure to BDE-47 in the sea cucumber body wall varied with the concentration. The distinct metabolic changes in each concentration group suggest that BDE-47 might exert different mechanisms of toxicity at different concentrations, affecting various physiological processes, including energy metabolism, antioxidant defenses, cellular signaling, lipid metabolism, and hormone regulation in the sea cucumber body wall.
At the lower concentration, several metabolites associated with key physiological processes exhibited significant changes. For instance, ADP, a fundamental molecule in energy transfer, showed notable alterations, potentially suggesting disruptions in energy metabolism (Tokarska-Schlattner et al., 2005; Fu et al., 2022). The antioxidant compound ergothioneine also demonstrated significant changes, which may be indicative of heightened oxidative stress within the sea cucumber body wall in response to BDE-47 (Cheah and Halliwell, 2012). Similarly, changes in the levels of Lucidenic acid J, Madlongiside C, Agavasaponin C, and pantothenic acid all of which are known to participate in cellular signaling processes (Su et al., 2011; Wang et al., 2016), might reflect a response to toxicant-induced stress. Moreover, acetylcarnitine, another significantly altered metabolite, has a role in transporting acetyl groups from the mitochondria (Izzo et al., 2023), suggesting potential disruption in cellular energy management in the body wall of sea cucumbers that exposed to low concentration of BDE-47. The alterations observed in key metabolites such as pantothenic acid and acetylcarnitine corroborate findings from previous studies conducted on mice subjected to BDE-47 treatment (Ji et al., 2019; Scoville et al., 2019), further substantiating our results.
In the moderate concentration group, altered levels of guanosine triphosphate adenosine, a molecule central to purine metabolism (Huang and Hsiao, 2018; Chua and Fraser, 2020), suggest potential disruptions in this crucial metabolic pathway. Similarly, significant changes in the levels of MG(18:0/0:0/0:0), a key player in lipid metabolism (Listenberger et al., 2003), may indicate alterations in lipid-related metabolic processes. Epiandrosterone, an endogenous steroid hormone (Labrie et al., 2001; Labrie et al., 2011), showed significant changes, suggesting potential endocrine-disrupting effects of BDE-47 (Hamers et al., 2008; Mclntyre et al., 2015). Betaine, known for its role in osmotic regulation and methylation processes (Craig, 2004; Rosas-Rodríguez and Valenzuela-Soto, 2021), also exhibited significant increases, highlighting a potential impact of BDE-47 on cellular homeostasis of body wall. Similarly, the elevation of betaine, as reported in mussel gills post BDE-47 exposure (Ji et al., 2013b), aligns with our observations, thereby contributing to a growing body of evidence on the impacts of BDE-47 exposure.
In the high concentration group, significant changes in the levels of PE(20:5/0:0) and PE(22:0/0:0) suggest potential effects on cell membrane integrity and signal transduction. Changes in 3a,20b-Pregnanediol and Stearoylcarnitine levels indicate potential disruptions in steroid metabolism and fatty acid metabolism. Goyasaponin I, known for its antioxidant and anti-inflammatory effects, had significant changes, indicating potential alterations in the antioxidant defenses of sea cucumbers. In the high concentration group, major changes in PE(20:5/0:0) and PE(22:0/0:0) may suggest potential effects on cell membrane integrity and lipid signaling (Van Meer et al., 2008). The altered levels of 3a,20b-Pregnanediol and Stearoylcarnitine might reflect disruptions in steroid and fatty acid metabolism, respectively (Bieber, 1988; Miller, 2013). Notably, Goyasaponin I, a known antioxidant and anti-inflammatory compound (Zhou et al., 2019), demonstrated significant changes, potentially signifying compromised antioxidant defenses and heightened inflammation. Lastly, Frangulanine and Avenestergenin A2, both known for their antioxidative properties, showed significant changes (Takai et al., 1975; Crombie et al., 1984), suggesting that BDE-47 exposure could lead to oxidative stress in the sea cucumber body wall (Stapleton et al., 2012; Ji et al., 2011).
In summary, the metabolic alterations observed at different concentrations of BDE-47 exposure in the sea cucumber body wall suggest a complex, concentration-dependent response to toxicity. At lower concentrations, metabolite changes indicate potential disruptions in energy metabolism, heightened oxidative stress, and alterations in cellular signaling, suggesting early compensatory responses or initial damage. Moderate concentration exposure appears to disturb more diverse physiological processes, including purine and lipid metabolism, and cellular homeostasis, possibly indicating increased physiological strain. High concentration exposure led to significant changes in metabolites involved in membrane integrity, steroid and fatty acid metabolism, and antioxidant responses, indicating more severe or systemic disturbances. These findings underline the importance of considering the concentration-dependent nature of toxicant responses and the need for comprehensive multi-omics approaches to fully understand the impact of environmental toxicants on marine organisms.
Other key metabolites related to the BDE-47 effect
According to our findings, there were unique overlaps of differentially altered metabolites among the concentration groups: 20 metabolites were concurrently modified in the low and moderate concentration groups (Table S3), 11 metabolites were changed in both the low and high concentration groups (Table S4), and 17 metabolites were jointly affected in the moderate and high concentration groups (Table S5). These overlaps are beyond the 9 metabolites that were found altered across all three concentration groups. The shared metabolites between each two concentration groups might reflect conserved physiological reactions and adaptive mechanisms employed by the sea cucumber under different levels of BDE-47 exposure. Among these altered metabolites, we chose to focus on inosine, avocadene, and normeperidinic acid for further discussion, given their key roles in metabolic pathways that are likely to be influenced by BDE-47 exposure.
Inosine is a naturally occurring purine nucleoside, which is formed by the breakdown of adenosine. It is involved in various biological processes, including RNA synthesis, immune system regulation and energy metabolism (Haskó et al., 2004; Ghiringhelli et al., 2012; Nakahama and Kawahara, 2020). Adenosine-to-inosine (A-to-I) RNA editing, catalyzed by adenosine deaminase acting on RNA (ADAR) enzymes, is a common RNA modification that prevents false activation of the innate immune system (Roth et al., 2019; Nakano and Nakajima, 2022). In the context of A. japonicus, the changes in inosine levels upon exposure to BDE-47 may suggest alterations in the sea cucumber’s energy metabolism and immune system. BDE-47 has been reported to induce oxidative stress in various organisms (Costa et al., 2014; Wang W. et al., 2020). Oxidative stress can affect energy metabolism, leading to disruptions in the balance between adenosine and inosine levels (Newsholme et al., 2003). The observed changes in inosine levels in A. japonicus exposed to BDE-47 might reflect a response to these metabolic alterations, potentially affecting the energy supply in the sea cucumber’s body wall. Moreover, inosine has been demonstrated to modulate immune responses through the activation of adenosine receptors, which play crucial roles in the regulation of inflammation and immune cell function (Haskó et al., 2004; Ghiringhelli et al., 2012; Samami et al., 2023). BDE-47 exposure has been linked to inflammation in various organisms (Zhang et al., 2015; Xu et al., 2021). The changes in inosine levels in A. japonicus exposed to low and medium concentrations of BDE-47, where inosine levels decreased and increased, respectively, might indicate a response to inflammation and immune system alterations in the body wall. In conclusion, the observed changes in inosine levels in sea cucumbers exposed to BDE-47 may be indicative of alterations in energy metabolism and immune system regulation in the body wall.
Avocadene, also known as (Z,Z,Z)-9,12,15-icosenetriol, is a naturally occurring polyhydroxylated fatty alcohol derived from avocado (Persea americana) and has been reported to possess several health-promoting properties, such as antioxidant, anti-inflammatory, antidiabetic, and anticancer activities (Lu et al., 2015; Bello-Pérez et al., 2020; Mu et al., 2021). The increase in avocadene levels upon exposure to low and moderate concentrations of BDE-47 in A. japonicus might suggest a possible response to oxidative stress in the sea cucumber’s body wall. Oxidative stress has been shown to be a significant factor in the toxic effects of BDE-47 (Costa et al., 2014; Xie et al., 2022). The increase in avocadene levels might represent an attempt by the sea cucumber’s body wall to counteract the oxidative stress induced by BDE-47. In a study conducted by Rodríguez-Sánchez et al. (2013), avocadene demonstrated a strong antioxidant capacity, which could help protect the cell membranes of the sea cucumber’s body wall from oxidative damage. Additionally, avocadene has been reported to possess anti-inflammatory properties (Lu et al., 2015). BDE-47 has been shown to cause inflammation in various organisms (Xu et al., 2017). Therefore, the elevated avocadene levels might suggest a response to inflammation in the sea cucumber’s body wall caused by BDE-47 exposure. In summary, the increase in avocadene levels in sea cucumbers exposed to low and moderate concentrations of BDE-47 might indicate a protective response to oxidative stress and inflammation in the body wall.
Normeperidinic acid is an important bioactive compound that has been reported to exhibit various biological activities, such as antioxidant and anti-inflammatory effects (Kosuge et al., 1998; Yang et al., 2015b; Ali et al., 2020). In our study, we observed a decrease in normeperidinic acid levels in the sea cucumber body wall after exposure to low levels of BDE-47, while an increase was observed in the high-level treatment group. The body wall of sea cucumbers, as an essential structural component, plays a crucial role in maintaining physiological functions and overall health (Wang et al., 2010). As a persistent organic pollutant, BDE-47 has been reported to induce neurotoxicity in animals (Ji et al., 2019; Li et al., 2020c). The observed decrease in normeperidinic acid levels in the body wall of sea cucumbers exposed to low levels of BDE-47 may be associated with the neurotoxic effects of BDE-47, as normeperidinic acid has been suggested to play a role in the modulation of nervous system function (Kosuge et al., 1998; Fan et al., 2021). Furthermore, the antioxidant properties of normeperidinic acid might be involved in the defense mechanisms against BDE-47-induced oxidative stress (Yang et al., 2015b), which could help to maintain the structural integrity and physiological functions of the sea cucumber body wall. On the other hand, the increase in normeperidinic acid levels observed in the high-level treatment group might represent a compensatory response to the increased BDE-47 exposure. This increase could potentially enhance the protective effects of normeperidinic acid against BDE-47-induced neurotoxicity and oxidative stress, thereby preserving the health and quality of the sea cucumber body wall (Yang et al., 2015b; Lee et al., 2021).
Conclusion
Our findings revealed that exposure to BDE-47 induced significant changes in the metabolites and metabolic pathways present in the body wall of A. japonicus. Compared to the control group, 9 metabolites were decreased in all the BDE-47 treated groups. Specifically, the down-regulations of elaidamide, epigallocatechin gallate, and adenosine in the body wall of sea cucumbers were associated with lipid metabolism, energy metabolism, and oxidative damage. The decrease of astragalin and vidarabine levels in the sea cucumber body wall exposed to BDE-47 suggests compromised antioxidant defenses, increased inflammatory response, and potential impacts on antiviral defenses and viral replication processes. In addition, Specifically altered metabolites, including ADP, Guanosine triphosphate adenosine, and PE(20:5/0:0), in individual BDE-47 concentration groups suggest a complex, concentration-dependent response to toxicity. Moreover, the alterations of inosine, avocadene, and normeperidinic acid revealed that BDE-47 exposure could also affect the metabolism of purine and fatty acids, and caused oxidative stress, inflammation, and neurotoxicity. These results indicate that BDE-47 exposure can disrupt the metabolic profiles of the body wall of sea cucumbers, potentially leading to harmful effects on the health and well-being of A. japonicus.
Data availability statement
The data presented in the study are deposited in the MetaboLights repository, accession number MTBLS7730.
Ethics statement
The animal study was reviewed and approved by The Experimental Animal Ethics Committee of Institute of Oceanology, Chinese Academy of Sciences.
Author contributions
Conceptualization: KD, PZ, QX and XZ. Methodology: KD, MG. Software: KD, XL, JM. Validation: QX and XZ. Formal analysis: KD, XL and MG. Investigation: KD and SL. Resources: PZ. Data curation: PZ, MG, QX and XZ. Writing—original draft preparation: KD. Writing—review and editing: KD and QX. Visualization: PZ. Supervision: XZ. Project administration: KD. Funding acquisition: SL. All authors contributed to the article and approved the submitted version.
Funding
This research was funded by the National Natural Science Foundation of China (Grant No. 42106131).
Acknowledgments
We would like to express our gratitude to Xiang Liu for assisting with data analysis. We are also grateful to Dr. Qiming Feng and Huanxin Gao for their help in sample collection.
Conflict of interest
The authors declare that the research was conducted in the absence of any commercial or financial relationships that could be construed as a potential conflict of interest.
Publisher’s note
All claims expressed in this article are solely those of the authors and do not necessarily represent those of their affiliated organizations, or those of the publisher, the editors and the reviewers. Any product that may be evaluated in this article, or claim that may be made by its manufacturer, is not guaranteed or endorsed by the publisher.
Supplementary material
The Supplementary Material for this article can be found online at: https://www.frontiersin.org/articles/10.3389/fmars.2023.1205538/full#supplementary-material
References
Ali H. M., El-Gizawy S. A., Abd El-Kader M. A., Abdel-Sattar E. A. (2020). Normeperidinic acid, a major metabolite of the antipsychotic drug perphenazine, ameliorates carbon tetrachloride-induced liver damage in rats. J. Biochem. Mol. Toxicol. 34 (10), e22541. doi: 10.1002/jbt.22541
Antonioli L., Pacher P., Haskó G. (2021). Adenosine and inflammation: it's time to (re) solve the problem. Trends Pharmacol. Sci. 42 (12), 961–976. doi: 10.1016/j.tips.2021.08.008
Beavo J. A., Brunton L. L. (2002). Cyclic nucleotide research [[/amp]]mdash; still expanding after half a century. Nat. Rev. Mol. Cell Biol. 3 (8), 710–718. doi: 10.1038/nrm907
Bello-Pérez M., Sánchez-Rivera M. M., Piñón-Zárate G. (2020). Health benefits and bioactive compounds of avocado (Persea americana mill.): a review. J. Food Biochem. 44 (11), e13421. doi: 10.1111/jfbc.13421
Bieber L. L. (1988). Carnitine. Annu. Rev. Biochem. 57 (1), 261–283. doi: 10.1146/annurev.bi.57.070188.001401
Biswas S. K. (2016). Does the interdependence between oxidative stress and inflammation explain the antioxidant paradox? Oxid. Med. Cell. Longevity 2016, 5698931. doi: 10.1155/2016/5698931
Bradshaw H. B., Rimmerman N., Krey J. F., Walker J. M. (2009). Sex and hormonal cycle differences in rat brain levels of pain-related cannabimimetic lipid mediators. Am. J. Physiology-Regulatory Integr. Comp. Physiol. 297 (2), R599–R608. doi: 10.1152/ajpregu.00183.2009
Byer J. D., Lebeuf M., Alaee M., Stephen B. R., Trottier S., Backus S., et al. (2013). Spatial trends of organochlorinated pesticides, polychlorinated biphenyls, and polybrominated diphenyl ethers in Atlantic anguillid eels. Chemosphere 90 (5), 1719–1728. doi: 10.1016/j.chemosphere.2012.09.044
Cheah I. K., Halliwell B. (2012). Ergothioneine; antioxidant potential, physiological function and role in disease. Biochim. Biophys. Acta (BBA)-Molecular Basis Dis. 1822 (5), 784–793. doi: 10.1016/j.bbadis.2011.09.017
Chen X., Yang W., Li Y., Zhu C., Qin Y., Hu H., et al. (2020). Antiviral activity of a purine synthesis enzyme reveals a key role of purine metabolism in aedes aegypti antiviral defense. Proc. Natl. Acad. Sci. 117 (33), 20075–20083. doi: 10.1073/pnas.2005583117
Chua S. M. H., Fraser J. A. (2020). Surveying purine biosynthesis across the domains of life unveils promising drug targets in pathogens. Immunol. Cell Biol. 98 (10), 819–831. doi: 10.1111/imcb.12350
Costa L. G., Giordano G., Guizzetti M. (2014). In vitro neurotoxicity of polybrominated diphenyl ethers (PBDEs). Neurotoxicology 43, 132–139. doi: 10.1016/j.neuro.2014.02.008
Craig S. A. (2004). Betaine in human nutrition. Am. J. Clin. Nutr. 80 (3), 539–549. doi: 10.1093/ajcn/80.3.539
Crombie L., Crombie W. M. L., Whiting D. A. (1984). Isolation of avenacins A -1, A -2, B -1, and B -2 from oat roots: structures of their ‘aglycones’, the avenestergenins. Journal of the Chemical Society, Chemical Communications, (4), 244–246.
Ding K., Zhang L., Huo D., Guo X., Liu X., Zhang S. (2021). Metabolomic analysis of coelomic fluids reveals the physiological mechanisms underlying evisceration behavior in the sea cucumber Apostichopus japonicus. Aquaculture 543, 736960. doi: 10.1016/j.aquaculture.2021.736960
Duan X., Zhang M., Mujumdar A. S., Wang S. (2010). Microwave freeze drying of sea cucumber (Stichopus japonicus). J. Food Eng. 96 (4), 491–497. doi: 10.1016/j.jfoodeng.2009.08.021
Fan H., Zhang Y., Cui Y., Zhang H., Liu Y., Qu S. (2021). Neuroprotective effects of normeperidinic acid on β-Amyloid-Induced cognitive impairment in mice. J. Alzheimer's Dis. 80 (3), 1187–1198. doi: 10.3233/JAD-200935
Ferrari E., Bettuzzi S., Naponelli V. (2022). The potential of epigallocatechin gallate (EGCG) in targeting autophagy for cancer treatment: a narrative review. Int. J. Mol. Sci. 23 (3), 1215. doi: 10.3390/ijms23031215
Fredholm B. B., IJzerman A. P., Jacobson K. A., Klotz K.-N., Linden J. (2001). International union of pharmacology. XXV. nomenclature and classification of adenosine receptors. Pharmacol. Rev. 53 (4), 527–552. Available at: https://pharmrev.aspetjournals.org/content/pharmrev/53/4/527.full.pdf
Fu J., Zhou M., Gritsenko M. A., Nakayasu E. S., Song L., Luo Z. Q. (2022). Legionella pneumophila modulates host energy metabolism by ADP-ribosylation of ADP/ATP translocases. Elife 11, e73611. doi: 10.7554/eLife.73611
Geng Q. Q., Guo M. M., Li F. L., Liu X. Y., Wu F., Yu X., et al. (2022). Tissue distribution, accumulation, elimination characteristics and toxicity of 2,2',4,4'-tetrabromodiphenyl ether in blue mussel. China Environ. Sci. 42 (3), 1385–1393. (In Chinese)
Ghiringhelli F., Bruchard M., Chalmin F., Rébé C. (2012). Production of adenosine by ectonucleotidases: a key factor in tumor immunoescape. J. Biomedicine Biotechnol. 2012, 473712. doi: 10.1155/2012/473712
Glazer L., Wells C. N., Drastal M., Odamah K. A., Galat R. E., Behl M., et al. (2017). Developmental exposure to low concentrations of two brominated flame retardants, BDE-47 and BDE-99, causes life-long behavioral alterations in zebrafish. Neurotoxicology 66, 221–232. doi: 10.1016/j.neuro.2017.02.002
Hamers T., Kamstra J. H., Sonneveld E., Murk A. J., Visser T. J., Van Velzen M. J., et al. (2008). Biotransformation of brominated flame retardants into potentially endocrine-disrupting metabolites, with special attention to 2, 2′, 4, 4′-tetrabromodiphenyl ether (BDE-47). Molecular nutrition & food research 52 (2), 284–298. doi: 10.1002/mnfr.200700104
Hardie D. G. (2011). AMP-activated protein kinase: an energy sensor that comes in 12 flavours. FEBS J. 278 (15), 2857–2861. doi: 10.1111/j.1742-4658.2011.08193.x
Haskó G., Cronstein B. N., Szabó C. (2004). Adenosine receptor agonists differentially regulate IL-10, TNF-alpha, and nitric oxide production in RAW 264.7 macrophages and in endotoxemic mice. J. Immunol. 173 (12), 4635–4645. doi: 10.4049/jimmunol.173.12.4635
Haskó G., Kuhel D. G., Chen J. F., Schwarzschild M. A., Deitch E. A., Mable y J. G., et al. (2000). Adenosine inhibits IL-12 and TNF-[alpha] production via adenosine A2a receptor-dependent and independent mechanisms. FASEB J. 14 (13), 2065–2074. doi: 10.1096/fj.00-0249com
Haug K., Cochrane K., Nainala V. C., Williams M., Chang J., Jayaseelan K. V., et al. (2020). MetaboLights: a resource evolving in response to the needs of its scientific community. Nucleic acids research 48 (D1), D440–D444. doi: 10.1093/nar/gkz1019
Hayakawa K., Takatsuki H., Watanabe I., Sakai S. I. (2004). Polybrominated diphenyl ethers (PBDEs), polybrominated dibenzo-p-dioxins/dibenzofurans (PBDD/Fs) and monobromo-polychlorinated dibenzo-p-dioxins/dibenzofurans (MoBPXDD/Fs) in the atmosphere and bulk deposition in Kyoto, Japan. Chemosphere 57 (5), 343–356. doi: 10.1016/j.chemosphere.2004.05.027
He H., Shi X., Lawrence A., Hrovat J., Turner C., Cui J. Y., et al. (2020). 2, 2′, 4, 4′-tetrabromodiphenyl ether (BDE-47) induces wide metabolic changes including attenuated mitochondrial function and enhanced glycolysis in PC12 cells. Ecotoxicology Environ. Saf. 201, 110849. doi: 10.1016/j.ecoenv.2020.110849
Herzke D., Berger U., Kallenborn R., Nygård T., Vetter W. (2005). Brominated flame retardants and other bromines in Norwegian predatory bird eggs. Chemosphere 61 (3), 441–449. doi: 10.1016/j.chemosphere.2005.03.086
Huang C. C., Hsiao K. M. (2018). Metabolic pathways and crosstalk in metabolically healthy obesity and metabolically unhealthy obesity. Chin. J. Physiol. 61 (3), 149–158. doi: 10.4077/CJP.2018.BAG589
Ikonomou M. G., Rayne S., Fischer M., Fernandez M. P., Cretney W. (2002). Occurrence and congener profiles of polybrominated diphenyl ethers (PBDEs) in environmental samples from coastal British Columbia, Canada. Chemosphere 46 (5), 649–663. doi: 10.1016/S0045-6535(01)00229-6
Izzo L. T., Trefely S., Demetriadou C., Drummond J. M., Mizukami T., Kuprasertkul N. (2023). Acetylcarnitine shuttling links mitochondrial metabolism to histone acetylation and lipogenesis. Sci. Adv. 9 (18), eadf0115. doi: 10.1126/sciadv.adf0115
Jakobsson K., Thuresson K., Rylander L., Sjödin A., Hagmar L., Bergman Å. (2002). Exposure to polybrominated diphenyl ethers and tetrabromobisphenol a among computer technicians. Chemosphere 46 (5), 709–716. doi: 10.1016/S0045-6535(01)00243-0
Jang K., Westbay J. H., Asher S. A. (2022). DNA-Crosslinked 2D photonic crystal hydrogels for detection of adenosine actuated by an adenosine-binding aptamer. ACS Sensors 7 (3), 1024–1030. doi: 10.1021/acssensors.2c00136
Ji K., Hong S., Kho Y., Choi K. (2013a). Effects of bisphenol s exposure on endocrine functions and reproduction of zebrafish. Environ. Sci. Technol. 47 (15), 8793–8800. doi: 10.1021/es400329t
Ji K., Liu X., Lee S., Kang S., Kho Y., Giesy J. P., et al. (2011). Effects of non-ortho-substituted polychlorinated biphenyls, PCP, and PBDEs on thyroid hormone levels in rats. Environ. Toxicol. Chem. 30 (4), 862–869. doi: 10.1002/etc.471
Ji F., Sreenivasmurthy S. G., Wei J., Shao X., Luan H., Zhu L., et al. (2019). Study of BDE-47 induced parkinson's disease-like metabolic changes in C57BL/6 mice by integrated metabolomic, lipidomic and proteomic analysis. J. Hazardous Materials 378, 120738. doi: 10.1016/j.jhazmat.2019.120738
Ji C., Wu H., Wei L., Zhao J., Yu J. (2013b). Proteomic and metabolomic analysis reveal gender-specific responses of mussel Mytilus galloprovincialis to 2, 2', 4, 4'-tetrabromodiphenyl ether (BDE 47). Aquat. Toxicol. 140-141, 449–457. doi: 10.1016/j.aquatox.2013.06.016
Ji C., Wu H., Wei L., Zhao J., Lu H., Yu J. (2013c). Proteomic and metabolomic analysis of earthworm Eisenia fetida exposed to different concentrations of 2, 2', 4, 4'-tetrabromodiphenyl ether. J. Proteomics 91, 405–416. doi: 10.1016/j.jprot.2013.07.026
Jiang Y., Tang X., Sun T., Wang Y. (2017). BDE-47 exposure changed the immune function of haemocytes in mytilus edulis: an explanation based on ROS-mediated pathway. Aquat. Toxicol. 182, 58–66. doi: 10.1016/j.aquatox.2016.11.016
Jin J., Liu W., Wang Y., Tang X. Y. (2008). Levels and distribution of polybrominated diphenyl ethers in plant, shellfish and sediment samples from laizhou bay in China. Chemosphere 71, 1043–1050. doi: 10.1016/j.chemosphere.2007.11.013
Kanehisa M., Sato Y., Kawashima M., Furumichi M., Tanabe M. (2016). KEGG as a reference resource for gene and protein annotation. Nucleic Acids Res. 44 (D1), D457–D462. doi: 10.1093/nar/gkv1070
Kim M. S., Kim S. H. (2011). Inhibitory effect of astragalin on expression of lipopolysaccharide-induced inflammatory mediators through NF-κB in macrophages. Arch Pharm Res 34, 2101–2107. doi: 10.1007/s12272-011-1213-x
Kim S. R., Seong K. J., Kim W. J., Jung J. Y. (2022). Epigallocatechin gallate protects against hypoxia-induced inflammation in microglia via NF-κB suppression and nrf-2/HO-1 activation. Int. J. Mol. Sci. 23 (3), 1128. doi: 10.3390/ijms23031128
Kosuge T., Yokota M., Sugiyama K., Yamamoto T., Yamaguchi T. (1998). Isolation of normeperidinic acid as an inhibitor of superoxide anion generation by activated neutrophils from the leaves of Mallotus repandus. J. Natural Products 61 (4), 508–510. doi: 10.1021/np970533f
Labrie F., Luu-The V., Labrie C., Simard J. (2001). DHEA and its transformation into androgens and estrogens in peripheral target tissues: intracrinology. Front. Neuroendocrinol. 22 (3), 185–212. doi: 10.1006/frne.2001.0210
Labrie F., Martel C., Balser J. (2011). Wide distribution of the serum dehydroepiandrosterone and sex steroid levels in postmenopausal women: role of the ovary? Menopause 18 (1), 30–43. doi: 10.1097/gme.0b013e3181e8f880
Lambert J. D., Yang C. S. (2003). Mechanisms of cancer prevention by tea constituents. J. Nutr. 133 (10), 3262S–3267S. doi: 10.1093/jn/133.10.3262S
Lee S. H., Kim S. Y., Lee S. H., Moon J. Y., Jeon Y. J. (2021). Normeperidine, a metabolite of meperidine, exhibits neuroprotective effects against oxidative stress-induced neuronal cell death. Biomolecules 11 (5), 718. doi: 10.3390/biom11050718
Leggett D. A., Mudalige T. K., Brown H. A. (2016). N-ethyl elaidamide: a surrogate analytical standard for the quantification of elaidamide and oleamide. Analytical Chem. 88 (10), 5435–5442. doi: 10.1021/acs.analchem.6b00760
Lema S. C., Schultz I. R., Scholz N. L., Incardona J. P., Swanson P. (2007). Neural defects and cardiac arrhythmia in fish larvae following embryonic exposure to 2, 2’, 4, 4’-tetrabromodiphenyl ether (PBDE 47). Aquat. Toxicol. 82 (4), 296–307. doi: 10.1016/j.aquatox.2007.03.002
Li Y., Chen L., Zhao X., Cui L., Chen H., Xiao X. (2020c). The neurotoxicity of BDE-47 and its effect on dopamine β-hydroxylase activity and expression in mice. Chemosphere 240, 124944. doi: 10.1016/j.chemosphere.2019.124944
Li R., Guo C., Tse W. K. F., Su M., Zhang X., Lai K. P. (2020a). Metabolomic analysis reveals metabolic alterations of human peripheral blood lymphocytes by perfluorooctanoic acid. Chemosphere 239, 124810. doi: 10.1016/j.chemosphere.2019.124810
Li Y., Yu N., Li M., Li K., Shi W., Yu H., et al. (2020b). Metabolomic insights into the lasting impacts of early-life exposure to BDE-47 in mice. Environ. pollut. 263, 114524. doi: 10.1016/j.envpol.2020.114524
Liu X., Jiao Y., Lin C., Sun K., Zhao Y. (2014). PBDEs, hydroxylated PBDEs and methoxylated PBDEs in bivalves from Beijing markets. Chemosphere 110, 97–103. doi: 10.1016/j.chemosphere.2014.03.017
Liu Z., Zhou D., Wang Y. (2016). Lipid metabolism in sea cucumber: implications for aquaculture. Aquaculture Res. 47 (9), 2658–2669. doi: 10.1111/are.12767
Listenberger L. L., Han X., Lewis S. E., Cases S., Farese Jr, R. V., Ory D. S., et al. (2003). Triglyceride accumulation protects against fatty acid-induced lipotoxicity. Proc Natl Acad Sci 100 (6), 3077–3082. doi: 10.1073/pnas.0630588100
Lorber M. (2008). Exposure of americans to polybrominated diphenyl ethers. J. Exposure Sci. Environ. Epidemiol. 18 (1), 2–19. doi: 10.1038/sj.jes.7500572
Lu Q. Y., Arteaga J. R., Zhang Q., Huerta S., Go V. L., Heber D. (2015). Inhibition of prostate cancer cell growth by an avocado extract: role of lipid-soluble bioactive substances. J. Nutr. Biochem. 16 (1), 23–30. doi: 10.1016/j.jnutbio.2004.08.001
McIntyre R. L., Kenerson H. L., Subramanian S., Wang S. A., Kazami M., Stapleton H. M., et al. (2015). Polybrominated diphenyl ether congener, BDE-47, impairs insulin sensitivity in mice with liver-specific Pten deficiency. BMC obesity 2 (1), 1–8. doi: 10.1186/s40608-014-0031-3
Mehmood S., Maqsood M., Mahtab N., Abbas S., Bashari M. (2022). Epigallocatechin gallate: phytochemistry, bioavailability, utilization challenges, and strategies. J. Food Biochem. 46 (1), e13914. doi: 10.1111/jfbc.13914
Messina C. M., Ruiz C. E., Regoli F., Manuguerra S., D'Agostino F., Avellone G., et al. (2020). BDE-47 exposure modulates cellular responses, oxidative stress and biotransformation related-genes in Mytilus galloprovincialis. Fish Shellfish Immunol 107, 537–546. doi: 10.1016/j.fsi.2020.11.015
Miller W. L. (2013). Steroid hormone synthesis in mitochondria. Mol. Cell. Endocrinol. 379 (1-2), 62–73. doi: 10.1016/j.mce.2013.05.003
Mo J., Prévost S. F., Blowes L. M., Egertová M., Terrill N. J., Wang W., et al. (2016). Interfibrillar stiffening of echinoderm mutable collagenous tissue demonstrated at the nanoscale. Proc. Natl. Acad. Sci. 113, E6362–E6371. doi: 10.1073/pnas.1608486113
Mu L., Xie C., Liu Y., Ma W. (2021). A systematic review on the pharmacological effects of avocado: implications for health and disease. Phytotherapy Res. 35 (6), 2756–2772. doi: 10.1002/ptr.7025
Nakahama T., Kawahara Y. (2020). Adenosine-to-inosine RNA editing in the immune system: friend or foe? Cell. Mol. Life Sci. 77, 2931–2948. doi: 10.1007/s00018-020-03508-6
Nakano M., Nakajima M. (2022). Adenosine-to-Inosine RNA editing and N6-methyladenosine modification modulating expression of drug metabolizing enzymes. Drug Metab. Disposition 50 (5), 624–633. doi: 10.1124/dmd.121.000583
Newsholme P., Procopio J., Lima M. M., Pithon-Curi T. C., Curi R. (2003). Glutamine and glutamate–their central role in cell metabolism and function. Cell Biochem. Funct. 21 (1), 1–9. doi: 10.1002/cbf.1003
Nicholson J. K. (2006). Global systems biology, personalized medicine and molecular epidemiology. Mol. Syst. Biol. 2, 52. doi: 10.1038/msb4100095
Qiu Y. W., Zhang G., Guo L. L., Cheng H. R., Wang W. X., Li X. D., et al. (2009). Polybrominated diphenyl ethers in sediments, suspended particulate matter and fish from the pearl river estuary, south China. Environ. pollut. 157 (3), 918–925. doi: 10.1016/j.envpol.2008.11.013
Rajput I. R., Yaqoob S., Sun Y., Sanganyado E., Liu W. (2021). Polybrominated diphenyl ethers exert genotoxic effects in pantropic spotted dolphin fibroblast cell lines. Environ. pollut. 271, 116131. doi: 10.1016/j.envpol.2021.116131
Ramu K., Kajiwara N., Tanabe S., Lam P. K., Jefferson T. A. (2005). Polybrominated diphenyl ethers (PBDEs) and organochlorines in small cetaceans from Hong Kong waters: levels, profiles and distribution. Mar. pollut. Bull. 51 (8/12), 669–676. doi: 10.1016/j.marpolbul.2005.02.049
Rodríguez-Sánchez D., Silva-Platas C., Rojo R. P., García N., Cisneros-Zevallos L., García-Rivas G., Hernández-Brenes, C. (2013). Activity-guided identification of acetogenins as novel lipophilic antioxidants present in avocado pulp (Persea americana). Journal of Chromatography B 942, 37–47. doi: 10.1016/j.jchromb.2013.10.013
Ronnenberg A. G., Wood R. J., Wang X., Xing H., Chen C., Chen D., et al. (2018). Preconception heme and nonheme iron intake and birth outcomes: a prospective cohort study. Am. J. Clin. Nutr. 108 (2), 467–476. doi: 10.1093/ajcn/nqy122
Rosas-Rodríguez J. A., Valenzuela-Soto E. M. (2021). The glycine betaine role in neurodegenerative, cardiovascular, hepatic, and renal diseases: insights into disease and dysfunction networks. Life Sci. 285, 119943. doi: 10.1016/j.lfs.2021.119943
Roth S. H., Levanon E. Y., Eisenberg E. (2019). Genome-wide quantification of ADAR adenosine-to-inosine RNA editing activity. Nat. Methods 16 (5), 435–440. doi: 10.1038/s41592-019-0362-3
Sachindra N. M., Sato E., Maeda H., Hosokawa M., Niwano Y., Kohno M., et al. (2010). Radical scavenging and singlet oxygen quenching activity of marine carotenoid fucoxanthin and its metabolites. J. Agric. Food Chem. 58 (19), 10516–10522. doi: 10.1021/jf102285b
Saito M., Kunisaki N., Urano N., Kimura S. (2002). Collagen as the Major Edible Component of Sea Cucumber (Stichopus japonicus). J Food Sci 67 (4), 1319–1322. doi: 10.1111/j.1365-2621.2002.tb10281.x
Samami E., Aleebrahim-Dehkordi E., Mohebalizadeh M., Yaribash S., Saghazadeh A., Rezaei N. (2023). Inosine, gut microbiota, and cancer immunometabolism. Am. J. Physiology-Endocrinology Metab. 324 (1), E1–E8. doi: 10.1152/ajpendo.00211.2022
Scoville D. K., Li C. Y., Wang D., Dempsey J. L., Raftery D., Mani S., et al. (2019). Polybrominated diphenyl ethers and gut microbiome modulate metabolic syndrome-related aqueous metabolites in mice. Drug Metab. Disposition 47, 928–940. doi: 10.1124/dmd.119.087338
Shockcor J. P., Holmes E. (2002). Metabonomic applications in toxicity screening and disease diagnosis. Curr. Topics Medicinal Chem. 2, 35–51. doi: 10.2174/1568026023394403
Singh B. N., Shankar S., Srivastava R. K. (2011). Green tea catechin, epigallocatechin-3-gallate (EGCG): mechanisms, perspectives and clinical applications. Biochem. Pharmacol. 82 (12), 1807–1821. doi: 10.1016/j.bcp.2011.07.093
Stapleton H. M., Eagle S., Sjödin A., Webster T. F. (2012). Serum PBDEs in a North Carolina toddler cohort: associations with handwipes, house dust, and socioeconomic variables. Environ Health Perspect 120 (7), 1049–1054. doi: 10.1289/ehp.1104802
Sun J., Yu Y., Zhao Z., Yin D., Chang Y., Zhao C. (2022). Ecological niche models for the assessment of site suitability of sea cucumbers and sea urchins in China. Sci. Rep. 12 (1), 1–10. doi: 10.1038/s41598-022-09130-0
Su Z. H., Li S. Q., Zou G. A., Yu C. Y., Sun Y. G., Zhang H. W., et al. (2011). Urinary metabonomics study of anti-depressive effect of Chaihu-Shu-Gan-San on an experimental model of depression induced by chronic variable stress in rats. Journal of pharmaceutical and biomedical analysis 55 (3), 533–539. doi: 10.1016/j.jpba.2011.02.013
Takai Z. H., Ogihara S. Q., Iitaka G. A., Shibata C. Y. (1975). Peptides in Higher Plants. I. The Conformation of Frangulanine. Chemical and Pharmaceutical Bulletin 23 (11), 2556–2559.
Thangapazham R. L., Singh A. K., Sharma A., Warren J., Gaddipati J. P., Maheshwari R. K. (2007). Green tea polyphenols and its constituent epigallocatechin gallate inhibits proliferation of human breast cancer cells in vitro and in vivo. Cancer Lett. 245 (1-2), 232–241. doi: 10.1016/j.canlet.2006.01.027
Tokarska-Schlattner M., Lucchinetti E., Zaugg M., Kay L., Gratia S., Guzun R., et al. (2005). Early effects of doxorubicin in perfused heart: transcriptional profiling reveals inhibition of cellular stress response genes. Am. J. Physiology-Regulatory Integr. Comp. Physiol. 288 (6), R1765–R1775. doi: 10.1152/ajpregu.00654.2004
Van Calker D., Biber K., Domschke K., Eckstein N. (2019). The role of adenosine receptors in mood and anxiety disorders. J. Neurochemistry 151 (1), 11–27. doi: 10.1111/jnc.14649
Van de Merwe J. P., Chan A. K. Y., Lei E. N. Y., Yau M. S., Lam M. H., Wu R. S. (2011). Bioaccumulation and maternal transfer of PBDE 47 in the marine medaka (Oryzias melastigma) following dietary exposure. Aquat. Toxicol. 103, 199–204. doi: 10.1016/j.aquatox.2011.03.008
Van Meer G., Voelker D. R., Feigenson G. W. (2008). Membrane lipids: where they are and how they behave. Nat. Rev. Mol. Cell Biol. 9 (2), 112–124. doi: 10.1038/nrm2330
Vasiliou V., Vasiliou K., Nebert D. W. (2009). Human ATP-binding cassette (ABC) transporter family. Hum. Genomics 3 (3), 281–290. doi: 10.1186/1479-7364-3-3-281
Vendel Nielsen L., Krogager T. P., Young C., Ferreri C., Chatgilialoglu C., Nørregaard Jensen O., et al. (2013). Effects of elaidic acid on lipid metabolism in HepG2 cells, investigated by an integrated approach of lipidomics, transcriptomics and proteomics. PLoS One 8 (9), e74283. doi: 10.1371/journal.pone.0074283
Wang Y., Tian M., Chang Y., Xue C., Li Z. (2020). Investigation of structural proteins in sea cucumber (Apostichopus japonicus) body wall. Sci. Rep. 10, 1–12. doi: 10.1038/s41598-020-67137-z
Wang H., Wang M., Chen J., Tang Y., Dou J., Yu J. (2015). Lipidomics reveals a link between CYP1A1 and SCD1 in promoting obesity. J. Proteome Res. 14 (11), 4566–4574. doi: 10.1021/acs.jproteome.5b00530
Wang X., Wang C., Wang J., Zhao S., Zhang K. Q. (2016). Antibiotic properties of the endophytic streptomyces sp. from the roots of glycyrrhiza uralensis and characterization of two biosynthetic gene clusters. World J. Microbiol. Biotechnol. 32 (6), 98. doi: 10.1007/s11274-016-2042-7
Wang F., Yang H., Gao F., Liu G. (2010). Body wall of the sea cucumber: a review. J. Fisheries China 34 (2), 299–305. (In Chinese)
Wang W., Zhao X., Ren X., Duan X. (2020). Antagonistic effects of multi-walled carbon nanotubes and BDE-47 in zebrafish (Danio rerio): oxidative stress, apoptosis and DNA damage. Aquat. Toxicol. 225, 105546. doi: 10.1016/j.aquatox.2020.105546
Wang Z., Zang R., Niu Z., Wang W., Wang X., Tang Y. (2021). Synthesis and antiviral effect of phosphamide modified vidarabine for treating HSV 1 infections. Bioorg Med Chem Lett 52 (4), 128405. doi: 10.1016/j.bmcl.2021.128405
Wilkie I. (2005). Mutable collagenous tissue: overview and biotechnological perspective. Echinodermata, 221–250.
Xie Q., Huang X., Chen J., Liu Y. (2022). PBDEs induce oxidative stress and inflammatory responses in rat liver: a proteomic study. Environ. Sci. pollut. Res. 29 (6), 6956–6968. doi: 10.1007/s11356-022-18572-1
Xing L., Sun L., Liu S., Zhang L., Yang H. (2021). Comparative metabolomic analysis of the body wall from four varieties of the sea cucumber Apostichopus japonicus. Food Chem. 352, 129339. doi: 10.1016/j.foodchem.2021.129339
Xu L., Gao S., Zhao H., Wang L., Cao Y., Xi J., et al. (2021). Integrated proteomic and metabolomic analysis of the testes characterizes BDE-47-induced reproductive toxicity in mice. Biomolecules 11 (6), 821. doi: 10.3390/biom11060821
Xu F., Giovanoulis G., van Waes S., Padilla-Sánchez J. A., Papadopoulou E., Magnér J. (2017). Comprehensive study of human external exposure to organophosphate flame retardants via air, dust, and hand wipes: the Swedish PentaBDE replacement study. Environ. Int. 102, 112–123. doi: 10.1016/j.envint.2017.02.015
Yan X., Zhang S., Fu H., Qu H. (2020). Combining convolutional neural networks and on-line raman spectroscopy for monitoring the cornu caprae hircus hydrolysis process. Spectrochimica Acta Part A: Mol. Biomolecular Spectrosc. 226, 117589. doi: 10.1016/j.saa.2019.117589
Yang C. S., Chen L., Lee M. J., Balentine D., Kuo M. C., Schantz S. P. (1998). Blood and urine levels of tea catechins after ingestion of different amounts of green tea by human volunteers. Cancer Epidemiology Biomarkers Prev. 7 (4), 351–354. Available at: https://citeseerx.ist.psu.edu/document?repid=rep1&type=pdf&doi=245b58410bac2c74e4a1ae91c3d1900a54a5385e
Yang H. S., Hamel J. F., Mercier A. (2015a). The Sea cucumber apostichopus japonicus: history, biology and aquaculture (Amsterdam, The Netherlands: Academic Press), 353–365.
Yang Q., Li S., Li P., Wang Y. (2015b). A new norlignan and the anti-inflammatory activities of two isolated compounds from Mallotus repandus. Fitoterapia 100, 102–108. doi: 10.1016/j.fitote.2014.11.013
Yang C., Wong C. M., Wei J., Chung A., Cai Z. (2018). The brominated flame retardant BDE-47 upregulates purine metabolism and mitochondrial respiration to promote adipocyte differentiation. Sci. Total Environ. 644, 1312–1322. doi: 10.1016/j.scitotenv.2018.06.381
Zhang Z. F., Zhang Y., Fan S. H., Zhuang J., Zheng Y. L., Lu J., et al. (2015). Troxerutin protects against 2,2′,4,4′-tetrabromodiphenyl ether (BDE-47)-induced liver inflammation by attenuating oxidative stress-mediated NAD+-depletion. J. hazardous materials 283, 98–109. doi: 10.1016/j.jhazmat.2014.09.012
Zhao Y., Wang X., Tang X., Zhao Y. (2022). Toxicity of 2, 2', 4, 4'-tetrabromodiphenyl ether (BDE-47) on the green microalgae chlorella sp. and the role of cellular oxidative stress. Mar. pollut. Bull. 180, 113810. doi: 10.1016/j.marpolbul.2022.113810
Zheng B., Zhao X., Liu L., Li Z., Lei K., Zhang L., et al. (2011). Effects of hydrodynamics on the distribution of trace persistent organic pollutants and macrobenthic communities in Bohai Bay. Chemosphere 84 (3), 336–341. doi: 10.1016/j.chemosphere.2011.04.006
Zhou M. Y., Zhang H. Z., Xia B., Cui Y., Qiao X. Y., Chen B. J., et al. (2010). Residue levels and distribution character of PBDEs in aquaculture zone of jiaozhou bay. Mar. Environ. Sci. 29 (6), 884–888. (In Chinese)
Zhou R., Zhu L., Kong Q., Luo Y. (2008). Levels and distribution of polybrominated diphenyl ethers in plant, shellfish and sediment samples from laizhou bay in China. Chemosphere 71 (6), 1043–1050. doi: 10.1016/j.chemosphere.2007.11.011
Zhuo P., Ding K., Deng B., Lai K., Zhang S., Zhang L., et al. (2022). The effect of 2,2',4,4'-tetrabromodiphenyl ether (BDE-47) on locomotor behaviour and muscle physiology of the sea cucumber Apostichopus japonicus. Mar. pollut. Bull. 185, 114198. doi: 10.1016/j.marpolbul.2022.114198
Keywords: sea cucumber, BDE-47, body wall, metabolite, metabolic pathway
Citation: Ding K, Zhuo P, Ge M, Liao X, Mo J, Liu S, Xu Q and Zhang X (2023) Metabolic profiling of Apostichopus japonicus body wall exposed to a typical type of PBDEs: potential health risks and impact on sea cucumber health. Front. Mar. Sci. 10:1205538. doi: 10.3389/fmars.2023.1205538
Received: 14 April 2023; Accepted: 20 June 2023;
Published: 28 September 2023.
Edited by:
Ming Li, Ningbo University, ChinaReviewed by:
Linbao Zhang, South China Sea Fisheries Research Institute, Chinese Academy of Fishery Sciences (CAFS), ChinaMohamed Mohsen, Jimei University, China
Copyright © 2023 Ding, Zhuo, Ge, Liao, Mo, Liu, Xu and Zhang. This is an open-access article distributed under the terms of the Creative Commons Attribution License (CC BY). The use, distribution or reproduction in other forums is permitted, provided the original author(s) and the copyright owner(s) are credited and that the original publication in this journal is cited, in accordance with accepted academic practice. No use, distribution or reproduction is permitted which does not comply with these terms.
*Correspondence: Qinzeng Xu, eHVxaW56ZW5nQGZpby5vcmcuY24=; Xuelei Zhang, emhhbmd4bEBmaW8ub3JnLmNu