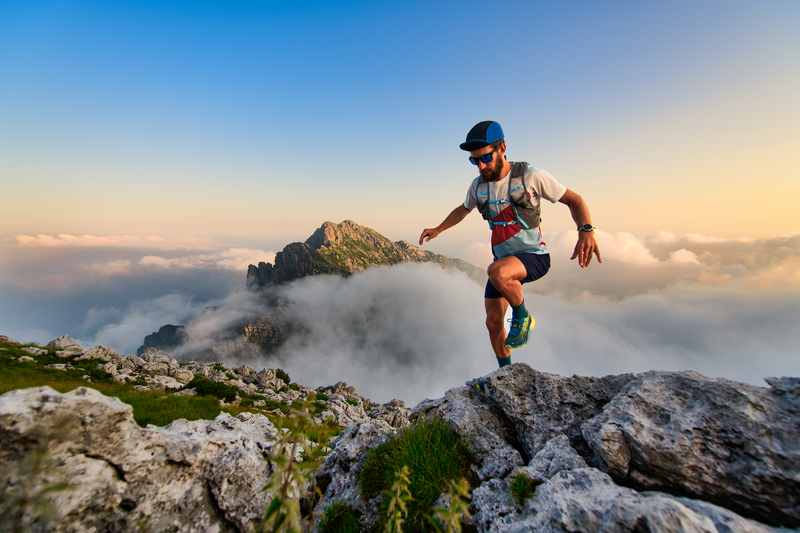
94% of researchers rate our articles as excellent or good
Learn more about the work of our research integrity team to safeguard the quality of each article we publish.
Find out more
ORIGINAL RESEARCH article
Front. Mar. Sci. , 11 December 2023
Sec. Deep-Sea Environments and Ecology
Volume 10 - 2023 | https://doi.org/10.3389/fmars.2023.1204992
Chemosymbiotic species inhabiting deep-sea hydrothermal vents are known to rely on microbial symbionts for nutrition. However, the relative contributions of heterotrophic energy sources to their diets remain poorly understood. In this study, we investigate the trophic positions (TP) of symbiont-bearing taxa, including vent mussels, snails, and shrimps, and examine the contribution of copepods and detrital organic matter (OM) to the food chain. Amino acid nitrogen isotopic compositions (δ15NAA) were used to investigate the TP of vent mussels (Bathymodiolus septemdierum and Gigantidas vrijenhoeki), snails (Alviniconcha spp.), and shrimps (Alvinocaris sp. and Rimicaris kairei) from two different vent environments. δ15NAA values in copepods and OM were also measured. Microbial resynthesis index (ΣV) was calculated to predict the contribution of reworked OM as an energy source to the hydrothermal vent ecosystem. Variations in TP were observed among vent mussels and snails from different vent environments, with higher TP in species from diffusing vents than in those from black smoker vents. Shrimps dwelling in a single diffusing vent exhibited distinct TP, suggesting that microhabitat and phylogeny may influence their energy acquisition. Notably, copepods occupied higher TPs than expected, possibly owing to the consumption of detrital OM. Our findings provide new insights into the trophic diversity of chemosymbiotic species in deep-sea hydrothermal vents and demonstrate the utility of δ15NAA analysis as a tool for unraveling food web dynamics and ecosystem functioning in these unique environments.
The discovery of lightless ecosystems in hydrothermal vents in the Galapagos Ridge in 1977 revolutionized our understanding of primary production on Earth (Lonsdale, 1977; Corliss et al., 1979; Jannasch and Wirsen, 1979; Fisher et al., 1988; Childress and Fisher, 1992). A notable feature of these ecosystems is the symbiotic partnership between chemosynthetic bacteria and their eukaryotic hosts (Childress and Fisher, 1992). Vent microbes, including both free-living and symbiotic bacteria, fix CO2 using the energy produced during the redox reaction of inorganic substrates, such as sulfide or methane from vents (Rau and Hedges, 1979; Rau, 1981a). Host animals harvest symbionts with the advantage of gaining access to energy sources that they cannot use directly; however, the relative contribution of heterotrophic energy sources and nutrients consumed by hosts to meet their nutritional demands remains unknown.
Understanding the nutritional relationship between symbiotic bacteria and host animals is critical for comprehending the energy flow and community structure of hydrothermal vent ecosystems. Prior studies utilized stable isotopic compositions, fatty acids, stomach content analysis, and observations to unravel nutritional sources and trophic relations in vent fauna (Kennicutt et al., 1992; Fisher et al., 1994; Pond et al., 1997; Polz et al., 1998; Colaço et al., 2002; Naraoka et al., 2008; Erickson et al., 2009; Wang et al., 2018; Salcedo et al., 2021; Suh et al., 2022a). Among these methods, bulk carbon (δ15Cbulk) and nitrogen isotope (δ15Nbulk) values have been extensively utilized in investigating vent food webs (Fisher et al., 1994; Colaço et al., 2002; Suh et al., 2022a). These methods offer the advantage of elucidating diet compositions over preceding weeks or months. Isotopic signatures demonstrate a consistent fractionation of approximately 1‰ for carbon and 3-4‰ for nitrogen between prey and predator, thereby enabling the interpretation of prey-predator relationships (Post 2002).
However, δ15Cbulk and δ15Nbulk values are not exclusively sensitive to TP; they are also influenced by the energy source and metabolic pathways of organisms. Although the isotope fractionation in δ15Cbulk values in vent fauna are relatively well known, the factors that affect δ15Nbulk values are poorly understood. Vents have both, inorganic (NH4+, NO3-, NO2-, and N2) and organic nitrogen (dissolved organic nitrogen and amino acids) sources (Bourbonnais et al., 2012), which may have distinct δ15Nbulk values and isotope fractionation during nitrogen cycling (Bourbonnais et al., 2012; Charoenpong, 2019). For instance, vent fauna that mainly utilize NO3- have δ15Nbulk values above 5‰, whereas those that assimilate ammonium have values below 5‰ (Lee and Childress, 1994; Bourbonnais et al., 2012). Furthermore, N2 fixation has been reported to reduce δ15N values (Fry, 2006), and ammonium assimilation can cause fractionation up to 20-30‰ depending on the NH4+ concentration (Bourbonnais et al., 2012; Portail et al., 2018), although in situ quantification is yet to be established. The presence of various nitrogen sources utilized by vent fauna, coupled with the uncertainty in δ15N values, poses a challenge when using δ15Nbulk values to accurately estimate their TP. If the TP of basal consumers is not accurately established, the entire food web structure may be poorly understood.
For example, δ15Nbulk values of vent mussels exhibit variability across mussel populations among distinct vent sites (Colaço et al., 2002; Naraoka et al., 2008; Van Audenhaege et al., 2019). It is challenging to determine whether such fluctuations arise from variation in source δ15N values or TP solely based on δ15Nbulk values. To address this, Vokhshoori et al. (2021) employed amino acid nitrogen stable isotope (δ15NAA) analysis to unravel dietary characteristics of a mussel (B. childressi) from seep sites located at water depths of 390-1490m. This investigation revealed that B. childressi exhibits mixotrophic behavior, with the contribution of heterotrophic sources linked to venting intensity and mussel bed size. Similarly, Alviniconcha snails also harvest symbiotic bacteria from their gill tissues and are often categorized as primary consumers (Suzuki et al., 2005a; Suzuki et al., 2005b; Suzuki et al., 2006). While several studies reported carbon fixation pathways of Alviniconcha snails’ symbionts (Suh et al., 2022b; Jang et al., 2023), investigation of the precise TP of the host snail remains limited.
In this study, the TP of ecologically important and symbiont-bearing vent species, such as vent mussels (Bathymodiolus septemdierum and Gigantidas vrijenhoeki), hairy snails (Alviniconcha spp.), and shrimps (Rimicaris kairei and Alvinocaris sp.), were estimated using δ15NAA. The advantage of δ15NAA analyses is that they provide TPs for organisms, from primary producers to top predators, without requiring source nitrogen δ15N values (Chikaraishi et al., 2009; McMahon and McCarthy, 2016; Ohkouchi et al., 2017). This study also compared congeners collected from two ecologically different vent sites (a diffusive vent in the Central Indian Ridge and a black smoker vent from the North Fiji Basin, Southwest Pacific) to investigate environmental impacts on TP and reveal phylogenetic variation in the TP of shrimps within a single vent site. Additionally, detritus and copepod spp. were collected and analyzed for δ15NAA values to examine their contribution to the macrofaunal community as possible energy sources.
Microbes play crucial roles in energy production and decomposition in vents. To understand the consumption of microbially resynthesized energy by host taxa, the study calculated δ15NAA based ΣV parameter, a proxy for total heterotrophic resynthesis proposed by McCarthy et al. (2007). If symbiont hosts consume microbially resynthesized energy in addition to the energy newly synthesized by symbionts, this suggests that they play a dual role as primary consumers and detritivores, contributing to vent energy recycling. The use of δ15NAA values as an indicator of chemosynthetic sources and mechanisms of nitrogen utilization has been proven in cold seeps (Vokhshoori et al., 2021; Wang et al., 2022) and shallow water hydrothermal vents (depth > 30m) (Chang et al., 2018), This study is the first to explore TP in deep-sea (> 2,000 m) hydrothermal vents with no or negligible contribution of photosynthetic OM. We believe that this study contributes to improving the interpretation of δ15NAA values to better understand the function and energetics of deep-sea hydrothermal vent ecosystems.
The Onnuri Vent Field (OVF; 11°24.9′ S, 66°25.4′ E) is a diffusive vent located in the Central Indian Ridge characterized by high biological density, high concentrations of dissolved methane, and a lack of plume particles (Kim et al., 2020; Suh et al., 2022b). During the KIOST Indian Ocean cruise conducted in June 2018 using R/V Isabu, megafaunal samples were collected from the OVF using a video-guided hydraulic grab (Oktopus, Germany). This study focused on analyzing a few symbiont-bearing endemic vent fauna species, including two mussel species (Gigantidas vrijenhoeki and Bathymodiolus septemdierum), a hairy snail (Alviniconcha marisindica), and two shrimp species (Rimicaris kairei and Alvinocaris sp.). In November 2021, the OVF was revisited using a remotely operated platform for ocean science (ROPOS) to collect samples of possible dietary sources of megafauna by suctioning settled particles and OM in and around the biological community. The copepod Stygiopontius spinifer (Lee et al., 2020) was manually isolated from the suctioned sample, and the remaining suctioned sample was size-fractioned using nested sieves of 100 μm and 38 μm mesh. The particles with a size between 38 and 100 μm were transferred to pre-ashed (450°C, for 5h) glass jars and the remaining particles smaller than 38μm size (detritus hereafter) was filtered using pre-ashed (450°C, for 5h) 47 mm GF/F. Bacterial mats on the outer shells of the mussel B. septemdierum were collected separately and stored in pre-ashed (45°C, for 5h) glass vials. All samples were stored at -80°C until analysis.
Mussels (B. septemdierum) and snails (Alviniconcha boucheti) were collected from an active black smoker chimney (R1964; 18° 51′ S, 173° 29′ E) located in the North Fiji Basin (NFB) to compare their trophic niches with congeners from the OVF diffusing vent. Samples were collected using ROPOS ROV during the KIOST expedition cruise in November and December 2016. Additionally, the copepod Isaacsicalanus paucisetus was sampled using a suction sampler of the ROPOS ROV from a diffusing vent (R1971; 16° 59′ S, 173° 55′ E) in the NFB (Park et al., 2020). Megafaunal density was higher in the diffusing vents (OVF and R1971) than in the active black smoker vent (R1964) based on the ROV video camera observation. Upon collection, megafauna samples were frozen at – 80°C and copepods were preserved in 4% formalin. Unfortunately, there were no frozen samples of copepod or settled particulate OM from the NFB for bulk isotope analyses.
We compared the stable isotopic compositions of carbon (δ13C) and nitrogen (δ15N) between the gill and muscle tissues of mollusks collected from the NFB, specifically B. septemdierum and A. boucheti. The isotopic values for the gill and muscle tissues of mollusks from OVF have already been reported in Suh et al. (2022b). The tissues were freeze-dried (FD8518, Ilshin Lab Co., Ltd., Korea) at – 80°C and 5 mTorr for 72 h and homogenized. Subsequently, the samples were treated with a mixture of dichloromethane (DCM): Methanol (MeOH) (2:1, v/v) with sonication to facilitate the lipid removal, following the method described by Bligh and Dyer (1959) with modifications. The copepods (S. spinifer) from the OVF was freeze-dried and defatted. Particle samples and bacterial mats from the OVF were freeze-dried and treated with 1N HCl to remove inorganic carbon. Lipid extraction and acid treatment were not performed for δ15N analysis to prevent treatment effects (Kim et al., 2016). The samples were wrapped in tin capsules for stable isotope analysis.
Stable isotope ratios were determined using an Elemental Analyzer (Vario MicroCube Elementar, Germany) connected to an isotope ratio mass spectrometer (Isoprime Vision Plus, Elementar GV Instrument, Germany). Isotope ratios are reported per mille (‰) using standard delta notation. The carbon and nitrogen references were Vienna PeeDee Belemnite and atmospheric nitrogen (N2), respectively.
The stable isotope ratios of each element were expressed as values relative to international reference materials as follows (1):
Where R = 13C/12C or 15N/14N
Reference materials were IAEA-CH-6 (Sucrose, -10.449‰) for 13C/12C, and IAEA-N-1 (Ammonium sulfate, 0.4‰) for 15N/14N. The analytical precision was within 0.1‰ for C and 0.2‰ for N.
Water samples were collected from the OVF in June 2022, using Niskin bottles attached to the ROPOS ROV. Water samples were then analyzed for inorganic nitrogen concentrations, including NH4+, NO2-, and NO3-, using a Smart Chem200 nutrient auto-analyzer (Proxima, Alliance Instruments, Italy). The nutrient concentrations in the NFB were previously reported by Suh et al. (2022a).
Powdered biota samples were used to measure the nitrogen isotope ratios of individual amino acids (AAs). Each sample (approximately 3 mg) was hydrolyzed for 12-24 h with 6M HCl at 110°C. Subsequently, the hydrophobic content of the samples was removed using 6:5 n-hexane/DCM (v/v). The residual HCl was dried under the flow of N2 gas at 70°C. Next, AA molecules were derivatized through N-pivaloyl-AA-isopropyl ester using 1:4 thionyl chloride/isopropanol (v/v) at 110°C for 2 h. and 1:4 pivaloyl chloride/DCM (v/v) at 110°C for 2 h in sequence. The AA derivatives were extracted with 6:5 n-hexane/DCM (v/v) to remove the residual hydrophilic content. The AA derivatives were redissolved in DCM and stored in a freezer at -20°C before analysis.
The δ15NAA values were measured using a gas chromatograph (HP 6890N, Agilent, US) connected to a furnace (GC5 Interface, Elementar, Germany) and an isotope ratio mass spectrometer (Isoprime 100, Elementar, Germany). The analytical precision of δ15N values was checked every 5–6 runs of samples, using standard mixture of nine AAs, including alanine, glycine, valine, leucine, norleucine, aspartic acid, methionine, glutamic acid, and phenylalanine (SHOKO-Science and Indiana University). During the analysis, δ15NAA values of standard mixture showed a standard deviation of less than 1‰.
We used the following equations proposed by Chikaraishi et al. (2009) to calculate TP based on δ15N values of the AAs:
Where δ15NGlu and δ15NPhe are nitrogen isotope values of Glu and Phe, respectively. β denotes the difference in δ15N values between four trophic AAs and Phe of primary producers, and TDF is the trophic enrichment factor of δ15NTrp-Phe relative to δ15NPhe in a trophic transfer. As no isotope fractionation data from chemosynthetic ecosystems were available, we used previously published values of βGlu-Phe (3.4‰; Chikaraishi et al., 2009) and TDFGlu-Phe (7.6‰; Vokhshoori et al., 2021). Although the absolute TP values may change slightly, we believe that the interpretation of the overall trend remains unaffected.
For chemosynthetic ecosystem consumers, including this study, Leu and Ile showed low δ15N values, and Pro exhibited notably high δ15N values (Vokhshoori et al., 2021), unlike the consumers from photosynthetic ecosystems. Thus, we compared TPs estimated using three different sets of trophic AAs with the TPGlu-Phe values, each employing a varying set of trophic AAs and corresponding βTrp-Phe and TDFTrp-Phe values sourced from Chikaraishi et al. (2009): inclusion of (1) all six trophic AAs (βTrp-Phe = 3.3‰, TDFTrp-Phe = 5.4‰), (2) Ala, Glu, Val, and Pro (βTrp-Phe = 3.6‰, TDFTrp-Phe = 5.9‰), and (3) Ala, Glu, and Val (βTrp-Phe = 3.7‰, TDFTrp-Phe = 6.0‰). δ15NTrp indicates the average nitrogen isotope values of trophic AAs. Moreover, we calculated propagation of errors on TP values using the equation in Jarman et al. (2017).
We also calculated ΣV parameter using six trophic amino acids (Ala, Glu, Ile, Leu, Pro, and Val), as an indication of microbial AA resynthesis, following the equation proposed by McCarthy et al. (2007):
where χAA is the deviation of each trophic AA = δ15NAA - average δ15N of trophic AAs, and n is the number of trophic AAs used in the calculation. However, variability in ΣV values in hydrothermal vent consumers would vary by characteristic distributions of δ15N values of trophic AAs (Vokhshoori et al., 2021). Consequently, it becomes challenging to discern whether high ΣV values are a result of microbial resynthesis or species-specific metabolism. We thereby calculated another parameter to evaluate the average isotopic offset in low δ15NAA values (Leu and Ile) within trophic AAs of hydrothermal vent consumers from the high δ15NAA (Ala, Val, Pro, and Glu) using the following equation:
Among trophic AAs, high δ15NPro and low δ15NLeu and δ15NIle values may serve as distinctive δ15N patterns indicative of methane-oxidizing bacteria, as proposed by Vokhshoori et al. (2021). Thus, we anticipate that a reliance on hydrothermal vent microbes among consumers would be reflected in both ΣV and Leu-Ile index.
The statistical analysis of variance in mean TP of vent fauna was performed using the statistical software package JMP (version 10.0, SAS Institute Inc., Cary, NC, USA). The mean TPs of the three bivalve mussel species were compared using ANOVA (p< 0.05) and Tukey-Kramer HSD test (p< 0.05; See Supplementary Material), whereas snails, shrimps, and copepods were compared using the t-test (p< 0.05).
The muscle δ15N values of the snail A. boucheti and the mussel B. septemdierum from the NFB were 11.3 ± 0.2‰ and 10.8 ± 1.9‰, respectively, which are consistently higher than their respective gill δ15N values by 0.5‰ and 0.4‰ on average (Supplementary Table A1). However, there was no clear pattern observed for δ13C values between muscle and gill tissues of the gastropods, with differences ranging from -0.06 to 0.7‰.
The detritus (0.7–38 μm) samples (Figure 1A) had a mean δ13C value of -22.7 ± 0.4‰, but had no detectable δ15N values (Table 1). Settled particles with a larger size range (38–100 μm) had mean δ13C value of -17.5 ± 0.0‰, which is ~5‰ higher than detritus (-22.7 ± 0.4‰), with δ15N value of 2.8 ± 0.0‰. The bacterial mats found on the outer shells of B. septemdierum mussels (Figure 1C) had δ13C and δ15N values of -27.0‰ and 3.3 ± 0.5‰, respectively. Furthermore, the copepod S. spinifer (Figure 1B) had mean δ15N value of 10.8 ± 0.1‰, which is the highest value among the community members, with also relatively high δ13C values (-12.8 ± 0.1‰).
Figure 1 (A) Suction sampling on and around biological community in the Onnuri Vent Field (OVF), (B) copepod Stygiopontius spinifer collected from the OVF, and (C) bacterial mats on the outer shells of Bathymodiolus septemdierum mussels.
Table 1 Bulk carbon (δ13C) and nitrogen (δ15N) isotopic compositions of the copepod Stygiopontius spinifer and particulate organic matter suction sampled near the biological community as well as bacterial mats on outer shells of Bathymodiolus septemdierum mussels (See Figure 1).
The δ15NAA values for mollusks, shrimps, copepods, and detritus ranged from -14.2‰ to 25.2‰ (Figure 2, Supplementary Table A2). The copepod I. paucisetus and shrimp R. kairei had only positive δ15NAA values, with I. paucisetus yielding 3.4 to 23.9‰ and R. kairei yielding 2.6 to 25.2‰. The mean δ15NAA values of trophic AAs (Glu, Ala, Ile, Leu, Pro, Val) ranged from -2.1 ± 1.4 ‰ (shrimp Alvinocaris sp.) to 18.7 ± 0.5‰ (copepod I. paucisetus), having consistently higher average δ15NAA values than source AAs (Gly, Ser, Met, Phe) by 5.5‰ to 12.6‰, with some variation by species. The δ15NAA offset between trophic AAs and source AAs was higher in A. marisindica snail and I. paucisetus copepod, with offsets of 12.6 ± 0.6‰ and 11.2 ± 0.5‰, respectively, whereas detritus and shrimp Alvinocaris sp. had smaller offsets of 5.5 ± 0.8‰ and 7.6 ± 0.4‰, respectively.
Figure 2 Individual amino acid nitrogen isotope values (δ15N) of vent fauna from the Onnuri Vent Field and North Fiji Basin (R1964, R1971) for two or three individual specimens for each species. Gray bars indicate standard deviation. Amino acid abbreviations are: Glu, glutamic acids; Ala, alanine; Ile, isoleucine; Leu, leucine; Pro, proline; Val, valine; Gly, glycine; Ser, serine; Met, methionine; Phe, phenylalanine.
Furthermore, δ15NLeu and δ15NIle values were consistently lower than other trophic AAs in all species and detritus samples, and these values were lower than δ15NGlu (ΔGlu-Leu and ΔGlu-Ile) by 5.8 ± 3.6‰ and 6.6 ± 3.6‰ on average, respectively. The shrimp R. kairei, and copepods I. paucisetus and S. spinifer had a greater difference in ΔGlu-Leu and ΔGlu-Ile values than the B. septemdierum mussels, ranging from 11.3 ± 1.2‰ to 15.0 ± 1.6‰. Additionally, we found scattered δ15NPro values against δ15NGlu (ΔGlu-Pro) among vent fauna, with A. boucheti snail and B. septemdierum mussel having higher δ15NPro values than δ15NGlu values by 9.1 ± 0.6‰ and 7.4 ± 0.9‰, respectively, while a copepod S. spinifer and snail A. marisindica had 15N-depletion in δ15NPro values than δ15NGlu by 6.0 ± 1.0‰ and 5.5 ± 1.4‰, respectively. The other vent fauna had ΔGlu-Pro values in-between.
The TPTrp-Phe values calculated using three trophic AAs (Ala, Glu, and Val) have a close alignment with 1:1 line and demonstrate a strong correlation with TPGlu-Phe values, compared to the other two estimations of TPTrp-Phe values involving Leu, Ile, and Pro (Figure 3). This discrepancy arises due to the notable variability of the three trophic AAs (Leu, Ile, and Pro) among species. While there may be marginal fluctuations in the absolute TPTrp-Phe values derived from the three trophic AAs against TPGlu-Phe values, the overall trend remains consistent and without significant difference. Consequently, our TP dataset will be predicted using TPGlu-Phe values.
Figure 3 Relationship between TPTrp-Phe and TPGlu-Phe calculated by three different sets of trophic AAs and corresponding βTrp-Phe and TDFTrp-Phe values sourced from Chikaraishi et al. (2009). Dotted line denote 1:1 line, where TPTrp-Phe is equal to TPGlu-Phe.
The TPGlu-Phe ranged from 1.8 ± 0.2 to 3.3 ± 0.1 (Figure 4, Table A2). The two mussel species found in the OVF, G. vrijenhoeki and B. septemdierum, had different mean TPs, with the former having a value of 1.8 ± 0.2, and the latter having a slightly higher value of 2.3 ± 0.2. The mussel B. septemdierum found in the NFB had an intermediate mean value of 2.0 ± 0.4. However, their variation was not statistically significant (ANOVA, p< 0.109; Supplementary Material). Among mollusks, A. marisindica snail from the OVF had the highest TP of 3.1 ± 0.1, while A. boucheti snail from NFB had a slightly lower value of 2.2 ± 0.1, with statistically significant difference (t-test, p< 0.001). The two shrimp species found in the OVF had significantly different TPs (Alvinocaris sp. = 1.8 ± 0.1; R. kairei = 3.3 ± 0.1; t-test, p< 0.001), while the copepods found at the two vent sites had similarly high TPs of 2.9 ± 0.1 (OVF) and 3.2 ± 0.1 (NFB), with statistical difference (t-test, p< 0.035). The detritus collected from the OVF had the lowest TP at 1.5 ± 0.1.
Figure 4 Trophic position of vent fauna from the Onnuri Vent Field and North Fiji Basin (R1964, R1971). Gray bars indicate standard deviations calculated using propagated errors (Jarman et al., 2017).
The δ15N of muscle tissues in A. boucheti snail and B. septemdierum mussel from the NFB were higher compared to their gill tissues, which corresponds to the values observed in the OVF, where another snail species (A. marisindica), and mussel species B. septemdierum and G. vrijenhoeki had consistently higher muscle δ15N values than gill tissues by 1.0 to 2.2‰ (Suh et al., 2022b), indicating a high transfer of nitrogen from the endosymbiotic bacteria to the host. Conversely, the δ13C values of gill and muscle tissues did not exhibit consistent patterns, having offsets between -0.6 and 0.7‰ in this study and in Suh et al. (2022b). If muscle tissues are 13C-enriched in δ13C values than gill tissues, it implies that carbon source for hosts is likely originating from endosymbiotic bacteria within the gill tissues. Since we observed both high and low δ13C values in muscle tissues of host individuals relative to those in gill tissues, hosts have the capacity to access carbon not only from endosymbiotic bacteria but also from ambient materials that they may have filter-fed, including POM, detritus, or free-living bacteria that could be either heterotrophs or autotrophs. The δ13C and δ15N values of bacterial mats (Table 1) scraped off the outer shells of mussel B. septemdierum suggested that hosts might use them as a carbon source but not for nitrogen metabolism, as indicated by a large δ15N offset between the mussels and bacterial mats (offset = 13-18‰). This suggests that B. septemdierum has different energy sources or different proportions of carbon and nitrogen, as suggested for other vent fauna (Lee and Childress, 1994).
The δ13C values of snails (Alviniconcha spp.) and mussels (B. septemdierum) showed only a small variation (~1‰) between the NFB and OVF, indicating that the carbon fixation pathways of the endosymbionts were consistent across both sites despite differing environmental conditions. However, there was a significant difference in δ15N values of B. septemdierum between the two vents, with NFB having a mean δ15N value of 2.4 ± 0.6‰ (Suh et al., 2022a) and OVF having a mean δ15N value of -12.1 ± 2.2‰ (Suh et al., 2022b). In contrast, the δ15N values of Alviniconcha spp. did not show such a variation. Low δ15N values in mussels from different vent locations (Colaço et al., 2002; Naraoka et al., 2008; Van Audenhaege et al., 2019) have frequently been attributed to incomplete utilization of NH4+ by bacteria (Rau, 1981b; Van Dover and Fry, 1994; Liao et al., 2014). Seawater samples from both vents were found to have similarly low NH4+ concentrations (Table 2), suggesting that endosymbionts may utilize biologically produced ammonium as the main source of nitrogen metabolism instead of using it in seawater. The observation of higher mussel density in the OVF, which has a higher biological production of ammonium, provides further evidence to suggest that lower δ15N values are associated with biomass (Demopoulos et al., 2019). Another potential factor contributing to 15N-depletion in vent or seep fauna is N2 fixation, which can occur at sites with methane availability (Dekas et al., 2014), such as OVF. This suggestion can be tested using metagenomic analysis.
Table 2 Nutrient concentrations (μM) in the water collected from the Onnuri Vent Field and North Fiji Basin (Suh et al., 2022a).
The copepod S. spinifer from the OVF exhibited the highest δ15N values (10.8 ± 0.1‰), surpassing those of the shrimp R. kairei (7.4 ± 1.8‰; Suh et al., 2022b) and vent crab Austinograea rodriguezensis (4.3 ± 2.0‰; Suh et al., 2022b) that are known to be omnivores or carnivores. This copepod species was found residing near an A. marisindica snail community and may have obtained some of its carbon energy from the snails, as evidenced by the similar δ13C values between the two species (A. marisindica = -11.4 ± 0.6‰ (Suh et al., 2022b); S. spinifer = -12.8‰ (this study)). Moreover, the copepods S. spinifer and R. kairei utilized isotopically similar sources of carbon and nitrogen, which was supported by the frequent clustering of R. kairei around the A. marisindica snail community.
Previous studies on vent fauna from the OVF and NFB (Suh et al., 2022a; Suh et al., 2022b) were unable to accurately determine the TP because of uncertainties in the source nitrogen δ15N values and isotope fractionation during chemosynthetic metabolism. In this study, we employed the δ15NAA values to estimate the TP of the vent fauna (Figure 4, Table A2). Our results revealed that the two mussel species from the OVF (B. septemdierum and G. vrijenhoeki) have slightly different TP (2.3 ± 0.2 and 1.8 ± 0.2, respectively), although the difference was not statistically significant (ANOVA, p = 0.101). Furthermore, the δ15NPhe values of B. septemdierum (-1.7 ± 0.8‰) and G. vrijenhoeki (-5.6 ± 0.5‰) suggest that the two species have different nitrogen sources (Figure 5). We posit that this variation could be attributed to differences in their associated symbionts, potentially influencing the nitrogen assimilation. Jang et al. (2020) reported that B. septemdierum from the OVF forms a symbiotic relationship with Candidatus Thioglobus, while G. vrijenhoeki is associated with Thioglobus-related Gammaproteobacteria, Methyloprofundus-related Gammaproteobacteria, and Sulfurovum-related Gammaproteobacteria. Different bacterial diversity in G. vrijenhoeki and B. septemdierum can lead to different nutrient synthesizing capability. We speculate that the slightly higher TP in B. septemdierum could be attributed to energy acquisition not solely from the endosymbionts but also from ambient materials including heterotrophic sources and/or detritus. Conversely, G. vrijenhoeki mainly relies on energy sources derived from its symbionts.
Figure 5 δ15NPhe values, proxy for baseline δ15N values, of vent fauna from the Onnuri Vent Field and North Fiji Basin (R1964, R1971). Gray bars indicate standard deviation.
Another factor to consider when interpreting trophic relationships between endosymbionts and host animals is the nutrition transfer mechanism that can lead to variations in the TP of the host animal as suggested by Cavanaugh et al. (2006). In one scenario, metabolites synthesized by the autotrophic endosymbionts undergo direct assimilation within the host animal’s tissues, resulting in the TP of the host indicative of a primary producer. Another scenario involves the digestion of endosymbiont cells by the host, leading to the TP of the host reflecting that of a consumer, rather than a primary producer. In both scenarios, the host animal’s nutrition ultimately comes from the autotrophic endosymbiont. However, the key distinction lies in the processing of the metabolites synthesized by the endosymbiont. These scenarios hold particular significance for herbivores (TP ≈ 2) that mainly rely on endosymbionts for energy and nutrients, as opposed to the omnivores (TP ≥ 2.5) that assimilate heterotrophic or detrital matter as part of their energy sources. Given the available data, the precise mechanism governing the nutrient transfer between endosymbionts and host animals remains challenging to ascertain. However, we note that these underlying factors may partially influence TP variations in herbivores such as the shrimp Alvinocaris sp., as well as the mussel species G. vrijenhoeki and B. septemdierum in this study.
The TP of mussel B. septemdierum in the NFB varied greatly from 1.6 to 2.3, suggesting that there is a high degree of intra-species nutritional variability, ranging from consuming symbiont-supported nutrition to ambient sources. This could also be linked to changes in feeding behavior during growth, as smaller mussels (6.7 cm) had a lower TP (1.6) than larger mussels (TPs < 2.0). Previous research has shown that mollusks can shift their diet depending on their body size, although this pattern varies among different locations (Van Dover, 2002; Reid et al., 2016; Reid et al., 2020). Owing to the small sample size (n = 3) and small variation in shell size (~1.3 cm), further investigation is necessary to confirm trophic plasticity in vent mussels. Nevertheless, it is clear that Bathymodiolus species in the OVF and NFB feed on both symbiont-derived nutrition and ambient materials (non-symbiont microbes and detritus), with some intra-specific variation.
Several studies have reported on the diversity of symbiotic bacteria and their carbon fixation pathways in Alviniconcha snails, but little is known about the contribution of heterotrophic energy to the host and their TP (Suzuki et al., 2005a; Suzuki et al., 2005b; Suzuki et al., 2006; Erickson et al., 2009; Sanders et al., 2013; Beinart et al., 2019; Van Audenhaege et al., 2019; Lee et al., 2021). Based on this study’s results, A. marisindica in the OVF is an omnivore (TP = 3.1 ± 0.1) that appears to consume detrital sources as part of their diet. The snails (A. boucheti) in the NFB, however, is not as omnivorous (TP = 2.2 ± 0.1) as their OVF counterparts (t-test, p< 0.0001). The reason for this variation between sites may be that OVF has higher biomass, which in turn leads to larger amounts of detritus or heterotrophic OM. This allows organisms to occupy higher TPs because they consume a greater proportion of heterotrophic or detrital OM. We also cannot rule out the variation in gill-associated symbiotic bacteria between the two Alviniconcha snail species that may influence their energy acquisition. The predominant symbiotic bacteria of A. marisindica snails from the OVF was Sulfurovum-related Campylobacteria (Jang et al., 2023), whereas the symbiotic bacteria associated with A. boucheti snails was Epsilonproteobacteria genus Sufurimonas (unpublished data).
Suh et al. (2022b) classified the shrimp Alvinocaris sp. as an omnivorous and opportunistic feeder in the OVF based on its isotopic affinity to omnivores, such as Munidopsis sp., and sedimentary OM, which represents the average value of the available food sources in the community. However, in this study, we discovered that Alvinocaris sp. is a primary consumer (TP = 1.8 ± 0.1) that mostly relies on symbiotic bacteria for energy (Tokuda et al., 2008; Sun et al., 2016). However, the shrimp R. kairei had the highest TP (3.3 ± 0.1) among all the species we analyzed, indicating that they are scavengers or carnivores. One notable pattern that we observed was that organisms that were often clustered together had similar TPs. For example, the TP of Alvinocaris sp. shrimps was similar to that of the G. vrijenhoeki mussel (TP = 1.8 ± 0.2), and we collected them from the same locality. The R. kairei shrimps were consistently found in hot shimmering areas, and the same was true for A. marisindica snails, which are also scavengers with a high TP (3.1 ± 0.1). We speculate that not only the feeding guild but also the energy availability in the microhabitat in which they choose to reside may influence their trophic niche (Versteegh et al., 2022). Variations in symbiotic bacterial community may also have influenced energy assimilation of the host species. Although this study did not analyze symbiotic bacterial diversity in shrimps, a recent study reported a dominance of Campylobacterota symbionts in R. kairei from the Indian Ocean (Methou et al., 2022). To our knowledge, no reports exist regarding the symbiotic bacterial diversity of Alvinocaris sp. from the Indian Ocean. However, it is noteworthy that an Alvinocaris congener from Okinawa Trough (A. longirostris) exhibited the prevalence of Sulfurovum spp. of Epsilonproteobacteria as gill symbionts (Tokuda et al., 2008; Sun et al., 2016).
The copepods had a high TP (2.9 ± 0.1 in OVF, 3.2 ± 0.1 in NFB), contrary to our expectation of them being a link between primary producer and higher TP consumers. The baseline δ15N values of S. spinifer copepods and R. kairei shrimps in the OVF were similar (S. spinifer δ15NPhe = 3.9 ± 0.1, R. kairei δ15NPhe = 3.6 ± 0.8), suggesting that they had similar diet sources. Moreover, the copepod S. spinifer was found around Alviniconcha snails, where Rimicaris shrimp was also present, indicating that they utilized detritus or heterotrophic sources available near Alviniconcha snail aggregates. The copepod I. paucisetus from the diffusive vent in the NFB was also classified as a detritivore or carnivore (TP = 3.2 ± 0.1), and we speculate that they consume detritus derived from the megafaunal community, positioning themselves above the mussels (B. septemdierum) and the snails (A. boucheti).
Trophic AAs were more enriched in 15N than source AAs in the vent fauna, as observed in all heterotrophs. However, the variation in δ15N of Leu and Ile compared to that of Glu (ΔGlu-Leu and ΔGlu-Ile, respectively; Figures 6A, B) in some vent fauna differed from that typically observed in heterotrophs from other marine environments. Leu and Ile had lower δ15N values compared to Glu, with offsets ranging from 1.9‰ to 15.0‰ for ΔGlu-Leu and from 1.5‰ to 13.3‰ for ΔGlu-Ile on average. This finding is consistent with those of Vokhshoori et al. (2021), that reported low δ15NIle and δ15NLeu values in seep mussels and to a lesser extent, in bacteria and archaea (Yamaguchi et al., 2017). We suggest that this could be due to isotope fractionation during the utilization of Glu to synthesize other AAs as bacteria can utilize Glu to resynthesize other AAs (Davies and Humphrey, 1978; Ponnudurai et al., 2020). The transamination and deamination processes involved in this metabolic pathway preferentially incorporate lighter isotopes, consequently increasing the δ15N values of the remaining “Glu pool.” Furthermore, the high energetic costs associated with the degradation of Ile and Leu in organisms can result in 15N-depletion in these AAs (Akashi and Gojobori, 2002).
Figure 6 Nitrogen isotopic offsets of Leu (A), Ile (B), and Pro (C) against Glu versus trophic position of vent fauna from the Onnuri Vent Field and North Fiji Basin (R1964, R1971). ΔGlu-Leu = δ15NGlu – δ15NLeu; ΔGlu-Ile = δ15NGlu – δ15NIle, ΔGlu-Pro = δ15NGlu – δ15NPro. Linear regression coefficient (r2) is indicated for each plot.
Another distinct δ15NAA pattern observed in vent fauna is the variable offsets of δ15N values of Pro relative to Glu (ΔGlu-Pro), which ranged from -9.1 ± 0.6‰ to 6.0 ± 1.0‰ across different species (Figure 6C). Low ΔGlu-Pro values were found in B. septemdierum mussel (-7.4 ± 0.9‰) and A. boucheti snail (-9.1 ± 0.6‰), while high values were recorded in A. marisindica snail (5.5 ± 1.4‰) and S. spinifer copepod (6.0 ± 1.0‰), with other organisms falling in-between. The underlying mechanism behind such scattered δ15NAA values among species is still unclear. However, we propose plausible explanations based on the hypotheses presented by Ohkouchi et al. (2017) on scattered δ15NAA patterns. First, the variability in δ15NAA values may arise from differences in the quality of OM substrates utilized by microorganisms in the energy biosynthesis process, which are subsequently incorporated into vent fauna (Chikaraishi et al., 2015). Second, it is possible that only selected AAs were microbially resynthesized, resulting from a combination of de novo AA synthesis from inorganic nitrogen and salvage incorporation of AAs. This scenario is likely to occur in hydrothermal vents owing to the availability of abundant inorganic nitrogen sources (NO3-, NO2-, N2 gas, and NH4+) and high biomass concentrations. Third, differences in the metabolic pathways of AAs among species could also contribute to variation in δ15NAA values. Based on the large variation in bulk δ15N values observed in vent fauna (-12.1 to 10.8‰ in OVF and -0.8 to 10.4‰ in NFB), it is inferred that multiple nitrogen sources and metabolic pathways are utilized by vent fauna in a single vent field.
A strong correlation between ΣV and Leu-Ile index has been demonstrated (r2 = 0.91), suggesting that the low δ15N in Leu and Ile play a substantial role in the variance within trophic AAs (Figure 7) as was also reported in Vokhshoori et al. (2021). These patterns have been observed in all vent fauna, with some variation in the extent of 15N-depletion in δ15N of Leu and Ile between species. We found a high ΣV value in detritus (3.4 ± 0.2), indicative of a substantial contribution from reworked OM by heterotrophic bacteria in the OVF. Vent fauna with ΣV values lower than those of detritus, such as Alvinocaris sp. and G. vrijenhoeki, may have minimal dependence on detrital OM, whereas those with ΣV values similar to or higher than those of detritus likely have mixed dependence on symbiotic and detrital OM. This suggests that host taxa with lower TP rely more on autotrophic chemotrophs for energy, whereas those with higher TPs increasingly rely on heterotrophic OM. However, it is important to note that ΣV index was initially developed in epipelagic detritus that could be presumed to be reworked by heterotrophic bacteria (McCarthy et al., 2007). Although this index can be applied to consumers that rely on bacteria for energy as suggested by Vokhshoori et al. (2021), we take note that metabolism by consumers can potentially influence δ15NAA distribution and subsequently ΣV values. This aspect necessitates thorough investigation in future studies.
Figure 7 Relationship between ΣV and Leu-Ile index in vent fauna from the Onnuri Vent Field (OVF) and North Fiji Basin (NFB).
An increase in the contribution of bacterial AA biomass with increasing TP has been typically observed in littoral environments (Ohkouchi et al., 2017; Vokhshoori et al., 2021), whereas the opposite pattern has been reported in methane seeps (Vokhshoori et al., 2021), where low-TP mussels were suggested to be autotrophic diet feeders and high-TP mussels consumed heterotrophic diets. However, in our study, we did not find such an inverse relationship despite the similarity in primary production processes (i.e., chemosynthesis) between seeps and vents. This discrepancy may be attributed to interspecific variations in the degree of heterotrophic energy contribution. Vokhshoori et al. (2021) looked at ΣV variation across sites for a single species, whereas this study focused on the variation of ΣV between sites for multiple species, at an ecosystem-level. This implies that some symbiont-bearing fauna rely on detritus (i.e., brown food web) as a crucial source of nourishment, with varying degrees of dependence among species. As a result, such effects are reflected in the food web, as demonstrated by the AA-based TP (Steffan et al., 2017). Detritivores are functionally omnivorous, and the omnivorous behavior of hosts suggests that diet flexibility may be a strategy for survival in an ecosystem that is temporally and spatially dynamic.
Using δ15NAA analysis, we determined the TP and extent of heterotrophy in symbiont-bearing taxa found in the hydrothermal vents of the OVF and NFB. These taxa occupied various TPs, ranging from primary consumers to omnivores or carnivores, depending on their feeding guild, environmental conditions, and the species with which they clustered. Our findings showed that the contribution of microbially resynthesized energy increased with TP, particularly in species found in biologically dense ecosystems, such as diffusing vents. This suggests that symbiont-derived energy and detrital or reworked biomass are crucial energy sources for vent fauna. Copepods, which we expected to occupy lower TPs, were found to be carnivores or detritivores, possibly because of trophic inflation caused by the consumption of detrital substrates. Furthermore, we observed low δ15NLeu and δ15NIle, and scattered δ15NPro values against δ15NGlu in the vent fauna, with some variation between species. These patterns may serve as indicators of chemosynthetic ecosystems that are distinct from photosynthetic environments. However, it is important to note that δ15NAA variation in chemosynthetic metabolisms are relatively unexplored compared to photosynthetic systems. Hence, future research efforts should be directed towards unraveling underlying factors contributing to the δ15NAA patterns in chemosynthetic ecosystem.
The original contributions presented in the study are included in the article/Supplementary Material. Further inquiries can be directed to the corresponding author.
The manuscript presents research on animals that do not require ethical approval for their study.
YS, S-JJ, and K-HS contributed to conception and design of the study. M-SK and HC analyzed stable isotopes. YS wrote the first draft of the manuscript. All authors contributed to the article and approved the submitted version.
The author(s) declare financial support was received for the research, authorship, and/or publication of this article. This research was supported by the Korean Institute of Marine Science and Technology (KIMST) and funded by the Ministry of Oceans and Fisheries (20170411 and 20210634). The isotope analysis was supported by the National Institute of Environmental Research (2022-01-01-072).
We are grateful to all crew members and scientists who participated in the exploration cruise and helped with sample collection. We extend our gratitude to Dr. Rho Taekeun and Ms. Son Purena for analyzing nutrient concentrations for this study.
The authors declare that the research was conducted in the absence of any commercial or financial relationships that could be construed as a potential conflict of interest.
All claims expressed in this article are solely those of the authors and do not necessarily represent those of their affiliated organizations, or those of the publisher, the editors and the reviewers. Any product that may be evaluated in this article, or claim that may be made by its manufacturer, is not guaranteed or endorsed by the publisher.
The Supplementary Material for this article can be found online at: https://www.frontiersin.org/articles/10.3389/fmars.2023.1204992/full#supplementary-material
Akashi H., Gojobori T. (2002). Metabolic efficiency and amino acid composition in the proteomes of Escherichia coli and Bacillus subtilis. Proc. Natl. Acad. Sci. 99, 3695–3700. doi: 10.1073/pnas.062526999
Beinart R. A., Luo C., Konstantinidis K. T., Stewart F. J., Girguis P. R. (2019). The bacterial symbionts of closely related hydrothermal vent snails with distinct geochemical habitats show broad similarity in chemoautotrophic gene content. Front. Microbiol. 10. doi: 10.3389/fmicb.2019.01818
Bligh E. G., Dyer W. J. (1959). A rapid method of total lipid extraction and purification. Can. J. Biochem. Physiol. 37, 911–917. doi: 10.1139/o59-099
Bourbonnais A., Lehmann M. F., Butterfield D. A., Juniper S. K. (2012). Subseafloor nitrogen transformations in diffuse hydrothermal vent fluids of the Juan de Fuca Ridge evidenced by the isotopic composition of nitrate and ammonium. Geochemistry Geophysics Geosystems 13, 4661-4678. doi: 10.1029/2011GC003863
Cavanaugh C. M., McKiness Z. P., Newton I. L., Stewart F. J. (2006). Marine chemosynthetic symbioses. prokaryotes 1, 475–507. doi: 10.1007/0-387-30741-9_18
Chang N.-N., Lin L.-H., Tu T.-H., Jeng M.-S., Chikaraishi Y., Wang P.-L. (2018). Trophic structure and energy flow in a shallow-water hydrothermal vent: Insights from a stable isotope approach. PLoS One 13, e0204753. doi: 10.1371/journal.pone.0204753
Charoenpong C. C. N. (2019). The production and fate of nitrogen species in deep-sea hydrothermal environments.
Chikaraishi Y., Ogawa N. O., Kashiyama Y., Takano Y., Suga H., Tomitani A., et al. (2009). Determination of aquatic food-web structure based on compound-specific nitrogen isotopic composition of amino acids. Limnology Oceanography: Methods 7, 740–750. doi: 10.4319/lom.2009.7.740
Chikaraishi Y., Steffan S. A., Takano Y., Ohkouchi N. (2015). Diet quality influences isotopic discrimination among amino acids in an aquatic vertebrate. Ecol. Evol. 5, 2048–2059. doi: 10.1002/ece3.1491
Childress J., Fisher C. R. (1992). The biology of hydrothermal vent animals: Physiology, biochemistry, and autotrophic symbioses. Oceanography Mar. Biology: Annu. Rev. 30, 337–441.
Colaço A., Dehairs F., Desbruyères D. (2002). Nutritional relations of deep-sea hydrothermal fields at the Mid-Atlantic Ridge: A stable isotope approach. Deep-Sea Res. Part I: Oceanographic Res. Papers 49, 395–412. doi: 10.1016/S0967-0637(01)00060-7
Corliss J. B., Dymond J., Gordon L. I., Edmond J. M., von Herzen R. P., Ballard R. D., et al. (1979). Submarine thermal springs on the galápagos rift. Science 203, 1073–1083. doi: 10.1126/science.203.4385.1073
Davies D. D., Humphrey T. J. (1978). Amino Acid recycling in relation to protein turnover. Plant Physiol. 61, 54–58. doi: 10.1104/pp.61.1.54
Dekas A. E., Chadwick G. L., Bowles M. W., Joye S. B., Orphan V. J. (2014). Spatial distribution of nitrogen fixation in methane seep sediment and the role of the ANME archaea. Environ. Microbiol. 16, 3012–3029. doi: 10.1111/1462-2920.12247
Demopoulos A. W. J., McClain-Counts J. P., Bourque J. R., Prouty N. G., Smith B. J., Brooke S., et al. (2019). Examination of Bathymodiolus childressi nutritional sources, isotopic niches, and food-web linkages at two seeps in the US Atlantic margin using stable isotope analysis and mixing models. Deep Sea Res. Part I: Oceanographic Res. Papers 148, 53–66. doi: 10.1016/j.dsr.2019.04.002
Erickson K., Macko S., Van Dover C. (2009). Evidence for a chemoautotrophically based food web at inactive hydrothermal vents (Manus Basin). Deep Sea Res. Part II: Topical Stud. Oceanography 56, 1577–1585. doi: 10.1016/j.dsr2.2009.05.002
Fisher C. R., Childress J. J., Arp A. J., Brooks J. M., Distel D., Favuzzi J. A., et al. (1988). Microhabitat variation in the hydrothermal vent mussel, Bathymodiolus thermophilus, at the Rose Garden vent on the Galapagos Rift. Deep Sea Res. Part A Oceanographic Res. Papers 35, 1769–1791. doi: 10.1016/0198-0149(88)90049-0
Fisher C. R., Childress J. J., Macko S. A., Brooks J. M. (1994). Nutritional interactions in Galapagos rift hydrothermal vent communities: Inferences from stable carbon and nitrogen isotope analyses. Mar. Ecol. Prog. Ser. 103, 45–56. doi: 10.3354/meps103045
Jang S.-J., Cho S.-Y., Li C., Zhou Y., Wang H., Sun J., et al. (2023). Geographical subdivision of Alviniconcha snail populations in the Indian Ocean hydrothermal vent regions. Front. Mar. Sci. 10. doi: 10.3389/fmars.2023.1139190
Jang S.-J., Ho P.-T., Jun S.-Y., Kim D., Won Y.-J. (2020). A newly discovered Gigantidas bivalve mussel from the Onnuri Vent Field in the northern Central Indian Ridge. Deep Sea Res. Part I: Oceanographic Res. Papers 161, 103299. doi: 10.1016/j.dsr.2020.103299
Jannasch H. W., Wirsen C. O. (1979). Chemosynthetic primary production at East Pacific sea floor spreading centers. Bioscience 29, 592–598. doi: 10.2307/1307765
Jarman C. L., Larsen T., Hunt T., Lipo C., Solsvik R., Wallsgrove N., et al. (2017). Diet of the prehistoric population of Rapa Nui (Easter Island, Chile) shows environmental adaptation and resilience. Am. J. Phys. Anthropology 164, 343–361. doi: 10.1002/ajpa.23273
Kennicutt M. C., Burke R. A., MacDonald I. R., Brooks J. M., Denoux G. J., Macko S. A. (1992). Stable isotope partitioning in seep and vent organisms: chemical and ecological significance. Chem. Geology: Isotope Geosci. section 101, 293–310. doi: 10.1016/0009-2541(92)90009-T
Kim J., Son S.-K., Kim D., Pak S.-J., Yu O. H., Walker S. L., et al. (2020). Discovery of active hydrothermal vent fields along the central Indian ridge, 8–12°S. Geochemistry Geophysics Geosystems 21, e2020GC009058. doi: 10.1029/2020gc009058
Kim M.-S., Lee W.-S., Suresh Kumar K., Shin K.-H., Robarge W., Kim M., et al. (2016). Effects of HCl pretreatment, drying, and storage on the stable isotope ratios of soil and sediment samples. Rapid Commun. Mass Spectrometry 30, 1567–1575. doi: 10.1002/rcm.7600
Lee J., Kim D., Kim I.-H. (2020). Copepoda (Siphonostomatoida: Dirivultidae) from hydrothermal vent fields on the central Indian Ridge, Indian Ocean. Zootaxa 4759, 301–337-301–337. doi: 10.11646/zootaxa.4759.3.1
Lee R. W., Childress J. J. (1994). Assimilation of inorganic nitrogen by marine invertebrates and their chemoautotrophic and methanotrophic symbionts. Appl. Environ. Microbiol. 60, 1852–1858. doi: 10.1128/AEM.60.6.1852-1858.1994
Lee W.-K., Juniper S. K., Perez M., Ju S.-J., Kim S.-J. (2021). Diversity and characterization of bacterial communities of five co-occurring species at a hydrothermal vent on the Tonga Arc. Ecol. Evol. 11, 4481–4493. doi: 10.1002/ece3.7343
Liao L., Wankel S. D., Wu M., Cavanaugh C. M., Girguis P. R. (2014). Characterizing the plasticity of nitrogen metabolism by the host and symbionts of the hydrothermal vent chemoautotrophic symbioses Ridgeia piscesae. Mol. Ecol. 23, 1544–1557. doi: 10.1111/mec.12460
Lonsdale P. (1977). Clustering of suspension-feeding macrobenthos near abyssal hydrothermal vents at oceanic spreading centers. Deep Sea Res. 24, 857–863. doi: 10.1016/0146-6291(77)90478-7
McCarthy M. D., Benner R., Lee C., Fogel M. L. (2007). Amino acid nitrogen isotopic fractionation patterns as indicators of heterotrophy in plankton, particulate, and dissolved organic matter. Geochimica Cosmochimica Acta 71, 4727–4744. doi: 10.1016/j.gca.2007.06.061
McMahon K., McCarthy M. (2016). Embracing variability in amino acid δ15N fractionation: Mechanisms, implications, and applications for trophic ecology. Ecosphere 7, e01511. doi: 10.1002/ecs2.1511
Methou P., Hikosaka M., Chen C., Watanabe H. K., Miyamoto N., Makita H., et al. (2022). Symbiont community composition in rimicaris kairei shrimps from Indian ocean vents with notes on mineralogy. Appl. Environ. Microbiol. 88, e0018522. doi: 10.1128/aem.00185-22
Naraoka H., Naito T., Yamanaka T., Tsunogai U., Fujikura K. (2008). A multi-isotope study of deep-sea mussels at three different hydrothermal vent sites in the northwestern Pacific. Chem. Geology 255, 25–32. doi: 10.1016/j.chemgeo.2008.05.015
Ohkouchi N., Chikaraishi Y., Close H. G., Fry B., Larsen T., Madigan D. J., et al. (2017). Advances in the application of amino acid nitrogen isotopic analysis in ecological and biogeochemical studies. Organic Geochemistry 113, 150–174. doi: 10.1016/j.orggeochem.2017.07.009
Park C., Lee W.-K., Kim S.-J., Ju S.-J. (2020). DNA barcoding of isaacsicalanus paucisetus (Copepoda: calanoida: spinocalanidae) from the hydrothermal vent in the North Fiji Basin, Southwestern Pacific Ocean. Anim. Systematics Evol. Diversity 36, 182–184. doi: 10.5635/ASED.2020.36.2.013
Polz M. F., Robinson J. J., Cavanaugh C. M., Van Dover C. L. (1998). Trophic ecology of massive shrimp aggregations at a Mid-Atlantic Ridge hydrothermal vent site. Limnology Oceanography 43, 1631–1638. doi: 10.4319/lo.1998.43.7.1631
Pond D. W., Dixon D. R., Bell M. V., Fallick A. E., Sargent J. R. (1997). Occurrence of 16: 2 (n-4) and 18: 2 (n-4) fatty acids in the lipids of the hydrothermal vent shrimps Rimicaris exoculata and Alvinocaris markensis: nutritional and trophic implications. Mar. Ecol. Prog. Ser. 156, 167–174. doi: 10.3354/meps156167
Ponnudurai R., Heiden S. E., Sayavedra L., Hinzke T., Kleiner M., Hentschker C., et al. (2020). Comparative proteomics of related symbiotic mussel species reveals high variability of host–symbiont interactions. ISME J. 14, 649–656. doi: 10.1038/s41396-019-0517-6
Portail M., Brandily C., Cathalot C., Colaço A., Gélinas Y., Husson B., et al. (2018). Food-web complexity across hydrothermal vents on the Azores triple junction. Deep Sea Res. Part I: Oceanographic Res. Papers 131, 101–120. doi: 10.1016/j.dsr.2017.11.010
Rau G. H. (1981a). Hydrothermal vent clam and tube worm 13C/12C: Further evidence of nonphotosynthetic food sources. Science 213, 338–340. doi: 10.1126/science.213.4505.338
Rau G. H. (1981b). Low 15N/14N in hydrothermal vent animals: ecological implications. Nature 289, 484–485. doi: 10.1038/289484a0
Rau G. H., Hedges J. I. (1979). Carbon-13 depletion in a hydrothermal vent mussel: suggestion of a chemosynthetic food source. Science 203, 648–649. doi: 10.1126/science.203.4381.648
Reid W. D. K., Sweeting C. J., Wigham B. D., McGill R. A. R., Polunin N. V. C. (2016). Isotopic niche variability in macroconsumers of the East Scotia Ridge (Southern Ocean) hydrothermal vents: What more can we learn from an ellipse? Mar. Ecol. Prog. Ser. 542, 13–24. doi: 10.3354/meps11571
Reid W. D. K., Wigham B. D., Marsh L., Weston J. N. J., Zhu Y., Copley J. T. (2020). Trophodynamics at the Longqi hydrothermal vent field and comparison with the East Scotia and Central Indian Ridges. Mar. Biol. 167, 141. doi: 10.1007/s00227-020-03755-1
Salcedo D. L., Soto L. A., Paduan J. B. (2021). Trophic interactions among the macrofauna of the deep-sea hydrothermal vents of Alarcón Rise, Southern Gulf of California. Deep Sea Res. Part I: Oceanographic Res. Papers 176, 103609. doi: 10.1016/j.dsr.2021.103609
Sanders J. G., Beinart R. A., Stewart F. J., Delong E. F., Girguis P. R. (2013). Metatranscriptomics reveal differences in in situ energy and nitrogen metabolism among hydrothermal vent snail symbionts. ISME J. 7, 1556–1567. doi: 10.1038/ismej.2013.45
Steffan S. A., Chikaraishi Y., Dharampal P. S., Pauli J. N., Guédot C., Ohkouchi N. (2017). Unpacking brown food-webs: Animal trophic identity reflects rampant microbivory. Ecol. Evol. 7, 3532–3541. doi: 10.1002/ece3.2951
Suh Y. J., Kim M.-S., Kim S.-J., Kim D., Ju S.-J. (2022b). Carbon sources and trophic interactions of vent fauna in the Onnuri Vent Field, Indian Ocean, inferred from stable isotopes. Deep Sea Res. Part I: Oceanographic Res. Papers 182, 103683. doi: 10.1016/j.dsr.2021.103683
Suh Y., Kim M.-S., Lee W. K., Yoon H., Moon I., Jung J., et al. (2022a). Niche partitioning of hydrothermal vent fauna in the North Fiji Basin, Southwest Pacific inferred from stable isotopes. Mar. Biol. 169, 149. doi: 10.1007/s00227-022-04129-5
Sun Q.-l., Zeng Z.-g., Chen S., Sun L. (2016). First comparative analysis of the community structures and carbon metabolic pathways of the bacteria associated with alvinocaris longirostris in a hydrothermal vent of okinawa trough. PLoS One 11, e0154359. doi: 10.1371/journal.pone.0154359
Suzuki Y., Kojima S., Sasaki T., Suzuki M., Utsumi T., Watanabe H., et al. (2006). Host-symbiont relationships in hydrothermal vent gastropods of the genus Alviniconcha from the Southwest Pacific. Appl. Environ. Microbiol. 72, 1388–1393. doi: 10.1128/aem.72.2.1388-1393.2006
Suzuki Y., Sasaki T., Suzuki M., Nogi Y., Miwa T., Takai K., et al. (2005a). Novel chemoautotrophic endosymbiosis between a member of the Epsilonproteobacteria and the hydrothermal-vent gastropod Alviniconcha aff. hessleri (Gastropoda: Provannidae) from the Indian Ocean. Appl. Environ. Microbiol. 71, 5440–5450. doi: 10.1128/aem.71.9.5440-5450.2005
Suzuki Y., Sasaki T., Suzuki M., Tsuchida S., Nealson K. H., Horikoshi K. (2005b). Molecular phylogenetic and isotopic evidence of two lineages of chemoautotrophic endosymbionts distinct at the subdivision level harbored in one host-animal type: the genus Alviniconcha (Gastropoda: Provannidae). FEMS Microbiol. Lett. 249, 105–112. doi: 10.1016/j.femsle.2005.06.023
Tokuda G., Yamada A., Nakano K., Arita N. O., Yamasaki H. (2008). Colonization of Sulfurovum sp. on the gill surfaces of Alvinocaris longirostris, a deep-sea hydrothermal vent shrimp. Mar. Ecol. 29, 106–114. doi: 10.1111/j.1439-0485.2007.00211.x
Van Audenhaege L., Fariñas-Bermejo A., Schultz T., Lee Van Dover C. (2019). An environmental baseline for food webs at deep-sea hydrothermal vents in Manus Basin (Papua New Guinea). Deep Sea Res. Part I: Oceanographic Res. Papers 148, 88–99. doi: 10.1016/j.dsr.2019.04.018
Van Dover C. (2002). Trophic relationships among invertebrates at the Kairei hydrothermal vent field (Central Indian Ridge). Mar. Biol. 141, 761–772. doi: 10.1007/s00227-002-0865-y
Van Dover C. L., Fry B. (1994). Microorganisms as food resources at deep-sea hydrothermal vents. Limnology Oceanography 39, 51–57. doi: 10.4319/lo.1994.39.1.0051
Versteegh E. A. A., Van Dover C. L., Van Audenhaege L., Coleman M. (2022). Multiple nutritional strategies of hydrothermal vent shrimp (Rimicaris hybisae) assemblages at the Mid-Cayman Rise. Deep Sea Res. Part I: Oceanographic Res. Papers 192, 103915. doi: 10.1016/j.dsr.2022.103915
Vokhshoori N. L., McCarthy M. D., Close H. G., Demopoulos A. W. J., Prouty N. G. (2021). New geochemical tools for investigating resource and energy functions at deep-sea cold seeps using amino acid δ15N in chemosymbiotic mussels (Bathymodiolus childressi). Geobiology 19, 601–617. doi: 10.1111/gbi.12458
Wang X., Li C., Wang M., Zheng P. (2018). Stable isotope signatures and nutritional sources of some dominant species from the PACManus hydrothermal area and the Desmos caldera. PLoS One 13, e0208887. doi: 10.1371/journal.pone.0208887
Wang F., Wu Y., Feng D. (2022). Different nitrogen sources fuel symbiotic mussels at cold seeps. Front. Mar. Sci. 9. doi: 10.3389/fmars.2022.869226
Yamaguchi Y., Chikaraishi Y., Takano Y., Ogawa N., Imachi H., Yokoyama Y., et al. (2017). Fractionation of nitrogen isotopes during amino acid metabolism in heterotrophic and chemolithoautotrophic microbes across Eukarya, Bacteria, and Archaea: Effects of nitrogen sources and metabolic pathways. Organic Geochemistry 111, 101-112. doi: 10.1016/j.orggeochem.2017.04.004
Keywords: food web, trophic position, compound-specific amino acid analysis, δ15N, chemosynthesis, diet source, heterotrophic source
Citation: Suh YJ, Ju S-J, Kim M-S, Choi H and Shin K-H (2023) Trophic diversity of chemosymbiont hosts in deep-sea hydrothermal vents using amino acid nitrogen isotopes. Front. Mar. Sci. 10:1204992. doi: 10.3389/fmars.2023.1204992
Received: 13 April 2023; Accepted: 27 November 2023;
Published: 11 December 2023.
Edited by:
Elva G. Escobar-Briones, National Autonomous University of Mexico, MexicoReviewed by:
Wang Minxiao, Chinese Academy of Sciences (CAS), ChinaCopyright © 2023 Suh, Ju, Kim, Choi and Shin. This is an open-access article distributed under the terms of the Creative Commons Attribution License (CC BY). The use, distribution or reproduction in other forums is permitted, provided the original author(s) and the copyright owner(s) are credited and that the original publication in this journal is cited, in accordance with accepted academic practice. No use, distribution or reproduction is permitted which does not comply with these terms.
*Correspondence: Kyung-Hoon Shin, c2hpbmtoQGhhbnlhbmcuYWMua3I=
Disclaimer: All claims expressed in this article are solely those of the authors and do not necessarily represent those of their affiliated organizations, or those of the publisher, the editors and the reviewers. Any product that may be evaluated in this article or claim that may be made by its manufacturer is not guaranteed or endorsed by the publisher.
Research integrity at Frontiers
Learn more about the work of our research integrity team to safeguard the quality of each article we publish.