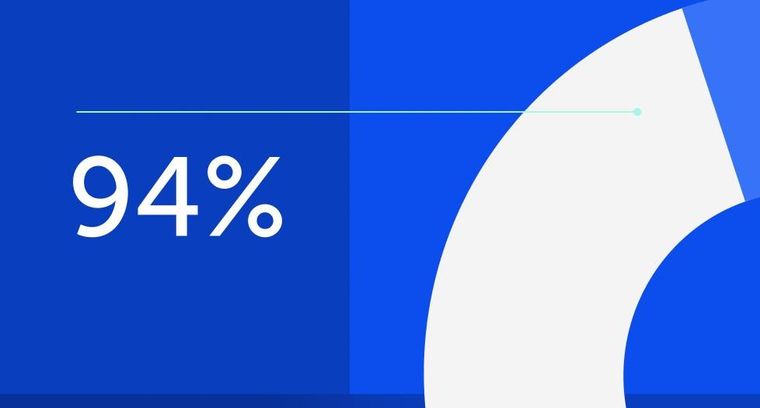
94% of researchers rate our articles as excellent or good
Learn more about the work of our research integrity team to safeguard the quality of each article we publish.
Find out more
REVIEW article
Front. Mar. Sci., 19 July 2023
Sec. Coral Reef Research
Volume 10 - 2023 | https://doi.org/10.3389/fmars.2023.1204843
This article is part of the Research TopicOptics and Ecophysiology of Coral Reef Organisms: Volume IIView all 6 articles
Corals have the ability to synthesize various pigments, responsible for their characteristic vivid coloration. Most coral host pigments are green fluorescent protein (GFP)-like pigments exhibiting diverse spectral properties covering almost the entire visible spectrum, with pigments fluorescing from cyan to red. The type of pigment a coral can synthesize varies inter- and intraspecifically. However, the precise role of host pigments in coral biology has not been fully elucidated. Host pigments have the ability to modify local light fields and could thus contribute to optimizing the light exposure of the photosymbionts. Such fine-tuning of the light microenvironment could enable the holobiont to adapt to broader environmental conditions. Putative mechanisms include energy transfer between host pigments, as well as modulation of their scattering properties via tissue plasticity and granule formation that affect the distribution and organization of host pigments in coral tissue. These mechanisms can enable either photoprotection or photoenhancement depending on the coral’s environment. In this review, we summarize and discuss current knowledge about the link between host pigments and symbiont photosynthesis in reef-building corals, and discuss limitations and challenges of experimental investigation of this connection.
Symbiont-bearing corals live in close association with intracellular, dinoflagellate microalgae belonging to the family Symbiodiniaceae (LaJeunesse et al., 2018), also named zooxanthellae when in hospite. Zooxanthellae are contained by the cnidarian host in its endodermis, bound by membrane-enclosed vacuolar compartments called symbiosomes (Sheppard et al., 2017). This endosymbiosis allows the coral host to become polytrophic, with photosynthates covering up to >95% of the organic carbon requirements of the host, supplemented by carbon and nutrients obtained by feeding on zooplankton (Muscatine et al., 1984) and phytoplankton (Seemann et al., 2013; Leal et al., 2014; Conlan et al., 2019), as well as vitamins and other compounds provided by the coral microbiome (van Oppen and Blackall, 2019). Zooxanthellae benefit from the absence of grazers in the host tissue, where light and inorganic carbon is provided for their photosynthesis along with a stable, yet limited access to nutrients in an otherwise oligotrophic marine environment (Tanaka et al., 2018; Ferrier-Pagès et al., 2021). Moreover, symbiont photosynthesis stimulates skeletogenesis of the coral via light-enhanced calcification (Goreau, 1959; Tambutté et al., 2011), and scleractinian, hermatypic corals are the main builders of coral reefs in tropical waters down to about 75 m (Jarrett et al., 2005). Some corals are able to harbor photosymbionts even deeper in the water column, i.e., down to 172 m (Rouzé et al., 2021), but reef formation at these depths is limited (Schlichter and Fricke, 1991). Optimizing the photosynthetic efficiency of their symbionts, while ensuring protection against high solar irradiation in shallow waters and alleviating light limitation in deeper waters, is a key element of coral fitness. Thus, both the symbionts and the host have evolved mechanisms to optimize photosynthesis (Roth, 2014; Iluz and Dubinsky, 2015), several of which involve the photopigments of the symbionts as well as coral host pigmentation. The colors of corals are partly due to the yellow-brownish coloration of the zooxanthellae containing chlorophylls (a and c) and carotenoids (Jeffrey and Haxo, 1968), while the more spectacular color hues of many corals are due to pigments synthesized by the coral host (Figure 1) (Salih et al., 2000; Dove et al., 2001; Oswald et al., 2007;). Coral host pigments can contribute up to ~7–14% of the soluble cellular proteins in coral tissues even under conditions of low energy supply (Leutenegger et al., 2007; Oswald et al., 2007; Bollati et al., 2020), suggesting that they fulfill a significant function within the coral host that remains to be fully resolved.
Figure 1 Spectral variety of fluorescent host pigments in corals. (A) RGB image under broad spectral illumination. The scale bars represent 2cm (B) RGB image taken under blue (450nm) illumination using a 500nm long pass filter, showing coral FPs (green, orange) and chlorophyll fluorescence (dark-red). Montipora sp. shows green fluorescence (517nm emission peak) and cyan fluorescence (485nm emmission peak). Acanthastrea sp. shows green (516nm emission peak) and orange fluorescence (579nm emission peak). (C) Excitation (emission = 516/517nm, dotted line) and emission (excitation = 450 nm, solid line) spectra of the three coral species, with peak emission wavelength highlighted. Fluorescence was measured on clarified tissue extracts from each coral colony, using a Hitachi F-2500 FL spectrophotometer.
The majority of coral host pigments are related to the green fluorescent protein (GFP) (Dove et al., 2001; Leutenegger et al., 2007; Oswald et al., 2007), first discovered in the bioluminescent hydromedusa Aequorea victoria (Shimomura et al., 1962). Based on their absorption/emission properties, they can be broadly classified as i) fluorescent proteins (FP) absorbing light in the visible spectrum and re-emitting it at longer wavelengths, or ii) non-fluorescent chromoproteins (CP) with characteristic absorption spectra. There are several hypotheses attributing non-photosynthetic roles to host pigments in the ecophysiology of corals such as thermal dissipation (Lyndby et al., 2016), larval settlement (Kenkel et al., 2011), antioxidant activity (Bou-Abdallah et al., 2006), camouflage (Matz et al., 2006), and attraction of symbionts (Hollingsworth et al., 2005; Aihara et al., 2019) or prey (Ben-Zvi et al., 2022) from the environment. However, due to the ability of host pigments to alter the coral tissue light field, they are foremost presumed to interact with coral photosynthesis by providing photoprotection (Salih et al., 2000; Smith et al., 2013; Gittins et al., 2014; Quick et al., 2018; Bollati et al., 2020) or light enhancement (Schlichter et al., 1986; Salih et al., 2000; Smith et al., 2017; Bollati et al., 2022). Indeed, even before the exact nature of coral host pigments was known, it was suggested that these pigments could i) protect the coral against harmful radiation in shallow waters, and ii) transform radiation into wavelengths useful for photosynthesis, thus improving the photosynthetic efficiency of symbiont-bearing corals in deeper waters (Kawaguti, 1969; Schlichter et al., 1986; Schlichter and Fricke, 1991). In recent years, detailed investigations of the optical properties of corals have expanded our knowledge of how host pigments are involved in coral photobiology and stress response, but many open questions remain. Here, we review the current understanding of the roles host pigments play in coral photobiology, and highlight knowledge gaps and potential future research directions.
Coral host pigments are often collectively referred to as GFP-like proteins, due to their structural homology with the A. victoria GFP (avGFP) (Shimomura et al., 1962). While avGFP emits green fluorescence under UV or blue illumination (Shimomura et al., 1962), coral GFP-like host pigments are not necessarily all green, nor fluorescent. GFP-like pigments with diverse absorption/emission spectra were cloned and characterized from non-bioluminescent Anthozoa for the first time in 1999 (Matz et al., 1999). Since then, a plethora of host pigments have been found in corals (Labas et al., 2002; Alieva et al., 2008; Macel et al., 2020). At the time of writing, 251 different host pigments in 48 coral species have been sequenced and recorded in Genbank Dec 12, 2022 (Figure 2, sequences available in Supplementary Data). Additionally, there are many observations of fluorescent and non-fluorescent pigments that have not yet been cloned or characterized in detail (Gruber et al., 2008; Eyal et al., 2015).
Figure 2 Coral host pigments in sequencing databases. Overview of fluorescent and non-fluorescent host pigments sequenced in corals. The total number of different proteins and species was determined from the query “fluorescent protein OR chromoprotein” on the NCBI Genbank database (https://www.ncbi.nlm.nih.gov/genbank/ accessed on Dec 12, 2022). Only sequences from “Stony corals” were used. Duplicated or partial sequences, as well as sequences without species asignment were removed. (A) Classification of FPs according to their predicted fluorescence emission maxima. Proteins with an emission maxima <500nm were classified as CFPs, 500-570nm as GFPs and >570nm as RFPs. The number of FPs emitting inside the limits of the 10nm bins are indicated on top the histogram bars. (B) Coral CPs according their absorption maxima. The total number of CPs counted within 10nm intervals are indicated on top of the histogram bars. Emission and absorption maxima were predicted from translated sequences using FPredX (Tam and Zhang, 2022). Results of the query and functional predictions are available as Supplementary Data.
There are many structural similarities between coral GFP-like proteins and the well-characterized wild-type avGFP (wt-avGFP), where 238 amino acids form a 11-stranded-beta-barrel surrounding the chromophore, creating a very stable 27 kDa large unit (Yang et al., 1996; Yarbrough et al., 2001; Nienhaus and Wiedenmann, 2009). However, while the quaternary structure of wt-avGFP is usually a homodimer (Phillips, 1997), most wild-type coral GFP-like proteins (with the exception of some CPs) form tetramers under physiological conditions (Alieva et al., 2008; Nienhaus and Wiedenmann, 2009; Ahmed et al., 2022). The wt-avGFP chromophore can exist in a neutral or anionic form, and these two forms have different spectral properties. The neutral form has a maximum absorption in the UV, while the anionic form absorbs mainly in the blue-green spectral region (Brejc et al., 1997). Under physiological conditions, the chromophores of host pigments in corals are mainly found in anionic forms (Alieva et al., 2008), therefore they absorb predominantly in the visible range (Figure 2), as opposed to wt-avGFP, which absorbs mostly UV via its neutral chromophore (Heim et al., 1994). Based on their spectral properties, several types of FPs can be distinguished in corals (Figure 2). Cyan FPs (CFPs) are characterized by an emission maximum <500 nm, commonly between 485-495 nm and sometimes have spectral properties that are indicative of chromophores in the neutral state (Alieva et al., 2008; Salih, 2019). Green FPs (GFPs) have an emission maximum >500 nm (Figure 2) (Alieva et al., 2008; Salih, 2019). Some red FPs (RFPs), known as “DsRed-type” after the first protein of this kind to be described (Matz et al., 1999), have a chromophore that emits green light when immature, while spontaneous chromophore maturation in the presence of oxygen leads to the emission of red light (Gross et al., 2000; Nienhaus and Wiedenmann, 2009) (Figure 2). Photoconvertible red FPs (pcRFPs) or Kaede-type RFPs, on the other hand, have an immature green-emitting chromophore that undergoes irreversible photoconversion from green to red emission upon exposure to UV radiation (~390 nm) (Ando et al., 2002; Nienhaus and Wiedenmann, 2009). CPs are characterized by a trans non-coplanar chromophore with a high extinction coefficient but no or very low fluorescence emission (Prescott et al., 2003; Alieva et al., 2008; Ahmed et al., 2022). CPs can sometimes become fluorescent in a light or pH-induced conformational change known as kindling (Lukyanov et al., 2000; Battad et al., 2007), but the implications of this phenomenon for coral biology have not been studied in detail (Salih et al., 2004).
The common presence within a single coral colony of several host pigments with overlapping absorption and fluorescence emission spectra suggests that they could interact with each other via energy transfer to modify the light environment experienced by the symbionts (Salih et al., 2000; Gilmore et al., 2003; Salih et al., 2003; Salih et al., 2004). Energy transfer between chromophores with overlapping excitation/emission spectra can occur via radiative transfer (i.e., emission and reabsorption) or via Förster resonance energy transfer (FRET), which is the non-radiative transfer of energy from an excited donor chromophore to an acceptor chromophore via intermolecular long-range dipole-dipole coupling (Clegg, 1995). FRET requires that i) the distance between the donor and the acceptor must be between 10 – 100 Å, ii) the excitation and emission spectrum of the two molecules must overlap, iii) their dipoles must be appropriately aligned, and iv) the quantum yield of the donor and the absorption coefficient of the acceptor must be high enough (Clegg, 1995). Histological and spectroscopic studies have shown that in some coral species, the spectral combination and spatial arrangement of host pigments are suitable for the formation of FRET pairs (Salih et al., 2003, 2004). Indeed, measurements of fluorescence lifetime indicate that FRET can occur between host pigments, although the relative contribution of this mechanism to overall emission has not been quantified (Gilmore et al., 2003; Cox et al., 2007). Importantly, FRET between coral FPs and zooxanthellae photosynthetic pigments has not been observed, as they are likely organized too far away from each other (Gilmore et al., 2003).
However, intramolecular FRET can be found in coral RFPs. Since maturation of many DsRed-type proteins is incomplete, immature and mature chromophores can be found within the same tetramer (Gross et al., 2000; Nienhaus and Wiedenmann, 2009) that, therefore, emits red fluorescence via direct excitation of the mature red chromophore, as well as via FRET between immature and mature chromophores of the same tetramer (Baird et al., 2000; Gross et al., 2000; Nienhaus and Wiedenmann, 2009). Similar to DsRed-type RFPs, in pcRFPs both unconverted and converted chromophores can exist within the same tetramer. Thus, pcRFPs that have been exposed to UV light emit mainly red fluorescence, either via direct excitation of the red chromophore or via FRET between the green immature chromophore and the red photoconverted chromophore (Ando et al., 2002; Nienhaus and Wiedenmann, 2009; Bollati et al., 2017). In both DsRed-type and in pcRFPs, intra-tetrameric FRET is virtually 100% efficient and green emission disappears upon maturation of a single chromophore; green emission arises mostly from tetramers composed of only immature chromophores (Baird et al., 2000; Wiedenmann et al., 2004).
While these spectral and biochemical properties have been well described for some coral host pigments in vitro, in many cases it remains elusive how these properties affect the light environment inside coral tissue. In fact, considering their in vitro biochemistry is not sufficient in order to understand the potential involvement of host pigments in coral photobiology. The distribution of host pigments relative to each other and to the zooxanthellae, as well as the dynamic regulation of pigments, play a key role in determining the in vivo light environment and must also be taken into account.
The expression and distribution of host pigments vary between coral species, and even between individuals of the same species, representative of different color morphs living side by side on the same reef (Salih et al., 2000; Kelmanson & Matz, 2003; Dove et al., 2006; Oswald et al., 2007; Gittins et al., 2014). At the same time, host pigments with similar optical and biochemical properties can be expressed by different species (Alieva et al., 2008). Two mechanisms can generate such diversity: i) polymorphism, characterized by differences in the genome that were determined during zygote formation leading to phenotypic traits that do not change but can exhibit varying intensity during the lifespan of the organism, and ii) polyphenism, where organisms have the same gene set but develop different phenotypic traits that can vary over time due to differences in the levels of gene expression or post-translational mechanisms (Kelmanson and Matz, 2003). Both polyphenism and polymorphism are involved in determining coral coloration (Takabayashi and Hoegh-Guldberg, 1995; Gittins et al., 2014). For example, the different color morphs of Montastraea cavernosa are a result of polyphenism, where the green and red color morphs possess the same gene set but exhibit differences in expression levels of their genes (mRNA abundance) (Kelmanson and Matz, 2003). In red morphs of Acropora millepora, the intensity of red fluorescence is determined by the copy number of the RFP gene as well as the expression level, a combination of polymorphism and polyphenism (Gittins et al., 2014).
The expression of coral GFP-like host pigment genes can be regulated by a number of environmental factors, such as heat, light, and wounding (D’Angelo et al., 2008; Smith-Keune and Dove, 2008; Kenkel et al., 2011; Smith et al., 2013). For some coral host pigments, gene transcription is driven by light intensity, particularly of light in the blue part of the solar spectrum (D’Angelo et al., 2008). Such “light-induced” GFP-like proteins are thus not expressed in darkness or under spectral light fields lacking blue wavelengths, and have often been reported in shallow water corals from high light environments (D’Angelo et al., 2008; Roth et al., 2010; Smith et al., 2013; Bollati et al., 2020). The relationship between light intensity and gene expression can vary between different light-induced host pigments. Some are expressed under relatively low light and saturate at intermediate photon irradiance levels of around 400 µmol photons m-2 s-1 of photosynthetically active radiation (PAR, 400-700 nm) (low induction threshold), while others exhibit an almost linear relationship between photon irradiance and pigment production (high induction threshold) (D’Angelo et al., 2008). Many CFPs belong to the former group, and many CPs to the latter (D’Angelo et al., 2008), but we note that the light regulation of host pigment gene expression has only been characterized for a very small selection of host pigments and/or coral species (D’Angelo et al., 2008; Bay et al., 2009; Kenkel et al., 2011). The signaling pathway that links blue light to GFP-like protein transcription also remains unknown. Corals are known to possess a number of putative photoreceptors, including blue light sensing cryptochromes and opsins, as well as G-protein coupled receptors thought to play a role in light signal transduction (Levy et al., 2007; Mason et al., 2012; Kaniewska et al., 2015; Mason et al., 2023). However, none of these pathways have yet been directly linked to the transcriptional induction of host pigments.
For other coral host pigments, transcription appears to be light-independent and high pigment concentrations are therefore maintained even when corals are kept in darkness (Leutenegger et al., 2007; Eyal et al., 2015); many FPs detected/observed in mesophotic corals belong to this group (Eyal et al., 2015). While transcriptionally independent from light levels, pcRFPs are to an extent still subject to light regulation via the post-translational photoconversion process, which does not affect protein concentration but does affect protein structure and spectral properties (Ando et al., 2002; Wiedenmann et al., 2004; Leutenegger et al., 2007).
Gene expression of host pigments, and therefore overall fluorescence of coral tissue, are also under regulation by water temperature (Dove et al., 2006; Desalvo et al., 2008; Smith-Keune and Dove, 2008; Hume et al., 2013; Roth and Deheyn, 2013; Mayfield et al., 2014). Specifically, temperature stress has been shown to induce a decrease in host pigment expression, sometimes as a precursor to bleaching (Desalvo et al., 2008; Smith-Keune and Dove, 2008; Mayfield et al., 2014). Additionally, cold exposure resulted in a reduction in host pigment fluorescence in the coral Acropora yongei (Roth & Deheyn, 2013). Since coral bleaching alters the internal light environment of corals (Wangpraseurt et al., 2012, 2017a), the interplay between light-induced upregulation and heat-induced downregulation is a critical factor in determining whether host pigment production will increase or decrease during a bleaching event, as described in later sections (Bollati et al., 2020).
Finally, an ontogenetic shift is sometimes observed in the expression of host pigments, with either an increase, a decrease or a spectral shift in fluorescence being reported during larval settlement and metamorphosis (Roth et al., 2013; Haryanti and Hidaka, 2019). For this review, we focus on the roles of host pigments in the photobiology of adult corals, therefore potential functions in aposymbiotic life stages will not be discussed further.
The studies reviewed so far indicate that the light conditions around and within corals are heavily involved in regulating the expression of host pigments. The light microclimate inside the coral tissue is determined by the interaction between solar radiation and the fundamental optical properties of coral tissue and skeleton, i.e., their absorption and scattering coefficient, and the directionality of scattering. These optical properties are important not only because they affect host pigment regulation, but also because they determine how light travels between host pigments and symbionts, how fluorescence emission is propagated in the tissue, and ultimately how light modulation by host pigments impacts symbiont photosynthesis.
In thick-tissued corals such as Lobophyllidae and Merulinidae, steep light gradients form from the tissue surface towards the skeleton, where the upper tissue layers can experience light levels reaching >200% of the incident, downwelling photon irradiance of PAR, which is then attenuated to 10% or less of the surface photon irradiance at the tissue-skeleton interface (Wangpraseurt et al., 2012, 2016b). This creates distinct light microhabitats across the coral tissue that can drive differential photoacclimation of symbionts in different compartments of thick-tissued corals (Lichtenberg et al., 2016; Wangpraseurt et al., 2016b), and might provide niche space for symbiont genotypes with different light requirements (Wangpraseurt et al., 2016a, 2016b). In contrast, the light field in thin-tissued corals like Pocillopora damicornis becomes more affected by the diffuse backscatter from the coral skeleton that also contributes strongly to light enhancement (Enríquez et al., 2005a; Wangpraseurt et al., 2016b). Despite the absence of steep vertical gradients in such thin-tissued corals, the complex architecture of the colony and scattering characteristics of the coral skeleton still enable homogenization across the coral colony (Enríquez et al., 2017), as well as the formation of shaded microhabitats (Kaniewska et al., 2014; Wangpraseurt et al., 2017b). The relative importance of tissue and skeleton optical properties for the light field of corals and their symbionts thus differs between different coral morphotypes and species, but also across different areas or tissue layers within the same colony.
The spatial position and organization of host pigments within the tissue affect their optical properties. The macroscale distribution of host pigments across coral colonies can vary greatly (Figure 3). Some pigments are distributed homogeneously across the coenosarc, while others are limited to the tip of the polyp tentacles, around the oral disk of the polyp, or concentrated at the colony margin or tip of branches (Salih et al., 2000; Gruber et al., 2008; D’Angelo et al., 2012; Smith et al., 2013; Eyal et al., 2015). In shallow-water corals, host pigments are often found in the ectoderm or in the gastrodermis above the symbiont (Salih et al., 2000, 2004) (Figure 3). But e.g. in depth-generalist and mesophotic corals, they can also be found in the gastrodermis distributed below or around the symbiont (Schlichter et al., 1986; Salih et al., 2000; Mazel et al., 2003; Salih et al., 2004; Oswald et al., 2007). The distribution patterns of host pigments can be either granular, i.e. concentrated in discrete granules (approximately 1 µm in size), which can aggregate to dense chromatophore systems, or diffuse, i.e. homogeneously distributed in the host cells (Salih et al., 2000; Salih et al., 2004; Wangpraseurt et al., 2017b; Wangpraseurt et al., 2019) (Figure 3). Granules, which can contain either a single host pigment or a combination (Salih et al., 2003, 2004), exhibit a more diffuse light scattering leading to enhancement of light closer to the coral tissue surface and a more rapid vertical attenuation of the light as compared to corals with more loosely distributed FPs (Lyndby et al., 2016; Wangpraseurt et al., 2017b). When present in the ectoderm, the scattering of FP chromatophores can thus reduce light exposure of deeper tissue layers shielding the zooxanthellae against excess irradiance and heat deposition (Lyndby et al., 2016). This can be further enhanced by high light induced contraction of coral tissue concentrating FP granules and enhancing the backscatter of light in the upper tissue layers (Wangpraseurt et al., 2017b). Ectodermal granules are uncommon in deep water corals (Salih et al., 2004), which can instead present chromatophore aggregations within or underneath the symbiont layer (Salih et al., 2000; Mazel et al., 2003; Salih et al., 2004; Oswald et al., 2007; Eyal et al., 2015). Specimens of some deep-water species can present granule shapes that are modified into needle- or rod-like structures (Salih et al., 2004), but whether and how such structures affect light propagation and harvesting remains to be studied. Host pigments with diffuse (non-granular), cytoplasmic arrangement are mostly found in ectodermal cells; they are common in corals from all depths (Salih et al., 2004; Eyal et al., 2015), and non-fluorescent CPs from shallow water corals seem to only assume this arrangement (Salih et al., 2000, 2004). In many coral species, multiple host pigments with different arrangement (granular and diffuse, see Figure 3) and localization (ectodermal or endodermal; above, within or below symbionts) coexist, creating complex and unique distribution patterns that contribute to the immense diversity of coral coloration.
Figure 3 Scattering and distribution of host and symbiont pigments in Dipsastraea sp. (A) Optical coherence tomography (OCT) 3D scan of Dipsastraea sp. (see Wangpraseurt et al., 2017b for methodology). Granules (on the right) and diffuse FPs can be observed in the ectoderm of the specimen. The grey framed area represents the region of scanning, while the color scale indicates intensity of scattering. (B) Confocal microscopy image of a 100µm thick tissue section of mouth tissue in Dipsastraea sp. Diffuse and granular FPs can be observed inside the ectoderm above the symbionts comprised in the gastrodermis. The red line represents the location of the section. Green represents GFPs (emission maxima 500-570nm), blue represents CFPs (emission maxima 460-500nm), and red represents chlorophyll fluorescence in the zooxanthellae. The emission of the laser used to acquire the microscopy image was set at 450nm. A live sample of Dipsastraea sp. was used for the OCT scan. The same sample was then fixed in 4% paraformaldehyde dissolved in a solution of 50 mM PBS. The sample was then decalcified using EDTA (10% EDTA), and was mounted on microscope slide for confocal microscopy imaging right after decalcification.
In shallow waters, corals can experience great variations in temperature and solar irradiance (e.g. Jimenez et al., 2012; Wangpraseurt et al., 2014b). Excessive heat stress and high light exposure induce an inhibition of the photosystem II repair mechanism and lower the photoinhibition threshold of the symbiont (Takahashi et al., 2009), which results in increased damage of photosystem II (Aro et al., 1993). Also, excessive light and heat can lead to increasing oxidative stress and production of harmful reactive oxygen species (ROS) in both host and symbiont cells (Rehman et al., 2016). Such stress can play a role in the breakdown of the coral symbiosis known as coral bleaching, where zooxanthellae are either broken down or expelled leaving the translucent coral animal tissue on top of the whitish coral skeleton (Brown, 1997). To cope with excessive temperature and light and avoid the breakdown of the symbiosis, several photoprotection mechanisms are employed either by the coral host or by the symbiont. Photoprotection in corals is in part ensured by the symbiont, using accessory pigments from the xanthophyll cycle (Ambarsari et al., 1997), alternative electron pathways (Reynolds et al., 2008), or by downregulating the activity of the photosystem II reaction centers (Gorbunov et al., 2001), and therefore decreasing the photosynthetic activity. The host also employs various mechanisms to protect their symbionts from excessive light and heat stress. One example is the self-shading morphology commonly found in shallow water corals (Kaniewska et al., 2014). This is typical of branched colonies, where the complex geometry creates more shade among the branches as the colony develops, reducing the total percentage of light absorbed per surface area compared to a spherical or plate shaped coral (Stambler and Dubinsky, 2005).
Host pigments have been shown to exert a photoprotective action on the symbiont, and this is thought to be one of the primary roles for host pigments distributed above the symbiont layer in shallow water corals (Salih et al., 2000); particularly CPs, (Smith et al., 2013) but also CFPs (Quick et al., 2018), GFPs (Lyndby et al., 2016) and RFPs (Gittins et al., 2014). One proposed mechanism for photoprotection is absorption (or “screening”) of excess light by host pigments before it reaches the symbiont layer (Salih et al., 2000; Smith et al., 2013). This is of high relevance for CPs found in thin-tissued corals, where in the absence of host pigments the symbionts experience a light-enhancing environment in hospite, meaning light inside the tissue is higher than outside thanks to the scattering properties of tissue and skeleton (Enríquez et al., 2005; Bollati et al., 2022; Wangpraseurt et al., 2017a). The presence of CPs can change this environment to become more light attenuating within the pigment-specific absorption spectrum (Bollati et al., 2022; Galindo-Martínez et al., 2022), potentially resulting in a 50% reduction in chlorophyll excitation as estimated from in vitro studies (Smith et al., 2013). Besides absorption, coral host pigments can also dissipate excess light energy via elastic scattering (Lyndby et al., 2016; Wangpraseurt et al., 2017b), and FRET between different host pigments might also serve as a photoprotective mechanism via energy conversion (Gilmore et al., 2003; Salih et al., 2004). In shallow water corals such as Acropora spp., FRET was indirectly observed between blue and green FPs (Gilmore et al., 2003; Cox et al., 2007), and groups of pigments with spectral characteristics that are suitable for FRET coupling have been observed within the tightly packed arrangement of FP granules (Salih et al., 2004). FRET between CFPs and GFPs could facilitate a greater Stokes shift than in an individual chromophore, enabling conversion of blue light to yellow-green, which is less readily absorbed by symbiont photosynthetic pigments (Gilmore et al., 2003; Salih et al., 2004; Cox et al., 2007).
In high light environments, conspecific morphs with high host pigment content show i) higher growth rates (Quick et al., 2018), ii) lower photodamage under light stress (Salih et al., 2000; Smith et al., 2013; Gittins et al., 2014), and iii) lower susceptibility to light-induced bleaching (Quick et al., 2018), as compared to less pigmented morphs. Some studies have suggested a positive relationship between pigmentation and resistance to thermal bleaching (Salih et al., 2000; Paley and Bay, 2012; Satoh et al., 2021), while other studies and some anecdotal evidence have reported the opposite relationship (Dove, 2004). Coral FPs can facilitate heat dissipation when they are highly concentrated as granules in the coral’s ectoderm (Lyndby et al., 2016). Such aggregation of FP can be made more dramatic by coral tissue contraction (Wangpraseurt et al., 2017b) leading to intense scattering and light enhancement in the outermost tissue layer and strong vertical light attenuation, which decreases light exposure of the zooxanthellae and leads to less radiative heat dissipation in the symbiont layer (Lyndby et al., 2016). For the particular case of CPs, the effect of pigment concentration on the coral heat budget has not yet been explored in detail. However, it is likely to be very different from that of FPs due to the high extinction coefficient and very low (or zero) fluorescence quantum yield of CPs (Alieva et al., 2008), which results in the majority of excitation energy being dissipated as heat.
Photoprotection by host pigments has also been suggested to facilitate the (re)colonization of coral tissue by symbionts, both during colony expansion and after bleaching events. Bleached corals can accumulate high concentrations of host pigments as a result of an optical feedback loop caused by the loss of symbionts increasing exposure to blue wavelengths that lead to upregulation of host pigmentation, in a process named “colorful bleaching” (Bollati et al., 2020). In healthy corals, blue light inducing host pigment expression is rapidly attenuated in the coral tissue as it is absorbed by symbiont pigments. Therefore, the production of light-induced host pigments is low in a healthy coral with symbionts. However, upon bleaching, light absorption by symbionts decreases greatly leading to enhancement of blue light (Lyndby et al., 2016; Wangpraseurt et al., 2017a) and upregulation of host pigments. The highly concentrated host pigment layer creates a light-attenuating environment, which has been proposed to facilitate the recovery of the symbiont population (Bollati et al., 2020, 2022). A similar feedback mechanism has been proposed to occur in low-symbiont areas of healthy colonies such as axial polyps and plate coral margins (D’Angelo et al., 2012; Bollati et al., 2020).
While photoprotection is now a well-demonstrated role of coral host pigments in shallow water, a different type of potential involvement in photobiology has been proposed for host pigments found in corals that live in low light conditions, such as mesophotic environments. Mesophotic reefs are located in deeper waters (>30 – 150 m), where corals grow under low and strongly blue-shifted solar irradiance (Kahng et al., 2019). Such habitats are also characterized by low mechanical energy, more stable and lower water temperatures and a higher abundance of nutrients (Lesser et al., 2018; Kahng et al., 2019). It has been proposed that mesophotic habitats could be a refuge for corals under the ongoing climate crisis that impacts shallow water reefs with increasing frequency of mass coral bleaching (Bongaerts et al., 2017; Lesser et al., 2018). This “deep reef refuge” hypothesis stipulates that, since corals experience less intense environmental variations (temperature, wave activity, irradiance) in deeper waters, mesophotic reef habitats could become the preferred habitat of species able to adapt and/or acclimate to the mesophotic environment e.g. in terms of low irradiance, a narrow spectral light regime, and lower temperatures (Bongaerts and Smith, 2019). An important prerequisite for such acclimation involves optimization of light harvesting and coral photosynthesis in these blue-green light dominated shaded environments. Only ~3-10% of the surface solar irradiance remains at 65-150 m, depending on the angular position of the sun, and the prevailing light is strongly shifted towards blue wavelengths (Lesser et al., 2018; Kahng et al., 2019). To cope with such reduced light availability and quality, mesophotic corals show lower energy requirements (e.g. lower respiration), an increased dependence on heterotrophic feeding, a change in colony morphology towards more “plate-like” flattened shapes, and an increased spacing between branches that reduces self-shading and might increase light harvesting (Lesser et al., 2018; Kahng et al., 2019).
Optimization of photosynthesis in these light-limited environments has long been proposed as one of the functions of coral host pigments (Schlichter et al., 1986; Salih et al., 2000; Enríquez et al., 2005; Dove et al., 2008). Host pigments are widespread in deep water corals and for some depth specialist species, fluorescent color morphs can represent >70% of the specimens in mesophotic environments (Roth et al., 2015). However, the distribution patterns of color morphs with depth can be very variable. Generally, CFP-containing morphs tend to decrease towards mesophotic depths (Eyal et al., 2015; Roth et al., 2015), while the number of orange-red fluorescent corals increases (Eyal et al., 2015; Smith et al., 2017). However, such surveys have so far only been carried out for a limited number of species and reef locations (Salih et al., 2004; Eyal et al., 2015; Roth et al., 2015; Smith et al., 2017).
Despite the abundance of fluorescent morphs at depth, direct evidence for a role of host pigments in enhancing photosynthesis in mesophotic corals is still lacking. For some mesophotic species, measurements of oxygen production and electron transport rates at the colony level did not show a difference in net photosynthesis between different fluorescent morphs (Ben-Zvi et al., 2019, 2021). However, it was experimentally shown that corals expressing pcRFPs had a greater survival rate in aquarium settings after extended (2 years) low blue light treatment as compared to their non-fluorescent counterparts, which showed 100% mortality at the end of the experiment (Smith et al., 2017). The presence of pcRFPs in corals enables transformation of blue-green wavelengths, abundant in mesophotic environments, to orange-red light, which penetrates deeper in corals tissue, but is otherwise limited at mesophotic depth (Eyal et al., 2015; Lichtenberg et al., 2016; Smith et al., 2017; Bollati et al., 2022). Such transformation is possible and highly efficient due to FRET between green and orange subunits of partially converted pcRFPs (Wiedenmann et al., 2004; Bollati et al., 2017). An ambient light spectrum with reduced (but not zero) UV irradiance, as encountered on mesophotic reefs (Eyal et al., 2015; Kahng et al., 2019), is ideally suited to maintaining the pcRFP pool in a partially converted state, thus maximizing this long range wavelength conversion mechanism (Bollati et al., 2017). A similar partially converted state with high intratetrameric FRET can be found in non-photoconvertible coral RFPs (i.e. DsRed type) due to incomplete maturation of the red chromophore under physiological conditions (Matz et al., 1999; Baird et al., 2000), suggesting that this mechanism may constitute an integral part of the function of RFPs in corals and other Anthozoa (Bollati et al., 2017).
It may appear counterintuitive that the transformation of actinic blue light into orange light could facilitate coral photosynthesis in a mesophotic environment. Blue light is quickly absorbed by the chlorophyll pigment of the symbiont (Wangpraseurt et al., 2012; Wangpraseurt et al., 2014b), while orange light is not as efficiently absorbed, and is thus able to penetrate deeper into the coral tissue, where it can be absorbed by symbionts contained in deeper tissue layers (Lichtenberg et al., 2016; Smith et al., 2017; Bollati et al., 2022). By transforming blue light into orange, the residence time of photons in the tissue is increased, increasing the chances for the symbionts to capture photons for photosynthesis (Wangpraseurt et al., 2014a; Bollati et al., 2017; Smith et al., 2017; Bollati et al., 2022). Such contribution of pcRFPs to the internal light field of corals may be negligible under broad-spectrum, high irradiance conditions, but may become an asset under the ambient, narrow spectral light conditions found in mesophotic environments, allowing to illuminate microenvironments in the coral tissue that, without this contribution, would remain shaded (Bollati et al., 2017; Smith et al., 2017; Bollati et al., 2022). However, a direct stimulation of symbiont photosynthesis via this mechanism remains to be demonstrated, and any potential benefit remains to be quantified in terms of the overall energy budget of the holobiont.
It has been shown that coral morphs harboring GFPs can better attract prey compared to non-fluorescent or orange fluorescent morphs (Ben-Zvi et al., 2022). This mechanism could thus have an important impact on the energy budgets of mesophotic corals that increasingly rely on predation and heterotrophic feeding (Kahng et al., 2019). While this and other non-photobiological roles of host pigments fall outside the scope of this review, we note that the two are not mutually exclusive and it is plausible that coral host pigments can fulfil multiple functions at the same time.
In this review, we have highlighted how various properties of GFP-like pigments may be linked to a diversity of functions. Beyond their well-characterized spectral properties, host pigment regulation mechanisms, microscale spatial distribution, and optical context vary dramatically across habitats, between coral species and even within a single species (Figure 4). Such astounding diversity indicates that pigments with different combinations of these properties may be involved in different physiological functions. Therefore, all these properties should be taken into account when attempting to classify host pigments, and to attribute functions to specific pigment groups. Specifically, we recommend that pigments be characterized in terms of their emission properties (e.g. GFP vs RFP vs CP), but also by establishing whether they are light-induced or constitutive, whether they are distributed above or below the symbiont, diffusely or as granules, and whether they are placed in light-enhancing or light-attenuating tissue (Figure 4). In this context, it would be timely to build on previous surveys of host pigments (Salih et al., 2004; Alieva et al., 2008; Gruber et al., 2008; Eyal et al., 2015) by including all these properties in a comprehensive survey. By establishing how these properties are distributed across taxa and environments, such survey would yield important insights into the diverse ecological and physiological functions of this pigment group.
Figure 4 Key features of host pigments involved in coral photobiology. Based on their spectral properties, host pigments can be broadly categorised as CFPs, GFPs, RFPs and non-fluorescent CPs. Based on their regulation properties, host pigments can be categorised as light-induced (such as the CFPs and GFPs found in some Montipora sp.) or constitutive (such as the GFP found in some Euphyllia sp.), depending on whether transcription of their respective genes is induced by blue light or not. Post-translational photoconversion can also alter the spectral properties of host pigments by changing their emission colour from green to red after exposure to UV light (as observed in the pcRFP from Echinophyllia sp.). Host pigments can be distributed ectodermally or gastrodermally, above or below the symbionts, and they can be arranged diffusely in the cytoplasm or packed into dense granules. Additionally, contraction and expansion can dynamically change the optical properties of the tissue and the arrangement of host pigments. Combined together, these properties ultimately determine the quality and quantity of light that reaches the symbiont cell and is thus available for photosynthesis.
Many aspects of host pigment regulation have still not been explored in detail. While we have an understanding of light-driven pigment regulation (D’Angelo et al., 2008), the heat-driven downregulation of host pigment genes (Desalvo et al., 2008; Smith-Keune and Dove, 2008; Mayfield et al., 2014) has not been explored extensively. The temperature thresholds at which such downregulation occurs are important in determining whether corals can increase host pigment production in response to bleaching, thus potentially affecting their recovery and survival (Bollati et al., 2020). These thresholds should be determined experimentally, perhaps using a standardized rapid thermal stress assay such as CBASS (Voolstra et al., 2020). Such assays could be used to test how heat stress interacts with other factors (light, nutrients, oxygen, feeding), which might impact host pigment regulation.
A key element needed to progress our understanding of the role of host pigments in photophysiology is direct measurements of symbiont photosynthesis at high spatial resolution in relation to the distribution of coral host pigments. These measurements are technically challenging, as the symbionts are contained under layers of tissue and operate under distinct microenvironmental conditions. This complicates the use of commonly applied methods for studying photosynthetic performance in coral ecophysiology such as variable chlorophyll fluorescence (see e.g. (Wangpraseurt et al., 2019). A more promising approach would entail local quantification of the photosynthesis of endosymbionts in the coral endoderm under controlled spectral regimes using microsensors for O2, light and variable chlorophyll fluorescence (e.g. Lichtenberg et al., 2016). Microsensor measurements, sometimes combined with nanoSIMS analyses, have so far been used to show i) that the optical properties of coral tissue allow the creation of micro-light environments for symbiont photosynthesis in hospite (Lichtenberg et al., 2016; Wangpraseurt et al., 2016b), and ii) that pcRFPs can provide a significant contribution to the photosynthetically active light spectrum available for the photosymbionts in mesophotic corals (Bollati et al., 2022). However, a direct measurement of how symbiont photosynthesis is affected by the presence and organization of coral host pigments around the zooaxanthellae is still lacking. So it remains to be determined whether and how fluorescent host pigments can stimulate coral photosynthesis, and what specific roles their spectral and scattering properties as well as their distribution inside the tissue might play in this process.
Furthermore, energy transfer between different FPs in vivo remains a process that has been little explored. While in vitro studies have clearly shown that highly efficient intra-tetrameric FRET occurs in RFPs (Baird et al., 2000; Wiedenmann et al., 2004), any non-radiative energy transfer between separate FP molecules will be highly dependent on the intermolecular distance. In this context, the distribution and arrangement of FPs in the tissue becomes extremely important, as do any mechanisms that might dynamically increase or decrease pigment concentration and therefore determine whether two molecules can be close enough for non-radiative wavelength conversion. Confocal microscopy combined with lifetime imaging can help determine if any particular sets of pigments and pigment arrangements are positioned in a way that is conducive to energy transfer (Gilmore et al., 2003; Salih et al., 2003; Salih et al., 2004; Cox et al., 2007). Additionally, in vivo measurements of fluorescence lifetime would help establish the magnitude of energy transfer under physiological conditions. Eventually, such studies should lead to the quantification of the effects of energy transfer mechanisms on symbiont photosynthesis.
Besides more detailed in hospite measurements of microenvironmental and physiological variables of the host and symbiont, novel in vitro and in silico approaches will also be useful tools to help unravel the role of host pigments for coral photobiology. The use of 3D bioprinting approaches has enabled the construction of bionic corals composed of biopolymers and microalgae mimicking key structural and optical traits of real corals (Wangpraseurt et al., 2020, 2022). Inclusion of host pigments in bionic corals in combination with novel means to read out metabolic activity in 3D bioprinted constructs (Trampe et al., 2018) would enable experimental exploration of how the spatial organization of these pigments relative to the microalgae and the skeleton affects light availability and photosynthesis.
Finally, multiphysics modeling of radiative, heat and mass transfer has recently enabled the simulation of light, temperature and oxygen distributions in corals of known tissue composition and 3D morphology (Taylor Parkins et al., 2021; Murthy et al., 2023). Including the elastic scattering properties of FPs, such numerical modeling was able to replicate experimental measurements of light and temperature enhancement in coral tissue areas with different FP content (Taylor Parkins et al., 2021), but so far the fluorescence properties of FPs have not been included in such simulations. Such modelling approach would enable predictive simulation of how different FP configurations affect the coral microenvironment and function in the context of the coral holobiont. This approach appears particularly promising to understand some of the trade-offs of host pigment production, which are likely to be a major selection driver for color polymorphism (Gittins et al., 2014; Quick et al., 2018). For example, the trade-off between light modulation (Bollati et al., 2022; Galindo-Martínez et al., 2022) and heat budgets (Lyndby et al., 2019) is likely critical in determining recovery potential and eventually survival of corals during bleaching events (Bollati et al., 2020).
In conclusion, a multidisciplinary approach is needed to fully appreciate the diversity of host pigment functions in corals. While many knowledge gaps remain, microscale methods and other emerging technologies offer a great potential for advancing our understanding of the role of coral GFP-like pigments in coral ecophysiology. We hope that by adding to our current knowledge of how corals can acclimate and adapt to diverse habitats, these advances will help guide future conservation and reef management efforts.
EB, GF, and MK contributed to conception and design of the review. GF wrote the first draft of the manuscript. EB, GF, and MK wrote sections of the manuscript. GF designed the figures. All authors contributed to the article and approved the submitted version.
This study was supported a grant from the European Union’s Horizon 2020 research and innovation programme BEEP (Marie Skłodowska-Curie grant agreement No 860125), and an investigator award from the Gordon and Betty Moore Foundation (MK; grant no. GBMF9206; https://doi.org/10.37807/GBMF9206). The material in this study reflects only the author’s views and the EU is not liable for any use that may be made of the information contained therein.
The authors declare that the research was conducted in the absence of any commercial or financial relationships that could be construed as a potential conflict of interest.
All claims expressed in this article are solely those of the authors and do not necessarily represent those of their affiliated organizations, or those of the publisher, the editors and the reviewers. Any product that may be evaluated in this article, or claim that may be made by its manufacturer, is not guaranteed or endorsed by the publisher.
The Supplementary Material for this article can be found online at: https://www.frontiersin.org/articles/10.3389/fmars.2023.1204843/full#supplementary-material
Ahmed F. H., Caputo A. T., French N. G., Peat T. S., Whitfield J., Warden A. C., et al. (2022). Over the rainbow: structural characterization of the chromoproteins gfasPurple, amilCP, spisPink and eforRed. Acta Crystallogr. Sect. Struct. Biol. 78, 599–612. doi: 10.1107/S2059798322002625
Aihara Y., Maruyama S., Baird A. H., Iguchi A., Takahashi S., Minagawa J. (2019). Green fluorescence from cnidarian hosts attracts symbiotic algae. Proc. Natl. Acad. Sci. 116, 2118–2123. doi: 10.1073/pnas.1812257116
Alieva N. O., Konzen K. A., Field S. F., Meleshkevitch E. A., Hunt M. E., Beltran-Ramirez V., et al. (2008). Diversity and evolution of coral fluorescent proteins. PloS One 3, e2680. doi: 10.1371/journal.pone.0002680
Ambarsari I., Brown B. E., Barlow R. G., Britton G., Cummings D. (1997). Fluctuations in algal chlorophyll and carotenoid pigments during solar bleaching in the coral Goniastrea aspera at Phuket, Thailand. Mar. Ecol. Prog. Ser. 159, 303–307. doi: 10.3354/meps159303
Ando R., Hama H., Yamamoto-Hino M., Mizuno H., Miyawaki A. (2002). An optical marker based on the UV-induced green-to-red photoconversion of a fluorescent protein. Proc. Natl. Acad. Sci. 99, 12651–12656. doi: 10.1073/pnas.202320599
Aro E. M., Virgin I., Andersson B. (1993). Photoinhibition of photosystem II. inactivation, protein damage and turnover. Biochim. Biophys. Acta BBA - Bioenerg. 1143, 113–134. doi: 10.1016/0005-2728(93)90134-2
Baird G. S., Zacharias D. A., Tsien R. Y. (2000). Biochemistry, mutagenesis, and oligomerization of DsRed, a red fluorescent protein from coral. Proc. Natl. Acad. Sci. 97, 11984–11989. doi: 10.1073/pnas.97.22.11984
Battad J. M., Wilmann P. G., Olsen S., Byres E., Smith S. C., Dove S. G., et al. (2007). A structural basis for the ph-dependent increase in fluorescence efficiency of chromoproteins. J. Mol. Biol. 368, 998–1010. doi: 10.1016/j.jmb.2007.02.007
Bay L. K., Ulstrup K. E., Nielsen H. B., Jarmer H., Goffard N., Willis B. L., et al. (2009). Microarray analysis reveals transcriptional plasticity in the reef building coral Acropora millepora. Mol. Ecol. 18, 3062–3075. doi: 10.1111/j.1365-294X.2009.04257.x
Ben-Zvi O., Eyal G., Loya Y. (2019). Response of fluorescence morphs of the mesophotic coral Euphyllia paradivisa to ultra-violet radiation. Sci. Rep. 9, 5245. doi: 10.1038/s41598-019-41710-3
Ben-Zvi O., Lindemann Y., Eyal G., Loya Y. (2022). Coral fluorescence: a prey-lure in deep habitats. Commun. Biol. 5, 1–8. doi: 10.1038/s42003-022-03460-3
Ben-Zvi O., Wangpraseurt D., Bronstein O., Eyal G., Loya Y. (2021). Photosynthesis and bio-optical properties of fluorescent mesophotic corals. Front. Mar. Sci. 8. doi: 10.3389/fmars.2021.651601
Bollati E., D’Angelo C., Alderdice R., Pratchett M., Ziegler M., Wiedenmann J. (2020). Optical feedback loop involving dinoflagellate symbiont and scleractinian host drives colorful coral bleaching. Curr. Biol. 30, 2433–2445.e3. doi: 10.1016/j.cub.2020.04.055
Bollati E., Lyndby N. H., D’Angelo C., Kühl M., Wiedenmann J., Wangpraseurt D. (2022). Green fluorescent protein-like pigments optimize the internal light environment in symbiotic reef building corals. eLife 11, e73521. doi: 10.7554/eLife.73521. 2021.10.05.463211.
Bollati E., Plimmer D., D’Angelo C., Wiedenmann J. (2017). FRET-mediated long-range wavelength transformation by photoconvertible fluorescent proteins as an efficient mechanism to generate orange-red light in symbiotic deep water corals. Int. J. Mol. Sci. 18, 1174. doi: 10.3390/ijms18071174
Bongaerts P., Riginos C., Brunner R., Englebert N., Smith S. R., Hoegh-Guldberg O. (2017). Deep reefs are not universal refuges: reseeding potential varies among coral species. Sci. Adv. 3, e1602373. doi: 10.1126/sciadv.1602373
Bongaerts P., Smith T. B. (2019). “Beyond the ‘deep reef refuge’ hypothesis: a conceptual framework to characterize persistence at depth,” in Mesophotic coral ecosystems coral reefs of the world. Eds. Loya Y., Puglise K. A., Bridge T. C. L. (Cham: Springer International Publishing), 881–895. doi: 10.1007/978-3-319-92735-0_45
Bou-Abdallah F., Chasteen N. D., Lesser M. P. (2006). Quenching of superoxide radicals by green fluorescent protein. Biochim. Biophys. Acta BBA - Gen. Subj. 1760, 1690–1695. doi: 10.1016/j.bbagen.2006.08.014
Brejc K., Sixma T. K., Kitts P. A., Kain S. R., Tsien R. Y., Ormö M., et al. (1997). Structural basis for dual excitation and photoisomerization of the Aequorea victoria green fluorescent protein. Proc. Natl. Acad. Sci. 94, 2306–2311. doi: 10.1073/pnas.94.6.2306
Brown B. E. (1997). Coral bleaching: causes and consequences. Coral Reefs 16, S129–S138. doi: 10.1007/s003380050249
Clegg R. M. (1995). Fluorescence resonance energy transfer. Curr. Opin. Biotechnol. 6, 103–110. doi: 10.1016/0958-1669(95)80016-6
Conlan J. A., Humphrey C. A., Severati A., Parrish C. C., Francis D. S. (2019). Elucidating an optimal diet for captive acropora corals. Aquaculture 513, 734420. doi: 10.1016/j.aquaculture.2019.734420
Cox G., Matz M., Salih A. (2007). Fluorescence lifetime imaging of coral fluorescent proteins. Microsc. Res. Tech. 70, 243–251. doi: 10.1002/jemt.20410
D’Angelo C., Denzel A., Vogt A., Matz M. V., Oswald F., Salih A., et al. (2008). Blue light regulation of host pigment in reef-building corals. Mar. Ecol. Prog. Ser. 364, 97–106. doi: 10.3354/meps07588
D’Angelo C., Smith E. G., Oswald F., Burt J., Tchernov D., Wiedenmann J. (2012). Locally accelerated growth is part of the innate immune response and repair mechanisms in reef-building corals as detected by green fluorescent protein (GFP)-like pigments. Coral Reefs 31, 1045–1056. doi: 10.1007/s00338-012-0926-8
Desalvo M. K., Voolstra C. R., Sunagawa S., Schwarz J. A., Stillman J. H., Coffroth M. A., et al. (2008). Differential gene expression during thermal stress and bleaching in the Caribbean coral Montastraea faveolata. Mol. Ecol. 17, 3952–3971. doi: 10.1111/j.1365-294X.2008.03879.x
Dove S. (2004). Scleractinian corals with photoprotective host pigments are hypersensitive to thermal bleaching. Mar. Ecol. Prog. Ser. 272, 99–116. doi: 10.3354/meps272099
Dove S. G., Hoegh-Guldberg O., Ranganathan S. (2001). Major colour patterns of reef-building corals are due to a family of GFP-like proteins. Coral Reefs 19, 197–204. doi: 10.1007/PL00006956
Dove S. G., Lovell C., Fine M., Deckenback J., Hoegh-Guldberg O., Iglesias-Prieto R., et al. (2008). Host pigments: potential facilitators of photosynthesis in coral symbioses. Plant Cell Environ. 31, 1523–1533. doi: 10.1111/j.1365-3040.2008.01852.x
Dove S., Ortiz J. C., Enríquez S., Fine M., Fisher P., Iglesias -Prieto R., et al. (2006). Response of holosymbiont pigments from the scleractinian coral Montipora monasteriata to short-term heat stress. Limnol. Oceanogr. 51, 1149–1158. doi: 10.4319/lo.2006.51.2.1149
Enríquez S., Méndez E. R., Hoegh-Guldberg O., -Prieto R. I. (2017). Key functional role of the optical properties of coral skeletons in coral ecology and evolution. Proc. R. Soc B Biol. Sci. 284, 20161667. doi: 10.1098/rspb.2016.1667
Enríquez S., Méndez E. R., -Prieto R. I. (2005). Multiple scattering on coral skeletons enhances light absorption by symbiotic algae. Limnol. Oceanogr. 50, 1025–1032. doi: 10.4319/lo.2005.50.4.1025
Eyal G., Wiedenmann J., Grinblat M., D’Angelo C., Kramarsky-Winter E., Treibitz T., et al. (2015). Spectral diversity and regulation of coral fluorescence in a mesophotic reef habitat in the red Sea. PloS One 10, e0128697. doi: 10.1371/journal.pone.0128697
Ferrier-Pagès C., Martinez S., Grover R., Cybulski J., Shemesh E., Tchernov D. (2021). Tracing the trophic plasticity of the coral–dinoflagellate symbiosis using amino acid compound-specific stable isotope analysis. Microorganisms 9, 182. doi: 10.3390/microorganisms9010182
Galindo-Martínez C. T., Chaparro A., Enríquez S., Iglesias-Prieto R. (2022). Modulation of the symbionts light environment in hospite in scleractinian corals. Front. Mar. Sci. 9. doi: 10.3389/fmars.2022.1029201
Gilmore A. M., Larkum A. W. D., Salih A., Itoh S., Shibata Y., Bena C., et al. (2003). Simultaneous time resolution of the emission spectra of fluorescent proteins and zooxanthellar chlorophyll in reef-building corals. Photochem. Photobiol. 77, 515–523. doi: 10.1562/0031-8655(2003)0770515STROTE2.0.CO2
Gittins J. R., D’Angelo C., Oswald F., Edwards R. J., Wiedenmann J. (2014). Fluorescent protein-mediated colour polymorphism in reef corals: multicopy genes extend the adaptation/acclimatization potential to variable light environments. Mol. Ecol. 24, 453–465. doi: 10.1111/mec.13041
Gorbunov M. Y., Kolber Z. S., Lesser M. P., Falkowski P. G. (2001). Photosynthesis and photoprotection in symbiotic corals. Limnol. Oceanogr. 46, 75–85. doi: 10.4319/lo.2001.46.1.0075
Goreau T. F. (1959). The physiology of skeleton formation in corals. i. a method for measuring the rate of calcium deposition by corals under different conditions. Biol. Bull. 116, 59–75. doi: 10.2307/1539156
Gross L. A., Baird G. S., Hoffman R. C., Baldridge K. K., Tsien R. Y. (2000). The structure of the chromophore within DsRed, a red fluorescent protein from coral. Proc. Natl. Acad. Sci. 97, 11990–11995. doi: 10.1073/pnas.97.22.11990
Gruber D. F., Kao H.-T., Janoschka S., Tsai J., Pieribone V. A. (2008). Patterns of fluorescent protein expression in scleractinian corals. Biol. Bull. 215, 143–154. doi: 10.2307/25470695
Haryanti D., Hidaka M. (2019). Developmental changes in the intensity and distribution pattern of green fluorescence in coral larvae and juveniles. Galaxea J. Coral Reef Stud. 21, 13–25. doi: 10.3755/galaxea.21.1_13
Heim R., Prasher D. C., Tsien R. Y. (1994). Wavelength mutations and posttranslational autoxidation of green fluorescent protein. Proc. Natl. Acad. Sci. USA 91, 12501–12504. doi: 10.1073/pnas.91.26.12501
Hollingsworth L. L., Kinzie R. A., Lewis T. D., Krupp D. A., Leong J.-A. C. (2005). Phototaxis of motile zooxanthellae to green light may facilitate symbiont capture by coral larvae. Coral Reefs 24, 523–523. doi: 10.1007/s00338-005-0063-8
Hume B., D’Angelo C., Burt J., Baker A. C., Riegl B., Wiedenmann J. (2013). Corals from the Persian/Arabian gulf as models for thermotolerant reef-builders: prevalence of clade C3 Symbiodinium, host fluorescence and ex situ temperature tolerance. Mar. pollut. Bull. 72, 313–322. doi: 10.1016/j.marpolbul.2012.11.032
Iluz D., Dubinsky Z. (2015). Coral photobiology: new light on old views. Zool. Jena Ger. 118, 71–78. doi: 10.1016/j.zool.2014.08.003
Jarrett B. D., Hine A. C., Halley R. B., Naar D. F., Locker S. D., Neumann A. C., et al. (2005). Strange bedfellows - a deep-water hermatypic coral reef superimposed on a drowned barrier island; southern pulley ridge, SW Florida platform margin. Mar. Geol. 214, 295–307. doi: 10.1016/j.margeo.2004.11.012
Jeffrey S. W., Haxo F. T. (1968). Photosynthetic pigments of symbiotic dinoflagellates (zooxanthellae) from corals and clams. Biol. Bull. 135, 149–165. doi: 10.2307/1539622
Jimenez I. M., Larkum A. W. D., Ralph P. J., Kühl M. (2012). In situ thermal dynamics of shallow water corals is affected by tidal patterns and irradiance. Mar. Biol. 159, 1773–1782. doi: 10.1007/s00227-012-1968-8
Kahng S. E., Akkaynak D., Shlesinger T., Hochberg E. J., Wiedenmann J., Tamir R., et al. (2019). “Light, temperature, photosynthesis, heterotrophy, and the lower depth limits of mesophotic coral ecosystems,” in Mesophotic coral ecosystems coral reefs of the world. Eds. Loya Y., Puglise K. A., Bridge T. C. L. (Cham: Springer International Publishing), 801–828. doi: 10.1007/978-3-319-92735-0_42
Kaniewska P., Alon S., Karako-Lampert S., Hoegh-Guldberg O., Levy O. (2015). Signaling cascades and the importance of moonlight in coral broadcast mass spawning. eLife 4, e09991. doi: 10.7554/eLife.09991
Kaniewska P., Anthony K. R. N., Sampayo E. M., Campbell P. R., Hoegh-Guldberg O. (2014). Implications of geometric plasticity for maximizing photosynthesis in branching corals. Mar. Biol. 161, 313–328. doi: 10.1007/s00227-013-2336-z
Kawaguti S. (1969). Effect of the green fluorescent pigment on the productivity of the reef corals. Micronesica 5, 121.
Kelmanson I. V., Matz M. V. (2003). Molecular basis and evolutionary origins of color diversity in great star coral Montastraea cavernosa (Scleractinia: faviida). Mol. Biol. Evol. 20, 1125–1133. doi: 10.1093/molbev/msg130
Kenkel C. D., Aglyamova G., Alamaru A., Bhagooli R., Capper R., Cunning R., et al. (2011). Development of gene expression markers of acute heat-light stress in reef-building corals of the genus Porites. PloS One 6, e26914. doi: 10.1371/journal.pone.0026914
Labas Y. A., Gurskaya N. G., Yanushevich Y. G., Fradkov A. F., Lukyanov K. A., Lukyanov S. A., et al. (2002). Diversity and evolution of the green fluorescent protein family. Proc. Natl. Acad. Sci. 99, 4256–4261. doi: 10.1073/pnas.062552299
LaJeunesse T. C., Parkinson J. E., Gabrielson P. W., Jeong H. J., Reimer J. D., Voolstra C. R., et al. (2018). Systematic revision of symbiodiniaceae highlights the antiquity and diversity of coral endosymbionts. Curr. Biol. 28, 2570–2580.e6. doi: 10.1016/j.cub.2018.07.008
Leal M. C., Ferrier-Pagès C., Calado R., Thompson M. E., Frischer M. E., Nejstgaard J. C. (2014). Coral feeding on microalgae assessed with molecular trophic markers. Mol. Ecol. 23 (15), 3870–3876. doi: 10.1111/mec.12486
Lesser M. P., Slattery M., Mobley C. D. (2018). Biodiversity and functional ecology of mesophotic coral reefs. Annu. Rev. Ecol. Evol. Syst. 49, 49–71. doi: 10.1146/annurev-ecolsys-110617-062423
Leutenegger A., D’Angelo C., Matz M. V., Denzel A., Oswald F., Salih A., et al. (2007). It’s cheap to be colorful. FEBS J. 274, 2496–2505. doi: 10.1111/j.1742-4658.2007.05785.x
Levy O., Appelbaum L., Leggat W., Gothlif Y., Hayward D. C., Miller D. J., et al. (2007). Light-responsive cryptochromes from a simple multicellular animal, the coral Acropora millepora. Science 318, 467–470. doi: 10.1126/science.1145432
Lichtenberg M., Larkum A. W. D., Kühl M. (2016). Photosynthetic acclimation of symbiodinium in hospite depends on vertical position in the tissue of the scleractinian coral Montastrea curta. Front. Microbiol. 7. doi: 10.3389/fmicb.2016.00230
Lukyanov K. A., Fradkov A. F., Gurskaya N. G., Matz M. V., Labas Y. A., Savitsky A. P., et al. (2000). Natural animal coloration can be determined by a nonfluorescent green fluorescent protein homolog. J. Biol. Chem. 275, 25879–25882. doi: 10.1074/jbc.C000338200
Lyndby N. H., Holm J. B., Wangpraseurt D., Ferrier-Pagès C., Kühl M. (2019). Bio-optical properties and radiative energy budgets in fed and starved scleractinian corals (Pocillopora damicornis) during thermal bleaching. Marine Ecology Progress Series 629, 1–17. doi: 10.3354/meps13146
Lyndby N. H., Kühl M., Wangpraseurt D. (2016). Heat generation and light scattering of green fluorescent protein-like pigments in coral tissue. Sci. Rep. 6, 26599. doi: 10.1038/srep26599
Macel M. L., Ristoratore F., Locascio A., Spagnuolo A., Sordino P., D’Aniello S. (2020). Sea As a color palette: the ecology and evolution of fluorescence. Zool. Lett. 6, 9. doi: 10.1186/s40851-020-00161-9
Mason B. M., Koyanagi M., Sugihara T., Iwasaki M., Slepak V., Miller D. J., et al. (2023). Multiple opsins in a reef-building coral, Acropora millepora. Sci. Rep. 13, 1628. doi: 10.1038/s41598-023-28476-5
Mason B., Schmale M., Gibbs P., Miller M. W., Wang Q., Levay K., et al. (2012). Evidence for multiple phototransduction pathways in a reef-building coral. PloS One 7, e50371. doi: 10.1371/journal.pone.0050371
Matz M. V., Fradkov A. F., Labas Y. A., Savitsky A. P., Zaraisky A. G., Markelov M. L., et al. (1999). Fluorescent proteins from nonbioluminescent anthozoa species. Nat. Biotechnol. 17, 969–973. doi: 10.1038/13657
Matz M. V., Marshall N. J., Vorobyev M. (2006). Are corals colorful? Photochem. Photobiol. 82, 345–350. doi: 10.1562/2005-08-18-RA-653
Mayfield A., Liu P.-J., Wang Y.-B., Chen C.-S. (2014). Decreased green fluorescent protein-like chromoprotein gene expression in specimens of the model reef-building coral Pocillopora damicornis undergoing high temperature-induced bleaching. Platax. 2014-12-01, 1–23. doi: 10.29926/PLATAX.201412_2014.0001
Mazel C. H., Lesser M. P., Gorbunov M. Y., Barry T. M., Farrell J. H., Wyman K. D., et al. (2003). Green-fluorescent proteins in Caribbean corals. Limnol. Oceanogr. 48, 402–411. doi: 10.4319/lo.2003.48.1_part_2.0402
Murthy S., Picioreanu C., Kühl M. (2023). Modeling the radiative, thermal and chemical microenvironment of 3D scanned corals. Front. Mar. Sci. 10, 1160208. doi: 10.3389/fmars.2023.1160208
Muscatine L., Falkowski P. G., Porter J. W., Dubinsky Z., Smith D. C. (1984). Fate of photosynthetic fixed carbon in light- and shade-adapted colonies of the symbiotic coral Stylophora pistillata. Proc. R. Soc Lond. B Biol. Sci. 222, 181–202. doi: 10.1098/rspb.1984.0058
Nienhaus G. U., Wiedenmann J. (2009). Structure, dynamics and optical properties of fluorescent proteins: perspectives for marker development. ChemPhysChem 10, 1369–1379. doi: 10.1002/cphc.200800839
Oswald F., Schmitt F., Leutenegger A., Ivanchenko S., D’Angelo C., Salih A., et al. (2007). Contributions of host and symbiont pigments to the coloration of reef corals. FEBS J. 274, 1102–1122. doi: 10.1111/j.1742-4658.2007.05661.x
Paley A., Bay L. (2012). “Bleaching condition varies among Acropora millepora color morphs,” in Proceedings of the 12th International Coral Reef Symposium, Cairns, QLD. 1–5.
Phillips G. N. (1997). Structure and dynamics of green fluorescent protein. Curr. Opin. Struct. Biol. 7, 821–827. doi: 10.1016/S0959-440X(97)80153-4
Prescott M., Ling M., Beddoe T., Oakley A. J., Dove S., Hoegh-Guldberg O., et al. (2003). The 2.2 Å crystal structure of a pocilloporin pigment reveals a nonplanar chromophore conformation. Structure 11, 275–284. doi: 10.1016/S0969-2126(03)00028-5
Quick C., D’Angelo C., Wiedenmann J. (2018). Trade-offs associated with photoprotective green fluorescent protein expression as potential drivers of balancing selection for color polymorphism in reef corals. Front. Mar. Sci. 5. doi: 10.3389/fmars.2018.00011
Rehman A. U., Szabó M., Deák Z., Sass L., Larkum A., Ralph P., et al. (2016). Symbiodinium sp. cells produce light-induced intra- and extracellular singlet oxygen, which mediates photodamage of the photosynthetic apparatus and has the potential to interact with the animal host in coral symbiosis. New Phytol. 212, 472–484. doi: 10.1111/nph.14056
Reynolds J. M., Bruns B. U., Fitt W. K., Schmidt G. W. (2008). Enhanced photoprotection pathways in symbiotic dinoflagellates of shallow-water corals and other cnidarians. Proc. Natl. Acad. Sci. 105, 13674–13678. doi: 10.1073/pnas.0805187105
Roth M. S. (2014). The engine of the reef: photobiology of the coral–algal symbiosis. Front. Microbiol. 5. doi: 10.3389/fmicb.2014.00422
Roth M. S., Deheyn D. D. (2013). Effects of cold stress and heat stress on coral fluorescence in reef-building corals. Sci. Rep. 3, 1421. doi: 10.1038/srep01421
Roth M., Fan T.-Y., Deheyn D. (2013). Life history changes in coral fluorescence and the effects of light intensity on larval physiology and settlement in Seriatopora hystrix. PloS One 8, e59476. doi: 10.1371/journal.pone.0059476
Roth M. S., Latz M. I., Goericke R., Deheyn D. D. (2010). Green fluorescent protein regulation in the coral Acropora yongei during photoacclimation. J. Exp. Biol. 213, 3644–3655. doi: 10.1242/jeb.040881
Roth M. S., Padilla-Gamiño J. L., Pochon X., Bidigare R. R., Gates R. D., Smith C. M., et al. (2015). Fluorescent proteins in dominant mesophotic reef-building corals. Mar. Ecol. Prog. Ser. 521, 63–79. doi: 10.3354/meps11108
Rouzé H., Galand P. E., Medina M., Bongaerts P., Pichon M., Pérez-Rosales G., et al. (2021). Symbiotic associations of the deepest recorded photosynthetic scleractinian coral (172 m depth). ISME J. 15, 1564–1568. doi: 10.1038/s41396-020-00857-y
Salih A. (2019). “Fluorescent proteins,” in Fundamentals of fluorescence imaging (Singapore:Jenny Stanford Publishing), 22. doi: 10.1201/9781351129404-6
Salih A., Cox G. C., Larkum A. W. (2003). “Cellular organization and spectral diversity of GFP-like proteins in live coral cells studied by single and multiphoton imaging and microspectroscopy,” in Multiphoton microscopy in the biomedical sciences III (SPIE), 194–200. doi: 10.1117/12.487618
Salih A., Larkum A., Cox G., Kühl M., Hoegh-Guldberg O. (2000). Fluorescent pigments in corals are photoprotective. Nature 408, 850–853. doi: 10.1038/35048564
Salih A., Larkum A. W., Cronin T. W., Wiedenmann J., Szymczak R., Cox G. C. (2004). “Biological properties of coral GFP-type proteins provide clues for engineering novel optical probes and biosensors,” in Genetically engineered and optical probes for biomedical applications II (SPIE), 61–72. doi: 10.1117/12.548926
Satoh N., Kinjo K., Shintaku K., Kezuka D., Ishimori H., Yokokura A., et al. (2021). Color morphs of the coral Acropora tenuis show different responses to environmental stress and different expression profiles of fluorescent-protein genes. G3 GenesGenomesGenetics 11, jkab018. doi: 10.1093/g3journal/jkab018
Schlichter D., Fricke H. W. (1991). Mechanisms of amplification of photosynthetically active radiation in the symbiotic deep-water coral Leptoseris fragilis. Hydrobiologia 216, 389–394. doi: 10.1007/BF00026491
Schlichter D., Fricke H. W., Weber W. (1986). Light harvesting by wavelength transformation in a symbiotic coral of the red Sea twilight zone. Mar. Biol. 91, 403–407. doi: 10.1007/BF00428634
Seemann J., Sawall Y., Auel H., Richter C. (2013). The use of lipids and fatty acids to measure the trophic plasticity of the coral stylophora subseriata. Lipids 48, 275–286. doi: 10.1007/s11745-012-3747-1
Sheppard C., Davy S., Pilling G., Graham N. (2017). The biology of coral reefs (Oxford:Oxford University Press).
Shimomura O., Johnson F. H., Saiga Y. (1962). Extraction, purification and properties of aequorin, a bioluminescent protein from the luminous hydromedusan, Aequorea. J. Cell. Comp. Physiol. 59, 223–239. doi: 10.1002/jcp.1030590302
Smith E. G., D’Angelo C., Salih A., Wiedenmann J. (2013). Screening by coral green fluorescent protein (GFP)-like chromoproteins supports a role in photoprotection of zooxanthellae. Coral Reefs 32, 463–474. doi: 10.1007/s00338-012-0994-9
Smith E. G., D’Angelo C., Sharon Y., Tchernov D., Wiedenmann J. (2017). Acclimatization of symbiotic corals to mesophotic light environments through wavelength transformation by fluorescent protein pigments. Proc. R. Soc B Biol. Sci. 284, 20170320. doi: 10.1098/rspb.2017.0320
Smith-Keune C., Dove S. (2008). Gene expression of a green fluorescent protein homolog as a host-specific biomarker of heat stress within a reef-building coral. Mar. Biotechnol. 10, 166–180. doi: 10.1007/s10126-007-9049-6
Stambler N., Dubinsky Z. (2005). Corals as light collectors: an integrating sphere approach. Coral Reefs 24, 1–9. doi: 10.1007/s00338-004-0452-4
Takabayashi M., Hoegh-Guldberg O. (1995). Ecological and physiological differences between two colour morphs of the coral Pocillopora damicornis. Mar. Biol. 123, 705–714. doi: 10.1007/BF00349113
Takahashi S., Whitney S. M., Badger M. R. (2009). Different thermal sensitivity of the repair of photodamaged photosynthetic machinery in cultured symbiodinium species. Proc. Natl. Acad. Sci. 106, 3237–3242. doi: 10.1073/pnas.0808363106
Tam C., Zhang K. Y. J. (2022). FPredX: interpretable models for the prediction of spectral maxima, brightness, and oligomeric states of fluorescent proteins. Proteins Struct. Funct. Bioinforma. 90, 732–746. doi: 10.1002/prot.26270
Tambutté S., Holcomb M., Ferrier-Pagès C., Reynaud S., Tambutté E., Zoccola D., et al. (2011). Coral biomineralization: from the gene to the environment. J. Exp. Mar. Biol. Ecol. 408, 58–78. doi: 10.1016/j.jembe.2011.07.026
Tanaka Y., Suzuki A., Sakai K. (2018). The stoichiometry of coral-dinoflagellate symbiosis: carbon and nitrogen cycles are balanced in the recycling and double translocation system. ISME J. 12, 860–868. doi: 10.1038/s41396-017-0019-3
Taylor Parkins S. K., Murthy S., Picioreanu C., Kühl M. (2021). Multiphysics modelling of photon, mass and heat transfer in coral microenvironments. J. R. Soc Interface 18, 20210532. doi: 10.1098/rsif.2021.0532
Trampe E., Koren K., Akkineni A. R., Senwitz C., Krujatz F., Lode A., et al. (2018). Functionalized bioink with optical sensor nanoparticles for O2 imaging in 3D bioprinted constructs. Adv. Func. Mat. 28, 1804411. doi: 10.1002/adfm.201804411
van Oppen M. J. H., Blackall L. L. (2019). Coral microbiome dynamics, functions and design in a changing world. Nat. Rev. Microbiol. 17, 557–567. doi: 10.1038/s41579-019-0223-4
Voolstra C. R., Buitrago-López C., Perna G., Cárdenas A., Hume B. C. C., Rädecker N., et al. (2020). Standardized short-term acute heat stress assays resolve historical differences in coral thermotolerance across microhabitat reef sites. Glob. Change Biol. 26, 4328–4343. doi: 10.1111/gcb.15148
Wangpraseurt D., Holm J. B., Larkum A. W. D., Pernice M., Ralph P. J., Suggett D. J., et al. (2017a). In vivo microscale measurements of light and photosynthesis during coral bleaching: evidence for the optical feedback loop? Front. Microbiol. 8. doi: 10.3389/fmicb.2017.00059
Wangpraseurt D., Wentzel C., Jacques S. L., Wagner M., Kühl M. (2017b). In vivo imaging of coral tissue and skeleton with optical coherence tomography. J. R. Soc Interface 14, 20161003. doi: 10.1098/rsif.2016.1003
Wangpraseurt D., Jacques S. L., Petrie T., Kühl M. (2016a). Monte Carlo Modeling of photon propagation reveals highly scattering coral tissue. Front. Plant Sci. 7. doi: 10.3389/fpls.2016.01404
Wangpraseurt D., Pernice M., Guagliardo P., Kilburn M. R., Clode P. L., Polerecky L., et al. (2016b). Light microenvironment and single-cell gradients of carbon fixation in tissues of symbiont-bearing corals. ISME J. 10, 788–792. doi: 10.1038/ismej.2015.133
Wangpraseurt D., Larkum A. W. D., Franklin J., Szabó M., Ralph P. J., Kühl M. (2014a). Lateral light transfer ensures efficient resource distribution in symbiont-bearing corals. J. Exp. Biol. 217, 489–498. doi: 10.1242/jeb.091116
Wangpraseurt D., Polerecky L., Larkum A. W. D., Ralph P. J., Nielsen D. A., Pernice M., et al. (2014b). The in situ light microenvironment of corals. Limnol. Oceanogr. 59, 917–926. doi: 10.4319/lo.2014.59.3.0917
Wangpraseurt D., Larkum A., Ralph P. J., Kühl M. (2012). Light gradients and optical microniches in coral tissues. Front. Microbiol. 3. doi: 10.3389/fmicb.2012.00316
Wangpraseurt D., Lichtenberg M., Jacques S. L., Larkum A. W. D., Kühl M. (2019). Optical properties of corals distort variable chlorophyll fluorescence measurements. Plant Physiol. 179, 1608–1619. doi: 10.1104/pp.18.01275
Wangpraseurt D., Sun Y., You S., Chua S.-T., Noel S. K., Willard H. F., et al. (2022). Bioprinted living coral microenvironments mimicking coral-algal symbiosis. Adv. Funct. Mater. 32, 2202273. doi: 10.1002/adfm.202202273
Wangpraseurt D., You S., Azam F., Jacucci G., Gaidarenko O., Hildebrand M., et al. (2020). Bionic 3D printed corals. Nat. Commun. 11, 1748. doi: 10.1038/s41467-020-15486-4
Wiedenmann J., Ivanchenko S., Oswald F., Schmitt F., Röcker C., Salih A., et al. (2004). EosFP, a fluorescent marker protein with UV-inducible green-to-red fluorescence conversion. Proc. Natl. Acad. Sci. USA 101, 15905–15910. doi: 10.1073/pnas.0403668101
Yang F., Moss L. G., Phillips G. N. (1996). The molecular structure of green fluorescent protein. Nat. Biotechnol. 14, 1246–1251. doi: 10.1038/nbt1096-1246
Keywords: fluorescence, GFP-like protein, photosynthesis, energy transfer, photoprotection, photo-enhancement, symbiosis
Citation: Ferreira G, Bollati E and Kühl M (2023) The role of host pigments in coral photobiology. Front. Mar. Sci. 10:1204843. doi: 10.3389/fmars.2023.1204843
Received: 12 April 2023; Accepted: 21 June 2023;
Published: 19 July 2023.
Edited by:
Virginia M. Weis, Oregon State University, United StatesReviewed by:
Renaud Grover, Centre Scientifique de Monaco, MonacoCopyright © 2023 Ferreira, Bollati and Kühl. This is an open-access article distributed under the terms of the Creative Commons Attribution License (CC BY). The use, distribution or reproduction in other forums is permitted, provided the original author(s) and the copyright owner(s) are credited and that the original publication in this journal is cited, in accordance with accepted academic practice. No use, distribution or reproduction is permitted which does not comply with these terms.
*Correspondence: Elena Bollati, ZWxlbmEuYm9sbGF0aUBiaW8ua3UuZGs=; Michael Kühl, bWt1aGxAYmlvLmt1LmRr
Disclaimer: All claims expressed in this article are solely those of the authors and do not necessarily represent those of their affiliated organizations, or those of the publisher, the editors and the reviewers. Any product that may be evaluated in this article or claim that may be made by its manufacturer is not guaranteed or endorsed by the publisher.
Research integrity at Frontiers
Learn more about the work of our research integrity team to safeguard the quality of each article we publish.