- Beneath The Waves, Herndon, VA, United States
Climate change is threatening marine ecosystems and the distribution of species which rely on them. Due to their capacity to sequester vast amounts of carbon, blue carbon ecosystems (BCEs; seagrass, mangroves, salt marshes, kelp forests) are becoming increasingly recognized as key nature-based solutions to climate change. Sharks are mobile species that can exert strong control of food webs and are also key to conservation efforts. BCEs are known to support shark life histories, but the drivers of these relationships remain poorly understood. Here, we highlight two key behavioural pathways directly linking BCEs and sharks (foraging and reproductive activities) and explore the influence of climate on them. Our evaluation of the literature suggested that the physical attributes of the blue carbon plant tissue itself serves as the key link between sharks and BCEs, facilitating high rates of prey biodiversity and a platform for reproductive behaviours. We revealed that shark body size likely has an influence on the nature of these relationships, and that climate may be a modulator of key interactions. We also use basic network theory to explore how ecological information flows throughout BCEs, with sharks as a prominent actor. We identify ways in which future studies can fill knowledge gaps; namely, a focus on smaller endemic species, and empirical assessments between sharks and organic carbon stocks. Maintaining the integrity of these relationships should preserve blue natural capital: BCEs’ capacity to sequester carbon, support local biodiversity, and the role of sharks in preserving resilience.
Introduction
Blue carbon ecosystems (hereafter referred to as “BCEs”) refer to those marine and coastal vegetated ecosystems (seagrass, mangroves, salt marshes, kelp forests) which contribute significantly to organic carbon sequestration; all of which have become increasingly valued in the broader discussion around climate change mitigation (Duarte et al., 2013; Lovelock and Duarte, 2019; Gao et al., 2022). Despite only occupying less than 0.2% of the world’s oceans, BCE’s are able to capture 50% of all carbon stored in the ocean (Duarte et al., 2013). Seagrass meadows, for example, not only capture organic carbon faster than tropical rainforests (Mcleod et al., 2011), but they store carbon for millennia compared to rainforests which do so for decades (Mateo et al., 1997; Macreadie et al., 2012; Serrano et al., 2012; Macreadie et al., 2015). The distribution of BCEs extends throughout virtually all marine environments from tropical to temperate environments, and as such their blue natural capital (the natural capital found in coastal and marine ecosystems) extends beyond their value solely as carbon sinks, as BCEs are critical hubs for mobile fish biodiversity and survival across ontogeny (Duarte, 2000; Vierros, 2017; Duarte and Gallagher, 2023). Global losses in BCEs due to human impacts – including the effects of climate change – however, have reduced the biomass and net productivity of BCEs (Crooks et al., 2011; Pendleton et al., 2012), reducing the capacity of the ocean as a carbon sink.
The identification of critical marine habitats to supporting threatened marine species remains a key objective for the conservation of biodiversity and this is particularly important for sharks, which are inherently vulnerable to overharvest due to life history traits which make their population recovery slow (e.g., low fecundity, slow growth rates; (Gallagher et al., 2012)). In many marine ecosystems worldwide, sharks continue to decline due to overexploitation and bycatch (Pimiento et al., 2020). Over the last two decades, this consistent level of threat and extinction risk has resulted in a heightened focus on shark behaviour, ecology, and fisheries interactions (Jorgensen et al., 2022). As a result, shark conservation has become a major contemporary theme in the broader marine conservation dialogue (Jorgensen et al., 2022) and the identification and protection of critical habitats which support important components of shark life-histories remains a critical research need (Hyde et al., 2022).
As mobile consumers, sharks can readily access BCEs and may influence the myriad of ecological processes they support, and as such, their degree of habitat use within BCEs should provide insights into how individuals or entire species utilize BCEs to meet their life history needs. Long-term ecological monitoring, through techniques such as biotelemetry, has provided insight into the relationships between shark habitat use within BCEs (Hussey et al., 2015). For example, studies conducted in the subtropical western Atlantic have demonstrated that BCEs acted as a key nursery and foraging ground for juvenile lemon sharks Negaprion brevirostris (Newman et al., 2010; Dhellemmes et al., 2021) and that seagrass ecosystems were preferentially selected by tiger sharks Galeocerdo cuvier over other habitat types, presumably for access to foraging opportunities (Gallagher et al., 2021). Studies on sharks and BCEs have also significantly enhanced our understanding of the ocean ecosystem more broadly. Specifically, tiger sharks recently guided the discovery and characterization of the world’s largest seagrass ecosystem in The Bahamas, a spatial extent estimated to be up to 93,000 km2 (Gallagher et al., 2022) which extended the total global estimate of seagrass cover by 41%.
These few examples show clear overlaps between shark life histories and BCEs, but the drivers and functions of these relationships are often less obvious, as is our understanding of the influence of a changing climate on these patterns. Food-web studies involving sharks and prey species found in BCEs (seagrasses primarily e.g., (Heithaus et al., 2002)) also point to the importance of shark-mediated blue carbon flux, with potential links to organic carbon stocks (Atwood et al., 2015). However, evaluating the role of sharks in these processes requires a holistic understanding of potential behavioural and trophic relationships and how they function in space and time. Without a proper overview of these relationships, it remains difficult to gauge the importance of sharks as vectors of blue carbon and predict their influence on BCE’s potential as a nature-based solution to climate change.
Here, we briefly summarize the current knowledge on the relationships between sharks and blue carbon ecosystems (BCEs), by identifying two direct, critical behavioural pathways in which they are linked. Within this framework, we summarize key research findings and identify existing data gaps, revealing opportunities for inspiring future research. We also apply network theory to describe the structure and function of BCEs, to aid in our understanding as to how ecological information (e.g., trophic, predation risk) is transmitted in BCEs and what the effects of climate, for example, may be on the species interactions therein. We recognize that this mini-review is not designed to be an exhaustive summary of the entire literature, but rather seeks to summarize important research findings to guide novel studies that evaluate the relationships between sharks and BCEs. We contend that expanding this emergent and timely research area should better contextualize the ecological role of sharks and the ecosystem services they provide, while adding new insights into the benefits of protecting BCEs, ultimately highlighting the contributions of sharks and BCEs to the blue economy wherever BCEs are found.
Foraging
The fast-growing vegetation comprising BCEs attract and support a wide array of marine species, therefore serving as a hub for organizing local biodiversity (Duarte, 2000; Vierros, 2017; Moraes, 2019). BCEs are often characterized by elevated habitat complexity which provide suitable quality habitat for prey species that rely upon these for refuge as well as foraging resources for prey themselves (Laegdsgaard and Johnson, 2001; Powter and Gladstone, 2008; Knip et al., 2010). In tropical ecosystems, the carbon inputs from all three BCEs were shown to link to the diet of multiple commercially-relevant species (Gorman et al., 2023). Seagrass meadows, for example, support 20% of the world’s largest 25 fisheries (Unsworth et al., 2019), highlighting their value as foraging grounds for larger predators like sharks due to high biomass and diversity of prey.
Adult sharks will also preferentially select mangrove lagoons (lemon sharks e.g., Pillans et al., 2021) and seagrass meadows (tiger sharks e.g.,Gallagher et al., 2021) over nearby reef habitats. Large, predatory sharks actively seek out BCEs when energetically-rich prey may be present. Tiger sharks monitored in the equatorial Indian Ocean directed their movements over long distances to overlap in time and space with green sea turtles (Chelonia mydas) at Raine Island, a remote seagrass-dominated BCE which supports the largest known aggregation of this prey species (Hammerschlag et al., 2016). In the subtropical Atlantic, tiger sharks with bio-loggers directed fine-scale movements towards seagrass meadows with the greatest densities throughout the seascape (Gallagher et al., 2021), presumably to increase encounter rates with sea turtles. Similarly, broadnose sevengill sharks (Notorynchus cepedianus), which are a top predator in temperate latitudes, show tight associations with kelp forests throughout their range (Ebert, 1996; Hammerschlag et al., 2019).
As the density and complexity of BCEs themselves may affect changes in local prey abundance or availability, the foraging behaviour of mobile marine consumers within these food-webs can also change (Harcourt et al., 2002). Large sharks demonstrate area-restricted searching (ARS) and tortuous movements when prey availability increases (Sims and Quayle, 1998), and this behaviour has been documented for multiple species within BCEs (Towner et al., 2016). Camera-equipped tiger sharks off Australia displayed tortuous movements in 27% of their tracks over seagrass meadows, which corresponded to turtles being detected and observed (Andrzejaczek et al., 2019). Similar work using animal-borne cameras revealed the extensive use of dense kelp forests (a temperate BCE) by white sharks Carcharodon carcharias at Dyer Island, South Africa, with significantly higher rates of ARS and turning associated with hunting for cape fur seals Arctocephalus pusillus whilst in the kelp forests (Jewell et al., 2019). Kelp ecosystems were previously thought to be a less-advantageous ecosystem for white sharks for actively hunting seals compared to open water drop-offs; these new data suggest that kelp forests may actually present white sharks with higher prey encounter rates, as well as the overlooked element of tactical camouflage and ambush facilitated by the three-dimensional nature of the BCE (Towner et al., 2016). Taken together, these studies indicate that fine-scale selection of BCE habitat by sharks may be used to increase encounter rates with prey species utilizing BCEs for their own fitness-related reasons.
The role of BCEs as key foraging grounds for sharks is further supported by the quantitative analysis of diet via stomach contents and molecular tracers such as stable isotopes and fatty acids. For example, in Shark Bay, Australia, stomach contents of tiger sharks revealed that sea turtles were common prey items (Heithaus, 2001) and in Bimini, Bahamas, mangrove associated prey were found to be a large component of lemon shark diet (Newman et al., 2010). Additionally, seagrass was found to make up a large component of bonnethead shark Sphyrna tiburo diet with mass gut content consisting of 62.1% seagrass (Leigh et al., 2018). Stable isotopes also revealed the community-wide importance of seagrass and mangrove resources for sharks across a variety of locales, including coastal Florida, USA (Gallagher et al., 2017b; Shipley et al., 2019) and Shark Bay, Western Australia (Vaudo and Heithaus, 2011). At the individual level, the presence of kelp BCEs elevated the trophic position of male redspotted catsharks Schroederichthys chilensis (Vásquez-Castillo et al., 2021). BCEs clearly provide important indirect (and in some cases, direct) energetic and nutritive roles for sharks, yet the strength of these interactions is likely to vary highly both locally and at the species level. Recent research also points to the important functional role of shark species which utilize BCEs (Shipley et al., 2023), whereby seagrass habitats served as important resource pools and geographical connections for mobile species such as blacknose sharks (Carcharhinus acronotus), bull sharks (Carcharhinus leucas), lemon sharks (Negaprion brevirostris), nurse sharks (Ginglymostoma cirratum), Caribbean reef (Carcharhinus perezi), and tiger sharks (Galeocerdo cuvier). These species served as important vectors of blue carbon in the marine environments they inhabited, more so than species which did not occupy BCEs, highlighting their importance to blue carbon flux, with likely implications for the carbon cycle.
Reproductive activities
The physical characteristics of BCEs which facilitate foraging-related species interactions should also provide additional benefits to sharks, specifically those linked to reproduction. Mangrove roots, for example, provide newborn and juvenile sharks with protection from predators, and it is these food-abundant lagoons which drive high residency in gravid female sharks. As a result, the value of BCEs (predominantly mangroves) as critical nursery ground for many species of sharks throughout their ontogeny has been widely documented in many regions (Heupel et al., 2007).
Shark nurseries can be described as geographically distinct areas where gravid females give birth or deposit eggs and where juveniles reside during the early stages of their lives (Castro, 1993; Heupel et al., 2007). In the Bahamas, the mangrove habitats of Bimini are long-known to provide critical nursery grounds for juvenile lemon sharks (Morrissey and Gruber, 1993; Kessel et al., 2016). Philopatry to specific natal mangrove nurseries for many years following birth has also been demonstrated for the species in The Bahamas (Feldheim et al., 2002)], the US Virgin Islands (Legare et al., 2015; Legare et al., 2020), and off Cuba (Ruiz-Abierno et al., 2020). Similarly, juvenile blacktip Carcharhinus melanopterus reef sharks (Carcharhinus melanopterus) have been shown to utilize shallow water mangrove ecosystems in Australia (George et al., 2019) and the US Virgin Islands (Deangelis et al., 2008; Legare et al., 2020). Scalloped hammerhead Sphyrna lewini sharks (Sphyrna lewini) are hypothesized to pup their young in coastal mangrove habitat in the Tropical Eastern Pacific (Robles et al., 2015), underscoring an important linkage in their life-history which alternates with offshore migrations at remote oceanic atolls (Salinas-De-León et al., 2017). Alongside viviparous shark species, evidence also suggests that BCEs are key habitats for oviparous (egg laying) species. At two sites along the coast of south-central Chile, catsharks (Scyliorhinidae spp.) selected for taller, thicker kelps when depositing their egg capsules (Trujillo et al., 2019). In this example, the structural complexity of the kelp was positively correlated with the abundance and persistence of egg capsules, as these traits provided better anchorage for oviposition and subsequent protection once the pups had hatched.
In addition to the fitness-level benefits afforded to sharks from the biotic factors of BCEs (prey availability, habitat complexity), several abiotic elements have also been shown to be advantageous. Due to their photosynthetic needs (e.g., access to light and suitable temperatures), most BCEs are restricted to shallow-water habitats. The abiotic components of shallow, nearshore waters can augment reproductive activities by aiding in thermoregulation and enhancing gestation and embryonic development (Hight and Lowe, 2007; Glaus et al., 2019). These patterns may explain why female tiger sharks in The Bahamas utilize shallow, patchy seagrass ecosystems as a gestation ground over multiple years (Sulikowski et al., 2016). Though observations of direct mating behaviour in large sharks remain limited, it is plausible that BCEs may serve as a seasonal encounter location for males and females. For example, nurse sharks are known to mate inside mangrove creeks in the Bahamas and throughout the shallow habitats of The Dry Tortugas, Florida, USA, where the benthic substrate can be dominated by turtle grass Thalassia testudinum (Kramer, 2006; Pratt and Carrier, 2001). However, it has also been hypothesized that the shallow seagrass habitats were used by females to avoid reproductive harassment by males and that mating events at the site could in fact be occurring in deeper waters (Whitney et al., 2010). While the value of BCEs for reproductive activities is supported by studies on tropical – and to a lesser extent temperate – shark species, the strength of these relationships appears to be species and sex-specific and may ultimately be influenced by an overlap of suitable abiotic conditions inherent to BCEs.
Climate change
Climate change is generating profound and widespread effects on marine ecosystems globally, whereby rising sea surface temperatures (SSTs) and heightened ocean acidity, for example, significantly challenge the survival of many marine species (Doney et al., 2012). These changes will affect marine ecosystems differentially; for example, rapid temperature fluctuations and sea level rise associated with climate change are likely to pose greater threats to shallow-water, coastal ecosystems, thus overlapping with many BCEs (Unsworth et al., 2019), and the species which may rely on them for foraging and reproduction.
Climate-related shifts in the spatial distributions of marine-megafauna populations have been documented in response to rising SSTs (Grose et al., 2020). The movements and behaviours of sharks are liable to change in response to climate change (Osgood et al., 2021), which, in turn may affect the manner in which they interact with BCEs. Over a period of ten years, tiger shark distributions in the northwest Atlantic appeared to be influenced by warming oceanographic conditions, as evidenced by a northerly latitudinal expansion in their migrations (Hammerschlag et al., 2022). Similarly, poleward shifts in tiger shark range as a result of rising SSTs were also predicted in the southern hemisphere (Niella et al., 2022). Ecological niche modelling of 25 shark species distributions under different climate change scenarios revealed losses in suitable habitat for 76% of species (Diaz-Carballido et al., 2022). While empirical data on the these effects are in general scarce, the evolutionary history of a given species may predict their responses (Gallagher et al., 2015). For example, for more reproductively specialized species that display philopatry, such as lemon sharks, shifting to new nursery habitats may be difficult (Dulvy et al., 2008). Although warming conditions are predicted to speed up embryonic development in smaller, egg-laying sharks, the combined effects of higher temperatures and elevated CO2 have been shown to significantly reduce metabolic efficiency and energetic demands in the Port Jackson shark (Heterodontus portusjacksoni), a small temperate species commonly found in kelp-dominated BCEs (Pistevos et al., 2015). Lower pH resulted in altered routine metabolic rates and a ~50% reduction in post-hatching survival in the brownbanded bamboo shark (Chiloscyllium punctatum) a small tropical species found in seagrass meadows (Rosa et al., 2014). Aside from the direct effects of oceanic warming on shark behaviour, the environmental perturbations associated with climate change may also indirectly affect shark populations through changes in habitat and prey availability (Chin et al., 2010), and it is predicted that coastal shark species will be most vulnerable to the changes associated with climate change (Knip et al., 2010).
The effects of climate change on BCEs themselves vary widely by region and may be BCE-specific. Yet, as a result of increased SSTs, rising sea levels and elevated atmospheric CO2, it is possible that the global distribution of BCEs will change. The primary producers comprising BCEs can be sensitive to temperature and light level changes due to their photosynthetic requirements, thus BCEs will most likely experience changes if ambient environmental conditions push them beyond natural tolerances (Short and Neckles, 1999). Extreme weather events induced by climate change, such as hurricanes and marine heatwaves, are recognized as some of the biggest drivers of environmental disturbance in coastal ecosystems (Wilson et al., 2020). The impacts of hurricanes on BCEs can vary regionally; however, the most common observation following an extreme weather event is the depletion or complete removal of seagrass beds as documented in the Atlantic and Caribbean (Van Tussenbroek et al., 2008; Wilson et al., 2020; respectively). However, the growth of seagrass species may actually increase following a major hurricane, as seen in multiple seagrass species in the Gulf of Mexico (Oppenheimer, 1963). Indeed, tropical seagrass ecosystems may be resilient to the effects of major storms, as meadows in The Bahamas have remained relatively stable despite being hit by multiple hurricanes over two decades (as discussed in Gallagher et al., 2022).
Protecting BCEs may provide a natural solution to help mitigate the broader impacts of climate change on coastal BCEs, as seagrass meadows have been shown to dissipate wave energy (Luhar et al., 2017), which in turn could limit the damage induced by extreme weather events. The recovery of seagrass ecosystems from storms is slow and can take many years, particularly for shallow-water seagrass species such as Thallasia testudiunum (Macreadie et al., 2015). However, deeper water seagrass meadows containing Halophila decipiens can recover rapidly due to frequent sexual reproduction (Williams, 1988). Thus, with increasing extreme weather events, the community structure of BCEs may change to become dominated by species with more prolific reproduction strategies. Interestingly, the movement of shark populations could potentially reflect these changes if residency within deeper waters increase. Evidence for this has already been highlighted and seasonal shifts of elasmobranch species to deeper waters in response to warming temperatures have been observed (Stebbing et al., 2002; Perry et al., 2005).
Discussion
The surging global interest in blue carbon is largely driven by its potential to serve as a nature-based solution to climate change, which, if implemented properly and transparently, should facilitate robust ocean protections and socioeconomic benefits for society (Macreadie et al., 2015). Blue carbon ecosystems also achieve co-benefits such as fisheries enhancement, coastal resilience, and maintenance of biodiversity. This mini-review suggests that sharks contribute to the topic of BCEs due to the ecological benefits resulting from their behaviour, specifically foraging and reproductive activities, which were identified as major pathways linking sharks and BCEs. While these relationships are relatively complex and likely to vary according to species and region, two main patterns regarding the relationships between BCEs and sharks emerged from our overview: (1) body size has a differential influence whether the nature of the relationship with the BCE will be biased towards foraging or reproductive activity; and (2) climate has a strong influence on the connectedness of these relationships.
Shark size appears to be a strong factor in driving the type of relationship demonstrated within a given BCE. While our review was not exhaustive, the studies highlighted throughout provide evidence for larger species (e.g., tiger and white sharks) utilizing BCEs for foraging benefits stemming from the presence of large, profitable prey items such as turtles and seals which are connected to the broader BCE network. In both of these cases, the primary prey items concomitantly utilize their respective BCE for fitness-related benefits: green sea turtles feed exclusively and selectively on turtle grass, and cape fur seals use the kelp forests for foraging on demersal prey, hiding from predators, and engaging in social behaviours. Smaller-bodied species of sharks also demonstrate extensive use of BCEs for foraging (Newman et al., 2010) and reproductive activities; however the latter tend to be most commonly associated with endemic species with restricted home ranges. Given that predator-prey interactions are a primary force in driving the structure and resilience of marine communities (Hunsicker et al., 2011), we suggest that BCEs offer highly profitable energy landscapes for large sharks (Gallagher et al., 2017a) and the strength of this connectivity to the broader BCE network should scale with size.
Climate change is likely to have a modulating effect on these relationships, as evidenced by the equivocal impacts on BCEs and sharks, with some species and regions exhibiting robust tolerance, whereas others may be sensitive and risk collapsing or becoming displaced. Existing data suggest that smaller, tropical species may experience greater metabolic effects and losses of suitable BCE habitat, whereas larger species may be less affected and could even gain access to new BCEs. The loss of blue carbon habitat due to the effects of climate change, however, illustrates a scenario where there can be detrimental knock-on effects to the broader BCE network. The seagrass ecosystem of Shark Bay, Australia, which collapsed in 2010/2011 following a marine heatwave (Thomson et al., 2015), corresponded with a decrease in the health of herbivores and indirect effects on multiple species throughout the area (Serrano et al., 2021). How carbon burial rates scale up the food-chain and the indirect link between organic carbon stock and shark residency has only ever been theoretically proposed, thus empirical evidence is needed to help explore this relationship. However, our overview also revealed that shark species inhabiting BCEs demonstrate high rates of ecosystem connectivity and carbon flux; the functional role of sharks using BCEs supports ecological resilience, which has direct implications for the health of BCEs in the face of human-induced threats.
BCEs as networks
The presence, direction, and strength of biological interactions – including those between BCEs and sharks, may be best represented as networks (Wey et al., 2008). By applying some of the basic principles of network theory (Jacoby et al., 2012), we can use various network metrics to better understand how ecological information (e.g., food-web) is transmitted in BCEs, and also determine which actors within the BCE play prominent roles in influencing structure of the overall species interaction network. In such a network, each principal actor would comprise an individual node, with interacting nodes connecting with one another to form edges. In this example, each edge could represent species interactions occurring in a locally-nested, non-random fashion (although we recognize this may not always be the case). Outlined above, the foraging ecology of sharks is a key link to BCEs, and BCEs therefore facilitate intense antagonistic networks, with prey species representing a spatially-variable node coupling sharks and BCEs. Networks are almost always temporally dynamic, with edges forming and breaking in response to numerous biotic and abiotic factors (Bartley et al., 2019). Thus, climate change could be considered a modulator to BCE species interaction networks as it will likely impose both direct and indirect impacts (Flores-Yeffal, 2013; D’alelio et al., 2019). To further illustrate this concept, we apply basic network theory to a blue carbon ecological network in The Bahamas, whereby a basic unipartite food-web comprising seven primary actors is structured as (Figure 1): basal BCE resource (seagrass), herbivorous guild (conch, herbivorous fish species, green sea turtle), predatory fishes (carnivorous fish, piscivorous fish), and apex predator (tiger shark). The resulting unipartite network is represented using an adjacency matrix B, where a given set of species S interacts with one another in a square matrix of dimensions (S, S). In this unweighted network, bi,j = 1 when species I and species j interact through direct one-way consumption, and bi,j= 0 when they do not. We recognize the relative simplicity of this model does not take into consideration additional actors nor any potential two-way interactions, nor does it capture spatial or temporal variation. However, we used this example because seagrass meadows in the Bahamas do not exhibit significant spatial or temporal variation, and all local and regional actors utilized therein are found consistently year-round (Gallagher et al., 2022). While more complex models of this system could certainly be constructed, our example follows the notion that most ecological networks are driven by relatively few, strong nodes and links (Ulanowicz et al., 2014).
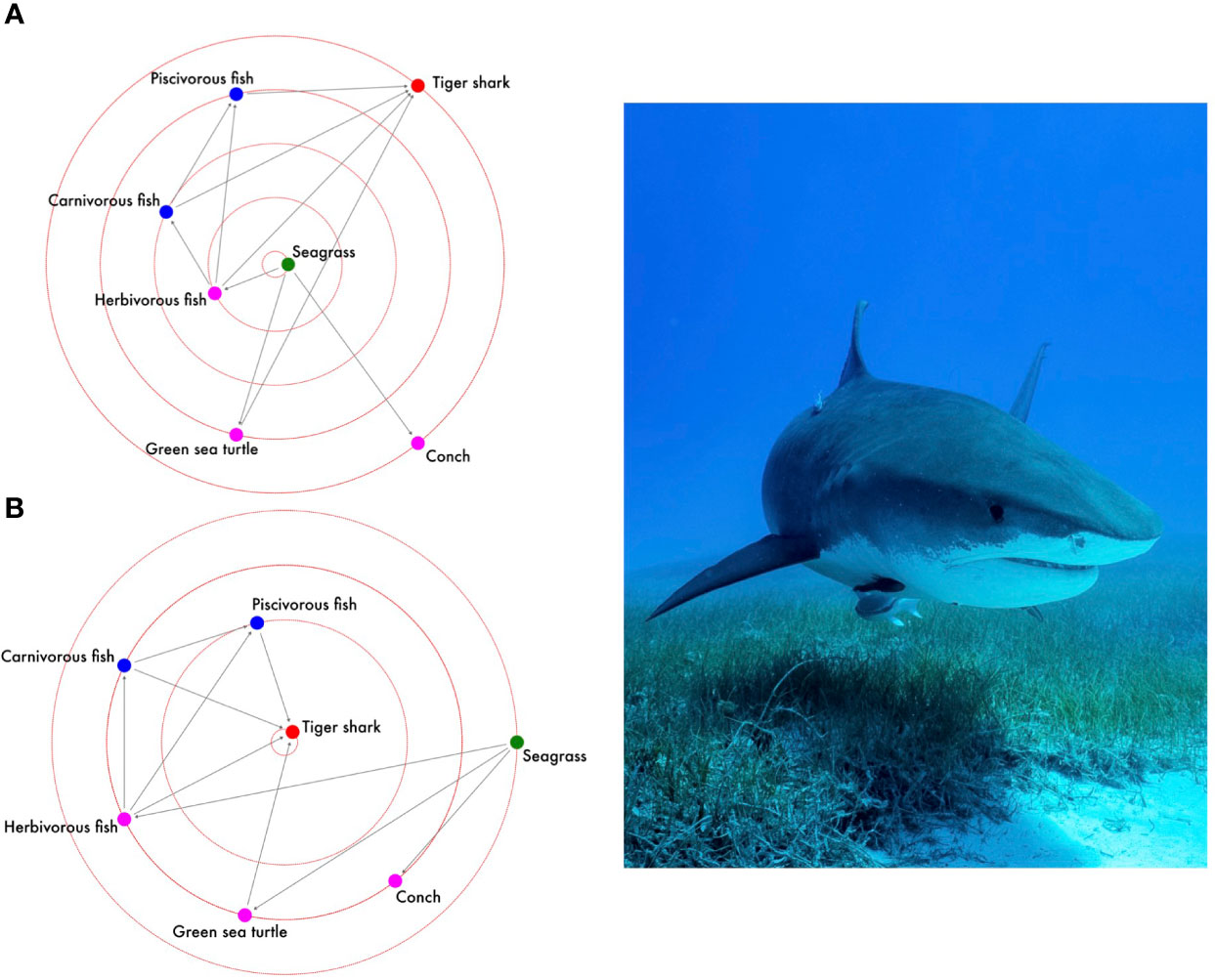
Figure 1 Application of basic social network theory to a tropical seagrass ecosystem, represented by seven key actors (nodes) interacting in a directional unipartite food-web network, highlighting elements of (A) closeness and (B) prestige. Nodes closer to the inside of the radius are associated with higher scores. Theoretical relationships around consumption (parentheses refer to species which are consumed by a given consumer) forming the adjacency matrix were as follows: tiger shark (piscivorous fish, carnivorous fish, herbivorous fish, green sea turtle); piscovorous fish (carnivorous fish, herbivorous fish), carnivorous fish (herbivorous fish), herbivorous fish (seagrass), green sea turtle (seagrass), conch (seagrass).
Here, we present two simple networks to illustrate varying types of prominence: centrality (identifying how food-web information is transmitted through the network) and prestige (identifying which actor is the most influential to network structure). We used influence range closeness centrality (IRCC) as an index for measuring the shortest distance between any two nodes in the network (Faber and Wasserman, 2002; Social Network Visualizer, 2015). For each node u, IRCC is the standardized inverse average distance between u and every other node reachable from it, and is calculated as follows:
where u represents each node, n represents the number of nodes in the network, J represents the nodes reachable by a given node, and d(u,j) represents the average distance of these nodes from node u.
To measure prestige, we used degree prestige to measure the relative prominence of each actor within the network (Wasserman and Fast 2000, Social Network Visualizer, 2015), and is calculated as follows:
where I is the total number of in-connections (edges) for a given node u.
Across both networks, modelled results suggested that seagrass itself served as the primary hub for the spreading of food-web information in the BCE (Figure 1A), and that tiger sharks were the most influential actor within the species interaction network (Figure 1B). Within the broader context of BCEs as complex networks, it is clear that climate change is likely to have a modular effect on the edges connecting sharks to prey species (D’alelio et al., 2019, Nowicki et al., 2019), as well as on prey species connected to seagrass resources. In the most extreme scenario, losses in seagrass abundance due to climate-driven warming of regional SSTs would propagate impacts throughout the BCE and could significantly reduce network connectivity (Nowicki et al., 2017).
Concluding remarks and future directions
BCEs and shark behaviour are a robust model for examining the linkages between biodiversity and climate. However, our knowledge of the relationships between BCEs and shark behaviour is largely restricted to studies performed in subtropical and tropical ecosystems, and also is biased towards those conducted on charismatic, larger bodied species such as tiger sharks and white sharks. Prominent examples within the literature underscore the value of integrative bio-logging approaches for exploring the relationships between sharks and BCEs; namely, that pairing real-time behavioural data obtained via onboard sensors with visual confirmation of habitat type and prey availability can help elucidate the complexity of BCE-shark species interactions. Yet, while larger study species serve as apex predators and likely play key roles in carbon cycling across large distances, there is a need in future studies to expand to smaller-bodied, endemic sharks, and to do so in a broader diversity of ecosystems such as temperate algal forests, and even the deep-sea. The importance of mangroves for facilitating nursery grounds and reproductive activities for coastal shark species is well established; however, there is a clear need to ask similar questions in other BCEs, and to focus on pelagic species’ (e.g. hammerheads), seasonal use of inshore BCEs for reproductive activities. Additionally, the importance of BCE health (e.g., dense vegetation) in driving foraging behaviours and reproductive activities in sharks is also apparent; thus, future studies should examine how variations in BCE quality may affect shark behaviour (and vice-versa). As the study of shark behaviour within BCEs is relatively nascent, and likely to expand, we recognize that a comprehensive assessment of the importance of BCEs to overall shark life histories relative to other habitats is yet to be fully resolved. The manner in which sharks could affect the organic carbon stocks buried or housed within BCEs is cryptic and more empirical data are needed to evaluate these relationships. However, as demonstrated by our topical application of social network theory to BCEs, we contend that the biodiversity supported by BCEs may indeed serve as a functional link between sharks and organic carbon stocks contained within BCEs. Nevertheless, how BCE organic carbon stocks scale up the food chain requires further investigation to establish whether organic carbon content is a key factor determining the use of BCEs for sharks, and if the residency of sharks impacts long-term carbon burial rates. Nevertheless, high rates of ecosystem connectivity are observed for shark species which utilize BCEs, therefore conserving these species is likely to yield ecological benefits that enhance BCE resilience to combat threats such as habitat loss and climate change. Additionally, with the discovery of the largest seagrass meadow in the Bahamas recently documented through long-term research with tiger sharks (consequently raising the known global extent of seagrass by over 40% (Gallagher et al., 2022)), new questions arise as to the true global extent of BCEs. Therefore, sharks should be considered as an important component of the broader topic of BCEs, and studies using sharks to explore BCEs should therefore be expanded, as doing so has clear benefits to satisfy the needs of this emergent field of research (Macreadie et al., 2019), while also providing new information to support the inclusion of BCEs and their demonstrated economic and ecological benefits into marine protected areas (Edgar et al., 2014; Howard et al., 2017).
Finally, two key conservation outcomes should emerge from the expansion of research into this domain: (1) the protection of BCEs will be critical to maintain blue natural capital at the local level, inclusive of biodiversity and carbon sequestration; and (2) the protection of shark species with clear associations to BCEs is imperative in order to sustain the integrity and resilience of BCEs to act long-term nature-based solutions to climate change. Through this lens, sharks may be highlighted as an adaptive climate ally, illuminating an otherwise dark ecosystem service they may provide though safeguarding blue carbon ecosystems.
Author contributions
OD and AG conceived and wrote the manuscript. All authors contributed to the article and approved the submitted version.
Acknowledgments
We thank D. Jacoby and O. Shipley for their thoughtful reviews of earlier drafts of this manuscript, and we are grateful to C. Duarte for productive discussions on this topic. We thank the staff of Beneath The Waves for their assistance with our overall blue carbon research program.
Conflict of interest
The authors declare that the research was conducted in the absence of any commercial or financial relationships that could be construed as a potential conflict of interest.
Publisher's note
All claims expressed in this article are solely those of the authors and do not necessarily represent those of their affiliated organizations, or those of the publisher, the editors and the reviewers. Any product that may be evaluated in this article, or claim that may be made by its manufacturer, is not guaranteed or endorsed by the publisher.
References
Andrzejaczek S., Gleiss A. C., Lear K. O., Pattiaratchi C. B., Chapple T. K., Meekan M. G. (2019). Biologging tags reveal links between fine-scale horizontal and vertical movement behaviors in tiger sharks (Galeocerdo cuvier). Front. Mar. Sci. 6, 229. doi: 10.3389/fmars.2019.00229
Atwood T. B., Connolly R. M., Ritchie E. G., Lovelock C. E., Heithaus M. R., Hays G. C., et al. (2015). Predators help protect carbon stocks in blue carbon ecosystems. Nat. Climate Change 5, 1038–1045. doi: 10.1038/nclimate2763
Bartley T. J., Mccann K. S., Bieg C., Cazelles K., Granados M., Guzzo M. M., et al. (2019). Food web rewiring in a changing world. Nat. Ecol. Evol. 3, 345–354. doi: 10.1038/s41559-018-0772-3
Castro J. I. (1993). The shark nursery of Bulls Bay, South Carolina, with a review of the shark nurseries of the southeastern coast of the United States. Environ. Biol. fishes 38, 37–48. doi: 10.1007/BF00842902
Chin A., Kyne P. M., Walker T. I., Mcauley R. B. (2010). An integrated risk assessment for climate change: analysing the vulnerability of sharks and rays on Australia’s Great Barrier Reef. Global Change Biol. 16, 1936–1953. doi: 10.1111/j.1365-2486.2009.02128.x
Crooks S., Herr D., Tamelander J., Laffoley D., Vandever J. (2011). Mitigating climate change through restoration and management of coastal wetlands and near-shore marine ecosystems: challenges and opportunities. (Washington, DC: Environment Department Paper 121, World Bank).
D’alelio D., Mele H., B. L., S. R., D’alcalà M., Jordán F. (2019). Rewiring and indirect effects underpin modularity reshuffling in a marine food web under environmental shifts. Ecol. Evol. 9, 11631–11646. doi: 10.1002/ece3.5641
Deangelis B. M., Mccandless C. T., Kohler N. E., Recksiek C. W., Skomal G. B. (2008). First characterization of shark nursery habitat in the United States Virgin Islands: evidence of habitat partitioning by two shark species. Mar. Ecol. Prog. Ser. 358, 257–271. doi: 10.3354/meps07308
Dhellemmes F., Smukall M. J., Guttridge T. L., Krause J., Hussey N. E. (2021). Predator abundance drives the association between exploratory personality and foraging habitat risk in a wild marine meso-predator. Funct. Ecol. 35, 1972–1984. doi: 10.1111/1365-2435.13874
Diaz-Carballido P. L., Mendoza-Gonzalez G., Yanez-Arenas C. A., Chiappa-Carrara X. (2022). Evaluation of shifts in the potential future distributions of carcharhinid sharks under different climate change scenarios. Front. Mar. Sci. 8, 2039. doi: 10.3389/fmars.2021.745501
Doney S. C., Ruckelshaus M., Emmett Duffy J., Barry J. P., Chan F., English C. A., et al. (2012). Climate change impacts on marine ecosystems. Annu. Rev. Mar. Sci. 4, 11–37. doi: 10.1146/annurev-marine-041911-111611
Duarte C. M. (2000). Marine biodiversity and ecosystem services: an elusive link. J. Exp. Mar. Biol. Ecol. 250, 117–131. doi: 10.1016/S0022-0981(00)00194-5
Duarte C. M., Losada I. J., Hendriks I. E., Mazarrasa I., Marbà N. (2013). The role of coastal plant communities for climate change mitigation and adaptation. Nat. Climate Change 3, 961–968. doi: 10.1038/nclimate1970
Dulvy N. K., Rogers S. I., Jennings S., Stelzenmüller V., Dye S. R., Skjoldal H. R. (2008). Climate change and deepening of the North Sea fish assemblage: a biotic indicator of warming seas. J. Appl. Ecol. 45, 1029–1039. doi: 10.1111/j.1365-2664.2008.01488.x
Ebert D. (1996). Biology of the sevengill shark Notorynchus cepedianus (PeroPeron 1807) in the temperate coastal waters of southern Africa. South Afr. J. Mar. Sci. 17, 93–103. doi: 10.2989/025776196784158545
Edgar G. J., Stuart-Smith R. D., Willis T. J., Kininmonth S., Baker S. C., Banks S., et al. (2014). Global conservation outcomes depend on marine protected areas with five key features. Nature 506, 216–220. doi: 10.1038/nature13022
Faber A. D., Wasserman S. (2002). Social support and social networks: Synthesis and review. Soc. Networks Health 8, 29–72. doi: 10.1016/S1057-6290(02)80020-1
Feldheim K. A., Gruber S. H., Ashley M. V. (2002). The breeding biology of lemon sharks at a tropical nursery lagoon. Proc. R. Soc. London. Ser. B: Biol. Sci. 269, 1655–1661. doi: 10.1098/rspb.2002.2051
Flores-Yeffal N. Y. (2013). Migration-trust networks: Social cohesion in Mexican US-bound emigration (Texas A&M University Press).
Gallagher A. J., Alsudairy N. A., Shea B. D., Payne N. L., Duarte C. M. (2021). First application of 360-degree camera technology to marine predator bio-logging. Front. Mar. Sci. 8, 707376. doi: 10.3389/fmars.2021.707376
Gallagher A. J., Brownscombe J. W., Alsudairy N. A., Casagrande A. B., Fu C., Harding L., et al. (2022). Tiger sharks support the characterization of the world’s largest seagrass ecosystem. Nat. Commun. 13, 6328. doi: 10.1038/s41467-022-33926-1
Gallagher A. J., Creel S., Wilson R. P., Cooke S. J. (2017a). Energy landscapes and the landscape of fear. Trends Ecol. Evol. 32, 88–96. doi: 10.1016/j.tree.2016.10.010
Gallagher A. J., Hammerschlag N., Cooke S. J., Costa D. P., Irschick D. J. (2015). Evolutionary theory as a tool for predicting extinction risk. Trends Ecol. Evol. 30, 61–65. doi: 10.1016/j.tree.2014.12.001
Gallagher A. J., Kyne P. M., Hammerschlag N. (2012). Ecological risk assessment and its application to elasmobranch conservation and management. J. Fish Biol. 80, 1727–1748. doi: 10.1111/j.1095-8649.2012.03235.x
Gallagher A. J., Shiffman D. S., Byrnes E. E., Hammerschlag-Peyer C., Hammerschlag N. (2017b). Patterns of resource use and isotopic niche overlap among three species of sharks occurring within a protected subtropical estuary. Aquat. Ecol. 51, 435–448. doi: 10.1007/s10452-017-9627-2
Gao G., Beardall J., Jin P., Gao L., Xie S., Gao K. (2022). A review of existing and potential blue carbon contributions to climate change mitigation in the Anthropocene. J. Appl. Ecol. 59, 1686–1699. doi: 10.1111/1365-2664.14173
George L. W., Martins A. P., Heupel M. R., Simpfendorfer C. A. (2019). Fine-scale movements of juvenile blacktip reef sharks Carcharhinus melanopterus in a shallow nearshore nursery. Mar. Ecol. Prog. Ser. 623, 85–97. doi: 10.3354/meps13010
Glaus K. B., Brunnschweiler J. M., Piovano S., Mescam G., Genter F., Fluekiger P., et al. (2019). Essential waters: Young bull sharks in Fiji’s largest riverine system. Ecol. Evol. 9, 7574–7585. doi: 10.1002/ece3.5304
Gorman D., Beale D. J., Crosswell J., Stephenson S. A., Shah R. M., Hillyer K. E., et al. (2023). Multiple-biomarkers show the importance of blue carbon to commercially important fishery species. Sci. Total Environ. 881, 163162. doi: 10.1016/j.scitotenv.2023.163162
Grose S. O., Pendleton L., Leathers A., Cornish A., Waitai S. (2020). Climate change will re-draw the map for marine megafauna and the people who depend on them. Front. Mar. Sci. 547. doi: 10.3389/fmars.2020.00547
Hammerschlag N., Bell I., Fitzpatrick R., Gallagher A. J., Hawkes L. A., Meekan M. G., et al. (2016). Behavioral evidence suggests facultative scavenging by a marine apex predator during a food pulse. Behav. Ecol. Sociobiol. 70, 1777–1788. doi: 10.1007/s00265-016-2183-2
Hammerschlag N., Mcdonnell L. H., Rider M. J., Street G. M., Hazen E. L., Natanson L. J., et al. (2022). Ocean warming alters the distributional range, migratory timing, and spatial protections of an apex predator, the tiger shark (Galeocerdo cuvier). Global Change Biol. 28, 1990–2005. doi: 10.1111/gcb.16045
Hammerschlag N., Williams L., Fallows M., Fallows C. (2019). Disappearance of white sharks leads to the novel emergence of an allopatric apex predator, the sevengill shark. Sci. Rep. 9, 1–6. doi: 10.1038/s41598-018-37576-6
Harcourt R. G., Bradshaw C. J., Dickson K., Davis L. S. (2002). Foraging ecology of a generalist predator, the female New Zealand fur seal. Mar. Ecol. Prog. Ser. 227, 11–24. doi: 10.3354/meps227011
Heithaus M. R. (2001). The biology of tiger sharks, Galeocerdo cuvier, in Shark Bay, Western Australia: sex ratio, size distribution, diet, and seasonal changes in catch rates. Environ. Biol. Fishes 61, 25–36. doi: 10.1023/A:1011021210685
Heithaus M., Dill L., Marshall G., Buhleier B. (2002). Habitat use and foraging behavior of tiger sharks (Galeocerdo cuvier) in a seagrass ecosystem. Mar. Biol. 140, 237–248. doi: 10.1007/s00227-001-0711-7
Heupel M. R., Carlson J. K., Simpfendorfer C. A. (2007). Shark nursery areas: concepts, definition, characterization and assumptions. Mar. Ecol. Prog. Ser. 337, 287–297. doi: 10.3354/meps337287
Hight B. V., Lowe C. G. (2007). Elevated body temperatures of adult female leopard sharks, Triakis semifasciata, while aggregating in shallow nearshore embayments: evidence for behavioral thermoregulation? J. Exp. Mar. Biol. Ecol. 352, 114–128. doi: 10.1016/j.jembe.2007.07.021
Howard J., Mcleod E., Thomas S., Eastwood E., Fox M., Wenzel L., et al. (2017). The potential to integrate blue carbon into MPA design and management. Aquat. Conserv.: Mar. Freshw. Ecosyst. 27, 100–115. doi: 10.1002/aqc.2809
Hunsicker M. E., Ciannelli L., Bailey K. M., Buckel J. A., Wilson White J., Link J. S., et al. (2011). Functional responses and scaling in predator–prey interactions of marine fishes: contemporary issues and emerging concepts. Ecol. Lett. 14, 1288–1299. doi: 10.1111/j.1461-0248.2011.01696.x
Hussey N. E., Kessel S. T., Aarestrup K., Cooke S. J., Cowley P. D., Fisk A. T., et al. (2015). Aquatic animal telemetry: a panoramic window into the underwater world. Science 348, 1255642. doi: 10.1126/science.1255642
Hyde C. A., Notarbartolo Di Sciara G., Sorrentino L., Boyd C., Finucci B., Fowler S. L., et al. (2022). Putting sharks on the map: A global standard for improving shark area-based conservation. Front. Mar. Sci. 1660. doi: 10.3389/fmars.2022.968853
Jacoby D. M., Brooks E. J., Croft D. P., Sims D. W. (2012). Developing a deeper understanding of animal movements and spatial dynamics through novel application of network analyses. Methods Ecol. Evol. 3, 574–583. doi: 10.1111/j.2041-210X.2012.00187.x
Jewell O. J., Gleiss A. C., Jorgensen S. J., Andrzejaczek S., Moxley J. H., Beatty S. J., et al. (2019). Cryptic habitat use of white sharks in kelp forest revealed by animal-borne video. Biol. Lett. 15, 20190085. doi: 10.1098/rsbl.2019.0085
Jorgensen S. J., Micheli F., White T. D., Van Houtan K. S., Alfaro-Shigueto J., Andrzejaczek S., et al. (2022). Emergent research and priorities for shark and ray conservation. Endangered species Res. 47, 171–203. doi: 10.3354/esr01169
Kessel S., Hansell A., Gruber S., Guttridge T., Hussey N. E., Perkins R. (2016). Three decades of longlining in Bimini, Bahamas, reveals long-term trends in lemon shark Negaprion brevirostris (Carcharhinidae) catch per unit effort. J. fish Biol. 88, 2144–2156. doi: 10.1111/jfb.12987
Knip D. M., Heupel M. R., Simpfendorfer C. A. (2010). Sharks in nearshore environments: models, importance, and consequences. Mar. Ecol. Prog. Ser. 402, 1–11. doi: 10.3354/meps08498
Kramer P. (2006). Mangrove creek fish assemblages. Rapid Ecol. Assess. WEST Coast. OF ANDROS THE BAHAMAS JUNE 19th-29th 2006, 19.
Laegdsgaard P., Johnson C. (2001). Why do juvenile fish utilise mangrove habitats? J. Exp. Mar. Biol. Ecol. 257, 229–253. doi: 10.1016/S0022-0981(00)00331-2
Legare B., Deangelis B., Skomal G. (2020). After the nursery: Regional and broad-scale movements of sharks tagged in the Caribbean. Mar. Ecol. 41, e12608. doi: 10.1111/maec.12608
Legare B., Kneebone J., Deangelis B., Skomal G. (2015). The spatiotemporal dynamics of habitat use by blacktip (Carcharhinus limbatus) and lemon (Negaprion brevirostris) sharks in nurseries of St. John, United States Virgin Islands. Mar. Biol. 162, 699–716. doi: 10.1007/s00227-015-2616-x
Leigh S. C., Papastamatiou Y. P., German D. P. (2018). Seagrass digestion by a notorious ‘carnivore’. Proc. R. Soc. B: Biol. Sci. 285, 20181583. doi: 10.1098/rspb.2018.1583
Lovelock C. E., Duarte C. M. (2019). Dimensions of blue carbon and emerging perspectives. Biol. Lett. 15, 20180781. doi: 10.1098/rsbl.2018.0781
Luhar M., Infantes E., Nepf H. (2017). Seagrass blade motion under waves and its impact on wave decay. J. Geophys. Res.: Oceans 122, 3736–3752. doi: 10.1002/2017JC012731
Macreadie P. I., Allen K., Kelaher B. P., Ralph P. J., Skilbeck C. G. (2012). Paleoreconstruction of estuarine sediments reveal human-induced weakening of coastal carbon sinks. Global Change Biol. 18, 891–901. doi: 10.1111/j.1365-2486.2011.02582.x
Macreadie P. I., Anton A., Raven J. A., Beaumont N., Connolly R. M., Friess D. A., et al. (2019). The future of Blue Carbon science. Nat. Commun. 10, 3998. doi: 10.1038/s41467-019-11693-w
Macreadie P. I., Trevathan-Tackett S. M., Skilbeck C. G., Sanderman J., Curlevski N., Jacobsen G., et al. (2015). Losses and recovery of organic carbon from a seagrass ecosystem following disturbance. Proc. R. Soc. B: Biol. Sci. 282, 20151537. doi: 10.1098/rspb.2015.1537
Mateo M., Romero J., Pérez M., Littler M. M., Littler D. S. (1997). Dynamics of millenary organic deposits resulting from the growth of the Mediterranean seagrassPosidonia oceanica. Estuarine Coast. Shelf Sci. 44, 103–110. doi: 10.1006/ecss.1996.0116
Mcleod E., Chmura G. L., Bouillon S., Salm R., Björk M., Duarte C. M., et al. (2011). A blueprint for blue carbon: toward an improved understanding of the role of vegetated coastal habitats in sequestering CO2. Front. Ecol. Environ. 9, 552–560. doi: 10.1890/110004
Moraes O. (2019). Blue carbon in area-based coastal and marine management schemes–a review. J. Indian Ocean Region 15, 193–212. doi: 10.1080/19480881.2019.1608672
Morrissey J. F., Gruber S. H. (1993). Home range of juvenile lemon sharks, Negaprion brevirostris. Copeia 2, 425–434. doi: 10.2307/1447141
Newman S. P., Handy R. D., Gruber S. H. (2010). Diet and prey preference of juvenile lemon sharks Negaprion brevirostris. Mar. Ecol. Prog. Ser. 398, 221–234. doi: 10.3354/meps08334
Niella Y., Butcher P., Holmes B., Barnett A., Harcourt R. (2022). Forecasting intraspecific changes in distribution of a wide-ranging marine predator under climate change. Oecologia 198, 111–124. doi: 10.1007/s00442-021-05075-7
Nowicki R., Heithaus M., Thomson J., Burkholder D., Gastrich K., Wirsing A. (2019). Indirect legacy effects of an extreme climatic event on a marine megafaunal community. Ecol. Monogr. 89, e01365. doi: 10.1002/ecm.1365
Nowicki R. J., Thomson J. A., Burkholder D. A., Fourqurean J. W., Heithaus M. R. (2017). Predicting seagrass recovery times and their implications following an extreme climate event. Mar. Ecol. Prog. Ser. 567, 79–93. doi: 10.3354/meps12029
Oppenheimer C. H. (1963). Effects of hurricane Carla on the ecology of Redfish Bay, Texas. Bull. Mar. Sci. 13, 59–72.
Osgood G. J., White E. R., Baum J. K. (2021). Effects of climate-change-driven gradual and acute temperature changes on shark and ray species. J. Anim. Ecol. 90, 2547–2559. doi: 10.1111/1365-2656.13560
Pendleton L., Donato D. C., Murray B. C., Crooks S., Jenkins W. A., Sifleet S., et al. (2012). Estimating global “blue carbon” emissions from conversion and degradation of vegetated coastal ecosystems. PLoS ONE 7, e43542.
Perry A. L., Low P. J., Ellis J. R., Reynolds J. D. (2005). Climate change and distribution shifts in marine fishes. science 308, 1912–1915. doi: 10.1126/science.1111322
Pillans R. D., Rochester W., Babcock R. C., Thomson D. P., Haywood M. D., Vanderklift M. A. (2021). Long-term acoustic monitoring reveals site fidelity, reproductive migrations, and sex specific differences in habitat use and migratory timing in a large coastal shark (Negaprion acutidens). Front. Mar. Sci. 8, 616633. doi: 10.3389/fmars.2021.616633
Pimiento C., Leprieur F., Silvestro D., Lefcheck J., Albouy C., Rasher D., et al. (2020). Functional diversity of marine megafauna in the Anthropocene. Sci. Adv. 6, eaay7650. doi: 10.1126/sciadv.aay7650
Pistevos J. C., Nagelkerken I., Rossi T., Olmos M., Connell S. D. (2015). Ocean acidification and global warming impair shark hunting behaviour and growth. Sci. Rep. 5, 1–10. doi: 10.1038/srep16293
Powter D. M., Gladstone W. (2008). “Habitat preferences of Port Jackson sharks, Heterodontus portusjacksoni, in the coastal waters of eastern Australia,” in Proceedings of the Linnean Society of New South Wales. 151–165.
Pratt H. L., Carrier J. C. (2001). A review of elasmobranch reproductive behavior with a case study on the nurse shark, Ginglymostoma cirratum. Environ. Biol. Fishes 60, 157–188. doi: 10.1023/A:1007656126281
Robles Y. A., Montes L. A., Vega Á.J. (2015). Caracterización de la captura de Tiburones por la Pesca Artesanal en Los Manglares de David, Golfo de Chiriqui, Pacifico de Panama. Tecnociencia 17, 11–30.
Rosa R., Baptista M., Lopes V. M., Pegado M. R., Ricardo Paula J., Trübenbach K., et al. (2014). Early-life exposure to climate change impairs tropical shark survival. Proc. R. Soc. B: Biol. Sci. 281, 20141738. doi: 10.1098/rspb.2014.1738
Ruiz-Abierno A., Márquez-Farías J. F., Hueter R. E., Macías-Romero L., Barros-García J. M., García-Córdova L., et al. (2020). Distribution and length composition of lemon sharks (Negaprion brevirostris) in a nursery ground in southern Cuba. Environ. Biol. Fishes 103, 1583–1594. doi: 10.1007/s10641-020-01050-y
Salinas-De-León P., Hoyos-Padilla E., Pochet F. (2017). First observation on the mating behaviour of the endangered scalloped hammerhead shark Sphyrna lewini in the Tropical Eastern Pacific. Environ. Biol. Fishes 100, 1603–1608. doi: 10.1007/s10641-017-0668-0
Serrano O., Arias-Ortiz A., Duarte C. M., Kendrick G. A., Lavery P. S. (2021). “Impact of marine heatwaves on seagrass ecosystems,” in Ecosystem Collapse and Climate Change (Springer).
Serrano O., Mateo M., Renom P., Julià R. (2012). Characterization of soils beneath a Posidonia oceanica meadow. Geoderma 185, 26–36. doi: 10.1016/j.geoderma.2012.03.020
Shipley O. N., Gallagher A. J., Shiffman D. S., Kaufman L., Hammerschlag N. (2019). Diverse resource-use strategies in a large-bodied marine predator guild: evidence from differential use of resource subsidies and intraspecific isotopic variation. Mar. Ecol. Prog. Ser. 623, 71–83. doi: 10.3354/meps12982
Shipley O. N., Matich P., Hussey N. E., Brooks A. M., Chapman D., Frisk M. G., et al. (2023). Energetic connectivity of diverse elasmobranch populations–implications for ecological resilience. Proc. R. Soc. B 290, 20230262. doi: 10.1098/rspb.2023.0262
Short F. T., Neckles H. A. (1999). The effects of global climate change on seagrasses. Aquat. Bot. 63, 169–196. doi: 10.1016/S0304-3770(98)00117-X
Sims D. W., Quayle V. A. (1998). Selective foraging behaviour of basking sharks on zooplankton in a small-scale front. Nature 393, 460–464. doi: 10.1038/30959
Social Network Visualizer (SocNetV). (2015). Social network analysis and visualization software. Home page: https://socnetv.org.
Stebbing A., Turk S., Wheeler A., Clarke K. (2002). Immigration of southern fish species to south-west England linked to warming of the North AtlantiAtlantic, (1960–2001). J. Mar. Biol. Assoc. United Kingdom 82, 177–180. doi: 10.1017/S0025315402005325
Sulikowski J. A., Wheeler C. R., Gallagher A. J., Prohaska B. K., Langan J. A., Hammerschlag N. (2016). Seasonal and life-stage variation in the reproductive ecology of a marine apex predator, the tiger shark Galeocerdo cuvier, at a protected female-dominated site. Aquat. Biol. 24, 175–184. doi: 10.3354/ab00648
Thomson J. A., Burkholder D. A., Heithaus M. R., Fourqurean J. W., Fraser M. W., Statton J., et al. (2015). Extreme temperatures, foundation species, and abrupt ecosystem change: an example from an iconic seagrass ecosystem. Global Change Biol. 21, 1463–1474. doi: 10.1111/gcb.12694
Towner A. V., Leos-Barajas V., Langrock R., Schick R. S., Smale M. J., Kaschke T., et al. (2016). Sex-specific and individual preferences for hunting strategies in white sharks. Funct. Ecol. 30, 1397–1407. doi: 10.1111/1365-2435.12613
Trujillo J. E., Pardo L. M., Vargas-Chacoff L., Valdivia N. (2019). Sharks in the forest: relationships between kelp physical-complexity attributes and egg deposition sites of the red-spotted catshark. Mar. Ecol. Prog. Ser. 610, 125–135. doi: 10.3354/meps12818
Ulanowicz R. E., Holt R. D., Barfield M. (2014). Limits on ecosystem trophic complexity: insights from ecological network analysis. Ecol. Lett. 17, 127–136. doi: 10.1111/ele.12216
Unsworth R. K., Mckenzie L. J., Collier C. J., Cullen-Unsworth L. C., Duarte C. M., Eklöf J. S., et al. (2019). Global challenges for seagrass conservation. Ambio 48, 801–815. doi: 10.1007/s13280-018-1115-y
Van Tussenbroek B. I., arba Santos B.M. G., Van Dijk J. K., Sanabria Alcaraz S. M., Téllez Calderón M. L. (2008). Selective elimination of rooted plants from a tropical seagrass bed in a back-reef lagoon: a hypothesis tested by hurricane Wilma, (2005). J. Coast. Res. 24, 278–281. doi: 10.2112/06-0777.1
Vásquez-Castillo S., Hinojosa I. A., Colin N., Poblete A. A., Górski K. (2021). The presence of kelp Lessonia trabeculata drives isotopic niche segregation of redspotted catshark Schroederichthys Chilensis. Estuarine Coast. Shelf Sci. 258, 107435. doi: 10.1016/j.ecss.2021.107435
Vaudo J. J., Heithaus M. R. (2011). Dietary niche overlap in a nearshore elasmobranch mesopredator community. Mar. Ecol. Prog. Ser. 425, 247–260. doi: 10.3354/meps08988
Vierros M. (2017). Communities and blue carbon: the role of traditional management systems in providing benefits for carbon storage, biodiversity conservation and livelihoods. Climatic Change 140, 89–100. doi: 10.1007/s10584-013-0920-3
Wey T., Blumstein D. T., Shen W., Jordán F. (2008). Social network analysis of animal behaviour: a promising tool for the study of sociality. Anim. Behav. 75, 333–344. doi: 10.1016/j.anbehav.2007.06.020
Whitney N. M., Pratt J. H.L., Pratt T. C., Carrier J. C. (2010). Identifying shark mating behaviour using three-dimensional acceleration loggers. Endangered Species Res. 10, 71–82. doi: 10.3354/esr00247
Williams S. L. (1988). Disturbance and recovery of a deep-water Caribbean seagrass bed. Mar. Ecol. Prog. series. Oldendorf 42, 63–71. doi: 10.3354/meps042063
Keywords: blue carbon, climate change, ecosystem, network, shark, predator-prey
Citation: Dixon OFL and Gallagher AJ (2023) Blue carbon ecosystems and shark behaviour: an overview of key relationships, network interactions, climate impacts, and future research needs. Front. Mar. Sci. 10:1202972. doi: 10.3389/fmars.2023.1202972
Received: 09 April 2023; Accepted: 01 August 2023;
Published: 01 September 2023.
Edited by:
Martin Gullström, Södertörn University, SwedenReviewed by:
Stuart James Kininmonth, The University of Queensland, AustraliaCopyright © 2023 Dixon and Gallagher. This is an open-access article distributed under the terms of the Creative Commons Attribution License (CC BY). The use, distribution or reproduction in other forums is permitted, provided the original author(s) and the copyright owner(s) are credited and that the original publication in this journal is cited, in accordance with accepted academic practice. No use, distribution or reproduction is permitted which does not comply with these terms.
*Correspondence: Olivia F. L. Dixon, bGl2QGJlbmVhdGh0aGV3YXZlcy5vcmc=