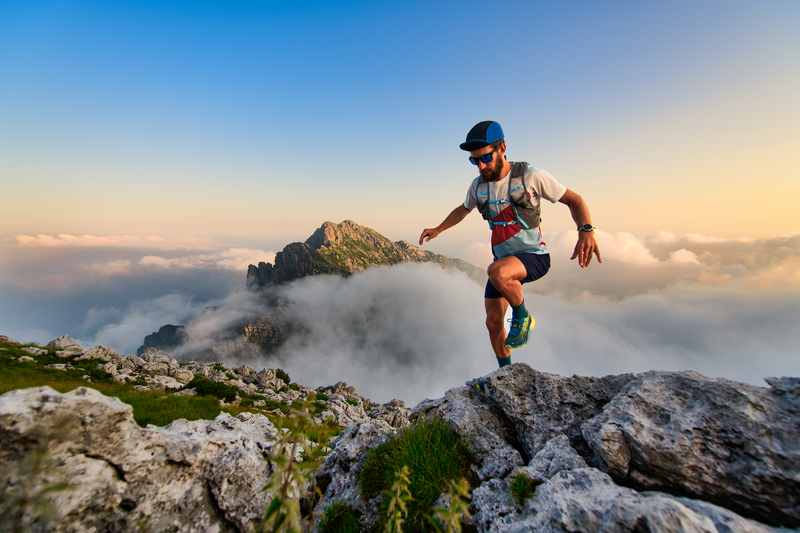
95% of researchers rate our articles as excellent or good
Learn more about the work of our research integrity team to safeguard the quality of each article we publish.
Find out more
ORIGINAL RESEARCH article
Front. Mar. Sci. , 08 May 2023
Sec. Marine Biotechnology and Bioproducts
Volume 10 - 2023 | https://doi.org/10.3389/fmars.2023.1202437
This article is part of the Research Topic Biological Macromolecules from Marine Organisms: Isolation, Characterization and Pharmacological Activities View all 6 articles
Scallops are one of the main marine products of Hokkaido, Japan. In addition to adductor muscle, scallop mantle tissue is often consumed in Japan. Previously, we showed that feeding mice a diet containing 1% mantle tissue resulted in lower food consumption and, ultimately, death. In this study, we isolated and identified toxic substances from scallop mantle tissue. The isolated toxic substances were protein complexes with molecular weights of 18 kDa and 29 kDa. Feeding mice a diet containing 0.05% toxic substances led to their death at five weeks. Based on LC-MS/MS analysis, the 29-kDa and 18-kDa proteins were identified as an actin fragment and the N-terminal fragment of the gelsolin-like protein, respectively. The 18-kDa protein was expressed in the mantle, gill, and ovary but not in the adductor muscle, testis, or midgland. Toxicity was observed only in mouse tissues expressing the 18-kDa protein. Feeding mice a diet containing only the 18-kDa protein did not induce decreased food consumption or death, implying that both the 29-kDa and 18-kDa complexes are essential for toxicity. This is the first study to identify a novel toxin in scallop tissues.
Shellfish such as scallops, clams, and mussels that feed on toxic marine algae can accumulate algal toxins, and most toxins exclusively accumulate in the digestive glands (Kumar et al., 2021). The toxicity of paralytic shellfish poisoning (PSP), diarrhetic shellfish poisoning (DSP), and amnesic shellfish poisoning (ASP) toxins is well-known (Matsushima et al., 2015; Young et al., 2020; Pradhan et al., 2022). The most well-known PSP, ASP, and DSP toxins are saxitoxin, domoic acid, and okadaic acid, respectively. Most studies on these toxins have focused on acute toxicity. Only a few studies have been conducted on the chronic and subacute toxicities of these toxins, and no data have been reported in humans (Truelove et al., 1996; O’Neill et al., 2016; Ferriss et al., 2017). Repeated exposure of rats to subacute amounts of saxitoxin for 12 weeks caused a reduction in body weight and food intake during the treatment period; however, the animals recovered quickly when the treatment was interrupted (Zepeda et al., 2014). Although the oral administration of subacute doses of domoic acid to rats for 64 days did not induce any clinical changes, morphological changes were observed in the hippocampus (Pulido, 2008). Chronic exposure to DSP toxins increases the risk of gastrointestinal cancer (Manerio et al., 2008). Lopez-Rodas et al. reported correlations between the consumption of DSP toxins and the total incidence of tumors (Lopez-Rodas et al., 2006).
Many proteinaceous toxins from plant seeds and botulinum toxins have been identified (Sandvig et al., 2010; Janik et al., 2019; Polito et al., 2019). However, few studies have reported the oral toxicity of proteinaceous toxins owing to their degradation in the gastrointestinal tract. Notably, ricin and abrin from plants have been reported to induce acute oral toxicity in ICR mice at doses of approximately 5–20 mg/kg (Garber, 2008); nonetheless, there have been no reports of subacute or chronic toxicity.
Scallop mantle tissue is widely consumed in Japan as sashimi and smoked food. Our previous studies showed that continuously feeding mice a diet containing 0.1% mantle tissue causes death (Hasegawa et al., 2018; Kariya et al., 2019; Kariya and Hasegawa, 2020). The continuous consumption of scallop mantle tissue causes toxic changes in the liver and kidneys of rats. Subacute toxicity of scallop mantle tissue is associated with water-soluble proteins with molecular weights greater than 10 kDa (Hasegawa et al., 2018). This toxin from scallop mantle tissue may be the first proteinaceous toxin discovered to cause toxicity following oral and chronic administration.
In this study, we report the isolation and identification of a novel proteinaceous toxin from the scallop mantle.
Commercially available scallops of the species Patinopecten yessoensis, harvested from Mutsu Bay, Aomori, Japan, were purchased. Rat pheochromocytoma cells (PC12) and human fibroblasts (TIG-20) were purchased from the JCRB Cell Bank (Osaka, Japan).
Mantle tissue was isolated, washed with deionized water, then lyophilized, and ground in a mill. The product (20 g) was suspended in 500 mL of 20 mM Tris-HCl (pH 7.5) and centrifuged at 12,000 × g for 15 min at 4°C. The supernatant was used as the mantle extract. The extract was subjected to Q-Sepharose column chromatography and equilibrated with 20 mM Tris-HCl (pH 7.5). After washing with 20 mM Tris-HCl (pH 7.5), the adsorbed substances were eluted using an NaCl concentration gradient ranging from 0 to 300 mM. The eluent was fractionated using ammonium sulfate precipitation (45–60%), followed by gel filtration on a Sephacryl S-200 column equilibrated with a solution containing 0.5 M NaCl and 20 mM Tris-HCl (pH 7.5). The fractions with toxic activity were pooled. When contaminated proteins were detected, the fractions were further purified using a hydroxyl apatite column. After adsorbing the proteins onto a hydroxyl apatite column equilibrated with 20 mM potassium phosphate, the toxic substances were eluted with a concentration gradient of phosphate buffer from 20 to 200 mM. Purified toxic substances were pooled and lyophilized after dialysis against 20 mM ammonium bicarbonate.
The 18-kDa protein was isolated by subjecting a fraction of ammonium sulfate (45–60%) to phenyl-Sepharose column chromatography equilibrated with 2 M ammonium sulfate and eluted with a concentration gradient from 2 to 0 M ammonium sulfate. Fractions containing the 18-kDa protein were further fractionated using a Sephacryl S200 gel filtration column and purified. The 18-kDa fractions were pooled and lyophilized after dialysis against 20 mM ammonium bicarbonate.
Synthetic genes corresponding to the N-terminal region of a gelsolin-like protein (amino acid number 1–168) with XhoI and NdeI restriction sites at the 5’- and 3’-ends were purchased from Genscript (Tokyo, Japan). The synthetic DNA was ligated into the XhoI–NdeI sites of the pET24a vector with a polyhistidine tag (6xHis) (Takara, Shiga, Japan) and transformed into E. coli strain TOP10F. A single colony was grown on Luria–Bertani (LB)-kanamycin-agar plates at 37°C in an LB medium containing 100 μg/L of kanamycin. Gene expression was induced by the addition of 100 mL of 0.5 mM IPTG to the cultures, which were cultured for 6 h at 30°C. E. coli were recovered by centrifugation at 4,000 × g for 20 min at 4°C and were suspended in a solution containing 20 mM Tris-HCl (pH 7.4), 100 mM NaCl, and 1% Triton X-100 (solution A). Harvested E.coli were disrupted by sonication, and the precipitate (inclusion bodies) containing a recombinant protein was recovered by centrifugation at 12,000 × g for 10 min at 4°C. The precipitate was resuspended in solution A and sonicated. After centrifugation at 12,000 × g for 10 min at 4°C, the precipitate was solubilized in 6 M urea and 20 mM Tris-HCl (pH 7.5). The solution was dialyzed several times at 4°C against 20 mM Tris-HCl (pH 7.5) for refolding of a recombinant protein from inclusion bodies. The solution was centrifuged at 12,000 × g for a further 10 min at 4°C, and the soluble fraction was pooled. The solubilized proteins were subjected to nickel ion chelate affinity chromatography equilibrated with 20 mM imidazole. After washing with 20 mM imidazole, adsorbed recombinant proteins were eluted with 200 mM imidazole. The purity of the recombinant protein was confirmed using SDS-PAGE.
Four-week-old male ICR mice were purchased from CLEA (Tokyo, Japan). The mice were housed individually in a room at 22°C. Three or four mice were housed in cages and maintained at 22°C with free access to water and food. The mice were acclimatized for at least seven days and used for each experiment. The mice were fed either a normal AIN-93G based diet (control diet) or an AIN-93G based diet containing 0.5% mantle tissue or 0.05–0.25% of each fraction from column chromatography (mantle diet) at 4 g/d for 4–7 weeks (Hasegawa et al., 2018). The diet compositions are shown in Table 1. Food intake was monitored daily.
The toxicity of each fraction was evaluated in three mice. We assumed that a fraction contained toxic substances if all three mice died after six or seven weeks of consuming a diet containing that fraction. The amount of each fraction or tissue in the diet was determined based on previous studies [16] and preliminary experiments.
Animal experiments were conducted per the Guidelines of Experimental Animal Care issued by the Office of the Prime Minister of Japan and the Muroran Institute of Technology. The experiments were approved by the Committee on the Ethics, Care, and Use of Animal Experiments of the Muroran Institute of Technology (Permit Number: H29-KS04). All the mice were monitored for health status by checking daily food intake, water intake, and observing their appearance during the experimental period.
The partially purified toxic substance was subjected to HCl solution at pH 2 for 60 minutes at room temperature. The pH was subsequently restored to pH 7 with the addition of 1 M sodium hydroxide, and the precipitated denatured protein was separated by centrifugation at 12,000g for 30 minutes. The resulting supernatant was dialyzed against distilled water and lyophilized. The diet containing 0.5% of lyophilized product was administered to the mice.
Four mice were anesthetized four weeks after the initiation of the diet containing 0.5% mantle tissue or the control diet. Blood was collected from the heart, and the serum was separated via centrifugation at 500 × g for 5 min. Biochemical analyses were performed using a HITACHI automatic analyzer 7180 (Hitachi, Japan).
Rat pheochromocytoma cells (PC12) were maintained in a Roswell Park Memorial Institute (RPMI) 1640 medium supplemented with 10% horse serum and 5% FBS. TIG-20 cells were maintained in Eagle’s minimal essential medium containing 10% FBS. Cells were maintained at 37°C in a humidified atmosphere containing 5% CO2. Cells were seeded at a density of 4 × 103 cells/well in a 96-well plate. After the cells were treated with the mantle toxin at the indicated concentrations for 24 h, the cell morphology was photographed using phase-contrast microscopy. Mantle toxicity was estimated using (3-(4,5-dimethylthiazol-2-yl)-2,5-diphenyltetrazolium bromide (MTT) assay (Manthorpe et al., 1986).
SDS-PAGE was performed utilizing the method of Leammli (Laemmli, 1970). A sample buffer containing 2% SDS and bromophenol was added to each fraction after column chromatography. Native PAGE was performed as described for SDS-PAGE in the absence of SDS.
Western blotting was performed as follows. After SDS-PAGE, proteins were transferred onto nitrocellulose membranes. The membranes were blocked with 5% skim milk (w/v) in a solution containing 0.1 M NaCl, 20 mM Tris-HCl (pH 7.5), and 0.05% Tween 20 before the addition of the 18-kDa antibody, followed by incubation with secondary antibodies, alkaline phosphatase-conjugated goat anti-rabbit IgG. Color development was performed using nitro blue tetrazolium and 5-bromo-4-chloro-3-indoyl phosphate.
Rabbits were first immunized with 500 μg of the 18-kDa protein with Freund’s complete adjuvant. Three successive injections of 500 μg of 18-kDa protein in incomplete adjuvant were performed at intervals of two weeks. Blood was collected after the final immunization, and the antiserum was separated using centrifugation at 500 × g for 5 min. The antiserum was fractionated using 30–60% ammonium sulfate, dialyzed against phosphate-buffered saline and used as an 18-kDa antibody.
Following the SDS-PAGE of the toxic substances, stained bands of 29-kDa and 18-kDa proteins were excised from the gel and subjected to LC-MS/MS analysis (Gundry et al., 2009). The gel was treated with 50% acetonitrile for six hours, after which all the solutions were removed. After the addition of 10 mM dithiothreitol, the gel pieces were alkylated in 10 mM iodoacetamide in the dark for one hour. The solution was removed, and the gel was incubated with 10 ng/µL trypsin in 30% acetonitrile at 37°C overnight. Subsequently, the peptide products were extracted from the gel fragments using 50% acetonitrile and 0.1% formic acid, and the tryptic fragments were subjected to protein identification using QSTAR-XL (Thermo Fisher Scientific Inc., Tokyo, Japan). Mass spectrometry data were analyzed using the MASCOT search engine to identify amino acid sequences. Proteins with a significant match score (p < 0.05) were identified.
The scallop mantle, gonad, adductor muscle, testis, mantle, gill, midgland, and ovary tissues were excised from live scallops, and total RNA was extracted using an RNAiso Plus Kit (Takara, Shiga, Japan) and purified [17, 18]. After cDNA synthesis, PCR was performed using the following primers specific for genes encoding scallop actin and gelsolin-like protein: the forward primer 5’- GTTCGAGACCTTCAACGCTC -3’ and the reverse primer 5’- GTAATCGGTCAAGTCACGGC -3’ for actin and the forward primer 5’-ATGTTTTCAGGTTTGAGAAAGGCAAAGAAGTACAA-3’ and the reverse primer 5’-CTCCACTGTCCTACGACTACCGCTGAAGTGAAGCA-3’ for gelsolin-like protein.
Data were expressed as mean ± standard deviation (SD). Data were analyzed using one-way analysis of variance (one-way ANOVA), followed by Tukey’s multiple comparison test or Student’s t-test.
In a previous study, we showed that consumption of a diet containing scallop mantle tissue increased blood alanine aminotransferase (ALT) and urea nitrogen (BUN) levels in serum, which are markers of liver and kidney injury, and caused death in rats (Hasegawa et al., 2018). In this study, we performed a detail serum biochemistry, which we did not perform previously. We found that amylase secretion from the pancreas was significantly reduced, although parameters such as albumin, creatinine, and bilirubin levels did not change (Table 2).
We isolated and identified toxic substances in mantle tissue. The mantle extract was subjected to Q-Sepharose column chromatography, equilibrated with 20 mM Tris-HCl (pH 7.5), and eluted using a linear gradient of NaCl (Figure 1A). The four fractions were pooled, and diets containing 0.25% (w/w) of each fraction were fed to the mice. Feeding a diet containing fraction 4 reduced food intake, and all three mice died within five weeks (Figure 1B). In contrast, the mice fed diets containing fractions 1 to 3 did not die. Fraction 4, which exhibited toxic activity, was pooled, and fractionated using ammonium sulfate.
Figure 1 Separation of mantle extract using Q-Sepharose column chromatography and toxicity of each fraction. (A) Mantle extract was fractionated in four fractions using Q-Sepharose column chromatography. (B) Diets containing 0.5% of each fraction (1–4) or mantle tissue were fed to mice. The food intake for each week is shown. Data represent the means of three mice, and bars indicate standard deviation (SD).
The toxicity of the fractions containing 0–30%, 30–45%, and 45–60% ammonium sulfate was examined (Figures 2A, B). Although all mice fed the 0–30% and 45–60% fractions died by week 4, the 45–60% fraction was pooled and subjected to S200 gel filtration (Figure 3A). Two peaks were observed, and toxicity assays for peaks 1 and 2 were performed using diets containing 0.025% of each fraction. All three mice fed a diet containing peak 2 died within six weeks (Figure 3C). Sodium dodecyl sulfate-polyacrylamide gel electrophoresis (SDS-PAGE) analysis showed that two proteins with molecular weights of 29 kDa and 18 kDa co-eluted in Peak 2 (Figure 3B). When contaminated proteins, except 29-kDa and 18-kDa proteins, were observed in peak 2, the fraction was further purified using a hydroxyl apatite column (Figures 4A–C). The fraction adsorbed onto the hydroxyl apatite column contained 29-kDa and 18-kDa proteins, which showed toxicity.
Figure 2 Toxicity after fractionation using ammonium sulfate. Fraction 4 after Q-Sepharose column chromatography was fractionated at 0–30%, 30–45%, and 45–60% of ammonium sulfate. (A) Diets containing 0.5% of each fraction were fed to mice. (B) SDS-PAGE profile of each fraction is indicated. MW indicates molecular weight marker. The food intake for each week is shown. Data represent averages of three mice, and bars show standard deviation (SD).
Figure 3 Fractionation via S-200 gel filtration column chromatography and toxicity of each fraction. (A) 45–60% ammonium sulfate fraction was fractionated in two fractions (peak 1 and peak 2) using S-200 gel filtration column chromatography. (B) SDS-PAGE profile of each fraction is indicated. The gel was silver stained. MW and CA indicate molecular weight marker and carbonic anhydrase with a molecular weight of 29 kDa, respectively. (C) Diets containing 0.05% of peak 1 (closed bar) or peak 2 (open bar) were fed to mice. The food intake for each week is indicated. Data represent the means of three mice, and bars show standard deviation (SD).
Figure 4 Separation using hydroxyl apatite column and toxicity of each fraction. (A) Peak 2 after gel filtration was fractionated in two fractions (adsorbed and unadsorbed) using hydroxyl apatite column chromatography. (B) SDS-PAGE profile of each fraction is indicated. MW and CA indicate molecular weight markers and carbonic anhydrase, respectively. (C) Diets containing 0.5% of adsorbed fraction (open bar) or unadsorbed fraction (closed bar) were fed to mice. The food intake for each week is indicated. Data represent the means of three mice, and bars show SD.
To identify the 29-kDa and 18-kDa proteins, we performed LC-MS/MS analysis of the tryptic fragments of each protein. LC-MS/MS analysis of the 29-kDa and 18-kDa fragments matched with actin, the cytoplasm of scallop Mizuhopecten yessoensis (OWF37106.1) at positions 97–327, and gelsolin-like protein 2 isoform X1 of scallop Mizuhopecten yessoensis (XP_021369890.1) at positions 32–168 (Figures 5A, B). These results implied that the 29-kDa and 18-kDa proteins were fragments of scallop actin with a molecular weight of 42 kDa and scallop gelsolin-like protein with a molecular weight of 39 kDa, respectively. The 18-kDa protein showed approximately 47% homology with the N-terminal region called the G1 domain of mouse gelsolin (NP_666232) and contained a sequence homologous to the G-actin-binding region of mouse gelsolin (Figures 5C, D) (Burtnick et al., 2004; Spinardi and Witke, 2007).
Figure 5 Identification of the 29-kDa and 18-kDa protein using LC-MS/MS. (A) Amino acid sequences of scallop gelsolin-like protein (XP_021369890.1) and (B) scallop actin (OWF37106.1) gelsolin-like protein (XP_021369890.1). Fragments identified using LC-MS/MS are indicated by red letters. The sequence homologous to the G-actin-binding region of mouse gelsolin is indicated by yellow shading. (C) Alignment of scallop gelsolin-like protein and mouse gelsolin. Asterisks indicate identical amino acids, while colons indicate similar amino acids. (D) Schematic comparison with mouse gelsolin which is composed of six domains.
We isolated and purified the 18-kDa protein as described in the “Materials and Methods” section to confirm that it was a gelsolin-like protein. In addition, we produced a recombinant protein corresponding to the N-terminal region (amino acid number 1–168) of the gelsolin-like protein. Isolated 18-kDa protein and the recombinant protein showed a single band on SDS-PAGE (Figures 6A, B). The polyclonal antibody produced against the 18-kDa protein recognized the recombinant protein and the 18-kDa protein in the isolated toxic substance (Figures 6C, D), showing that the 18-kDa protein is the N-terminal region of the gelsolin-like protein. The antibody recognized two bands of 18-kDa and 35-kDa proteins in the mantle extract (Figure 6D), which implies that the 18-kDa protein was not a degradation product generated during the purification process.
Figure 6 Reactivity of the 18-kDa protein and a recombinant protein with anti-18-kDa protein antibody. (A) SDS-PAGE profile of purified 18-kDa protein, which was used as an antigen and (B) the isolated recombinant protein. (C) Western blot profile of a recombinant protein. (D) Western blot profile of the isolated toxic substance and mantle extract. MW indicates molecular weight marker.
Next, we investigated whether the 29- and 18-kDa proteins form a complex (Figures 7A, B). Native PAGE of fractions containing both 29-kDa and 18-kDa proteins showed two bands different from the band of the 18-kDa protein alone (Figure 7B), suggesting that the isolated toxic substances are a complex of 29-kDa and 18-kDa proteins.
Figure 7 Binding of 29-kDa and-18 kDa proteins. (A) SDS-PAGE and (B) native PAGE profiles of isolated 18-kDa protein and fraction containing 29-kDa and 18-kDa proteins. Isolated 18-kDa protein shows doublet bands which may be a degradation product. MW and CA indicate molecular weight markers and carbonic anhydrase, respectively.
We examined the expression of a gelsolin-like protein and actin fragments in each scallop tissue sample using RT-PCR. Although actin was expressed in all tissues, the gelsolin-like protein was expressed in the gill, mantle, and ovary but not in the adductor muscle, testis, and midgland (Figure 8A). We have previously shown that diets containing gills and ovaries but not adductor muscles or testis are toxic (Hasegawa et al., 2018). In this study, we investigated the toxicity of the midgland. The mice fed a diet containing 0.5% midgland did not show a decrease in food intake (Figure 8B). These results indicated that only tissues of the gill, mantle, and ovary, which express gelsolin-like proteins, exhibited toxicity, strongly supporting the hypothesis that the 18-kDa protein is a toxic substance.
Figure 8 The expression of the gelsolin-like protein in each tissue was investigated using RT-PCR. (A) Actin and gelsolin-like protein in each tissue was amplified using specific primers as described in “Materials and Methods.” (B) Diets containing 0.5% of midgland (hatched bar) or mantle (open bar) were fed to three mice. The control diet is indicated by a closed bar. The food intake for each week is indicated. (C) A diet containing 0.5% of 18-kDa protein or a recombinant protein was fed to three mice. The food intake for each week is indicated. Data represent the means of three mice, and bars show SD.
Finally, we examined whether the 29- and 18-kDa complexes were essential for toxicity. Diets containing 0.25% of the 18-kDa protein alone or a recombinant protein were fed to mice for five or six weeks; however, toxicity was not observed (Figure 8C), indicating that the 29-kDa and 18-kDa complex is essential for toxicity in mice.
We investigated whether the toxic substance is stable under the acidic conditions of stomach. The partially purified toxic substance was treated with a HCl solution at pH 2 for 60 minutes at room temperature and then restored to pH 7. After centrifugation, the supernatant and precipitate fractions were analyzed using SDS-PAGE and western blot (Figures 9A, B). The 18 kDa protein was recovered in the supernatant fraction and mice fed a diet containing the supernatant fraction died at four weeks (Figure 9C). This result shows that the 18 kDa protein is stable even after acidic treatment.
Figure 9 Stability of toxic substance under acidic condition was investigated. (A) SDS-PAGE and (B) western blot profiles of supernatant (S) and precipitate (P) fractions after acidic treatment. (C) Diets containing 0.5% of fraction containing the supernatant fraction (closed bar) or mantle extract (open bar) were fed to mice. The food intake for each week is indicated. Data represent the means of three mice, and bars show SD.
The action of toxic substances on cultured cells was examined using PC12 and fibroblast cells (Figures 10A–E). In this study, we used PC12 and fibroblast cells with characteristic morphology to investigate whether the 29- and 18-kDa complexes or the recombinant 18-kDa protein acts on actin stress fibers and alters cell morphology. The addition of toxic substances to the culture medium induced toxicity against fibroblasts and PC12 cells (Figures 10A, D) and altered the morphology of PC12 and fibroblast cells to round forms (Figures 10B, E). The number of round PC12 cells increased by approximately three-fold in the presence of the recombinant 18-kDa protein and ten-fold in the presence of the 29- and 18-kDa complexes compared with the control cells (Figure 10C). The long diameter of fibroblast cells decreased to approximately one-seventh in the presence of the recombinant 18-kDa protein and one-eighth in the presence of the 29- and 18-kDa complexes compared with the control cells (Figure 10F).
Figure 10 Cell toxicity of the 29-kDa and 18-kDa protein complexes or recombinant 18 kDa protein against PC12 cells (A–C) and fibroblast cells (D–F). Cells were treated in the absence or presence of 0.05 mg/mL of 29-kDa and 18-kDa protein complexes (closed bar) or recombinant 18 kDa protein (open bar) for 24 hr. Control cells is indicated by hatched bar. (A) Toxicity against PC12 cells was estimated via MTT assay. Data were combined from six wells. (B) The photograph was taken in the absence and presence of 29-kDa and 18-kDa protein complexes or recombinant 18 kDa protein under phase-contrast microscopy. (C) The ratio of round cells per field was estimated in six fields. (D) Toxicity against fibroblast cells was estimated via MTT assay. Data were combined from six wells. (E) The photograph was taken in the absence and presence of 29-kDa and 18-kDa protein complexes or recombinant 18 kDa protein under phase-contrast microscopy. (F) The long diameter of fibroblast cells was measured for 100 cells. (B, E) Arrowheads show round cells. Bars show SD. Statistical significance was determined using a one-way ANOVA: *p < 0.05 relative to the control.
Consistent with the previous result (Hasegawa et al., 2018), feeding the mantle tissue caused an increase in the values of liver injury markers, AST and ALT, and kidney injury marker, BUN. Some toxicants can cause injury to the liver and kidneys, which play a critical role in the process of detoxification and excretion of toxicants from the organism. The present toxic substance may be also absorbed through the small intestine and then reach the liver and kidney tissues, causing cellular damage and cell death. In addition, we found that amylase content decreased distinctly. Nakajima et al. showed that low serum amylase is associated with increased risk of metabolic abnormalities and diabetes (Nakajima, 2016). Decrease of amylase may be due to the metabolic abnormality. On the other hand, Oh et al. showed that low serum levels of amylase have been infrequently observed in advanced stage of chronic pancreatitis. Feeding of mantle tissue may causes major injury in the pancreas (Oh et al., 2017). Further studies are necessary to better understand the processes by which toxic substances are absorbed and distributed.
Our previous studies have shown that scallop mantle tissue contains a proteinaceous toxin with a molecular weight above 10 kDa (Hasegawa et al., 2018). Clarifying the mechanism of toxicity to isolate and identify toxic substances is important. Thus, in this study, we isolated and identified 29-kDa and 18-kDa protein complexes from mantle tissue as novel toxic substances. The antibody against the separately isolated 18-kDa protein recognized two bands of 18 and 35 kDa in the mantle extract, suggesting that the 18-kDa protein may be a spliced form of the 35-kDa protein. However, we cannot determine whether the 35 kDa protein is a complete gelsolin-like protein and is toxic. During the preparation of the toxic substance, we found that two fractions of 0–30% and 45–60% ammonium sulfate showed toxicity. Although we used the 45–60% fraction in this study, a toxic substance different from the 29-kDa and 18-kDa complex may exist in the 0–30% fraction. Currently, the identification of toxic substances in the 0–30% fraction and 35-kDa protein is underway.
Although the function of the gelsolin-like protein is unclear, the 18-kDa protein is homologous to the G1 domain of mouse gelsolin (Burtnick et al., 2004; Spinardi and Witke, 2007). The G1 domain has a G-actin-binding region and actin-cleaving activity (Nag et al., 2013). The 18-kDa protein may also have actin-cleaving activity because the 18-kDa protein is isolated with an actin fragment. Many toxins inhibit or promote actin polymerization by interacting directly with actin. For example, latrunculin, mycalolide B, and aplyronine toxins bind to G-actin monomers in a 1:1 complex, rendering actin unable to polymerize and resulting in cell toxicity (Lenz et al., 2021; Gao and Nakamura, 2022). The addition of 29-kDa and 18-kDa protein complexes or recombinant 18 kDa protein to PC12 and fibroblast cells altered the cell morphology to a round form, suggesting that the 18-kDa protein may inhibit actin polymerization by interacting with actin.
Our results showed that the 18-kDa and 29-kDa complexes are essential for in vivo toxicity because the purified 18-kDa protein or a recombinant protein corresponding to the N-terminal region of the gelsolin-like protein alone did not show in vivo toxicity. Although the reasons remain unclear, the 18-kDa and 29-kDa complex may be stable for protease in the gastrointestinal tract and necessary for absorbance from the intestine. Native PAGE of the 29-kDa and 18-kDa protein complexes revealed faster migration than a mixture of 20-kDa molecular weight marker and carbonic anhydrase, with a molecular weight of 29 kDa. In native PAGE, proteins are separated based on the net charge, size, and shape of their native structures. The 18-kDa and 29-kDa protein complexes may have a compact structure and high net charges compared with the 29-kDa molecular weight marker and carbonic anhydrase mixture. Several protease-resistant proteins have been reported to have compact, globular structures (Jacobson and Rosenbusch, 1976; Tretyachenko et al., 2022). Further investigation is necessary to determine the protease-stability of these complexes.
Previously, we reported that treatment of the human hepatoma cell line HepG2 with mantle tissue extract suppressed the insulin-stimulated phosphorylation of Akt, a key protein in the insulin signaling pathway (Kariya et al., 2019). In addition, we showed that mantle tissue ingestion might inhibit insulin signaling by decreasing the glycogen content and increasing the mRNA expression of gluconeogenic enzymes in liver tissue (Kariya and Hasegawa, 2020). These results suggest that mantle tissue consumption causes glucose metabolism abnormalities by inhibiting insulin signaling in the liver. In this study, we performed detailed serum biochemical analyses. Consistent with previous results (Hasegawa et al., 2018), we confirmed that feeding mice mantle tissue increased the levels of liver and kidney injury markers. In addition, we found that the amylase content decreased substantially. Low serum amylase levels have been observed in advanced stages of chronic pancreatitis (Oh et al., 2017) and in patients with diabetes, particularly those with insulin-dependent diabetes (Nakajima, 2016). Mantle tissue consumption may also cause major injury to the pancreas in addition to the liver, resulting in glucose metabolism abnormalities.
Our results have shown that chronic administration of mantle tissue, gills, and ovaries of scallops to mice causes toxicity (Hasegawa et al., 2018). In addition to the adductor muscle, scallop mantle tissues and ovaries are consumed regularly in Japan. Therefore, determining whether scallop mantle tissue is toxic to humans is important. However, there are no reports of mantle tissue ingestion causing serious toxicity. Although it may be rare to consume the mantle and ovary tissues of scallops daily, the possibility that excessive consumption of these tissues causes toxicity in the liver and kidneys of humans cannot be ruled out. Investigating the relationship between the consumption of scallop mantle and ovarian tissue and disorders of glucose metabolism, such as diabetes, by conducting epidemiological studies is necessary.
In this study, we isolated and identified a novel toxic substance. This toxin was the first proteinaceous toxin to exhibit subacute toxicity after oral administration. Therefore, investigating the pharmacokinetics of this toxin seems interesting.
The original contributions presented in the study are included in the article/supplementary material. Further inquiries can be directed to the corresponding author.
Animal experiments were conducted per the Guidelines of Experimental Animal Care issued by the Office of the Prime Minister of Japan and the Muroran Institute of Technology. The experiments were approved by the Committee on the Ethics, Care, and Use of Animal Experiments of the Muroran Institute of Technology (Permit Number: H29-KS04).
Research design: YH; experiment and data analysis: NM, FY, KM, ST, and GX; manuscript preparation: YH. All authors contributed to the article and approved the submitted version.
The authors would like to thank FUJIFILM Wako (Osaka, Japan) for assistance with the LC-MS/MS analysis.
The authors declare that the research was conducted in the absence of any commercial or financial relationships that could be construed as a potential conflict of interest.
All claims expressed in this article are solely those of the authors and do not necessarily represent those of their affiliated organizations, or those of the publisher, the editors and the reviewers. Any product that may be evaluated in this article, or claim that may be made by its manufacturer, is not guaranteed or endorsed by the publisher.
Burtnick L. D., Urosev D., Irobi E., Narayan K., Robinson R. C. (2004). Structure of the n-terminal half of gelsolin bound to actin: roles in severing, apoptosis and FAF. EMBO J. 23, 2713–2722. doi: 10.1038/sj.emboj.7600280
Ferriss B. E., Marcinek D. J., Ayres D., Borchert J., Lefebvre K. A. (2017). Acute and chronic dietary exposure to domoic acid in recreational harvesters: a survey of shellfish consumption behavior. Environ. Int. 101, 70–79. doi: 10.1016/j.envint.2017.01.006
Gao J., Nakamura F. (2022). Actin-associated proteins and small molecules targeting the actin cytoskeleton. Int. J. Mol. Sci. 23, 2118. doi: 10.3390/ijms23042118
Garber E. A. (2008). Toxicity and detection of ricin and abrin in beverages. J. Food Prot. 71, 1875–1883. doi: 10.4315/0362-028X-71.9.1875
Gundry R. L., White M. Y., Murray C. I., Kane L. A., Fu Q., Stanley B. A., et al. (2009). Preparation of proteins and peptides for mass spectrometry analysis in a bottom-up proteomics workflow. Curr. Protoc. Mol. Biol. Chapter 10, Unit 25. doi: 10.1002/0471142727.mb1025s88
Hasegawa Y., Itagaki D., Konno K., Hasegawa C. (2018). Feeding of scallop mantle epithelial cell layer causes subacute toxicity against rodents. Fish Sci. 84, 91–100. doi: 10.1007/s12562-017-1156-3
Jacobson G. R., Rosenbusch J. P. (1976). ATP binding to a protease-resistant core of actin. Proc. Natl. Acad. Sc. U.S.A. 73, 2742–2746. doi: 10.1073/pnas.73.8.2742
Janik E., Ceremuga M., Saluk-Bijak J., Bijak M. (2019). Biological toxins as the potential tools for bioterrorism. Int. J. Mol. Sci. 20, 1181. doi: 10.3390/ijms20051181
Kariya T., Hasegawa Y. (2020). Scallop mantle toxin induces apoptosis in liver tissues of mice. Food Sci. Nutr. 8, 3308–3316. doi: 10.1002/fsn3.1608
Kariya T., Takahashi K., Itagaki D., Hasegawa Y. (2019). Scallop mantle extract inhibits insulin signaling in HepG2 cells. Food Sci. Nutr. 7, 2159–2166. doi: 10.1002/fsn3.1061
Kumar T., Ghosh A., Nandi J. (2021). Seafood and shellfish poisoning: a review. Int. J. Sci. Dev. Res. 6, 2455–2631.
Laemmli U. K. (1970). Cleavage of structural proteins during the assembly of the head of bacteriophage T4. Nature 227, 680–685. doi: 10.1038/227680a0
Lenz K. D., Klosterman K. E., Mukundan H., Kubicek-Sutherland J. Z. (2021). Macrolides: from toxins to therapeutics. Toxins 13, 347. doi: 10.3390/toxins13050347
Lopez-Rodas V., Maneiro E., Martinez J., Navarro M., Costas E. (2006). Harmful algal blooms, red tides and human health: diarrhetic shellfish poisoning and colorectal cancer. Anal. Real Acad. Nac. Farm. 72, 391–408.
Manerio E., Rodas V. L., Costas E., Hernandez J. M. (2008). Shellfish consumption: a major risk factor for colorectal cancer. Med. Hypotheses. 70, 409–412. doi: 10.1016/j.mehy.2007.03.041
Manthorpe M., Fagnani R., Skaper S. D., Varon S. (1986). An automated colorimetric microassay for neuronotrophic factors. Brain Res. 390, 191–198. doi: 10.1016/0165-3806(86)90208-7
Matsushima R., Uchida H., Nagai S., Watanabe R., Kamio M., Kaneiwa M., et al. (2015). Assimilation, accumulation, and metabolism of dinophysistoxins (DTXs) and pectenotoxins (PTXs) in the several tissues of Japanese scallop patinopecten yessoensis. Toxins 7, 5141–5154. doi: 10.3390/toxins7124870
Nag S., Larsson M., Robinson R. C., Burtnick L. D. (2013). Gelsolin: the tail of a molecular gymnast. Cytoskeleton 70, 360–384. doi: 10.1002/cm.21117
Nakajima K. (2016). Low serum amylase and obesity, diabetes and metabolic syndrome: a novel interpretation. World J. Diabetes 7, 112–121. doi: 10.4239/wjd.v7.i6.112
Oh H. C., Kwon C. I., El Hajj I. I., Easler J. J., Watkins J., Fogel E. L., et al. (2017). Low serum pancreatic amylase and lipase values are simple and useful predictors to diagnose chronic pancreatitis. Gut Liver 11, 878–883. doi: 10.5009/gnl17066
O’Neill K., Musgrave I. F., Humpage A. (2016). Low dose extended exposure to saxitoxin and its potential neurodevelopmental effects: a review. Environ. Toxicol. Pharmacol. 48, 7–16. doi: 10.1016/j.etap.2016.09.020
Polito L., Bortolotti M., Battelli M. G., Calafato G., Bolognesi A. (2019). Ricin: an ancient story for a timeless plant toxin. Toxins 11, 324. doi: 10.3390/toxins11060324
Pradhan B., Kim H., Abassi S., Ki J. S. (2022). Toxic effects and tumor promotion activity of marine phytoplankton toxins: a review. Toxins 14, 397. doi: 10.3390/toxins14060397
Pulido O. M. (2008). Domoic acid toxicologic pathology: a review. Mar. Drugs 6, 180–219. doi: 10.3390/md6020180
Sandvig K., Torgersen M. L., Engedal N., Skotland T., Iversen T. G. (2010). Protein toxins from plants and bacteria: probes for intracellular transport and tools in medicine. FEBS Lett. 584, 2626–2634. doi: 10.1016/j.febslet.2010.04.008
Spinardi L., Witke W. (2007). “Gelsolin and diseases”. In: Carafoli E., Brini M. editors. Calcium Signalling and Disease. Springer. 45, 55–69.
Tretyachenko V., Vymětal J., Neuwirthová T., Vondrášek J., Fujishima K., Hlouchová K. (2022). Modern and prebiotic amino acids support distinct structural profiles in proteins. Open Biol. 12, 220040. doi: 10.1098/rsob.220040
Truelove J., Mueller R., Pulido O., Iverson F. (1996). Subchronic toxicity study of domoic acid in the rat. Food Chem. Toxicol. 34, 525–529. doi: 10.1016/0278-6915(96)81814-X
Young N., Sharpe R. A., Barciela R., Nichols G., Davidson K., Berdalet E., et al. (2020). Marine harmful algal blooms and human health: a systematic scoping review. Harmful Algae 98, 101901. doi: 10.1016/j.hal.2020.101901
Keywords: actin, gelsolin-like protein, mantle tissue, novel toxin, scallop
Citation: Maeda N, Yoshida F, Matsumoto K, Takahashi S, Xiong G and Hasegawa Y (2023) Isolation and identification of a novel toxin in scallop mantle tissue. Front. Mar. Sci. 10:1202437. doi: 10.3389/fmars.2023.1202437
Received: 08 April 2023; Accepted: 26 April 2023;
Published: 08 May 2023.
Edited by:
Bin Wang, Zhejiang Ocean University, ChinaReviewed by:
Yoshimasa Sagane, Tokyo University of Agriculture, JapanCopyright © 2023 Maeda, Yoshida, Matsumoto, Takahashi, Xiong and Hasegawa. This is an open-access article distributed under the terms of the Creative Commons Attribution License (CC BY). The use, distribution or reproduction in other forums is permitted, provided the original author(s) and the copyright owner(s) are credited and that the original publication in this journal is cited, in accordance with accepted academic practice. No use, distribution or reproduction is permitted which does not comply with these terms.
*Correspondence: Yasushi Hasegawa, aGFzZWdhd2FAbW1tLm11cm9yYW4taXQuYWMuanA=
Disclaimer: All claims expressed in this article are solely those of the authors and do not necessarily represent those of their affiliated organizations, or those of the publisher, the editors and the reviewers. Any product that may be evaluated in this article or claim that may be made by its manufacturer is not guaranteed or endorsed by the publisher.
Research integrity at Frontiers
Learn more about the work of our research integrity team to safeguard the quality of each article we publish.