- 1Geospatial Ecology of Marine Megafauna Lab, Marine Mammal Institute, and Department of Fisheries, Wildlife, and Conservation Sciences, Oregon State University, Newport, OR, United States
- 2Department of Fisheries, Wildlife, and Conservation Sciences, Coastal Oregon Marine Experiment Station, Oregon State University, Newport, OR, United States
- 3Department of Integrative Biology, Oregon State University, Corvallis, OR, United States
- 4College of Engineering, School of Chemical, Biological, and Environmental Engineering, Oregon State University, Corvallis, OR, United States
- 5Department of Integrative Biology, Benthic Ecology Lab, Oregon State University, Newport, OR, United States
- 6Department of Fisheries, Wildlife, and Conservation Sciences, Oregon State University, Corvallis, OR, United States
The ocean continues to be a sink for microparticle (MP) pollution, which includes microplastics and other anthropogenic debris. While documentation of MP in marine systems is now common, we lack information on rates of MP ingestion by baleen whales and their prey. We collected and assessed MP loads in zooplankton prey and fecal samples of gray whales (Eschrichtius robustus) feeding in coastal Oregon, USA and produced the first estimates of baleen whale MP consumption rates from empirical data of zooplankton MP loads (i.e., not modeled). All zooplankton species examined were documented gray whale prey items (Atylus tridens, Holmesimysis sculpta, Neomysis rayii) and contained an average of 4 MP per gram of tissue, mostly of the microfiber morphotype. We extrapolated MP loads in zooplankton prey to estimate the daily MP consumption rates of pregnant and lactating gray whales, which ranged between 6.5 and 21 million MP/day. However, these estimates do not account for MP ingested from ambient water or benthic sediments, which may be high for gray whales given their benthic foraging strategy. We also assessed MP loads in fecal samples from gray whales feeding in the same spatio-temporal area and detected MP in all samples examined, which included microfibers and significantly larger morphotypes than in the zooplankton. We theorize that gray whales ingest MP via both indirect trophic transfer from their zooplankton prey and directly through indiscriminate consumption of ambient MPs when foraging benthically where they consume larger MP morphotypes that have sunk and accumulated on the seafloor. Hence, our estimated daily MP consumption rates for gray whales are likely conservative because they are only based on indirect MP ingestion via prey. Our results improve the understanding of MP loads in marine ecosystems and highlight the need to assess the health impacts of MP consumption on zooplankton and baleen whales, particularly due to the predominance of microfibers in samples, which may be more toxic and difficult to excrete than other MP types. Furthermore, the high estimated rates of MP consumption by gray whales highlights the need to assess health consequences to individuals and subsequent scaled-up effects on population vital rates.
1 Introduction
As intensive research on microplastics enters its second decade, the issue of plastic pollution has emerged alongside climate change as one of the most pressing environmental challenges. Plastic waste is predicted to increase exponentially out to 2050 and beyond (Borrelle et al., 2020), and scientists have recently called for a cap on virgin plastic production by 2040 (Bergmann et al., 2022). The ocean continues to be a sink for microplastic pollution, and is now documented to be a secondary source of microplastics to atmospheric and terrestrial ecosystems as well (Allen et al., 2020). Marine organisms are exposed to this complex suite of microplastic pollutants (Rochman et al., 2019) via water, sediment, and consumption of prey items, with a sizeable portion of these microscopic particles tracked back to stormwater, road wear, and laundering/waste treatment practices (Brahney et al., 2021; Brander et al., 2021; Miller et al., 2021; Ross et al., 2021; Granek et al., 2022). The impacts of microplastics on organisms are diverse and dependent partially on trophic level and life history, but the mechanisms of toxicity most consistently observed include (1) food dilution, resulting in reduced nutrient absorption and thus reduced growth, and (2) oxidative damage caused by small microplastics that can potentially translocate between organs of the organisms that ingest or inhale them (Jacob et al., 2020; Mehinto et al., 2022; Thornton Hampton et al., 2022). Microplastic presence has been demonstrated in many marine organisms, particularly those consumed as seafood (Li et al., 2019; Baechler et al., 2020a), but also in wild zooplankton that form the base of many marine trophic webs (Desforges et al., 2015; Botterell et al., 2022). Given that lower trophic level organisms are estimated to ingest higher levels of microplastics (Walkinshaw et al., 2020) and that microplastics-driven food dilution is occurring across organisms of all sizes (Siddiqui et al., 2022), investigations of microplastic loads in zooplankton and their potential impact on food webs are critical to more comprehensively understand ecological impacts.
The Pacific Northwest (PNW) seaboard of North America is an important habitat for a variety of ecologically important marine species, including gray whales (Eschrichtius robustus) that use the coastal region as a foraging ground from June through October each year (Calambokidis et al., 2019). Although much of the PNW coast is relatively pristine compared to more industrialized regions, microplastics have been confirmed in a number of different sample types collected in the region (Baechler et al., 2020b; Harris et al., 2021; Talbot et al., 2022; Lasdin et al., 2023). The majority of these microplastics are fibers, which aligns with other studies globally (Athey and Erdle, 2021; Granek et al., 2022). Given that fibers may be more toxic than other polymer shapes (Stienbarger et al., 2021; Mehinto et al., 2022), this abundance of fibers is of great concern, especially for zooplankton which form the base of marine food webs and are a primary dietary component for marine mammals including gray whales. [Hereafter we refer to microplastics as microparticles (MPs) given that not all microparticles are synthetic in composition, as recommended in (Miller et al., 2021; Lasdin et al., 2023)].
Zooplankton are important to multiple predator types, including invertebrates, fish, seabirds, and marine mammals that depend on their abundance to support their energetic demands. Therefore, trophic transfer of MP and the potential for bioaccumulation of associated contaminants from zooplankton through the food web is a significant concern (Nelms et al., 2018; Zantis et al., 2021). In the coastal region of Oregon, USA, zooplankton are key species in the nearshore ecosystem where they form the food base for commercially and recreationally harvested fish (Bosley et al., 2014) and gray whales during their ~6 month foraging season (Torres et al., 2018; Hildebrand et al., 2021). Fishing and whale watching in coastal Oregon play critical economic and food resource roles for coastal communities (O'Connor et al., 2009; Cramer et al., 2018). Thus, it is important to understand the rates and variability of MP loads in this coastal food web to establish baselines, identify species or areas of concern, and inform next steps of research and management to reduce harmful impacts of MP on wildlife populations and human communities.
Baleen whales are mega-filter feeders that engulf large amounts of ambient water while consuming their target prey. This feeding method exposes baleen whales to both direct ingestion of MP from ambient water, and indirect MP ingestion via consumption of contaminated prey through trophic transfer (Germanov et al., 2018; Zantis et al., 2021). Gray whales are a unique lineage of baleen whales, as they use suction feeding (rather than ram or lunge feeding; Goldbogen et al., 2017) to feed benthically on zooplankton and amphipods. Thus, gray whales frequently ingest benthic substrate (e.g., mud, sand, shell) while feeding in addition to ambient water and prey items, which may increase this taxa’s MP exposure. This study focuses on the Pacific Coast Feeding Group (PCFG) of gray whales, which is a small sub-group (abundance estimated to be 230 individuals) of the larger Eastern North Pacific (ENP) population of gray whales that migrate from breeding grounds in Baja California, Mexico to the Arctic where they feed (Rice and Wolman, 1971). The PCFG diverges from this migration pattern by foraging in coastal habitats from northern California, USA, to southern British Columbia, Canada (Calambokidis et al., 2002). PCFG whales are generalist feeders, foraging in nearshore areas <20 m (Hildebrand et al., 2021) with primary prey in our study region of coastal Oregon, USA being benthic and epibenthic mysids and amphipods (Hildebrand et al., 2021; Hildebrand et al., 2022).
Research on cetacean exposure to MPs is still in its relative infancy, especially on baleen whales. MPs have been found in the gastrointestinal tract of one humpback whale (Megaptera novaeangliae, Besseling et al., 2015) and in the blubber of fin whales (Balaenoptera physalus; Fossi et al., 2012; Fossi et al., 2014). Additionally, fin whale foraging grounds in the Mediterranean Sea were found to have high spatial overlap with areas containing elevated densities of microplastics (Fossi et al., 2016; Fossi et al., 2017). A recent study quantified MP ingestion rates of Bryde’s (Balaenoptera edeni brydei) and sei (Balaenoptera borealis) whales that feed on pelagic zooplankton in the Hauraki Gulf of New Zealand (Zantis et al., 2022). These initial studies demonstrate baleen whale exposure to MPs and the potential for negative consequences, including sub-lethal individual level effects that may impact energetic gains and health (Nelms et al., 2018). MPs can impact whales and other marine mammals by blockage of internal organs, mechanical damage of digestive tract, false feeling of satiation, and potentially leaching of toxicants depending on the length of the digestive period (Donohue et al., 2019; Hudak and Sette, 2019; Zhu et al., 2019; Novillo et al., 2020). These impacts could reduce an individual’s overall resilience to injury and disturbance, reproductive capacity, and possibly even survival. While it is critical to consider the population level effects of MP exposure to cetacean populations for a holistic and cumulative assessment of risks, we must first reliably estimate individual MP exposure rates and understand these impacts on vital rates.
Despite the likelihood of high MP ingestion by baleen whales, there remains a paucity of studies on rates of ingestion or impacts, likely due to the inherent challenges of ethically and effectively sampling such a large, free ranging, marine animal. However, fecal sample collection is effectively used to non-invasively assess hormone variation from baleen whales (Hunt et al., 2013; Lemos et al., 2020a) and recently to estimate MP ingestion rates (Zantis et al., 2022). Here, we quantify MP loads in Oregon coastal zooplankton, including from within primary PCFG gray whale foraging habitat and their known target prey species, and we extrapolate these findings to estimate the daily MP ingestion rates of gray whales based on energetic demands. As a case study of trophic transfer, we also conduct MP analysis of fecal samples collected from several PCFG gray whales feeding in the same spatio-temporal area. We hypothesize that MPs with similar characteristics will be detected in both sample types. To our knowledge, this study is the first to look at MP exposure of baleen whales from “zoop to poop” and to quantify baleen whale MP consumption rates from empirically counted MP loads in zooplankton prey (i.e., not modeled). Our results further develop the understanding of MP loads in marine ecosystems across trophic levels.
2 Materials and methods
2.1 Sample collection
2.1.1 Zooplankton
Zooplankton samples were collected using a light trap (modified from design in Chan et al., 2016; described in Hildebrand et al., 2021) in nearshore waters off Newport, Oregon, USA, between June and October from 2017 to 2019 as part of a larger study on gray whale ecology (Lemos et al., 2020b). The aim of zooplankton sample collection was to collect epibenthic PCFG gray whale prey items to gain a better understanding of the quality of prey available to foraging gray whales (Hildebrand et al., 2021). The light trap was deployed <1m above the seafloor at rocky reef sites where gray whales had previously been observed feeding on a given survey day and left to soak overnight before collection the following day. Zooplankton in the light trap were transferred to sterile plastic jars and frozen at -20°C for subsequent sorting and processing.
2.1.2 Gray whale feces
Opportunistic fecal samples of gray whales were collected when defecations were observed (Lemos et al., 2020a). Fecal material was captured using two 300 μm nylon mesh dipnets that were dragged through the fecal plume several times to capture as much material as possible. Collected fecal material was flushed out of the nets using ambient seawater in squeeze bottles into sterile plastic jars. Sample jars were put on ice until the field team returned to shore where jars were frozen at -20°C for later analysis. Photographs were taken of whales from which samples were collected in order to attribute samples to unique individuals. Location, date, and time of sampling were also recorded. Seawater samples were also collected from the same area to measure ambient MP levels.
2.2 Sample preparation
Under a laminar flow hood with HEPA filtration to 0.3 μm, defrosted zooplankton samples were sorted to species level under a Leica EZ4W stereoscope using LAS EZ imaging software (version 3.4.0). A minimum of 2 g wet weight per sample was required for the sample to have a critical mass for further analysis. Zooplankton samples were filtered through a set of two successive sieves to filter out the parts unable to be fully digested by the KOH (exoskeletons, larger pieces). The first/top sieve was a size of 1 mm and the second/bottom was 63 um. Samples digested in KOH were poured over the top sieve and rinsed with reverse osmosis (RO) water. All pieces caught by either of the sieves were collected into a petri dish to be searched for microparticles under the microscope. For each sample, a filtrate and a wash were collected: the filtrate being everything that went through the sieves, and after this was collected, the wash being what was collected from the sieves being rinsed with RO water. Both were collected in a glass pan below the sieves and transferred to their respective jars and labelled “filtrate” or “rinse from sieve” (wash). The filtrate and wash were vacuum filtered using a 5 μm polycarbonate filter (no polycarbonate was found in samples). Subsequently, samples were transferred and chemically digested for 64 hours at 50°C with 25 ml of 10% KOH added per gram of zooplankton to ensure complete digestion following protocols of (Enders et al., 2017; Pfeiffer and Fischer, 2020). All reagents were filtered to 5 μm prior to use to remove potential MP contamination. All glassware was muffle furnaced at 450°C and cleaned per protocols described in Brander et al., 2020 and Lasdin et al., 2023.
Fecal samples were filtered through unbleached coffee filters (confirmed to be cotton via FTIR) to drain saltwater from the samples (Lemos et al., 2020a). Filtered samples were then centrifuged for 10 min at 3,000 rpm (i.e., 1,000 RCF - relative centrifugal force [g]) to extract any remaining salt. Overlying water was removed using a pipette and samples were then lyophilized for 72 hr to remove all water content. Only fecal sample with dry mass weight ≥ 0.2 g were included in the subsequent MP analysis. Fecal samples were then chemically digested for 24-48 hours with 100 ml of 10% KOH in sterilized glass jars to ensure breakdown of organic material, per previously described methods in (Brander et al., 2020). The primary aim of MP analysis of fecal samples was to assess variation in MP type relative to zooplankton results, rather than estimation of MP loads due to limitations in sampling and contamination control. We acknowledge that some MPs may have been lost and/or added from fecal samples during collection with a larger mesh size net and potentially during filtration using cellulose coffee filters.
Prior to vacuum-filtration, 5 μm polycarbonate filters were inspected under a stereomicroscope for potential MP contamination. Digested samples were then vacuum-filtered through the inspected papers. Filtration units were rinsed with reverse osmosis (referred to henceforth as RO) filtered water to ensure the capture of all potential plastics. Liquid waste was poured back into the jars while used filter papers were stored in sterilized glass petri dishes for later MP processing.
2.3 Microparticle processing
Sample and control (see Section 2.4 Quality assurance/quality control for details) petri dishes were photographed prior to analysis under a microscope. Next, potential microplastics identified under the microscope were transferred to glass slides, photographed, and categorized by color and morphotype, and subsequently measured using LAS EZ imaging software (version 3.4.0), per methods described in Lasdin et al. (2023). To confirm chemical composition, a subset of MPs from the zooplankton (39%) and fecal (100%) samples, and seawater (100%) and lab control (43%) samples were analyzed using μFourier Transform Infrared (FTIR) spectroscopy and matched to the library housed within a commonly used open source software program - Open Specy (Cowger et al., 2021). MPs for FTIR analysis were selected to obtain a representative subsample of the different morphologies and colors found in each sample type (zooplankton, fecal, seawater, controls). All particles analyzed via FTIR were sorted into three categories: synthetic (plastic), anthropogenic (human generated but not clearly plastic, eg. cotton textile), and natural (e.g. plant matter, bone fragment). Methods followed for FTIR analysis are described in detail in: Harris et al., 2021; Caldwell et al., 2022; Talbot et al., 2022.
2.4 Quality assurance/quality control
All sample processing was conducted in a HEPA-filtered laminar flow hood.
Glass jars were filled with 100 ml of KOH and the lids removed during pre-vacuuming preparation to measure airborne contaminants during the sample processing stage under the laminar flow hood. These KOH blanks were filtered and picked following the same protocols used for zooplankton and whale fecal samples. During the picking process, air quality controls were made by stamping grids on Whatman filters, just as they were for all samples analyzed, mounting the paper in open glass petri dishes, and wetting the paper with RO water. These were placed in the workstation and the lids removed when samples were analyzed to measure possible background contaminants.
The number of background contaminants per gram of sample was calculated using the following equation:
2.5 Data analysis
We determined the number of MPs per gram for each zooplankton species and whale fecal samples by dividing the total number of MPs identified in each sample by the wet weight of the sample prior to digestion. We also calculated the number of MPs per individual zooplankton by multiplying the average wet weight (g) of an individual zooplankton for each species by the number of MPs per 1 g of that species.
Differences in MPs per gram and length of MPs of the three zooplankton species were assessed using analyses of variance (ANOVA; ‘stats’ R-package, version 4.0.2). Lengths of common MP morphotypes found in zooplankton and gray whale fecal samples were compared using Kruskal-Wallis tests (due to unequal variances and sample sizes between groups). We applied a Bonferroni correction to account for multiple comparisons. A total of three Kruskal-Wallis tests were performed, resulting in a new Bonferroni adjusted alpha value of 0.02 (0.05/3).
One of our study aims was to estimate the amount of MPs that gray whales ingest in one day. Since it is unknown what proportion of each gray whale defecation was collected at each sampling event or the digestion rate between the amount a whale consumes and the amount defecated per day, we were unable to use the MP per fecal gram results to extrapolate to daily MP intake by gray whales. Instead, we used the MPs per individual zooplankton results to extrapolate MP consumption rates per day based on published values of the number of individual zooplankton (by species) pregnant and lactating gray whales would need to consume per day to reach their daily energetic requirements (Villegas-Amtmann et al., 2017; Hildebrand et al., 2021). Our extrapolations were limited to pregnant and lactating females because these are the only gray whale demographic units with available information on daily energetic requirements (Villegas-Amtmann et al., 2017). While pregnant and lactating females were present in our study area and period overlapping with our prey sampling effort, we recognize that these groups may have higher energetic demands than other demographic units (considered further in Discussion). We multiplied these daily requirements by the number of MPs in an individual zooplankton by species to determine the estimated number of MPs that pregnant and lactating gray whales ingest daily. We also performed this calculation using an average value of the three zooplankton species (“composite prey”), as the proportions of gray whale diet are unknown. Pregnancy and lactation are life history stages with the highest energetic demands and are crucial periods that affect population dynamics (Lockyer, 1984; Villegas-Amtmann et al., 2015).
3 Results
3.1 Sample collection and preparation
Of the 36 light traps deployed, 31 successfully captured zooplankton. Since these light trap samples were also collected for the purpose of energetic value assessment (Hildebrand et al., 2021) and therefore needed to be divided equally, 16 light trap samples were analyzed for this study. Within each light trap sample, zooplankton were identified and sorted by species to obtain species-specific groups of 2 g wet weight for MP analysis. Three zooplankton species composed 99% of catch: the amphipod Atylus tridens, and the mysid shrimp Holmesimysis sculpta and Neomysis rayii, all of which are known PCFG gray whale prey items (Hildebrand et al., 2021). Due to the different catch amounts and wet weights of these three zooplankton species, the number of 2 g samples available for MP analysis varied by species; A total of 26 zooplankton samples were analyzed: (A. tridens = 4, H. sculpta = 9, N. rayii = 13; approximately 20, 20, and 10 individuals per sample respectively).
Since whale fecal samples were collected as part of a larger ecological study with the primary objective of quantifying hormones (Lemos et al., 2020a), only a small number of fecal samples of a critical minimum mass (0.2 g) for microparticle analysis were available for this study (n=5). These five samples were collected from four unique individual whales.
3.2 Microparticles in zooplankton
MPs were present in all 26 zooplankton samples analyzed. There were no statistically significant differences in the number of MPs per gram between the three zooplankton species (ANOVA: Sum of squares=7.98, F=0.382, p=0.687), with the mean number of MPs per gram being very similar across species, at approximately 4 MPs (3.92) per gram after accounting for background contamination (2.04 MPs per gram, Figure 1A).
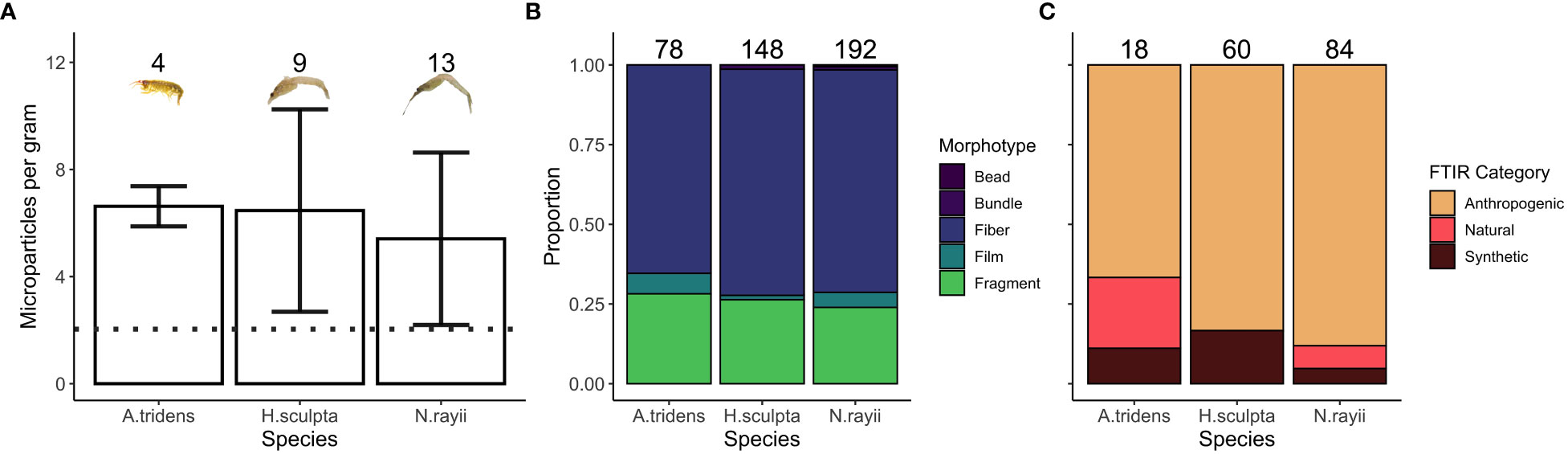
Figure 1 Microparticle (MP) loads and morphotypes by zooplankton species. (A) the number of MPs per 1 gram per species, with the dotted line representing the average MP level in controls. (B) the proportion of MP morphotypes found in each zooplankton species. (C) the proportion of Fourier transform infrared (FTIR) spectroscopy categories of MPs found in each zooplankton species. The sample size for each sample is denoted above all columns.
A total of 418 suspected MPs were identified from the 26 zooplankton samples. The morphotypes identified were similar across the three zooplankton species, with fibers accounting for over 50% of all MPs identified for each species (Figure 1B). The representative subset of MPs (n=162) analyzed via FTIR also revealed similar proportions by category across species, with anthropogenic MPs comprising more than half of the MPs identified for each species (Figure 1C). [Complete spectral matching and categorization data of zooplankton samples and controls can be found in Supplementary Material (Tables S1)]. No significant differences were found in the lengths of MPs between the three zooplankton species (ANOVA: Sum of squares=2.31, F=1.32, p=0.268). The size range of MPs found in the zooplankton (all species combined) was between 0.04 and 6.59 mm, with fibers showing a long tail of outliers toward larger items (Figure 2). Only one fiber that was 13 mm in length was excluded from analysis. Lengths of MPs detected in zooplankton were similar to MPs found in sea water control samples (Figure 2).
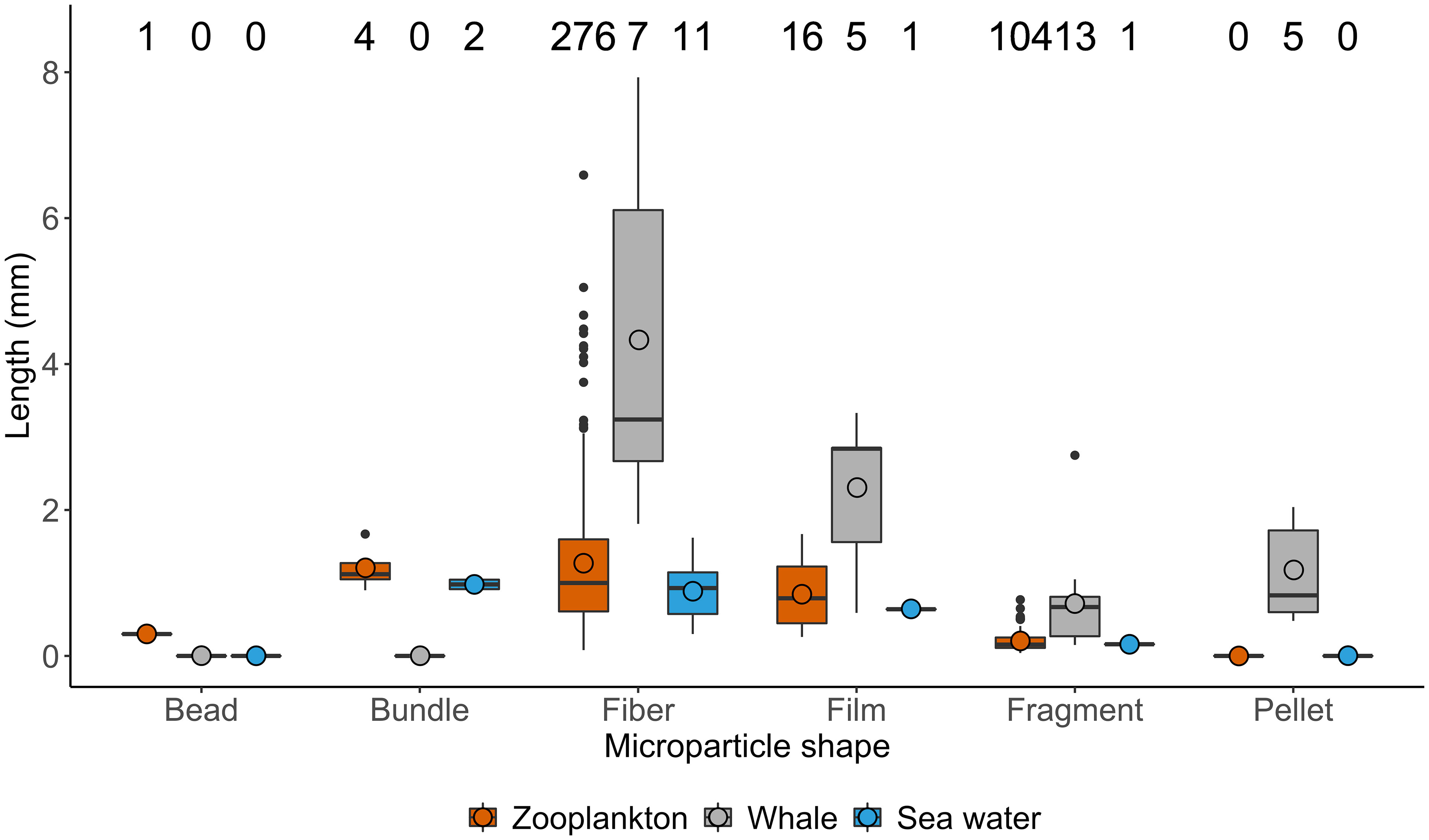
Figure 2 Size distribution of microparticles found in zooplankton samples (all species combined), the five gray whale fecal samples, and the sea water control samples. Note that 1 data point has been removed (outlier for fiber from zooplankton sample that was > 13 mm). The sample size for each sample is denoted above all columns. The box spans the 25 to 75% quartile range, the thick horizontal line indicates the median, whiskers extend to the 95% range, the circle indicates the mean, and points are outliers.
Despite the fact that N. rayii has a lower mean number of MPs per gram than the other two prey species (Figure 1A; though this difference is non-significant), this species had the highest number of MPs found per individual zooplankton (Table 1). In fact, the number of MPs per individual N. rayii (0.36) is between 3-4 times larger than the values for A. tridens (0.10) and H. sculpta (0.09). These differences are likely due to the fact that individual N. rayii are on average 10-14 mm longer than individuals of the other two species (Chapman, 2007; Burnham, 2015).
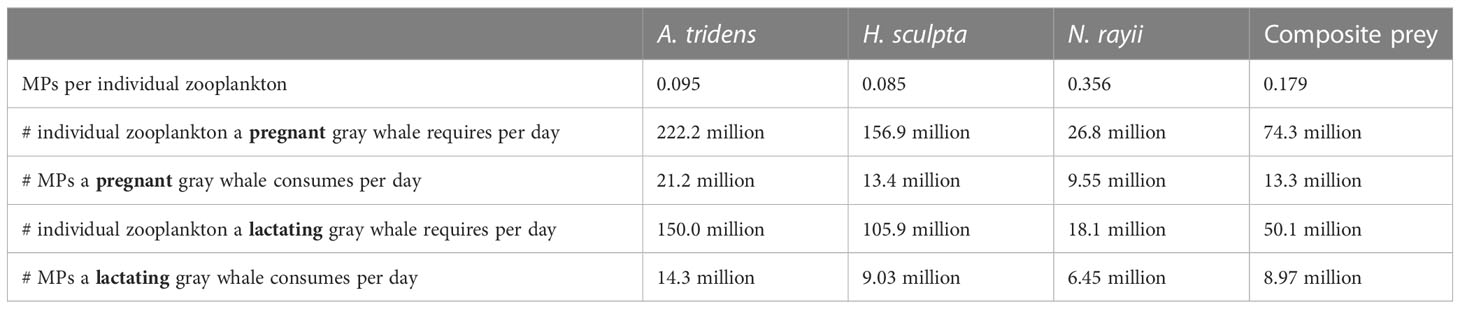
Table 1 Estimates of the number of microparticles (MPs) that a pregnant and lactating female gray whale consumes per day generated through extrapolation of results from this study (Microparticles per individual zooplankton; first row) to their daily energetic needs by zooplankton prey species from Hildebrand et al., 2021.
3.3 Microparticles in gray whale feces
MPs were present in all five fecal samples analyzed (Figure 3A). A total of 37 suspected MPs were identified in the five fecal samples. The morphotypes identified were similar across the five samples, with fragments found in all five and a mix of fibers, films, and pellets (Figure 3B). Given the small number of identified fecal MPs, all were analyzed via FTIR. Similar to the zooplankton samples, the majority of MPs were from an anthropogenic, followed by natural, and synthetic source (Figure 3C). [Complete spectral matching and categorization data of fecal samples and controls can be found in Supplementary Material (Table S2)]. Background contamination was appreciably higher in fecal samples (mean = 15.4; Figure 3A) than in the zooplankton samples. Results from the Kruskal-Wallis tests revealed that the three common morphotypes were significantly longer in gray whale fecal samples than in their zooplankton prey (fibers: χ2 = 15.6, df=1, p<0.001; films: χ2 = 5.35, df=1, p=0.02; fragments: χ2 = 18.3, df=1, p<0.001), and longer than MP detected in sea water control samples (Figure 2).
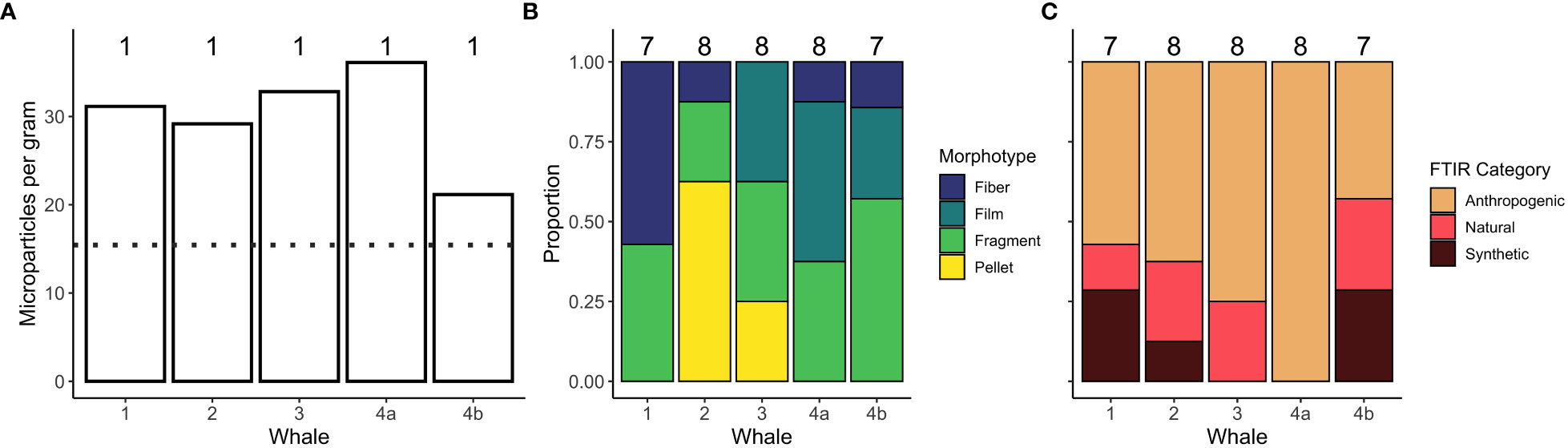
Figure 3 Microparticle (MP) loads and morphotypes found in each of the five gray whale fecal samples analyzed. (A) the number of MPs per gram of fecal sample, with the dotted line representing the average MP level in controls. (B) the proportion of MP morphotypes found in each fecal sample. (C) the proportion of Fourier transform infrared (FTIR) spectroscopy categories of MPs found in each fecal sample. The sample size for each sample is denoted above all columns.
3.4 Extrapolation to daily caloric needs of whales
We estimated the number of microparticles that gray whales consume per day through extrapolation of the number of MPs per individual zooplankton by species to daily gray whale energetic needs for pregnant and lactating females for each prey species (Table 1). Results indicate that if a pregnant gray whale ate only the energetically rich N. rayii in a day, it would consume 9.55 million MP per day. If a lactating whale ate a varied prey diet of “composite prey”, it would consume 8.97 million MP per day.
3.5 Microparticles in seawater and control samples
Five seawater samples were collected in proximity to each fecal sample (but outside of fecal plume). Six KOH controls and 10 air filter controls were collected during lab processing. In summary, of the 15 potential MPs identified in seawater samples, fibers were the dominant morphology (Figure S1). All 15 MPs were analyzed via FTIR that determined an almost even split between anthropogenic and synthetic sources (Figure S2). For the KOH controls, 38 potential MPs were identified, which were also dominated by fibers (Figure S1). FTIR results of the 14 MPs from KOH controls found mostly anthropogenic sources (Figure S2). From the 10 air filter controls, 16 potential MPs were identified, all of which were fibers (Figure S1). FTIR analysis of seven of these fiber MPs determined they were all from an anthropogenic source (Figure S2).
4 Discussion
Our estimated daily consumption of MPs by a pregnant or lactating PCFG gray whale is astounding, ranging between 6.45 and 21.2 million, especially when considering that a gray whale’s foraging season lasts ~6 months (180 days). Although this amount may appear to be an overestimate, it is aligned with the modeled estimates for other baleen whales that target zooplankton, such as Kahane-Rapport et al. (2022); a blue whale consumes 10 million MP per day) and Zantis et al. (2022) that estimated Bryde’s and sei whales consume 3.4 million MP per day (95% CI: 295,810 – 10,031,370). Blue, Bryde’s, and sei whales are all pelagic feeding whales rather than benthic feeding like gray whales. While MPs are commonly detected in surface water and throughout the water column, it is widely documented that the benthos is the biggest sink for marine MPs due to weathering and aggregation processes that increase particle density over time (Bergmann et al., 2017; Zhang, 2017; Shupe et al., 2021). Additionally, we produced estimates for the most energetically demanding life history phases of pregnant and lactating females. However, our estimates are derived from MP loads found in the main prey items of gray whales and do not account for MP ingested due to consumption of either ambient water or benthic sediments. Therefore, it is possible that the actual daily consumption rate of MP by gray whales during these sensitive life history stages is higher.
The differences in morphology and size of MPs found in the zooplankton and fecal samples are interesting. We theorize that these differences may be due to (1) the gape size limitation of zooplankton compared to whales, and (2) ambient consumption by benthically feeding gray whales of the heavier and larger MPs that are primarily found in the benthos (Zhang, 2017). Although the large mesh size (300 μm) of the net used to collect fecal samples may have under sampled smaller MPs, our protocols for zooplankton assessment (immediate digestion and filtration) would have collected larger MPs had they been present. Therefore, the finding of larger MPs in gray whale fecal samples than their zooplankton prey is likely accurate. Also, given that the amount of anthropogenic cellulosic material (e.g., cotton) was similar between whales and zooplankton, we do not think that the coffee filters (identified as cellulose via FTIR) added any contamination to the fecal samples.
The majority of MPs found in zooplankton were smaller fibers (<2 mm), which are likely more easily ingestible compared to larger items. While whale fecal samples also contained fibers, there was also a large amount of films, fragments, and pellets. The sea water blanks we collected at the surface were dominated by fibers with few films and fragments, indicating that the heavier and larger MPs may sink faster and accumulate in the benthos (ter Halle et al., 2016). This difference in sinking rates has also been widely documented in the literature (Horton and Dixon, 2018). Fibers also eventually sink to the bottom once weathered (Woodall et al., 2015). Therefore, we hypothesize that gray whales are exposed to MPs via trophic transfer from their zooplankton prey and indiscriminate consumption of ambient MPs in the benthos while foraging benthically (Figure 4). Yet our estimated daily MP consumption rates are only based on prey ingestion and therefore are likely conservative.
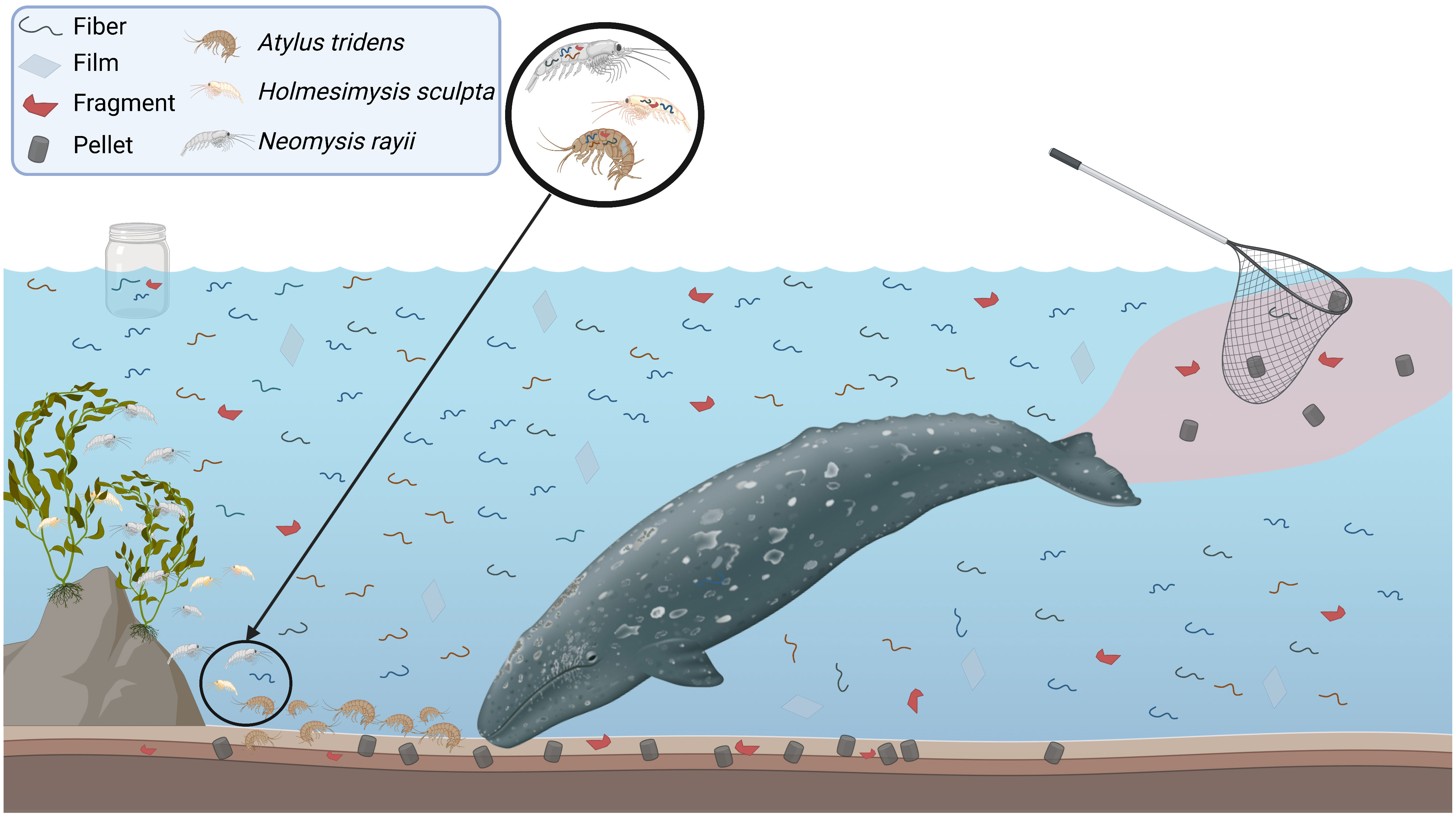
Figure 4 Theoretical microparticle (MP) exposure pathway schematic illustrating why gray whales have relatively more film, fragment and pellets in their fecal samples than were detected in their zooplankton prey that was dominated by fibers. Film, fragments, and pellets are heavier than fibers and sink to the bottom where gray whales feed benthically and thus likely consume these MPs within ambient benthic material. In contrast, fibers are found throughout the water column and benthos, including surface where we collect “sea water control samples” as indicated by the glass jar. The circle above the seafloor indicates where the light trap was placed in the water column and the primary zooplankton species caught. Adapted from “Virtual Background - Zebrafish”, by BioRender.com (2022). Retrieved from https://app.biorender.com/biorender-templates.
Gray whales feeding in this region use variable foraging tactics to feed on a variety of prey (Torres et al., 2018; Hildebrand et al., 2021), which may explain the variation in MP types within fecal samples and also implies that the “composite prey” estimate of daily MP consumption (Table 1) may be the most realistic to consider MP exposure across a whole foraging season. While the examination of MP morphotypes and size in fecal samples is useful, we do not attempt to calculate MP loads or consumption rates based on the fecal samples due to several reasons. We only collected a small and unknown portion of each fecal plume, so the estimation of MP consumption rate is fraught. Furthermore, whale fecal samples were collected using a 300 um net that may not have retained smaller MP types and sizes. Additionally, in contrast to the zooplankton samples which were processed under HEPA in a laminar flow hood, contamination of fecal samples may have occurred because we used plastic jars to store these samples and HEPA filters were not used during initial step of sample filtration through a coffee filter (HEPA was used for additional processing steps that followed). Thus, the five fecal samples analyzed in this study represent a pilot effort to better understand MP morphotype and sizes consumed, and as a proof of methods.
The predominance of microfibers in zooplankton species consumed by PCFG gray whales is concerning as evidence is accumulating across taxa that fibers may be more toxic and more difficult to excrete than other MP types (Stienbarger et al., 2021; Bucci and Rochman, 2022; Granek et al., 2022; Thornton Hampton et al., 2022). Although evidence of fiber impacts in megafauna such as whales is lacking, it could be predicated that fibers are similarly problematic. Further, the accumulation and internalization of microfibers by zooplankton likely impacts other important coastal species in addition to whales. The same zooplankton species are commonly fed upon by salmonids and other fishes commonly consumed by humans. Concerningly, a recent study focused on Oregon seafood species found that microfibers are common in the fillet tissue of salmonids, lingcod, herring (Brander pers. obs.), and in the fillet and gut of rockfish (Lasdin et al., 2023) frequently consumed by humans. Additionally, our estimates of MPs in zooplankton are likely also conservative, since current microscopy and FTIR approaches are limited to the detection of MPs > 20 microns in size (Cowger et al., 2020).
Although this study provides a first, baseline estimate of MP consumption by gray whales, there are many necessary next steps. Methodologically, whale fecal samples should be collected with a smaller mesh net and processed in a more controlled manner to minimize contamination. This added control would provide more robust estimates of MP types consumed, yet abundance estimates of MP consumption rates will remain challenging without estimates of the fecal sample proportion collected. More importantly though, is to extend these and other findings on the high rates of MP consumption by baleen whales to assess the population level impacts on the health of individuals and subsequent scaled-up effects on population dynamics (Senko et al., 2020). MP consumption may significantly impair the fitness of individuals through multiple pathways (e.g., reduced energetic gain, increased pollutant loads, organ damage), which may have long-term impacts across a population if reproductive capacity and calf survival is negatively impacted. Additionally, the impacts of MPs on zooplankton prey are also unknown; zooplankton with unsustainable levels of MP ingestion may not survive or reproduce as well as less contaminated individuals, and these impacts would have prey availability consequences to whales. Therefore, much more research is needed on the physiological impacts of MP ingestion on both zooplankton prey and whale predators. While our study demonstrates the potential for alarming rates of MP consumption by gray whales, it is also important to recognize that MP exposure is just one of many threats whales face in an increasingly anthropogenically impacted ocean. Hence, it is the cumulative impacts of these threats that must be holistically evaluated and managed to truly sustain and improve the health and viability of whale populations.
Data availability statement
The original contributions presented in the study are included in the article and Supplementary Material. Further inquiries can be directed to the corresponding author.
Ethics statement
The animal study was reviewed and approved by Oregon State University Animal Program Office.
Author contributions
LT, SB, and LH contributed to conception and design of the study. LT led field work and sample collection. SB oversaw laboratory analysis, which LH led with assistance from JP, EB, RN, JVB, and KL. LH conducted statistical analyses. LT wrote the first draft of the manuscript, with contributions from SB and LH. All authors contributed to manuscript revision, read, and approved the submitted version. Project funding was acquired and managed by LT.
Funding
The Coastal Oregon Zooplankton Investigation (COZI) was supported by the Agricultural Research Foundation at Oregon State University, with additional support from the OSU Marine Mammal Institute, Oregon Sea Grant, the Office of Naval Research Marine Mammals and Biology Program (#N00014-20-1-2760), and the NSF Growing Convergence Research Grant (#1935028).
Acknowledgments
We are grateful to our additional collaborators Kim Bernard, Sarah Henkel, Emily Pedersen, Haley Kent, and Noah Goodwin-Rice. Fecal samples collected under NOAA/NMFS permit #21678 issued to John Calambokidis.
Conflict of interest
The authors declare that the research was conducted in the absence of any commercial or financial relationships that could be construed as a potential conflict of interest.
Publisher’s note
All claims expressed in this article are solely those of the authors and do not necessarily represent those of their affiliated organizations, or those of the publisher, the editors and the reviewers. Any product that may be evaluated in this article, or claim that may be made by its manufacturer, is not guaranteed or endorsed by the publisher.
Supplementary material
The Supplementary Material for this article can be found online at: https://www.frontiersin.org/articles/10.3389/fmars.2023.1201078/full#supplementary-material
References
Allen S., Allen D., Moss K., Le Roux G., Phoenix V. R., Sonke J. E. (2020). Examination of the ocean as a source for atmospheric microplastics. PloS One 15, e0232746. doi: 10.1371/journal.pone.0232746
Athey S. N., Erdle L. M. (2021). Are we underestimating anthropogenic microfiber pollution? a critical review of occurrence, methods and reporting. Environ. Toxicol. Chem. 41(4), 822–837. doi: 10.1002/etc.5173
Baechler B. R., Granek E. F., Mazzone S. J., Nielsen-Pincus M., Brander S. M. (2020b). Microplastic exposure by razor clam recreational harvester-consumers along a sparsely populated coastline. Front. Mar. Sci. 7. doi: 10.3389/fmars.2020.588481
Baechler B. R., Stienbarger C. D., Horn D. A., Joseph J., Taylor A. R., Granek E. F., et al. (2020a). Microplastic occurrence and effects in commercially harvested north American finfish and shellfish: current knowledge and future directions. Limnology Oceanography Lett. 5, 113–136. doi: 10.1002/lol2.10122
Bergmann M., Almroth B. C., Brander S. M., Dey T., Green D. S., Gundogdu S., et al. (2022). A global plastic treaty must cap production. Science 376, 469–470. doi: 10.1126/science.abq0082
Bergmann M., Wirzberger V., Krumpen T., Lorenz C., Primpke S., Tekman M. B., et al. (2017). High quantities of microplastic in Arctic deep-Sea sediments from the HAUSGARTEN observatory. Environ. Sci. Technol. 51, 11000–11010. doi: 10.1021/acs.est.7b03331
Besseling E., Foekema E. M., Van Franeker J. A., Leopold M. F., Kühn S., Bravo Rebolledo E. L., et al. (2015). Microplastic in a macro filter feeder: humpback whale Megaptera novaeangliae. Mar. pollut. Bull. 95, 248–252. doi: 10.1016/j.marpolbul.2015.04.007
Borrelle S. B., Ringma J., Lavender Law K., Monnahan C. C., Lebreton L., McGivern A., et al. (2020). Predicted growth in plastic waste exceeds efforts to mitigate plastic pollution. Science 369, 1515–1518. doi: 10.1126/science.aba3656
Bosley K. L., Miller T. W., Brodeur R. D., Bosley K. M., Van Gaest A., Elz A. (2014). Feeding ecology of juvenile rockfishes off Oregon and Washington based on stomach content and stable isotope analysis. Mar. Biol. 161, 2381–2393. doi: 10.1007/s00227-014-2513-8
Botterell Z. L. R., Bergman M., Hildebrandt N., Krumpen T., Steinke M., Thompson R. C., et al. (2022). Microplastic ingestion in zooplankton from the fram strait in the Arctic. Sci. Total Environ. 831, 154886. doi: 10.1016/j.scitotenv.2022.154886
Brahney J., Mahowald N., Prank M., Cornwell G., Klimont Z., Matsui H., et al. (2021). Constraining the atmospheric limb of the plastic cycle. Proc. Natl. Acad. Sci. 118. doi: 10.1073/pnas.2020719118
Brander S. M., Hoh E., Unice K. M., Cook A., Holleman R. C., Kone D. V., et al. (2021). Microplastic pollution in California: a precautionary framework and scientifc guidance to assess and address risk to the marine environment (Sacramento, California, USA: California Ocean Science Trust).
Brander S. M., Renick V. C., Foley M. M., Steele C., Woo M., Lusher A., et al. (2020). Sampling and quality assurance and quality control: a guide for scientists investigating the occurrence of microplastics across matrices. Appl. Spectrosc 74, 1099–1125. doi: 10.1177/0003702820945713
Bucci K., Rochman C. M. (2022). Microplastics: a multidimensional contaminant requires a multidimensional framework for assessing risk. Microplastics Nanoplastics 2, 7. doi: 10.1186/s43591-022-00028-0
Burnham R. E. (2015). Reproductive strategies conferring species dominance in marine mysid (Peracarida, mysida) species in coastal waters off Vancouver island, BC. Crustaceana 88, 1421–1438. doi: 10.1163/15685403-0003502
Calambokidis J., Darling J. D., Deecke V., Gearin P., Gosho M., Megill W., et al. (2002). Abundance, range and movements of a feeding aggregationof gray whales (Eschrichtius robustus) from California to southeastern Alaska in 1998. J. Cetacean Res. Manage. 4, 267–276. doi: 10.47536/jcrm.v4i3.839
Calambokidis J., Pérez A., Laake J. (2019). Updated analysis of abundance and population structure of seasonal gray whales in the pacific Northwest 1996-2017 (Seattle, Washington: Final Report to NOAA), 1–72.
Caldwell A., Brander S., Wiedenmann J., Clucas G., Craig E. (2022). Incidence of microplastic fiber ingestion by common terns (Sterna hirundo) and roseate terns (S. dougallii) breeding in the northwestern Atlantic. Mar. pollut. Bull. 177, 113560. doi: 10.1016/j.marpolbul.2022.113560
Chan B. K. K., Shao K.-T., Shao Y.-T., Chang Y.-W. (2016). A simplified, economical, and robust light trap for capturing benthic and pelagic zooplankton. J. Exp. Mar. Biol. Ecol. 482, 25–32. doi: 10.1016/j.jembe.2016.04.003
Chapman J. C. (2007). Amphipoda, in: the light and smith manual – intertidal invertebrates from central California to Oregon. 4th Edition (Berkeley, CA, USA: UC Press).
Cowger W., Booth A. M., Hamilton B. M., Thaysen C., Primpke S., Munno K., et al. (2020). Reporting guidelines to increase the reproducibility and comparability of research on microplastics. Appl. Spectrosc. 74(9), 1066–1077. doi: 10.1177/0003702820930292
Cowger W., Steinmetz Z., Gray A., Munno K., Lynch J., Hapich H., et al. (2021). Microplastic spectral classification needs an open source community: open specy to the rescue! Analytical Chem. 93, 7543–7548. doi: 10.1021/acs.analchem.1c00123
Cramer L. A., Flathers C., Caracciolo D., Russell S. M., Conway F. (2018). Graying of the fleet: perceived impacts on coastal resilience and local policy. Mar. Policy 96, 27–35. doi: 10.1016/j.marpol.2018.07.012
Desforges J.-P. W., Galbraith M., Ross P. S. (2015). Ingestion of microplastics by zooplankton in the northeast pacific ocean. Arch. Environ. Contamination Toxicol. 69, 320–330. doi: 10.1007/s00244-015-0172-5
Donohue M. J., Masura J., Gelatt T., Ream R., Baker J. D., Faulhaber K., et al. (2019). Evaluating exposure of northern fur seals, callorhinus ursinus, to microplastic pollution through fecal analysis. Mar. pollut. Bull. 138, 213–221. doi: 10.1016/j.marpolbul.2018.11.036
Enders K., Lenz R., Beer S., Stedmon C. A. (2017). Extraction of microplastic from biota: recommended acidic digestion destroys common plastic polymers. ICES J. Mar. Sci. 74, 326–331. doi: 10.1093/icesjms/fsw173
Fossi M. C., Coppola D., Baini M., Giannetti M., Guerranti C., Marsili L., et al. (2014). Large Filter feeding marine organisms as indicators of microplastic in the pelagic environment: the case studies of the Mediterranean basking shark (Cetorhinus maximus) and fin whale (Balaenoptera physalus). Mar. Environ. Res. 100, 17–24. doi: 10.1016/j.marenvres.2014.02.002
Fossi M. C., Marsili L., Baini M., Giannetti M., Coppola D., Guerranti C., et al. (2016). Fin whales and microplastics: the Mediterranean Sea and the Sea of cortez scenarios. Environ. pollut. 209, 68–78. doi: 10.1016/j.envpol.2015.11.022
Fossi M. C., Panti C., Guerranti C., Coppola D., Giannetti M., Marsili L., et al. (2012). Are baleen whales exposed to the threat of microplastics? a case study of the Mediterranean fin whale (Balaenoptera physalus). Mar. pollut. Bull. 64, 2374–2379. doi: 10.1016/j.marpolbul.2012.08.013
Fossi M. C., Romeo T., Baini M., Panti C., Marsili L., Campani T., et al. (2017). Plastic debris occurrence, convergence areas and fin whales feeding ground in the Mediterranean marine protected area pelagos sanctuary: a modeling approach. Front. Mar. Sci. 4. doi: 10.3389/fmars.2017.00167
Germanov E. S., Marshall A. D., Bejder L., Fossi M. C., Loneragan N. R. (2018). Microplastics: no small problem for filter-feeding megafauna. Trends Ecol. Evol. 33, 227–232. doi: 10.1016/j.tree.2018.01.005
Goldbogen J. A., Cade D. E., Calambokidis J., Friedlaender A. S., Potvin J., Segre P. S., et al. (2017). How baleen whales feed: the biomechanics of engulfment and filtration. Annu. Rev. Mar. Sci. 9, 367–386. doi: 10.1146/annurev-marine-122414-033905
Granek E. F., Traylor S. D., Tissot A. G., Hurst P. T., Wood R. S., Brander S. M. (2022). Clothes encounters of the microfibre kind: the effects of natural and synthetic textiles on organisms, in: polluting textiles. Routledge, 63–99.
Harris L. S. T., La Beur L., Olsen A. Y., Smith A., Eggers L., Pedersen E., et al. (2021). Temporal variability of microparticles under the Seattle aquarium, WA: documenting the global covid-19 pandemic. Environ. Toxicol. Chem. 41(4), 917–930. doi: 10.1002/etc.5190
Hildebrand L., Bernard K. S., Torres L. G. (2021). Do gray whales count calories? comparing energetic values of gray whale prey across two different feeding grounds in the eastern north pacific. Front. Mar. Sci. 8. doi: 10.3389/fmars.2021.683634
Hildebrand L., Sullivan F. A., Orben R. A., Derville S., Torres L. G. (2022). Trade-offs in prey quantity and quality in gray whale foraging. Mar. Ecol. Prog. Ser. 695, 189–201. doi: 10.3354/meps14115
Horton A. A., Dixon S. J. (2018). Microplastics: an introduction to environmental transport processes. WIREs Water 5, e1268. doi: 10.1002/wat2.1268
Hudak C. A., Sette L. (2019). Opportunistic detection of anthropogenic micro debris in harbor seal (Phoca vitulina vitulina) and gray seal (Halichoerus grypus atlantica) fecal samples from haul-outs in southeastern Massachusetts, USA. Mar. pollut. Bull. 145, 390–395. doi: 10.1016/j.marpolbul.2019.06.020
Hunt K. E., Moore M. J., Rolland R. M., Kellar N. M., Hall A. J., Kershaw J., et al. (2013). Overcoming the challenges of studying conservation physiology in large whales: a review of available methods. Conserv. Physiol. 1, cot006. doi: 10.1093/conphys/cot006
Jacob H., Besson M., Swarzenski P. W., Lecchini D., Metian M. (2020). Effects of virgin micro-and nanoplastics on fish: trends, meta-analysis, and perspectives. Environ. Sci. Technol. 54, 4733–4745. doi: 10.1021/acs.est.9b05995
Kahane-Rapport S. R., Czapanskiy M. F., Fahlbusch J. A., Friednlaender A. S., Calambokidis J., Hazen E. L., et al. (2022). Field measurements reveal exposure risk to microplastic ingestion by filter-feeding megafauna. Nat. Commun. 13, 6327. doi: 10.1038/s41467-022-33334-5
Lasdin K. S., Arnold M., Agrawal A., Fennie H., Grorud-Colvert K., Sponaugle S., et al. (2023). Presence of microplastics and microparticles in Oregon black rockfish sampled near marine reserve areas. PeerJ 11, e14564. doi: 10.7717/peerj.14564
Lemos L. S., Burnett J. D., Chandler T. E., Sumich J. L., Torres L. G. (2020b). Intra- and inter-annual variation in gray whale body condition on a foraging ground. Ecosphere 11, e03094. doi: 10.1002/ecs2.3094
Lemos L. S., Olsen A., Smith A., Chandler T. E., Larson S., Hunt K., et al. (2020a). Assessment of fecal steroid and thyroid hormone metabolites in eastern north pacific gray whales. Conserv. Physiol. 8, coaa110. doi: 10.1093/conphys/coaa110
Li J., Lusher A. L., Rotchell J. M., Deudero S., Turra A., Bråte I. L. N., et al. (2019). Using mussel as a global bioindicator of coastal microplastic pollution. Environ. Pollut. 244, 522–533. doi: 10.1016/j.envpol.2018.10.032
Lockyer C. (1984). Review of baleen whale reproduction and implications for management. Rep. Int. Whaling Commission 6, 27–50.
Mehinto A. C., Coffin S., Koelmans A. A., Brander S. M., Wagner M., Thornton Hampton L. M., et al. (2022). Risk-based management framework for microplastics in aquatic ecosystems. Microplastics Nanoplastics 2, 17. doi: 10.1186/s43591-022-00033-3
Miller E., Sedlak M., Lin D., Box C., Holleman C., Rochman C. M., et al. (2021). Recommended best practices for collecting, analyzing, and reporting microplastics in environmental media: lessons learned from comprehensive monitoring of San Francisco bay. J. Hazardous Materials 409, 124770. doi: 10.1016/j.jhazmat.2020.124770
Nelms S. E., Galloway T. S., Godley B. J., Jarvis D. S., Lindeque P. K. (2018). Investigating microplastic trophic transfer in marine top predators. Environ. pollut. 238, 999–1007. doi: 10.1016/j.envpol.2018.02.016
Novillo O., Raga J. A., Tomás J. (2020). Evaluating the presence of microplastics in striped dolphins (Stenella coeruleoalba) stranded in the Western Mediterranean Sea. Mar. pollut. Bull. 160, 111557. doi: 10.1016/j.marpolbul.2020.111557
O'Connor S., Campbell R., Cortez H., Knowles T. (2009). Whale watching worldwide: tourism numbers, expenditures and economic benefits (Yarmouth, Massachusetts: International Fund for Animal Welfare).
Pfeiffer F., Fischer E. K. (2020). Various digestion protocols within microplastic sample processing–evaluating the resistance of different synthetic polymers and the efficiency of biogenic organic matter destruction. Front. Environ. Sci. 8. doi: 10.3389/fenvs.2020.572424
Rice D. W., Wolman A. A. (1971). The life history and ecology of the gray whale (Eschrichtius robustus) (Stillwater, Oklahoma: American Society of Mammalogists).
Rochman C. M., Brookson C., Bikker J., Djuric N., Earn A., Bucci K., et al. (2019). Rethinking microplastics as a diverse contaminant suite. Environ. Toxicol. Chem. 38, 703–711. doi: 10.1002/etc.4371
Ross P. S., Chastain S., Vassilenko E., Etemadifar A., Zimmermann S., Quesnel S.-A., et al. (2021). Pervasive distribution of polyester fibres in the Arctic ocean is driven by Atlantic inputs. Nat. Commun. 12, 106. doi: 10.1038/s41467-020-20347-1
Senko J. F., Nelms S. E., Reavis J. L., Witherington B., Godley B. J., Wallace B. P. (2020). Understanding individual and population-level effects of plastic pollution on marine megafauna. Endangered Species Res. 43, 234–252. doi: 10.3354/esr01064
Shupe H. J., Boenisch K. M., Harper B. J., Brander S. M., Harper S. L. (2021). Effect of nanoplastic type and surface chemistry on particle agglomeration over a salinity gradient. Environ. Toxicol. Chem. 40(7), 1820–1826. doi: 10.1002/etc.5030
Siddiqui S., Dickens J. M., Cunningham B. E., Hutton S. J., Pedersen E. I., Harper B., et al. (2022). Internalization, reduced growth, and behavioral effects following exposure to micro and nano tire particles in two estuarine indicator species. Chemosphere 296, 133934. doi: 10.1016/j.chemosphere.2022.133934
Stienbarger C. D., Joseph J., Athey S. N., Monteleone B., Andrady A. L., Watanabe W. O., et al. (2021). Direct ingestion, trophic transfer, and physiological effects of microplastics in the early life stages of centropristis striata, a commercially and recreationally valuable fishery species. Environ. Pollut. 285, 117653. doi: 10.1016/j.envpol.2021.117653
Talbot R., Granek E., Chang H., Wood R., Brander S. (2022). Spatial and temporal variations of microplastic concentrations in portland’s freshwater ecosystems. Sci. Total Environ. 833, 155143. doi: 10.1016/j.scitotenv.2022.155143
ter Halle A., Ladirat L., Gendre X., Goudouneche D., Pusineri C., Routaboul C., et al. (2016). Understanding the fragmentation pattern of marine plastic debris. Environ. Sci. Technol. 50, 5668–5675. doi: 10.1021/acs.est.6b00594
Thornton Hampton L. M., Bouwmeester H., Brander S. M., Coffin S., Cole M., Hermabessiere L., et al. (2022). Research recommendations to better understand the potential health impacts of microplastics to humans and aquatic ecosystems. Microplastics Nanoplastics 2, 18. doi: 10.1186/s43591-022-00038-y
Torres L. G., Nieukirk S. L., Lemos L., Chandler T. E. (2018). Drone up! quantifying whale behavior from a new perspective improves observational capacity. Front. Mar. Sci. 5. doi: 10.3389/fmars.2018.00319
Villegas-Amtmann S., Schwarz L. K., Gailey G., Sychenko O., Costa D. P. (2017). East Or west: the energetic cost of being a gray whale and the consequence of losing energy to disturbance. Endangered Species Res. 34, 167–183. doi: 10.3354/esr00843
Villegas-Amtmann S., Schwarz L. K., Sumich J. L., Costa D. P. (2015). A bioenergetics model to evaluate demographic consequences of disturbance in marine mammals applied to gray whales. Ecosphere 6, 1–19. doi: 10.1890/ES15-00146.1
Walkinshaw C., Lindeque P. K., Thompson R., Tolhurst T., Cole M. (2020). Microplastics and seafood: lower trophic organisms at highest risk of contamination. Ecotoxicology Environ. Saf. 190, 110066. doi: 10.1016/j.ecoenv.2019.110066
Woodall L. C., Gwinnett C., Packer M., Thompson R. C., Robinson L. F., Paterson G. L. J. (2015). Using a forensic science approach to minimize environmental contamination and to identify microfibres in marine sediments. Mar. Pollut. Bull. 95, 40–46. doi: 10.1016/j.marpolbul.2015.04.044
Zantis L. J., Bosker T., Lawler F., Nelms S. E., O'Rorke R., Constantine R., et al. (2022). Assessing microplastic exposure of large marine filter-feeders. Sci. Total Environ. 818, 151815. doi: 10.1016/j.scitotenv.2021.151815
Zantis L. J., Carroll E. L., Nelms S. E., Bosker T. (2021). Marine mammals and microplastics: a systematic review and call for standardisation. Environ. pollut. 269, 116142. doi: 10.1016/j.envpol.2020.116142
Zhang H. (2017). Transport of microplastics in coastal seas. Estuarine Coast. Shelf Sci. 199, 74–86. doi: 10.1016/j.ecss.2017.09.032
Keywords: microfiber, microparticles (MPs), microplastic (MP), gray whale, zooplankton, trophic transfer, benthic foraging, consumption rates
Citation: Torres LG, Brander SM, Parker JI, Bloom EM, Norman R, Van Brocklin JE, Lasdin KS and Hildebrand L (2023) Zoop to poop: assessment of microparticle loads in gray whale zooplankton prey and fecal matter reveal high daily consumption rates. Front. Mar. Sci. 10:1201078. doi: 10.3389/fmars.2023.1201078
Received: 06 April 2023; Accepted: 30 May 2023;
Published: 26 June 2023.
Edited by:
Lyne Morissette, M – Expertise Marine, CanadaReviewed by:
Penelope Kate Lindeque, Plymouth Marine Laboratory, United KingdomGiuseppe Suaria, National Research Council, Italy
Copyright © 2023 Torres, Brander, Parker, Bloom, Norman, Van Brocklin, Lasdin and Hildebrand. This is an open-access article distributed under the terms of the Creative Commons Attribution License (CC BY). The use, distribution or reproduction in other forums is permitted, provided the original author(s) and the copyright owner(s) are credited and that the original publication in this journal is cited, in accordance with accepted academic practice. No use, distribution or reproduction is permitted which does not comply with these terms.
*Correspondence: Leigh G. Torres, bGVpZ2gudG9ycmVzQG9yZWdvbnN0YXRlLmVkdQ==
†Present addresses: Elissa M. Bloom, Allogene Therapeutics, South San Francisco, CA, United States
Robyn Norman, Department of Biological Sciences, Cal Poly Humboldt, Arcata, CA, United States
Katherine S. Lasdin, School of Aquatic and Fishery Science, University of Washington, Seattle, WA, United States
‡ORCID: Leigh G. Torres, orcid.org/0000-0002-2643-3950
Susanne M. Brander, orcid.org/0000-0002-2305-5659
Elissa M. Bloom, orcid.org/0000-0001-8976-2578
Robyn Norman, orcid/org/0000-0003-3127-5915
Lisa Hildebrand, orcid.org/0000-0001-5688-1116
‡These authors have contributed equally to this work and share first authorship