- 1Hazardous Substances and New Organisms Group, Environmental Protection Authority, Wellington, New Zealand
- 2Marine Invasive Species Program, California State Lands Commission, Long Beach, CA, United States
- 3Chesapeake Biological Laboratory, University of Maryland Center for Environmental Science, Solomons, MD, United States
Biofouling (including initial microbial biofilms) of submerged ship surfaces can directly impact vessel operations, leading to increases in fuel usage, greenhouse gas emissions, and the likelihood of non-indigenous species (NIS) transfer and impacts. Considerations of attainable and consistent biosecurity goals are paramount to the success of the widespread adoption of biofouling management policy. Proactive in-water cleaning (IWC) of biofilms from submerged ship surfaces may provide a viable option from a biosecurity and ship operations standpoint, however these benefits need to be balanced against other environmental costs, including the potential for increased biosecurity risks associated with the elevated release of diverse microbes from ship surfaces.
1 Introduction
Microorganisms are ubiquitous in the marine environment. The rapid microbial colonization of submerged surfaces followed by the development of biofilms is a well-known process that remains particularly problematic for the maritime shipping industry (Cassé and Swain, 2006; Cacabelos et al., 2020; Swain et al., 2022; Tuck et al., 2022). The formation of biofilms, also referred to as microfouling or “slime layer,” on ship hulls has been associated with increased fuel usage, due to higher frictional drag (Schultz et al., 2011; Farkas et al., 2018), and greenhouse gas emissions (International Maritime Organization [IMO], 2011). The presence of biofilms is also associated with accelerated marine corrosion and reduced heat transfer efficiency (Jones and Little, 1990; Little et al., 2008; Dobretsov, 2010; Tuck et al., 2022). Further, biofilm presence and composition are considered key drivers in the settlement and establishment of macrofouling (i.e., multicellular individuals and colonies visible by eye, such as barnacles, tubeworms, and macroalgae; Tamburri et al., 2008; International Maritime Organization [IMO], 2011; Salta et al., 2013; Cacabelos et al., 2020).
In the natural marine environment, biofilms are dynamic and complex three-dimensional structures that contain heterogenous microbial populations (Dobretsov, 2010; Salta et al., 2013; Tuck et al., 2022). While biofilms are predominately made up of bacteria and diatoms, they may also include viruses, fungi, autotrophic and heterotrophic flagellates, ciliates, and protozoa (Cassé and Swain, 2006; Salta et al., 2013; De Carvalho, 2018; Cacabelos et al., 2020). The community within the biofilm is protected from the environment by the secretion of extracellular polymeric substances (EPS) which often contain polysaccharides, proteins, nucleic acids, and organic and inorganic substances (Dobretsov, 2010; Tuck et al., 2022). The EPS layer is an open system that allows for communication and co-operation (or antagonism) between community subpopulations, the exchange of nutrients and waste with surrounding waters, and the attraction or dispersal of individuals (Dobretsov, 2010; Tuck et al., 2022). Importantly, biofilms often also contain newly settled, microscopic stages of macrofouling organisms (i.e., invertebrate larvae and algal spores; Dobretsov, 2010).
Because of the ubiquity of microorganisms within the marine environment, biofilms are likely to be present under, between, and on macro-organism communities (i.e., macrofouling) settled on ship surfaces (e.g., epibiosis; Wahl et al., 2012). Microorganisms, including potentially harmful marine pathogens, are also present within the macrofouling (Georgiades et al., 2021; Evans et al., 2022). Depending on various factors, including host-pathogen dynamics and pathogen life-cycle, these microorganisms may present either in a non-replicative state in a carrier species or actively replicating within a susceptible host causing harm (Georgiades et al., 2021).
2 Knowns, unknowns, and biosecurity considerations
Similar to macrofouling communities, the composition of biofilms on ships is widely varied and is driven by numerous factors that influence recruitment, establishment, and spread (Inglis et al., 2010; Zargiel et al., 2011; Hunsucker et al., 2014; Sweat et al., 2017). For example, on statically immersed panels coated with eight commercial antifouling paints, Zargiel et al. (2011) observed more than 120 diatom species from more than 40 genera among three sites along the east coast of Florida.
Biofilm community composition is heavily influenced by geographic location, local biotic and abiotic conditions (e.g., ultra-violet (UV) light, temperature, desiccation, hydrodynamics, competition and predation, water chemistry, and nutrient availability), seasonal variations (Molino et al., 2009; Dobretsov, 2010; Zargiel et al., 2011; Salta et al., 2013; Sweat et al., 2017; Qian et al., 2022), port environments, and local anthropogenic discharges (Shikuma and Hadfield, 2010; Melchers, 2014; Cacabelos et al., 2020). Shikuma and Hadfield, (2010) observed the human pathogens Escherichia coli and Vibrio cholerae within biofilms sourced from ships hulls sampled in Hawaii. These findings are of little surprise given the vast volumes of wastewater entering the coastal marine environment from sewers, septic tank systems, agricultural runoff, and other direct inputs (Tuholske et al., 2021; Webber et al., 2021).
The submerged surfaces of ships provide a wide range of potential habitats that influence the type and presence of microfouling present (Salta et al., 2013; Hunsucker et al., 2014; Sweat et al., 2017; Georgiades and Kluza, 2020; Tuck et al., 2022). For example, diatom assemblages observed within the sheltered niche areas (e.g., bilge keels, sea chests, rudders) of cruise ships included genera with known weak adhesion strengths (Hunsucker et al., 2014). Similarly, surfaces containing copper or zinc, or those coated in biocidal antifouling paints, are likely to support microorganisms resistant to such active ingredients (Jones and Little, 1990; Little et al., 2008; Zargiel et al., 2011; Hunsucker et al., 2014; Sweat et al., 2017). These resistant microbes may facilitate the growth of less resistant microfouling and macrofouling species (Jones and Little, 1990; Tuck et al., 2022). Coating type also influences biofilm retention on surfaces during transport and is the leading factor for macrofouling recruitment and community composition (Sweat et al., 2017). Dobretsov et al. (2013) demonstrated that color can also influence the formation of both micro- and macrofouling communities. Further research is required regarding the influence of material composition on biofilm formation (Tuck et al., 2022).
As the composition of biofilms varies with substrate, location, space, and time (Qian et al., 2022), the exchange and release rates of microbes from biofilms on ship surfaces, either stationary, underway, or when surfaces are disturbed (e.g., contact with dock fenders and lines, tug and pilot boats, and bunkering barges), remains unknown. However, similar to macrofouling (Davidson et al., 2020), significant declines in the number of cells (organisms) and the diversity of biofilm composition on exposed ship surfaces decreases under dynamic conditions (i.e., the shear stress created when ships are underway) (Cassé and Swain, 2006; Zargiel and Swain, 2014; Sweat et al., 2017). Diatoms from the genera Amphora and Navicula, so called tenacious diatoms, are well known for their resistance to dynamic conditions, including on fouling release coatings (Hunsucker et al., 2014; Sweat et al., 2017). Added to those that are found in sheltered niche areas (i.e., more benign hydrodynamic conditions), the fouling of ships’ surfaces is a viable transport pathway for species within a biofilm community (Hunsucker et al., 2014; Sweat et al., 2017). The heterogeneity of biofilm organisms among ships, ports, and over time remains a key information gap (Sweat et al., 2017).
3 Policy implications
The complete prevention of biofilm formation on ship submerged surfaces is not possible (Dobretsov, 2010; Tuck et al., 2022). Microbes are subsequently released from these surfaces by natural processes (e.g., dispersal) or during normal ship operations (e.g., high speed transit and interactions with tugs, barges, and fenders) (Dobretsov, 2010; Morrisey et al., 2013). Indeed, some residual biofouling (i.e., both micro- and macro- fouling) is unavoidable even when best practices are applied (Georgiades and Kluza, 2017).
Ship in-water cleaning (IWC) has been promoted as a management measure to prevent or remove biofouling (Naval Sea Systems Command [NAVSEA], 2006; International Maritime Organization [IMO], 2011; Department of the Environment [DOE] and New Zealand Ministry for Primary Industries [MPI], 2015; Swain et al., 2022). Ship IWC is typically separated into two categories, namely proactive IWC or reactive IWC (Scianni and Georgiades, 2019; Tamburri et al., 2021). Proactive IWC represents the periodic removal or reduction of biofilms on ship surfaces. The accumulation of macrofouling is also prevented by proactive IWC as both the prerequisite biofilms for macrofouling colonization are less available and newly settled or attached microscopic stages of macrofouling organisms can be removed (Tribou and Swain, 2010; Scianni and Georgiades, 2019; Swain et al., 2022). Established macrofouling organisms are removed by reactive IWC (Scianni and Georgiades, 2019; Tamburri et al., 2020), as are the biofilms and associated microbes present on, under, around, and within the macrofouling community (Figure 1).
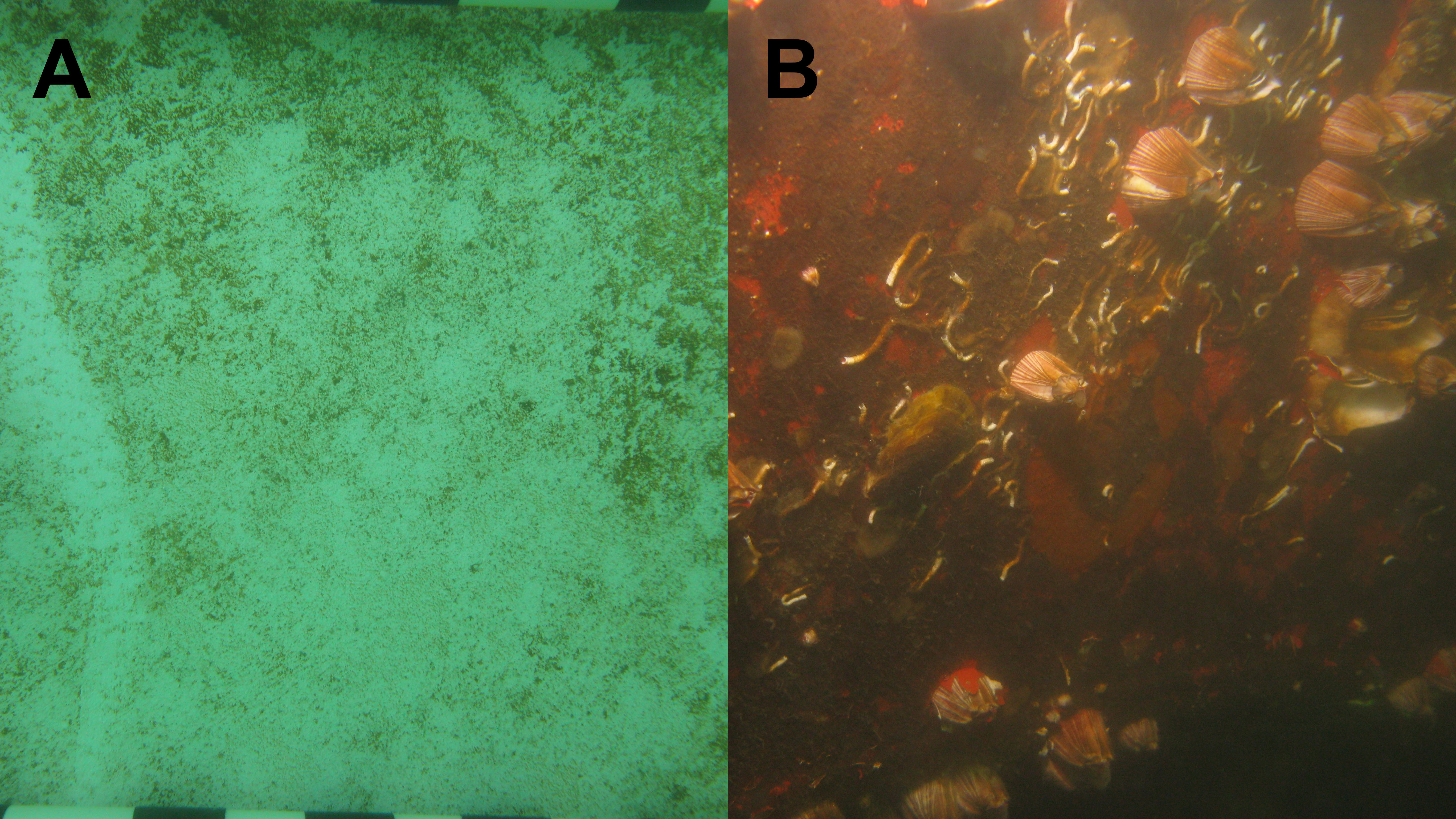
Figure 1 Fouling of vessel immersed surfaces (A) biofilm only; (B) biofilms in the presence of macrofouling (Photo credit: Ian Davidson).
Proactive IWC has become increasingly topical for having the potential as a rare win-win for both supporting ship operational needs and environmental protection goals (Tamburri et al., 2021). While proactive IWC has been the subject of a lot of small-scale experimental field work (Tribou and Swain, 2010; Tribou and Swain, 2017; Ralston et al., 2022; Swain et al., 2022), to the authors’ knowledge there has yet to be comprehensive, science-based, independent testing of specific systems under real-world conditions (Tamburri et al., 2021; ACT/MERC, 2022a; ACT/MERC, 2022b). While proactive IWC approaches may reduce or eliminate many of the concerns compared to reactive IWC (Department of the Environment [DOE] and New Zealand Ministry for Primary Industries [MPI], 2015; Scianni and Georgiades, 2019; Georgiades et al., 2021), it is critical to ensure these practices do not result in unintended consequences such as the enhanced release of ship antifouling coating related biocides and microplastics (Tamburri et al., 2021; ACT/MERC, 2022a; Tamburri et al., 2022). This is particularly so if proactive IWC is to be used frequently and at a global scale.
An apparent disconnect has become evident between views on the release of biofilm constituents from proactive and reactive IWC. For reactive IWC, biosecurity regulators have focused on retention of macrofouling, including fragments and propagules, with no real focus on biofilms and microorganisms inherently present on, in, around, and under macrofouling community. That is, biofilms are not considered as a great enough biosecurity risk to warrant mitigation. However, biosecurity concerns of biofilm constituents released from proactive IWC have recently been raised, including suggestions that capture and treatment of microbes should be required. One of the key benefits of proactive IWC is that the accumulation of macrofouling is minimised, which includes the preventive management of the potential replication and release of pathogenic microorganisms within host or carrier macrofouling species. The management of pathogenic microorganisms from reactive IWC is particularly challenging (Georgiades et al., 2021).
The terms “capture” and “treatment” are often used to describe actions associated with IWC systems that collect, retain, transport, and treat and/or dispose of some fraction of the removed organisms. However, these terms are not used in a uniform manner across IWC technologies or even regulatory bodies (Tamburri et al., 2021). This lack of consistency to describe important components of the IWC process leads to ambiguous understanding of the capabilities of various IWC approaches to minimize biosecurity and other environmental risks.
Given their sizes, microorganisms, including those associated with macrofouling, would require capture and/or treatment beyond the 10 µm physical separation currently considered for the containment of macrofouling (e.g., BIMCO/ICS, 2021; Department of Agriculture, Water and the Environment [DAWE], 2021). These treatments may include exposure to heat, ultraviolet radiation, biocides, or further filtration via organoclays (Georgiades et al., 2021). However, the development and implementation of such treatment systems should be based on a sound body of evidence and careful consideration of practicability and feasibility, including environmental acceptability. However, there exist two important caveats:
1. Treatment of captured debris (including microbes) is only beneficial if the material removed from the ship surface is effectively contained and collected at the cleaning head. Otherwise, even very effective treatment will only address the microbes released in the effluent and not those lost to surrounding waters.
2. Similar to the treatment of large quantities of effluent in other sectors, such as sewage wastewater (e.g., Chahal et al., 2016; Momba et al., 2019; Tuholske et al., 2021) and ship ballast water (International Maritime Organization [IMO], 2004; Hess-Erga et al., 2019; Studivan et al., 2022), complete sterilization is not currently feasible. Therefore, assessments and establishment of environmentally acceptable levels of microbial release would be needed, which includes the ability to monitor in real-time.
While harmful microalgae and pathogens can occur within ship biofilms (Drake et al., 2005; Drake et al., 2007; Molino and Wetherbee, 2008; Shikuma and Hadfield, 2010; Revilla-Castellanos et al., 2015), the possible risks associated with the release of biological material from the application of proactive IWC, above inherent release during normal ship operations, remain largely unknown (Scianni and Georgiades, 2019; Georgiades et al., 2021). However, due to the types of organisms targeted for removal and the typically less abrasive techniques required, the relative environmental risk of proactive IWC compared to reactive IWC (with or without capture and treatment of debris) is intuitive. Further, risk needs to be put into context of other anthropogenic inputs of biological concern (Tuholske et al., 2021; Webber et al., 2021).
4 Actionable recommendations
In relative terms, the biosecurity risk, and fuel usage, greenhouse gas emissions, antifouling system maintenance costs posed by macrofouling organisms is believed to be much higher compared to biofilms. If a zero-risk approach were to be considered for IWC, then the risks associated with the release of microorganisms and biofilms would require multi-staged processes, such as capture, primary treatment (e.g., filtration), and secondary treatment (e.g., disinfection with biocides, UV), to the greatest extent possible (e.g., best available technologies). Further, this approach would have to be equally applied to both proactive and reactive IWC technologies. The lower relative biosecurity risk of biofilms could allow their proactive removal without debris capture and treatment. While this may be true from a biosecurity perspective, other environmental considerations of proactive IWC would still need to be accounted for (e.g., environmental loads of antifouling biocides and microplastics; Tamburri et al., 2022) under a balanced cost-benefit analysis. Furthermore, biofilm formation on ship submerged surfaces, and the release of biofilm constituents during normal ship operations (independent of IWC), also needs further research (see Caruso, 2020) to better contextualize the risks associated with IWC. Other gaps include defining and agreement upon terminology (e.g., capture, treatment) to enable international uptake and implementation of safe and effective ship biofouling management.
5 Conclusions
A zero-risk approach is not currently practical or feasible when considering the IWC of biofouling from ships. Proactive IWC of biofilms without capture or treatment offers relative reductions in biosecurity risk, fuel usage, greenhouse gas emissions, and antifouling system maintenance costs. However, additional data and holistic considerations of potential environmental impacts are needed to ensure overall benefit of ship biofouling IWC.
Author contributions
The authors contributed equally to the conception and authorship of the article. Therefore, authorship is in alphabetical order, by last name.
Funding
This effort was supported, in part, by the U.S. Department of Transportation Maritime Administration and Maryland Department of Transportation’s Port Administration, through the University of Maryland Center for Environmental Science.
Acknowledgments
The authors acknowledge the fruitful discussions with Dr. Ralitsa Mihaylova (Safinah Group Ltd) which greatly improved the clarity of this manuscript.
Conflict of interest
The authors declare that the research was conducted in the absence of any commercial or financial relationships that could be construed as a potential conflict of interest.
Publisher’s note
All claims expressed in this article are solely those of the authors and do not necessarily represent those of their affiliated organizations, or those of the publisher, the editors and the reviewers. Any product that may be evaluated in this article, or claim that may be made by its manufacturer, is not guaranteed or endorsed by the publisher.
References
ACT/MERC (2022a). Evaluation of the jotun Hull skating solution: a proactive ship biofouling in-water cleaning system (Solomons, MD: ACT/MERC), TS-786-22. CBL/UMCES 2023-014.
ACT/MERC (2022b). Guidelines for testing ship biofouling in-water cleaning systems Solomons, MD: ACT/MERC, TS-788-22, CBL/UMCES 2023-017.
BIMCO/ICS (2021) Industry standard on in-water cleaning with capture. V1.01. Available at: www.bimco.org/-/media/bimco/ships-ports-and-voyage-planning/environment-protection/biofouling/2021-industry-standard-in-water-cleaning-final.ashx?rev=7e4ea382b7864d59951bf53f6595e4b2 (Accessed January, 2023).
Cacabelos E., Ramalhosa P., Canning-Clode J., Troncoso J. S., Olabarria C., Delgado C., et al. (2020). The role of biofilms developed under different anthropogenic pressure on recruitment of macro-invertebrates. Int. J. Mol. Sci. 21 (6), 2030. doi: 10.3390/ijms21062030
Caruso G. (2020). Microbial colonization in marine environments: overview of current knowledge and emerging research topics. J. Mar. Sci. Eng. 8 (2), 78. doi: 10.3390/jmse8020078
Cassé F., Swain G. (2006). The development of microfouling on four commercial antifouling coatings under static and dynamic immersion. International Biodeterioration & Biodegradation 57 (3), 179–185. doi: 10.1016/j.ibiod.2006.02.008
Chahal C., Van Den Akker B., Young F., Franco C., Blackbeard J., Monis P. (2016). Pathogen and particle associations in wastewater: significance and implications for treatment and disinfection processes. Adv. Appl. Microbiol. 97, 63–119. doi: 10.1016/bs.aambs.2016.08.001
Davidson I. C., Smith G., Ashton G. V., Ruiz G. M., Scianni C. (2020). An experimental test of stationary lay-up periods and simulated transit on biofouling accumulation and transfer on ships. Biofouling 36 (4), 455–466. doi: 10.1080/08927014.2020.1769612
De Carvalho C. C. (2018). Marine biofilms: a successful microbial strategy with economic implications. Front. Mar. Sci. 5, 126. doi: 10.3389/fmars.2018.00126
Department of Agriculture, Water and the Environment [DAWE] (2021). Australian In-water cleaning standards: consultation draft (Canberra: ACT: Department of Agriculture, Water and the Environment).
Department of the Environment [DOE] and New Zealand Ministry for Primary Industries [MPI] (2015). Antifouling and in-water cleaning guidelines Vol. 31 (Canberra: Department of Agriculture).
Dobretsov S. (2010). “Marine biofilms,” in Biofouling, vol. 123–136 . Eds. Dürr S., Thomasson J. C. (Oxford: Wiley-Blackwell).
Dobretsov S., Abed R. M., Voolstra C. R. (2013). The effect of surface colour on the formation of marine micro and macrofouling communities. Biofouling 29 (6), 617–627. doi: 10.1080/08927014.2013.784279
Drake L. A., Doblin M. A., Dobbs F. C. (2007). Potential microbial bioinvasions via ships’ ballast water, sediment, and biofilm. Mar. pollut. Bull. 55, 333–341. doi: 10.1016/j.marpolbul.2006.11.007
Drake L. A., Meyer A. E., Forsberg R. L., Baier R. E., Doblin M. A., Heinemann S., et al. (2005). Potential invasion of micro-organisms and pathogens via ‘interior hull fouling’: biofilms inside ballast water tanks. Biol. Invasions 7, 969–982. doi: 10.1007/s10530-004-3001-8
Evans J. S., Paul V. J., Kellogg C. A. (2022). Biofilms as potential reservoirs of stony coral tissue loss disease. Front. Mar. Sci. 9, 1009407. doi: 10.3389/fmars.2022.1009407
Farkas A., Degiuli N., Martić I. (2018). Towards the prediction of the effect of biofilm on the ship resistance using CFD. Ocean Eng. 167, 169–186. doi: 10.1016/j.oceaneng.2018.08.055
Georgiades E., Kluza D. (2017). Evidence-based decision making to underpin the thresholds in new zealand’s CRMS: biofouling on vessels arriving to new Zealand. J. Mar. Sci. Technol. 51, 76–88. doi: 10.4031/MTSJ.51.2.5
Georgiades E., Kluza D. (2020). Technical advice: conduct of in-water biofouling surveys for domestic vessels. biosecurity new Zealand technical paper no: 2020/04 (Wellington: Ministry for Primary Industries).
Georgiades E., Scianni C., Davidson I., Tamburri M. N., First M. R., Ruiz G., et al. (2021). The role of vessel biofouling in the translocation of marine pathogens: management considerations and challenges. Front. Mar. Sci. 8, 435. doi: 10.3389/fmars.2021.660125
Hess-Erga O. K., Moreno-Andrés J., Enger Ø., Vadstein O. (2019). Microorganisms in ballast water: disinfection, community dynamics, and implications for management. Sci. Total Environ. 657, 704–716. doi: 10.1016/j.scitotenv.2018.12.004
Hunsucker K. Z., Koka A., Lund G., Swain G. (2014). Diatom community structure on in-service cruise ship hulls. Biofouling 30 (9), 1133–1140. doi: 10.1080/08927014.2014.974576
Inglis G. J., Floerl O., Ahyong S., Cox S., Unwin M., Ponder-Sutton A., et al. (2010). The biosecurity risks associated with biofouling on international vessels arriving in new Zealand: summary of the patterns and predictors of fouling. a report prepared for ministry of agriculture biosecurity new Zealand biosecurity new Zealand (Wellington: Ministry of Agriculture Biosecurity New Zealand).
International Maritime Organization [IMO] (2004). International convention for the control and management of ships’ ballast water and sediments (London: International Maritime Organization).
International Maritime Organization [IMO] (2011). Guidelines for the control and management of ships’ biofouling to minimize the transfer of invasive aquatic species (London: International Maritime Organization).
Jones J. M., Little B. (1990). USS Princeton (CG 59): microbiologically influenced corrosion (MIC) and macrofouling status of seawater piping systems (Silver Spring, MD: Naval Surface Warfare Center Silver Spring, MD).
Little B. J., Lee J. S., Ray R. I. (2008). The influence of marine biofilms on corrosion: a concise review. Electrochimica Acta 54 (1), 2–7. doi: 10.1016/j.electacta.2008.02.071
Melchers R. E. (2014). Long-term immersion corrosion of steels in seawaters with elevated nutrient concentration. Corrosion Sci. 81, 110–116. doi: 10.1016/j.corsci.2013.12.009
Molino P. J., Childs S., Eason Hubbard M. R., Carey J. M., Burgman M. A., Wetherbee R. (2009). Development of the primary bacterial microfouling layer on antifouling and fouling release coatings in temperate and tropical environments in Eastern Australia. Biofouling 25 (2), 149–162. doi: 10.1080/08927010802592917
Molino P. J., Wetherbee R. (2008). The biology of biofouling diatoms and their role in the development of microbial slimes. Biofouling 24, 365–379. doi: 10.1080/08927010802254583
Momba M., Ebdon J., Kamika I., Verbyla M. (2019). ). using indicators to assess microbial treatment and disinfection efficacy (East Lansing, MI, USA: Global Water Pathogen Project; Michigan State University Press).
Morrisey D., Gadd J., Page M., Lewis J., Bell A., Georgiades E. (2013). In-water cleaning of vessels: biosecurity and chemical contamination risks. new Zealand ministry for primary industries technical paper no. 2013/11 (Wellington: Ministry for Primary Industries).
Naval Sea Systems Command [NAVSEA] (2006). “Naval ships’ technical manual chapter 081,” in Waterborne underwater Hull cleaning of navy ships, revision 5 (Washington: DC: Naval Sea Systems Command).
Qian P. Y., Cheng A., Wang R., Zhang R. (2022). Marine biofilms: diversity, interactions and biofouling. Nat. Rev. Microbiol. 20, 1–14. doi: 10.1038/s41579-022-00744-7
Ralston E., Gardner H., Hunsucker K. Z., Swain G. (2022). The effect of grooming on five commercial antifouling coatings. Front. Mar. Sci. 9, 836555. doi: 10.3389/fmars.2022.836555
Revilla-Castellanos V. J., Guerrero A., Gomez-Gil B., Navarro-Barrón E., Lizárraga-Partida M. L. (2015). Pathogenic Vibrio parahaemolyticus isolated from biofouling on commercial vessels and harbor structures. Biofouling 31, 275–282. doi: 10.1080/08927014.2015.1038526
Salta M., Wharton J. A., Blache Y., Stokes K. R., Briand J. F. (2013). Marine biofilms on artificial surfaces: structure and dynamics. Environ. Microbiol. 15 (11), 2879–2893. doi: 10.1111/1462-2920.12186
Shikuma N. J., Hadfield M. G. (2010). Marine biofilms on submerged surfaces are a reservoir for Escherichia coli and Vibrio cholerae. Biofouling 26 (1), 39–46. doi: 10.1080/08927010903282814
Schultz M. P., Bendick J. A., Holm E. R., Hertel W. M. (2011). Economic impact of biofouling on a naval surface ship. Biofouling 27, 87–98. doi: 10.1080/08927014.2010.542809
Scianni C., Georgiades E. (2019). Vessel in-water cleaning or treatment: identification of environmental risks and science needs for evidence-based decision making. Front. Mar. Sci. 6, 467. doi: 10.3389/fmars.2019.00467
Studivan M. S., Baptist M., Molina V., Riley S., First M., Soderberg N., et al. (2022). Transmission of stony coral tissue loss disease (SCTLD) in simulated ballast water confirms the potential for ship-born spread. Sci. Rep. 12 (1), 19248. doi: 10.1038/s41598-022-21868-z
Swain G., Erdogan C., Foy L., Gardner H., Harper M., Hearin J., et al. (2022). Proactive in-water ship hull grooming as a method to reduce the environmental footprint of ships. Front. Mar. Sci. 8, 808549. doi: 10.3389/fmars.2021.808549
Sweat L. H., Swain G. W., Hunsucker K. Z., Johnson K. B. (2017). Transported biofilms and their influence on subsequent macrofouling colonization. Biofouling 33 (5), 433–449. doi: 10.1080/08927014.2017.1320782
Tamburri M. N., Davidson I. C., First M. R., Scianni C., Newcomer K., Inglis G. J., et al. (2020). In-water cleaning and capture to remove ship biofouling: An initial evaluation of efficacy and environmental safety. Front. Mar. Sci., 7, 437. doi: 10.3389/fmars.2020.00437
Tamburri M. N., Breitburg D. L., Bonniwell S. M., Luckenbach M. W. (2008). Settlement of Crassostrea ariakensis larvae: effects of substrate, biofilms, sediment and adult chemical cues. J. Shellfish Res. 27, 601–608. doi: 10.2983/0730-8000(2008)27[601:SOCALE]2.0.CO;2
Tamburri M. N., Georgiades E. T., Scianni C., First M. R., Ruiz G. M., Junemann C. E. (2021). Technical considerations for development of policy and approvals for in-water cleaning of ship biofouling. Front. Mar. Sci. 8, 804766. doi: 10.3389/fmars.2021.804766
Tamburri M. N., Soon Z. Y., Scianni C., Øpstad C. L., Oxtoby N. S., Doran S., et al. (2022). Understanding the potential release of microplastics from coatings used on commercial ships. Front. Mar. Sci. 9, 1074654. doi: 10.3389/fmars.2022.1074654
Tribou M., Swain G. (2010). The use of proactive in-water grooming to improve the performance of ship hull antifouling coatings. Biofouling 26, 47–56. doi: 10.1080/08927010903290973
Tribou M., Swain G. (2017). The effects of grooming on a copper ablative coating: a six year study. Biofouling 33, 494–504. doi: 10.1080/08927014.2017.1328596
Tuck B., Watkin E., Somers A., Machuca L. L. (2022). A critical review of marine biofilms on metallic materials. NPJ Materials Degradation 6 (1), 25. doi: 10.1038/s41529-022-00234-4
Tuholske C., Halpern B. S., Blasco G., Villasenor J. C., Frazier M., Caylor K. (2021). Mapping global inputs and impacts from of human sewage in coastal ecosystems. PloS One 16 (11), e0258898. doi: 10.1371/journal.pone.0258898
Wahl M., Goecke F., Labes A., Dobretsov S., Weinberger F. (2012). The second skin: ecological role of epibiotic biofilms on marine organisms. Front. Microbiol. 3, 292. doi: 10.3389/fmicb.2012.00292
Webber J. L., Tyler C. R., Carless D., Jackson B., Tingley D., Stewart-Sinclair P., et al. (2021). Impacts of land use on water quality and the viability of bivalve shellfish mariculture in the UK: a case study and review for SW England. Environ. Sci. Policy 126, 122–131. doi: 10.1016/j.envsci.2021.09.027
Zargiel K. A., Coogan J. S., Swain G. W. (2011). Diatom community structure on commercially available ship hull coatings. Biofouling 27 (9), 955–965. doi: 10.1080/08927014.2011.618268
Keywords: biofilms, proactive in-water cleaning, biosecurity, biofouling management, ships
Citation: Georgiades E, Scianni C and Tamburri MN (2023) Biofilms associated with ship submerged surfaces: implications for ship biofouling management and the environment. Front. Mar. Sci. 10:1197366. doi: 10.3389/fmars.2023.1197366
Received: 30 March 2023; Accepted: 25 May 2023;
Published: 07 June 2023.
Edited by:
Laurent Urios, Université de Pau et des Pays de l’Adour, FranceReviewed by:
Daniel Rittschof, Duke University, United StatesSriyutha Murthy, Bhabha Atomic Research Centre, India
Copyright © 2023 Georgiades, Scianni and Tamburri. This is an open-access article distributed under the terms of the Creative Commons Attribution License (CC BY). The use, distribution or reproduction in other forums is permitted, provided the original author(s) and the copyright owner(s) are credited and that the original publication in this journal is cited, in accordance with accepted academic practice. No use, distribution or reproduction is permitted which does not comply with these terms.
*Correspondence: Mario N. Tamburri, dGFtYnVycmlAdW1jZXMuZWR1