- 1Fisheries Ecology Division, Southwest Fisheries Science Center, National Marine Fisheries Service, Santa Cruz, CA, United States
- 2Fish Ecology Division, Northwest Fisheries Science Center, National Marine Fisheries Service, Newport, OR, United States
- 3College of Earth, Ocean, and Atmospheric Sciences, Oregon State University, Corvallis, OR, United States
- 4Department of Applied Math, University of California, Santa Cruz, CA, United States
Building resilience in coastal resources and related communities is improved by a holistic ecosystem research approach for integrating socioecological system components; a key challenge in this process is capturing dynamic interactions between components. We present an application of ecosystem oceanography to address goals of marine conservation and management, including climate readiness and supporting a blue economy. Treating the ecosystem as the sample unit is fundamental to our research program. Specifically, spatiotemporal structure of relationships among taxa themselves is the study subject, not the individual members. Our approach outlines four steps toward successful implementation: 1) Build a conceptual ecosystem-oceanography model informed by previous science and human dimensions research to test hypotheses and identify gaps in our understanding; 2) Design survey and adaptive monitoring efforts, including data sharing protocols, to capture the spatiotemporal processes of ecosystem structure; 3) Use diet data and spatiotemporal variability in trophic interactions to quantify processes influencing ecosystem function, including persistent hotspots of abundance, biodiversity, and trophic transfer; 4) Link empirically-determined processes to improve parameterization of biophysical models to enable evaluation of ecosystem structure and functionality retrospectively and prospectively. Accomplishing these objectives requires a transdisciplinary team and will enable evaluation of specific management goals, develop indicators for tracking progress towards meeting them, and carry out scenario evaluation under near-term and long-term scenarios that explore key uncertainties (e.g., future climate and policy directions). We apply this four-step approach to identify key drivers for recent ecosystem and fishery surprises in the California Current Ecosystem. We propose this approach offers a means for anticipating future ecosystem states and increasing preparedness and capacity to overcome fishery surprises, and in doing so supporting the development of management approaches that are robust to uncertainty.
A role for ecosystem oceanography
Reductionist approaches commonly used in fishery and biological oceanography often fall short in providing management advice leading to robust actions for dealing with novel or surprising ecosystem change. Despite decades of researching the dynamics and behavior of marine ecosystems, there is a lack of demonstrated skill in fishery and ecosystem-level forecasting. Such a short-fall is apparent in the presence of increasing challenges brought on by climate change, successful predator recovery efforts, and additional anthropogenic pressures (e.g., fisheries, shipping, offshore wind development) (Hidalgo et al., 2022). Namely, predicting contemporary dynamics is underprepared for overcoming increasing risk and challenges to mitigating and adapting to adverse and interactive effects of climate change and human resource demand on ecosystem services. A holistic ecosystem oceanography approach to research that focuses on identifying complex and varying processes and mechanisms can provide context for quantifying the embedded and interactive effects of ecosystem components. This improves the evaluation and implementation of dynamic ocean management approaches that are robust to ‘ecological surprises’ (here defined as ecosystem behavior and events that deviate from our expectation) and better situates researchers and managers to proactively adapt to or mitigate pending conditions (Filbee-Dexter et al., 2017; Moore and Schindler, 2022).
The discipline of ecosystem oceanography provides a framework for achieving system-level understanding and parameterization of models for developing well-founded risk assessments and management strategies for contemporary and novel ecosystem issues. Ecosystem oceanography was first referenced by Cury (2005) and an initial framework for the approach was presented in Cury et al. (2008) incorporating empirical and numerical analyses to quantify ecosystem dynamics including influences of spatiotemporal variability in bottom-up, top-down, and middle-out control of the system. Our goal is to provide context and tractable advice on developing an ecosystem oceanography program: a discipline for elucidating variability in trophic structure related to climate change and human-related impacts. In building a transdisciplinary research program, we have found it essential to integrate personnel with skills pertaining to physical and biological oceanography, ecosystem modeling, and fisheries biology and management as components of an ecosystem oceanography approach (e.g., Wells et al., 2020; Santora et al., 2021a; Tommasi et al., 2021). Doing so permits diagnosing previous ecological conditions and anticipating looming changes in the ecosystem to optimize solutions for maintaining healthy socio-ecological systems.
As a discipline, several ecosystem oceanography stylized approaches have been successfully implemented globally, regardless of whether they have followed similar steps that we outline. All of these approaches have readily applied the use of a conceptual model for describing how ecosystem components interact and how they are scaled relative to biophysical drivers. The importance of the Stommel Diagram approach, which examines scales of spatiotemporal variability of biological and physical oceanography, is now a ubiquitous feature of biological, fisheries and ecosystem oceanography (Stommel, 1963; Haury et al., 1978; Levin, 1992; Schwing, 2023). This cornerstone approach to conceptualizing oceanographic variability extends to development of research programs invested in understanding the causal and ultimate drivers of marine ecosystem dynamics worldwide by pairing empirical and numerical modeling research priorities (e.g., Wells et al., 2020). For example, the Global Ocean Ecosystem Dynamics program invested in developing indicators and models to benefit fishery and ecosystem management (Turner et al., 2013). Further, in the Southern Ocean, fishery and ecosystem management involving Antarctic krill (Euphausia superba) and dependent predators (finfish, penguins, pinnipeds), relies upon ecosystem monitoring data that is explicitly connected to dynamical ecosystem models that are used to ensure overfishing of krill does not impact sensitive predator populations (Watters et al., 2013). In the North Sea, the sprat (Sprattus sprattus L.) fishery applies a coupled ocean ecosystem and individual-based model to assess fish survival (Daewel et al., 2008) and similar models have been developed for Atlantic cod (Gadus morhua) (Daewel et al., 2015). In the Baltic Sea, well-parameterized biophysical models are considered assets in the development of ecosystem-level management tactics and strategies for sprat, Atlantic cod, and herring (Clupea harengus) to ensure fisheries are sustainable (Möllmann et al., 2014). Although there are many examples of studies that develop ecological and oceanographic indicators from observations, and use numerical models to predict species patterns and response of fishery practices, we argue that implementing a well-defined and transferable ecosystem oceanography approach is more relevant than ever due to increasing climate change and variability effects on marine ecosystems.
Our ecosystem oceanography approach applied to the California Current Ecosystem has been of increasing importance as variability in trophic structure (i.e., variability of food web linkages between zooplankton and secondary consumers) linked to variability of environmental conditions result in consequential ecosystem-level impacts (Bindoff et al., 2019). Successful conservation of seabirds and marine mammals has resulted in many once-depleted populations recovering, a situation prompting resource managers to consider strategies that better balance the trade-off between the forage needs of predators and the impacts of human fisheries and removals (DeMaster et al., 2001; Samhouri et al., 2017; Ainley et al., 2018). The concomitant effect of varying and less persistent environment and forage populations with increasing consumptive demands of recovering predators (e.g., seabirds and marine mammals) has been linked to observed shifts of trophodynamics (Wells et al., 2008a; Mills et al., 2013; McClatchie et al., 2015; Fleming et al., 2016). The cost of ecological surprises to coastal human communities has been great and future costs may be greater as unanticipated ecosystem states continue to develop (Lam et al., 2016). Costs directly realized have included closures to fisheries due to recruitment failures (e.g., Chinook salmon (Oncorhynchus tshawytscha); Lindley et al., 2009; McCabe et al., 2016), domoic acid events (Holland and Leonard, 2020; Trainer et al., 2020), and mitigating risk of whale entanglement in fixed-gear fisheries (Santora et al., 2020). There have been monetary losses connected to droughts, fires and increasing river temperatures which directly relate to salmon adult abundance and the fishery (Lindley et al., 2009), and losses due to whale entanglement mitigation and the Dungeness crab (Metacarcinus magister) fishery (Seary et al., 2022). As current climate trends continue, we can expect occurrences of ecological surprises to increase and the development of chronically-unfavorable conditions that could be irreversible (e.g., freshwater habitat degradation, compression of coastal habitat, reduced productivity at trophic hotspots; Solomon et al., 2009; Santora et al., 2017; Schroeder et al., 2022).
Two events in the California Current Ecosystem demonstrate complicated consequences of spatiotemporal variability in ecosystem function, resulting in surprises that impacted socio-economic systems, and demonstrate the need for an ecosystem-oceanographic approach. These examples include different time periods, yet similar underlying unexpected ecosystem shifts: 1) the failure of the 2005 California Fall-run Chinook salmon cohort that led to a years-long collapse of a salmon fishery (Lindley et al., 2009); and 2) increased whale entanglements in fixed-gear fisheries during the Large Marine Heat Wave of 2014-2016 (Santora et al., 2020). In both cases, there were warm winter conditions in the Northeast Pacific Ocean basin and increased coastal stratification, consequences of reduced late-winter upwelling. This preconditioned the California Current Ecosystem for poor primary productivity, reduced production of euphausiids (hereafter krill) at persistent hotspots, and a switch by predators from typically well dispersed juvenile forage fishes associated with productive conditions (e.g., rockfishes, spp. Sebastes) and krill to adult Northern anchovy (Engraulis mordax) which are restricted nearshore in a narrow band of upwelled water (i.e., habitat compression) (Wells et al., 2017; Santora et al., 2020). As a result, seabirds aggregated nearshore to feed on the available Northern anchovy, but in doing so, increased incidental predation on emigrating Chinook salmon which typically only make up a nominal amount of their diet (Wells et al., 2017). Similarly, humpback whales (Megaptera novaeangliae) that typically feed on krill in more offshore locations (outer continental slope), foraged nearshore on Northern anchovy where the incidence of their entanglement with fixed fishing gears increased dramatically. Currently, in 2023 the Chinook salmon fishery for much of the US West Coast is closed for the second time in 15 years, resulting in another significant economic loss for coastal fishing communities. We refer back to these examples throughout to highlight how an integrative ecosystem oceanographic approach can inform and mitigate such surprises.
We contend that diagnosing and adapting to future ecosystem change, through use of single-species, multi-species or aggregated approaches (i.e., constructing ecosystem structure and process from disaggregated studies) are insufficient at capturing the variability in ecosystem structure and functionality, especially in cases where spatiotemporal variability in the trophic interactions is a dominant characteristic. What makes the ecosystem oceanographic approach novel is consideration of the underlying structure of the full system and building a study design accommodating this system and its varying structure with an eye toward modeling the variability in the interactions and functionality of ecosystem components and, ultimately, the consequences to fish, fisheries and protected resources. We advocate for a holistic and adaptive research and survey program that could allow for building capacity and preparedness for overcoming novel contemporary ecosystem management issues and unanticipated logistical constraints (e.g., coronavirus, reduced funding, ship malfunctions; Santora et al., 2021b). We provide a conceptual overview of our streamlined process to facilitate an effective approach to ecosystem-based management that we refer to throughout this perspective piece (Figure 1). Linking ecosystem services to data inputs, empirical analyses, coupling empirical results to biophysical models is integral to an ecosystem oceanographic approach. This is especially critical as the frequency of negative, novel conditions is greater than the evolution of the science needed to diagnose related impacts, develop decision tools, and resolve contemporary and future solutions.
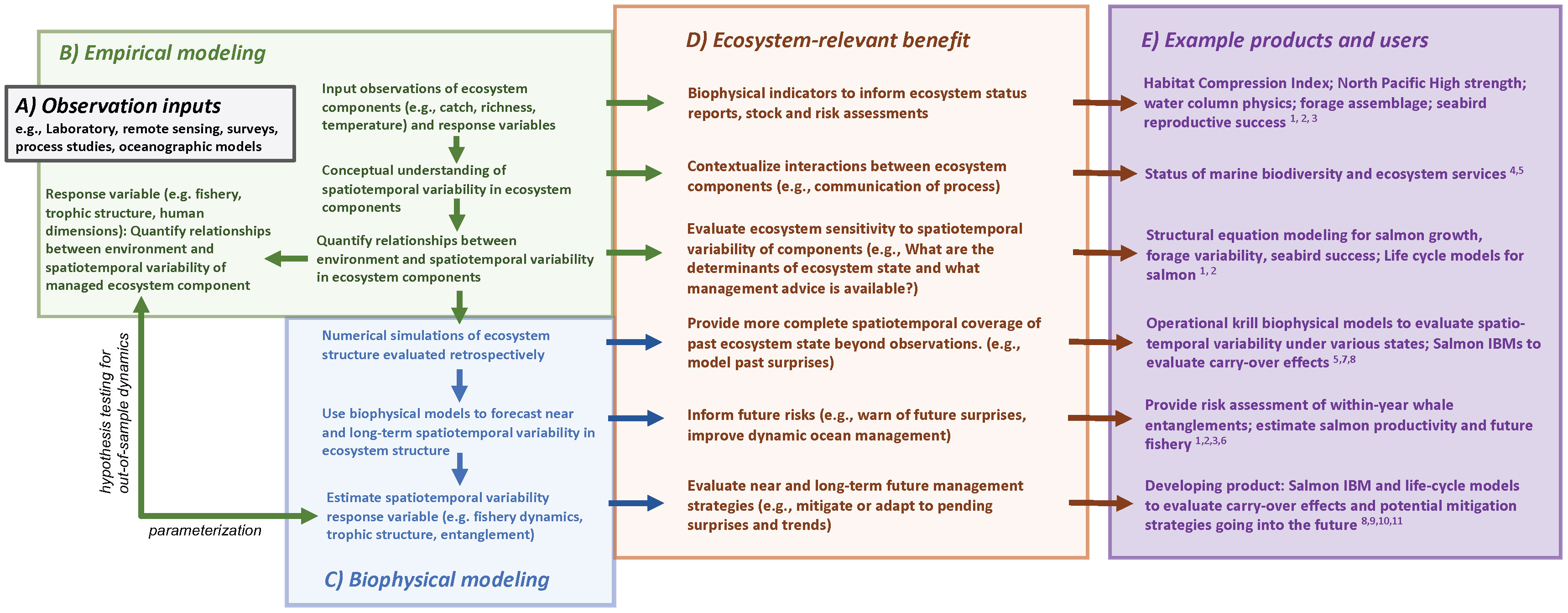
Figure 1 A flow diagram of ecosystem services linked to (A) data inputs, (B) empirical analyses, (C) coupling empirical results to biophysical models and the (D) ecosystem benefits that can result from each level of this ecosystem oceanographic approach. Empirical observations and statistical modeling provide measures of the states of individual ecosystem components, a conceptual understanding of the system, and the opportunity to develop functional relationships within the ecosystem. This provides indicators on which to assess risks to ecosystem components, the opportunity for semi-quantitative analyses from a conceptual model, and sensitivity analysis to evaluate the leverage ecosystem components have on accomplishing managerial goals or affecting additional ecosystem components. Empirical outputs and diagnosed processes can be coupled to biophysical models to evaluate spatiotemporal variability in ecosystem structure and functionality retrospectively and prospectively. Further, by quantifying the relationships between the environment and spatiotemporal variability of managed ecosystem components, we can estimate the spatiotemporal responses of these components to future climate trends and variability. These coupled, numerical approaches provide a more complete spatiotemporal coverage of ecosystem state that have been out-of-sample, inform near and long-term risks, and can be used to evaluate management strategies. We also provide example products and users (E): 1) Pacific Marine Fisheries Council (https://www.pcouncil.org/annual-california-current-ecosystem-status-report/), 2) California Current Integrated Ecosystem Assessment (https://www.integratedecosystemassessment.noaa.gov/regions/california-current/california-current-iea-indicators), 3) California Department of Fish and Wildlife, Ocean Protection Council (https://www.opc.ca.gov/risk-assessment-and-mitigation-program-ramp/), 4) National Marine Sanctuary Reports, Central California Ocean Observing System (CenCOOS), 5) Marine Biodiversity Observation Network: https://mbon.ioos.us/#default-data/5, 6) NOAA Fisheries (Wells et al 2020 and development of biophysical models and biological opinions), 7) Monterey Bay Aquarium Research Institute (https://www.mbari.org/data/krill-hotspots-in-the-california-current/), 8) Informing freshwater management actions (e.g., Gosselin et al., 2021, ), 9) Fiechter et al., 2015 (IBM is Individual Based Model), 10) Henderson et al., 2019, and 11) Friedman et al., 2019.
Although the California Current is particularly data rich, many similar observational and modeling data sets exist within large marine ecosystems worldwide and are increasingly accessible to researchers. We have access to several long-term data sets including physical and biological series, and fishery assessments, across trophic levels, from which to develop an integrative ecosystem observing and modeling approach. By treating the ecosystem as the sample unit and by evaluating these data series synthetically we achieve an ecosystem oceanography research effort. This underlying approach considers the spatiotemporal relationships between ecosystem components as the study subject. The implication of this assertion is that spatiotemporal variability in ecosystem function is best evaluated synthetically. Otherwise, constructing conceptual understandings and management options by reanimating previously disconnected research efforts would provide a false comfort in solving issues as idiosyncratic problems to be diagnosed and dealt with individually. Rather, we should build general approaches and solutions founded on researching underlying processes governing ecosystem structure, treating the ecosystem itself as the unit of study. If not, resulting cascades, synergistic outcomes and latent interactions may be unanticipated when the ecosystem is pushed in novel ways by environmental, internal, and human pressures. Therefore, a first step in an ecosystem-level approach is to define the ecosystem, including its community structure, the environmental drivers that impact trophic interactions, and the impacts to and resulting from humans (e.g., Figure 2).
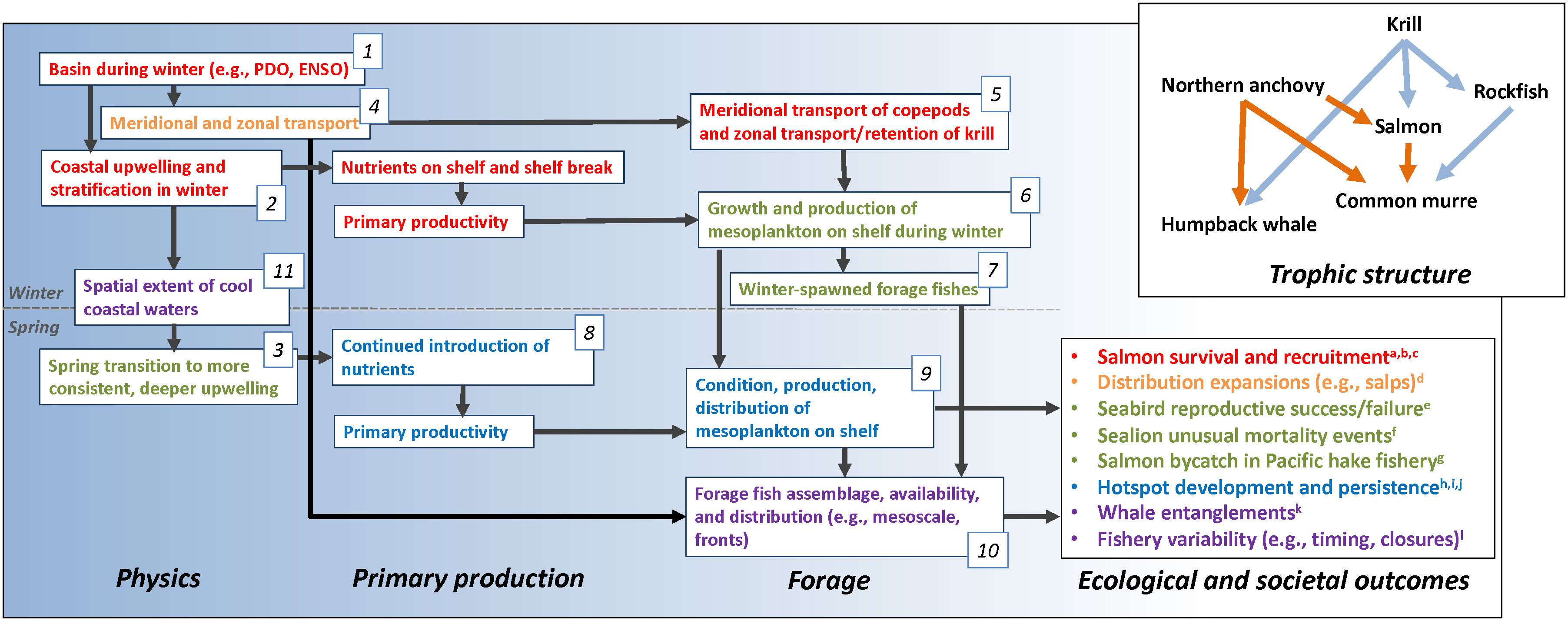
Figure 2 Our general conceptual model of the California Current Ecosystem as it currently stands relative to a number of recent and current ecological outcomes. Fonts are colored to match ecological outcomes and the various studies with which we refined the conceptual model: a growing suite of components and interactions were examined to elucidate the outcomes at hand. Numbers associated with components are referenced as specific studies in the text. Literature cited in “Ecological and societal outcomes”: a) Wells et al., 2012, b) Woodson et al., 2013b, c) Wells et al., 2017, d) Miller et al., 2019, e) Wells et al., 2008a, f) McClatchie et al., 2015, g) Wells et al., 2023, h) Santora et al., 2011, i) Santora et al., 2018, j) Santora et al., 2017, k) Santora et al., 2020 and l) Seary et al., 2022. From upper left to lower right the scale of processes declines from basin-scale to meso-scale. Also shown are two roughly organized trophic structures of central California sentinel species discussed here related to cool, productive conditions (blue arrows) and less productive, warmer conditions (orange arrows) during spring and summer.
An ecosystem oceanography effort is improved by integrating research goals and human dimensions directly. Sole consideration to natural resource variability marginalizes and impacts stakeholders and reduces effective participation in implementation of management decisions that aim for resilient fisheries and communities (Conroy and Peterson, 2013). An integrated socio-ecological conceptual model is a first step in developing a transdisciplinary program inclusive of human dimensions such that the results are mapped to identified needs, climate vulnerability assessments, and the diagnosis of resolutions (Harvey et al., 2016; Wells et al., 2020; Dudley et al., 2021). For example, Chinook salmon fisheries are linked to additional fisheries, such as the fixed-gear Dungeness crab fishery, through cross-participation which is an aspect that is likely of importance beyond a salmon-oriented ecosystem (Fisher et al., 2021). Developing and executing a research program in collaboration with the community through methods such as Structured Decisions Making reduces unforeseen conflicts that could hinder the application of an academically valuable but misdirected research effort (Conroy and Peterson, 2013).
As we consider a holistic program, we recognize Cury et al. (2008) provide tenets on which we base our guideposts. 1) Problems should be approached and addressed at the ecosystem level. 2) Ecosystem oceanography treats bottom-up (e.g., productivity) and top-down (e.g., fishery, predation) influences as acting simultaneously on components of the ecosystem, albeit, with different relative influence under different conditions. 3) Spatial and temporal processes act simultaneously to determine ecosystem state including, among other aspects, recruitment of forage fish, forage species assemblages, predator behaviors, trophic dynamics and fisheries. 4) While ecosystem processes are dependent on larger-scale conditions (e.g., pressure cells), the proximate determinants of ecosystem structure and function are the result of mesoscale processes (e.g., fronts, upwelling, eddies). 5) Explicit representation of predation allows for the estimation of the proximate outcomes of environmental variability and trends. 6) Quantitative analysis of long-term data series allows a greater scope of conditions and provides more confidence in the estimation of out-of-sample predictions. 7) Ecosystem models should only be as complex as needed to address the overarching ecosystem processes. In such, appropriate model complexity should be evaluated by comparing nested model results to observed condition. 8) Model evaluation is essential to develop trust in the hindcasts and forecasts of ecosystem structure and functionality. Here, we provide direction in the development of an ecosystem oceanography program inclusive of, and building on, Cury et al.’s principles.
To build and implement an ecosystem model, assessment or management strategy evaluation properly, requires an integrative observing and modeling process for parametrization and evaluation (e.g., from samples of fish in trawls and observations of predators and sea state to parameterizing an individual-based model for juvenile salmonids). While already existing ecosystem models may be capable of accommodating our approach, they typically do not follow the sequence of steps outlined in this conceptual review. We provide a path with four guideposts for accomplishing an ecosystem oceanography research effort and applying its results based on decades of research in the California Current Ecosystem (Figure 1). This perspective targets and may benefit existing research programs with intermediate (e.g., 10-20 years) or mature time series, yet with limited integration between them (Edwards et al., 2010). Development and application of the effort begins with recognizing the ecosystem as it relates to questions, observing and quantifying processes regulating the ecosystem, recognizing current and future risks, and providing guidance for management evaluation to avoid, adapt to, or mitigate risk. While we focus on an Eastern Boundary Upwelling Ecosystem, any marine system (e.g., coral reefs, mangrove, and high latitude sea-ice ecosystems) can be examined if the totality of the system is treated as a sample unit and the fundamental data series and conceptual models are considered. The limiting element to the general application of this approach is data availability. We hope to build a case for increased investment in data collection and, where possible, adding value to existing survey efforts by including additional platforms (e.g., remotely-sensed data) and observations (e.g., predators, eDNA). What is not specifically addressed here, but is implicit in the examples we provide throughout, is the need to build a transdisciplinary team including representation from physical and biological oceanography, ecosystem modeling, fisheries biology, and management. We are fortunate to have identified and maintained such a collective (represented in the Acknowledgements), which we consider to be an ecosystem oceanography program. We hope that our experiences and comments, organized as 4 interrelated steps, make the path to such a synthetic effort shorter for those interested in developing such a program.
Develop conceptual models of the marine ecosystem structure and processes
We see a first step in problem solving requiring the development of conceptual models of presumed ecosystem structure and processes relative to the hypothesis for the ecosystem dynamics at hand. The clarity of these models makes complicated objectives appear more manageable, solutions more probable, and increases the motivation to work on the issues collaboratively (Harvey et al., 2016). It is also useful for defining a strategy for identifying research gaps (Wells et al., 2020). Importantly, conceptual models allow the identification of process-oriented indicators for individual components and of ecosystem function at the scales at which processes are dependent and occur (Figures 1A, B). Basin-scale drivers (e.g., atmospheric pressure systems and related surface winds) influence regional-scale processes (e.g., upwelling and nutrient introduction) that structure mesoscale trophic interactions (e.g., forage availability near fronts) (Di Lorenzo et al., 2013). These connections and processes have been, in the California Current Ecosystem, approached as a series of more specific research efforts to fill the knowledge gaps between correlative relationships between basin-scale drivers (upper left of Figure 2) and direct proximate drivers of mortality such as predation at fronts (lower right of Figure 2) (Wells et al., 2006; Wells et al., 2016; Sabal et al., 2020). Further, the conceptual model can be built in a way to incorporate management drivers (e.g., climate change, Ecosystem Based Fisheries Management, restoration and recovery, forecasting) to provide a research map for developing efforts to inform management needs in the context of the whole system (e.g., Wells et al., 2020).
Importantly, the model we present is general and serves as a guide for framing hypotheses and facilitating initial diagnosis of ecological surprises and changes in ecosystem state (Figure 2). The realism and successful inclusion of conceptual models in a long-term ecosystem study program is dependent on combining process studies and lab experiments with observations and numerical modeling. Such a model acts as an evolving starting point in diagnosing and modeling drivers and consequences of novel conditions and has been demonstrably capable of providing context for a number of such surprises (Figure 2). Generally, Di Lorenzo et al. (2013) developed correlations that highlight the roles of basin-scale variability and small-scale variability (e.g., eddies) structuring the proximate drivers of ecosystem function. Similarly, our current general conceptual model builds on this and focusses on evaluating basin-scale to regional changes from winter to predict development of the subsequent spring coastal ecosystem. Its current structure results from a collection of studies through the years, shown roughly in Figure 2 as colored elements to highlight particular research and deduction pathways. It should be considered dynamic to address contemporary understanding and inclusive of components and functional relationships relevant to individual objectives, hypotheses, or management needs (Harvey et al., 2016; Wells et al., 2020). The model is built collaboratively by synthesizing the body of previous research and management objectives relative to the issue of concern. In doing so, the conceptual model becomes the hypothesis of ecosystem structure and dynamics. As research progresses a conceptual model is refined through a growing body of research effort to fill knowledge gaps. Our general conceptual model was seeded with a long history of foundational research (e.g., Hickey, 1979; Cury and Roy, 1989; Ainley and Boekelheide, 1990; Pearcy, 1992; Bakun, 1996; Checkley and Barth, 2009) on which we expand the understanding through researching successively more complicated and embedded processes and research directions (Santora et al., 2014; Wells et al., 2016; Wells et al., 2017; Friedman et al., 2018).
To explore processes related to salmon growth, we developed simplified conceptual models for hypothesized regional ecosystem function of the system and tested that structure with path analysis. For example, Wells et al. (2008b), linked Chinook salmon growth patterns to variability in basin-scale (e.g., Aleutian Low Pressure Index), regional- scale (i.e., wind stress, turbulence, upwelling, sea surface temperature), and local-scale (i.e., freshwater plumes) environmental influences. Cool basin conditions, increased regional upwelling (Figure 2, Boxes 1, 2), and a reduced plume size were related to increased salmon growth. The structured equation methodology, which arranges variables in a manner representing their sequential role in ecosystem structure, inherently required a hypothesized conceptual model and, when complete, provided context for the factors determining growth and the relative interactions and sensitivities of each factor and spatial scale affecting salmon.
We extended the structured equation approach to relate variability in forage fish recruitment (e.g., pelagic juvenile rockfishes; Sebastes spp.), seabird productivity and trophic dynamics in central California (Wells et al., 2008b). Namely, we developed a conceptual model of the system and tested hypotheses pertaining to effects of regional winds that determine local upwelling (Figure 2, Box 3), sea surface temperature and offshore advection (Figure 2, Boxes 4, 5) and examined the variability in the interacting dynamics between zooplankton, forage fish and seabird productivity. Path analysis allows us to quantify the relative roles of each environmental and biological ecosystem component on one another. As expected, cool conditions and increased upwelling increased prey resources and local seabird reproductive success. Our conceptual model indicated that certain ecosystem components were insignificant contributors to the trophic dynamics we examined, including wind speed (turbulence) and coastal sea level height (as an indicator of geostrophic flow and meridional transport). Importantly, variables, when examined individually (as bivariate relationships) to biological times series can often be significant due to correlations with other variables. Our approach, embedding them as components driving and being driven by additional variables, provided process-oriented context. We paired this analysis with Partial Least Squares regression to develop predictive models of ecosystem function and resultant biological variability (Fan et al., 2016). Therefore, with pathways and connected processes evaluated, an ecosystem sample unit of information is identified to assess relative state (high and low variability responses) as well as influence adaptive survey monitoring to inform decisions to mitigate or adapt to effects of novel states on ecosystem structure, fisheries, and communities (Figures 1A–C).
At the midpoint of our program, we focused on research and spearheaded efforts that evaluated the ecosystem structure as a consequence of current conditions. We knew then that the forage availability observed today results from previous spawning (Figure 2, Boxes 6, 7), nutrient (Figure 2, Box 8), and transport conditions (Figure 2, Boxes 9, 10). Schroeder et al. (2009; Schroeder et al., 2013) provides an excellent, and still standing example, of estimating local ecosystem productivity in spring and summer (including seabirds and forage fishes) by its relationship to the size and location of the North Pacific High pressure system in late winter (Figure 2, Box 1). Schroeder et al. (2013) demonstrated that a larger, more inshore North Pacific High-pressure cell in February and March results in increased upwelling and reduced coastal stratification in winter (Figure 2, Box 2) and, hence, increased nutrient introduction at the same time local forage fish are spawned (Figure 2, Boxes 6, 7) and seabirds prepare for the breeding season.
Upwelling phenology is a critical driver of California Current Ecosystem structure and function including associated stratification, nutrient input, and later productivity in the summer (Bograd et al., 2009). This work, in consideration of previous salmon models presented above, was expanded by Wells et al. (2017) to demonstrate that increased late-winter upwelling (Figure 2, Box 2), increased spring freshwater flow, and increased alternative prey available to foraging seabirds provides greater survival of emigrating Chinook salmon smolts. Specifically, cool, productive conditions couched in an appropriate conceptual model allowed us to diagnose the spatiotemporal role of the environment on local forage availability, seabird foraging behavior and the potential predation pressure on emigrating Chinook salmon (Figure 2 ‘Trophic structure’). This work was critical in demonstrating that the collapse of the 2005 Chinook salmon cohort was, in significant part, due to predation on salmon when alternative dispersed prey was unavailable and replaced with adult Northern anchovy which were inshore overlaying the distribution of emigrating salmon. Similarly, warming conditions in the North Pacific Ocean and reduced upwelling efficacy, lead to habitat compression of cool thermal habitat in coastal areas (Figure 2, Box 11), that relate to reduced widely dispersed prey (i.e., juvenile rockfishes) in favor of concentrations of Northern anchovy shoreward that overlap with commercial crab fishing areas (Figure 2 ‘Trophic structure’). As a result of these dynamics and reduced productivity of krill, whale entanglements in fixed gears during spring and summer is more likely when habitat compression is persistent and upwelling conditions are poor going into spring (Santora et al., 2020; Schroeder et al., 2022).
Develop an integrated survey plan that can document spatiotemporal variability of trophic levels and their interactions
Surveys are critical to evaluate the spatiotemporal dynamics of the ecosystem (Figure 1C). For illustrative purposes we can consider three types of surveys: stock assessment, environmentally-coupled, and integrative. Stock assessment surveys typically support estimation of abundance, demographic structure and distribution of individual or a suite of managed taxa. Such surveys can also provide guidance on population dynamics including distribution, growth, maturation, age-structure and diet. These surveys are generally designed to match the management-relevant spatial organization (e.g., management regions) and range of the taxa of interest which may not be the scale at which the ecosystem processes of interest are occurring (e.g., mesoscale). Coupled surveys, often arise from a modest alteration of a single-taxon survey in an attempt to address a specific question or concern (e.g., include environmental data collected synoptically with taxa of interest). Such a pairing allows researchers to explore bivariate functional responses of population dynamics and the proximate environment as well as provide physical indicators relevant to the taxa of biological interest as covariates in statistical or assessment models (e.g., species distribution models) (Figures 1A, B) (Schroeder et al., 2019; Malick et al., 2020; Muhling et al. 2020).
A more integrative, synthetic, spatiotemporally-appropriate survey design is required to examine the bottom-up, top-down, and middle-out aspects of the ecosystem and switches between the relative importance of processes (Figure 3). The California Current Ecosystem is one of the best surveyed systems worldwide with a number of large-scale, regional-scale, and small-scale surveys collecting predator data (e.g., distribution, diet), fishery data (e.g., distribution of Pacific hake (Merluccius productus), coastal pelagic species), as well as oceanographic data (McClatchie, 2016, Figures 3A, B). We rely a great deal on two surveys along the California Current Ecosystem that sample the ecosystem as a sample unit: Rockfish Recruitment and Ecosystem Assessment Survey (RREAS) in coastal California (Sakuma et al., 2016; Santora et al., 2021a), and the Pre-recruit survey in Oregon and Washington (Auth et al., 2017; Field et al., 2021)(Figures 3A, B). The combination of these surveys allows diagnosis of coastwide and meso-scale processes related to recruitment variability of groundfish and other epipelagic micronekton (Friedman et al., 2018; Field et al., 2021). The survey effort occurs in late spring, when the key coastal surface mixed layer properties (e.g., nutrients, stratification, temperature and salinity) and lower trophic levels (e.g., phytoplankton, zooplankton, larval forage fish) have developed in response to winter time physical forcing (Figures 3A–C). Acoustics are used to monitor forage distribution and visual surveys of seabird and marine mammal abundance and distribution are enumerated synoptically at the meso- and coastwide scales (Santora et al., 2012; Santora et al., 2021a; Santora et al., 2021b). The survey design allows for a full coastwide evaluation of typical and rare community states, such as geographic expansions of salps, pyrosomes, and Humboldt squid (Dosidicus gigas) (Wells et al., 2013, Field et al., 2013; Miller et al., 2019; Stewart et al. 2014).
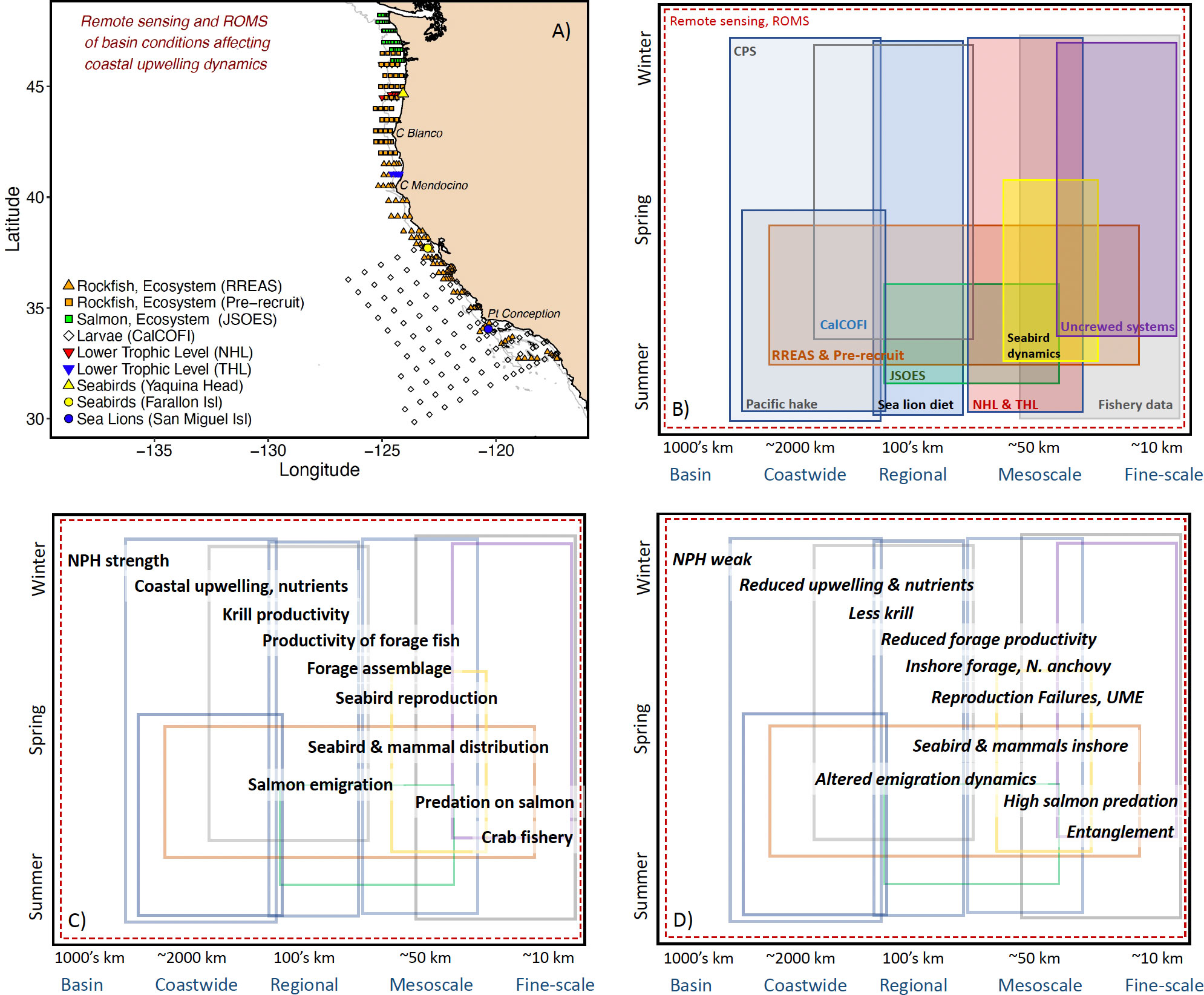
Figure 3 (A) A non-exhaustive accounting of the spatial scale of surveys and geographic prominences along the California Current Ecosystem. In addition to the surveys shown are the spatially-adaptive coastal pelagic survey (CPS) and Pacific hake acoustic surveys that encompass nearly the full California Current. As well, a number of uncrewed and mooring systems are implemented along the California Current. RREAS is Rockfish Recruitment and Ecosystem Assessment Survey, JSOES is Juvenile Salmon Ocean and Ecosystem Survey, CalCOFI is the California Cooperative Oceanic Fisheries Investigations Survey, NHL and THL are the Newport and Trinidad Hydrographic Lines. ROMS is Regional Ocean Modeling System. (B) Approximation of the spatiotemporal coverage of these data series. (C) An example of the spatiotemporal (e.g., phenological) dynamics of a subset of the ecosystem. This is, ultimately, a component of the general conceptual model (Figure 2) paired with data streams to demonstrate the importance of integration across surveys to address ecosystem-level questions and goals. NPH is the North Pacific High pressure system: modulates coastal upwelling. (D) An example of the development of select ecological surprises overlaying the survey and data streams available informing the sea-state and risk of these ecological outcomes. UME is Unusual Mortality Events of seabirds and mammals. Altered emigration dynamics can include timing, size, or condition of the salmon. Whale entanglements can occur at any time of the crab fishery (November – July) but we focus here on spring.
Survey designs need to accommodate various approaches and integrate with resources that provide greater spatiotemporal coverage than at-sea surveys alone can provide such as remote sensing, numerical models and an extensive uncrewed network beyond and deeper within the bounds of the ecosystem of direct interest. The RREAS and Pre-recruit efforts occur at a particularly important period in the evolution of the ecosystem, when a synoptic survey is critical (Figure 3C). To explore the evolution of the ecosystem we rely on other platforms collecting data on ecosystem components that are critical to the evolution of the California Current leading up to the spring and following it (e.g., satellite observations, moorings and data-assimilative ocean models)(Figure 3C). Autonomous vehicles (e.g., gliders) have provided context for the spatiotemporal oceanography within and without the boundaries of our ecosystem surveys. It is also important to consider means to include collection of data, when possible, on trophic levels that are underrepresented. For example, the inclusion of predator observers, diet data, acoustics and eDNA are simple cost-effective additions to surveys that provide greater context to the data collected from the individual survey and aids in filling spatiotemporal gaps in observations currently existing between surveys (Closek et al., 2019; Santora et al., 2021b). We have also partnered with California sea lion (Zalophus californianus) scat collections, seabird colony demography and diet, salmon weir counts, and remote sensing to contextualize the effects of the seascape observations on top-predators and their prey (e.g., environmental- and forage-dependent drivers of a California sea lion unusual mortality event in 2013; Wells et al., 2013; McClatchie et al., 2015; Warzybok et al., 2018; Figures 3C, D). Such an overarching effort includes collaboration with a number of academic, nongovernmental, and governmental groups dedicated to specific areas of research. Critical to the success of a multi-survey analytical approach is well-executed data sharing and a transdisciplinary approach to generation of hypotheses.
We examine spatiotemporal scales relevant to ecosystem dynamics across the survey efforts to inform the dynamics underpinning observed ecosystem states. In doing so, we inform the functional relationships with the over-arching conceptual model and provide spatiotemporal context for our observations (Figures 3C, D). A fundamental dynamic modulating ecosystem functioning along the California Current is the productivity and distribution of krill availability to foraging seabirds, whales and mesopredators such as Pacific hake and Chinook salmon. Santora et al. (2012; Santora et al., 2018) used data from the RREAS to demonstrate that krill productivity and distribution is largely determined by bathymetry, including canyon heads and additional areas where nutrient rich, cool water is persistently upwelled. Similarly, Friedman et al. (2018) examined the distribution of forage taxa and assemblages along the West Coast to demonstrate the mechanistic role and the conceptual model of environmental drivers of forage. This work was possible due to the extensive forage time series linked to in situ and remotely-sensed environmental data. These analyses have informed a mechanistic understanding of how prey species organize spatially along the California Current which is used as a starting point for describing spatiotemporal trophic dynamics. With the inclusion of visual predator surveys (i.e., at-sea observations), we can examine how variability in the assemblage and availability of forage leads to variability in seabird and mammal foraging behavior and distribution patterns that may relate to and result in ecological surprises such as whale entanglements or Chinook salmon cohort collapse (Figures 3C, D).
Quantify spatiotemporal variability of trophic interactions and processes
Over the past few decades, researchers and the managers they inform have focused on indicators of ocean climate state with the assumption that these metrics (e.g., sea surface temperature, sea level height) are indicators of ecosystem function (Williams et al., 2021), but they could also be simply a constellation of values providing presumed structure but isolated from any interactivity. Beyond a simple concern of unstable correlations, they can fail to capture the process and, hence, the bottom-up, middle out- and top-down consequences within the seascape on spatiotemporal variability in forage assemblages (Myers, 1998). Such improved understanding of functional relationships from basin-scale conditions to fine-scale reactions, as contextualized within ecosystem processes, allows the estimation of spatiotemporal ecosystem responses to variability of a3subsets of ecosystem components (Figures 1A, B, 3C, D). Hence, we promote quantification of functional responses linking spatiotemporal drivers and ecological impacts for the evaluation and parameterization of mechanistic ecosystem models. At the core of this assertion is that a driver of ecosystem state and resulting trophic structure acts at multiple scales and is not in isolation of additional drivers. For example, we have quantified the effects of size and strength of North Pacific High in winter on coastal and community productivity (Schroeder et al., 2009; Black et al., 2010; Schroeder et al., 2013), the varying functional relationships between the resulting forage availability, regionally and at fronts, on salmon diet and growth (e.g., Wells et al., 2012; Sabal et al., 2020), and quantified the potential for size-selective predation by seabirds on salmon in response to spatiotemporal variability in forage (Woodson et al., 2013b; Wells et al., 2017). These results serve to evaluate in-season risk but importantly parameterize ecosystem-level numerical models to diagnose past events and, potentially, explore future conditions (Figures 1A, B, D, 3D)(Henderson et al., 2019).
With an increased understanding of the environmental determinants of species abundance and distribution, including forage and predators, we explore spatiotemporal variability in trophic structure (e.g., Figure 2 ‘Trophic structure’). As forage availability and assemblages vary dependent on the environment, we have diagnosed how this covaries with salmon growth and survival, predator behaviors and human consequences, and we parameterize biophysical models with this observed environmentally-dependent variability (e.g., Fiechter et al., 2015; Friedman et al., 2019; Henderson et al., 2019; Cimino et al., 2020). In our case, salmon dynamics relative to the ecosystem state have largely been observed by returning salmon condition and abundance (Wells et al., 2006), coded-wire-tag recoveries (Henderson et al., 2019), and by condition of juvenile salmon collected at sea (Woodson et al., 2013b). Variability in prey assemblages and predator distributions and abundances has been quantified by at-sea surveys (Figure 3). Salmon diet has been examined by gut content analysis (e.g., Sabal et al., 2020: Wells et al. 2023). Observations of diet of returning seabirds on nesting sites has allowed us to quantify shifts in diet associated with shifts in the environment and forage availability (Mills et al., 2007; Roth et al., 2007; Wells et al., 2017; Warzybok et al., 2018). We have also led the development of a California Current Ecosystem Trophic Database with extensive time series of predator diets throughout the coast in anticipation of providing tools for others to explore the approaches we profess (Bizzarro et al., 2023). This database can improve our understanding and application of trophic structure and guilds to meet regional fishery science and management needs by improving process-oriented risk assessment and parameterization of ecosystem models (Figures 1A, B, D).
The starting point for exploring the ecosystem and trophic structural variability is developing an understanding of the determinants of distribution, abundance and assemblages of forage species at regional (~100-1000 km) and mesoscales (~10-100 km). Along the California Current, variability in forage fish assemblages is coherent at the scale of bioregions defined by oceanographic processes dominated by coastal promontories that shape the size and intensity of upwelling habitats (Gottscho, 2014; Friedman et al., 2018). Within these regions, forage species availability, biodiversity, and assemblage are dependent on mesoscale structuring around upwelling plumes, freshwater plumes, and bathymetry (Kaltenberg et al., 2010; Woodson et al. 2013a; Litz et al., 2014; Friedman et al., 2018; Santora et al., 2021a; Suca et al. 2022). The available habitat of cool nutrient-rich water within each of the primary bioregions varies relative to the strength of upwelling and transport (Schroeder et al., 2022). Forage communities are distributed relative to the mesoscale distributions of available upwelling, shadows, and plume habitats along the coast, compressing and expanding accordingly. At fine scales (~1-10km), bathymetric features act to concentrate cool nutrient-rich waters when there exist appropriate oceanographic conditions such as upwelling of deep waters and retention. Bakun’s Triad summarizes this concept well as enrichment, concentration and retention (Bakun, 1996) and Santora et al. (2017) provide an example of how this Triad relates to the development of trophic hotspots and the interactions they offer to human activities such as fishery extraction patterns and shipping practices. A well-defined research effort should be developed in consideration of these dynamics and capable of defining processes that are conflated within.
We develop climatologies of species distribution and develop an improved understanding of trophic hotspots where, by definition, trophic interactions are greatest. Quantifying the scale at which ecosystem processes occur is an important component of this understanding and management application (Figures 1A, B, D). Persistence, in a combined spatiotemporal way, indicates areas of trophic importance based on the recurrence of biophysical properties influencing species relative abundance patterns and their interactions. With access to at least 5-10 years of survey data with repeated coverage, we can determine where mesoscale trophic hotspots (~10-100 km) are likely to form and persist, which can better inform ecosystem monitoring and modeling studies (Santora et al., 2011; Santora et al., 2013; Santora et al., 2017). There exist a number of persistent hotspots within the California Current Ecosystem that, except under the most anomalous conditions (especially those related to excessive transport and turbulence), have a consistent supply of nutrient-rich deep and retained waters and relatively greater concentrations of forage fishes and krill (Graham and Largier, 1997; Wing et al., 1998; Santora et al., 2017; Wernberg et al. 2013). These aggregations are sought out by resident and migratory predators. While sub-mesoscale hotspots can be mobile, such as eddy cores and freshwater plume fronts, stationary hotspots, including seamounts, canyon breaks, and wind shadows are more prevalent along the West Coast (Hickey and Banas, 2008; Santora et al., 2011; Santora et al., 2018; Fiechter et al., 2020). We explored the role of submarine canyons as conduits for localized upwelling, retention of nutrients and enhancing trophic interactions between pelagic and coastal food webs (Hickey and Banas, 2008; Santora et al., 2018).
The ability for trophic hotspots to persist in the face of increased climate variability associated with climate change (e.g., stronger and longer marine heat waves, increased intensity and variability in upwelling) should be specifically considered. Important to having defined hotspots is quantifying the processes determining them and using numerical models to assess their spatiotemporal variability retrospectively and prospectively (Cimino et al., 2020; Fiechter et al., 2020; Messié et al., 2022). Statistical, biophysical and ecosystem modeling efforts should consider the role of trophic hotspots as buffers to negative environmental impacts going forward and management strategies should accommodate any positive roles (Figures 1A, B, D). They should also be considered from their functional and ecosystem services standpoint when management choices are made as they may provide an oasis and require specific protections from shipping, fishing, and other human impacts (e.g., resource extraction and renewable energy).
Link empirically-determined processes to biophysical models
A critical link between biophysical modeling, empirical studies, and management application is to first structure a biophysical model with the conceptual model and then parameterize its embedded functional relationships with empirical data (Figures 1A, B, D, E) (Collie et al., 2016). In doing this, the conceptual model acts as the wiring diagram for the numerical model which can then be used for evaluation of management strategies (Figures 1A, B, D, E). Therefore, the model is founded in empirically and mechanically quantified relationships. Fundamental to the ecosystem oceanographic approach is that the relationships change and can covary with environmental and fishery dynamics (e.g., effort, catches, fleet dynamics, targeting) (Cury et al., 2008). Therefore, rather than static rules underpinning the numerical model we argue that the wiring of the numerical model should allow for different structures under different conditions. The ecosystem oceanographic approach, founded in sampling and studying the ecosystem as a sample unit synthetically, provides a path for providing embedded evidence-based process-oriented variability in the modeled ecosystem structure. This provides an advance and increased trust when building and relying on approaches such as Ecosim, Ecopath and individual-based models; all of which can be parameterized to accommodate varying ecosystem trophic structure. For example, we have found that spatiotemporal variability in coastal upwelling conditions can alter the distribution and makeup of the forage base leading to changes in distribution, behavior, and the potential impact of predators. We show this in the inset of Figure 2 as the dominant prey and predator relationships under cool, upwelling and warm conditions. With an appropriately quantified model supporting observed structural shifts we can treat the wiring of the biophysical model as a switch board, varying appropriately with the environment; in this effort we have relied largely on individual based models (Fiechter et al., 2015; Henderson et al., 2019) and life-cycle models (Friedman et al., 2019) (Figures 1, 2). Hence, this approach provides the potential for more realistic scenario, risk, and management strategy evaluation. Biophysical modeling and empirical studies should be used in a complementary fashion to address hypotheses, assess ecosystem state, quantify functional relationships, and provide well-founded management actions (Figures 1B, D) (Seidl, 2017). Spatially-explicit numerical models can be used to investigate and manipulate ecosystem processes and their sensitivities to spatiotemporal variability in the environment (Cury et al., 2008). Modeling allows for a broader spatiotemporal perspective of the system and assessment of the processes embedded in it. By comparing modeled outcomes to observational data, we can also help to refine both the model parameterization and hypotheses pertaining to ecosystem functionality (Santora et al., 2013; Cimino et al., 2020; Fiechter et al., 2020; Santora et al., 2021a; Messié et al., 2022).
With increasing complexity and variability of ecosystem interactions in the face of climate change, a combined approach of observational data and models allows us to evaluate out-of-sample states and build informed, process-oriented conceptual models and hypotheses (Seidl, 2017; Wells et al., 2020). Given the importance of examining the spatiotemporal aspects of an ecosystem and the impossibility of covering it entirely with empirical observations, numerical models provide a potential avenue for filling in the gaps. Comparing model output and observational data, where available, we evaluate models for structural realism of modeled trophic interactions at the correct spatiotemporal scale (Cury et al., 2008; Santora et al., 2013). Two specific model comparisons we spearheaded include evaluations of ROMS-CoSiNE to capture spatiotemporal dynamics of krill (Chai et al., 2002; Santora et al., 2013) and output from ROMS-NEMURO to represent the physical environment determining variability in the coastal forage base (Schroeder et al., 2014). Once realism was confirmed, we also relied on model output to observe that distribution of modeled krill aggregations and observed predators are tied to bathymetry and associated oceanographic features (Santora et al., 2013; Fiechter et al. 2016; Fiechter et al., 2020). When predator observations, including seabirds and mammals, are related to the modeled mesoplankton, the distribution of predators is coherent with observed krill hotpots (Fiechter et al., 2020; Messié et al., 2022). This is a measurable success of integrating modeling and observations to describe potential spatiotemporal structure of trophic interactions.
As a specific example, we use biophysical models to predict and measure uncertainty of spatiotemporal variability of Chinook salmon growth and survival. We applied, with the inclusion of empirical study results, an individual-based model for Chinook salmon coupled to a dynamic energy budget model in which modeled salmon used a restricted search pattern for modeled krill (Fiechter et al., 2015; Henderson et al., 2019). The results confirmed aspects of our conceptual model (Figure 2) by demonstrating the roles preconditioning and krill availability have on Chinook salmon survival and the dynamics of poor salmon growth described through empirical analyses. We have current efforts to parameterize these models for seabird behavior dependent on a varying trophic structure (Figure 2, sensu Wells et al., 2017) and we are demonstrating that variability in growth of juvenile Chinook salmon across years can be substantial enough to leave salmon susceptible to predation for extended periods (K. Vasbinder, UCSC, unpublished data). This modeling framework enables a direct examination of the importance of synchronous recovery of predators and prey to protect Chinook salmon sustainability and recovery. Finally, our framework can be used to identify needs for supporting new and existing models to benefit ecosystem-based management and novel fishery challenges. For example, using existing species diet data and trophic databases, we are poised to develop spatially-explicit trophic models that can predict trophoscapes (i.e., spatially-explicit predictions of trophic relationships) to situate us to explore functionally-based distribution models, novel trophic interactions, and potential negative consequences of a varying predator behaviors across varying environmental conditions (e.g., Wells et al., 2017; Santora et al., 2020).
Example applications
A key objective of this ecosystem oceanography program is to inform management, reduce conflict with and among invested stakeholders (Conroy and Peterson, 2013; Townsend et al., 2019; Santora et al., 2020; Wells et al., 2020), and provide ecosystem-level context for contemporary and future ecosystem states to inform actions that might mitigate negative consequences. Specifically, Figure 1E provides examples of direct applications resulting from this program, ranging from co-developing risk assessment plans with stakeholders and fishery managers, efficient and rapid biodiversity and ecosystem assessments, building capacity to model and predict salmon growth and survival, to identifying gaps that can be addressed with new observing and modeling studies. As the program developed, we were able to provide disaggregated, but relevant, indicators of the state of ecosystem components such as forage assemblage characteristics, seabird reproductive success and water column properties. Based on previous observations, these indicators provide some consideration for the state of the ecosystem and are currently used qualitatively by our regional management council in consideration of management advice for setting salmon harvest and also provide inputs for improving rockfish assessment. These indicators, overtime, were embedded in a conceptual model (Figure 2) that provides an improved understanding of how they relate to one another and their sensitivity to basin and regional scale perturbations. In our case, the National Marine Sanctuaries and the Marine Biodiversity Observation Network use this model implicitly in their presentation of ecosystem state and standardized condition reports to communicate changes to manager and importantly, the public through outreach. Using the conceptual model as a foundation for structural equation modeling provides additional support for representing the relative importance of variability of any given indicator in risk assessments for salmon and whale entanglement, each provided to our regional management council and to the California Department of Fish and Wildlife, respectively. Our conceptual models have also been used to provide guidance in defining research gaps (Wells et al., 2008a; Wells et al., 2008b, Wells et al., 2020).
With a trusted conceptual model evaluated through extensive empirical observations and process studies, we have parameterized coupled ocean-ecosystem and biophysical models, permitting the exploration of ecosystem processes across multiple trophic levels. These biophysical models are tools that provide efficiency for estimation of spatial and temporal structure of the ecosystem that are now used to inform the productivity and distribution of krill and the potential of increased predation of salmon at sea. This information is used by the California Current Integrated Ecosystem Assessment to provide advice to the regional management council for risk assessments for survival of salmon in their first year at sea. Using these biophysical models, within-year risk assessments of potential whale entanglements are provided and used by California Department of Fish and Wildlife to develop spatial and temporal rules for fixed-gear fisheries. Finally, while still in development, these models are being expanded, with success, to provide a test bed for evaluating retrospective and prospective management strategies. Namely, our current objective is to evaluate the role of freshwater conditions on out-migration demographics (i.e., carry-over effects; Gosselin et al., 2021) and resulting survival of salmon in the first year at sea.
The path to application
Our specific applications are the result of what can be considered a general approach to applying ecosystem oceanography to management. Survey observations, lab experiments, uncrewed systems (e.g., gliders), numerical modeling output and suites of additional research endeavors are at the foundation of useful and applicable outcomes (Figures 1C, 3). As we inform the individual elements in the conceptual model through survey efforts and retrospective and near-real time biophysical model output, we provide early, non-integrative indications of ecosystem state alluded to through the conditions of each ecosystem component (Figures 1A–C, 2). As more data is accumulated and the components of the conceptual framework is fleshed out through the year, individual components and interactions can be contextualized and provide a system-level perspective of risk (Figure 3D). Examples of this approach along the California Current include observations of larval fish assemblages in winter to indicate future forage conditions faced by emigrating juvenile salmon (Daly et al., 2013) and seasonal observations on the developing basin, coastwide and regional ecosystem states to estimate risk of whale entanglements (Santora et al., 2020; Figure 3D). The risk assessment of whale entanglement and ecosystem conditions synthesized by Santora et al. (2020) was supported by Ocean Protection Council (https://www.opc.ca.gov/risk-assessment-and-mitigation-program-ramp/) and was developed in collaboration with fishers, port processors, federal resource managers and the California Department of Fish and Wildlife for monitoring periods of elevated entanglement risk throughout the Dungeness crab fishery season. This applied ecosystem oceanography approach has led to more effective communication and decision making regarding changing ocean-climate conditions and a decline in whale entanglements in recent years, however substantial economic challenges remain to mitigate the effect of fishery closures on coastal communities due to whale entanglements (Seary et al., 2022).
Ultimately, by understanding the ecosystem’s phenology and response to climate perturbations (e.g., expected outcomes from El Niño), we no longer need to treat each anomalous ecological event linked to Large Marine Heat Waves, El Niño, or poor upwelling conditions as an idiosyncratic outcome. In doing so, we can be ahead of issues even if the specifics of the issues themselves may be unique (e.g., dynamic ocean management; Lewison et al., 2015; Becker et al., 2016; Jacox et al., 2022; Stepanuk et al., 2022). Although research is leading to increased capacity to identify, classify and predict marine heat waves in near real time based on observed and modeled sea temperature anomalies, there is little effort to understand ocean processes (other than global warming trends) that govern dynamics between a heat wave event and dynamic socio-ecosystem outcomes. We currently do not fully understand the actual mechanisms driving thermal habitat compression in upwelling systems, but we do know that there are certain ecosystem shifts related to forage species and protected species that coincide with heat waves. We encourage researchers to not only classify temperature anomalies as heat wave products, but to link them to actual ecosystem indicators, and our guideposts may serve as a process for further strengthening our ecosystem modeling and forecast capacity. Cautiously, just focusing on correlations among indicators without an understanding of their connectivity and inter-relationships may lead to breakdowns in supporting dynamic ocean management initiatives. For example, due to previous indicator development for predicting rockfish recruitment strength, based on decades of observations, we were anticipating significantly lower rockfish recruitment during the 2014-2016 Large Marine Heat wave, when in fact, it was just the opposite. Specifically, late winter sea level height, which has no direct connection to rockfish recruitment (i.e., indictor of surface transport strength), had been used as an indicator for 20 years yet during the heat wave it failed as an indicator of one of the largest juvenile rockfish recruitment events in the past 40 years. This was a negative ecosystem surprise in the sense of prediction, but provided an exciting opportunity to mend the indicator and make it more conceptually sound and reflective of conditions adult rockfish experience in their benthic habitats (i.e., groundfish). In retrospect, having revisited the process of juvenile rockfish recruitment during the heat wave, we found that ocean basin scale of drivers demonstrated as water mass properties (e.g. source water variability) entering the coastal region are the actual determinant of rockfish recruitments and hence more capable at capturing rockfish recruitment and their biodiversity variability (Schroeder et al., 2019; Santora et al., 2021a). We learned from this surprise and increased our preparedness for developing models involving source waters as drivers of predicting rockfish recruitment which will ultimately benefit future stock assessments aimed at forecasting recruitment strength of year classes.
We can use the example ecosystem benefits flow diagram (Figure 1), informed by the conceptual model and empirical results, to illustrate the path between sampling, research and model development to provide advice on the risk of ecosystem shifts. This approach also provides realistic parameterization of ecosystem conditions for examining management strategies (Figures 1B, D). We can refine conceptual models and parameterize biophysical models with a number of years of observations, and use these models to evaluate the probable success of different management strategies retrospectively and prospectively. Such an approach provides potential paths forward to reduce socio-economic impacts resulting from recognized general ecosystem realizations. A management scenario evaluation can be in two phases: 1) retrospective analysis to develop an understanding of past conditions and processes and the development of strategies that had the potential of mitigating past ecological surprises and 2) a prospective analysis and strategy evaluation inclusive of ecosystem change scenario modeling informed by the results of the retrospective analysis. Critical to this approach is having as complete as possible understanding of spatiotemporal ecosystem-level processes. The inclusion of demonstrable mechanisms, considered in the context of a variable ecosystem structure, improves the realism of emergent properties from the future scenarios. However, more complexity in a modeling approach can reduce bias but uncertainty in output increases as a result of parameter uncertainty (Collie et al., 2012). Models attempting to represent an ecosystem realistically can be more poorly parameterized and unfocused for addressing objectives. However, models that are too focused may neglect to capture processes inherent in the ecosystem (Cury et al., 2008).
Conclusion
It has been our goal to provide context, based on California Current Ecosystem examples, of how to accomplish an ecosystem oceanography research approach and a programmatic effort that can provide general solutions and risk assessments for unique ecological conditions. We recognize that there are complex non-linearities within an ecosystem that make prediction, especially outside of observed conditions, imperfect. However, without an approach built on empirical observations and biophysical models capable of representing emergent qualities of the ecosystem we would be left in a considerably worse position when novel conditions arise from any such undiagnosed non-linearities. Namely, while ecosystem shifts and novel fishery management challenges themselves may be idiosyncratic, they are embedded in a generally understood ecosystem that can be observed as it evolves to diagnose issues in environmental and biological conditions from the basin- to meso-scales. It should be clear that as we developed this program, we did not have a straight-line path between our goals and the synthetic approach; much of our perspective was the result of dead ends and missteps and re-envisioning of conceptual models and hypothesis testing. Our perspective extends the Cury et al. (2008) framework to a body of work that has been initiated and will continue to be expanded in the California Current and hopefully provides a starting point for other ecosystems by removing some of the realistic and imagined barriers to its implementation. Key to the success of such an effort is data sharing, synthesis across research, surveys, and modeling approaches to capture the processes determining ecosystem state and structure in the context of its spatiotemporal evolution. Fundamental to this approach is treating the ecosystem as the sample unit and ecological surprises as having arisen from an understood process. Importantly, such ecosystem processes are system dependent but some can be generalized across disconnected ecosystems (e.g., prediction of trophic hotspot locations in upwelling systems). To accomplish this, we provide a few simple directions. 1) Develop a conceptual model informed by the previous science and human dimensions to identify gaps in our understanding. 2) Structure a well-designed survey synthesis that captures the spatiotemporal processes of the ecosystem of interest including those drivers outside of its bounds. 3) Use diet data and spatiotemporal variability in trophic interactions to quantify processes within and forcing of the ecosystem structure. 4) Tie empirically-determined processes to biophysical models to enable out-of-sample evaluation of ecosystem structure and functionality retrospectively and prospectively. We must provide management-relevant ecosystem products and strategic portfolios including process-oriented indicators of state and prioritization of management goals. Ultimately, research and management actions should be based on consideration of the potential frequency and likelihood of disruptive events over near and long-term time scales pertaining to predicted climate trends and ecosystem shifts impacting fishery socio-economic systems.
Author contributions
BW and JS contributed equally to the conception and writing of the manuscript. All authors contributed to the article and approved the submitted version.
Funding
JS acknowledges support by NASA US MBON 80NSSC20M0001.
Acknowledgments
As we have noted, this work is the result of many hands and collaborations. We thank D. Ainley, S. Bograd, R. Brodeur, F. Chavez, M. Cimino, J. Fiechter, J. Field, W. Friedman, K. Forney, E. Hazen, D. Huff, N. Mantua, M. Messié, W. Peterson, K. Richerson, I. Schroeder, and W. Sydeman.
Conflict of interest
The authors declare that the research was conducted in the absence of any commercial or financial relationships that could be construed as a potential conflict of interest.
Publisher’s note
All claims expressed in this article are solely those of the authors and do not necessarily represent those of their affiliated organizations, or those of the publisher, the editors and the reviewers. Any product that may be evaluated in this article, or claim that may be made by its manufacturer, is not guaranteed or endorsed by the publisher.
References
Ainley D. G., Boekelheide R. J. (1990). Seabirds of the Farallon Islands: Ecology, Dynamics, and Structure of an Upwelling-System Community (Stanford, CA: Stanford University Press).
Ainley D. G., Santora J. A., Capitolo P. J., Field J. C., Beck J. N., Carle R. D., et al. (2018). Ecosystem-based management affecting Brandt's Cormorant resources and populations in the central California Current region. Biol. Conserv. 217, 407–418. doi: 10.1016/j.biocon.2017.11.021
Auth T. D., Daly E. A., Brodeur R. D., Fisher J. L. (2017). Phenological and distributional shifts in ichthyoplankton associated with recent warming in the northeast Pacific Ocean. Global Change Biol. 24, 259–272. doi: 10.1111/gcb.13872
Bakun A. (1996). Patterns in the Ocean: Ocean Processes and the Marine Population Dynamics (National Oceanic and Atmospheric Administration in Cooperation with Centro de Investigaciones Biologicas del Noroeste: California Sea Grant College System).
Becker E. A., Forney K. A., Fiedler P. C., Barlow J., Chivers S. J., Edwards C. A., et al. (2016). Moving towards dynamic ocean management: How well do modeled ocean products predict species distributions? Remote Sensing. 8, 149. doi: 10.3390/rs8020149
Bindoff N. L., Cheung W. W. L., Kairo J. G., Arístegui J., Guinder V. A., Hallberg R., et al. (2019). “Changing Ocean, Marine Ecosystems, and Dependent Communities,” in IPCC Special Report on the Ocean and Cryosphere in a Changing Climate. Eds. Pörtner H.-O., Roberts D. C., Masson-Delmotte V., Zhai P., Tignor M., Poloczanska E., Mintenbeck K., Alegría A., Nicolai M., Okem A., Petzold J., Rama B., Weyer N. M. (Cambridge, UK and New York, NY, USA: Cambridge University Press), 447–587.
Bizzarro J. J., DeWitt L., Wells B. K., Curtis K. A., Santora J. A, Field J. C., et al. 2023. A multi-predator trophic database for the California Current Large Marine Ecosystem. Scientific Data. 10, 496. doi: 10.1038/s41597-023-02399-2
Black B. A., Schroeder I. D., Sydeman W. J., Bograd S. J., Lawson P. W. (2010). Wintertime ocean conditions synchronize rockfish growth and seabird reproduction in the central California Current ecosystem. Can. J. Fisheries Aquat. Sci. 67, 1149–1158. doi: 10.1139/F10-055
Bograd S. J., Schroeder I., Sarkar N., Qiu X. M., Sydeman W. J., Schwing F. B. (2009). Phenology of coastal upwelling in the California Current. Geophysical Res. Lett. 36, L01602. doi: 10.1029/2008gl035933
Chai F., Dugdale R. C., Peng T.-H., Wilkerson F. P., Barber R. T. (2002). One dimensional ecosystem model of the equatorial Pacific upwelling system, part I: model development and silicon and nitrogen Cycle. Deep-Sea Res. II 49, 2713–2745. doi: 10.1016/S0967-0645(02)00055-3
Checkley D. M., Barth J. A. (2009). Patterns and processes in the California current system. Prog. Oceanography 83, 49–64. doi: 10.1016/j.pocean.2009.07.028
Cimino M. A., Santora J. A., Schroeder I., Sydeman W., Jacox M. G., Hazen E. L., et al. (2020). Essential krill species habitat resolved by seasonal upwelling and ocean circulation models within the large marine ecosystem of the California Current System. Ecography 43, 1536–1549. doi: 10.1111/ecog.05204
Closek C. J., Santora J. A., Starks H. A., Schroeder I. D., Andruszkiewicz E. A., Sakuma K. M., et al. (2019). Marine vertebrate biodiversity and distribution within the central California Current using environmental DNA (eDNA) metabarcoding and ecosystem surveys. Front. Mar. Science. 6. doi: 10.3389/fmars.2019.00732
Collie J. S., Botsford L. W., Hastings A., Kaplan I. C., Largier J. L., Livingston P. A., et al. (2016). Ecosystem models for fisheries management: finding the sweet spot. Fish Fisheries 17, 101–125. doi: 10.1111/faf.12093
Collie J. S., Peterman R. M., Zuehlke B. M. (2012). A fisheries risk-assessment framework to evaluate trade-offs among management options in the presence of time-varying productivity. Can. J. Fisheries Aquat. Sci. 69, 209–223. doi: 10.1139/f2011-148
Conroy M. J., Peterson J. T. (2013). Decision Making in Natural Resource Management: A Structured, Adaptive Approach (Hoboken: First. John Wiley and Sons).
Cury P., Roy C. (1989). Optimal environmental window and pelagic fish recruitment success in upwelling areas. Can. J. Fisheries Aquat. Sci. 46, 670–680. doi: 10.1139/f89-086
Cury P. M., Shin Y. J., Planque B., Durant J. M., Fromentin J. M., Kramer-SChadt S., et al. (2008). Ecosystem oceanography for global change in fisheries. Trends Ecol. Evol. 23, 338–346. doi: 10.1016/j.tree.2008.02.005
Daewel U. T. E., Peck M. A., Kuehn W., ST. JOHN M., Alekseeva I., Schrum C. (2008). Coupling ecosystem and individual-based models to simulate the influence of environmental variability on potential growth and survival of larval sprat (Sprattus sprattus L.) in the North Sea. Fisheries Oceanography 17, 333–351. doi: 10.1111/j.1365-2419.2008.00482.x
Daewel U., Schrum C., Gupta A. K. (2015). The predictive potential of early life stage individual-based models (IBMs): an example for Atlantic cod Gadus morhua in the North Sea. Mar. Ecol. Prog. Ser. 534, 199–219. doi: 10.3354/meps11367
Daly E. A., Auth T. D., Brodeur R. D., Peterson W. T. (2013). Winter ichthyoplankton biomass as a predictor of early summer prey fields and survival of juvenile salmon in the northern California Current. Mar. Ecol. Prog. Ser. 484, 203–217. doi: 10.3354/meps10320
DeMaster D. P., Fowler C. W., Perry S. L., Richlen M. E. (2001). Predation and competition: The impact of fisheries on marine-mammal populations over the next one hundred years. J. Mammalogy 82, 641–651. doi: 10.1644/1545-1542(2001)082<0641:PACTIO>2.0.CO;2
Di Lorenzo E., Combes V., Keister J. E., Strub P. T., Thomas A. ,. C., Franks P. J., et al. (2013). Synthesis of Pacific Ocean climate and ecosystem dynamics. Oceanography 26, 68–81. doi: 10.5670/oceanog.2013.76
Dudley P. N., Rogers T. L., Morales M. M., Stoltz A. D., Sheridan C. J., Beulke A. K., et al. (2021). A more comprehensive climate vulnerability assessment framework for fisheries social-ecological systems. Front. Mar. Sci. 8, 674. doi: 10.3389/fmars.2021.678099
Edwards M., Beaugrand G., Hays G. C., Koslow J. A., Richardson A. J. (2010). Multi-decadal oceanic ecological datasets and their application in marine policy and management. Trends Ecol. Evol. 25, 602–610. doi: 10.1016/j.tree.2010.07.007
Fan Y., Chen J., Shirkey G., John R., Wu S. R., Park H., et al. (2016). Applications of structural equation modeling (SEM) in ecological studies: an updated review. Ecol. Processes 5, 19. doi: 10.1186/s13717-016-0063-3
Fiechter J., Huckstadt L. A., Rose K. A., Costa D. P. (2016). A fully coupled ecosystem model to predict the foraging ecology of apex predators in the California Current. Mar. Ecol. Prog. Ser. 556, 273–285. doi: 10.3354/meps11849
Fiechter J., Huff D. D., Martin B. T., Jackson D. W., Edwards C. A., Rose K. A., et al. (2015). Environmental conditions impacting juvenile Chinook salmon growth off central California: An ecosystem model analysis. Geophysical Res. Lett. 42, 2910–2917. doi: 10.1002/2015GL063046
Fiechter J., Santora J. A., Chavez F., Northcott D., Messié M. (2020). Krill hotspot formation and phenology in the California current ecosystem. Geophysical Res. Lett. 47, e2020GL088039. doi: 10.1029/2020GL088039
Field J. C., Elliger C., Baltz K., Gillespie G. E., Gilly W. F., Ruiz-Cooley R. I., et al. (2013). Foraging ecology and movement patterns of jumbo squid (Dosidicus gigas) in the California Current System. Deep Sea Res. Part II: Topical Stud. Oceanography 95, 37–51.
Field J. C., Miller R. R., Santora J., Tolimieri N., Haltuch M., Brodeur R., et al. (2021). Saptiotemporal patterns of variability in the abundance and distribution of winter-spawned pelagic juvenile rockfish in the California Current. PLOS ONE 6, e0251638. doi: 10.1371/journal.pone.0251638
Filbee-Dexter K., Pittman J., Haig H. A., Alexander S. M., Symons C. C., Burke M. J. (2017). Ecological surprise: Concept, synthesis, and social dimensions. Ecosphere 8, e02005. doi: 10.1002/ecs2.2005
Fisher M. C., Moore S. K., Jardine S. L., Watson J. R., Samhouri J. F. (2021). Climate shock effects and mediation in fisheries. Proc. Natl. Acad. Sci. 118, e2014379117. doi: 10.1073/pnas.2014379117
Fleming A. H., Clark C. T., Calambokidis J., Barlow J. (2016). Humpback whale diets respond to variance in ocean climate and ecosystem conditions in the California Current. Global Change Biol. 22, 1214–1224. doi: 10.1111/gcb.13171
Friedman W. R., Martin B. T., Wells B. K., Michel C., Warzybok P., Danner E. M., et al. (2019). Composite effects of marine and freshwater processes on salmon population dynamics. Ecosphere 10, e02743. doi: 10.1002/ecs2.2743
Friedman W. R., Santora J. A., Schroeder I. D., Huff D. D., Brodeur R. D., Field J. C., et al. (2018). Environmental and geographic relationships among salmon forage assemblages along the continental shelf of the California Current. Mar. Ecol. Prog. Ser. 596, 181–198. doi: 10.3354/meps12598
Gosselin J. L., Buhle E. R., Van Holmes C., Beer W. N., Iltis S., Anderson J. J. (2021). Role of carryover effects in conservation of wild Pacific salmon migrating regulated rivers. Ecosphere. 12, e03618. doi: 10.1002/ecs2.3618
Gottscho A. D. (2014). Zoogeography of the San Andreas Fault system: Great Pacific Fracture Zones correspond with spatially concordant phylogeographic boundaries in western North America. Biol. Rev. 91, 235–254. doi: 10.1111/brv.12167
Graham W. W., Largier J. L. (1997). Upwelling shadows as nearshore retention sites: the example of northern Monterey Bay. Continental Shelf Res. 17, 509–532. doi: 10.1016/S0278-4343(96)00045-3
Harvey C. J., Reum J. C. P., Poe M. R., Williams G. D., Kim S. J. (2016). Using conceptual models and qualitative network models to advance integrative assessments of marine ecosystems. Coast. Manage. 44, 486–503. doi: 10.1080/08920753.2016.1208881
Haury L. R., McGowan J. A., Wiebe P. H. (1978). Patterns and processes in the time-space scales of plankton distributions. Ed. Steele J. H. (Plenum, New York, New York, USA: Spatial pattern in plankton communities), 277–327.
Henderson M., Fiechter J., Huff D. D., Wells B. K. (2019). Spatial variability in ocean-mediated growth potential is linked to Chinook salmon survival. Fisheries Oceanography 28, 334–344. doi: 10.1111/fog.12415
Hickey B. M. (1979). The California current system–hypotheses and facts. Prog. Oceanography 8, 191–279. doi: 10.1016/0079-6611(79)90002-8
Hickey B. M., Banas N. S. (2008). Why is the northern end of the California Current System so productive? Oceanography 21, 90–107. doi: 10.5670/oceanog.2008.07
Hidalgo M., Bartolino V., Coll M., Hunsicker M. E., Travers-Trolet M., Browman H. I. (2022). Adaptation science’ is needed to inform the sustainable management of the world's oceans in the face of climate change. ICES J. Mar. Sci. 79, 457–462. doi: 10.1093/icesjms/fsac014
Holland D. S., Leonard J. (2020). Is a delay a disaster? economic impacts of the delay of the California dungeness crab fishery due to a harmful algal bloom. Harmful Algae. 98, 101904. doi: 10.1016/j.hal.2020.101904
Jacox M., Alexander M., Amaya D., Becker E., Bograd S., Steven S., et al. (2022). Global seasonal forecasts of marine heatwaves. Nature. 604, 486–490. doi: 10.1038/s41586-022-04573-9
Kaltenberg A. M., Emmett R. L., Benoit-Bird K. J. (2010). Timing of forage fish seasonal appearance in the Columbia River plume and link to ocean conditions. Mar. Ecol. Prog. Ser. 419, 171–184. doi: 10.3354/meps08848
Lam V., Cheung. W., Reygondeau G., Sumaila U. R. (2016). Projected change in global fisheries revenues under climate change. Sci. Rep. 6, 32607. doi: 10.1038/srep32607
Levin S. A. (1992). The problem of pattern and scale in ecology: the Robert H. MacArthur award lecture. Ecol. 73, 1943–1967. doi: 10.2307/1941447
Lewison R., Hobday A. J., Maxwell S., Hazen E., Hartog J. R., Dunn D. C., et al. (2015). Dynamic ocean management: Identifying the critical ingredients of dynamic approaches to ocean resource management. BioScience 65, 486–498. doi: 10.1093/biosci/biv018
Lindley S. T., Grimes C. B., Mohr M. S., Peterson W., Stein J., Anderson J. T., et al. (2009). What caused the Sacramento River fall Chinook stock collapse. (Silver Spring, MD: NOAA)
Litz M. N. C., Emmett R. L., Bentley P. J., Claiborne A. M., Barceló C. (2014). Biotic and abiotic factors influencing forage fish and pelagic nekton community in the Columbia River plume (USA) throughout the upwelling season 1999–2009. ICES J. Mar. Sci. 71, 5–18. doi: 10.1093/icesjms/fst082
Malick M. J., Hunsicker M. E., Haltuch M. A., Parker-Stetter S. L., Berger A. M., Marshall K. N. (2020). Relationships between temperature and Pacific hake distribution vary across latitude and life-history stage. Mar. Ecol. Prog. Ser. 639, 185–197. doi: 10.3354/meps13286
McCabe R. M., Hickey B. M., Kudela R. M., Lefebvre K. A., Adams N. G., Bill B. D., et al. (2016). An unprecedented coastwide toxic algal bloom linked to anomalous ocean conditions. Geophysical Res. Lett. 43, 10,310–366,376. doi: 10.1002/2016GL070023
McClatchie S. (2016). Regional fisheries oceanography of the California Current System (New York, NY: Springer).
McClatchie S., Field J., Thompson A. R., Gerrodette T., Lowry M., Fiedler P. C., et al. (2015). Food limitation of sea lion pups and the decline of forage off central and southern California. R. Soc. Open Sci. 3, 1–9. doi: 10.1098/rsos.150628
Messié M., Sancho-Gallegos D., Fiechter J., Santora J. A., Chavez F. (2022). Satellite-based lagrangian model reveals how upwelling and oceanic circulation shape krill hotspots in the California current system. Front. Mar. Science. 9. doi: 10.3389/fmars.2022.835813
Miller R. R., Santora J. A., Auth T. D., Sakuma K. M., Wells B. K., Field J. C., et al. (2019). Distribution of pelagic thaliaceans, Thetys vagina and Pyrosoma atlanticum, during a period of mass occurrence within the California Current. California Cooperative Oceanic Investigations (CalCOFI) Rep. 60, 94–108.
Mills K. L., Laidig T., Ralston S., Sydeman W. J. (2007). Diets of top predators indicate pelagic juvenile rockfish (Sebastes spp.) abundance in the California Current System. Fisheries Oceanography 16, 273–283. doi: 10.1111/j.1365-2419.2007.00429.x
Mills K. E., Pershing A. J., Brown C. J., Chen Y., Chiang F. S., Holland D. S., et al. (2013). Fisheries management in a changing climate lessons from the 2012 ocean heat wave in the Northwest Atlantic. Oceanography 26, 191–195. doi: 10.5670/oceanog.2013.27
Möllmann C., Lindegren M., Blenckner T., Bergström L., Casini M., Diekmann R., et al. (2014). Implementing ecosystem-based fisheries management: from single-species to integrated ecosystem assessment and advice for Baltic Sea fish stocks. ICES J. Mar. Sci. 71, 1187–1197. doi: 10.1093/icesjms/fst123
Moore J. W., Schindler D. E. (2022). Getting ahead of climate change for ecological adaptation and resilience. Science. 376, 1421–1426. doi: 10.1126/science.abo3608
Muhling B. A., Brodie S., Smith J. A., Tommasi D., Gaitan C. F., Hazen E. L., et al. (2020). Predictability of species distributions deteriorates under novel environmental conditions in the california current system. Front. Mar. Sci. 7, 589. doi: 10.3389/fmars.2020.00589
Myers R. A. (1998). When do environment–recruitment correlations work? Rev. Fish Biol. Fisheries 8, 285–305. doi: 10.1023/A:1008828730759
Pearcy W. G. (1992). Ocean ecology of North Pacific salmonids (Seattle, WA: Washington Sea Grant Program).
Roth J. E., Mills K. L., Sydeman W. J. (2007). Chinook salmon (Oncorhynchu’s tshawytscha) – seabird covariation off central California and possible forecasting applications. Can. J. Fisheries Aquat. Sci. 64, 1080–1090. doi: 10.1139/f07-081
Sabal M. C., Hazen E. L., Bograd S. J., MacFarlane R. B., Schroeder I. D., Hayes S. A., et al. (2020). California Current seascape influences juvenile salmon foraging ecology at multiple scales. Mar. Ecol. Prog. Series 634, 159–173. doi: 10.3354/meps13185
Sakuma K. M., Field J. C., Mantua N. J., Ralston S., Marinovic B. B., Carrion C. N. (2016). Anomalous epipelagic micronekton assemblage patterns in the neritic waters of the California Current in spring 2015 during a period of extreme ocean conditions. California Cooperative Oceanic Investigations (CalCOFI) Rep. 57, 163–183.
Samhouri J. F., Stier A. C., Hennessey S. M., Novak M., Halpern B. S., Levin P. S. (2017). Rapid and direct recoveries of predators and prey through synchronized ecosystem management. Nat. Ecol. Evol. 1, 68. doi: 10.1038/s41559-016-0068
Santora J. A., Field J. C., Schroeder I. D., Sakuma K. M., Wells B. K., Sydeman W. J. (2012). Spatial ecology of krill, micronekton and top predators in the central California Current: Implications for defining ecologically important areas. Prog. Oceanography 106, 154–174. doi: 10.1016/j.pocean.2012.08.005
Santora J. A., Mantua N. J., Schroeder I. D., Field J. C., Hazen E. L., Bograd S. J., et al. (2020). Habitat compression and ecosystem shifts as potential links between marine heatwave and record whale entanglements. Nat. Commun. 2020 (11), 1 11:1–1 1112. doi: 10.1038/s41467-019-14215-w
Santora J. A., Rogers T. L., Cimino M. A., Sakuma K. M., Hansen K. D., Dick E. J., et al. (2021b). Diverse integrated ecosystem approach overcomes pandemic-related fisheries monitoring challenges. Nat. Commun. 12, 6492. doi: 10.1038/s41467-021-26484-5
Santora J. A., Schroeder I. D., Bograd S. J., Chavez F. P., Cimino M. A., Fiechter J., et al. (2021a). Pelagic biodiversity, ecosystem function, and services: an integrated observing and modeling approach. Oceanography 34, 16–37. doi: 10.5670/oceanog.2021.212
Santora J. A., Schroeder I. D., Field J. C., Wells B. K., Sydeman W. J. (2014). Spatio-temporal dynamics of ocean conditions and forage taxa reveals regional structuring of seabird-prey relationships. Ecol. Appl. 24, 1730–1747. doi: 10.1890/13-1605.1
Santora J. A., Sydeman W. J., Messié M., Chai F., Chao Y., Thompson S. A., et al. (2013). Triple check: Observations verify structural realism of an ocean ecosystem model. Geophysical Res. Lett. 40, 1367–1372. doi: 10.1002/grl.50312
Santora J. A., Sydeman W. J., Schroeder I. D., Field J. C., Miller R. R., Wells B. K. (2017). Persistence of trophic hotspots and relation to human impacts within an upwelling marine ecosystem. Ecol. Applications. 27, 560–574. doi: 10.1002/eap.1466
Santora J. A., Sydeman W. J., Schroeder I. D., Wells B. K., Field J. C. (2011). Mesoscale structure and oceanographic determinants of krill hotspots in the California Current: Implications for trophic transfer and conservation. Prog. Oceanography 91, 397–409. doi: 10.1016/j.pocean.2011.04.002
Santora J. A., Zeno R., Dorman J. G., Sydeman W. J. (2018). Submarine canyons represent an essential habitat network for krill hotspots in a Large Marine Ecosystem. Sci. Rep. 2018 (8), 1 8:1–1 8:9. doi: 10.1038/s41598-018-25742-9
Schroeder I. D., Black B. A., Sydeman W. J., Bograd S. J., Hazen E. L., Santora J. A., et al. (2013). The North Pacific High and wintertime pre-conditioning of California Current productivity. Geophysical Res. Lett. 40, 541–546. doi: 10.1002/grl.50100
Schroeder I. D., Santora J. A., Bograd S. J., Hazen E. L., Sakuma K. M., Moore A. M., et al. (2019). Source water variability as a driver of rockfish recruitment in the California Current ecosystem. Can. J. Fisheries Aquat. Sci. 76, 950–960. doi: 10.1139/cjfas-2017-0480
Schroeder I. D., Santora J. A., Mantua N., Field J. C., Wells B. K., Hazen E. L., et al. (2022). Habitat compression indices for monitoring ocean conditions and ecosystem impacts within coastal upwelling systems. Ecol. Indicators. 144, 109520. doi: 10.1016/j.ecolind.2022.109520
Schroeder I. D., Santora J. A., Moore A. M., Edwards C. A., Fiechter J., Hazen E. L., et al. (2014). Application of a data-assimilative regional ocean modeling system for assessing California Current System ocean conditions, krill, and juvenile rockfish interannual variability. Geophysical Res. Letters. 41, 5942–5950. doi: 10.1002/2014GL061045
Schroeder I. D., Sydeman W. J., Sarkar N., Thompson S. A., Bograd S. J., Schwing F. B. (2009). Winter pre-conditioning of seabird phenology in the California Current. Mar. Ecol. Prog. Ser. 393, 211–223. doi: 10.3354/meps08103
Schwing F. B. (2023). Modern technologies and integrated observing systems are “instrumental” to fisheries oceanography: A brief history of ocean data collection. Fisheries Oceanography. 32, 28–69. doi: 10.1111/fog.12619
Seary R., Santora J. A., Tommasi D., Thompson A., Bograd S. J., Richerson K., et al. (2022). Revenue loss due to whale entanglement mitigation and fishery closures. Sci. Rep. 12, 21554. doi: 10.1038/s41598-022-24867-2
Seidl R. (2017). To Model or not to Model, That is no Longer the Question for Ecologists. Ecosystems 20, 222–228. doi: 10.1007/s10021-016-0068-x
Solomon S., Plattner G., Knutti R., Friedlingstein P. (2009). Irreversible climate change due to carbon dioxide emmisions. Procedures Natural Acad. Science. 106, 1704–1709. doi: 10.1073/pnas.0812721106
Stepanuk J. E., Kim H., Nye J. A., Roberts J. J., Halpin P. N., Palka D. L., et al. (2022). Subseasonal forecasts provide a powerful tool for dynamic marine mammal management. Front. Ecol. Environment 21, 117–2509. doi: 10.1002/fee.2506
Stewart J. S., Hazen E. L., Bograd S. J., Byrnes J. E., Foley D. G., Gilly W. F., et al. (2014). Combined climate-and prey-mediated range expansion of Humboldt squid (Dosidicus gigas), a large marine predator in the California Current System. Global Change Biol. 20, 1832–1843. doi: 10.1111/gcb.12502
Stommel H. (1963). Varieties of Oceanographic Experience: The ocean can be investigated as a hydrodynamical phenomenon as well as explored geographically. Science 139, 572–576. doi: 10.1126/science.139.3555.572
Suca J. J., Santora J. A., Field J. C., Curtis K. A., Muhling B. A., Cimino M. A., et al. (2022). Temperature and upwelling dynamics drive market squid (Doryteuthis opalescens) distribution and abundance in the California Current. ICES J. Mar. Science. 79, 2489–2509. doi: 10.1093/icesjms/fsac186
Tommasi D., deReynier Y., Townsend H., Harvey C. J., Satterthwaite W. H., Marshall K. N., et al. (2021). Connecting fisheries management challenges with models and analysis to support ecosystem-based management in the california current ecosystem. Front. Mar. Sci 8, 624161. doi: 10.3389/fmars.2021.624161
Townsend H., Harvey C. J., DeReynier Y., Davis D., Zador S. G., Gaichas S., et al. (2019). Progress on implementing ecosystem-based fisheries management in the United States through the use of ecosystem models and analysis. Front. Mar. Sci. 6, 641. doi: 10.3389/fmars.2019.00641
Trainer V. L., Kudela R. M., Hunter M. V., Adams N. G., McCabe R. M. (2020). Climate extreme seeds a new domoic acid hotspot on the US West Coast. Front. Climate 2, 23. doi: 10.3389/fclim.2020.571836
Turner E., Haidvogel D. B., Hofmann E. E., Batchelder H. P., Fogarty M. J., Powell T. (2013). US GLOBEC: Program goals, approaches, and advances. Oceanography 26, 12–21. doi: 10.5670/oceanog.2013.72
Warzybok P., Santora J. A., Ainley D. G., Bradley R. W., Field J. C., Capitolo P. J., et al. (2018). Prey switching and consumption by seabirds in the central California Current upwelling ecosystem: Implications for forage fish management. J. Mar. Syst. 185, 25–39. doi: 10.1016/j.jmarsys.2018.04.009
Watters G. M., Hill S. L., Hinke J. T., Matthews J., Reid K. (2013). Decision-making for ecosystem-based management: Evaluating options for a krill fishery with an ecosystem dynamics model. Ecol. Applications. 23, 710–725. doi: 10.1890/12-1371.1
Wells B. K., Field J. C., Thayer J. A., Grimes C. B., Bograd S. J., Sydeman W. J., et al. (2008a). Untangling the relationships among climate, prey and top predators in an ocean ecosystem. Mar. Ecol. Prog. Ser. 364, 15–29. doi: 10.3354/meps07486
Wells B. K., Grimes C. B., Field J. C., Reiss C. S. (2006). Covariation between the average lengths of mature coho (Oncorhynchus kisutch) and Chinook salmon (O-tshawytscha) and the ocean environment. Fisheries Oceanography 15, 67–79. doi: 10.1111/j.1365-2419.2005.00361.x
Wells B. K., Grimes C. B., Sneva J. G., McPherson S., Waldvogel J. B. (2008b). Relationships between oceanic conditions and growth of Chinook salmon (Oncorhynchus tshawytscha) from California, Washington, and Alaska, USA. Fisheries Oceanography 17, 101–125. doi: 10.1111/j.1365-2419.2008.00467.x
Wells B. K., Huff D. D., Burke B. J., Brodeur R. D., Santora J. A., Field J. C., et al. (2020). Implementing ecosystem-based management principles in the design of a salmon ocean ecology program. Front. Mar. Sci. 7, 342. doi: 10.3389/fmars.2020.00342
Wells B. K., Santora J. A., Bizzarro J., Billings A., Brodeur R. D., Daly E. A., et al. (2023). Trophoscapes of predatory fish reveal biogeographic structuring of spatial dietary overlap and inform fisheries bycatch patterns. Mar. Ecol. Prog. Series. doi: 10.3354/meps14319
Wells B. K., Santora J. A., Field J. C., MacFarlane R. B., Marinovic B. B., Sydeman W. J. (2012). Population dynamics of Chinook salmon Oncorhynchus tshawytscha relative to prey availability in the central California coastal region. Mar. Ecol. Prog. Ser. 457, 125–137. doi: 10.3354/meps09727
Wells B. K., Santora J. A., Henderson M. J., Warzybok P., Jahncke J., Bradley R. W., et al. (2017). Environmental conditions and prey-switching by a seabird predator impact juvenile salmon survival. J. Mar. Syst. 174, 54–63. doi: 10.1016/j.jmarsys.2017.05.008
Wells B. K., Santora J. A., Schroeder I. D., Mantua N., Sydeman W. J., Huff D. D., et al. (2016). Marine ecosystem perspectives on Chinook salmon recruitment: a synthesis of empirical and modeling studies from a California upwelling system. Mar. Ecol. Prog. Ser. 552, 271–284. doi: 10.3354/meps11757
Wells B. K., Schroeder I. D., Santora J. A., Hazen E. L., Bograd S. J., Bjorkstedt E. P., et al. (2013). State of the California Current 2012-2013: No such thing as an A’verage’ year. California Cooperative Oceanic Investigations (CalCOFI) Rep. 54, 37–71.
Wernberg T., Smale D. A., Tuya F., Thomsen M. S., Langlois T. J., De Bettignies T., et al. (2013). An extreme climatic event alters marine ecosystem structure in a global biodiversity hotspot. Nat. Climate Change. 3, 78–82. doi: 10.1038/nclimate1627
Williams G. D., Andrews K. S., Brown J. A., Gove J. M., Hazen E. L., Leong K. M., et al. (2021). Place-based ecosystem management: Adapting integrated ecosystem assessment processes for developing scientifically and socially relevant indicator portfolios. Coast. Management. 49, 46–71. doi: 10.1080/08920753.2021.1846154
Wing S. R., Botsford L. W., Ralston S. V., Largier J. L. (1998). Meroplanktonic distribution and circulation in a coastal retention zone of the northern California upwelling system. Limnology Oceanography 43, 1710–1721. doi: 10.4319/lo.1998.43.7.1710
Woodson L. E., Wells B. K., Grimes C. B., Franks R. P., Santora J. A., Carr M. H. (2013a). Water and otolith chemistry identify exposure of juvenile rockfish to upwelled waters in an open coastal system. Mar. Ecol. Prog. Ser. 473, 261–273. doi: 10.3354/meps10063
Keywords: ecosystem monitoring and management, salmon, whale entanglement, food webs, biophysical models
Citation: Wells BK and Santora JA (2023) Implementing an ecosystem oceanography program to increase capacity and preparedness for dynamic ocean management and fishery challenges. Front. Mar. Sci. 10:1192052. doi: 10.3389/fmars.2023.1192052
Received: 22 March 2023; Accepted: 31 July 2023;
Published: 17 August 2023.
Edited by:
Stelios Katsanevakis, University of the Aegean, GreeceReviewed by:
Jacqueline Mary Grebmeier, University of Maryland, College Park, United StatesUrsula Scharler, University of KwaZulu-Natal, South Africa
Copyright © 2023 Wells and Santora. This is an open-access article distributed under the terms of the Creative Commons Attribution License (CC BY). The use, distribution or reproduction in other forums is permitted, provided the original author(s) and the copyright owner(s) are credited and that the original publication in this journal is cited, in accordance with accepted academic practice. No use, distribution or reproduction is permitted which does not comply with these terms.
*Correspondence: Brian K. Wells, brian.wells@noaa.gov; Jarrod A. Santora, jarrod.santora@noaa.gov
†These authors have contributed equally to this work and share senior authorship