- 1Department of Biology, Faculty of Marine and Environmental Sciences, University of Cádiz, Puerto Real, Spain
- 2Institute of Marine Research (INMAR), Marine Campus of International Excellence (CEIMAR), Agrifood Campus of International Excellence (ceiA3), Puerto Real, Spain
- 3The European University of the Seas (SEA-EU), Cádiz, Spain
- 4Sorbonne Université, CNRS, Biologie Intégrative des Organismes Marins (BIOM), Banyuls-sur-Mer, France
- 5International Cancer Laboratory Co., Ltd, Chuo-ku, Tokyo, Japan
- 6Institute of Aquaculture of Torre la Sal, CSIC, Ribera de Cabanes, Castellón, Spain
Neurosteroids are involved in the regulation of multiple behavioral and physiological processes and metabolic activities in the vertebrate brain. However, central mechanisms of how neurosteroid synthesis is regulated is far to be understood. Gonadotropin-inhibitory hormone (GNIH) is a hypothalamic neuropeptide that negatively regulates gonadotropin secretion but also inhibits sexual and aggressive behaviors in birds and mammals by modulating aromatase enzyme and neuroestrogen synthesis. In a previous study performed in male sea bass, we reported that Gnih inhibited the reproductive axis by acting at the three levels of the brain-pituitary-gonad axis. Moreover, the presence of Gnih cells and fibers in the telencephalon, mesencephalon and rhombencephalon suggests a role of Gnih in regulating other important brain functions in sea bass, including behavior. In this study, we have analyzed the effects of the intracerebroventricular (icv) injection of sbGnih-2 on the brain and pituitary expression of the main neurosteroids-synthesizing enzymes (stAR, cyp17, 3β-hsd, 17β-hsd, cyp19b, cyp7b), as well as on estrogen and androgen receptors (erα, erβ1, erβ2, ar). A combination of immunohistochemistry and in situ hybridization was also used to identify putative interaction of Gnih- and aromatase-positive cells. We also performed a mirror test study as a proxy to measure aggression levels and agonistic behavior after icv injection of sbGnih-2. Central administration of sbGnih-2 at different doses reduced the transcript levels of 3β-hsd and 17β-hsd, and increased the expression of cyp19b (brain aromatase) in the sea bass brain. Neuroanatomical results suggest that paracrine and neuroendocrine actions could mediate Gnih effects on aromatase expression. Central administration of sbGnih-2 also decreased the pituitary expression of 17β-hsd and estrogen receptors (erβ2). The mirror test analysis showed that sbGnih-2 affected the agonistic/aggressive behavior of sea bass as revealed by the decreased interaction with the mirror, lower time spent in the mirror zone, increased latency to establish contact with the mirror and higher mean distance to the mirror zone. In contrast, locomotor activity parameters measured were not affected by sbGnih-2 injection. Taken together, our results showed for the first time in fish that Gnih inhibits social-aggressive behavior and affects the gene expression of neurosteroid-synthesizing enzymes giving rise to neuroandrogens and neuroestrogens in the sea bass brain.
Introduction
The brain is not only a target for steroid hormones produced in peripheral tissues such as the gonads, the interrenal/adrenal glands and the mammalian placenta but also has its local production by converting cholesterol and circulating steroids into neuroactive steroids known as neurosteroids (Ubuka and Tsutsui, 2022). Neurosteroids have been involved in the regulation of multiple physiological processes, metabolic activities and behaviors, including food intake, sexual activity, aggressiveness, anxiety, depression, body temperature, blood pressure, brain plasticity and neurogenesis in tetrapod vertebrates (Lapchak and Araujo, 2001; Belelli et al., 2006; Strous et al., 2006; Do Rego et al., 2009; Azcoitia et al., 2018; He et al., 2019). In teleost fish, neurosteroids also appear implicated on sex differentiation, reproduction, stress, neurogenesis, cell migration and social-aggressive behavioral responses (Diotel et al., 2013; Huffman et al., 2013; Haraguchi et al., 2015; Pellegrini et al., 2016; Ramallo et al., 2017). Although it has been established that aggressive behavior of male fish is largely dependent of testicular androgens (Oliveira et al., 2002; O’Connor et al., 2011; Teles and Oliveira, 2016), in some cases, plasma circulating androgen concentration was not related to the aggressive rating of teleosts (Vullioud et al., 2013; Almeida et al., 2014). It should be noted that the brain of fish is well known for its capacity to synthesize androgens and to convert testicular and locally-synthesized androgens (notably, testosterone) into estrogens (estradiol), whose actions are mediated by brain androgen and estrogen receptors (Callard et al., 1990; Diotel et al., 2011a). The transformation of C19 androgens, such as testosterone (T), into C18 estrogens, such as estradiol (E2), is elicited by the brain aromatase (cytochrome P450) enzyme, indicating that estrogens signaling could also play an important role in the brain of males (Perkins and Roselli, 2007; Ramallo et al., 2017; Azcoitia et al., 2018). Brain aromatase, encoded by the cyp19b gene, has been involved in the inhibition of aggressive behavior in different teleost species studied to date. For example, in a social species like the African cichlid fish Astatotilapia burtoni, subordinate males expressed higher concentration levels of aromatase than dominant males (Huffman et al., 2013). In the blue-banded goby (Lythrypnus dalli), a sex-changing fish, it was also observed that socially induced decreases in brain aromatase levels produced an increase in aggressive behavior, suggesting that aromatase activity may modulate changes in teleost social behavior (Black et al., 2005). Despite its functional significance, little is known about how aromatase synthesis and activity are regulated in relation to behavior in fish.
In 2000, a novel neuropeptide belonging to the RFamide family was identified in the hypothalamus of the quail (Coturnix japonica) and it was reported that it actively inhibited the gonadotropin secretion on the pituitary gland (Tsutsui et al., 2000). As a result, it was termed as gonadotropin-inhibitory hormone or GNIH. Within the mammalian brain, GNIH neurons were present in the dorsomedial (DMH), paraventricular (PVN) and ventromedial (VMH) nuclei of the hypothalamus (Kriegsfeld et al., 2006; Clarke et al., 2008; Dardente et al., 2008; Soga et al., 2014), whereas they were restricted to PVN neurons in birds (Tsutsui et al., 2000; Bentley et al., 2003; Ukena et al., 2003; Osugi et al., 2004). However, teleosts seem to exhibit a much wider distribution of Gnih cells, at least in some of the species analyzed so far (Muñoz-Cueto et al., 2017; Di Yorio et al., 2019).
On the other hand, increasing evidences are indicating that neuropeptides and neurosteroids interactions can play an important role in the regulation of brain functions (Do Rego et al., 2009). Recently, Ubuka and colleagues demonstrated that GNIH regulates neuroestrogen synthesis by stimulating the activity of cytochrome P450 aromatase in the quail brain, and proposed that GNIH could inhibit male socio-sexual behavior throughout its actions on aromatase-producing cells located in the preoptic area of male birds (Ubuka et al., 2014). So far, in teleost fish, which represent more than half of vertebrates, most of the reported effects of Gnih have been focused on the reproductive axis, i.e., regulation of gonadotropin synthesis and release, seasonal reproduction and steroidogenesis (Muñoz-Cueto et al., 2017; Di Yorio et al., 2019). However, the role that Gnih plays in the regulation of neurosteroids synthesis and behavior remains unknown in fish.
The European sea bass (Dicentrarchus labrax) is one of the most important species for European aquaculture and has been commonly used in our laboratory as model species for neuroanatomical, neuroendocrinological, physiological, cellular, molecular and behavioral studies (Cerdá-Reverter et al., 2001a; Cerdá-Reverter et al., 2001b; González-Martínez et al., 2001; González-Martínez et al., 2002; Cerdá-Reverter et al., 2008; Servili et al., 2009; Servili et al., 2010; Sánchez-Vázquez and Muñoz-Cueto, 2014; Paullada-Salmerón et al., 2016a; Paullada-Salmerón et al., 2016b; Paullada-Salmerón et al., 2016c; Cowan et al., 2017; Paullada-Salmerón et al., 2017; Paullada-Salmerón et al., 2019; Wang et al., 2022; Paullada-Salmerón et al., 2023). Recently, we identified a sea bass gnih gene encoding a precursor with two different RFamide peptides, named as sbGnih-1 and sbGnih-2, and elucidated the immunohistochemical localization of Gnih neurons and their projections in the brain and pituitary by using specific antibodies against both peptides (Paullada-Salmerón et al., 2016a). In agreement to other fish species (Biswas et al., 2015; Di Yorio et al., 2016; Ogawa et al., 2016; Aliaga-Guerrero et al., 2018), our results evidenced a profuse innervation of Gnih neurons entering the neurohypophysis to control gonadotropin secretion and targeting neuroendocrine brain regions such as the ventral telencephalon, preoptic area and hypothalamus but also reaching non-hypophysiotrophic regions as the habenula and midbrain/hindbrain areas, suggesting that Gnih might be also involved in the regulation of behavior in fish (Paullada-Salmerón et al., 2016a). Moreover, both sbGnih-1 and sbGnih-2 decreased androgen (T and 11-ketotestosterone, 11-KT) plasma levels at early- and mid-spermatogenesis stages, affecting the progression testicular gametogenesis (Paullada-Salmerón et al., 2016c).
In order to better understand the role of Gnih system on neurosteroids synthesis and function, in the present study we investigated the in vivo effects of intracerebroventricular (icv) injection of sbGnih-2 peptide on brain and pituitary expression of neurosteroidogenic enzymes (steroidogenic acute regulatory protein, star; cytochrome P450 17α-hydroxylase/C-17,20-lyase, cyp17; 3-beta-hydroxysteroid dehydrogenase, 3β-hsd; 17-beta-hydroxysteroid dehydrogenase, 17β-hsd; brain cytochrome P450 aromatase, cyp19b; cytochrome P450 7b, cyp7b), as well as estrogen and androgen receptors (erα, erβ1, erβ2, ar). Moreover, we also tried to elucidate the existence of potential interactions between Gnih-immunoreactive and aromatase-expressing cells by using a combination of immunohistochemical and in situ hybridization methods. Finally, in order to investigate putative behavioral actions of Gnih in sea bass, we performed a mirror test analysis to determine the sbGnih-2 effects on agonistic behavior and aggression levels in this species.
Materials and methods
Animals
For gene expression, immunohistochemical and in situ hybridization analyses, two-years-old adult spermiating male specimens of European sea bass, Dicentrarchus labrax (459 ± 103 g in weight), were obtained from CUPIMAR S.L (San Fernando, Spain) and housed in the “Laboratorio de Cultivos Marinos” (University of Cádiz, Puerto Real, Spain). All animals were kept under natural winter photoperiod conditions and constant temperature and salinity (19 ± 1°C and 39 ‰, respectively) and fed once per day with commercial dry pellets (1% body weight, Skretting España S.A, Burgos, Spain) using automatic feeders (EHEIM GmbH & Co. KG, Deizisau, Germany) 2 h after light onset.
For behavioral analysis, two-years-old adult spermiating male specimens of European sea bass, D. labrax (354 ± 53 g in weight), were obtained from “Méditerranée Pisciculture” (Salses-le-Château, France) and kept in tanks of the Mutualized Aquariology Service of the Oceanological Observatory of Banyuls-sur-Mer (Sorbonne Université, CNRS, Banyuls-sur-Mer, France). All animals were acclimated during 3 weeks in 1 m3 indoor tanks (35 fish per tank) supplied with Mediterranean sea water (∼37 ‰ salinity along the French Mediterranean coast) provided by an open circuit, at a flow rate of ∼400 l/h, and maintained under natural temperature and simulated natural photoperiod (light provided by fluorescent bulbs). Fish were fed ad libitum with commercial dry pellets (Skretting France, Fontaine-lès-Vervins, France), administered once per day 2 h after light onset.
The animal experimental protocols were designed and approved in accordance with the Animal Care and Use Committee of the University of Cádiz (Spain) and of the Oceanological Observatory of Banyuls-sur-Mer (France) and in agreement with European Union Regulation (EC. Directive 86/609/EEC) concerning the protection of experimental animals.
Hormones
The sea bass gonadotropin-inhibitory hormone-2 peptide (sbGnih-2, GenBank accession no. LN681205, SPNSTPNMPQRF-NH2, Paullada-Salmerón et al., 2016a) was synthesized by Metabion International AG (Planegg, Germany). Synthetic peptide was amidated at the C-terminal end, purified by high-performance liquid chromatography (>98% purity) and dissolved in phosphate buffer saline (PBS) 1x sterilized according to the manufacturer’s instructions.
Gnih administration
For the gene expression and mirror test analyses, animals were acclimatized for several weeks prior to the administration of the sbGnih-2 peptide in experimental tanks with identical photoperiod, temperature and salinity conditions to the housed tanks. Gnih peptide was injected to the fish intracerebroventricularly (icv) according to the procedure described by Paullada-Salmerón et al. (2016b). Briefly, sbGnih-2 was administrated in the third ventricle using a 25 μl Hamilton microsyringe fitted with a 26-G needle (Hamilton, Reno, NV, USA) and driven by a micromanipulator. Each fish was anesthetized and immobilized and the needle was immediately inserted into a small hole drilled in the dorsal surface of the skull to dispense the peptide or the vehicle into the sea bass third ventricle. No mortality was observed after icv injection.
Gene expression analysis
At the beginning of the reproductive season, forty male sea bass were used to determine the effect of sbGnih-2 on brain and pituitary expression of neurosteroids-synthesizing enzymes and steroid receptors genes. These animals were divided into four different groups: three experimental groups and one control group of ten fish each. The three experimental groups were injected with sbGnih-2 at 1, 2 and 4 μg doses dissolved in 10 μl of PBS, respectively, while the control group received only PBS. Sampling was carried out at 6 and 12 h post-injection (hpi), according to our established method and previous results (Paullada-Salmerón et al., 2016b). At the end of the experiment, all fish were anesthetized by immersion in MS-222 and the brain and pituitary gland were dissected, frozen in liquid nitrogen, and stored at -80°C until assay.
For real-time quantitative PCR analysis (RT-qPCR), the procedure for RNA isolation and reverse transcription was performed as previously described by Paullada-Salmerón et al. (2016b). Total RNA was isolated from sea bass brain and pituitary by TRIsure reagent (Bioline, London, UK), according to the manufacturer’s protocol. Tissues were homogenized in a mixer mill MM400 (Retsch GmbH, Haan, Germany) and total RNA concentration and quality were measured on a NanoDrop 2000 spectrophotometer (Thermo Fisher Scientific, Waltham, MA, USA). Total RNA (1μg) was retro-transcripted and DNA removed using a Primer ScripTM RT Reagent Kit with gDNA Eraser (Takara Bio Inc., Shiga, Japan). To analyze brain and pituitary expression of neurosteroidogenic enzymes (star, cyp17, 3β-hsd, 17β-hsd, cyp19b, cyp7b), estrogen and androgen receptors (erα, erβ1, erβ2, ar), RT-qPCR was conducted by using Bio-Rad CFX96 Touch detection system (Bio-Rad, Richmond, CA, USA) with the SYBR Premix ExTaqTM (Tli RNase H Plus; Takara Bio Inc.). Primers were purchased from Integrated DNA Technologies (Coralville, IA, USA) and sequences, amplicon size and PCR conditions are shown in Table 1. Experiments were done by duplicates in the same test. Standard curves were generated for each gene with 10-fold serial dilutions of cDNA, and all calibration curves exhibited slopes close to 3.32 and efficiencies around 100%. Melting curves were performed for each sample in order to confirm that a single product was amplified. As internal standard housekeeping genes l17 and elfα were used (Table 1). The relative expression of genes analyzed was calculated by the ΔΔ-Ct method (Livak and Schmittgen, 2001).
Immunohistochemical analysis
For the immunohistochemical localization of Gnih, we used immunofluorescence and a specific rabbit polyclonal antibody against sbGnih-2 generated and previously validated in our laboratory (Paullada-Salmerón et al., 2016a). Before immunostaining, sections were transferred for 15 min to Coons buffer pH 7.4 (0.01 M Veronal, 0.15 M NaCl) containing 0.1-0.2% Triton X-100 (CBT) and then saturated in CBT containing 0.5% bovine serum albumin for 30 min. Sections were incubated overnight in a moist chamber at room temperature with sbGnih-2 antibody (1:1500 dilution in CBT 0.5% bovine albumin serum). Sections were washed in CBT (3 x 10 min) and incubated for 2 h in a dark chamber at room temperature with goat anti-rabbit Alexa Fluor 594 or 488 (1:500 dilution, Jackson ImmunoResearch Laboratories, West Grove, PA, USA). After washing in CBT, slides were coverslipped with Vectashield mounting medium with DAPI (Vector laboratories, Burlingame, CA, USA). To confirm the specificity of the immunostaining, controls were performed by preabsorption of primary sbGnih-2 antiserum with synthetic sbGnih-1 (NH2-PLHLHANMPMRF-CONH2) and sbGnih-2 (NH2-SPNSTPNMPQRF-CONH2) peptides (100 ng/μl), replacement of primary antiserum with the corresponding preimmunize serum, and omission of primary or secondary antisera.
Double immunohistochemical and in situ hybridization detection of sbGnih-2 and aromatase-expressing cells in sea bass brain
In order to identify putative colocalization and/or interactions of sbGnih-2 and aromatase-expressing cells, we used a combination of immunohistochemical and in situ hybridization procedures. Three male sea bass specimens were anesthetized and transcardially perfused with 0.65% NaCl followed by 4% paraformaldehyde with 0.2% picric acid in PBS buffer pH 7.5 at 4°C. Then, brains were dissected, postfixed overnight at 4°C, cryoprotected in 15% sucrose solution and embedded in Tissue-Tek. Serial coronal brain sections obtained with a cryomicrotome were first processed for in situ hybridization and, subsequently, for sbGnih-2 immunostaining as indicated above.
For in situ hybridization, messenger RNA used for the synthesis of specific riboprobes corresponded to the ORF-coding region of the European sea bass brain aromatase (Blázquez and Piferrer, 2004). The specific riboprobes, of 584 nucleotides in length, were synthesized using a pSpark II vector (Canvax Biotech, Córdoba, Spain) containing DNA sequence of sea bass brain aromatase synthesized by Bio Basic Inc. (Markham, Canada). Antisense and sense single-stranded mRNA probes were obtained by transcription with T7 and SP6 RNA polymerases (Promega Biotech Ibérica S.L., Alcobendas, Spain) on plasmids linearized with SacI and SacII restriction enzymes using DIG RNA labelling MIX (Sigma Aldrich, Madrid, Spain). The size and integrity of the DIG-labelled antisense and sense probes were checked by 1% agarose gel electrophoresis under denaturing conditions, and their concentration was evaluated by spectrophotometric analysis.
In situ hybridization was performed as described previously (Frau et al., 2022). Cryostat sections were rinsed in 1x PBS pH 7,4 and post-fixed in PAF 4% diluted in PBS. After postfixation, the slides were washed two times in PBS and deproteinized with proteinase K at 37°C for 5 minutes in PBS, washed, and postfixed again in 4% PAF. Next, sections were rinsed at room temperature in 2x SSC buffer for 10 min. Before hybridization, the antisense and sense DIG-probes were preheated at 75°C for 5 minutes. The slides were then hybridized at 60°C overnight in a hybridization buffer (2 × SSC; 2.5% dextran sulphate; 50% deionized formamide; 5 × Denhardt’s solution; 50 μg/ml of yeast tRNA, pH 8; 4 mM EDTA) with riboprobes. Subsequently, the slides were rinsed in SSC at 60°C, followed by two rinses at 60 °C for 30 min in SSC/50% deionized formamide. The sections were rinsed in 0.2 and 0.1 x SSC at room temperature and blocked for 1 h in a solution of 100 mM Tris-HCl buffer, 150 mM NaCl (pH 7.5) with 0.5% BSA, and 0.2% Triton X-100. Afterwards, the slides were incubated overnight with an alkaline phosphatase-conjugated anti-DIG antibody (1:500; Sigma Aldrich). The third day, the sections were revealed with NBT/BCIP (stock solution; Sigma Aldrich) for 0.5-1 h. After washing with PBS, immunohistochemistry of Gnih was performed following the protocol described above, and sections were coverslipped with Vectashield mounting medium with DAPI (Vector Laboratories) and observed under a fluorescence photomicroscope as indicated below.
Analysis of images and figure production
Immunohistochemical and in situ hybridization sections were observed under a Zeiss Imager D2 photomicroscope (Zeiss, Oberkochen, Germany) and images were acquired with an Axiocam 503 camera (Zeiss) and processed using the ZEN software (Zeiss). Plates were assembled using Photoshop CS6 (Adobe Systems, San Jose, CA, USA) without alteration except for light or contrast adjustments. For the precise localization of immunoreactive sbGnih-2 and aromatase-expressing cells, a sea bass brain atlas was used (Cerdá-Reverter et al., 2001a; Cerdá-Reverter et al., 2001b; Cerdá-Reverter et al., 2008), with some changes in the nomenclature to adapt it to the current neuromeric/prosomeric models of brain organization (Puelles, 2019).
Behavioral analysis
During the reproductive period, thirty pairs of sea bass males were used to test the effect of sbGnih-2 administration on agonistic behavior and aggression levels by using the mirror test. Animals were anesthetized and sbGnih-2 was administered in the third ventricle between 8.30 and 11:00 h with a single dose of 2 μg dissolved in 10 μl of PBS, which was revealed as the most effective dose after icv injection in our previous study performed in sea bass (Paullada-Salmerón et al., 2016b), while the control group was injected with PBS alone. Subsequently, animals were placed in the experimental tank fifteen minutes before the behavioral test for recovery. For mirror test and behavioral data analysis, the experimental procedure described by Manciocco and coworkers (Manciocco et al., 2015) was used with slight modification. Fifteen minutes after administration of sbGnih-2 or vehicle, the behavior of each pair of male sea bass was recorded for 30 minutes using a digital camera (Supplemental Material 1). The position of the camera was defined to allow video coverage of the whole surface of the experimental tank (110 x 70 x 50 cm). The camera was turned on avoiding sudden movements and no evidence of stress behavior (e.g., no change in swimming movements or change in pigmentation) was observed. All experimental fish pairs were tested out of their home tanks, isolated from the social group, and the behavior of both fishes from each pair was recorded and analyzed (15 pairs, n = 30 for control fish; 15 pairs, n = 30 for sbGnih-2 injected fish). Behavioral analysis was performed using Noldus EthoVision XT software and some parameters defined by Noldus system (proximity, relative movement, mobility, acceleration state, velocity and distance moved, latency to the first event, C-shape bending of the body in front of the mirror) were analyzed. All parameters measured are shown in Table 2. In each pair of animals, the first animal that moved was defined as the focal subject and its recognition was carried out based on slight body differences (e.g., the size, color, shape of the caudal fin), while the second individual was defined as the companion. There were no statistical differences in the behavioral parameters analyzed between the focal and the companion subjects of each pair of fish and, therefore, both individuals were used for the statistical analysis presented in the results section.
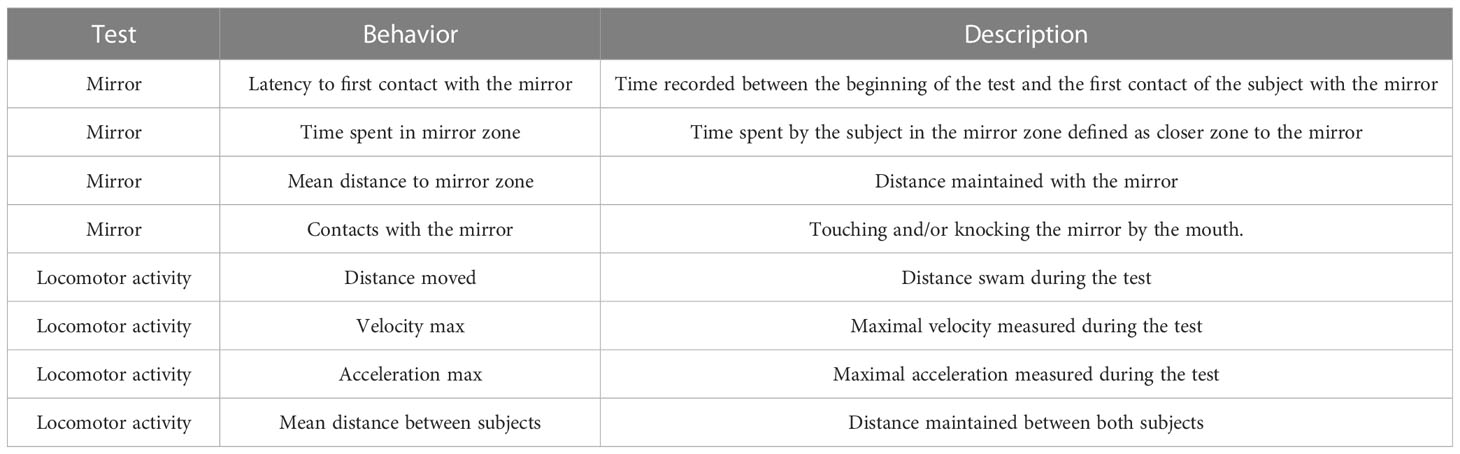
Table 2 Behaviors recorded in the mirror test, including parameters related to the mirror and locomotor activity of male sea bass specimens.
Data analysis
All results are expressed as mean ± standard error of the mean (SEM). Statistical differences in relative gene expression levels, hormonal levels and behavioral response were examined by one-way ANOVA followed by Tukey post hoc test using Statgraphics Centurion XVI software (Statpoint Technologies, Warrenton, VA, USA) and GraphPad Prims 9 Software (San Diego, USA). Before the analysis, datasets were tested for normality and homoscedasticity of variance, and if necessary, were log- or square route-transformed. When data did not fulfil the requirements of the ANOVA, data were analyzed using the Kruskal Wallis non-parametric test. In all cases, statistical significance was established at p<0.05.
Results
Physiological effects of sbGnih-2 on the expression of brain and pituitary neurosteroids-synthesizing enzymes and steroid receptors
In the brain, icv injection of sbGnih-2 provoked a reduction of 17β-hsd mRNA levels at 6 hpi with the higher doses (2 and 4 μg; p<0.0088 and 0.0452, respectively; Figure 1D) and a significant decrease on 3β-hsd expression at 12 hpi with the three doses tested (1, 2 and 4 μg; p<0.0073, 0.0056 and 0.0222, respectively; Figure 2C), as well as an increased expression of cyp19b at 6 hpi with the lower dose (1 μg; p<0.0002; Figure 1E). No significant effects on brain mRNAs levels of star (Figures 1A, 2A), cyp17 (Figures 1B, 2B), 3β-hsd (at 6 hpi, Figure 1C), 17β-hsd (at 12 hpi, Figure 2D), cyp19b (at 12 hpi, Figure 2E), cyp7b (Figures 1F, 2F), erα (Figures 1G, 2G), erβ1 (Figures 1H, 2H), erβ2 (Figures 1I, 2I) and ar (Figures 1J, 2J) were observed in fish treated with sbGnih-2 peptide.
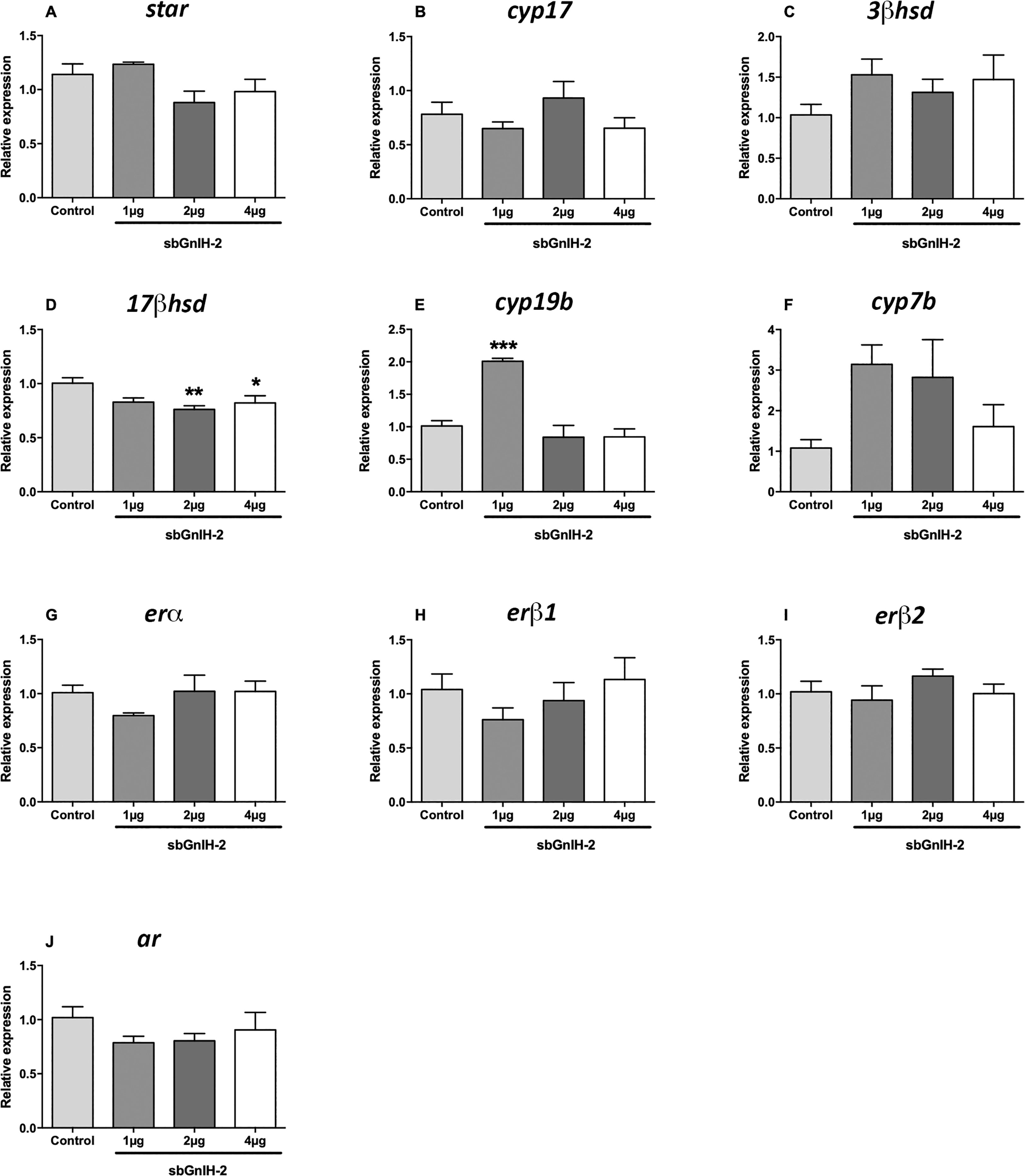
Figure 1 Effects of in vivo intracerebroventricular (icv) injection of different doses (1μg, 2μg and 4μg) of sbGnih-2 on the relative expression of neurosteroids-synthesizing enzymes and steroid receptors in the brain of male sea bass at 6 hours post-injection. (A). Steroidogenic acute regulatory protein (star); (B). Cytochrome P450 17alpha-hydroxylase/17,20-lyase (cyp17); (C). 3β-hydroxysteroid dehydrogenase (3β-hsd); (D). 17β-hydroxysteroid dehydrogenase (17β-hsd); (E). Brain cytochrome P450 aromatase (cyp19b); (F). Cytochrome P450 7α-hydroxylase (cyp7b); (G). Estrogen receptor alpha (erα); (H). Estrogen receptor beta 1 (erβ1); (I). Estrogen receptor beta 2 (erβ2). (J). Androgen receptor (ar). Results are expressed as mean ± SEM (n=5). Asterisks denote significant differences between mean values of Gnih-treated animals and controls by one-way ANOVA followed by Tukey post hoc test (*, p<0.05; **, p<0.01; ***, p<0.001).
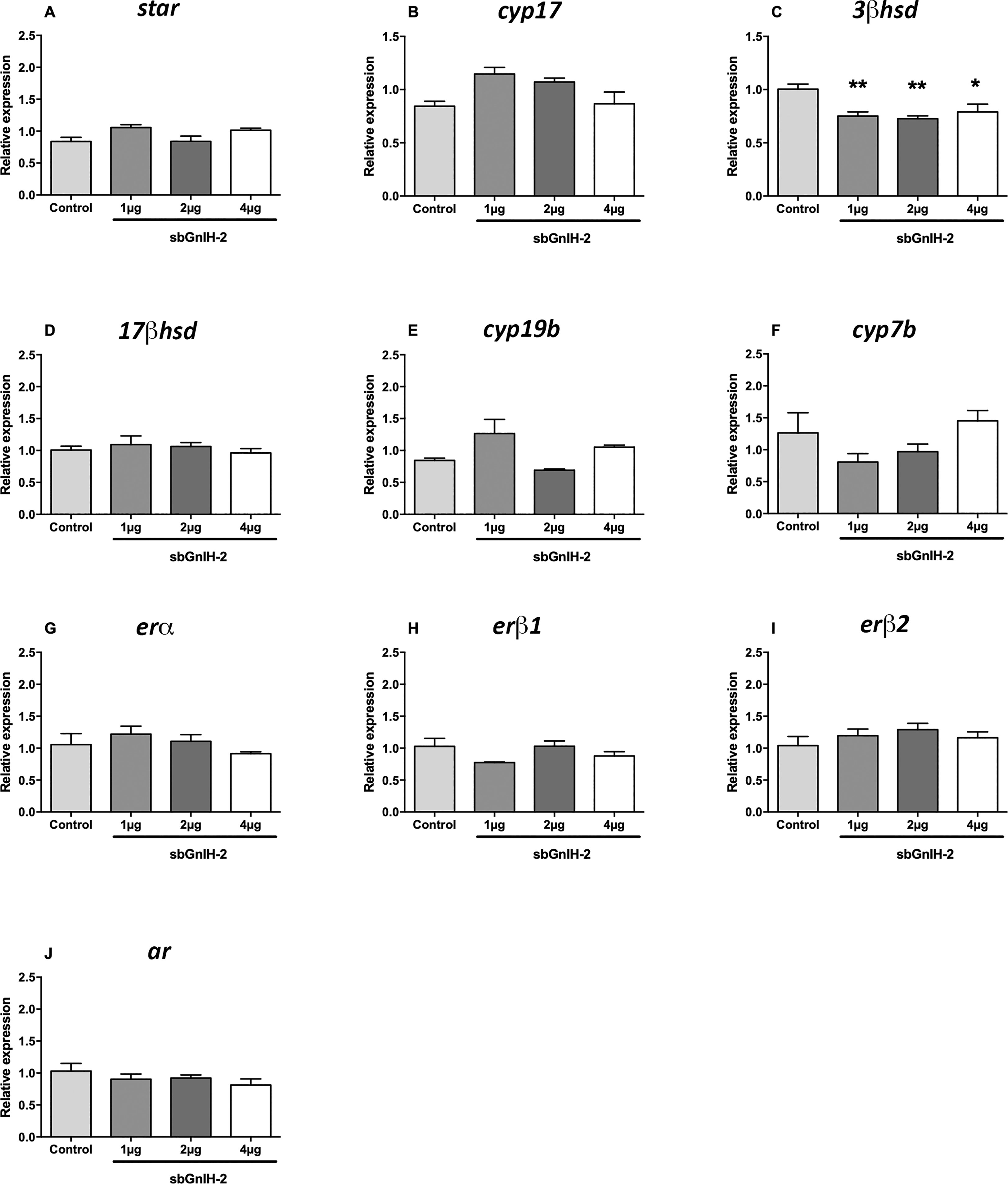
Figure 2 Effects of in vivo intracerebroventricular (icv) injection of different doses (1μg, 2μg and 4μg) of sbGnih-2 on the relative expression of neurosteroids-synthesizing enzymes and steroid receptors in the brain of male sea bass at 12 hours post-injection. (A). Steroidogenic acute regulatory protein (star); (B). Cytochrome P450 17alpha-hydroxylase/17,20-lyase (cyp17); (C). 3β-hydroxysteroid dehydrogenase (3β-hsd); (D). 17β-hydroxysteroid dehydrogenase (17β-hsd); (E). Brain cytochrome P450 aromatase (cyp19b); (F). Cytochrome P450 7α-hydroxylase (cyp7b); (G). Estrogen receptor alpha (erα); (H). Estrogen receptor beta 1 (erβ1); (I). Estrogen receptor beta 2 (erβ2). (J). Androgen receptor (ar). Results are expressed as mean ± SEM (n=5). Asterisks denote significant differences between mean values of Gnih-treated animals and controls by one-way ANOVA followed by Tukey post hoc test (*, p<0.05; **, p<0.01).
The effect of sbGnih-2 at pituitary level was less evident than in the brain. Both 17β-hsd mRNA levels (p<0.0216; Figure 3B) and erβ2 expression (p<0.0053; Figure 3F) were significantly decreased in fish treated with the lower dose of sbGnih-2 (1 μg) at 6 hpi. The expression of star, cyp17 and cyp7b enzymes was not detected in sea bass pituitary and no significant effect of sbGnih-2 was detected for the remaining neurosteroids-synthesizing enzymes and steroid receptors analyzed (Figures 3, 4).
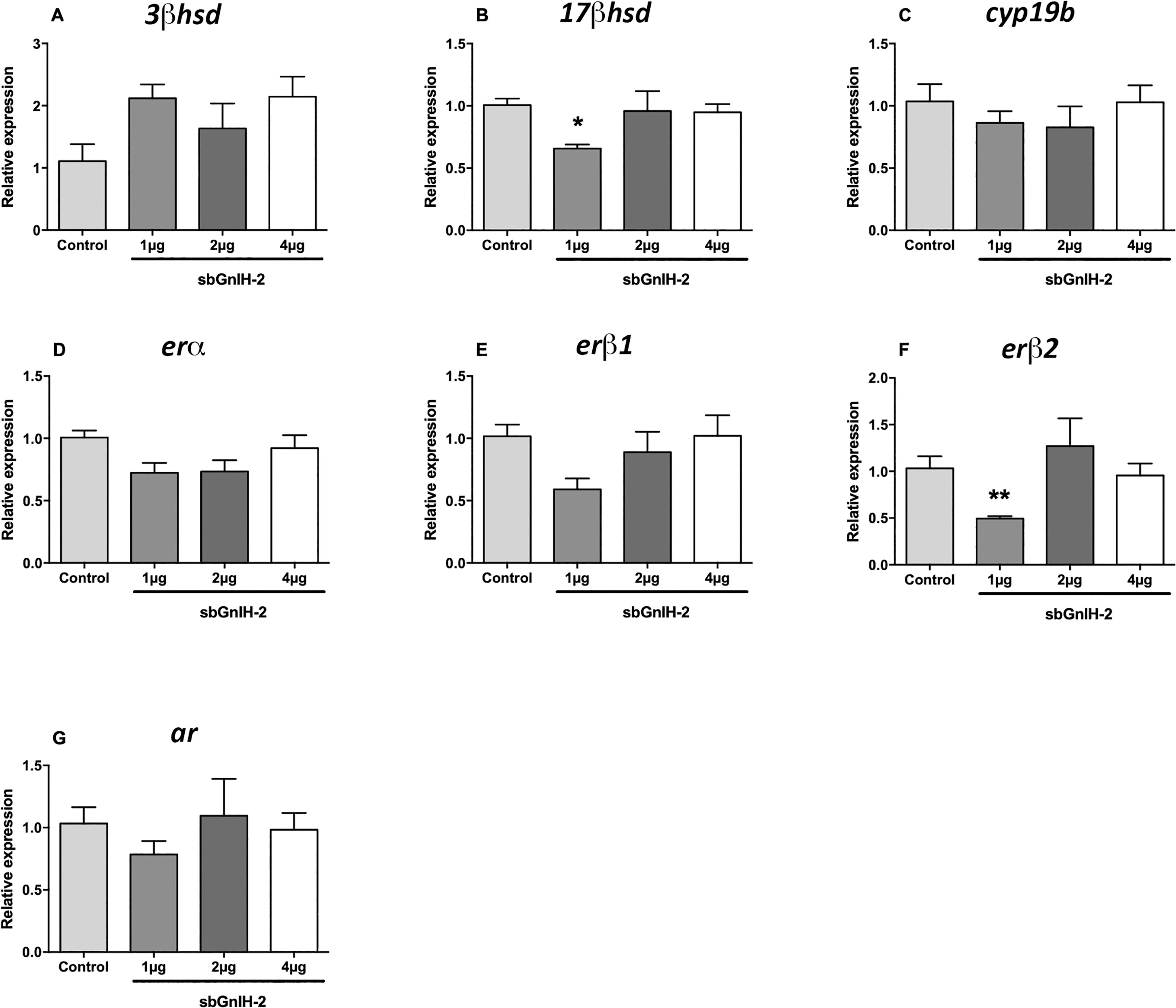
Figure 3 Effects of in vivo intracerebroventricular (icv) injection of different doses (1μg, 2μg and 4μg) of sbGnih-2 on the relative expression of neurosteroids-synthesizing enzymes and steroid receptors in the pituitary of male sea bass at 6 hours post-injection. (A). 3β-hydroxysteroid dehydrogenase (3β-hsd); (B). 17β-hydroxysteroid dehydrogenase (17β-hsd); (C). Brain cytochrome P450 aromatase (cyp19b); (D). Estrogen receptor alpha (erα); (E). Estrogen receptor beta 1 (erβ1); (F). Estrogen receptor beta 2 (erβ2). (G). Androgen receptor (ar). Results are expressed as mean ± SEM (n=5). Asterisks denote significant differences between mean values of Gnih-treated animals and controls by one-way ANOVA followed by Tukey post hoc test (*, p<0.05; **, p<0.01).
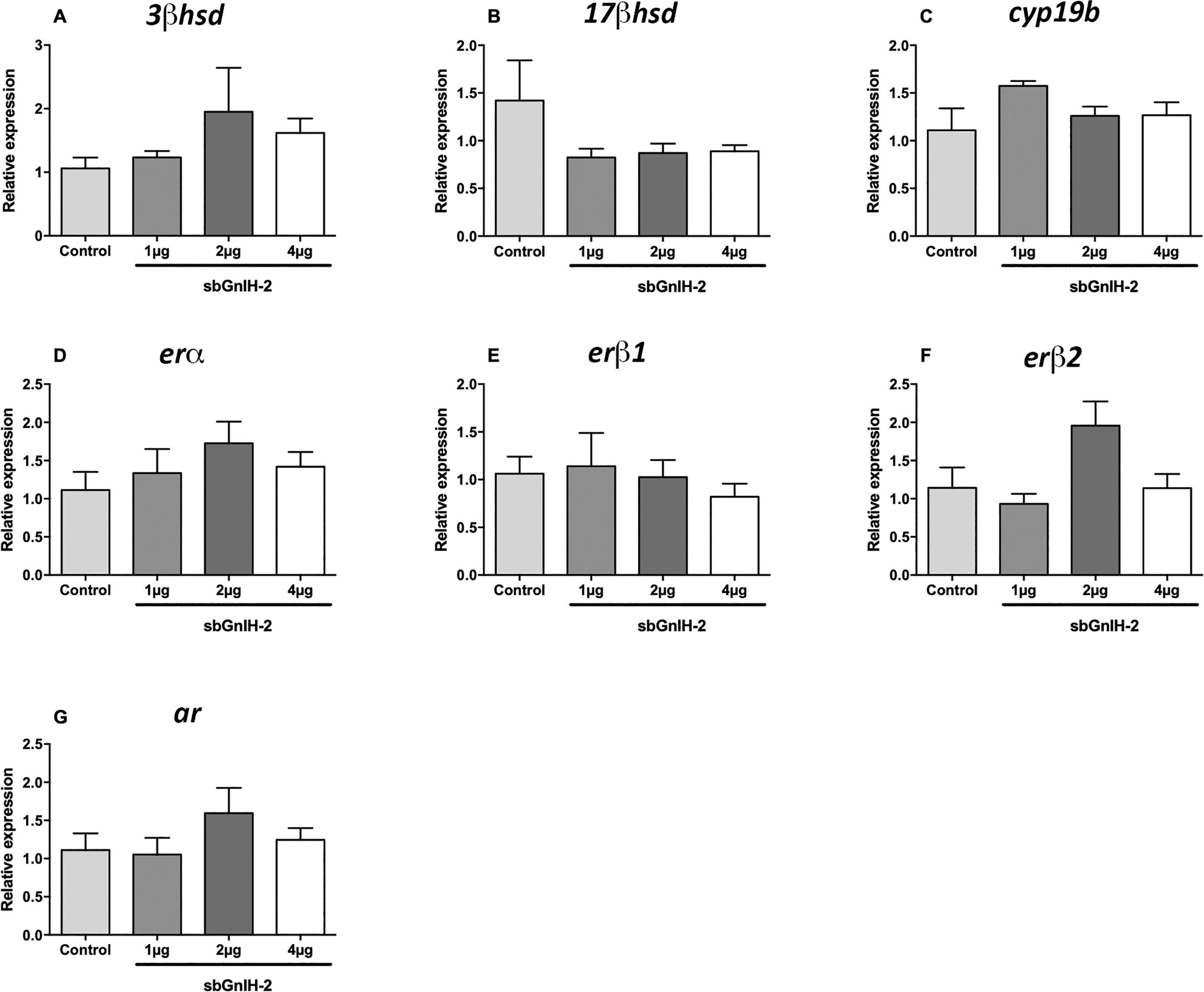
Figure 4 Effects of in vivo intracerebroventricular (icv) injection of different doses (1μg, 2μg and 4μg) of sbGnih-2 on the relative expression of neurosteroids-synthesizing enzymes and steroid receptors in the pituitary of male sea bass at 12 hours post-injection. (A). 3β-hydroxysteroid dehydrogenase (3β-hsd); (B). 17β-hydroxysteroid dehydrogenase (17β-hsd); (C). Brain cytochrome P450 aromatase (cyp19b); (D). Estrogen receptor alpha (erα); (E). Estrogen receptor beta 1 (erβ1); (F). Estrogen receptor beta 2 (erβ2). (G). Androgen receptor (ar). Results are expressed as mean ± SEM (n=5). No significant differences between mean values of Gnih-treated animals and controls were detected by one-way ANOVA (p>0.05).
Overlapping distribution of Gnih-immunoreactivity and aromatase expression in the sea bass forebrain
In addition to that reported in our previous immunohistochemical study (Paullada-Salmerón et al., 2016a), the immunohistochemical analysis using the anti-sbGnih-2 antiserum revealed the presence of immunostained cells adopting a peripheral distribution along the telencephalic, preoptic and prethalamic/thalamic ventricles (Figure 5). These Gnih-immunoreactive (ir) cells were particularly evident in the caudo-medial region of the dorsal (Dm4) and ventral (Vs, Vp) telencephalon (Figures 5A, B, E, F), the preoptic area (NPOpc, NPOav; Figures 5C, F) and prethalamus (VM; Figures 5D, G).
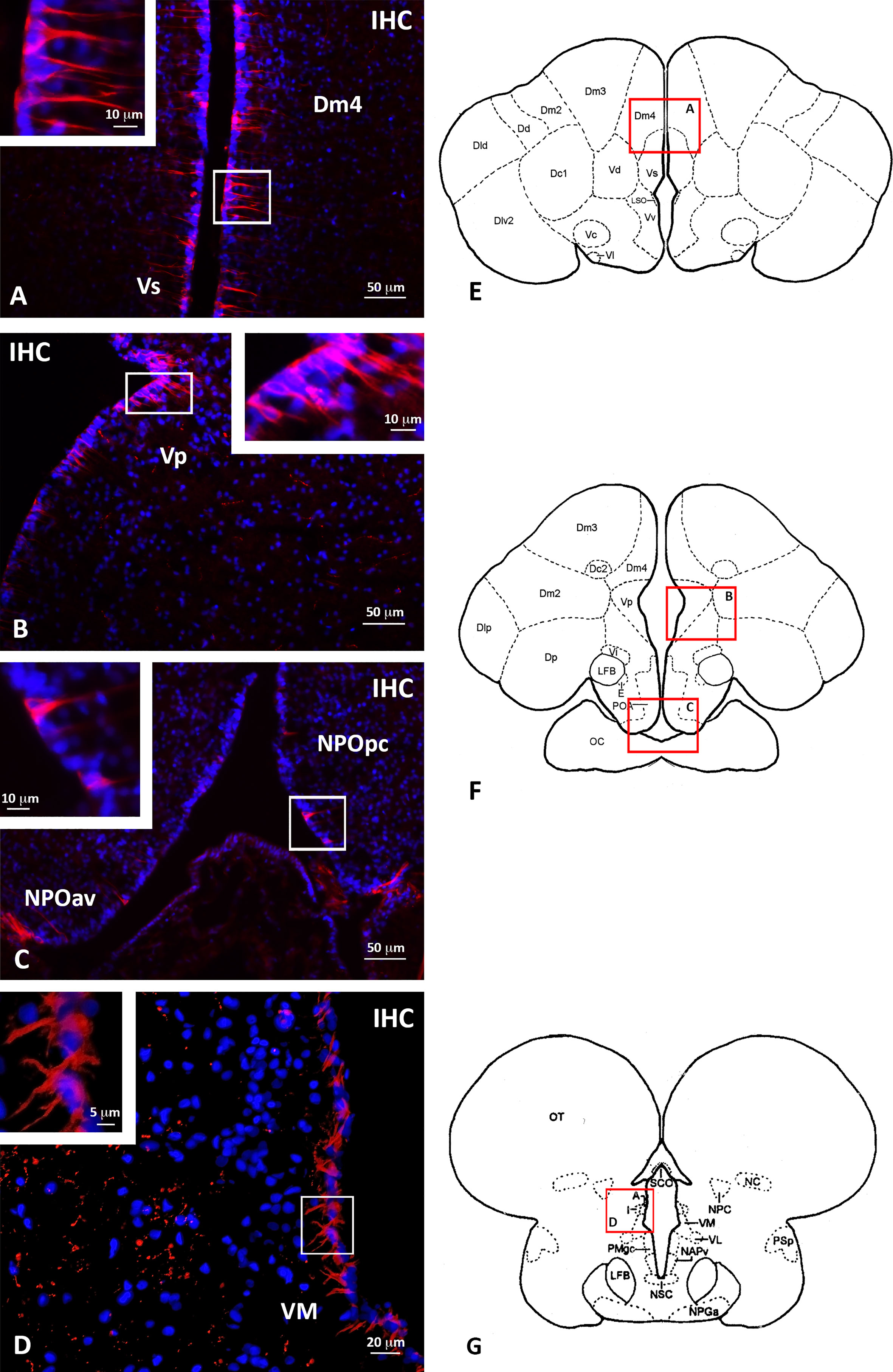
Figure 5 Localization of periventricular Gnih-immunoreactive (ir) cells in the telencephalon (A, B), preoptic area (C) and rostral prethalamus (D) of male sea bass. Schematic transverse sections through the brain of sea bass are shown in panels (E-G), indicating the areas with Gnih-ir cells magnified in panels (A-D, red rectangles). Insets in (A-D) show details of immunopositive Gnih perikarya present in white rectangles of these panels. Scale bars represent 50 μm in (A-C), 20 μm in (D), 10 μm in insets of panels (A-C), and 5 μm in inset of panel (D). Dm4, subdivision 4 of the medial part of the dorsal telencephalon; IHC, immunohistochemistry; NPOav, anteroventral part of the parvocellular preoptic nucleus; NPOpc, parvocellular part of the parvocellular preoptic nucleus; VM, ventromedial prethalamic nucleus; Vp, postcommissural nucleus of the ventral telencephalon; Vs, supracommissural nucleus of the ventral telencephalon. For further abbreviations in panels (E-G) see Cerdá-Reverter et al., 2001a; Cerdá-Reverter et al., 2001b.
Double labelling using immunohistochemistry and in situ hybridization revealed the overlapping of Gnih immunoreactivity and brain cytochrome P450 aromatase (cyp19b) expression along the forebrain ventricle (Figures 6, 7). Gnih-ir cells were mostly found lining the subventricular zone, while aromatase-positive cells were located at the edge of the brain, lining the ventricular surface (see Figures 6C, F). In the telencephalon, the close apposition of Gnih-ir cells and aromatase-expressing cells was particularly evident in the caudo-medial subdivisions of the dorsal telencephalon (Dm4, Figures 6A-C, G) and in the supracommissural (Vs, Figures 6A-C, G) and postcommissural (Vp, Figures 6D-F, H) nuclei of the ventral telencephalon. In the prethalamus, Gnih-ir cells were also in close proximity to aromatase-expressing cells along the ventricular surface of the ventromedial nucleus (VM, Figures 7A-D, G, black arrowheads in 7D). Moreover, Gnih-ir fiber terminals were evident in the vicinity of some aromatase-positive cells located in the most internal part of VM (Figure 7D, white arrowheads). No labelling was observed with sense riboprobes (Figure 7E) or when the primary sbGnih-2 antiserum was preabsorbed with the synthetic sbGnih-2 peptide (Figure 7F).
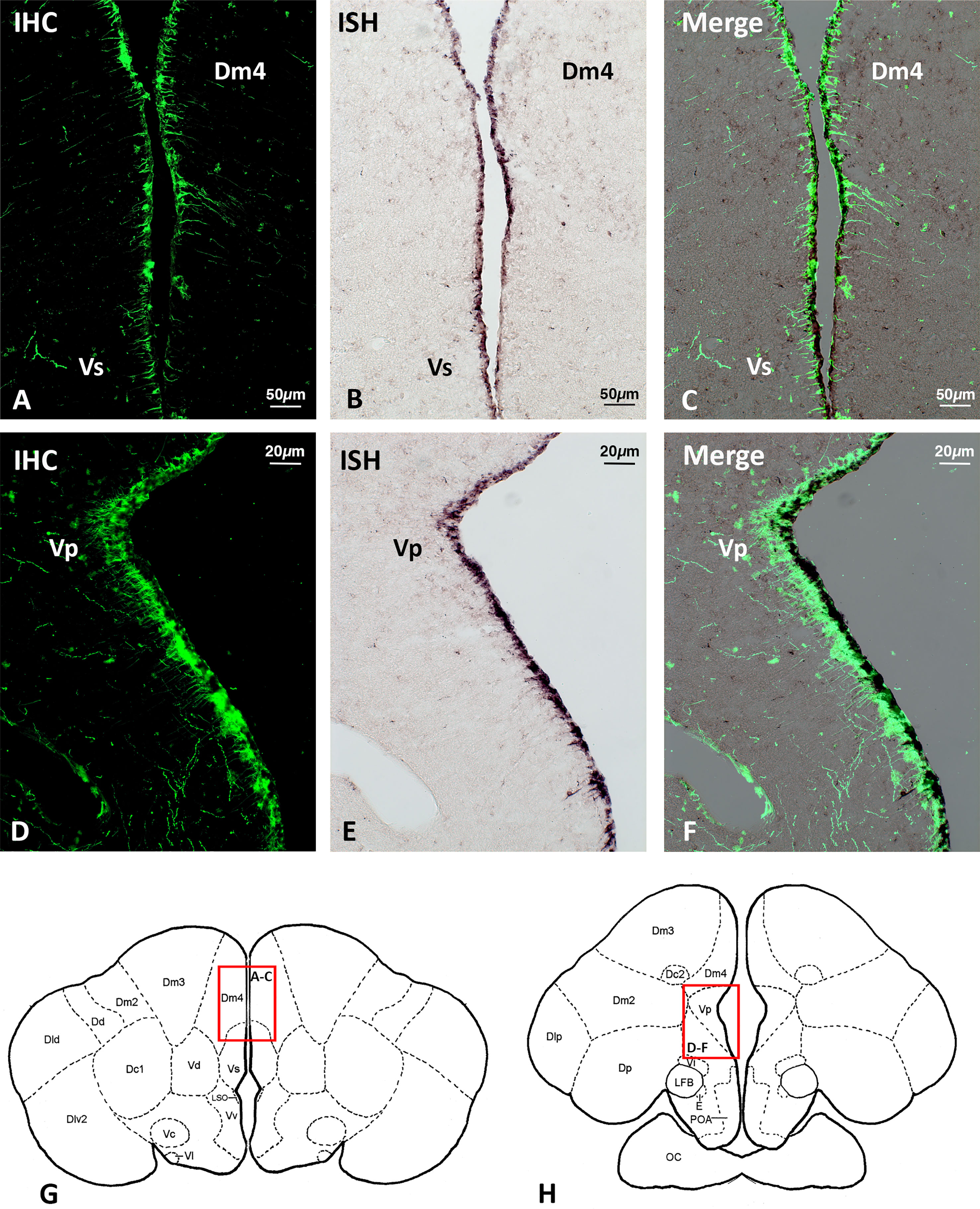
Figure 6 Localization of Gnih-immunoreactive (ir) cells (A, D) and brain cytochrome P450 aromatase (cyp19b)-expressing cells (B, E) in the periventricular telencephalon of male sea bass. Merge sections photomicrographs are provided in panels (C, F). Schematic transverse sections through the brain of sea bass are shown in panels (G, H), indicating the areas with Gnih-ir cells and aromatase-positive cells magnified in panels (A-C, G) and (D-F, H) (red rectangles). Note the overlapping distribution of subventricular Gnih-ir cells and ventricular aromatase-expressing cells along the telencephalic ventricle. Scale bars represent 50 μm in (A-C), and 20 μm in (D-F). Dm4, subdivision 4 of the medial part of the dorsal telencephalon; IHC, immunohistochemistry; ISH, in situ hybridization; Vp, postcommissural nucleus of the ventral telencephalon; Vs, supracommissural nucleus of the ventral telencephalon. For further abbreviations in panels (E-G) see Cerdá-Reverter et al., 2001a.
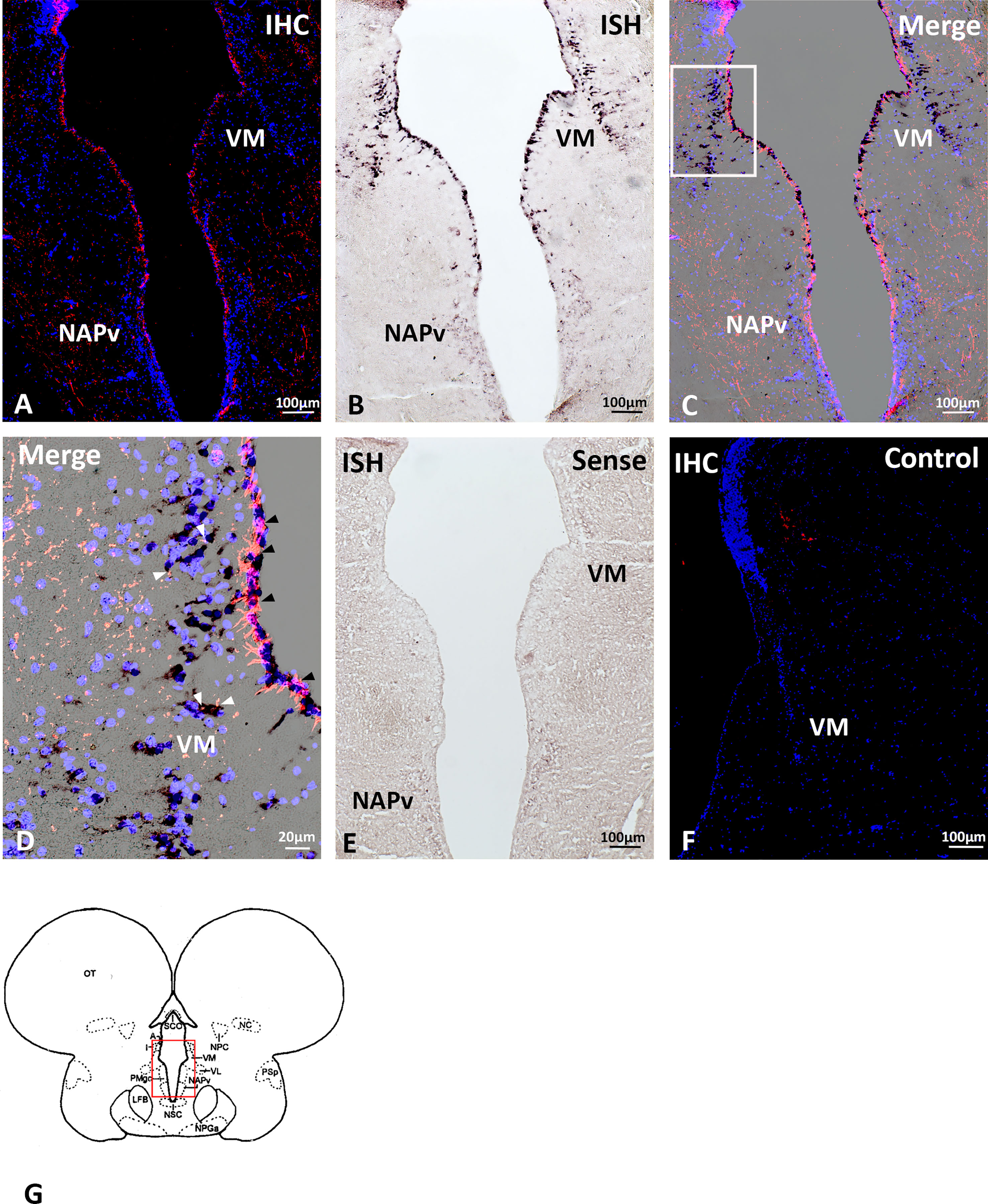
Figure 7 Localization of periventricular Gnih-immunoreactive (ir) cells (A) and brain cytochrome P450 aromatase (cyp19b)-expressing cells (B) in the prethalamus and caudal preoptic area of male sea bass. Merge sections photomicrographs are provided in panels (C, D). Photomicrograph in panel (D) shows a detail of immunopositive Gnih cells and fibers and aromatase-expressing perikarya present in white rectangle of panel (C). Note the close apposition of Gnih immunoreactivity and aromatase-positive labelling in perikarya along the periventricular zone of the ventral prethalamic nucleus (black arrowheads). In turn, more internal aromatase-expressing cells of this nucleus appear innervated by immunopositive Gnih axon terminals (white arrowheads). Photomicrograph of panels (E, F) represent control sections of in situ hybridization and immunohistochemistry, respectively. Schematic transverse section through the brain of sea bass is shown in panel (G), indicating the area with Gnih-ir cells and aromatase expressing cells magnified in panels (A-D, red rectangle). Scale bars represent 100 μm in (A-C, E, F), and 20 μm in (D). IHC, immunohistochemistry; ISH, in situ hybridization; NAPv, anterior periventricular nucleus; VM, ventromedial prethalamic nucleus. For further abbreviations in panels (G), see Cerdá-Reverter et al., 2001b.
Gnih effects on agonistic/aggressive behavior and locomotor activity
We also tested the effect of central administration of sbGnih-2 on agonistic/aggressive behavior and locomotor activity of male sea bass (Figure 8, Supplementary Material). Icv injection of sbGnih-2 reduced the number of contacts with the mirror and the time spent in the mirror zone (p<0.0016 and p<0.001, respectively, Figures 8A, B). These results were consistent with the movement heat map analysis of control and sbGnih-2 treated animals (Figures 8I, J). In turn, sbGnih-2 administered fish showed an increase in time spent to establish the first contact with the mirror and in the mean distance to the mirror zone (p<0.0032 and p<0.003, respectively, Figures 8C, D). Concerning the locomotor activity, we did not observe any significant effect of sbGnih-2 on distance moved, maximal velocity of swimming, maximal acceleration nor mean distance between subjects (Figures 8E–H).
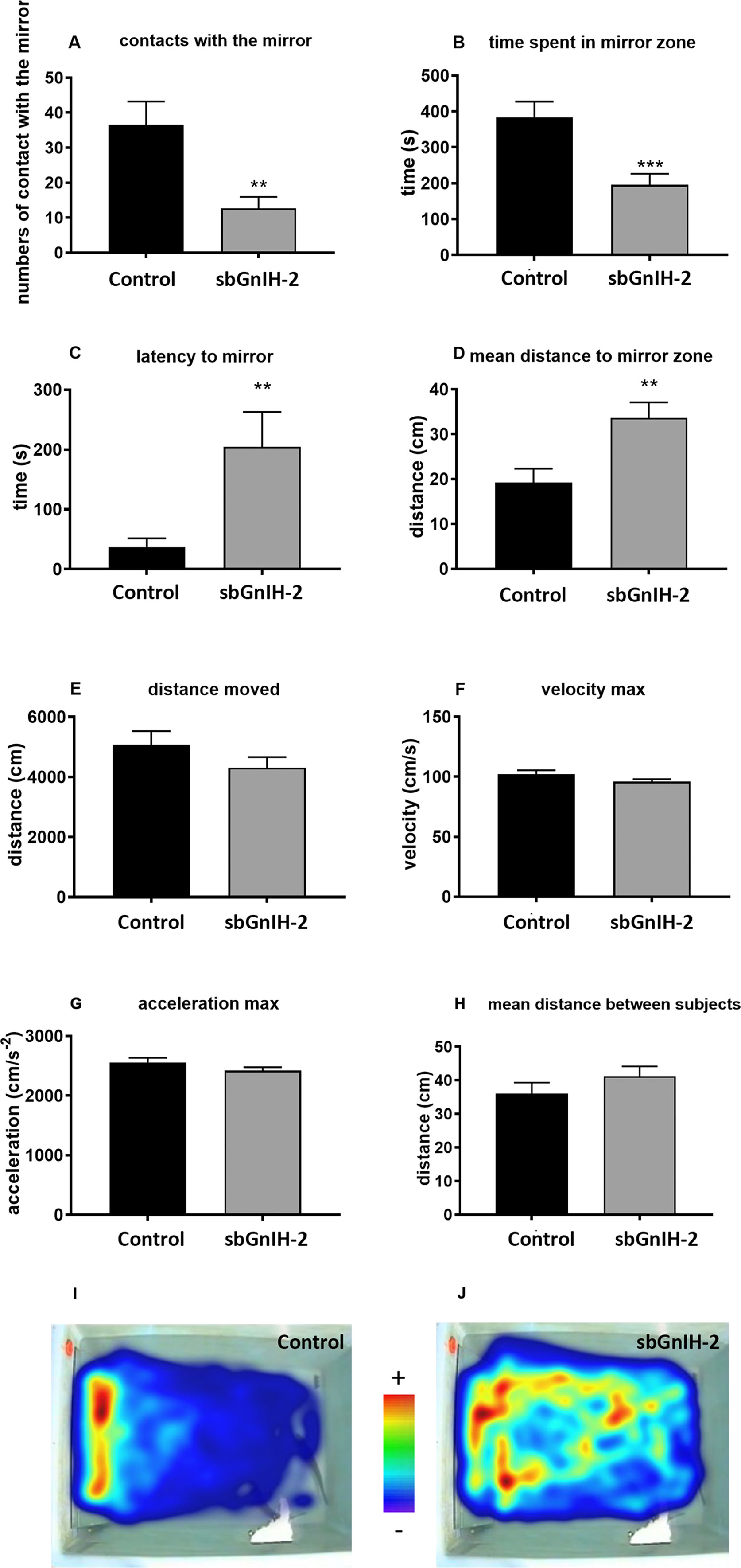
Figure 8 Effects of in vivo intracerebroventricular (icv) injection of sbGnih-2 (dose 2μg) on agonistic behavior and locomotor activity parameters of male sea bass as revealed by the mirror test. (A). Contacts with the mirror; (B). Time spent in the mirror zone; (C). Latency to the first contact with the mirror; (D). Mean distance to the mirror zone; (E). Distance moved during the test; (F). Maximal velocity measured during the test; (G). Maximal acceleration measured during the test; (H). Mean distance between subjects. (I). Movement heat map of control animals. (J). Movement heat map of sbGnih-2-treated animals. Results are expressed as mean ± SEM of 15 couples per condition (n=30). Asterisks denote significant differences between mean values of Gnih-treated animals and controls by T-Student test (**, p<0.01; ***, p<0.001).
Discussion
Although the regulation of neurosteroid biosynthesis by neuropeptides and neurotransmitters has been extensively reported in tetrapod vertebrates (Do Rego et al., 2009; Ubuka and Tsutsui, 2022), studies in fish have mainly focused on the effects of temperature, social interactions and steroid hormones on aromatase activity and/or expression (Menuet et al., 2005; Piferrer and Blázquez, 2005; Diotel et al., 2010; Coumailleau et al., 2015). Gonadotropin-inhibitory hormone (GNIH/Gnih) is well known for playing a crucial role in the regulation of reproduction by acting on the brain and pituitary hormone synthesis and secretion (Kriegsfeld et al., 2006; Ubuka et al., 2014; Choi et al., 2016; Di Yorio et al., 2016; Ogawa et al., 2016; Paullada-Salmerón et al., 2016b; Aliaga-Guerrero et al., 2018). In light of the studies performed, this neuropeptide has also emerged as a key factor in regulating steroid hormone production both in the brain and gonads (Bentley et al., 2006; Piekarski et al., 2013; Paullada-Salmerón et al., 2016c; Ubuka and Tsutsui, 2022). Particularly, GNIH may act as a regulator of neuroestrogen synthesis in birds and mammals, suggesting a role of this neurohormone in the control of social-sexual behaviors in these groups of vertebrates (Ubuka et al., 2014; Abdel-Aleem et al., 2019; Ubuka and Tsutsui, 2022). Despite remarkable advances in the knowledge of fish Gnih system in recent years, the role of this neuropeptide in the regulation of neurosteroid biosynthesis and its relationships in mediating social/aggressive behavior has not been addressed in fish to date. The current study revealed that intracerebroventricular administration of sbGnih-2 affected the transcript levels of some of the main enzymes involved in neurosteroids biosynthesis (3β-hsd, 17β-hsd, cyp19b), but also evoked changes in the expression levels of estrogen receptors (erβ2) at the brain and/or pituitary level. The present study also provides the first evidence of the inhibitory effect of Gnih on aggressive behavior in a teleost fish.
In the present study, we showed that central administration of sbGnih-2 peptide increased the transcripts levels of cyp19b (brain aromatase) in the brain of sea bass. Brain aromatase is responsible for converting androstenedione in estrone and T in E2 (Figure 9). Therefore, this Gnih-induced increase in the expression of brain aromatase might lead to an elevation in the synthesis of neuroestrogens in the sea bass brain. In quail, GNIH stimulated in a dose-dependent manner the aromatase activity and increased E2 concentration in the preoptic area (POA), which was inhibited if GNIH was co-administered with the GNIH receptor antagonist, RF9 or the aromatase inhibitor, fadrozole (Ubuka et al., 2014). Similar results have been obtained in mice, in which RFRP-1 stimulates in vitro aromatase activity in the POA (Ubuka and Tsutsui, 2022). It has been suggested that regulation of neuroestrogen synthesis by GNIH could be mediated by a decrease in the phosphorylation of the aromatase protein and the subsequent increase in its enzymatic activity (Balthazart et al., 2006; Ubuka et al., 2014). Therefore, the stimulatory actions of GNIH/Gnih on brain aromatase synthesis and/or activity appear to represent a conserved feature of vertebrate neurosteroidogenesis.
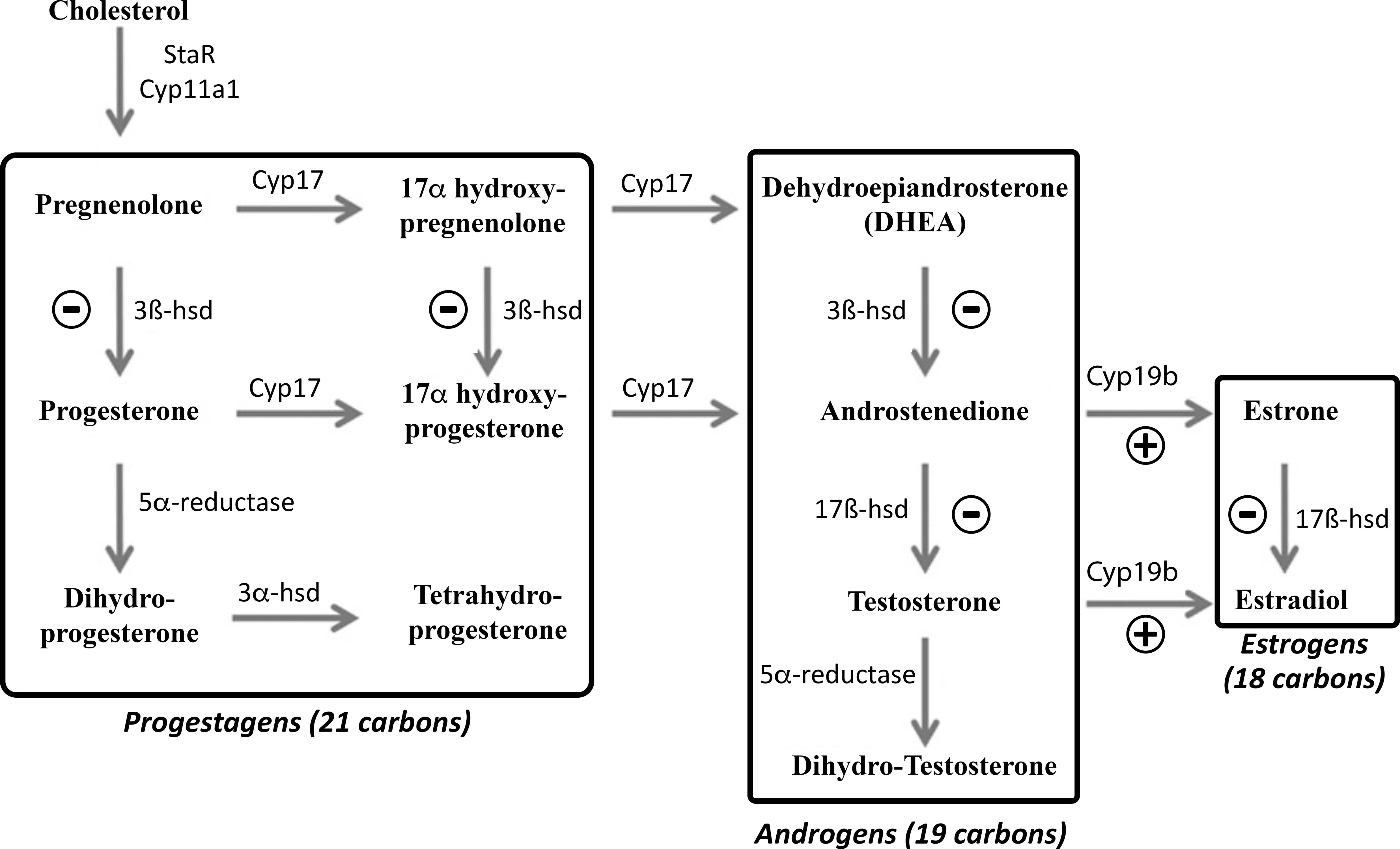
Figure 9 Pathways of neurosteroids biosynthesis in the fish brain (modified from Diotel et al., 2011a). 3α-hsd, 3α-hydroxysteroid dehydrogenase; 3β-hsd, 3β-hydroxysteroid dehydrogenase; 17β-hsd, 17β-hydroxysteroid dehydrogenase. Cyp11a1, Cytochrome P450 cholesterol side-chain cleavage enzyme; Cyp17, Cytochrome P450 17α-hydroxylase/C17, 20-lyase; Cyp19b, brain cytochrome P450 aromatase; StAR, Steroidogenic acute regulatory protein. The symbols ⊖ and ⊕ indicate inhibitory and stimulatory actions of sbGnih-2 on the transcript levels of the neurosteroid-synthesizing enzymes, respectively, as revealed in this study.
In fish, brain aromatase-expressing cells were detected mainly in the ventricular forebrain regions including the peripheral layer of the dorsal telencephalon, ventral telencephalon, preoptic area, prethalamus, thalamus, hypothalamus, and in the cerebellum (Menuet et al., 2003; Strobl-Mazzulla et al., 2005; Pellegrini et al., 2007; Okubo et al., 2011; Diotel et al., 2011a; Ramallo et al., 2017). In the sea bass, we found a similar distribution of aromatase-expressing cells in the periventricular layers of the anterior brain and particularly in the caudo-medial subdivisions of the dorsal and ventral telencephalon, preoptic area, prethalamus, thalamus and hypothalamus. In contrast to what is reported in birds and mammals, in which aromatase expression is mainly confined to neurons, brain aromatase is massively expressed in periventricular radial glial progenitor cells of teleost brain (Diotel et al., 2011a; Coumailleau et al., 2015). Considering the distribution and appearance of aromatase-expressing cells detected in the present study by in situ hybridization, this seems to be true also in the sea bass brain, although some aromatase-positive cells, which adopt a more internal position in the prethalamic ventromedial nucleus, could correspond to neurons. Nevertheless, it has been shown that aromatase mRNA can be transported internally along radial processes in fish (Diotel et al., 2011b). In a previous study in the European sea bass, Gnih-ir fibers were detected in the periventricular preoptic area, but also in the dorsal and ventral telencephalon, prethalamus, thalamus and hypothalamus (Paullada-Salmerón et al., 2016a). Thus, a direct action of Gnih on aromatase-expressing cells could be envisaged in this species, as it was suggested in male quail, in which GNIH-ir neuronal fibers reach aromatase-ir cells present in the POA, which also expressed GNIH receptor mRNA (Ubuka et al., 2014). In fact, in the present study closely apposed Gnih-ir and aromatase-expressing cell bodies were detected along the periventricular surface of the dorsal (Dm4) and ventral (Vs, Vp) telencephalon and prethalamus (VM) of sea bass, whereas some aromatase-positive cells lying more internally in the VM were innervated by Gnih-ir fibers. These forebrain regions are also known for their high concentrations of estrogen receptor (ER) mRNAs or protein in sea bass and other teleost fish (Pellegrini et al., 2005; Strobl-Mazzulla et al., 2008; Muriach et al., 2008a; Muriach et al., 2008b). Further morphofunctional studies seem necessary to characterize the presence of Gnih receptors in aromatase-expressing cells in fish.
Our research showed that treatments with sbGnih-2 resulted in a decrease in 3β-hsd expression mRNA levels in the sea bass brain. The 3β-HSD protein is an important steroidogenic enzyme that is essential for the synthesis of progesterone from pregnenolone and 17α-hydroxyprogesterone from 17α-hydroxypregnenolone, but also converts dehydroepiandrosterone (DHEA) to androstenedione, which is, in turn, a relevant precursor for the production of testosterone (Figure 9; Sakamoto et al., 2001; Schlinger et al., 2008; Diotel et al., 2011a). Studies developed in birds have reported that 3β-HSD, working in concert with aromatase, could be involved in neurobehavioral functions through its actions on DHEA for the synthesis of estrogens (Schlinger et al., 2008; Pradhan et al., 2010). In zebrafish, 3β-hsd mRNA expression and/or protein activity were characterized in numerous brain regions including the olfactory bulbs, ventral and dorsomedial telencephalon, preoptic area, hypothalamus, optic tectum, cerebellum and lateral recess, demonstrating that the teleost brain can synthesize neurosteroids (Sakamoto et al., 2001; Diotel et al., 2011a; Diotel et al., 2011b). It should be noted that sea bass Gnih innervation reaches the same neural areas where 3β-hsd steroidogenic enzyme-expressing cells have been reported in the fish brain (Paullada-Salmerón et al., 2016a), which could suggest the existence of putative interactions of Gnih system with 3β-Hsd in the brain of sea bass. Further studies should be directed to identifying 3β-hsd-expressing cells in the brain of sea bass and its tentative colocalization with Gnih cells and projections.
On the other hand, our study also evidenced a significant reduction of 17β-hsd transcript levels in the brain and pituitary of sea bass after sbGnih-2 administration. Together with 3β-HSD, the 17β-HSD enzyme is also essential for androgen biosynthesis, by promoting the conversion of androstenedione to T, but also converts estrone into E2 in the brain of mammalian and non-mammalian vertebrates (Figure 9; Steckelbroeck et al., 1999; Mindnich et al., 2004, Hojo et al., 2004, Diotel et al., 2011a). Previous studies in fish have demonstrated that androgens arising from gonads, such as T and/or 11-KT, are involved in the regulation of aggressive and sexual behavior, possibly through their actions on brain areas related to motivational or motor pattern regulation (Remage-Healey and Bass, 2006; Remage-Healey and Bass, 2007; Lord et al., 2009; Chang et al., 2012). It is not known whether the effects of Gnih on aggressive behavior observed in the present study are mediated by peripheral E2 or, alternatively, by peripheral T converted locally to E2 by Cyp19, which could act on androgen and estrogen receptors present in the sea bass brain. However, reproductive but not aggressive behavior is affected in castrated tilapia, suggesting the involvement of independent central mechanisms in aggressive behavior (Almeida et al., 2014), which could be, at least in part, due to local production of androgens from DHEA into the fish brain (Soma et al., 2008; Wingfield et al., 2018). It is plausible to think that DHEA may also be converted to active neuroandrogens in sea bass brain through the enzymatic activities of 3β-Hsd and 17β-Hsd and exert an influence over aggressive behavior. Therefore, Gnih might be involved in the neuroandrogen-mediated regulation of different behavioral traits by inhibiting 3β-hsd/17β-hsd expression in sea bass brain. The concomitant Gnih-induced inhibition of 3β-hsd and 17β-hsd gene transcription without a reduction on cyp17 and cyp7b transcript levels could determine the accumulation of DHEA. Whether alternative pathways from DHEA leading to the production of DHEA sulphate and/or 7α-hydroxy DHEA also operate in the fish brain, as it has been described in mammals (Maninger et al., 2009), needs further investigation.
Our work also showed that central administration of sbGnih-2 elicited a decrease of 17β-hsd and erβ2 expression at the pituitary level. The pituitary gland contains a high density of estrogen and androgen receptors in fish, including sea bass (Piferrer and Blázquez, 2005; Harbott et al., 2007; Strobl-Mazzulla et al., 2008; Muriach et al., 2008b), and neurosteroids-synthesizing enzymes such as brain aromatase (Cyp19b) have also been detected in the pituitary of rainbow trout (Menuet et al., 2003), zebrafish (Goto-Kazeto et al., 2004), pejerrey (Strobl-Mazzulla et al., 2005), sea bass (Alvarado et al., 2016) and the weakly electric fish, Apteronotus leptorhynchus (Shaw and Krahe, 2018). As 17β-Hsd transforms androstenedione in T and estrone in E2 (Figure 9), Gnih actions on 17β-hsd expression could reduce T and E2 availability in the pituitary gland. In a previous study, we reported the presence of Gnih-ir fibers reaching the proximal pars distalis (PPD) of the sea bass pituitary gland (Paullada-Salmerón et al., 2016a). Moreover, we showed that peripherally-administered Gnih decreased T and 11-KT plasma levels at early- and mid-spermatogenesis stages, affecting the testicular gametogenesis of sea bass (Paullada-Salmerón et al., 2016c). Interestingly, a study performed in sea bass showed that castration also reduced neurosteroid-synthesizing enzymes (cyp19b) and estrogen receptor (erα) expression in the pituitary while T replacement after gonadectomy stimulated its expression to a level that was comparable to that of the control fish (Alvarado et al., 2016). Whether the Gnih actions on the pituitary reported in the present study represent direct or indirect (through gonadal feedback) effects should be elucidated. In any case, our results suggest that Gnih might be regulating the synthesis and actions of steroids not only at the brain level but also in the pituitary.
Finally, we have shown early inhibitory actions of sbGnih-2 in agonistic/aggressive behavior of sea bass using the mirror test (decreased interaction with the mirror, lower time spent in the mirror zone, increased latency to establish contact with the mirror and greater mean distance to the mirror zone) without affecting the locomotor activity (no difference in distance moved, maximum velocity and acceleration and mean distance between subjects). The short-term effects of Gnih in agonistic/aggressive behavior (15 to 45 min after Gnih administration) could be due to rapid actions on brain aromatase activity, as it has been previously reported in birds (Ubuka et al., 2014), while the subsequent effects on the transcription of 3β-hsd, 17β-hsd and cyp19b genes (6- or 12-hours post administration) could sustain long-lasting actions of Gnih on brain neurosteroids repertoire. Several studies have reported a possible effect of the social environment on aromatase mRNA expression and/or activity within the brain of teleosts and there is strong evidence that brain aromatase is involved in the regulation of socio-sexual and aggressive behavior in vertebrates, including fish (Black et al., 2005; Black et al., 2011; Iwata et al., 2012; Huffman et al., 2013; Ubuka and Tsutsui, 2014; Ubuka et al., 2014; Ramallo et al., 2017; Quintana et al., 2021). In the weakly electric banded knifefish (Gymnotus omarorum), brain aromatase is upregulated in dominant but not subordinate fish (Quintana et al., 2021). Treatment with fadrozole (an aromatase inhibitor) in dominant males of the African cichlid fish Astatotilapia burtoni, increased aromatase expression in the preoptic area and decreased aggressive but not reproductive behavior (Huffman et al., 2013). Moreover, high aromatase mRNA expression was found in the magnocellular and gigantocellular regions of the preoptic area of subordinate male of the African cichlid fish compared to dominant fish (Huffman et al., 2013). In turn, in the simultaneous hermaphroditic bluebanded goby Lythrypnus dalli, a low brain aromatase activity is related to increased aggression (Black et al., 2005). As mentioned above, GNIH seems to represent a key factor in the regulation of social and reproductive behaviors in birds and mammals (Kriegsfeld et al., 2006; Ubuka et al., 2014; Abdel-Aleem et al., 2019). Thus, as reported in sea bass in the current study, icv injection of GNIH suppressed the male aggressive behavior in male quail as quickly as 30 min after its administration (Ubuka et al., 2014). As mentioned above, centrally administered GNIH also activates short term aromatase activity (probably by dephosphorylation of phosphorylated aromatase) and increases neuroestrogen synthesis in the POA and the administration of GNIH antagonist RF9 inhibited the action of GNIH on aromatase and neuroestrogen synthesis in the brain of quail. Furthermore, RNA interference of GNIH gene decreased diencephalic GNIH mRNA expression and concentration, as well as the density of GNIH-immunoreactive fibers in the ventral tegmental area, increasing the locomotor activity and aggressive-sexual behavior, which suggests that GNIH may play a role in the social interactions controlling these behavioral responses in birds (Ubuka et al., 2013; Ubuka et al., 2014).
In conclusion, our molecular results provide the first evidence of the Gnih actions on the gene expression of neurosteroid-synthesizing enzymes (3β-hsd, 17β-hsd and cyp19b) in a fish species, the European sea bass, which could inhibit the production of aromatizable neuroandrogens (i.e., androstenedione and testosterone) and promote their conversion into neuroestrogens (i.e., estrone and estradiol). In turn, neuroanatomical data suggest that paracrine but also neuroendocrine actions could mediate the Gnih effects on brain aromatase expression in this species. The elucidation of the mechanisms underlying the short-term behavioral effects of Gnih, the subsequent actions of this neuropeptide on the gene expression of neurosteroidogenic enzymes, and whether Gnih actions on the transcription of neurosteroid-synthesizing genes and/or neurosteroids levels are also involved in other processes (e.g., neurogenesis, sex differentiation, puberty and/or seasonal reproduction) in sea bass will require further investigation in future studies.
Data availability statement
The original contributions presented in the study are included in the article/Supplementary Materials, further inquiries can be directed to the corresponding author/s.
Ethics statement
The animal study was reviewed and approved by Ethics Committee of the University of Cádiz (Spain), Ethics Committee of the Oceanological Observatory of Banyuls-sur-Mer (France), and the General Directorate for Agricultural and Livestock Production of the Regional Government of Andalusia (approval number 15/12/2020/143 and 15/12/2020/144).
Author contributions
JAM-C contributed to the conception of the study, with the help of TU and ELM. JAP-S and GHL performed the icv Gnih injections and gene expression analysis. JAP-S performed the immunohistochemical and in situ hybridization analyses. GHL, MF and LB planned and performed the behavioral study. JAP-S and JAM-C wrote the manuscript. All authors contributed to the article and approved the submitted version.
Funding
Funded by grants from PAIDI2020-Junta de Andalucía (Grant no P18-RT-5152) and FEDER-UCA (Grant no 18‐107538) to JAM-C, and a grant from the European Union (FP7-INFRASTRUCTURES-2010-1, Grant no 262280. Ref. OOB–EMBRC-FR–AAP2018–no 2200) to GL, LB and JAM-C.
Acknowledgments
We would like to thank to staff from the “Planta de Cultivos Marinos” (University of Cádiz) and from the Mutualized Aquariology Service of the Oceanological Observatory of Banyuls-sur-Mer (Sorbonne Université, Banyuls-sur-Mer, France) for their assistance in the maintaining and care of experimental animals used in this study. We thank Dr. Olivier Kah for his critical reading of the manuscript and useful comments.
Conflict of interest
Author TU is employed by International Cancer Laboratory Co., Ltd.
The remaining authors declare that the research was conducted in the absence of any commercial or financial relationships that could be construed as a potential conflict of interest.
Publisher’s note
All claims expressed in this article are solely those of the authors and do not necessarily represent those of their affiliated organizations, or those of the publisher, the editors and the reviewers. Any product that may be evaluated in this article, or claim that may be made by its manufacturer, is not guaranteed or endorsed by the publisher.
Supplementary material
The Supplementary Material for this article can be found online at: https://www.frontiersin.org/articles/10.3389/fmars.2023.1185652/full#supplementary-material
Supplementary material | Behavioral video recording of control and sbGnih-2 intracerebroventricularly-injected male sea bass.
References
Abdel-Aleem G. A., Shafik N. M., El-Magd M. A., Mohamed D. A. (2019). Soya bean rich diet is associated with adult male rat aggressive behavior: relation to RF amide-related peptide 3-aromatase-neuroestrogen pathway in the brain. Metab. Brain. Dis. 34, 1103–1115. doi: 10.1007/s11011-019-00431-2
Aliaga-Guerrero M., Paullada-Salmerón J. A., Piquer V., Mañanós E. L., Muñoz- Cueto J. A. (2018). Gonadotropin-inhibitory hormone in the flatfish, Solea senegalensis: molecular cloning, brain localization and physiological effects. J. Comp. Neurol. 526, 349–370. doi: 10.1002/cne.24339
Almeida O., Canário A. V., Oliveira R. F. (2014). Castration affects reproductive but not aggressive behavior in a cichlid fish. Gen. Comp. Endocrinol. 207, 34–40. doi: 10.1016/j.ygcen.2014.03.018
Alvarado M. V., Servili A., Molés G., Gueguen M. M., Carrillo M., Kah O., et al. (2016). Actions of sex steroids on kisspeptin expression and other reproduction-related genes in the brain of the teleost fish European sea bass. J. Exp. Biol. 219, 3353–3365. doi: 10.1242/jeb.137364
Azcoitia I., Arevalo M. A., Garcia-Segura L. M. (2018). Neural-derived estradiol regulates brain plasticity. J. Chem. Neuroanat. 89, 53–59. doi: 10.1016/j.jchemneu.2017.04.004
Balthazart J., Baillien M., Ball G. F. (2006). Rapid control of brain aromatase activity by glutamatergic inputs. Endocrinology 147, 359–366. doi: 10.1210/en.2005-0845
Belelli D., Herd M. B., Mitchell E. A., Peden D. R., Vardy A. W., Gentet L., et al. (2006). Neuroactive steroids and inhibitory neurotransmission: mechanisms of action and physiological relevance. Neuroscience 138, 821–829. doi: 10.1016/j.neuroscience.2005.07.021
Bentley G., Jensen J., Kaur G., Wacker D., Tsutsui K., Wingfield J. (2006). Rapid inhibition of female sexual behavior by gonadotropin-inhibitory hormone (GnIH). Horm. Behav. 49, 550–555. doi: 10.1016/j.yhbeh.2005.12.005
Bentley G. E., Perfito N., Ukena K., Tsutsui K., Wingfield J. C. (2003). Gonadotropin-inhibitory peptide in song sparrows (Melospiza melodia) in different reproductive conditions, and in house sparrows (Passer domesticus) relative to chicken- gonadotropin-releasing hormone. J. Neuroendocrinol. 15, 794–802. doi: 10.1046/j.1365-2826.2003.01062.x
Biswas S., Jadhao A. G., Pinelli C., Palande N. V., Tsutsui K. (2015). GnIH and GnRH expressions in the central nervous system and pituitary of Indian major carp, Labeo rohita during ontogeny: an immunocytochemical study. Gen. Comp. Endocrinol. 220, 88–92. doi: 10.1016/j.ygcen.2014.06.005
Black M. P., Balthazart J., Baillien M., Grober M. S. (2005). Socially induced and rapid increases in aggression are inversely related to brain aromatase activity in a sex-changing fish, Lythrypnus dalli. Proc. Biol. Sci 272, 2435–2440. doi: 10.1098/rspb.2005.3210
Black M. P., Balthazart J., Baillien M., Grober M. S. (2011). Rapid increase in aggressive behavior precedes the decrease in brain aromatase activity during socially mediated sex change in Lythrypnus dalli. Gen. Comp. Endocrinol. 170, 119–124. doi: 10.1016/j.ygcen.2010.09.019
Blázquez M., Piferrer F. (2004). Cloning, sequence analysis, tissue distribution, and sex-specific expression of the neural form of P450 aromatase in juvenile sea bass (Dicentrarchus labrax). Mol. Cell. Endocrinol. 19, 83–94. doi: 10.1016/j.mce.2004.01.006
Callard G., Schlinger B., Pasmanik M. (1990). Nonmammalian vertebrate models in studies of brain-steroid interactions. J. Exp. Zool. Suppl. 4, 6–16. doi: 10.1002/jez.1402560404
Cerdá-Reverter J. M., Muriach B., Zanuy S., Muñoz-Cueto J. A. (2008). A cytoarchitectonic study of the brain of a perciform species, the sea bass (Dicentrarchus labrax): the midbrain and hindbrain. Acta Histochem. 110, 433–450. doi: 10.1016/j.acthis.2008.01.001
Cerdá-Reverter J. M., Zanuy S., Muñoz-Cueto J. A. (2001a). Cytoarchitectonic study of the brain of a perciform species, the sea bass (Dicentrarchus labrax). I. telencephalon. J. Morphol. 247, 217–228. doi: 10.1002/1097-4687(200103)247:3<217::AID-JMOR1013>3.0.CO;2-U
Cerdá-Reverter J. M., Zanuy S., Muñoz-Cueto J. A. (2001b). Cytoarchitectonic study of the brain of a perciform species, the sea bass (Dicentrarchus labrax). II. The diencephalon. J. Morphol. 247, 299–251. doi: 10.1002/1097-4687(200103)247:3<229::AID-JMOR1014>3.0.CO;2-K
Chang C., Li C. Y., Earley R. L., Hsu Y. (2012). Aggression and related behavioral traits: the impact of winning and losing and the role of hormones. Integr. Comp. Biol. 52, 801–813. doi: 10.1093/icb/ics057
Choi Y. J., Kim N. N., Habibi H. R., Choi C. Y. (2016). Effects of gonadotropin-inhibitory hormone or gonadotropin-releasing hormone on reproduction-related genes in the protandrous cinnamon clown-fish, Amphiprion melanopus. Gen. Comp. Endocrinol. 235, 89–99. doi: 10.1016/j.ygcen.2016.06.010
Clarke I. J., Sari I. P., Qi Y., Smith J. T., Parkington H. C., Ubuka T., et al. (2008). Potent action of RFamide-related peptide-3 on pituitary gonadotropes indicative of a hypophysiotropic role in the negative regulation of gonadotropin secretion. Endocrinology 149, 5811–5821. doi: 10.1210/en.2008-0575
Coumailleau P., Pellegrini E., Adrio F., Diotel N., Cano-Nicolau J., Nasri A., et al. (2015). Aromatase, estrogen receptors and brain development in fish and amphibians. Biochim. Biophys. Acta 1849, 152–162. doi: 10.1016/j.bbagrm.2014.07.002
Cowan M., Paullada-Salmerón J. A., López-Olmeda J. F., Sánchez-Vázquez F. J., Muñoz-Cueto J. A. (2017). Effects of pinealectomy on the neuroendocrine reproductive system and locomotor activity in male European sea bass, Dicentrarchus labrax. Comp. Biochem. Physiol. A. Mol. Integr. Physiol. 207, 1–12. doi: 10.1016/j.cbpa.2017.02.008
Dardente H., Birnie M., Lincoln G. A., Hazlerigg D. G. (2008). RFamide-related peptide and its cognate receptor in the sheep: cDNA cloning, mRNA distribution in the hypothalamus and the effect of photoperiod. J. Neuroendocrinol. 20, 1252–1259. doi: 10.1111/j.1365-2826.2008.01784.x
Diotel N., Do Rego J. L., Anglade I., Vaillant C., Pellegrini E., Gueguen M. M., et al. (2011a). Activity and expression of steroidogenic enzymes in the brain of adult zebrafish. Eur. J. Neurosci. 34, 45–56. doi: 10.1111/j.1460-9568.2011.07731.x
Diotel N., Do Rego J. L., Anglade I., Vaillant C., Pellegrini E., Vaudry H., et al. (2011b). The brain of teleost fish, a source, and a target of sexual steroids. Front. Neurosci. 5, 137. doi: 10.3389/fnins.2011.00137
Diotel N., Le Page Y., Mouriec K., Tong S. K., Pellegrini E., Vaillant C., et al. (2010). Aromatase in the brain of teleost fish: expression, regulation and putative functions. Front. Neuroendocrinol. 31, 172–192. doi: 10.1016/j.yfrne.2010.01.003
Diotel N., Vaillant C., Gabbero C., Mironov S., Fostier A., Gueguen M. M., et al. (2013). Effects of estradiol in adult neurogenesis and brain repair in zebrafish. Horm. Behav. 63, 193–207. doi: 10.1016/j.yhbeh.2012.04.003
Di Yorio M. P., Muñoz-Cueto J. A., Paullada-Salmerón J. A., Somoza G. M., Tsutsui K., Vissio P. G. (2019). The gonadotropin-inhibitory hormone: what we know and what we still have to learn from fish. Front. Endocrinol. 10, 78. doi: 10.3389/fendo.2019.00078
Di Yorio M. P., Pérez Sirkin D. I., Delgadin T. H., Shimizu A., Tsutsui K., Somoza G. M., et al. (2016). Gonadotropin-inhibitory hormone in the cichlid fish Cichlasoma dimerus: structure, brain distribution and differential effects on the secretion of gonadotropins and growth hormone. J. Neuroendocrinol. 28, 12377. doi: 10.1111/jne.12377
Do Rego J. L., Seong J. Y., Burel D., Leprince J., Luu-The V., Tsutsui K., et al. (2009). Neurosteroid biosynthesis: enzymatic pathways and neuroendocrine regulation by neurotransmitters and neuropeptides. Front. Neuroendocrinol. 30, 259–301. doi: 10.1016/j.yfrne.2009.05.006
Frau S., Paullada-Salmerón J. A., Paradiso I., Cowan M. E., Martín-Robles Á. J., Muñoz-Cueto J. A. (2022). From embryo to adult life: differential expression of visual opsins in the flatfish Solea senegalensis under different light spectra and photoperiods. Front. Mar. Sci 9, 797507. doi: 10.3389/fmars.2022.797507
González-Martínez D., Madigou T., Zmora N., Anglade I., Zanuy S., Zohar Y., et al. (2001). Differential expression of three different prepro-GnRH (gonadotrophin-releasing hormone) messengers in the brain of the european sea bass (Dicentrarchus labrax). J. Comp. Neurol. 429, 144–155. doi: 10.1002/1096-9861(20000101)429:1<144::aid-cne11>3.0.co;2-b
González-Martínez D., Zmora N., Mañanos E., Saligaut D., Zanuy S., Zohar Y., et al. (2002). Immunohistochemical localization of three different prepro-GnRHs in the brain and pituitary of the European sea bass (Dicentrarchus labrax) using antibodies to the corresponding GnRH-associated peptides. J. Comp. Neurol. 446, 95–113. doi: 10.1002/cne.10190
Goto-Kazeto R., Kight K. E., Zohar Y., Place A. R., Trant J. M. (2004). Localization and expression of aromatase mRNA in adult zebrafish. Gen. Comp. Endocrinol. 139, 72–84. doi: 10.1016/j.ygcen.2004.07.003
Haraguchi S., Yamamoto Y., Suzuki Y., Chang J.H., Koyama T., Sato M., et al. (2015). 7α-hydroxypregnenolone, a key neuronal modulator of locomotion, stimulates upstream migration by means of the dopaminergic system in salmon. Sci. Rep. 5, 12546. doi: 10.1038/srep12546
Harbott L. K., Burmeister S. S., White R. B., Vagell M., Fernald R. D. (2007). Androgen receptors in a cichlid fish, Astatotilapia burtoni: structure, localization, and expression levels. J. Comp. Neurol. 504, 57–73. doi: 10.1002/cne.21435
He X. Y., Dobkin C., Yang S. Y. (2019). 17β-hydroxysteroid dehydrogenases and neurosteroid metabolism in the central nervous system. Mol. Cell. Endocrinol. 489, 92–97. doi: 10.1016/j.mce.2018.10.002
Hojo Y., Hattori T. A., Enami T., Furukawa A., Suzuki K., Ishii H. T., et al. (2004). Adult male rat hippocampus synthesizes estradiol from pregnenolone by cytochromes P45017a and P450 aromatase localized in neurons. Proc. Natl. Acad. Sci. U.S.A. 101, 865–870. doi: 10.1073/pnas.2630225100
Huffman L. S., O'Connell L. A., Hofmann H. A. (2013). Aromatase regulates aggression in the African cichlid fish Astatotilapia burtoni. Physiol. Behav 112-113, 77–83. doi: 10.1016/j.physbeh.2013.02.004
Iwata E., Mikami K., Manbo J., Moriya-Ito K., Sasaki H. (2012). Social interaction influences blood cortisol values and brain aromatase genes in the protandrous false clown anemonefish, Amphiprion ocellaris. Zool. Sci 29, 849–855. doi: 10.2108/zsj.29.849
Kriegsfeld L. J., Mei D. F., Bentley G. E., Ubuka T., Mason A. O., Inoue K., et al. (2006). Identification and characterization of a gonadotropin-inhibitory system in the brains of mammals. Proc. Natl. Acad. Sci. U.S.A. 103, 2410–2415. doi: 10.1073/pnas.0511003103
Lapchak P. A., Araujo D. M. (2001). Preclinical development of neurosteroids as neuroprotective agents for the treatment of neurodegenerative diseases. Int. Rev. Neurobiol. 46, 379–397. doi: 10.1016/s0074-7742(01)46069-7
Livak K. J., Schmittgen T. D. (2001). Analysis of relative gene expression data using real-time quantitative PCR and the 2(-ΔΔC(T)) method. Methods 25, 402–408. doi: 10.1006/meth.2001.1262
Lord L. D., Bond J., Thompson R. R. (2009). Rapid steroid influences on visually guided sexual behaviour in male goldfish. Horm. Behav. 56, 519–526. doi: 10.1016/j.yhbeh.2009.09.002
Manciocco A., Toni M., Tedesco A., Malavasi S., Alleva E., Cioni C. (2015). The acclimation of European sea bass (Dicentrarchus labrax) to temperature: behavioural and neurochemical responses. Ethology 121, 68–83. doi: 10.1111/eth.12315
Maninger N., Wolkowitz O. M., Reus V. I., Epel E. S., Mellon S. H. (2009). Neurobiological and neuropsychiatric effects of dehydroepiandrosterone (DHEA) and DHEA sulfate (DHEAS). Front. Neuroendocrinol. 30, 65–91. doi: 10.1016/j.yfrne.2008.11.002
Menuet A., Anglade I., Le Guevel R., Pellegrini E., Pakdel F., Kah O. (2003). Distribution of aromatase mRNA and protein in the brain and pituitary of female rainbow trout: comparison with estrogen receptor alpha. J. Comp. Neurol. 462, 180–193. doi: 10.1002/cne.10726
Menuet A., Pellegrini E., Brion F., Gueguen M. M., Anglade I., Pakdel F., et al. (2005). Expression and estrogen-dependent regulation of the zebrafish brain aromatase gene. J. Comp. Neurol. 485, 304–320. doi: 10.1002/cne.20497
Mindnich R., Deluca D., Adamski J. (2004). Identification and characterization of 17 beta-hydroxysteroid dehydrogenases in the zebrafish, Danio rerio. Mol. Cell. Endocrinol. 215, 19–30. doi: 10.1016/j.mce.2003.11.010
Muñoz-Cueto J. A., Paullada-Salmerón J. A., Aliaga-Guerrero M., Cowan M. E., Parhar I. S., Ubuka T. (2017). A journey through the gonadotropin-inhibitory hormone system of fish. Front. Endocrinol. 8, 285. doi: 10.3389/fendo.2017.00285
Muriach B., Carrillo M., Zanuy S., Cerdá-Reverter J. M. (2008b). Distribution of estrogen receptor 2 mRNAs (Esr2a and Esr2b) in the brain and pituitary of the sea bass (Dicentrarchus labrax). Brain. Res. 1210, 126–141. doi: 10.1016/j.brainres.2008.02.053
Muriach B., Cerdá-Reverter J. M., Gómez A., Zanuy S., Carrillo M. (2008a). Molecular characterization and central distribution of the estradiol receptor alpha (ERalpha) in the sea bass (Dicentrarchus labrax). J. Chem. Neuroanat. 35, 33–48. doi: 10.1016/j.jchemneu.2007.05.010
O’Connor C. M., Gilmour K. M., van der Kraak G., Cooke S. J. (2011). Circulating androgens are influenced by parental nest defense in a wild teleost fish. J. Comp. Physiol. A. 197, 711–715. doi: 10.1007/s00359-011-0629-6
Ogawa S., Sivalingam M., Biran J., Golan M., Anthonysamy R. S., Levavi-Sivan B., et al. (2016). Distribution of LPXRFa, a gonadotropin-inhibitory hormone ortholog peptide, and LPXRFa receptor in the brain and pituitary of the tilapia. J. Comp. Neurol. 524, 2753–2775. doi: 10.1002/cne.23990
Okubo K., Takeuchi A., Chaube R., Paul-Prasanth B., Kanda S., Oka Y., et al. (2011). Sex differences in aromatase gene expression in the medaka brain. J. Neuroendocrinol. 23, 412–423. doi: 10.1111/j.1365-2826.2011.02120.x
Oliveira R. F., Hirschenhauser K., Carneiro L. A., Canario A. V. (2002). Social modulation of androgen levels in male teleost fish. Comp. Biochem. Physiol. B. Biochem. Mol. Biol. 132, 203–215. doi: 10.1016/s1096-4959(01)00523-1
Osugi T., Ukena K., Bentley G. E., O’Brien S., Moore I. T., Wingfield J. C., et al. (2004). Gonadotropin-inhibitory hormone in gambel’s white-crowned sparrows: cDNA identification, transcript localization and functional effects in laboratory and field experiments. J. Endocrinol. 182, 33–42. doi: 10.1677/joe.0.1820033
Paullada-Salmerón J. A., Cowan M., Aliaga-Guerrero M., Gómez A., Zanuy S., Mañanos E., et al. (2016a). LPXRFa peptide system in the European sea bass: a molecular and immunohistochemical approach. J. Comp. Neurol. 524, 176–198. doi: 10.1002/cne.23833
Paullada-Salmerón J. A., Cowan M., Aliaga-Guerrero M., López-Olmeda J. F., Mañanós E. L., Zanuy S., et al. (2016c). Testicular steroidogenesis and locomotor activity are regulated by gonadotropin-inhibitory hormone in male European sea bass. PLoS. One 11, e0165494. doi: 10.1371/journal.pone.0165494
Paullada-Salmerón J. A., Cowan M., Aliaga-Guerrero M., Morano F., Zanuy S., Muñoz-Cueto J. A. (2016b). Gonadotropin inhibitory hormone down-regulates the brain-pituitary reproductive axis of male European sea bass (Dicentrarchus labrax). Biol. Reprod. 94, 121. doi: 10.1095/biolreprod.116.139022
Paullada-Salmerón J. A., Cowan M. E., Loentgen G. H., Aliaga-Guerrero M., Zanuy S., Mañanós E. L., et al. (2019). The gonadotropin-inhibitory hormone system of fish: the case of sea bass (Dicentrarchus labrax). Gen. Comp. Endocrinol. 279, 184–195. doi: 10.1016/j.ygcen.2019.03.015
Paullada-Salmerón J. A., Loentgen G. H., Cowan M., Aliaga-Guerrero M., Rendón-Unceta M. D., Muñoz-Cueto J. A. (2017). Developmental changes and day-night expression of the gonadotropin-inhibitory hormone system in the European sea bass: effects of rearing temperature. Comp. Biochem. Physiol. A. Mol. Integr. Physiol. 206, 54–62. doi: 10.1016/j.cbpa.2017.01.009
Paullada-Salmerón J. A., Wang B., Muñoz-Cueto J. A. (2023). Spexin in the European sea bass, Dicentrarchus labrax: characterization, brain distribution, and interaction with gnrh and gnih neurons. J. Comp. Neurol. 531, 314–335. doi: 10.1002/cne.25428
Pellegrini E., Diotel N., Vaillant-Capitaine C., Pérez Maria R., Gueguen M. M., Nasri A., et al. (2016). Steroid modulation of neurogenesis: focus on radial glial cells in zebrafish. J. Steroid. Biochem. Mol. Biol. 160, 27–36. doi: 10.1016/j.jsbmb.2015.06.011
Pellegrini E., Menuet A., Lethimonier C., Adrio F., Gueguen M. M., Tascon C., et al. (2005). Relationships between aromatase and estrogen receptors in the brain of teleost fish. Gen. Comp. Endocrinol. 142, 60–66. doi: 10.1016/j.ygcen.2004.12.003
Pellegrini E., Mouriec K., Anglade I., Menuet A., Le Page Y., Gueguen M. M., et al. (2007). Identification of aromatase-positive radial glial cells as progenitor cells in the ventricular layer of the forebrain in zebrafish. J. Comp. Neurol. 501 (1), 150–167. doi: 10.1002/cne.21222
Perkins A., Roselli C. E. (2007). The ram as a model for behavioral neuroendocrinology. Horm. Behav. 52, 70–77. doi: 10.1016/j.yhbeh.2007.03.016
Piekarski D. J., Zhao S., Jennings K. J., Iwasa T., Legan S. J., Mikkelsen J. D., et al. (2013). Gonadotropin-inhibitory hormone reduces sexual motivation but not lordosis behaviour in female Syrian hamsters (Mesocricetus auratus). Horm. Behav. 64, 501–510. doi: 10.1016/j.yhbeh.2013.06.006
Piferrer F., Blázquez M. (2005). Aromatase distribution and regulation in fish. Fish. Physiol. Biochem. 31, 215–226. doi: 10.1007/s10695-006-0027-0
Pradhan D. S., Lau L. Y. M., Schmidt K. L., Soma K. K. (2010). 3βhsd in songbird brain: subcellular localization and rapid regulation by estradiol. J. Neurochem. 115, 667–675. doi: 10.1111/j.1471-4159.2010.06954.x
Puelles L. (2019). Survey of midbrain, diencephalon, and hypothalamus neuroanatomic terms whose prosomeric definition conflicts with columnar tradition. Front. Neuroanat. 13, 20. doi: 10.3389/fnana.2019.00020
Quintana L., Jalabert C., Fokidis H. B., Soma K. K., Zubizarreta L. (2021). Neuroendocrine mechanisms underlying non-breeding aggression: common strategies between birds and fish. Front. Neural. Circuits. 15, 716605. doi: 10.3389/fncir.2021.716605
Ramallo M. R., Morandini L., Birba A., Somoza G. M., Pandolfi M. (2017). From molecule to behavior: brain aromatase (cyp19a1b) characterization, expression analysis and its relation with social status and male agonistic behavior in a Neotropical cichlid fish. Horm. Behav. 89, 176–188. doi: 10.1016/j.yhbeh.2017.02.005
Remage-Healey L., Bass A. H. (2006). From social behavior to neural circuitry: steroid hormones rapidly modulate advertisement calling via a vocal pattern generator. Horm. Behav. 50, 432–441. doi: 10.1016/j.yhbeh.2006.05.007
Remage-Healey L., Bass A. H. (2007). Plasticity in brain sexuality is revealed by the rapid actions of steroid hormones. J. Neurosci. 27, 1114–1122. doi: 10.1523/JNEUROSCI.4282-06.2007
Sakamoto H., Ukena K., Tsutsui K. (2001). Activity and localization of 3beta-hydroxysteroid dehydrogenase/Delta5-Delta4- isomerase in the zebrafish central nervous system. J. Comp. Neurol. 439, 291–305. doi: 10.1002/cne.1351
Sánchez-Vázquez F. J., Muñoz-Cueto J. A. (2014). Biology of European seabass (CRC Press, Boca Raton: United States of America).
Schlinger B. A., Pradhan D. S., Soma K. K. (2008). 3b-HSD activates DHEA in the songbird brain. Neurochem. Int. 52, 611–620. doi: 10.1016/j.neuint.2007.05.003
Servili A., Bufalino M. R., Nishikawa R., Sanchez de Melo I., Muñoz-Cueto J. A., Lee L. E. (2009). Establishment of long term cultures of neural stem cells from adult sea bass, Dicentrarchus labrax. Comp. Biochem. Physiol. A. Mol. Integr. Physiol. 152, 245–254. doi: 10.1016/j.cbpa.2008.10.018
Servili A., Lethimonier C., Lareyre J. J., López-Olmeda J. F., Sánchez-Vázquez F. J., Kah O., et al. (2010). The highly conserved gonadotropin-releasing hormone-2 form acts as a melatonin-releasing factor in the pineal of a teleost fish, the european sea bass Dicentrarchus labrax. Endocrinology 151, 2265–2275. doi: 10.1210/en.2009-1207
Shaw K., Krahe R. (2018). Pattern of aromatase mRNA expression in the brain of a weakly electric fish, Apteronotus leptorhynchus. J. Chem. Neuroanat. 90, 70–79. doi: 10.1016/j.jchemneu.2017.12.009
Soga T., Kitahashi T., Clarke I. J., Parhar I. S. (2014). Gonadotropin-inhibitory hormone promoter-driven enhanced green fluorescent protein expression decreases during aging in female rats. Endocrinology 155, 1944–1955. doi: 10.1210/en.2013-1786
Soma K. K., Scotti M. A., Newman A. E., Charlier T. D., Demas G. E. (2008). Novel mechanisms for neuroendocrine regulation of aggression. Front. Neuroendocrinol. 29, 476–489. doi: 10.1016/j.yfrne.2007.12.003
Steckelbroeck S., Stoffel-Wagner B., Reichelt R., Schramm J., Bidlingmaier F., Siekmann L., et al. (1999). Characterization of 17b-hydroxysteroid dehydrogenase activity in brain tissue: testosterone formation in the human temporal lobe. J. Neuroendocrinol. 11, 457–464. doi: 10.1046/j.1365-2826.1999.00363.x
Strobl-Mazzulla P. H., Lethimonier C., Gueguen M. M., Karube M., Fernandino J. I., Yoshizaki G., et al. (2008). Brain aromatase (Cyp19A2) and estrogen receptors, in larvae and adult pejerrey fish Odontesthes bonariensis: neuroanatomical and functional relations. Gen. Comp. Endocrinol. 158, 191–201. doi: 10.1016/j.ygcen.2008.07.006
Strobl-Mazzulla P. H., Moncaut N. P., López G. C., Miranda L. A., Canario A. V., Somoza G. M. (2005). Brain aromatase from pejerrey fish (Odontesthes bonariensis): cDNA cloning, tissue expression, and immunohistochemical localization. Gen. Comp. Endocrinol. 143, 21–32. doi: 10.1016/j.ygcen.2005.02.026
Strous R. D., Maayan R., Weizman A. (2006). The relevance of neurosteroids to clinical psychiatry: from the laboratory to the bedside. Eur. Neuropsychopharmacol. 16, 155–169. doi: 10.1016/j.euroneuro.2005.09.005
Teles M. C., Oliveira R. F. (2016). Androgen response to social competition in a shoaling fish. Horm. Behav. 78, 8–12. doi: 10.1016/j.yhbeh.2015.10.009
Tsutsui K., Saigoh E., Ukena K., Teranishi H., Fujisawa Y., Kikuchi M., et al. (2000). A novel avian hypothalamic peptide inhibiting gonadotropin release. Biochem. Biophys. Res. Commun. 275, 661–667. doi: 10.1006/bbrc.2000.3350
Ubuka T., Haraguchi S., Tobari Y., Narihiro M., Ishikawa K., Hayashi T., et al. (2014). Hypothalamic inhibition of socio-sexual behaviours by increasing neuroestrogen synthesis. Nat. Commun. 5, 3061. doi: 10.1038/ncomms4061
Ubuka T., Mizuno T., Fukuda Y., Bentley G. E., Wingfield J. C., Tsutsui K. (2013). RNA interference of gonadotropin-inhibitory hormone gene induces aggressive and sexual behaviors in birds. Gen. Comp. Endocrinol. 181, 179–186. doi: 10.1016/j.ygcen.2012.09.010
Ubuka T., Tsutsui K. (2014). Neuroestrogen regulation of socio-sexual behavior of males. Front. Neurosci. 8, 323. doi: 10.3389/fnins.2014.00323
Ubuka T., Tsutsui K. (2022). Neuropeptidergic control of neurosteroids biosynthesis. Front. Neuroendocrinol. 65, 100976. doi: 10.1016/j.yfrne.2021.100976
Ukena K., Koda A., Yamamoto K., Kobayashi T., Iwakoshi-Ukena E., Minakata H., et al. (2003). Novel neuropeptides related to frog growth hormone-releasing peptide: isolation, sequence, and functional analysis. Endocrinology 144, 3879–3884. doi: 10.1210/en.2003-0359
Vullioud P., Bshary R., Ros A. F. (2013). Intra- and interspecific aggression do not modulate androgen levels in dusky gregories, yet male aggression is reduced by an androgen blocker. Horm. Behav. 64, 430–438. doi: 10.1016/j.yhbeh.2013.06.007
Wang B., Paullada-Salmerón J. A., Vergès-Castillo A., Gómez A., Muñoz-Cueto J. A. (2022). Signaling pathways activated by sea bass gonadotropin-inhibitory hormone peptides in COS-7 cells transfected with their cognate receptor. Front. Endocrinol. 13, 982246. doi: 10.3389/fendo.2022.982246
Keywords: gonadotropin-inhibitory hormone, aggressive behavior, aromatase, neuroestrogens, neuroandrogens, fish, brain
Citation: Paullada-Salmerón JA, Loentgen GH, Fuentès M, Besseau L, Ubuka T, Mañanos EL and Muñoz-Cueto JA (2023) Gonadotropin inhibitory-hormone modulates neurosteroids-synthesizing enzymes expression and aggressive behavior in male sea bass, Dicentrarchus labrax. Front. Mar. Sci. 10:1185652. doi: 10.3389/fmars.2023.1185652
Received: 13 March 2023; Accepted: 06 June 2023;
Published: 30 June 2023.
Edited by:
Alejandro S. Mechaly, CONICET Instituto de Investigaciones en Biodiversidad y Biotecnología (INBIOTEC), ArgentinaReviewed by:
Fabián Canosa, CONICET Institute of Biotechnological Research (IIB-INTECH), ArgentinaHuapu Chen, Guangdong Ocean University, China
Satoshi Ogawa, Monash University Malaysia, Malaysia
Copyright © 2023 Paullada-Salmerón, Loentgen, Fuentès, Besseau, Ubuka, Mañanos and Muñoz-Cueto. This is an open-access article distributed under the terms of the Creative Commons Attribution License (CC BY). The use, distribution or reproduction in other forums is permitted, provided the original author(s) and the copyright owner(s) are credited and that the original publication in this journal is cited, in accordance with accepted academic practice. No use, distribution or reproduction is permitted which does not comply with these terms.
*Correspondence: José Antonio Muñoz-Cueto, bXVub3ouY3VldG9AdWNhLmVz; José Antonio Paullada-Salmerón, am9zZWFudG9uaW8ucGF1bGxhZGFAdWNhLmVz
†These authors have contributed equally to this work and share first authorship