- 1Department of Paleoceanography, Institute of Oceanology Polish Academy of Sciences, Sopot, Poland
- 2Department of Geosciences, University of Tromsø (UiT) The Arctic University of Norway, Tromsø, Norway
- 3NORCE Climate and Environment, NORCE Norwegian Research Centre AS and Bjerknes Centre for Climate Research, Bergen, Norway
Sedimentary ancient DNA (sedaDNA) offers a novel retrospective approach to reconstructing the history of marine ecosystems over geological timescales. Until now, the biological proxies used to reconstruct paleoceanographic and paleoecological conditions were limited to organisms whose remains are preserved in the fossil record. The development of ancient DNA analysis techniques substantially expands the range of studied taxa, providing a holistic overview of past biodiversity. Future development of marine sedaDNA research is expected to dramatically improve our understanding of how the marine biota responded to changing environmental conditions. However, as an emerging approach, marine sedaDNA holds many challenges, and its ability to recover reliable past biodiversity information needs to be carefully assessed. This review aims to highlight current advances in marine sedaDNA research and to discuss potential methodological pitfalls and limitations.
1 Introduction
Marine sedimentary archives are an important source of information for understanding the environment in which marine organisms lived over geological time scales. The analysis of these archives adopts a multidisciplinary approach, which requires the engagement of experts in geology, organic and inorganic geochemistry, geomorphology, paleoceanography, and micropaleontology, (e.g., Backman et al. (2009); Łącka et al. (2019); Łącka et al. (2020); Pawłowska et al. (2020a); Marino et al. (2022)). Records of past environmental change can be accessed by analyzing indirect sources of information (so-called proxies) retrieved from marine sediment cores. These proxies can be fossil assemblages, indicator species, or geochemical proxies such as lipid biomarkers, pigments, or sedimentological properties. Such multidisciplinary approaches have greatly expanded our knowledge of marine paleo-communities and their physical and chemical environments, providing invaluable information for predictions on marine ecosystem response to future climate changes and anthropogenic pressures. However, our current view of past environmental changes and evolutionary responses of marine organisms has, until recently, been limited to selected groups of organisms whose remains are preserved in the fossil record. Examples of such fossilizing taxa are mollusks (Machado et al., 2018; Mcgann and Powell, 2022), coccolithophores (Jacques and Luc, 2007; Marino et al., 2022), foraminifera (Kujawa et al., 2021; Pados-Dibattista et al., 2022), diatoms (Oksman et al., 2017; Miettinen, 2018), and dinoflagellate cysts (Ellegaard et al., 2017; Hennissen et al., 2017; Gussone and Friedrich, 2018; Aubry et al., 2020). The compositional variation of these taxa reflects transformations in marine environmental conditions. Our current understanding of the past ocean is largely based on traditional proxies, such as foraminifera, for which oxygen isotope records provided detailed insight into the climate history during the Cenozoic (Zachos et al., 2008) and Mg/Ca ratios provided sea water temperature estimates (Mcclymont et al., 2020). However, these proxies are often used to infer a specific environmental variable (e.g., temperature, salinity) and do not provide an overview of biodiversity changes. Moreover, their taxonomic analysis is often labor-intensive and requires expertise that is not readily available making impractical their applications for long-term or large-scale assessments.
Over the last decade, advances in high-throughput DNA sequencing technologies and laboratory techniques for working with ancient DNA have offered richer, higher-quality analyses that better facilitate the reconstruction and understanding of paleo-communities. An initial study by Coolen and Overmann (1998) successfully used ancient 16S rDNA fragments from lake sediments and the carotenoid okenone to show that bottom-water anoxia and a sulfidic chemocline persisted in Mahoney Lake, Canada, during most of the Holocene. After this study, the number of sedaDNA-based publications remained relatively low until 2014. Since then, their number has been consistently increasing, yet studies of marine sediments remain less numerous than those of freshwater environments (Figure 1A). Early marine sedaDNA studies tested the burial of DNA and compared the microbial communities of sedaDNA and fossil assemblages exploring various marine habitats from coastal polar regions (Coolen et al., 2004; Boere et al., 2009; Pawłowska et al., 2014), to anoxic basins (Coolen et al., 2009; Boere et al., 2011; Coolen, 2011; Coolen et al., 2013) and abyssal plains (Lejzerowicz et al., 2013). An increasing number of studies is now combining sedaDNA analyses with other proxy data to gain insight into past oceanographic conditions, such as sea-ice cover (Boere et al., 2009; De Schepper et al., 2019; Pawłowska et al., 2020a; Zimmermann et al., 2020; Zimmermann et al., 2021), sea-surface temperatures and subsurface salinities (Zimmermann et al., 2021). As shown by the main keywords associated with sedaDNA publications, their main focus so far has been: “paleoenvironments”, changes in “biodiversity”, “climate change” and “long-term dynamics” (Figure 1B).
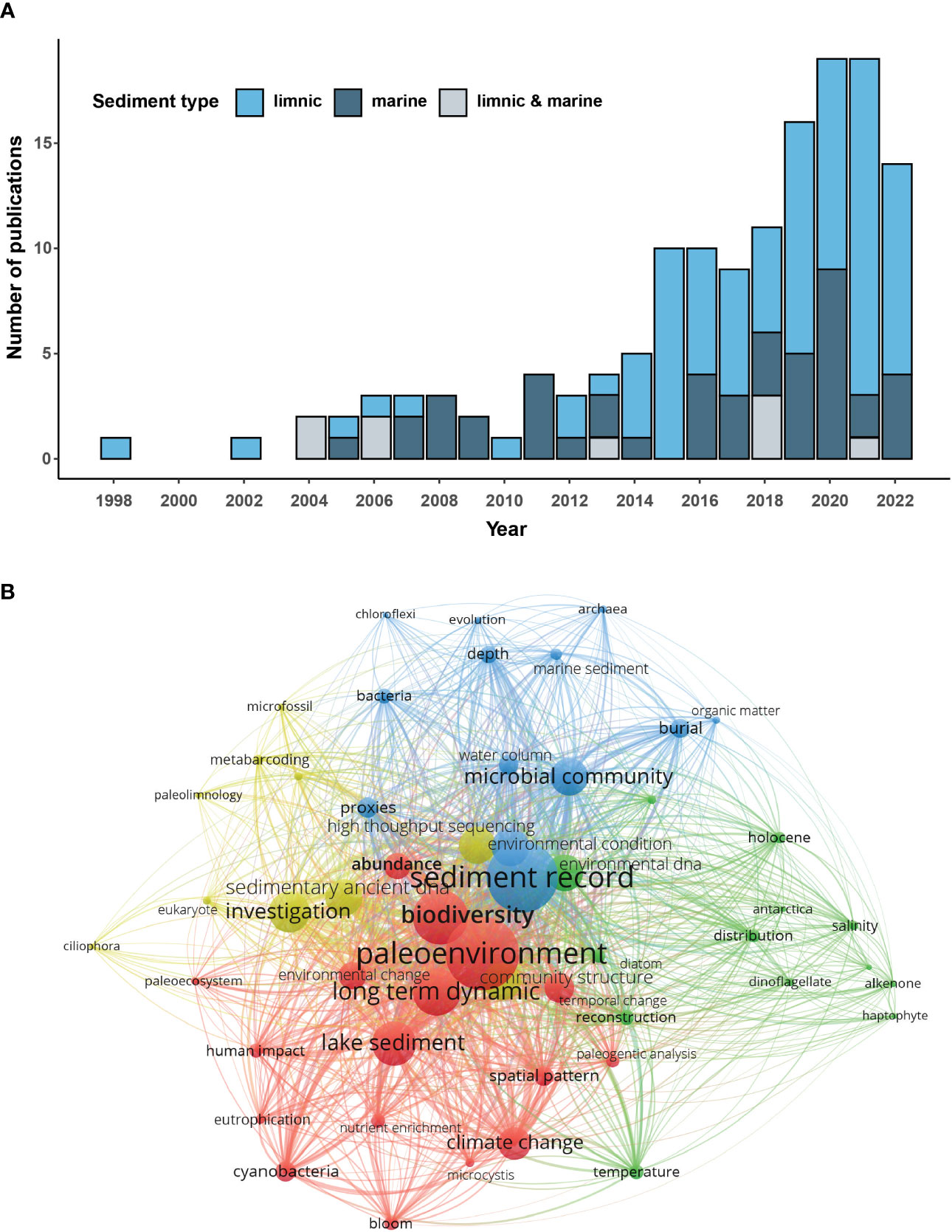
Figure 1 (A) Bar chart showing the annual number of published sedaDNA research articles retrieved from Google Scholar and cross-checked with the literature database of the sedaDNA scientific society (https://sedadna.github.io/). We queried for papers that (1) presented sedaDNA data, and (2) targeted either bacteria, archaea, micro-eukaryotes (protists, fungi), meiofauna, or small zooplankton, and verified them manually. (B) Co-occurrence network map of keywords found in abstracts of sedaDNA research papers. The network map was generated using the VOSviewer software (Perianes-Rodriguez et al., 2016). Size of the nodes and font are proportional to the eigenvector centrality; the nodes were colored to show different clusters. A total of 142 original studies on limnic and/or marine sedaDNA were included in this review (Table S1).
This review aims to highlight the potential of sedimentary ancient DNA-based research for the characterization of past marine biodiversity and its response to environmental changes. We describe taphonomic or preservation processes and discuss key issues related to procedures for sampling, laboratory processing, and computational techniques involved in marine sedaDNA investigation. We focus on both planktonic and benthic microbial (prokaryotes and single-cell eukaryotes) and meiofaunal organisms deposited in marine sediments and discuss their potential in studying biodiversity changes across geological timescales.
2 Taphonomy and characteristics of marine sedaDNA
Marine sedaDNA research involves the analysis of genetic material preserved in marine sediments from organisms that once lived in the water column or on the ocean floor. Several investigations in different sedimentary basins shed some light on the taphonomy of sedaDNA in marine settings (Sunday et al., 2014; Kelly et al., 2018; Ellegaard et al., 2020) (Figure 2). The processes that regulate the accumulation and preservation of environmental DNA (eDNA) in marine environments are more complex than in the freshwater-terrestrial system. This is because the marine environment with its surface and bottom water currents, sinking water masses, lateral sediment transports, and sometimes very active benthic communities, is more dynamic than freshwater systems (Pedersen et al., 2015; Barrenechea Angeles et al., 2020; Allan et al., 2021).
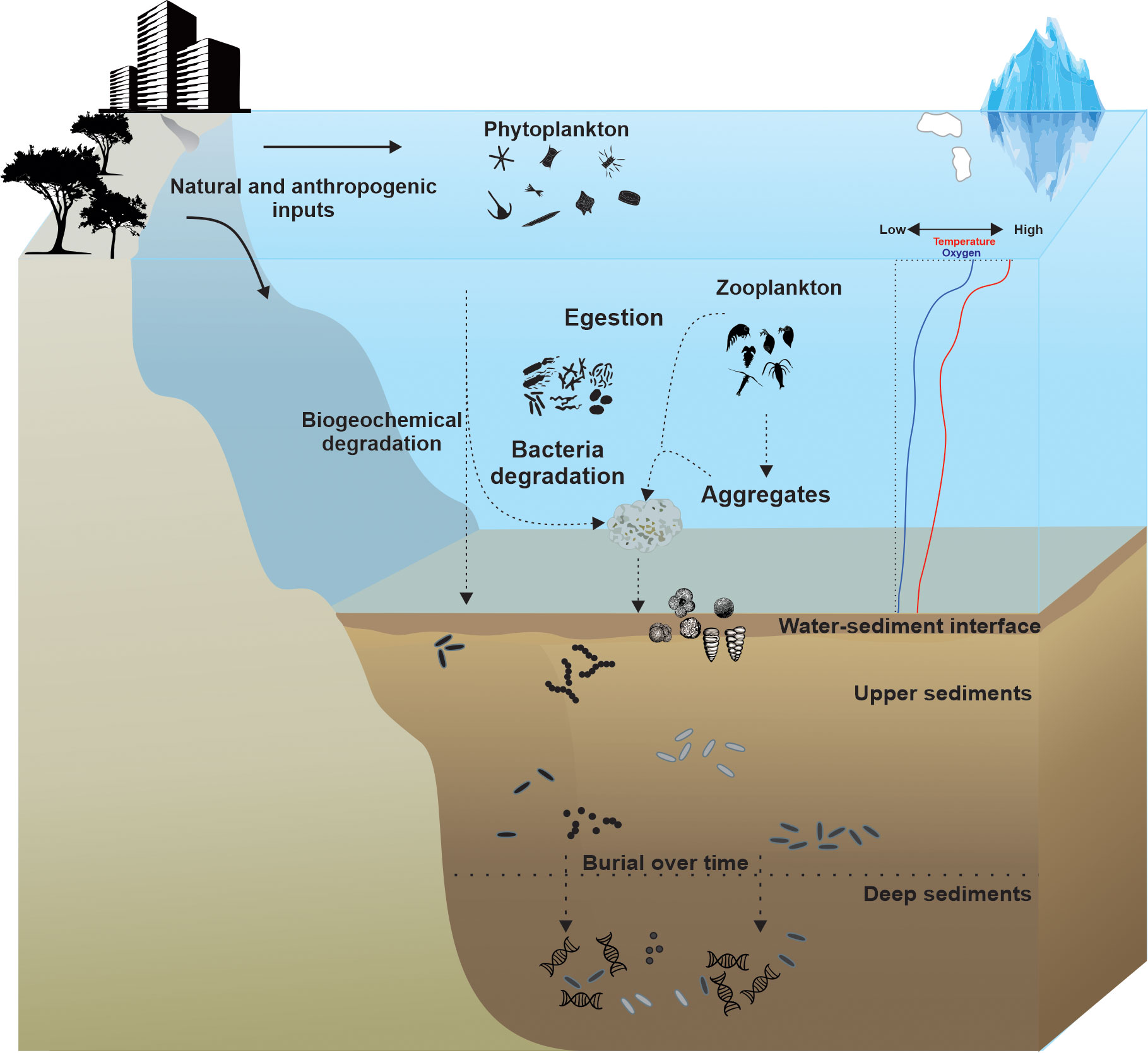
Figure 2 Schematic illustration of eDNA taphonomic processes in the marine environment. These processes involve eDNA distribution, degradation, and/or preferential preservation during the transition from the pelagic to the benthic zones, and from the water-sediment interface into subsurface sediment.
The planktonic community, dominated by prokaryotes (Kallmeyer et al., 2012), protists (Vargas et al., 2015), and zooplankton (Murrell and Lores, 2004), is responsible for a large portion of the marine sedaDNA (Barrenechea Angeles et al., 2020). Several studies have described how planktonic DNA is deposited and preserved in sediments (Corinaldesi et al., 2011; Torti et al., 2018). As shown in Figure 2, after the death of planktonic organisms, their DNA has to travel through the water column for tens (shallow shelf) to thousands (open ocean) of meters to reach the sea floor (Smayda, 1971; Bach et al., 2016). During this journey, the planktonic DNA can be associated with organism remains or attached to particulate organic matter, skeletons, bound detritus, or inorganic mineral grains (Iversen and Ploug, 2010; Herndl and Reinthaler, 2013; Wood et al., 2020). Depending on the size of the sinking particles (Cael and White, 2020) and a range of physical and biological mechanisms (aggregation, downwelling and density inversion currents, packaging of cells in fecal pellets) in situ (Bach et al., 2016), the plankton sinking can take several days or even longer time to reach the seafloor (Bach et al., 2019; Nooteboom et al., 2019). The degree of planktonic DNA degradation depends on various factors such as organic matter load, temperature, pH, salinity, water depth, light intensity, and organismal activity through the water column (Corinaldesi et al., 2008; Torti et al., 2015; Andruszkiewicz et al., 2017; Collins et al., 2018; Mccartin et al., 2022). Some authors suggested that macrobial eDNA degradation rates differ between marine, brackish, and freshwater systems (Thomsen et al., 2012; Collins et al., 2018). An experimental and comparative study by Collins et al. (2018) indicated the rate of decay to be 1.6 times faster in inshore waters than in offshore waters. Salinity seemed to be an important factor in steering decay rates, but it could also be related to microbial activities (Collins et al., 2018). In general, degradation rates of eDNA decrease in colder seawater (Okabe and Shimazu, 2007; Mccartin et al., 2022) with a higher pH (Collins et al., 2018), high dissolved organic carbon content, and low bacterial activity (Corinaldesi et al., 2008). However, very little is known about how the degradation of planktonic DNA that is going through the water column and deposited on the seafloor can impact the composition of planktonic communities retrieved from sedaDNA analyses (Morard et al., 2017; Barrenechea Angeles et al., 2020).
The physicochemical characteristics of the sediment in concert with environmental conditions are considered to play important roles in the preservation of sedaDNA on the seafloor (Corinaldesi et al., 2008; Vuillemin et al., 2019). Early ancient DNA studies indicated that the sediment’s characteristics such as high clay, borate, and organic content under cold/frozen and anoxic conditions facilitate optimal DNA preservation (Willerslev et al., 2004; Coolen and Overmann, 2007; Coolen et al., 2013; Furukawa et al., 2013; Torti et al., 2015). Indeed, it has been demonstrated experimentally that organic matter and clay increase the DNA adsorption capacity of sediment (Xue and Feng, 2018). In addition, the adsorption of DNA to sand is also well documented (Lorenz and Wackernagel, 1987; Robert et al., 2005). However, overall, the relation between marine sediment characteristics and sedaDNA preservation over time is poorly understood. Similarly, it remains unclear how bottom water temperature impacts sedaDNA preservation. Since the bottom water temperature is remarkably stable and colder than 4°C below a depth of 700 m (Locarnini et al., 2018) it is possible that the influence of the bottom water temperature on the preservation of sedaDNA may be comparable over large parts of the ocean. To date, the majority of sedaDNA studies have been performed in high-latitude, cold environments, particularly the polar regions (Pawłowska et al., 2014; De Schepper et al., 2019), or deep-sea subsurface sediments (Corinaldesi et al., 2011; More et al., 2018; Armbrecht et al., 2021a). Warmer, shallow, or marginal seas and oceanic regions with strong seasonality were long assumed to be less favorable for the long-term preservation of eDNA. However, some studies show that eDNA can also be well preserved in temperate and tropical regions, beneath toxic water columns (Coolen et al., 2013; More et al., 2018), in well−oxygenated deep-sea sediments (Lejzerowicz et al., 2013), and tropical reef sediments (Del Carmen Gomez Cabrera et al., 2019).
Although no accurate timeline predicting sedaDNA preservation potential in various sediment types or geographical regions exists, marine sedaDNA has been recovered back to the early Quaternary (Figure 3A). Micro-eukaryotic DNA has been recovered from 800-year-old fjord sediments (Pawłowska et al., 2014), 30,000-year-old deep-sea sediments (Lejzerowicz et al., 2013), 43,000-year-old oxygen-minimum zone sediments (More et al., 2018), and 100,000-year-old Greenland Sea sediments (De Schepper et al., 2019). Even longer preservation was documented in sediments dating back to 1–1.4 Ma (Kirkpatrick et al., 2016; Armbrecht et al., 2022). Compared to modern sedimentary DNA, the sedaDNA molecules are typically degraded into short fragments and may have extensive chemical damage (Armbrecht et al., 2020). However, some studies have shown that ancient DNA fragments longer than 500 base pairs (bp) can be recovered from subsurface sediments, for example, from the eastern Mediterranean Holocene and Pleistocene sapropels with aforementioned conditions for preservation (Coolen and Overmann, 2007; Boere et al., 2011) (see also Table S1; Figure 3A). This could be explained by the ability of some microbes such as bacteria and archaea (Vuillemin et al., 2019; Thomas et al., 2020; Capo et al., 2022), dinoflagellates, and diatoms (Ribeiro et al., 2011; Sanyal et al., 2022), to form dormant or resting stages for extended periods following sediment burial.
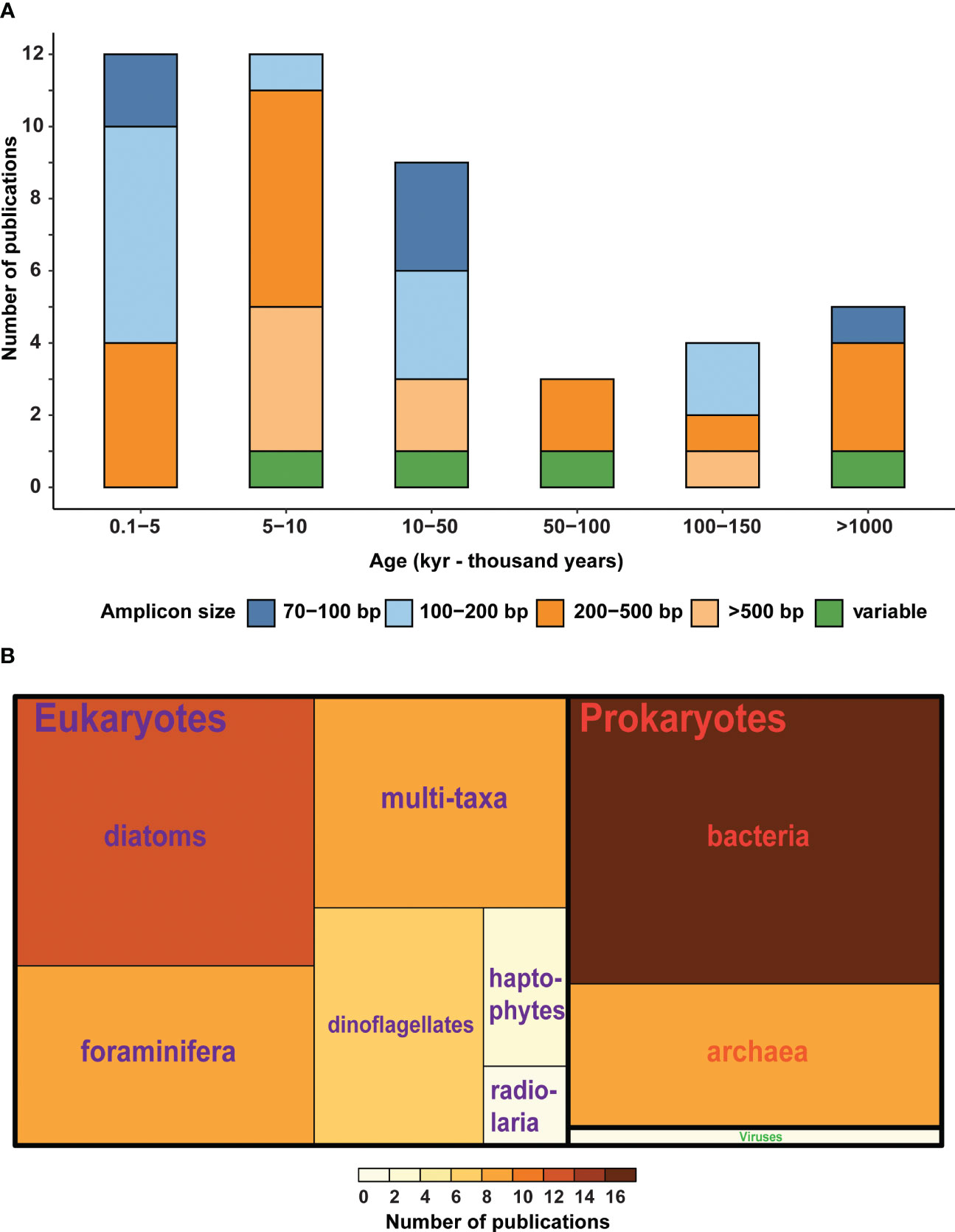
Figure 3 (A) Bar chart showing the number of published articles on marine sedaDNA, indicating the age of the sediment and the fragment length of the analyzed amplicons, variable: a range of fragment sizes is obtained through metagenomics. (B) Treemap showing the number of published articles targeting different taxonomic groups of prokaryotes and eukaryotes in marine sedaDNA. A total of 55 marine sedaDNA studies were included in this figure (Table S1).
3 Marine sedaDNA processing and analysis
The methods used in sedaDNA processing and analysis have been reviewed by several authors, e.g. Epp et al. (2019); Fulton and Shapiro (2019); Capo et al. (2021). However, only a few papers concern specifically the marine environment, focusing on the collection, storage, and manipulation of marine sediments for sedaDNA analysis (Armbrecht et al., 2019; Armbrecht et al., 2020; Selway et al., 2022). Here we will shortly discuss some of these methods with a special focus on challenges related to marine sediments.
3.1 Sediment coring
The sediment core sampling strategy is the major difference between marine sedaDNA and other studies involving ancient environmental DNA. In particular, sampling long cores (> 10-meter-long) from depths of hundreds to thousands of meters below the sea surface, while avoiding contamination is the main challenge of marine sedaDNA. Depending on the functional mechanisms that ensure penetration into the sediment, the following coring methods are commonly used in marine sedaDNA research: gravity corer (Coolen et al., 2013; Torti et al., 2018; De Schepper et al., 2019; More et al., 2021), piston corer (Zimmermann et al., 2021; Armbrecht et al., 2022), multicorer (Coolen et al., 2013; Lejzerowicz et al., 2013; De Schepper et al., 2019), and boxcorer (Coolen et al., 2006). The gravity and piston corers are used for obtaining sediment records of several meters in length to provide insights into past environmental change over decades, centuries to millennia. Multicore systems, on the other hand, are typically used for short-term records of the seafloor and allow for simultaneous collection of 2, 4 or even 6 short cores (< 1 m).
Since collecting multiple gravity or piston cores in the marine environment can be problematic and costly, splitting the core lengthwise and taking multiple subsamples from the same core is usually the best way to obtain replicate samples for sedaDNA analysis. Subsequent steps of sedaDNA analysis should be performed in a sterile environment, ideally in a dedicated ancient DNA laboratory. Subsampling of sediment cores can be performed with sterile knives, spoons, and spatulas (Lejzerowicz et al., 2013; Hou et al., 2014; Szczuciński et al., 2016) or using mini-cores or cut-open syringes (Coolen et al., 2013; Capo et al., 2021; Talas et al., 2021; Armbrecht et al., 2022). The sampling intervals vary according to the objectives of each study, the sedimentation rates in marine systems, and the weight/volume of samples required for further analysis. Subsampling for sedaDNA should be conducted rapidly in cold, still-air conditions to avoid exposure to oxygen after the core is opened (Elbaum et al., 2006; Ogata et al., 2021). A recent study shows that although sedaDNA can be obtained from cores that were split and stored in the dark at 4°C in plastic containers for several years, modern contamination from fungi and other eukaryotes are likely to be observed in such material (Selway et al., 2022). To avoid serious contamination issues, sediment cores should be transported and stored under cold, or even freezing temperatures. Alternatively, sediment cores for sedaDNA analyses could be subsampled onboard the vessel or immediately upon arrival at the laboratory and stored frozen (Llamas et al., 2017; Selway et al., 2022).
3.2 sedaDNA extraction
Due to the complexity of marine sediment composition and the wide range of target organisms to be analyzed, choosing a universally optimal sedaDNA extraction method is challenging (Armbrecht et al., 2020; Murchie et al., 2020; Kang et al., 2021). Extraction of the highly degraded fragments of sedaDNA has to ensure efficient removal of inhibitors, such as humic acids, while retaining a maximum amount of DNA in the solution. The efficiency of sedaDNA extraction depends on the complex and variable mineral composition of the sediment (Lekang et al., 2015), and DNA extraction methods (Kang et al., 2021). Due to the interaction between DNA molecules and sediment colloids, different extraction methods can influence the final quantity and quality of total DNA and biodiversity assessments.
Commercially available spin column-based DNA extraction kits (e.g., Qiagen, MP biomedicals, Omega, etc.) are commonly used in marine sedaDNA studies (Table S1). These products ensure a greater degree of uniformity and consistency than homemade solution-based protocols, especially when extracting DNA from a large number of samples. Yet, the latter, such as the phenol-chloroform method, have other advantages, including being cheaper than commercial kits, usually obtaining good quality and quantity of the extracted DNA, being easily optimized to the sample material, and preferentially recovering longer sedaDNA fragments (Direito et al., 2012; Armbrecht et al., 2020). However, solution-based DNA extraction protocols can be quite laborious, since toxic chemicals are used and all steps are to be processed manually in a fume hood, which can be inconvenient in a clean laboratory.
It should be noted that using different DNA extraction methods can significantly affect DNA yield and quality and species assemblages (Deiner et al., 2018; Pearman et al., 2020; Kang et al., 2021; Brauer and Bengtsson, 2022). For example, the Qiagen DNeasy PowerMax Soil Kit that allows extraction of up to 10 g of material is recommended by some studies for eukaryote biodiversity surveys from the surface (Pearman et al., 2020; Kang et al., 2021; Pawlowski et al., 2022) and downcore (Epp et al., 2019) sediment samples. The use of DNA-binding spin columns tends to selectively recover large DNA fragments (Armbrecht et al., 2020). Whereas non-column-based methods are more efficient for recovering smaller fragments, particularly when dealing with highly fragmented and degraded sedaDNA (Armbrecht et al., 2020). Thus, when selecting a DNA extraction protocol, it is important to consider various factors such as the type and quantity of samples, the intended purpose of the study, the availability of equipment, and financial limitations.
3.3 Metabarcoding vs metagenomics
Most sedaDNA studies to date have utilized metabarcoding approaches for the characterization of paleobiodiversity from sediment records. Metabarcoding consists of high-throughput sequencing of PCR-amplified marker genes, thus the success of DNA metabarcoding depends on the selection of an appropriate DNA marker. Ideally, such markers should have sufficiently conserved flanking primer-binding sites to minimize taxonomic biases during PCR amplification (Liu et al., 2020), distinguish targeted taxa, and possess a reference database for assigning taxonomy (Deagle et al., 2014). There are several marker genes commonly used for sedaDNA metabarcoding studies, including nuclear ribosomal genes for eukaryotic organisms (Pawłowska et al., 2014; De Schepper et al., 2019; Thomas et al., 2019; More et al., 2021), prokaryotes (Torti et al., 2018; Vuillemin et al., 2019), the mitochondrial cytochrome oxidase I (COI) gene for animals (Coolen, 2011; Der Sarkissian et al., 2017), the chloroplast ribulose bisphosphate carboxylase large chain (rcbL) gene for diatoms (Zimmermann et al., 2020; Armbrecht et al., 2021a), and major capsid protein (MCP) for double stranded DNA viruses infecting algae (Coolen, 2011). Based on the research questions, the sequence length of DNA markers must be considered: longer fragments (e.g., 200 bp to 500 bp) usually provide a better ability to discriminate taxa, while shorter fragments (e.g. 100 bp or less) are more likely to be preserved in sedaDNA (Figure 3A). In some cases, short fragments were also shown to provide effective taxonomic resolution (Meusnier et al., 2008; Pawłowska et al., 2014).
Currently, shotgun metagenomic sequencing is becoming more accessible and popular in marine sedaDNA research (Armbrecht et al., 2021a; Armbrecht et al., 2021b; Armbrecht et al., 2022; Selway et al., 2022) because it allows detection of all genomic fragments preserved in the sediment independent of length and without the primer bias of metabarcoding (Armbrecht et al., 2021a). In addition, it preserves DNA damage patterns and thus allows for assessing sedaDNA authenticity. On the other hand, the targeted group of organisms may constitute only a small portion of sequences in shotgun-sequencing samples, making this approach computationally challenging and costly. A recent study showed hybridization capture to be a promising compromise as it increases the yield of target eukaryote sedaDNA while maintaining the possibility for ancient DNA authentication via damage patterns (Armbrecht et al., 2021b). Since metagenomics approaches allow sequencing all DNA present in an ancient environmental sample, they have the potential to recover the full paleo-genetic record and provide the most holistic view of past marine biodiversity (Fellows Yates et al., 2021a; Armbrecht et al., 2022; Capo et al., 2022).
3.4 Bioinformatics challenges
The quality assessment and quality control of sequences produced by sedaDNA metabarcoding-metagenomics studies are particularly challenging given the potential damage of DNA preserved in the sediments. It is critical to select a bioinformatic pipeline that addresses the challenges specific to the recovery of sedaDNA signals. In the context of ancient environmental samples, ASV-based DADA2 (Callahan et al., 2019) may be more accurate than Operational Taxonomical Units (OTUs) for low abundance sedaDNA containing damage patterns (substitution) as it allows to detect small variants (Callahan et al., 2019; Porter and Hajibabaei, 2020). It is also important to ensure that the taxonomic classification of sedaDNA sequences is carried out using highly curated taxonomic reference databases. Although continuously updated (Guillou et al., 2012; Quast et al., 2012), such databases are largely incomplete, especially for marine meio- and microorganisms. The gaps in reference databases can be a key challenge associated with eDNA studies of both paleo- and modern communities since those are often dominated by taxonomically unassigned or unknown sequence fragments (Vargas et al., 2015; Cordier et al., 2022). The situation is also difficult in the case of metagenomic studies targeting marine eukaryotes as the number of reference genomes remains limited (Delmont et al., 2022).
In response to the rapidly growing production of ancient metagenomics data, new bioinformatics techniques have been developed to deal with the particular features of ancient DNA sequences. These techniques encompass a range of approaches, such as the integration of damage detection algorithms, e.g. in mapDamage (Jónsson et al., 2013; Kistler et al., 2017; Weyrich et al., 2017) and metaDMG (Michelsen et al., 2022). They also involve optimizing ancient DNA mapping through the analysis of cytosine residues deamination, utilizing algorithms such as BWA (Schubert et al., 2012; Weyrich et al., 2017), PyDamage (Borry et al., 2021). Furthermore, new tools have been developed to enhance the accuracy of taxonomic assignment of metagenomic reads (PIA, Cribdon et al. (2020), and for processing and analyzing ancient metagenomics shotgun data, specifically targeting ultra-short molecules, (e.g. Collin et al. (2020); Fellows Yates et al. (2021b); Pochon et al. (2022); Neuenschwander et al. (2023)). However, identifying ancient sequences is still a challenge due to the lack of standard bioinformatics pipelines to analyze DNA metabarcoding or shotgun metagenomics data. Using different bioinformatics procedures or genetic databases that lack consistent standards increases ambiguity and bias. Additionally, to ensure in silico reproducibility and facilitate further research, it is crucial to emphasize the need for publishing comprehensive bioinformatics analysis reports. The application and optimization of bioinformatics pipelines should be the focus of future research to accurately identify and authenticate marine microbiota in sedaDNA.
4 Current applications of the marine sedaDNA approach
The main application of marine sedaDNA is the reconstruction of past biodiversity in relation to environmental changes at geological time scales (Figure 4). In this context, the sedaDNA approach provides a unique insight into the marine paleo-ecological communities. Its application allows reconstructing a detailed record of community changes from individuals to populations and species that occurred across time, increasing the accuracy of prediction models in anticipating how climate/environmental change affects biodiversity (De Schepper et al., 2019; Epp, 2019). Several studies have demonstrated the potential of sedaDNA for screening genetic signals of multiple taxa (as in Figure 3B), including non-fossilizing organisms (Pawłowska et al., 2014; Selway et al., 2022; Barrenechea Angeles et al., 2023). Most studies focused on marine microbial and meiofaunal communities, revealing a huge and largely undescribed diversity of viruses (Coolen, 2011; Pratas and Pinho, 2018; Zheng et al., 2021), bacteria (Hou et al., 2014; More et al., 2019; Nwosu et al., 2021), archaea (Torti et al., 2018; Vuillemin et al., 2019), and protists (Lejzerowicz et al., 2013; Pawłowska et al., 2014; More et al., 2018; Zimmermann et al., 2020; Egge et al., 2021).
The aims of sedaDNA studies vary from comparing the diversity of molecular and microfossil assemblages to analyzing the past diversity of non-fossilized taxa. Several studies demonstrated the lack of congruence between molecular and microfossil assemblages (Pawłowska et al., 2014; Barrenechea Angeles et al., 2020). This lack of congruence could be explained by difficulties in extracting DNA from hard-shelled, fossilized taxa, multiple copies of the target gene in the genome, PCR primers biases, or PCR inhibitors, e.g., in the case of several planktonic taxa (Barrenechea Angeles et al., 2020; Pierella Karlusich et al., 2023). Another possible explanation would be that the non-fossilized taxa are much more abundant, and their DNA dominates in sediment samples (Lejzerowicz et al., 2013). This could be the case for non-fossilized monothalamous foraminifera and the acantharian radiolarians that usually are more dominant in sedaDNA datasets compared to fossilizing species of the same lineages (Lejzerowicz et al., 2013; Pawłowska et al., 2014). Indeed, they also dominate in modern sediment analyses (Lecroq et al., 2011; Pawłowska et al., 2014; Cordier et al., 2022). Despite this lack of congruence in community composition, the patterns inferred from metabarcoding and microfossil data are often similar. This was the case in one study of planktonic foraminifera microfossil and metabarcoding assemblages that exhibited congruent regional biogeographical patterns (Barrenechea Angeles et al., 2020). Similarly, sedaDNA data of non-fossilized soft-walled foraminifera show comparable patterns as microfossil foraminiferal assemblage in 800-year-old fjord sediments (Pawłowska et al., 2014).
The most promising application of sedaDNA is to investigate past climates and their impact on oceanographic circulation and species migration. Until now, these studies were mainly based on macro- and microfossils, ignoring a large diversity of non-fossilized species, in particular those belonging to microbial and meiofaunal communities, which are essential for ecosystem functioning. This issue could be overcome by analyzing sedaDNA from marine sediment cores. For example, changes in ocean circulation patterns were investigated by targeting sedaDNA of non-fossilized foraminifera in cores collected east of Svalbard (Pawłowska et al., 2020a). Several studies integrate sedaDNA data with geochemical proxies, including stable and radiogenic isotopes (More et al., 2018; Voldstad et al., 2020), and biomarkers (De Schepper et al., 2019). For example, through analysis of sedaDNA and hydrogen isotopes of haptophyte-derived alkenones preserved in early Holocene oxygenated lacustrine and mid-to-late-Holocene anoxic coastal Black Sea sediments, planktonic community structures were reconstructed and associated with Holocene climate phases and transitions (Coolen et al., 2013). Paired analysis of sedaDNA and the sea ice biomarker IP25 was used as a proxy for sea-ice reconstructions by targeting diatom sedaDNA composition in the Fram Strait (Zimmermann et al., 2020), Subarctic North Pacific (Zimmermann et al., 2021), and to trace a sea-ice dinoflagellate east of Greenland (De Schepper et al., 2019). All of these studies provide complementary insights into ecosystem-climate histories, extending our current view of marine communities beyond the diversity found in modern populations and advancing our understanding of their past biogeographic patterns and adaptations to new environmental conditions.
At a shorter timescale, marine sedaDNA studies also represent a promising complement to traditional biomonitoring surveys. Currently, modern sedimentary DNA metabarcoding is becoming a routine tool for monitoring the human impact on marine systems e.g., through construction, overexploitation, agriculture, habitat loss, and pollution (Bálint et al., 2018; Cordier et al., 2021; Pawlowski et al., 2021). A few studies have shown that the sedaDNA approach can be used to investigate the impact of anthropogenic activities on the ecosystem over the past century. One of these studies recovered the composition of marine plankton communities from hundred-year-old sediment samples and showed irreversible shifts after the cumulative effect of war and agricultural pollution (Siano et al., 2021). Another study demonstrated dramatic ecosystem changes resulting from a multi-level cascade effect of impacts associated with industrial activities, urbanization, water circulation, and land-use changes in one of the most polluted marine sites in Europe (Barrenechea Angeles et al., 2023). Both studies demonstrate the potential of sedaDNA to elucidate the effects of human pollution on marine communities, contributing to the reconstruction of reference conditions and helping the conservation and management of marine and coastal ecosystems.
5 Future perspectives
Future developments in the marine sedaDNA research field will make it easier to integrate paleogenetic signals preserved in the marine seafloor with other proxies (Figure 4). Based on the best understanding of each proxy, we can use the strengths of each approach to their best advantage (i.e., complementary patterns of DNA metabarcoding and morphology). The marine sedaDNA allows recovering a wide range of organisms that will provide valuable material for searching for new paleo-bioindicators. These could include non-fossilized microbial and meiofaunal taxa that present various kinds of ecological characteristics, or the genotypes of fossilized species, the distribution of which can be used to detect paleo-environmental changes (Pawłowska et al., 2020b). In the future, sedaDNA studies could also pay more attention to marine mammals, fish, and macroinvertebrates, traces of which can be detected in sediment samples (Kuwae et al., 2020).
However, several technical challenges inherent to sedaDNA research need to be considered for future developments in the field. First, incomplete reference databases are the major factor limiting the assignment of sequences to taxonomic names and might lead to divergent results (Balvociute and Huson, 2017). The continuous addition of new reference sequences from modern marine organisms to taxonomic databases is important to further complete the taxonomic picture of marine paleo communities. Second, marine sedaDNA sampling and laboratory procedures may need further optimization (Armbrecht et al., 2019). In particular, the combination of amplicon sequencing (metabarcoding) with metagenomics through the further development of HTS technologies may improve the authentication of ancient signals in genetic data (Armbrecht et al., 2021a) and allow getting more diverse data from assembled sequences, at least for prokaryotes. Finally, more studies are needed to evaluate the limitations of the sedaDNA approach, especially the time limits of DNA preservation in marine sediments. There is also a lack of evidence on which physicochemical characteristics of sediments are optimal for long-time DNA preservation.
Given the developments in the field, we foresee that the sedaDNA approach will be rapidly integrated into routine paleoceanographic research. The approach is technologically mature, thus the costs of including a sedaDNA module in research projects should continue to decrease, thereby increasing the feasibility of sedaDNA as a regular inclusion in multi-proxy investigations. Even though there are still some challenging issues to be solved, the information provided by the sedaDNA data is highly valuable. The DNA-based holistic overview of biodiversity changes through time is unique. Its various applications, from the studies of climate changes and water mass circulation at geological time scales to the monitoring of recent anthropogenic impacts, are of key importance to understanding the past and present state of marine ecosystems. Further development of the sedaDNA field and its wider integration will not only help to improve our knowledge of past changes affecting the ocean and coastal ecosystems, but it will also help to establish and optimize strategies for their conservation and management.
Author contributions
NL-N, JaP and JoP conceived the review; all authors drafted the manuscript. MG and DD drew the figures. All authors contributed to the article and approved the submitted version.
Funding
The research leading to these results has been funded by Norwegian Financial Mechanism for 2014-2021, project no. 2019/34/H/ST10/00682 in the frame of project “Sedimentary ancient DNA - a new proxy to investigate the impact of environmental change on past and present biodiversity in Nordic Seas”. IBA was supported by the Norwegian Petroleum Directorate and the project AKMA, project no. 287869.
Conflict of interest
The authors declare that the research was conducted in the absence of any commercial or financial relationships that could be construed as a potential conflict of interest.
Publisher’s note
All claims expressed in this article are solely those of the authors and do not necessarily represent those of their affiliated organizations, or those of the publisher, the editors and the reviewers. Any product that may be evaluated in this article, or claim that may be made by its manufacturer, is not guaranteed or endorsed by the publisher.
Supplementary material
The Supplementary Material for this article can be found online at: https://www.frontiersin.org/articles/10.3389/fmars.2023.1185435/full#supplementary-material
References
Allan E. A., Dibenedetto M. H., Lavery A. C., Govindarajan A. F., Zhang W. G. (2021). Modeling characterization of the vertical and temporal variability of environmental DNA in the mesopelagic ocean. Sci. Rep. 11, 21273. doi: 10.1038/s41598-021-00288-5
Andruszkiewicz E. A., Sassoubre L. M., Boehm A. B. (2017). Persistence of marine fish environmental DNA and the influence of sunlight. PloS One 12, e0185043. doi: 10.1371/journal.pone.0185043
Armbrecht L. H., Coolen M. J. L., Lejzerowicz F., George S. C., Negandhi K., Suzuki Y., et al. (2019). Ancient DNA from marine sediments: precautions and considerations for seafloor coring, sample handling and data generation. Earth-Sci. Rev. 196, 102887. doi: 10.1016/j.earscirev.2019.102887
Armbrecht L., Eisenhofer R., Utge J., Sibert E. C., Rocha F., Ward R., et al. (2021a). Paleo-diatom composition from Santa Barbara Basin deep-sea sediments: a comparison of 18S-V9 and diat-rbcL metabarcoding vs shotgun metagenomics. ISME Commun. 1, 66. doi: 10.1038/s43705-021-00070-8
Armbrecht L., Hallegraeff G., Bolch C. J. S., Woodward C., Cooper A. (2021b). Hybridisation capture allows DNA damage analysis of ancient marine eukaryotes. Sci. Rep. 11, 3220. doi: 10.1038/s41598-021-82578-6
Armbrecht L., Herrando-Pérez S., Eisenhofer R., Hallegraeff G. M., Bolch C. J. S., Cooper A. (2020). An optimized method for the extraction of ancient eukaryote DNA from marine sediments. Mol. Ecol. Resour. 20, 906–919. doi: 10.1111/1755-0998.13162
Armbrecht L., Weber M. E., Raymo M. E., Peck V. L., Williams T., Warnock J., et al. (2022). Ancient marine sediment DNA reveals diatom transition in Antarctica. Nat. Commun. 13, 5787. doi: 10.1038/s41467-022-33494-4
Aubry A. M. R., De Schepper S., De Vernal A. (2020). Dinocyst and acritarch biostratigraphy of the late Pliocene to early Pleistocene at integrated ocean drilling program site U1307 in the Labrador Sea. J. Micropalaeontol. 39, 41–60. doi: 10.5194/jm-39-41-2020
Bach L. T., Boxhammer T., Larsen A., Hildebrandt N., Schulz K. G., Riebesell U. (2016). Influence of plankton community structure on the sinking velocity of marine aggregates. Glob. Biogeochem. Cycles 30, 1145–1165. doi: 10.1002/2016GB005372
Bach L. T., Stange P., Taucher J., Achterberg E. P., Algueró-Muñiz M., Horn H., et al. (2019). The influence of plankton community structure on sinking velocity and remineralization rate of marine aggregates. Global Biogeochem. Cycles 33, 971–994. doi: 10.1029/2019GB006256
Backman J., Fornaciari E., Rio D. (2009). Biochronology and paleoceanography of late Pleistocene and Holocene calcareous nannofossil abundances across the Arctic Basin. Mar. Micropaleontol. 72, 86–98. doi: 10.1016/j.marmicro.2009.04.001
Bálint M., Pfenninger M., Grossart H.-P., Taberlet P., Vellend M., Leibold M. A., et al. (2018). Environmental DNA time series in ecology. Trends Ecol. Evol. 33, 945–957. doi: 10.1016/j.tree.2018.09.003
Balvociute M., Huson D. H. (2017). SILVA, RDP, Green genes, NCBI and OTT - how do these taxonomies compare? BMC Genom. 18, 114. doi: 10.1186/s12864-017-3501-4
Barrenechea Angeles I., Lejzerowicz F., Cordier T., Scheplitz J., Kucera M., Ariztegui D., et al. (2020). Planktonic foraminifera eDNA signature deposited on the seafloor remains preserved after burial in marine sediments. Sci. Rep. 10, 20351. doi: 10.1038/s41598-020-77179-8
Barrenechea Angeles I., Romero-Martínez M. L., Cavaliere M., Varrella S., Francescangeli F., Piredda R., et al. (2023). Encapsulated in sediments: eDNA deciphers the ecosystem history of one of the most polluted European marine sites. Environ. Int. 172, 107738. doi: 10.1016/j.envint.2023.107738
Boere A. C., Abbas B., Rijpstra W. I. C., Versteegh G. J. M., Volkman J. K., Sinninghe Damsté J. S., et al. (2009). Late-Holocene succession of dinoflagellates in an Antarctic fjord using a multi-proxy approach: paleoenvironmental genomics, lipid biomarkers and palynomorphs. Geobiology 7, 265–281. doi: 10.1111/j.1472-4669.2009.00202.x
Boere A. C., Rijpstra W. I. C., De Lange G. J., Sinninghe Damsté J. S., Coolen M. J. L. (2011). Preservation potential of ancient plankton DNA in Pleistocene marine sediments. Geobiology 9, 377–393. doi: 10.1111/j.1472-4669.2011.00290.x
Borry M., Hübner A., Rohrlach A. B., Warinner C. (2021). PyDamage: automated ancient damage identification and estimation for contigs in ancient DNA de novo assembly. PeerJ 9, e11845. doi: 10.7717/peerj.11845
Brauer A., Bengtsson M. M. (2022). DNA Extraction bias is more pronounced for microbial eukaryotes than for prokaryotes. MicrobiologyOpen 11, e1323. doi: 10.1002/mbo3.1323
Cael B. B., White A. E. (2020). Sinking versus suspended particle size distributions in the North Pacific subtropical gyre. Geophys. Res. Lett. 47, e2020GL087825. doi: 10.1029/2020GL087825
Callahan B. J., Wong J., Heiner C., Oh S., Theriot C. M., Gulati A. S., et al. (2019). High-throughput amplicon sequencing of the full-length 16S rRNA gene with single-nucleotide resolution. Nucleic Acids Res. 47, e103–e103. doi: 10.1093/nar/gkz569
Capo E., Giguet-Covex C., Rouillard A., Nota K., Heintzman P. D., Vuillemin A., et al. (2021). Lake sedimentary DNA research on past terrestrial and aquatic biodiversity: overview and recommendations. Quaternary 4, 6. doi: 10.3390/quat4010006
Capo E., Monchamp M.-E., Coolen M. J. L., Domaizon I., Armbrecht L., Bertilsson S. (2022). Environmental paleomicrobiology: using DNA preserved in aquatic sediments to its full potential. Environ. Microbiol 24, 2201–2209. doi: 10.1111/1462-2920.15913
Collin T. C., Drosou K., O Riordan J. D., Meshveliani T., Pinhasi R., Feeney R. N. M. (2020). An open-sourced bioinformatic pipeline for the processing of next-generation sequencing derived nucleotide reads: identification and authentication of ancient metagenomic DNA. bioRxiv. doi: 10.1101/2020.04.20.050369
Collins R. A., Wangensteen O. S., O’gorman E. J., Mariani S., Sims D. W., Genner M. J. (2018). Persistence of environmental DNA in marine systems. Commun. Biol. 1, 185. doi: 10.1038/s42003-018-0192-6
Coolen M. J. (2011). 7000 years of Emiliania huxleyi viruses in the Black Sea. Science 333, 451–452. doi: 10.1126/science.1200072
Coolen M. J. L., Boere A., Abbas B., Baas M., Wakeham S. G., Sinninghe Damsté J. S. (2006). Ancient DNA derived from alkenone-biosynthesizing haptophytes and other algae in Holocene sediments from the Black Sea. Paleoceanography 21. doi: 10.1029/2005PA001188
Coolen M. J. L., Muyzer G., Rijpstra W. I. C., Schouten S., Volkman J. K., Sinninghe Damsté J. S. (2004). Combined DNA and lipid analyses of sediments reveal changes in Holocene haptophyte and diatom populations in an Antarctic lake. Earth Planet. Sci. Lett. 223, 225–239. doi: 10.1016/j.epsl.2004.04.014
Coolen M. J., Orsi W. D., Balkema C., Quince C., Harris K., Sylva S. P., et al. (2013). Evolution of the plankton paleome in the Black Sea from the deglacial to anthropocene. Proc. Natl. Acad. Sci. U.S.A. 110, 8609–8614. doi: 10.1073/pnas.1219283110
Coolen M. J. L., Overmann J. (1998). Analysis of subfossil molecular remains of purple sulfur bacteria in a lake sediment. Appl. Environ. Microbiol. 64, 4513–4521. doi: 10.1128/AEM.64.11.4513-4521.1998
Coolen M. J. L., Overmann J. (2007). 217 000-year-old DNA sequences of green sulfur bacteria in Mediterranean sapropels and their implications for the reconstruction of the paleoenvironment. Environ. Microbiol. 9, 238–249. doi: 10.1111/j.1462-2920.2006.01134.x
Coolen M. J. L., Saenz J. P., Giosan L., Trowbridge N. Y., Dimitrov P., Dimitrov D., et al. (2009). DNA and lipid molecular stratigraphic records of haptophyte succession in the Black Sea during the Holocene. Earth Planet. Sci. Lett. 284, 610–621. doi: 10.1016/j.epsl.2009.05.029
Cordier T., Alonso-Sáez L., Apothéloz-Perret-Gentil L., Aylagas E., Bohan D. A., Bouchez A., et al. (2021). Ecosystems monitoring powered by environmental genomics: a review of current strategies with an implementation roadmap. Mol. Ecol. 30, 2937–2958. doi: 10.1111/mec.15472
Cordier T., Angeles I. B., Henry N., Lejzerowicz F., Berney C., Morard R., et al. (2022). Patterns of eukaryotic diversity from the surface to the deep-ocean sediment. Sci. Adv. 8, eabj9309. doi: 10.1126/sciadv.abj9309
Corinaldesi C., Barucca M., Luna G. M., Dell’anno A. (2011). Preservation, origin and genetic imprint of extracellular DNA in permanently anoxic deep-sea sediments. Mol. Ecol. 20, 642–654. doi: 10.1111/j.1365-294X.2010.04958.x
Corinaldesi C., Beolchini F., Dell’anno A. (2008). Damage and degradation rates of extracellular DNA in marine sediments: implications for the preservation of gene sequences. Mol. Ecol. 17, 3939–3951. doi: 10.1111/j.1365-294X.2008.03880.x
Cribdon B., Ware R., Smith O., Gaffney V., Allaby R. G. (2020). PIA: more accurate taxonomic assignment of metagenomic data demonstrated on sedaDNA from the North Sea. Front. Ecol. Evol. 8. doi: 10.3389/fevo.2020.00084
Deagle B. E., Jarman S. N., Coissac E., Pompanon F., Taberlet P. (2014). DNA metabarcoding and the cytochrome c oxidase subunit I marker: not a perfect match. Biol. Lett. 10 (9), 20140562. doi: 10.1098/rsbl.2014.0562
Deiner K., Lopez J., Bourne S., Holman L., Seymour M., Grey E. K., et al. (2018). Optimising the detection of marine taxonomic richness using environmental DNA metabarcoding: the effects of filter material, pore size and extraction method. Metabarcoding Metagenom. 2, e28963. doi: 10.3897/mbmg.2.28963
Del Carmen Gomez Cabrera M., Young J. M., Roff G., Staples T., Ortiz J. C., Pandolfi J. M., et al. (2019). Broadening the taxonomic scope of coral reef palaeoecological studies using ancient DNA. Mol. Ecol. 28, 2636–2652. doi: 10.1111/mec.15038
Delmont T. O., Gaia M., Hinsinger D. D., Frémont P., Vanni C., Fernandez-Guerra A., et al. (2022). Functional repertoire convergence of distantly related eukaryotic plankton lineages abundant in the sunlit ocean. Cell Genom. 2, 100123. doi: 10.1016/j.xgen.2022.100123
Der Sarkissian C., Pichereau V., Dupont C., Ilsoe P. C., Perrigault M., Butler P., et al. (2017). Ancient DNA analysis identifies marine mollusc shells as new metagenomic archives of the past. Mol. Ecol. Resour. 17, 835–853. doi: 10.1111/1755-0998.12679
De Schepper S., Ray J. L., Skaar K. S., Sadatzki H., Ijaz U. Z., Stein R., et al. (2019). The potential of sedimentary ancient DNA for reconstructing past sea ice evolution. ISME J. 13, 2566–2577. doi: 10.1038/s41396-019-0457-1
Direito S. O. L., Marees A., Röling W. F. M. (2012). Sensitive life detection strategies for low-biomass environments: optimizing extraction of nucleic acids adsorbing to terrestrial and Mars analogue minerals. FEMS Microbiol. Ecol. 81, 111–123. doi: 10.1111/j.1574-6941.2012.01325.x
Egge E., Elferink S., Vaulot D., John U., Bratbak G., Larsen A., et al. (2021). An 18S V4 rRNA metabarcoding dataset of protist diversity in the Atlantic inflow to the Arctic Ocean, through the year and down to 1000 m depth. Earth Syst. Sci. Data 13, 4913–4928. doi: 10.5194/essd-13-4913-2021
Elbaum R., Melamed-Bessudo C., Boaretto E., Galili E., Lev-Yadun S., Levy A. A., et al. (2006). Ancient olive DNA in pits: preservation, amplification and sequence analysis. J. Archaeol. Sci. 33, 77–88. doi: 10.1016/j.jas.2005.06.011
Ellegaard M., Clokie M. R. J., Czypionka T., Frisch D., Godhe A., Kremp A., et al. (2020). Dead or alive: sediment DNA archives as tools for tracking aquatic evolution and adaptation. Commun. Biol. 3, 169. doi: 10.1038/s42003-020-0899-z
Ellegaard M., Dale B., Mertens K. N., Pospelova V., Ribeiro S. (2017). “Dinoflagellate cysts as proxies for Holocene environmental change in estuaries: diversity, abundance and morphology,” in Applications of paleoenvironmental techniques in estuarine studies. Eds. Weckström K., Saunders K. M., Gell P. A., Skilbeck C. G. (Dordrecht: Springer Netherlands), 295–312.
Epp L. S. (2019). A global perspective for biodiversity history with ancient environmental DNA. Mol. Ecol. 28, 2456–2458. doi: 10.1111/mec.15118
Epp L. S., Zimmermann H. H., Stoof-Leichsenring K. R. (2019). Sampling and extraction of ancient DNA from sediments. Methods Mol. Biol. 1963, 31–44. doi: 10.1007/978-1-4939-9176-1_5
Fellows Yates J. A., Andrades Valtueña A., Vågene Å.J., Cribdon B., Velsko I. M., Borry M., et al. (2021a). Community-curated and standardised metadata of published ancient metagenomic samples with AncientMetagenomeDir. Sci. Data 8, 31. doi: 10.1038/s41597-021-00816-y
Fellows Yates J. A., Lamnidis T. C., Borry M., Andrades Valtueña A., Fagernäs Z., Clayton S., et al. (2021b). Reproducible, portable, and efficient ancient genome reconstruction with nf-core/eager. PeerJ 9, e10947. doi: 10.7717/peerj.10947
Fulton T. L., Shapiro B. (2019). “Setting up an ancient DNA laboratory,” in Ancient DNA: methods and protocols. Eds. Shapiro B., Barlow A., Heintzman P. D., Hofreiter M., Paijmans J. L. A., Soares A. E. R. (New York, NY: Springer New York), 1–13.
Furukawa Y., Horiuchi M., Kakegawa T. (2013). Selective stabilization of ribose by borate. Orig. Life Evol. Biosph. 43, 353–361. doi: 10.1007/s11084-013-9350-5
Guillou L., Bachar D., Audic S., Bass D., Berney C., Bittner L., et al. (2012). The Protist Ribosomal Reference database (PR2): a catalog of unicellular eukaryote small sub-unit rRNA sequences with curated taxonomy. Nucleic Acids Res. 41, D597–D604. doi: 10.1093/nar/gks1160
Gussone N., Friedrich O. (2018). Cretaceous calcareous dinoflagellate cysts as recorder of δ44/40Caseawater and paleo-temperature using Sr/Ca thermometry. Chem. Geol. 488, 138–148. doi: 10.1016/j.chemgeo.2018.04.020
Hennissen J., Head M. J., De Schepper S., Groeneveld J. (2017). Dinoflagellate cyst paleoecology during the Pliocene–Pleistocene climatic transition in the North Atlantic. Palaeogeogr. Palaeoclimatol. Palaeoecol. 470, 81–108. doi: 10.1016/j.palaeo.2016.12.023
Herndl G. J., Reinthaler T. (2013). Microbial control of the dark end of the biological pump. Nat. Geosci. 6, 718–724. doi: 10.1038/ngeo1921
Hou W., Dong H., Li G., Yang J., Coolen M. J. L., Liu X., et al. (2014). Identification of photosynthetic plankton communities using sedimentary ancient DNA and their response to late-Holocene climate change on the Tibetan Plateau. Sci. Rep. 4, 6648. doi: 10.1038/srep06648
Iversen M. H., Ploug H. (2010). Ballast minerals and the sinking carbon flux in the ocean: carbon-specific respiration rates and sinking velocity of marine snow aggregates. Biogeosciences 7, 2613–2624. doi: 10.5194/bg-7-2613-2010
Jacques G., Luc B. (2007). “Chapter ten coccolithophores: from extant populations to fossil assemblages,” in Developments in marine geology. Eds. Hillaire–Marcel C., Vernal A. (Elsevier), 409–439.
Jónsson H., Ginolhac A., Schubert M., Johnson P. L. F., Orlando L. (2013). mapDamage2.0: fast approximate Bayesian estimates of ancient DNA damage parameters. Bioinformatics 29, 1682–1684. doi: 10.1093/bioinformatics/btt193
Kallmeyer J., Pockalny R., Adhikari R. R., Smith D. C., D’hondt S. (2012). Global distribution of microbial abundance and biomass in subseafloor sediment. Proc. Natl. Acad. Sci. U.S.A. 109, 16213–16216. doi: 10.1073/pnas.1203849109
Kang W., Anslan S., Börner N., Schwarz A., Schmidt R., Künzel S., et al. (2021). Diatom metabarcoding and microscopic analyses from sediment samples at Lake Nam Co, Tibet: the effect of sample-size and bioinformatics on the identified communities. Ecol. Indic. 121, 107070. doi: 10.1016/j.ecolind.2020.107070
Kelly R. P., Gallego R., Jacobs-Palmer E. (2018). The effect of tides on nearshore environmental DNA. PeerJ 6, e4521. doi: 10.7717/peerj.4521
Kirkpatrick J. B., Walsh E. A., D’hondt S. (2016). Fossil DNA persistence and decay in marine sediment over hundred-thousand-year to million-year time scales. Geology 44, 615–618. doi: 10.1130/G37933.1
Kistler L., Ware R., Smith O., Collins M., Allaby R. G. (2017). A new model for ancient DNA decay based on paleogenomic meta-analysis. Nucleic Acids Res. 45, 6310–6320. doi: 10.1093/nar/gkx361
Kujawa A., Łącka M., Szymańska N., Pawłowska J., Telesiński M. M., Zajączkowski M. (2021). Could Norwegian fjords serve as an analogue for the future of the Svalbard fjords? state and fate of high latitude fjords in the face of progressive “atlantification”. Polar Biol. 44, 2217–2233. doi: 10.1007/s00300-021-02951-z
Kuwae M., Tamai H., Doi H., Sakata M. K., Minamoto T., Suzuki Y. (2020). Sedimentary DNA tracks decadal-centennial changes in fish abundance. Commun. Biol. 3, 558. doi: 10.1038/s42003-020-01282-9
Łącka M., Cao M., Rosell-Melé A., Pawłowska J., Kucharska M., Forwick M., et al. (2019). Postglacial paleoceanography of the western Barents Sea: implications for alkenone-based sea surface temperatures and primary productivity. Quat. Sci. Rev. 224, 105973. doi: 10.1016/j.quascirev.2019.105973
Łącka M., Michalska D., Pawłowska J., Szymańska N., Szczuciński W., Forwick M., et al. (2020). Multiproxy paleoceanographic study from the western Barents Sea reveals dramatic younger dryas onset followed by oscillatory warming trend. Sci. Rep. 10, 15667. doi: 10.1038/s41598-020-72747-4
Lecroq B., Lejzerowicz F., Bachar D., Christen R., Esling P., Baerlocher L., et al. (2011). Ultra-deep sequencing of foraminiferal microbarcodes unveils hidden richness of early monothalamous lineages in deep-sea sediments. Proc. Natl. Acad. Sci. U.S.A. 108, 13177–13182. doi: 10.1073/pnas.1018426108
Lejzerowicz F., Esling P., Majewski W., Szczucinski W., Decelle J., Obadia C., et al. (2013). Ancient DNA complements microfossil record in deep-sea subsurface sediments. Biol. Lett. 9, 20130283. doi: 10.1098/rsbl.2013.0283
Lekang K., Thompson E., Troedsson C. (2015). A comparison of DNA extraction methods for biodiversity studies of eukaryotes in marine sediments. Aquat. Microb. Ecol. 75, 15–25. doi: 10.3354/ame01741
Liu M., Clarke L. J., Baker S. C., Jordan G. J., Burridge C. P. (2020). A practical guide to DNA metabarcoding for entomological ecologists. Ecol. Entomol. 45, 373–385. doi: 10.1111/een.12831
Llamas B., Valverde G., Fehren-Schmitz L., Weyrich L. S., Cooper A., Haak W. (2017). From the field to the laboratory: controlling DNA contamination in human ancient DNA research in the high-throughput sequencing era. STAR: Sci. Technol. Archaeol. Res. 3, 1–14. doi: 10.1080/20548923.2016.1258824
Locarnini M., Mishonov A., Baranova O., Boyer T., Zweng M., Garcia H., et al. (2018). “World ocean atlas 2018,” in Temperature, vol. 1. (Silver Spring, MD: NOAA Atlas NESDIS), 81.
Lorenz M. G., Wackernagel W. (1987). Adsorption of DNA to sand and variable degradation rates of adsorbed DNA. Appl. Environ. Microbiol. 53, 2948–2952. doi: 10.1128/aem.53.12.2948-2952.1987
Machado G. M. V., Bastos A. C., Da Silva De Freitas A., Baptista Neto J. A. (2018). Sedimentary, geochemical and micropaleontological responses to sea level variations in the Vitoria Estuary, Espírito Santo. Radiocarbon 60, 583–600. doi: 10.1017/RDC.2018.5
Marino M., Rodrigues T., Quivelli O., Girone A., Maiorano P., Bassinot F. (2022). Paleoproductivity proxies and alkenone precursors in the Western Mediterranean during the early-middle Pleistocene transition. Palaeogeogr. Palaeoclimatol. Palaeoecol. 601, 111104. doi: 10.1016/j.palaeo.2022.111104
Mccartin L. J., Vohsen S. A., Ambrose S. W., Layden M., Mcfadden C. S., Cordes E. E., et al. (2022). Temperature controls eDNA persistence across physicochemical conditions in seawater. Environ. Sci. Technol. 56, 8629–8639. doi: 10.1021/acs.est.2c01672
Mcclymont E. L., Ford H. L., Ho S. L., Tindall J. C., Haywood A. M., Alonso-Garcia M., et al. (2020). Lessons from a high-CO2 world: an ocean view from ∼ 3 million years ago. Climate Past 16, 1599–1615. doi: 10.5194/cp-16-1599-2020
Mcgann M., Powell C. L. (2022). Impact of climate change on mollusks and other invertebrate resources at the Dominican University of California archaeological site (CA-MRN-254), Marin County, California. Quat. Int. 628, 64–78. doi: 10.1016/j.quaint.2022.02.030
Meusnier I., Singer G. A., Landry J. F., Hickey D. A., Hebert P. D., Hajibabaei M. (2008). A universal DNA mini-barcode for biodiversity analysis. BMC Genom. 9, 214. doi: 10.1186/1471-2164-9-214
Michelsen C., Pedersen M. W., Fernandez-Guerra A., Zhao L., Petersen T. C., Korneliussen T. S. (2022). metaDMG – a fast and accurate ancient DNA damage toolkit for metagenomic data. bioRxiv 2022, 2012. doi: 10.1101/2022.12.06.519264
Miettinen A. (2018). Diatoms in Arctic regions: potential tools to decipher environmental changes. Polar Sci. 18, 220–226. doi: 10.1016/j.polar.2018.04.001
Morard R., Lejzerowicz F., Darling K. F., Lecroq-Bennet B., Winther Pedersen M., Orlando L., et al. (2017). Planktonic foraminifera-derived environmental DNA extracted from abyssal sediments preserves patterns of plankton macroecology. Biogeosciences 14, 2741–2754. doi: 10.5194/bg-14-2741-2017
More K. D., Giosan L., Grice K., Coolen M. J. L. (2019). Holocene paleodepositional changes reflected in the sedimentary microbiome of the Black Sea. Geobiology 17, 436–448. doi: 10.1111/gbi.12338
More K. D., Orsi W. D., Galy V., Giosan L., He L., Grice K., et al. (2018). A 43 kyr record of protist communities and their response to oxygen minimum zone variability in the Northeastern Arabian Sea. Earth Planet. Sci. Lett. 496, 248–256. doi: 10.1016/j.epsl.2018.05.045
More K. D., Wuchter C., Irigoien X., Tierney J. E., Giosan L., Grice K., et al. (2021). Subseafloor archaea reflect 139 kyrs of paleodepositional changes in the northern Red Sea. Geobiology 19, 162–172. doi: 10.1111/gbi.12421
Murchie T. J., Kuch M., Duggan A. T., Ledger M. L., Roche K., Klunk J., et al. (2020). Optimizing extraction and targeted capture of ancient environmental DNA for reconstructing past environments using the PalaeoChip Arctic-1.0 bait-set. Quat. Res. 99, 305–328. doi: 10.1017/qua.2020.59
Murrell M. C., Lores E. M. (2004). Phytoplankton and zooplankton seasonal dynamics in a subtropical estuary: importance of cyanobacteria. J. Plankton Res. 26, 371–382. doi: 10.1093/plankt/fbh038
Neuenschwander S., Cruz Dávalos D. I., Anchieri L., Sousa Da Mota B., Bozzi D., Rubinacci S., et al. (2023). Mapache: a flexible pipeline to map ancient DNA. Bioinformatics 39, btad028. doi: 10.1093/bioinformatics/btad028
Nooteboom P. D., Bijl P. K., Van Sebille E., Von Der Heydt A. S., Dijkstra H. A. (2019). Transport bias by ocean currents in sedimentary microplankton assemblages: implications for paleoceanographic reconstructions. Paleoceanogr. Paleoclimatology 34, 1178–1194. doi: 10.1029/2019PA003606
Nwosu E. C., Brauer A., Kaiser J., Horn F., Wagner D., Liebner S. (2021). Evaluating sedimentary DNA for tracing changes in cyanobacteria dynamics from sediments spanning the last 350 years of Lake Tiefer See, NE Germany. J. Paleolimnol. 66, 279–296. doi: 10.1007/s10933-021-00206-9
Ogata M., Masuda R., Harino H., Sakata M. K., Hatakeyama M., Yokoyama K., et al. (2021). Environmental DNA preserved in marine sediment for detecting jellyfish blooms after a tsunami. Sci. Rep. 11, 16830. doi: 10.1038/s41598-021-94286-2
Okabe S., Shimazu Y. (2007). Persistence of host-specific bacteroides–prevotella 16S rRNA genetic markers in environmental waters: effects of temperature and salinity. Appl. Microbiol. Biotechnol. 76, 935–944. doi: 10.1007/s00253-007-1048-z
Oksman M., Weckström K., Miettinen A., Juggins S., Divine D. V., Jackson R., et al. (2017). Younger Dryas ice margin retreat triggered by ocean surface warming in central-eastern Baffin Bay. Nat. Commun. 8, 1017. doi: 10.1038/s41467-017-01155-6
Pados-Dibattista T., Pearce C., Detlef H., Bendtsen J., Seidenkrantz M. S. (2022). Holocene palaeoceanography of the Northeast Greenland shelf. Clim. Past. 18, 103–127. doi: 10.5194/cp-18-103-2022
Pawłowska J., Łącka M., Kucharska M., Pawlowski J., Zajączkowski M. (2020a). Multiproxy evidence of the Neoglacial expansion of Atlantic water to eastern Svalbard. Clim. Past. 16, 487–501. doi: 10.5194/cp-16-487-2020
Pawłowska J., Lejzerowicz F., Esling P., Szczucinski W., Zajaczkowski M., Pawlowski J. (2014). Ancient DNA sheds new light on the Svalbard foraminiferal fossil record of the last millennium. Geobiology 12, 277–288. doi: 10.1111/gbi.12087
Pawłowska J., Wollenburg J. E., Zajączkowski M., Pawlowski J. (2020b). Planktonic foraminifera genomic variations reflect paleoceanographic changes in the Arctic: evidence from sedimentary ancient DNA. Sci. Rep. 10, 15102. doi: 10.1038/s41598-020-72146-9
Pawlowski J., Bonin A., Boyer F., Cordier T., Taberlet P. (2021). Environmental DNA for biomonitoring. Mol. Ecol. 30, 2931–2936. doi: 10.1111/mec.16023
Pawlowski J., Bruce K., Panksep K., Aguirre F. I., Amalfitano S., Apothéloz-Perret-Gentil L., et al. (2022). Environmental DNA metabarcoding for benthic monitoring: a review of sediment sampling and DNA extraction methods. Sci. Total Environ. 818, 151783. doi: 10.1016/j.scitotenv.2021.151783
Pearman J. K., Keeley N. B., Wood S. A., Laroche O., Zaiko A., Thomson-Laing G., et al. (2020). Comparing sediment DNA extraction methods for assessing organic enrichment associated with marine aquaculture. PeerJ 8, e10231. doi: 10.7717/peerj.10231
Pedersen M. W., Overballe-Petersen S., Ermini L., Sarkissian C. D., Haile J., Hellstrom M., et al. (2015). Ancient and modern environmental DNA. Philos. Trans. R. Soc Lond. B Biol. Sci. 370, 20130383. doi: 10.1098/rstb.2013.0383
Perianes-Rodriguez A., Waltman L., Van Eck N. J. (2016). Constructing bibliometric networks: a comparison between full and fractional counting. J. Informetr. 10, 1178–1195. doi: 10.1016/j.joi.2016.10.006
Pierella Karlusich J. J., Pelletier E., Zinger L., Lombard F., Zingone A., Colin S., et al. (2023). A robust approach to estimate relative phytoplankton cell abundances from metagenomes. Mol. Ecol. Resour. 23, 16–40. doi: 10.1111/1755-0998.13592
Pochon Z., Bergfeldt N., Kırdök E., Vicente M., Naidoo T., Valk T. V. D., et al. (2022). aMeta: an accurate and memory-efficient ancient metagenomic profiling workflow. bioRxiv 2022, 2010. doi: 10.1101/2022.10.03.510579
Porter T. M., Hajibabaei M. (2020). Putting COI metabarcoding in context: the utility of exact sequence variants (ESVs) in biodiversity analysis. Front. Ecol. Evol. 8. doi: 10.3389/fevo.2020.00248
Pratas D., Pinho A. (2018). “Metagenomic composition analysis of sedimentary ancient DNA from the Isle of Wight,” in 26th European Signal Processing Conference, EUSIPCO 2018 (Rome, Italy: IEEE).
Quast C., Pruesse E., Yilmaz P., Gerken J., Schweer T., Yarza P., et al. (2012). The SILVA ribosomal RNA gene database project: improved data processing and web-based tools. Nucleic Acids Res. 41, D590–D596. doi: 10.1093/nar/gks1219
Ribeiro S., Berge T., Lundholm N., Andersen T. J., Abrantes F., Ellegaard M. (2011). Phytoplankton growth after a century of dormancy illuminates past resilience to catastrophic darkness. Nat. Commun. 2, 311. doi: 10.1038/ncomms1314
Robert K. N., Benjamin G., John D. M., David L. S., David M., Barbara R., et al. (2005). Sand DNA - a genetic library of life at the water's edge. Mar. Ecol. Prog. Ser. 301, 9–22. doi: 10.3354/meps301009
Sanyal A., Larsson J., Van Wirdum F., Andrén T., Moros M., Lönn M., et al. (2022). Not dead yet: diatom resting spores can survive in nature for several millennia. Am. J. Bot. 109, 67–82. doi: 10.1002/ajb2.1780
Schubert M., Ginolhac A., Lindgreen S., Thompson J. F., Al-Rasheid K., Willerslev E., et al. (2012). Improving ancient DNA read mapping against modern reference genomes. BMC Genom. 13, 178. doi: 10.1186/1471-2164-13-178
Selway C. A., Armbrecht L., Thornalley D. (2022). An outlook for the acquisition of marine sedimentary ancient DNA (sedaDNA) from North Atlantic Ocean archive material. Paleoceanogr. Paleoclimatology 37, e2021PA004372. doi: 10.1029/2021PA004372
Siano R., Lassudrie M., Cuzin P., Briant N., Loizeau V., Schmidt S., et al. (2021). Sediment archives reveal irreversible shifts in plankton communities after World War II and agricultural pollution. Curr. Biol. 31, 2682–2689.e2687. doi: 10.1016/j.cub.2021.03.079
Smayda T. J. (1971). Normal and accelerated sinking of phytoplankton in the sea. Mar. Geol. 11, 105–122. doi: 10.1016/0025-3227(71)90070-3
Sunday J. M., Popovic I., Palen W. J., Foreman M. G. G., Hart M. W. (2014). Ocean circulation model predicts high genetic structure observed in a long-lived pelagic developer. Mol. Ecol. 23, 5036–5047. doi: 10.1111/mec.12924
Szczuciński W., Pawłowska J., Lejzerowicz F., Nishimura Y., Kokociński M., Majewski W., et al. (2016). Ancient sedimentary DNA reveals past tsunami deposits. Mar. Geol. 381, 29–33. doi: 10.1016/j.margeo.2016.08.006
Talas L., Stivrins N., Veski S., Tedersoo L., Kisand V. (2021). Sedimentary ancient DNA (sedaDNA) reveals fungal diversity and environmental drivers of community changes throughout the Holocene in the present boreal Lake Lielais Svētiņu (Eastern Latvia). Microorganisms 9, 719. doi: 10.3390/microorganisms9040719
Thomas C., Francke A., Vogel H., Wagner B., Ariztegui D. (2020). Weak influence of paleoenvironmental conditions on the subsurface biosphere of Lake Ohrid over the last 515 ka. Microorganisms 8, 1736. doi: 10.3390/microorganisms8111736
Thomas S. P., Shanmuganathan B., Jaiswal M. K., Kumaresan A., Sadasivam S. K. (2019). Legacy of a Pleistocene bacterial community: patterns in community dynamics through changing ecosystems. Microbiol. Res. 226, 65–73. doi: 10.1016/j.micres.2019.06.001
Thomsen P. F., Kielgast J., Iversen L. L., Møller P. R., Rasmussen M., Willerslev E. (2012). Detection of a diverse marine fish fauna using environmental DNA from seawater samples. PloS One 7, e41732. doi: 10.1371/journal.pone.0041732
Torti A., Jørgensen B. B., Lever M. A. (2018). Preservation of microbial DNA in marine sediments: insights from extracellular DNA pools. Environ. Microbiol. 20, 4526–4542. doi: 10.1111/1462-2920.14401
Torti A., Lever M. A., Jørgensen B. B. (2015). Origin, dynamics, and implications of extracellular DNA pools in marine sediments. Mar. Genom. 24, 185–196. doi: 10.1016/j.margen.2015.08.007
Vargas C. D., Audic S., Henry N., Decelle J., Mahé F., Logares R., et al. (2015). Eukaryotic plankton diversity in the sunlit ocean. Science 348, 1261605. doi: 10.1126/science.1261605
Voldstad L. H., Alsos I. G., Farnsworth W. R., Heintzman P. D., Håkansson L., Kjellman S. E., et al. (2020). A complete Holocene lake sediment ancient DNA record reveals long-standing high Arctic plant diversity hotspot in Northern Svalbard. Quat. Sci. Rev. 234, 106207. doi: 10.1016/j.quascirev.2020.106207
Vuillemin A., Wankel S. D., Coskun Ö.K., Magritsch T., Vargas S., Estes E. R., et al. (2019). Archaea dominate oxic subseafloor communities over multimillion-year time scales. Sci. Adv. 5, eaaw4108. doi: 10.1126/sciadv.aaw4108
Weyrich L. S., Duchene S., Soubrier J., Arriola L., Llamas B., Breen J., et al. (2017). Neanderthal behaviour, diet, and disease inferred from ancient DNA in dental calculus. Nature 544, 357–361. doi: 10.1038/nature21674
Willerslev E., Hansen A. J., Poinar H. N. (2004). Isolation of nucleic acids and cultures from fossil ice and permafrost. Trends Ecol. Evol. 19, 141–147. doi: 10.1016/j.tree.2003.11.010
Wood S. A., Biessy L., Latchford J. L., Zaiko A., Von Ammon U., Audrezet F., et al. (2020). Release and degradation of environmental DNA and RNA in a marine system. Sci. Total Environ. 704, 135314. doi: 10.1016/j.scitotenv.2019.135314
Xue J., Feng Y. (2018). Determination of adsorption and desorption of DNA molecules on freshwater and marine sediments. J. Appl. Microbiol. 124, 1480–1492. doi: 10.1111/jam.13739
Zachos J. C., Dickens G. R., Zeebe R. E. (2008). An early Cenozoic perspective on greenhouse warming and carbon-cycle dynamics. Nature 451, 279–283. doi: 10.1038/nature06588
Zheng X., Liu W., Dai X., Zhu Y., Wang J., Zhu Y., et al. (2021). Extraordinary diversity of viruses in deep-sea sediments as revealed by metagenomics without prior virion separation. Environ. Microbiol. 23, 728–743. doi: 10.1111/1462-2920.15154
Zimmermann H. H., Stoof-Leichsenring K. R., Kruse S., Müller J., Stein R., Tiedemann R., et al. (2020). Changes in the composition of marine and sea-ice diatoms derived from sedimentary ancient DNA of the eastern Fram Strait over the past 30 000 years. Ocean Sci. 16, 1017–1032. doi: 10.5194/os-16-1017-2020
Zimmermann H. H., Stoof-Leichsenring K. R., Kruse S., Nürnberg D., Tiedemann R., Herzschuh U. (2021). Sedimentary ancient DNA from the Subarctic North Pacific: how sea ice, salinity, and insolation dynamics have shaped diatom composition and richness over the past 20,000 years. Paleoceanogr. Paleoclimatology 36, e2020PA004091. doi: 10.1029/2020PA004091
Keywords: paleoceanography, sedaDNA, marine sediment, metabarcoding, metagenomics, marine biodiversity
Citation: Nguyen N-L, Devendra D, Szymańska N, Greco M, Angeles IB, Weiner AKM, Ray JL, Cordier T, De Schepper S, Pawłowski J and Pawłowska J (2023) Sedimentary ancient DNA: a new paleogenomic tool for reconstructing the history of marine ecosystems. Front. Mar. Sci. 10:1185435. doi: 10.3389/fmars.2023.1185435
Received: 13 March 2023; Accepted: 30 May 2023;
Published: 09 June 2023.
Edited by:
Christian Grenz, UMR7294 Institut Méditerranéen d’océanographie (MIO), FranceReviewed by:
Marco J. L. Coolen, Curtin University, AustraliaMathilde Bourreau, École Normale Supérieure, France
Copyright © 2023 Nguyen, Devendra, Szymańska, Greco, Angeles, Weiner, Ray, Cordier, De Schepper, Pawłowski and Pawłowska. This is an open-access article distributed under the terms of the Creative Commons Attribution License (CC BY). The use, distribution or reproduction in other forums is permitted, provided the original author(s) and the copyright owner(s) are credited and that the original publication in this journal is cited, in accordance with accepted academic practice. No use, distribution or reproduction is permitted which does not comply with these terms.
*Correspondence: Ngoc-Loi Nguyen, bG9pbmd1eWVuQGlvcGFuLnBs; Joanna Pawłowska, cGF3bG93c2thQGlvcGFuLnBs