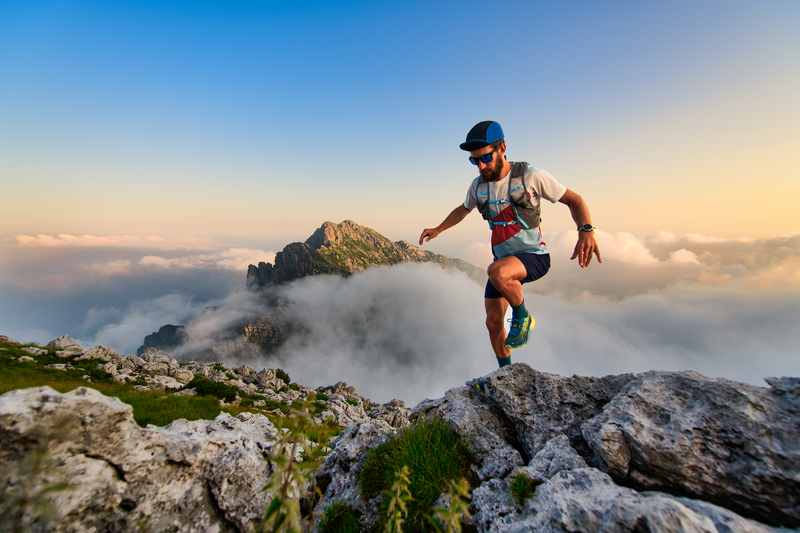
95% of researchers rate our articles as excellent or good
Learn more about the work of our research integrity team to safeguard the quality of each article we publish.
Find out more
ORIGINAL RESEARCH article
Front. Mar. Sci. , 15 May 2023
Sec. Marine Biogeochemistry
Volume 10 - 2023 | https://doi.org/10.3389/fmars.2023.1184397
This article is part of the Research Topic Seafloor Processes: Geomorphology, Sediment-Ocean Interaction and Natural Resources View all 11 articles
Internal solitary waves (ISWs) exert a significant influence on the deep-sea floor, yet little research has been conducted on their impact on benthic organisms. The objective of this study was to investigate the propagation characteristics of ISWs on the Shenhu continental slope in the northern South China Sea, as well as their mechanisms of influence on benthic activity. In-situ observation was performed at the head of the Shenhu Canyon (water depth 655m) to determine the physical characteristics of ISWs and the changes in benthic organisms. The study revealed that the abundance and density of benthic organisms were positively correlated with the time and intensity of ISWs. The abundance of benthic organisms affected by ISWs is 3-5 times that without ISWs, and the density of benthic organisms is 3-9 times. The impact induced by ISWs, including resuspension of bottom sediments, formation of marine snow, changes in the bottom boundary layer environment, and vertical transfer of seawater environmental factors, played a crucial role in the impact on benthic activity. To our limited knowledge, this is the first in-situ long-term observation study on the effects of ISWs on deep-sea benthic organisms on the continental slope of the northern South China Sea deeper than 600m, providing new insights for exploring the environmental impact of ISWs on the deep-sea bottom boundary layer.
Internal solitary waves (ISWs) are a type of nonlinear internal wave characterized by short periods, large amplitudes, and strong flow velocities that remain relatively constant during propagation (Bogucki and Redekopp, 1999; Helfrich et al., 2006; Alford et al., 2015). They are widely distributed in various terrains such as large lakes and marginal seas (Boegman and Stastna, 2019; Syamsudin et al., 2019) and can cause interactions between seawater and the seafloor, becoming a major driving force for perturbing the deep-sea benthic environment (Holligan et al., 1985; Jia et al., 2019). ISWs enhance material transport and vertical mixing processes on the continental slope and shelf. From a biological perspective, ISWs affect benthic biomass and activity on the continental slope and shelf (van Haren, 2020).
The distribution and dynamics of benthic communities depend on different hydrodynamic conditions (Shimeta et al., 2003). ISWs induce shear stress and turbulence, which promote vertical mixing and exchange of heat and nutrients, thereby increasing primary productivity (Chen et al., 2016b; Zhang et al., 2019; Hung et al., 2021). Marine snow, a major source of food for mobile organisms (Trudnowska et al., 2021), can enhance the feeding and survival of mobile organisms (MacKenzie, 2000; MacKenzie and Kiorboe, 2000). ISWs can affect overall biomass changes on a relatively small spatial scale (Pineda, 1991; Broitman et al., 2008), and this movement from deep to shallow waters can transport larvae and organisms to their adult habitats, thus altering population density (Roder et al., 2010). Flood et al. (2021) found that fish habitat locations change with the vertical movement of the thermocline induced by internal wave disturbances, and the time scale of fish response to physical environmental changes corresponds to the period of internal waves. Currently, the impact of ISWs on biological activity is mainly reported in shallow water areas, and their effects on deep water areas are unknown.
ISWs generated in the Luzon Strait of the South China Sea propagate northwestward, with a significant amount of energy dissipated on the continental slope and shelf (Cai et al., 2012; Fu et al., 2012). Reid et al. (2019) observed that ISWs caused a four-fold increase in instantaneous nitrate flux in the Dongsha Atoll in the northern South China Sea compared to periods without ISWs. The shoaling of ISWs transports nutrient-rich water to the shelf and coast, increasing nutrient concentrations and biological productivity, thereby improving the coral ecosystem of the Dongsha Atoll (Reid et al., 2019; Hung et al., 2021). The enhancement of biological activity by ISWs is not limited to shallow waters on the continental shelf, as ISWs can cause vertical mixing at depths of 600m and transfer nutrient-rich cold water to promote biological productivity (Pan et al., 2012). However, in situ observations of ISWs are mainly concentrated in the Dongsha Sea, and observations of the impact of ISWs on the marine environment and biological activity are lacking. The Shenhu Canyon is located in the central Pearl River Mouth Basin, where sediments and nutrients transported by the Pearl River greatly enrich the benthic ecosystem of the canyon (Yin et al., 2019; Zhang et al., 2022). There are many canyons and steep ridges on the Shenhu continental slope (Su et al., 2020), and the interaction between ISWs and steep terrain can increase the bottom flow velocity and shear stress (Lamb, 2014; Tian et al., 2021b), enhancing disturbances to the deep-sea benthic environment.
This study aimed to investigate the physical characteristics of ISWs and changes in benthic biological activity on the Shenhu continental slope in the northern South China Sea using long-term in situ observations. We explored the environmental impact of ISWs on the water column and deep-sea bottom boundary layer and analyzed the relevant mechanisms of the impact of ISWs on benthic biological activity.
The Shenhu continental slope, located in the northern South China Sea, is comprised of 17 submarine canyons oriented in NW-SE direction (Ding et al., 2013; Chen et al., 2016a). The canyons’ heads are affected by large-amplitude internal solitary waves (ISWs), which result in erosive turbidity currents (Yin et al., 2019), as depicted in Figures 1A, B, Observation station SH1 is situated at the head of the canyon at a depth of approximately 655m. Satellite data show the presence of numerous internal solitary waves during the observation period (Figure 1C). During the cruise, sediment samples were collected using a gravity corer, and the sediment’s physical properties were determined in the laboratory. As shown in Table 1, the sediment’s median particle size was 0.01 mm, and it exhibited high water content, high porosity, and high saturation. The surface sediment in the Shenhu region contained biogenic particles, such as foraminifera and algae, as well as flocculent material (Kuang et al., 2019). The Shenhu canyon’s topography results in more frequent and energetic internal solitary waves due to refraction, reflection, and interaction with the topography (Ma et al., 2016; Bai et al., 2019). These strong and frequent hydrodynamic phenomena are likely to affect changes in the seafloor environment (Zhu et al., 2023a; Zhu et al., 2023b).
Figure 1 (A) Location of the Shenhu Canyon System and study area in the Northern South China Sea, (B) The multi-beam geomorphology shadow map was modified from Ding et al. (2013), (C) Moderate-resolution Imaging Spectroradiometer (MODIS) image of the northern South China Sea on Aug 25, 2020, data source https://worldview.earthdata.nasa.gov.
W is the water content, ρs is the sediment natural density, n is the porosity, S is the specific weight of the particle, Sr is the sediment saturation, and D50 is the mean particle size.
The scientific research vessel “Dongfanghong 3” deployed a deep-sea in-situ observation system for the bottom boundary layer at observation station SH1. Continuous underwater observations were conducted from August 20 to September 2, 2020. As shown in Figures 2A, B, The observation system was equipped with a 75 kHz acoustic Doppler current profiler (75k-ADCP), a Nortek Vector Acoustic Doppler Velocimeter (ADV), RBR concerto3a Multi-Channel Logger, and a deep-sea camera. Using the ship-borne CTD profiling system, we measured the changes in environmental parameters in the vertical direction of the water column for 24 hours from 5:00 pm on September 1 to 5:00 pm on September 2. We collected water samples and transformed the instrument’s observed turbidity into suspended particulate matter concentration using filtration experimental methods (Wang et al., 2022). The turbidity and suspended particulate matter concentration conversion equation was SPM=5.64×Turbidity+0.12.
Figure 2 (A) Field working diagram of the observation system, (B) Schematic illustration of the observation instrument.
Observations of the dynamics of ISWs were conducted using a 75k-ADCP (Huang et al., 2022). Profile data were recorded every 1 minute, with unreasonable extreme points excluded, and vertically interpolated every 8 meters. The Multi-Channel Logger collected data every 10 seconds, recording changes in temperature, salinity, dissolved oxygen, and turbidity in the bottom boundary layer (Dato et al., 2021; Du et al., 2021; Kheili et al., 2021). The ADV had a sampling frequency of 64Hz and was continuously sampled.
Video images captured by the deep-sea camera were taken every 3 minutes for 7 seconds. Each image was an RGB image stored in a three-dimensional matrix with pixel values of “1080×1920” and dimensions of 378×672 mm. Although some shadows and micro-benthic organisms were not identified as biological species due to fixed camera observation, they were used for statistical calculations of biological abundance and density. Changes in biological abundance (A) and density (D) were characterized using formula (1).
where n is the number of individuals per hour, N is the total number of individuals in a certain time, s is the area occupied by the organism, and S is the image area.
In the bottom boundary layer, shear stress (τc) caused by velocity was calculated using the Turbulent Kinetic Energy (TKE) method, Covariance method (COV), and Logarithmic Velocity (RL) method. The TKE method (Stapleton and Huntley, 1995; Zhang et al., 2018), which does not require consideration of the height of the ADV above the seabed, was used (Pope et al., 2006). Velocity data in the three directions were averaged every minute, with the total average value subtracted from all the velocity data, and calculated the velocity fluctuations in the three directions were as follows:
shear stress τc was calculated by
where C1 is the empirical coefficient, generally taking a value of 0.19-0.2 (Stapleton and Huntley, 1995), and ρ is the seawater density.
The sediment in the study area was fine-grained cohesive sediments, and we used the equation applicable to cohesive sediments to determine the critical shear stress (τce) of the bottom sediment (Shi et al., 2012).
where β is a dimensionless coefficient, s is the specific weight of the particle, W is the water content, and ρs is the sediment particle density. When the particle size of the bottom sediment is less than a few tens of microns and the water content of the bottom sediment is relatively high, β is 0.3 (Taki, 2000).
Figure 3 shows the velocity profile for a total of 3 days from 8/21 00:00 to 8/24 12:00. The velocity profile (25-628 mab) observed by 75k-ADCP shows strong velocity at the study area, with a maximum velocity exceeding 0.5 m/s in both the u and v directions (Figures 3A, B). From an analysis of the entire water column, the horizontal velocity exhibits a three-layer structure with the same flow direction between the sea surface and the sea floor. In addition, the horizontal current exhibits an obvious diurnal variation cycle, showing internal tidal characteristics. The maximum vertical velocity (w) exceeds 0.1 m/s and exhibits clear diel vertical migration characteristics, with organisms migrating to a depth of around 400 m around 6:00 and returning to the surface around 18:00. ISWs exists in the form of wave packets, with one ISW packet arriving at the study area around 20:00 each day. The current is northwest with a maximum velocity of 0.5 m/s at the surface. In the bottom boundary layer deeper than 600 m, The current is southeast with a maximum velocity of 0.2 m/s.
Figure 3 Velocity profiles measured by 75 k-ADCP. (A) zonal velocity u, (B) meridional velocity v, (C) vertical velocity w.(ISWs-1, ISWs-2, and ISWs-3 indicate ISW packets. The black line indicates the time scale for the ISW packet).
As shown in Figure 4, the profile of velocity indicates the ISWs-2 includes five distinct ISWs of depression, which lasted for about 4 hours with each individual ISW lasting around 20 minutes. Combining with Figure 3, ISW-1 contains four distinct ISWs of depression with a duration of about 3 hours, and ISW-3 contains six distinct ISWs of depression with a duration of around 5 hours. Figures 4A, B indicates that the horizontal velocity profile presents an obvious two-layered structure in which the dividing depth is approximately 130 m. In the upper layer, the zonal current is westward and the meridional current is northward, the horizontal velocity caused by ISWs can reach up to 0.6 m/s. In the lower layer, the zonal current is eastward and the meridional current is southward, and the horizontal velocity caused by ISWs exceeds 0.2 m/s. Figure 4C shows that there are strong upwelling and downwelling flows before and after the arrival of each ISW, with the highest flow velocity reaching 0.1 m/s, and such flow can act on the seafloor. It is worth noting that the time scales of the downwelling and upwelling flows are not consistent. For example, the time scale of the downwelling flow of the first ISW is 12 minutes, while the time scale of the upwelling flow is 10 minutes, and the strength of the downwelling flow is higher than the upwelling flow. This asymmetry enhances the transport of heat and energy in the water column, which has an important impact on the marine ecological environment (Chen et al., 2019; Hu et al., 2023).
Figure 4 The velocity results when the ISWs-2 passed. (A) zonal velocity u, (B) meridional velocity v, (C) vertical velocity w.
Video images captured by the deep-sea camera demonstrate there are a variety of benthic organisms in the study area (Figure 5). A total of 6,320 video sequences were captured, and 890 of them contained biological specimens. According to the method of Li (2017), The analysis identified 23 different species of benthic organisms from six phyla, including Cnidaria, Arthropoda, Chordata, Nematoda, Mollusca, and Annelida.
Figure 6 shows the changes in benthic abundance and density for 3 days from 8/21 00:00 to 8/24 12:00. Because the undersea camera is fixed to the observation system, there were many times when no identifiable benthic organisms were captured, so the abundance and density of the organisms were 0 at such times. The abundance and density of benthic organisms showed three peak stages. The first peak occurred around 1:00-2:30 on August 22, the maximum values of abundance and density are 0.043 and 0.78, respectively. The second peak occurred from around 22:00 on August 22 to 2:00 on August 23, the maximum values of abundance and density are 0.044 and 1.2, respectively. The third peak occurred from around 22:00 on August 23 to 2:30 on August 24, the maximum values of abundance and density are 0.053 and 1.3, respectively. Combined with Figure 4, we can find that the increase in abundance and density of benthic organisms is closely related to the passage of ISWs, and the abundance and density of benthic organisms are positively correlated with the strength and period of ISWs. The abundance of benthic organisms affected by ISWs is 3-5 times that without ISWs, and the density of benthic organisms is 3-9 times.
The resuspension of sediment inevitably leads to an increase in suspended particulate matter in the water column. In some canyon trench terrains, the increase in organic matter sedimentation has led to a doubling of oxygen consumption by organisms (Muacho et al., 2013). In-situ observation results indicated that the suspended particulate matter in the bottom boundary layer was mainly marine snow (Figures 7A, B), which are sticky particles with a negative charge (Alldredge et al., 2002; Taucher et al., 2018). These particles can combine with sediment particles, mainly composed of diatoms, animal feces or debris, and mineral particles (Möller et al., 2012). Bottom water samples were collected using a CTD, and the suspended particle composition was mainly analyzed in the laboratory using a microscope, which revealed that diatoms and organic particles are the main components of flocculent particles(Figures 7C, D). The accumulation of marine snow in persistent, density-discontinuous nepheloid layer leads to environmental zoning in the water column area, which affects the distribution and interaction of planktonic organisms and the intensity of biological processes in the water column, and helps to maintain species diversity (Alldredge et al., 2002; Turner, 2015). Marine snow is the primary carrier of the biological carbon pump and has a significant impact on the settlement and feeding of planktonic organisms (Trudnowska et al., 2021). Therefore, the presence of suspended particles such as marine snow has become an important mechanism affecting biological activity.
Figure 7 (A, B) marine snow particles captured by underwater cameras, (C, D) show suspended particles observed under a microscope.
In the continental slope region of the deep sea, large-amplitude ISWs of depression can cause sediment resuspension through shear stress (Lamb, 1997; Boegman and Stastna, 2019; Jia et al., 2019). Figure 8A depicts the changes in the velocity of the bottom boundary layer during the passage of ISWs-2, as recorded by ADV. For each ISW of depression, the u and v velocities are almost symmetrical, with an average velocity of 0.1 m/s. The v-direction velocity is relatively higher, with a maximum velocity of 0.22 m/s. The variation in the w-direction velocity is not significant. The incipient flow velocity of deep-sea marine snow particles ranges between 0.06-0.08 m/s, while a velocity of approximately 0.1-0.2 m/s can suspend most clayey sands (Camenen, 2009; Gardner et al., 2017). Therefore, ISWs can cause large-scale suspension and transport of marine snow at a depth of around 655 m. The maximum bottom shear stress generated by ISWs is 1.48 Pa, while the critical bed shear stress of the seafloor sediment is 0.2 Pa (Figure 8B). The bottom shear stress produced by ISWs surpasses the critical bed shear stress of sediment, which is the condition for sediment erosion and resuspension (Quaresma et al., 2007; Valipour et al., 2017). Figure 8C illustrates that the average suspended particulate matter concentration caused by the ISWs is 4.1 mg/l, which is 2-3 times higher than the background concentration.
Figure 8 (A) Velocity characteristics of ISWs in the submarine boundary layer, (B) The bed shear stresses (τc)due to ISWs and the critical shear stress for erosion of the bottom sediment (τce), (C) Suspended particulate matter (SPM).
During the propagation of ISWs from the deep sea to the continental slope and shelf regions, they play a crucial role in transporting materials and delivering heat (or cold) energy and nutrients to the benthic communities (Pan et al., 2012; Li et al., 2018; Navarro et al., 2018). Additionally, ISWs can cause small-scale physical changes in the benthic environment (Leichter et al., 2003; Hung et al., 2021) In addition, ISWs can lead to the accumulation of surface organic films, phytoplankton, and zooplankton (Macías et al., 2010), which can alter benthic organism activity. To estimate nitrate concentration (), a linear fit between nitrate concentration and water temperature is used (Reid et al., 2019). Although this method does not consider biotransformation of the nutrient field, such as uptake or remineralization, it can reflect trends in nutrient changes. In this study, the passage of ISWs resulted in a change in bottom temperature of approximately 0.2 °C (Figure 9A) and a change in nitrate () concentration of 0.18 μM (Figure 9D). The bottom temperature and dissolved oxygen concentration decreased and then increased (Figures 9A, C), while salinity and nitrate concentration increased and then decreased (Figures 9B, D), indicating that ISWs can bring nutrient-rich cold water to the continental slope, thus impacting benthic organism activity.
Figure 9 The variability of bottom environmental elements when the ISWs passed. (A) temperature, (B) salinity, (C) dissolved oxygen, (D) nitrate ().
ISWs play a crucial role in enhancing vertical mixing and modifying the thermocline structure (Wang et al., 2001; Wang et al., 2007). ISWs of depression can transfer dissolved substances (CO2 and O2) and water containing biological particles from the upper layer to the benthic system, affecting benthic biological activity (Pan et al., 2012; Muacho et al., 2013). In this study, to understand the impact of ISWs on the water column environment in the study area, we conducted continuous CTD observations from 17:30 on September 1, 2020 to 17:30 on September 2, 2020, tracking the environmental impact of a single internal solitary wave on the water column (Figure 10). Similar observations have been made by Hung et al. (2021) with a water depth of 200 m on the Dongsha shoal.
Figure 10 Impact of single ISW on the vertical structure of the water column. (A–C) velocity profiles single ISW passage measured by 75 k-ADCP, (D) Temperature variation, (E) Turbidity variation (black boxes indicate the time scale for a single ISW).
Figures 10A, B, and C show that a single ISW arrived around 19:10 on September 1st, with a maximum velocity in the u and v directions of 0.3 m/s and a vertical velocity w of no more than 0.05 m/s. The duration of its action was approximately 20 minutes, and compared with the other observed ISWs, the energy of this single ISW was relatively weak. However, this single ISW still can force the surface water to move downward, causing vertical mixing outside of the thermocline, with a maximum displacement distance of 50 m (Figure 10D). The ISW of depression discovered by Huang et al. (2016) caused the maximum temperature to increase by over 6°C at the trough at a depth of 300m, and even at 1000 m depth, the temperature increase exceeded 0.5°C, which is likely to have a significant impact on benthic biological activity. Figure 10E shows an increase in turbidity at depths of 70 m, 300 m, and 600 m, indicating the presence of surface, middle, and bottom nepheloid layers (Masunaga et al., 2015). ISWs elevate the thickness of the surface nepheloid layer and increased the concentration of the middle and bottom nepheloid layers.
We summarize three main mechanisms of benthic organism activity affected by ISWs (Figure 11): (1) In steep terrain or shallow water, ISWs mainly break and form local vortices (Bogucki and Redekopp, 1999; Puig et al., 2001), resuspending a large amount of sediment and continuously migrating these suspended sediments to the deep sea, forming nepheloid layer (Zhang et al., 2019; Tian et al., 2021a). During migration, suspended sediment particles aggregate to form marine snow and other flocs that settle to the seafloor. In the deep-sea bottom boundary layer, ISWs cause sediment resuspension through large bottom shear forces, with marine snow being the dominant suspended particle type that serves as a food source for benthic organisms on the continental slope. This represents an important mechanism for impacting benthic organism activity. (2) During the propagation of ISWs from the open ocean to the continental slope, the transport of nutrient-rich cold water to benthic communities on the seafloor impacts benthic organism activity. Strong hydrodynamics also transport some larvae and organisms to the continental slope and shelf (Roder et al., 2010), thereby affecting changes in benthic organism abundance. (3) In the vertical direction, ISWs can enhance the vertical mixing of seawater, alter thermocline structure, and impact the habitat of upper-level organisms (Flood et al., 2021). Additionally, ISWs transfer heat and bioparticles from the upper layer to the benthic system, thereby affecting benthic organism activity.
In this study, we investigated the impact of ISWs on the deep-sea bottom boundary layer environment and benthic organisms through in-situ long-term observations for the first time on the continental slope of the northern South China. The results are summarized as follows:
(1) ISWs of depression with a period of one day were observed in the Shenhu continental slope, arriving at the study area around 19:00 to 21:00 each day. ISWs consisted of 5-6 individual wave groups in a wave packet form, with a maximum horizontal velocity exceeding 0.5m/s and vertical velocity exceeding 0.1m/s. ISWs induced velocity changes exceeding 0.2m/s in the bottom boundary layer.
(2) The abundance and density of benthic organisms were positively correlated with the duration and intensity of ISWs. The abundance of benthic organisms affected by ISWs was found to be 3-5 times higher than that in the absence of ISWs, while the density was 3-9 times higher.
(3) ISWs generated significant shear stress in the bottom boundary layer at a depth of 655m, causing sediment resuspension on the Shenhu continental slope and increasing the aggregation and transport of marine snow and other nutrients. This provided a food source for benthic organisms. The shoaling effect of ISWs on the continental slope also introduced cold nutrient-rich water, larvae, and organisms, which affected changes in benthic biomass. In the vertical direction, ISWs were found to move temperature, salinity, dissolved oxygen, and nutrients of surface water toward deeper layers, thereby influencing the position of benthic organisms in the water column. These factors collectively influenced changes in benthic organism activity.
The original contributions presented in the study are included in the article/supplementary material. Further inquiries can be directed to the corresponding authors.
XF took part in the in-situ observations, analyzed, and interpreted the data, and wrote the manuscript. LW revised the manuscript and provided suggestions for the manuscript. CJ took part in the in-situ observations and analyzed some of the data. HW revised part of the manuscript and provided suggestions for the manuscript. CZ presented the central ideas, revised the manuscript, discussed the results, and approved the submitted version. YJ presented the central idea, discussed the results, provided advice to the manuscript, and approved the submitted version. All authors contributed to the article and approved the submitted version.
This research was funded by the National Natural Science Foundation of China (No. 41831280, 42207173), the Natural Science Foundation of Shandong Province (No. ZR2022QD002, 2022RZB07052), the Shandong Provincial and Qingdao Postdoctoral Foundation (No. SDCX-ZG-202203089), and the Key Laboratory of Submarine Geosciences and Prospecting Techniques (MoE) (No. SGPT-2022OF-03).
We thank the Natural Science Foundation of China for the Open Research Cruise of the northern South China Sea (NORC2019-05, NORC2022-05). We appreciate all members for their efforts in the collection of the data in this study.
The authors declare that the research was conducted in the absence of any commercial or financial relationships that could be construed as a potential conflict of interest.
All claims expressed in this article are solely those of the authors and do not necessarily represent those of their affiliated organizations, or those of the publisher, the editors and the reviewers. Any product that may be evaluated in this article, or claim that may be made by its manufacturer, is not guaranteed or endorsed by the publisher.
Alford M. H., Peacock T., MacKinnon J. A., Nash J. D., Buijsman M. C., Centurioni L. R., et al. (2015). The formation and fate of internal waves in the south China Sea. Nature 521 (7550), 65–69. doi: 10.1038/nature14399
Alldredge A. L., Cowles T. J., MacIntyre S., Rines J. E. B., Donaghay P. L., Greenlaw C. F., et al. (2002). Occurrence and mechanisms of formation of a dramatic thin layer of marine snow in a shallow pacific fjord. Mar. Ecol. Prog. Ser. 233, 1–12. doi: 10.3354/meps233001
Bai X., Liu Z., Zheng Q., Hu J., Lamb K. G., Cai S. (2019). Fission of shoaling internal waves on the northeastern shelf of the south China Sea. J. Geophysical Research: Oceans 124 (7), 4529–4545. doi: 10.1029/2018jc014437
Boegman L., Stastna M. (2019). Sediment resuspension and transport by internal solitary waves. Annu. Rev. Fluid Mechanics 51 (1), 129–154. doi: 10.1146/annurev-fluid-122316-045049
Bogucki D. J., Redekopp L. G. (1999). A mechanism for sediment resuspension by internal solitary waves. Geophysical Res. Lett. 26 (9), 1317–1320. doi: 10.1029/1999gl900234
Broitman B. R., Blanchette C. A., Menge B. A., Lubchenco J., Krenz C., Foley M., et al. (2008). Spatial and temporal patterns of invertebrate recruitment along the West coast of the united states. Ecol. Monogr. 78 (3), 403–421. doi: 10.1890/06-1805.1
Cai S., Xie J., He J. (2012). An overview of internal solitary waves in the south China Sea. Surveys Geophysics 33 (5), 927–943. doi: 10.1007/s10712-012-9176-0
Camenen B. (2009). Estimation of the wave-related ripple characteristics and induced bed shear stress. Estuarine Coast. Shelf Sci. 84 (4), 553–564. doi: 10.1016/j.ecss.2009.07.022
Chen T. Y., Tai J. H., Ko C. Y., Hsieh C. H., Chen C. C., Jiao N., et al. (2016b). Nutrient pulses driven by internal solitary waves enhance heterotrophic bacterial growth in the south China Sea. Environ. Microbiol. 18 (12), 4312–4323. doi: 10.1111/1462-2920.13273
Chen D., Wang X., Völker D., Wu S., Wang L., Li W., et al. (2016a). Three dimensional seismic studies of deep-water hazard-related features on the northern slope of south China Sea. Mar. Petroleum Geology 77, 1125–1139. doi: 10.1016/j.marpetgeo.2016.08.012
Chen L., Zheng Q., Xiong X., Yuan Y., Xie H., Guo Y., et al. (2019). Dynamic and statistical features of internal solitary waves on the continental slope in the northern south China Sea derived from mooring observations. J. Geophysical Research: Oceans 124 (6), 4078–4097. doi: 10.1029/2018jc014843
Dato J. F., Fiore M. M. E., Donofrio E. E., Oreiro F. A., Grismeyer W. H. (2021). Comparison of the extreme surge estimation with the generalized extreme value distribution, using the maximum of positive storm surge and skew surge in two port areas with different hydrodynamic and tidal characteristics. J. Mar. Environ. Eng. 10 (4), 281–290.
Ding W., Li J., Li J., Fang Y., Tang Y. (2013). Morphotectonics and evolutionary controls on the pearl river canyon system, south China Sea. Mar. Geophysical Res. 34 (3-4), 221–238. doi: 10.1007/s11001-013-9173-9
Du X., Sun Y., Song Y., Zhu C. (2021). In-situ observation of wave-induced pore water pressure in seabed silt in the yellow river estuary of China. J. Mar. Environ. Eng. 10 (4), 305–317.
Flood B., Wells M., Dunlop E., Young J. (2021). Vertical oscillations of the thermocline caused by internal waves modify coldwater pelagic fish distribution: results from a large stratified lake. J. Great Lakes Res. 47 (10), 1386–1399. doi: 10.1016/j.jglr.2021.06.010
Fu K.-H., Wang Y.-H., St. Laurent L., Simmons H., Wang D.-P. (2012). Shoaling of large-amplitude nonlinear internal waves at dongsha atoll in the northern south China Sea. Continental Shelf Res. 37, 1–7. doi: 10.1016/j.csr.2012.01.010
Gardner W. D., Tucholke B. E., Richardson M. J., Biscaye P. E. (2017). Benthic storms, nepheloid layers, and linkage with upper ocean dynamics in the western north Atlantic. Mar. Geology 385, 304–327. doi: 10.1016/j.margeo.2016.12.012
Helfrich K. R., Melville W. K., Miles J. W. (2006). On interfacial solitary waves over slowly varying topography. J. Fluid Mechanics 149 (-1), 305–317. doi: 10.1017/s0022112084002664
Holligan P. M., Pingree R. D., Mardell G. T. (1985). Oceanic solitons, nutrient pulses and phytoplankton growth. Nature 314 (6009), 348–350. doi: 10.1038/314348a0
Hu C., Li X., Ji C., Jiao X., Jia Y. (2023). In-situ observation of seabed vertical deformation inYellow River Delta under storm surges. Mar. Pet. Geol. 152, 106250. doi: 10.1016/j.marpetgeo.2023.106250
Huang X., Chen Z., Zhao W., Zhang Z., Zhou Q., Yang, J. (2016). An extreme internal solitary wave event observed in the northern South China Sea. Sci Rep 6, 30041. doi: 10.1038/srep30041
Huang X., Huang S., Zhao W., Zhang Z., Zhou C., Tian J. (2022). Temporal variability of internal solitary waves in the northern south China Sea revealed by long-term mooring observations. Prog. Oceanography 201, 102716. doi: 10.1016/j.pocean.2021.102716
Hung J. J., Wang Y. H., Fu K. H., Lee I. H., Tsai S. S., Lee C. Y., et al. (2021). Biogeochemical responses to internal-wave impacts in the continental margin off dongsha atoll in the northern south China Sea. Prog. Oceanography 199, 102689. doi: 10.1016/j.pocean.2021.102689
Jia Y., Tian Z., Shi X., Liu J. P., Chen J., Liu X., et al. (2019). Deep-sea sediment resuspension by internal solitary waves in the northern south China Sea. Sci. Rep. 9 (1), 12137. doi: 10.1038/s41598-019-47886-y
Kheili A. G. K., Shafieefar M., Rezaei A., Nemati M. H., Bagheri M. (2021). Contributing factors to ocean currents modelling in the makran coastal region. J. Mar. Environ. Eng. 10 (4), 261–280.
Kuang Y., Yang L., Li Q., Lv X., Li Y., Yu B., et al. (2019). Physical characteristic analysis of unconsolidated sediments containing gas hydrate recovered from the shenhu area of the south China sea. J. Petroleum Sci. Eng. 181, 106173. doi: 10.1016/j.petrol.2019.06.037
Lamb K. G. (1997). Particle transport by nonbreaking, solitary internal waves. J. Geophysical Research: Oceans 102 (C8), 18641–18660. doi: 10.1029/97jc00441
Lamb K. G. (2014). Internal wave breaking and dissipation mechanisms on the continental Slope/Shelf. Annu. Rev. Fluid Mechanics 46 (1), 231–254. doi: 10.1146/annurev-fluid-011212-140701
Leichter J. J., Stewart H. L., Miller S. L. (2003). Episodic nutrient transport to Florida coral reefs. Limnology Oceanography 48 (4), 1394–1407. doi: 10.4319/lo.2003.48.4.1394
Li X. (2017). Taxonomic research on deep-sea macrofauna in the south China Sea using the Chinese deep-sea submersible jiaolong. Integr. Zool 12 (4), 270–282. doi: 10.1111/1749-4877.12254
Li D., Chou W. C., Shih Y. Y., Chen G. Y., Chang Y., Chow C. H., et al. (2018). Elevated particulate organic carbon export flux induced by internal waves in the oligotrophic northern south China Sea. Sci. Rep. 8 (1), 2042. doi: 10.1038/s41598-018-20184-9
Ma X., Yan J., Hou Y., Lin F., Zheng X. (2016). Footprints of obliquely incident internal solitary waves and internal tides near the shelf break in the northern south China Sea. J. Geophysical Research: Oceans 121 (12), 8706–8719. doi: 10.1002/2016jc012009
Macías D., Somavilla R., González-Gordillo J. I., Echevarría F. (2010). Physical control of zooplankton distribution at the strait of Gibraltar during an episode of internal wave generation. Mar. Ecol. Prog. Ser. 408, 79–95. doi: 10.3354/meps08566
MacKenzie B. R. (2000). Turbulence, larval fish ecology and fisheries recruitment: a review of field studies. Oceanologica Acta 23 (4), 357–375. doi: 10.1016/S0399-1784(00)00142-0
MacKenzie B. R., Kiorboe T. (2000). Larval fish feeding and turbulence: a case for the downside. Limnology Oceanography 45 (1), 1–10. doi: 10.4319/lo.2000.45.1.0001
Masunaga E., Homma H., Yamazaki H., Fringer O. B., Nagai T., Kitade Y., et al. (2015). Mixing and sediment resuspension associated with internal bores in a shallow bay. Continental Shelf Res. 110, 85–99. doi: 10.1016/j.csr.2015.09.022
Möller K. O., St John M., Temming A., Floeter J., Sell A. F., Herrmann J. P., et al. (2012). Marine snow, zooplankton and thin layers: indications of a trophic link from small-scale sampling with the video plankton recorder. Mar. Ecol. Prog. Ser. 468, 57–69. doi: 10.3354/meps09984
Muacho S., da Silva J. C. B., Brotas V., Oliveira P. B. (2013). Effect of internal waves on near-surface chlorophyll concentration and primary production in the nazaré canyon (west of the Iberian peninsula). Deep Sea Res. Part I: Oceanographic Res. Papers 81, 89–96. doi: 10.1016/j.dsr.2013.07.012
Navarro G., Vicent J., Caballero I., Gómez-Enri J., Morris E. P., Sabater N., et al. (2018). Improving the analysis of biogeochemical patterns associated with internal waves in the strait of Gibraltar using remote sensing images. Estuarine Coast. Shelf Sci. 204, 1–13. doi: 10.1016/j.ecss.2018.02.009
Pan X., Wong G. T. F., Shiah F.-K., Ho T.-Y. (2012). Enhancement of biological productivity by internal waves: observations in the summertime in the northern south China Sea. J. Oceanography 68 (3), 427–437. doi: 10.1007/s10872-012-0107-y
Pineda J. (1991). Predictable upwelling and the shoreward transport of planktonic larvae by internal tidal bores. Science 253 (5019), 548–549. doi: 10.1126/science.253.5019.548
Pope N. D., Widdows J., Brinsley M. D. (2006). Estimation of bed shear stress using the turbulent kinetic energy approach–a comparison of annular flume and field data. Continental Shelf Res. 26 (8), 959–970. doi: 10.1016/j.csr.2006.02.010
Puig P., Palanques A., Guillen J. (2001). Near-bottom suspended sediment variability caused by storms and near-inertial internal waves on the ebro mid continental shelf (NW Mediterranean). Mar. Geology 178 (1-4), 81–93. doi: 10.1016/S0025-3227(01)00186-4
Quaresma L. S., Vitorino J., Oliveira A., da Silva J. (2007). Evidence of sediment resuspension by nonlinear internal waves on the western Portuguese mid-shelf. Mar. Geology 246 (2-4), 123–143. doi: 10.1016/j.margeo.2007.04.019
Reid E. C., DeCarlo T. M., Cohen A. L., Wong G. T. F., Lentz S. J., Safaie A., et al. (2019). Internal waves influence the thermal and nutrient environment on a shallow coral reef. Limnology Oceanography 64 (5), 1949–1965. doi: 10.1002/lno.11162
Roder C., Fillinger L., Jantzen C., Schmidt G. M., Khokiattiwong S., Richter C. (2010). Trophic response of corals to large amplitude internal waves. Mar. Ecol. Prog. Ser. 412, 113–128. doi: 10.3354/meps08707
Shi B. W., Yang S. L., Wang Y. P., Bouma T. J., Zhu Q. (2012). Relating accretion and erosion at an exposed tidal wetland to the bottom shear stress of combined current–wave action. Geomorphology 138 (1), 380–389. doi: 10.1016/j.geomorph.2011.10.004
Shimeta J., Amos C. L., Beaulieu S. E., Katz S. L. (2003). Resuspension of benthic protists at subtidal coastal sites with differing sediment composition. Mar. Ecol. Prog. Ser. 259, 103–115. doi: 10.3354/meps259103
Stapleton K. R., Huntley D. A. (1995). Seabed stress determinations using the inertial dissipation method and the turbulent kinetic energy method. Earth Surface Processes Landforms 20 (9), 807–815. doi: 10.1002/esp.3290200906
Su M., Lin Z., Wang C., Kuang Z., Liang J., Chen H., et al. (2020). Geomorphologic and infilling characteristics of the slope-confined submarine canyons in the pearl river mouth basin, northern south China Sea. Mar. Geology 424, 106166. doi: 10.1016/j.margeo.2020.106166
Syamsudin F., Taniguchi N., Zhang C., Hanifa A. D., Li G., Chen M., et al. (2019). Observing internal solitary waves in the lombok strait by coastal acoustic tomography. Geophysical Res. Lett. 46 (17-18), 10475–10483. doi: 10.1029/2019gl084595
Taki K. (2000). Critical shear stress for cohesive sediment transport. Coast. Estuar. Fine Sediment Processes 3, 53–61. doi: 10.1016/s1568-2692(00)80112-6
Taucher J., Stange P., Algueró-Muñiz M., Bach L. T., Nauendorf A., Kolzenburg R., et al. (2018). In situ camera observations reveal major role of zooplankton in modulating marine snow formation during an upwelling-induced plankton bloom. Prog. Oceanography 164, 75–88. doi: 10.1016/j.pocean.2018.01.004
Tian Z., Jia Y., Chen J., Liu J. P., Zhang S., Ji C., et al. (2021a). Internal solitary waves induced deep-water nepheloid layers and seafloor geomorphic changes on the continental slope of the northern south China Sea. Phys. Fluids 33 (5), 053312. doi: 10.1063/5.0045124
Tian Z., Jia Y., Du Q., Zhang S., Guo X., Tian W., et al. (2021b). Shearing stress of shoaling internal solitary waves over the slope. Ocean Eng. 241, 110046. doi: 10.1016/j.oceaneng.2021.110046
Trudnowska E., Lacour L., Ardyna M., Rogge A., Irisson J. O., Waite A. M., et al. (2021). Marine snow morphology illuminates the evolution of phytoplankton blooms and determines their subsequent vertical export. Nat. Commun. 12 (1), 2816. doi: 10.1038/s41467-021-22994-4
Turner J. T. (2015). Zooplankton fecal pellets, marine snow, phytodetritus and the ocean’s biological pump. Prog. Oceanography 130, 205–248. doi: 10.1016/j.pocean.2014.08.005
Valipour R., Boegman L., Bouffard D., Rao Y. R. (2017). Sediment resuspension mechanisms and their contributions to high-turbidity events in a large lake. Limnology Oceanography 62 (3), 1045–1065. doi: 10.1002/lno.10485
van Haren H. (2020). Challenger deep internal wave turbulence events. Deep Sea Res. Part I: Oceanographic Res. Papers 165, 103400. doi: 10.1016/j.dsr.2020.103400
Wang B. J., Bogucki D. J., Redekopp L. G. (2001). Internal solitary waves in a structured thermocline with implications for resuspension and the formation of thin particle-laden layers. J. Geophysical Research: Oceans 106 (C5), 9565–9585. doi: 10.1029/2000jc900101
Wang Y.-H., Dai C.-F., Chen Y.-Y. (2007). Physical and ecological processes of internal waves on an isolated reef ecosystem in the south China Sea. Geophysical Res. Lett. 34 (18), L18609. doi: 10.1029/2007gl030658
Wang H., Jia Y., Ji C., Jiang W., Bian C. (2022). Internal tide-induced turbulent mixing and suspended sediment transport at the bottom boundary layer of the south China Sea slope. J. Mar. Syst. 230, 103723. doi: 10.1016/j.jmarsys.2022.103723
Yin S., Lin L., Pope E. L., Li J., Ding W., Wu Z., et al. (2019). Continental slope-confined canyons in the pearl river mouth basin in the south China Sea dominated by erosion, 2004–2018. Geomorphology 344, 60–74. doi: 10.1016/j.geomorph.2019.07.016
Zhang W., Didenkulova I., Kurkina O., Cui Y., Haberkern J., Aepfler R., et al. (2019). Internal solitary waves control offshore extension of mud depocenters on the NW Iberian shelf. Mar. Geology 409, 15–30. doi: 10.1016/j.margeo.2018.12.008
Zhang Q., Gong Z., Zhang C., Lacy J. R., Jaffe B. E., Xu B. (2018). Bed shear stress estimation under wave conditions using near-bottom measurements: comparison of methods. J. Coast. Res. 85, 241–245. doi: 10.2112/si85-049.1
Zhang S., Zhu J., Jia Y., Li S., Chen R., Chen X., et al. (2022). Submarine small-scale features of cyclic steps in the penghu canyon: implications for the migration of canyon. J. Mar. Sci. Eng. 10 (9), 1301. doi: 10.3390/jmse10091301
Zhu C., Li S., Chen J., Wang D., Song X., Li Z., et al. (2023b). Nepheloid layer generation by gas eruption: unexpected experimental results. J. Oceanology Limnology. 41(2). doi: 10.1007/s00343-022-2108-z
Keywords: internal solitary waves, benthic organisms, the Shenhu continental slope, in-situ observation, the bottom boundary layer
Citation: Feng X, Wang L, Ji C, Wang H, Zhu C and Jia Y (2023) The impact of internal solitary waves on deep-sea benthic organisms on the continental slope of the northern South China Sea. Front. Mar. Sci. 10:1184397. doi: 10.3389/fmars.2023.1184397
Received: 11 March 2023; Accepted: 28 April 2023;
Published: 15 May 2023.
Edited by:
Wei Li, South China Sea Institute of Oceanology (CAS), ChinaReviewed by:
Shumin Gao, Hainan Tropical Ocean University, ChinaCopyright © 2023 Feng, Wang, Ji, Wang, Zhu and Jia. This is an open-access article distributed under the terms of the Creative Commons Attribution License (CC BY). The use, distribution or reproduction in other forums is permitted, provided the original author(s) and the copyright owner(s) are credited and that the original publication in this journal is cited, in accordance with accepted academic practice. No use, distribution or reproduction is permitted which does not comply with these terms.
*Correspondence: Chaoqi Zhu, emh1Y2hhb3FpQG91Yy5lZHUuY24=; Yonggang Jia, eW9uZ2dhbmdAb3VjLmVkdS5jbg==
Disclaimer: All claims expressed in this article are solely those of the authors and do not necessarily represent those of their affiliated organizations, or those of the publisher, the editors and the reviewers. Any product that may be evaluated in this article or claim that may be made by its manufacturer is not guaranteed or endorsed by the publisher.
Research integrity at Frontiers
Learn more about the work of our research integrity team to safeguard the quality of each article we publish.