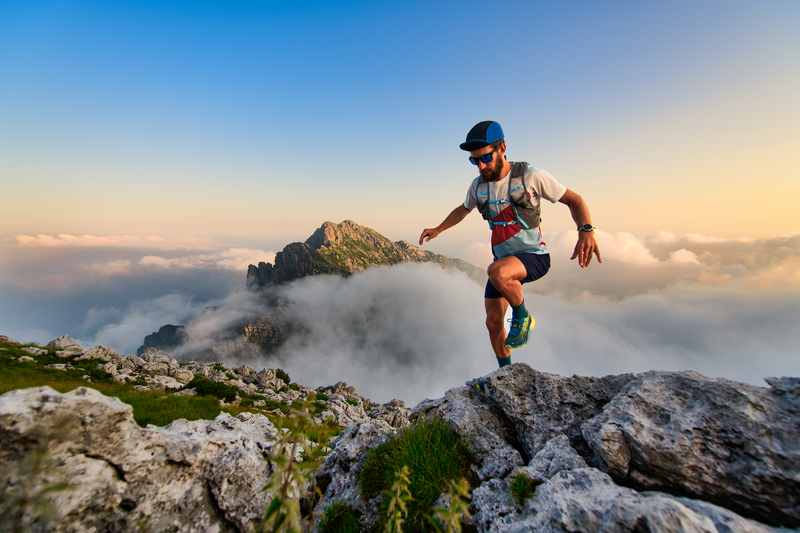
95% of researchers rate our articles as excellent or good
Learn more about the work of our research integrity team to safeguard the quality of each article we publish.
Find out more
ORIGINAL RESEARCH article
Front. Mar. Sci. , 19 July 2023
Sec. Marine Biology
Volume 10 - 2023 | https://doi.org/10.3389/fmars.2023.1183728
This article is part of the Research Topic Impact of Climate Change on Coastal Environmental Variability and Aquatic Physiology View all 5 articles
Introduction: The rapid increase in sea-water temperatures and frequency of extreme thermal events have amplified the risk of functional extinction of Mediterranean species such as the endemic long-lived seagrass Posidonia oceanica. Because of the valuable ecological functions and ecosystem services the species provides, understanding the life-stage specific thermal vulnerability is crucial to accurately predict the consequences of current and future global climate change and to protect and conserve existing meadows.
Methods: To this end, here we report a study on the ontogeny-specific thermal sensitivity of important physiological functions (i.e. respiration and net production) of three different early life history stages of P. oceanica, namely seed, seedling (4-month-old individuals) and 16-month-old plantlet by measuring thermal performance curves (eleven temperatures treatments between 15-36°C with n=8).
Results: All three stages examined showed photosynthetic activity during light exposure with similar optimal temperatures for both net and gross production. Gross photosynthesis increased with rising temperature up to 28-30°C, subsequently declining at higher temperatures until complete inhibition at 36°C. The metabolic response of seeds was found to be temperature-dependent up to 26°C, while respiration of seedlings and plantlets was almost stable up to 28-30°C, but increased markedly at higher temperatures, resulting in a negative whole-plant C balance at temperatures above 32°C. Overall, our results show that seedlings and plantlets tolerate a wider temperature range (15 - 32°C) than seeds, which experience metabolic and physiological dysfunction from 26-28°C onwards.
Discussion: These findings suggest that the impact of warming on recruitment in P. oceanica meadows may vary depending on the timing of marine heatwaves (i.e. mid-spring to mid-autumn) and provide useful knowledge to inform restoration programs using early life stages of the species. In conclusion, the study of physiological responses during the early life stages of species is key to identify life history stages that are particularly vulnerable to climate change, which is vital knowledge for ecosystem management and conservation.
The importance of the Mediterranean Sea as a hotspot for climate change has long been recognized, as this sea is a semi enclosed basin highly sensitive to the influences from surrounding land masses and local and global climate-induced drivers (Rilov et al., 2021). According to the IPCC (2022) latest report, the Mediterranean basin is warming at an average rate 20% faster than the global annual mean sea surface temperature and it is strongly experiencing the effects of global warming, especially in the form of heat waves, whose frequency and intensity are expected to increase in the coming decades (Darmaraki et al., 2019).
The impacts of climate change in this area are also exacerbated by strong non-climatic environmental stressors, such as pollution, unsustainable use of marine space and spread of non-indigenous species (Cramer et al., 2018; Ramírez et al., 2018), which further increase the risk of functional extinction or even decline of Mediterranean species (Coma et al., 2009; Garrabou et al., 2009). The Mediterranean endemic seagrass Posidonia oceanica is considered highly vulnerable (Marbà and Duarte, 2010; Boudouresque et al., 2021) and its meadows are currently facing alarming regression with major impact on both their extent and the ecosystems services they provide (Orth et al., 2006; Campagne et al., 2015; Telesca et al., 2015; Blanco-Murillo et al., 2022). Recent estimates project habitat losses of up to 75% by 2050 and even worst scenarios for 2100 (Chefaoui et al., 2018; IPCC, 2022).
Although P. oceanica meadows cover a very small area worldwide compared to other marine angiosperms, they are considered among the most valuable costal ecosystems in the Mediterranean (Personnic et al., 2014). The massive supra-littoral deposit of leaf litter from P. oceanica (i.e. banquettes) contribute to reduce coastal erosion, protect from sea level rise and storming events and, because of the structural complexity of the meadows, they 1) support high biodiversity serving as refuge and/or nursery ground for many vertebrates and invertebrates’ species and 2) enhance water quality by trapping suspended matter and limiting the negative effects associated with resuspension events (McKenzie, 2008; Campagne et al., 2015; Ruiz-Frau et al., 2017; Pergent et al., 2018). Over the last decades, P. oceanica meadows have earned more attention in terms of their role in Blue Carbon fixation and sequestration, which in many cases has been compared to or even considered higher than that of terrestrial forests (e.g. Costanza et al., 1997; Vassallo et al., 2013). It has been suggested that carbon sequestration by P. oceanica accounts for a significant portion of the CO2 emission/release of various Mediterranean countries (Pergent-Martini et al., 2021). In addition, P. oceanica meadows can buffer seawater acidification through their intense metabolic activity, benefiting the calcifying organisms living within the meadows (Hendriks et al., 2014). Therefore, despite being identified as potential victim of climate change, P. oceanica can also play a key role in coping with the effects of climate change, mitigating its impacts and contributing to future climate adaptation (Gattuso et al., 2018).
P. oceanica is a slow-growing, long-lived species that reproduces both asexually (i.e. clonal growth through rhizome propagation) and sexually (i.e. through flowering and seeds). Sexual reproduction of the species begins in autumn with the appearance of the first inflorescences, which, once pollinated, develop relatively large fruits (sea olives) that, when ripen, are released from the mother plant in the spring of the following year (Balestri, 2004). These fruits are buoyant and travel for some time, carried by winds and currents, before dehiscence and the release of the single non-dormant seed they contain, which sinks to the sea bottom where it germinates and roots (Guerrero-Meseguer et al., 2018). A particularity of P. oceanica seeds is their photosynthetic capacity, which is key for their germination and subsequent seedling development, as it contributes to satisfy part of the energy demand needed during this early life stage of the species (Celdrán and Marín, 2013; Guerrero-Meseguer et al., 2018). Sexual reproduction is vital to preserve genetic variability and thus the resilience of meadows to environmental changes (Procaccini et al., 2007), as well as to colonize new areas (McMahon et al., 2014). Flowering in natural populations, which is highly variable in space and time, has increased in frequency and intensity in correlation with warming in recent decades (Diaz-Almela et al., 2007) and the induction of flowering by heat has recently been experimentally demonstrated (Ruiz et al., 2018). Hence, the species could be more plastic and potentially more resilient than previously thought, offering a more hopeful future for its potential to adapt to ocean warming (Marín-Guirao et al., 2019; Martínez-Abraín et al., 2022). However, the early life stages of the species are apparently more vulnerable to increased temperatures than adults, which could affect population recruitment rates, with potential consequences for the species’ ability to adapt and survive in a future scenario of climate change (Guerrero-Meseguer et al., 2017; Hernán et al., 2017).
Exposure to heat reduces growth and carbohydrate content, while increasing leaf senescence and seedling mortality as consequence of the physiological and metabolic alterations produced in the seedlings by extreme temperatures (Guerrero-Meseguer et al., 2017; Hernán et al., 2017). In seagrasses, indeed, exposure to high temperatures increases respiration rate in comparison to photosynthesis, and consequently reduces the photosynthesis/respiration ratio, leading to carbon unbalances that can impact plant growth and survival (Lee et al., 2007; Nguyen et al., 2021).
P. oceanica is particularly sensitive to alterations in its carbon balance as the species relies on carbon stocks to meet its metabolic and growth needs during the winter season (Alcoverro et al., 2001). Since these reserves for long-term use are produced and stored during the spring-summer period, when abnormally high temperatures are most likely to occur, any warming-induced alterations on plant carbon metabolism during this critical phase of the species’ annual cycle could compromise its long-term survival (Marín-Guirao et al., 2018). In this case, although the whole energy production (i.e. gross production) may increase under warming conditions, most of this energy would be allocated to maintenance processes (i.e. respiration), resulting in an overall decrease in the energy available for storage (i.e. net production). In this perspective, metabolic rates and thermal performance (i.e. thermal reaction norms for organismal performance; Huey and Stevenson, 1979) of plants adequately reflect the energetic costs of adaptation to a particular thermal niche and not just a simple response to temperature (Magozzi and Calosi, 2015; Schulte, 2015). As a main consequence, it is imperative to disclose the functional response of the early life stages of P. oceanica to provide more accurate predictions about the resilience of this species to future climate trends and to inform about restoration strategies using seeds and seedlings.
To this end, here we report a study on the ontogeny-specific thermal sensitivity of important physiological functions (i.e. respiration and net production) of three different early life history stages of P. oceanica, namely seed, seedling (4-month-old individuals) and 16-month-old plantlet by measuring photosynthesis rate and respiration to build stage-dependent thermal performance curves, that is the explicit relation among the changes in temperature and the changes in the organisms performance (Kingsolver and Buckley, 2020).
For this study, more than 400 P. oceanica seeds were extracted from beach-cast fruits in late May 2021 and 2022 along the coast of Isola delle Femmine (Northern Sicily; N 38.187948, E 13.239630). According to genetic assignment (i.e. measures of genetic distance) and Lagrangian modelling (Procaccini et al., 2023), Posidonia fruits found in the area mostly derive from the extensive meadow growing nearby on different types of substratum.
After collection, seeds were immediately transported to the laboratory and transferred to tanks with continuous flow-through of natural seawater under a 12 h:12 h light:dark photoperiod (80 umol q m-2s-1), where they germinated and grew to obtain about 200 plantlets older than 1 year (i.e. 16-month- old plantlets starting from seed collected in 2021) and almost 200 4-month-old seedlings. In May 2022, a new sampling was carried out to obtain another 200 seeds from beach-collected fruits. Seeds were placed in experimental tanks under constant conditions (T 20°C; salinity 35-36 psu; 80 umol q m-2s-1) where they were left to acclimate for a few days to carry out physiological measurements prior to germination and seedling development. Seedling and plantlets were acclimated into 60-L aquaria, each equipped with lamps and connected to a heating/cooling system to maintain homogeneous conditions. During the acclimation period salinity and pH were monitored daily using a multiparameter probe.
Before starting metabolic and physiological measurements, individuals of the three developmental stages (i.e. seeds, seedlings and plantlets) were progressively warmed/cooled from the acclimation temperature (20°C; according to the sea surface temperatures [SST] measured about 1 meter below the surface by the Italian Oceanographic Buoy Network; www.mareografico.it; Supplementary Figure 1) to their respective experimental temperature using a temperature ramping of 1°C per day (Said et al., 2021). Once the experimental temperature was reached, eight individuals of similar size from each stage were selected, gently cleaned of epiphytes and used for physiological and metabolic measurements.
To assess the thermal tolerance of early life stages of P. oceanica, metabolic (respiration) and photosynthetic rates were measured after 24 hours of exposure to 11 different temperatures (15, 18, 20, 22, 24, 26, 28, 30, 32, 34 and 36°C). The maximum temperature used for the seeds was 30°C, as higher temperatures are considered outside the current and projected thermal range of the period when the seeds of the species are released (May).
Oxygen production/consumption was estimated for eight individuals of each developmental stage incubated in sealed, transparent glass chambers filled with filtered seawater (filter porosity 0.45 µm): 33 ml for seeds, 275 ml for seedlings and 560 ml for plantlets. Eight independent chambers were used for physiological measurements, each containing either one seed, seedling or plantlet of P. oceanica, while four additional control chambers, containing only filtered seawater, were used to correct for possible changes in oxygen concentration. To perform physiological and metabolic measurements, each replicate was tested against one temperature treatment only. As some assays were repeated, the number of replicates could vary. Chambers were placed in a seawater tank (150 L) connected to a chiller-heater unit (Hailea HC 300A), to keep the experimental temperature stable ( ± 0.5 °C) during the measurements. Light was provided by natural spectrum trisphosphor lamp units (Solarmax 20 W) suspended at a fixed height above the chambers to produce a homogeneous light field of 95 ( ± 5) μmol photons s-1m-2 and monitored throughout the whole experiment by means of the HOBO pendant MX2202 dataloggers. The water inside the chambers was continuously mixed by magnetic stirrers and dissolved oxygen concentrations were measured using Bare optical fiber REDFLASH technology sensors (Pyroscience) inserted through the chamber wall and connected via a 4-channel meter to a computer recording mg l-1 of O2. Before starting the oxygen recordings, the individuals inside the chambers were acclimatized to darkness for 15 min to limit the photorespiration (i.e. the process by which plants consume oxygen and release carbon dioxide during photosynthesis; Tait and Schiel, 2010). Dissolved oxygen concentration was then monitored every second for at least 30 min after the slope of dissolved oxygen versus time was stabilized, and then the light was turned on and photosynthetic rates were measured for another 30 min (Figure 1). Oxygen production (net primary production, NP) and respiration (Rd) were measured respectively under light and dark conditions in individuals and were calculated from the slope between the initial and final concentrations of oxygen, standardized by the incubation time, the volume of water within each chamber and the dry weight of incubated individuals. Gross-photosynthesis (GP) was calculated as the sum of NP and Rd, i.e. GP = NP + Rd (e.g Falkowski and Raven, 2013). Before each incubation, the oxygen electrodes were calibrated using the manufacturer’s 1-point method (100% air saturated water).
Figure 1 Schematic rappresentation of the physiological measurements performed on seeds, seedlings and plantlets. Curves are obtained by averaging the thermal response of each life stage within one hour interval recordings. Shaded areas represent the interval of 10 mins excluded in analyzing respiration data while the orange line indicate the time each life stage needed to respond to light stimulus (as indicated by the change in the slope) after light was turned-on.
At the end of the incubations, individuals were removed from the chambers and their wet and dry weights (48 h at 60°C) were determined (precision ±0.0001 g). For seedlings and plantlets, above-ground (leaves) and below-ground (roots and rhizomes) tissues were dissected to measure their weights separately.
Exponential regressions were used to fit the temperature-dependent respiration curves by using the software program OriginPro 2022 (OriginLab Corporation, Northampton MA, United States).
Thermal performance curves were estimated using the package rTPC (Padfield et al., 2021) in R environment, launching and comparing a total of 9 different non-linear least squares TPC models chosen as they include (at least) the T optimum as a parameter in the equation (Supplementary Figure 2). Whenever the experimental individuals were less responsive, they were omitted from the analysis.
Among the models satisfying such a criterion, the parameters obtained from model fitting were only kept by comparing the goodness of fit (i.e. presenting the lowest Akaike Information Criterion score) and if the predicted temperatures are close to the temperature range of the experiment (i.e., 15-36°C). Bootstrapping was used to calculate 95% prediction limits for the selected TPC model and confidence intervals around its optimum temperature.
The analysis of photosynthetic thermal performance curves (TPC) selected the Joehnk et al. (2008) model as the best fit for seed gross photosynthesis (GP) data, while the Lobry-Rosso-Flandros (LRF; Rosso et al., 1993) model and the Thomas et al. (2012) model were the best fits to seedlings and plantlets GP respectively (Figure 2; Supplementary Tables 1A–C). In general, gross photosynthesis increased with increasing temperature up to a threshold after which it began to decrease. In seeds, this threshold was observed at 26°C with an average rate of 0.168 ± 0.020 O2 mg gDW-1 h-1, but higher for seedlings and plantlets where GP rates respectively doubled and quadrupled (28-30°C; Figure 2). GP rates were only negative at the maximum experimental temperatures, between 34-35°C for seedlings and between 35-36°C for plantlets (Figure 2). In all three examined stages of the seagrass, the modelled TPCs indicated the optimum temperatures for GP values slightly above 26°C and predicted the maximum critical temperature of 32.1°C, 34.7°C and 35.5°C respectively in seeds, seedlings and plantlets (Table 1). Regardless of the model used, both the predicted thermal breadths (i.e. the range of temperatures over which a curve’s rate is at least 0.8 of peak) and the safety margin values (as expressed by the difference between the CTmax and the Topt) were higher in plantlets compared to the other two stages. Specifically, as shown in Table 1, the thermal breadth was 11.63°C and the thermal safety margin covered a range of 9.05°C, while the same values never exceeded 7.97°C and 9.86°C in seedlings.
Figure 2 Respiration rate (R[d], n = 4-16; left) and gross photosynthesis (GP, n = 3-15; right) of the three life stages of P. oceanica at increasing temperatures. Rates were base on total Dry Weight (DW), including roots. R[d] and GP data where fitted with exponential growth equations and a set of nonlinear least square regression models, respectively, see Methods for details; thick lines represent the regression while the faded bands represent the 95% confidence interval. R[d] indicates that respiration data are showed in absolute value. In seeds, respiration rates measured at temperatures of 28-30°C were removed from data fit since they represents the critical treshold and indicates the failure of compensatory mechanisms supporting the thermal response.
Table 1 Parameter estimation for GP modelled for each life stage. rmax = maximum rate; Topt, optimum temperature; CTmin, critical thermal minimum; CTmax, Critical thermal maximum; e, activation energy; eh, deactivation energy; Q10, Q10 value.
Seed respiratory metabolic activity increased with increasing temperature up to 26°C, where the maximum rate was 0.339 ± 0.013 O2 mg gDW-1 h-1, while respiration of seedlings and plantlets remained almost stable up to 28-30°C. Specifically, the average rate of respiration across the range of temperatures 15-30°C was 0.205 O2 mg gDW-1 h-1 and 0.249 O2 mg gDW-1 h-1 respectively for seedlings and plantlets. At higher temperatures, however, the rate increased exponentially to 2- and 3-fold higher values at 36°C (Figure 2).
Net production, as an indicator of plant carbon balance, remained stable in seeds throughout the experimental temperature range (15-30°C), but rates were always negative. In the case of seedlings and plantlets, NP remained positive up to 30°C and 32°C respectively, becoming negative at higher temperatures (Figure 3).
Figure 3 Photosynthesis (net primary production, NP; n = 4-16, mean ± SE) vs temperature obtained from oxygen measurements performed under light conditions for the three life stages of P. oceanica. Rates were base on total Dry Weight (DW), including roots.Values positioned below the dashed lines indicate the temperatures at which the carbon balance was negative.
The analysis of metabolic and (photo)physiological thermal performance of three early life stages of the iconic seagrass P. oceanica (seeds, 4-month-old seedlings and 16-month-old plantlets) along a comprehensive temperature gradient showed that vulnerability to rising temperatures is age-dependent as it varies with the developmental stage of individuals (Figure 4). The metabolic activity and physiology of the three stages show a close relationship with temperature, with photosynthetic yield and respiratory activity increasing following the rising of temperature, thus maintaining unaltered carbon balances over a relatively wide range of temperatures. However, at stage-specific thermal thresholds, which progressively increases during ontogenesis, the photosynthetic performance begins to decline, while respiration acutely rises in seedlings and plantlets. This heat sensitivity is likely due to heat-induced damage on the structure and functioning of the photosynthetic apparatus, inactivation of photosynthetic enzymes (rubisco activase) and/or pigments degradation (Wahid et al., 2007). Once these thermal thresholds are exceeded, the production rates (net photosynthesis) are impacted, compromising the carbon balance of individuals and threatening their survival. Notably, as the age of the individuals increases, this thermal threshold occurs at progressively higher temperatures. Our results confirmed that P. oceanica seeds are photosynthetically active (Celdrán and Marín, 2011; Celdrán and Marín, 2013; Guerrero-Meseguer et al., 2018) and highlighted an increase in gross photosynthetic rates up to a maximum at 26°C, and a subsequent decrease at higher temperatures. Interestingly, the respiratory metabolic activity of seeds showed a similar trend, responding positively to increasing temperature and decreasing at the same temperature threshold (26°C). The reduction in metabolic consumption rates along with the decrease in photosynthetic performance likely reflect the onset of an energetically conservative response rather than mitochondrial disfunction; in fact the temperature at which respiration peaks is usually much higher than the temperature at which photosynthesis peaks (Crous et al., 2022) as seen for seedlings and plantlets. A similar homeostatic response has been observed in adults of the same species subjected to increasing temperature as a possible strategy to conserve energy reserves, required by the species to overwinter, while maintaining an efficient response to cope with the effect of heat stress (Marín-Guirao et al., 2018). In seeds, energy reserves (starch) are fundamental during the first months of seedling development, contributing to up to 65% and 47% leaf and root biomass respectively (Celdrán and Marín, 2011) until a stage of maturity capable of fully supporting the carbon demands of the plant is reached (Celdrán and Marín, 2013). Therefore, regulating the process involved in respiration would provide the seeds the possibility to keep their production rates (net P) unaltered, and to spare their energy reserves, over the temperature range studied. Indeed, although net photosynthetic rates were negative at all experimental temperatures since the rate of oxygen production was lower than the rates of oxygen consumption, the resulting photosynthetic activity of the seeds not only helps to prevent the depletion of starch reserves and the initial development of the seedlings, but it is also key to their germination (Celdrán and Marín, 2011; Guerrero-Meseguer et al., 2018). This vital role of photosynthesis for P. oceanica seeds, together with the high sensitivity of this process to rising temperatures, suggest that warming or extreme heat events during the period when they are released from fruits could affect viability, by shrinking the window suitable for germination. It is therefore possible that in a future climate change scenario, seeds could represent a bottleneck for natural recruitment rates of P. oceanica meadows or the colonization of new areas.
Figure 4 Summary model of the stage-specific photosynthetic performance under increasing temperature. The figure shows age-dependent photosynthetic thermal range as indicated by the orange arrows for the seeds, seedlings and plantlets of P. oceanica. The photosynthetic performances were progressively higher during the development (blue arrow). The photosynthetic thermal tolerance of seedlings and plantlets was higher than that of seeds, increasing at rising temperature until complete inhibition (Lethal Thermal Threshold, red box). The green box reveals that the optimum Photosynthetic performance is consistent across all examined developmental stages (images are modified from vecta.io and actaplantarum.org).
The breadth of thermal performance for photosynthesis of seedlings and plantlets was larger than that of seeds, reaching maximum photosynthetic rates at 28-30°C and then decreasing to complete inhibition at 34-36°C. The photosynthetic TPC models, indeed, showed a clear age-dependent photosynthetic thermal sensitivity as indicated by the values of thermal tolerance, thermal safety margins, critical maximum temperatures (CT_max) and safety margin values, which were progressively higher with the developmental stage of the individuals (seeds < 4-month-old seedlings < 16-month-old plantlets; Table 1).
The thermal impact on gross photosynthesis of seedlings and plantlets at high temperatures could be associated with heat stress-induced damage to photosystems II (PSII) as significant reductions in photochemical rates of 5-month-old seedlings have been described at temperatures ≥ 31°C along with increases in basal fluorescence (F0) (Guerrero-Meseguer et al., 2017). Our results also suggest that juvenile stages of P. oceanica are more sensitive to increased temperatures than adult individuals, as the latter tolerate temperatures of 30-32°C without showing evidence of significant photosynthetic alterations or damage to the photosynthetic apparatus (Marín-Guirao et al., 2016; Ontoria et al., 2019), although this could vary depending on the local adaptation of the plants to their natural thermal environments (Marín-Guirao et al., 2017; Marín-Guirao et al., 2018; Bennett et al., 2022; Stipcich et al., 2022a). Deterioration of the photosynthetic apparatus when the temperature exceeds tolerance thresholds can lead to an increase in seedlings necrotic surfaces, as it has been described in 3 and 5-month-old seedlings after longer exposures (1-3 months) to temperatures between 29-33°C (Guerrero-Meseguer et al., 2017; Hernán et al., 2017; Pereda-Briones et al., 2019; Guerrero-Meseguer et al., 2020). These seedlings also showed a significant reduction in growth rates, biomass and leaf area, as well as in their root system, all symptoms of reduced energy status as a consequence of prolonged exposure to high temperatures (Wahid et al., 2007; Bita and Gerats, 2013). In our study, simultaneously with the reduction in photosynthetic yield, seedlings and plantlets exponentially increased their metabolic demands, resulting in a negative plant C balance. This suggests that exposures to temperatures above 32°C for more than 24h could represent a critical point for the maintenance of metabolic needs and thus for their longer-term survival. Increased metabolic demands at high temperatures (> 29°C) led to a significant reduction in the starch content of seedlings after several weeks, ultimately leading to increased mortality (Guerrero-Meseguer et al., 2017; Hernán et al., 2017).
Our results also indicate that the lethal temperature for early stages of P. oceanica should be between 34°C and 36°C. Indeed, within these temperatures range, seedling and plantlet showed a marked change in the responses. Specifically, no recovery signs were recorded after light was turned-on (i.e. NP was negative, as indicated by the slope of the respiration vs temperature curve; Supplementary Figure 3) and the water within the experimental chambers became viscous. Also, the leaves presented symptoms of deterioration losing their green colour, in some cases with the tips that were becoming transparent and overall resulting in a whole negative C-balance for both stages (Figure 3; Supplementary Figures 3, 4). Beyond that thermal threshold, no recovery was observed neither in those individuals re-acclimated at 20°C after one month from exposure to experimental temperature of 36°C (Supplementary Figure 5).
Temperature is widely known to be one of the main ecological factors that determines seagrass performance, survival and distribution limits. Temperatures close to the threshold of 30°C have been shown to negatively affect seedlings development, leaf formation rates and leaf biomass as well as to increase the vulnerability of newly germinated seedlings to extreme events (Olsen et al., 2012; Guerrero-Meseguer et al., 2017; Mutlu et al., 2022). High water temperatures significantly affect the photosynthetic rate of seagrass, decreasing oxygen production (Rasmusson et al., 2020), PSII performance and growth rates (Ontoria et al., 2019), while increasing leaf necrosis and mortality events (Collier and Waycott, 2014; Pazzaglia et al., 2022b). Raising temperatures may also alter seagrass distributions and meadow density, directly affecting flowering dynamics (Diaz-Almela et al., 2007).
Despite the recognized sensitivity of the species to warming, the modelled thermal optima and limits used here showed that sensitivity could vary among the different life stages of this species confirming that in seagrass ecosystems, intraspecific variation is likely to play a more important role for successful distribution than in terrestrial ecosystems, to compensate their low species richness worldwide (Moreira-Saporiti et al., 2023) Specifically, the relatively narrower tolerance and safety margin for seeds and seedlings compared to adults confirmed that these life stages may be more vulnerable to projected increases in temperature for the Mediterranean basin (Balestri et al., 2009; Guerrero-Meseguer et al., 2020), particularly considering their important role in ensuring dispersal, colonization of new sites and the establishing of new genotypes in existing seagrass populations (Balestri and Cinelli, 2003; Martínez-Abraín et al., 2022).
Since the beginning of 2000’s the Mediterranean Sea has experienced strong marine heat waves over more than 75% of its surface. As a result, several Mediterranean coastal species are increasingly subject to greater heat stress, increasing the risk of mortality. However, the experimental thermal limits used here are derived from seagrass physiological responses to temperature conditions and no other stressors, while instead we recognize that other additive interactions e.g. herbivory) or other sources of disturbance (e.g. anchoring) are commonly reported in seagrass studies and that species thermal limits could vary under multiple stressing conditions (e.g. Pereda-Briones et al., 2019; Guerrero-Meseguer et al., 2020).
Moreover, response to thermal stress can vary according to the environmental quality of single populations, where patches exposed to higher nutrient load can suffer more to increased water temperature (Pazzaglia et al., 2020; Pazzaglia et al., 2022b).
Also, as both adults and juveniles of P. oceanica have been shown to have thermal stress memory and epigenetic memory, growth in extreme environments would make the species more resilient to ocean warming with important repercussions for future restoration programs (Nguyen et al., 2020; Pazzaglia et al., 2022a; Stipcich et al., 2022b). Indeed, since sensitivity to warming decreases with age, the timing of a heat wave may have a different impact on new Posidonia recruits, i.e. the later in the autumn the extreme temperature event occurs, the lesser could be the impact. Additionally, marine heatwaves have occurred more frequently in recent years and are predicted to become more frequent as a result of the long-term warming SST, increasing the threat to marine ecosystems and socioeconomic activities that depend on them (Marbà and Duarte, 2010; Morley et al., 2017; Frölicher et al., 2018; Wernberg et al., 2021; Stipcich et al., 2022c).
In conclusion, our findings suggest that the impact of marine/ocean warming on recruitment in P. oceanica meadows may vary depending on the timing of marine heatwaves (i.e. mid-spring to mid-autumn) and provide useful knowledge to inform restoration programs using early life stages of the species. Therefore, the study of physiological responses during the early life stages of species is key to identify life history stages that are particularly vulnerable to climate change, which is vital knowledge for ecosystem management and conservation.
The raw data supporting the conclusions of this article will be made available by the authors, without undue reservation.
AR, MM, and VM conceived the study and performed the experiments. AR performed data analysis and with VM drafted the early version of this manuscript. MM conducted the fieldwork and the seagrass maintenance in the laboratory. FB, GD’A, SM, LM-G and GP contributed to manuscript revision. All authors contributed to the article and approved the submitted version.
This study was supported by the project Marine Hazard, PON03PE_00203_1, Italian Ministry of Education, University and Research (MIUR).
We are grateful to Dr. Arturo Zenone and Dr. Maximiliano Giacalone for the help in collecting seeds, and to Giuseppe Di Stefano for the support provided during fieldwork and for the assistance in the laboratory. We thank Dr. Evelyn Scicchigno for the advanced editing.
The authors declare that the research was conducted in the absence of any commercial or financial relationships that could be construed as a potential conflict of interest.
The reviewer CG declared a shared affiliation with the author GP to the handling editor at the time of review.
All claims expressed in this article are solely those of the authors and do not necessarily represent those of their affiliated organizations, or those of the publisher, the editors and the reviewers. Any product that may be evaluated in this article, or claim that may be made by its manufacturer, is not guaranteed or endorsed by the publisher.
The Supplementary Material for this article can be found online at: https://www.frontiersin.org/articles/10.3389/fmars.2023.1183728/full#supplementary-material
Alcoverro T., Manzanera M., Romero J. (2001). Annual metabolic carbon balance of the seagrass Posidonia oceanica: the importance of carbohydrate reserves. Mar. Ecol. Prog. Ser. 211, 105–116. doi: 10.3354/meps211105
Balestri E. (2004). Flowering of the seagrass Posidonia oceanica in a north-western Mediterranean coastal area: temporal and spatial variations. Mar. Biol. 145, 61–68. doi: 10.1007/s00227-004-1301-2
Balestri E., Cinelli F. (2003). Sexual reproductive success in Posidonia oceanica. Aquat. Bot. 75, 21–32. doi: 10.1016/S0304-3770(02)00151-1
Balestri E., Gobert S., Lepoint G., Lardicci C. (2009). Seed nutrient content and nutritional status of Posidonia oceanica seedlings in the northwestern Mediterranean Sea. Mar. Ecol. Progr. Ser. 388, 99–109. doi: 10.3354/meps08104
Bennett S., Alcoverro T., Kletou D., Antoniou C., Boada J., Buñuel X., et al. (2022). Resilience of seagrass populations to thermal stress does not reflect regional differences in ocean climate. New Phytol. 233, 1657–1666. doi: 10.1111/nph.17885
Bita C., Gerats T. (2013). Plant tolerance to high temperature in a changing environment: scientific fundamentals and production of heat stress-tolerant crops. Fron. Plant Sci. 4, 273. doi: 10.3389/fpls.2013.00273
Blanco-Murillo F., Fernández-Torquemada Y., Garrote-Moreno A., Sáez C. A., Sánchez-Lizaso J. L. (2022). Posidonia oceanica L.(Delile) meadows regression: long-term affection may be induced by multiple impacts. Mar. Environ. Res. 174, 105557. doi: 10.1016/j.marenvres.2022.105557
Boudouresque C. F., Blanfuné A., Pergent G., Thibaut T. (2021). Restoration of seagrass meadows in the Mediterranean Sea: a critical review of effectiveness and ethical issues. Water 13 (8), 1034. doi: 10.3390/w13081034
Campagne C. S., Salles J. M., Boissery P., Deter J. (2015). The seagrass Posidonia oceanica: ecosystem services identification and economic evaluation of goods and benefits. Mar. Poll. Bull. 97, 391–400. doi: 10.1016/j.marpolbul.2015.05.061
Celdrán D., Marín A. (2011). Photosynthetic activity of the non-dormant Posidonia oceanica seed. Mar. Biol. 158, 853–858. doi: 10.1007/s00227-010-1612-4
Celdrán D., Marín A. (2013). Seed photosynthesis enhances Posidonia oceanica seedling growth. Ecosphere 4, 149. doi: 10.1890/ES13-00104.1
Chefaoui R. M., Duarte C. M., Serrão E. A. (2018). Dramatic loss of seagrass habitat under projected climate change in the Mediterranean Sea. Glob. Change Biol. 24, 4919–4928. doi: 10.1111/gcb.14401
Collier C. J., Waycott M. (2014). Temperature extremes reduce seagrass growth and induce mortality. Mar. Poll. Bull. 83, 483–490. doi: 10.1016/j.marpolbul.2014.03.050
Coma R., Ribes M., Serrano E., Jiménez E., Salat J., Pascual J. (2009). Global warming-enhanced stratification and mass mortality events in the Mediterranean. Proc. Nat. Acad. Sci. 106, 6176–6181. doi: 10.1073/pnas.0805801106
Costanza R., d'Arge R., De Groot R., Farber S., Grasso M., Hannon B., et al. (1997). The value of the world's ecosystem services and natural capital. Nature 387, 253–260. doi: 10.1038/387253a
Cramer W., Guiot J., Fader M., Garrabou J., Gattuso J. P., Iglesias A., et al. (2018). Climate change and interconnected risks to sustainable development in the Mediterranean. Nat. Clim Change 8, 972–980. doi: 10.1038/s41558-018-0299-2
Crous K. Y., Uddling J., De Kauwe M. G. (2022). Temperature responses of photosynthesis and respiration in evergreen trees from boreal to tropical latitudes. New Phytol. 234, 353–374. doi: 10.1111/nph.17951
Darmaraki S., Somot S., Sevault F., Nabat P., Cabos Narvaez W. D., Cavicchia L., et al. (2019). Future evolution of marine heatwaves in the Mediterranean Sea. Clim. Dyn. 53, 1371–1392. doi: 10.1007/s00382-019-04661-z
Diaz-Almela E., Marbà N., Duarte C. M. (2007). Consequences of Mediterranean warming events in seagrass (Posidonia oceanica) flowering records. Glob. Change Biol. 13, 224–235. doi: 10.1111/j.1365-2486.2006.01260.x
Falkowski P. G., Raven J. A. (2013). Aquatic photosynthesis. 2nd ed. (United States: Princeton University Press).
Frölicher T. L., Fischer E. M., Gruber N. (2018). Marine heatwaves under global warming. Nature 560, 360–364. doi: 10.1038/s41586-018-0383-9
Garrabou J., Coma R., Bensoussan N., Bally M., Chevaldonné P., Cigliano M., et al. (2009). Mass mortality in northwestern Mediterranean rocky benthic communities: effects of the 2003 heat wave glob. Change Biol. 15, 1090–1103. doi: 10.1111/j.1365-2486.2008.01823.x
Gattuso J. P., Magnan A. K., Bopp L., Cheung W. W. L., Duarte C. M., Hinkel J., et al. (2018). Ocean solutions to address climate change and its effects on marine ecosystems. Front. Mar. Sci. 5. doi: 10.3389/fmars.2018.00337
Guerrero-Meseguer L., Marín A., Sanz-Lázaro C. (2017). Future heat waves due to climate change threaten the survival of Posidonia oceanica seedlings. Environ. pollut. 230, 40–45. doi: 10.1016/j.envpol.2017.06.039
Guerrero-Meseguer L., Marín A., Sanz-Lázaro C. (2020). Heat wave intensity can vary the cumulative effects of multiple environmental stressors on Posidonia oceanica seedlings. Mar. Environ. Res. 159, 105001. doi: 10.1016/j.marenvres.2020.105001
Guerrero-Meseguer L., Sanz-Lázaro C., Marín A. (2018). Understanding the sexual recruitment of one of the oldest and largest organisms on earth, the seagrass Posidonia oceanica. PloS One 13, e0207345. doi: 10.1371/journal.pone.0207345
Hendriks I. E., Olsen Y. S., Ramajo L., Basso L., Steckbauer A., Moore T. S., et al. (2014). Photosynthetic activity buffers ocean acidification in seagrass meadows. Biogeosciences 11, 333–346. doi: 10.5194/bg-11-333-2014
Hernán G., Ortega M. J., Gándara A. M., Castejón I., Terrados J., Tomas F. (2017). Future warmer seas: increased stress and susceptibility to grazing in seedlings of a marine habitat-forming species. Glob. Change Biol. 23, 4530–4543. doi: 10.1111/gcb.13768
Huey R. B., Stevenson R. D. (1979). Integrating thermal physiology and ecology of ectotherms: discussion of approaches. Am. Zool. 19, 357–366. doi: 10.1093/icb/19.1.357
IPCC (2022). Climate change 2022: impacts, adaptation and vulnerability. contribution of working group II to the sixth assessment report of the intergovernmental panel on climate change. Eds. Poürtner H.-O., Roberts D. C., Tignor M., Poloczanska E. S., Mintenbeck K., Alegriía A., Craig M., Langsdorf S., Loüschke S., Moüller V., Okem A., Rama B. (Cambridge, UK: Cambridge University Press). doi: 10.1017/9781009325844
Joehnk K. D., Huisman J. E. F., Sharples J., Sommeijer B. E. N., Visser P. M., Stroom J. M. (2008). Summer heatwaves promote blooms of harmful cyanobacteria. Glob. Change Biol. 14, 495–512. doi: 10.1111/j.1365-2486.2007.01510.x
Kingsolver J. G., Buckley L. B. Ontogenetic variation in thermal sensitivity shapes insect ecological responses to climate change. Curr Opin Insect Sci. (2020) 41, 17–24. doi: 10.1016/j.cois.2020.05.005
Lee K. S., Park S. R., Kim Y. K. (2007). Effects of irradiance, temperature, and nutrients on growth dynamics of seagrasses: a review. J. Exp. Mar. Biol. Ecol. 350, 144–175. doi: 10.1016/j.jembe.2007.06.016
Magozzi S., Calosi P. (2015). Integrating metabolic performance, thermal tolerance, and plasticity enables for more accurate predictions on species vulnerability to acute and chronic effects of global warming glob. Change Biol. 21, 181–194. doi: 10.1111/gcb.12695
Marbà N., Duarte C. M. (2010). Mediterranean Warming triggers seagrass (Posidonia oceanica) shoot mortality. Glob. Change Biol. 16, 2366–2375. doi: 10.1111/j.1365-2486.2009.02130.x
Marín-Guirao L., Bernardeau-Esteller J., García-Muñoz R., Ramos A., Ontoria Y., Romero J., et al. (2018). Carbon economy of Mediterranean seagrasses in response to thermal stress. Mar. pollut. Bull. 135, 617–629. doi: 10.1016/j.marpolbul.2018.07.050
Marín-Guirao L., Entrambasaguas L., Dattolo E., Ruiz J. M., Procaccini G. (2017). Molecular mechanisms behind the physiological resistance to intense transient warming in an iconic marine plant. Front. Plant Sci. 8, 1142. doi: 10.3389/fpls.2017.01142
Marín-Guirao L., Entrambasaguas L., Ruiz J. M., Procaccini G. (2019). Heat-stress induced flowering can be a potential adaptive response to ocean warming for the iconic seagrass Posidonia oceanica. Mol. Ecol. 28, 2486–2501. doi: 10.1111/mec.15089
Marín-Guirao L., Ruiz J. M., Dattolo E., Garcia-Munoz R., Procaccini G. (2016). Physiological and molecular evidence of differential short-term heat tolerance in Mediterranean seagrasses. Sci. Rep. 6 (1), 28615. doi: 10.1038/srep28615
Martínez-Abraín A., Castejón-Silvo I., Roiloa S. (2022). Foreseeing the future of Posidonia oceanica meadows by accounting for the past evolution of the Mediterranean Sea. ICES J. Mar. Sci. 79, 2597–2599. doi: 10.1093/icesjms/fsac212
McMahon K., Van Dijk K. J., Ruiz-Montoya L., Kendrick G. A., Krauss S. L., Waycott M. (2014). The movement ecology of seagrasses. Proc. Biol. Sci.B. 281, 20140878. doi: 10.1098/rspb.2014.0878
Moreira-Saporiti A., Teichberg M., Garnier E., Cornelissen J. H. C., Alcoverro T., Björk M., et al. (2023). A trait-based framework for seagrass ecology: trends and prospects. Front. Plant Sci. 14, 1088643. doi: 10.3389/fpls.2023.1088643
Morley J. W., Batt R. D., Pinsky M. L. (2017). Marine assemblages respond rapidly to winter climate variability. Glob. Change Biol. 23, 2590–2601. doi: 10.1111/gcb.13578
Mutlu E., Olguner C., Gökoğlu M., Ozvarol Y. (2022). Seasonal growth dynamics of Posidonia oceanica in a pristine Mediterranean gulf. Ocean Sci. J. 57, 381–397. doi: 10.1007/s12601-022-00078-8
Nguyen H. M., Kim M., Ralph P. J., Marín-Guirao L., Pernice M., Procaccini G. (2020). Stress memory in seagrasses: first insight into the effects of thermal priming and the role of epigenetic modifications front. Plant Sci. 11, 494. doi: 10.3389/fpls.2020.00494
Nguyen H. M., Ralph P. J., Marín-Guirao L., Pernice M., Procaccini G. (2021). Seagrasses in an era of ocean warming: a review. Biol. Rev. 96, 2009–2030. doi: 10.1111/brv.12736
Olsen Y. S., Sánchez-Camacho M., Marbà N., Duarte C. M. (2012). Mediterranean Seagrass growth and demography responses to experimental warming. Estuaries Coast. 35, 1205–1213. doi: 10.1007/s12237-012-9521-z
Ontoria Y., Cuesta-Gracia A., Ruiz J. M., Romero J., Perez M. (2019). The negative effects of short-term extreme thermal events on the seagrass Posidonia oceanica are exacerbated by ammonium additions. PloS One 14, e0222798. doi: 10.1371/journal.pone.0222798
Orth R. J., Carruthers T. J. B., Dennison W. C., Duarte C. M., Fourqurean J. W., Heck K. L., et al. (2006). A global crisis for seagrass ecosystems. BioScience 56, 987–996. doi: 10.1641/0006-3568
Padfield D., O'Sullivan H., Pawar S. (2021). rTPC and nls. multstart: a new pipeline to fit thermal performance curves in r. Methods Ecol. Evol. 12, 1138–1143. doi: 10.1111/2041-210X.13585
Pazzaglia J., Badalamenti F., Bernardeau-Esteller J., Ruiz J. M., Giacalone V. M., Procaccini G., et al. (2022a). Thermo-priming increases heat-stress tolerance in seedlings of the Mediterranean seagrass p. oceanica. Mar. pollut. Bull. 174, 113164. doi: 10.1016/j.marpolbul.2021.113164
Pazzaglia J., Santillán-Sarmiento A., Helber S. B., Ruocco M., Terlizzi A., Marín-Guirao L., et al. (2020). Does warming enhance the effects of eutrophication in the seagrass Posidonia oceanica? Front. Mar. Sci. 7, 564805. doi: 10.3389/fmars.2020.564805
Pazzaglia J., Santillán-Sarmiento A., Ruocco M., Dattolo E., Ambrosino L., Marín-Guirao L., et al. (2022b). Local environment modulates whole-transcriptome expression in the seagrass Posidonia oceanica under warming and nutrients excess. Environ. pollut. 303, 119077. doi: 10.1016/j.envpol.2022.119077
Pereda-Briones L., Terrados J., Tomas F. (2019). Negative effects of warming on seagrass seedlings are not exacerbated by invasive algae. Mar. pollut. Bull. 141, 36–45. doi: 10.1016/j.marpolbul.2019.01.049
Pergent G., Gerakaris V., Sghaier Y. R., Zakhama-Sraier R., Fernández Torquemada Y., Pergent-Martini C. (2018) Posidonia oceanica. the IUCN red list of threatened species. Available at: https://www.iucnredlist.org/species/153534/4516034.
Pergent-Martini C., Pergent G., Monnier B., Boudouresque C. F., Mori C., Valette-Sansevin A. (2021). Contribution of Posidonia oceanica meadows in the context of climate change mitigation in the Mediterranean Sea. Mar. Environ. Res. 165, 105236. doi: 10.1016/j.marenvres.2020.105236
Personnic S., Boudouresque C. F., Astruch P., Ballesteros E., Blouet S., Bellan-Santini D., et al. (2014). An ecosystem-based approach to assess the status of a Mediterranean ecosystem, the Posidonia oceanica seagrass meadow. PloS One 9, e98994. doi: 10.1371/journal.pone.0098994
Procaccini G., Dattolo E., Ruocco M. (2023). Genetic diversity and connectivity in the Mediterranean seagrass Posidonia oceanica: state of art and future directions. Cah. Biol. Mar. 64, 105–114. doi: 10.21411/CBM.A.18AC84C1
Procaccini G., Olsen J. L., Reusch T. B. H. (2007). Contribution of genetics and genomics to seagrass biology and conservation. J. Exp. Mar. Biol. Ecol. 350, 234–259. doi: 10.1016/j.jembe.2007.05.035
Ramírez F., Coll M., Navarro J., Bustamante J., Green A. J. (2018). Spatial congruence between multiple stressors in the Mediterranean Sea may reduce its resilience to climate impacts. Sci. Rep. 8, 14871. doi: 10.1038/s41598-018-33237-w
Rasmusson L. M., Buapet P., George R., Gullström M., Gunnarsson P. C., Björk M. (2020). Effects of temperature and hypoxia on respiration, photorespiration, and photosynthesis of seagrass leaves from contrasting temperature regimes. ICES J. Mar. Sci. 77, 2056–2065. doi: 10.1093/icesjms/fsaa093
Rilov G., David N., Guy-Haim T., Golomb D., Aravfv R., Filin S., et al. (2021). Sea Level rise can severely reduce biodiversity and community net production on rocky shores. Sci. Total Environ. 791, 148377. doi: 10.1016/j.scitotenv.2021.148377
Rosso L., Lobry J. R., Flandrois J. P. (1993). An unexpected correlation between cardinal temperatures of microbial growth highlighted by a new model. J. Theor. Biol. 162, 447–463. doi: 10.1006/jtbi.1993.1099
Ruiz J. M., Marín-Guirao L., García-Muñoz L., Ramos-Segura A., Bernardeau-Estellera J., Perez M., et al. (2018). Experimental evidence of warming-induced flowering in the Mediterranean seagrass Posidonia oceanica. Mar.Pollut. Bull. 134, 49–54. doi: 10.1016/j.marpolbul.2017.10.037
Ruiz-Frau A., Gelcich S., Hendriks I. E., Duarte C. M., Marbà N. (2017). Current state of seagrass ecosystem services: research and policy integration. Ocean Coast. Manage. 149, 107–115. doi: 10.1016/j.ocecoaman.2017.10.004
Said N. E., McMahon K., Lavery P. S. (2021). Accounting for the influence of temperature and location when predicting seagrass (Halophila ovalis) photosynthetic performance. Estuar. Coast. Shelf Sci. 257, 107414. doi: 10.1016/j.ecss.2021.107414
Schulte P. M. (2015). The effects of temperature on aerobic metabolism: towards a mechanistic understanding of the responses of ectotherms to a changing environment. J. Exp. Biol. 218, 1856–1866. doi: 10.1242/jeb.118851
Stipcich P., Apostolaki E. T., Chartosia N., Efthymiadis P. T., Jimenez C. E., La Manna G., et al. (2022c). Assessment of posidonia oceanica traits along a temperature gradient in the Mediterranean Sea shows impacts of marine warming and heat waves. Front. Mar. Sci. 9, 895354. doi: 10.3389/fmars.2022.895354
Stipcich P., Marín-Guirao L., Pansini A., Pinna F., Procaccini G., Pusceddu A., et al. (2022a). Effects of current and future summer marine heat waves on Posidonia oceanica: plant origin matters? Front. Clim. 4. doi: 10.3389/fclim.2022.844831
Stipcich P., Pansini A., Beca-Carretero P., Stengel D. B., Ceccherelli G. (2022b). Field thermo acclimation increases the resilience of Posidonia oceanica seedlings to marine heat waves. Mar. pollut. Bull. 184, 114230. doi: 10.1016/j.marpolbul.2022.114230
Tait L. W., Schiel D. R. (2010). Primary productivity of intertidal macroalgal assemblages: comparison of laboratory and in situ photorespirometry. Mar.Ecol. Progr. Ser. 416, 115–125. doi: 10.3354/meps08781
Telesca L., Belluscio A., Criscoli A., Ardizzone G., Apostolaki E. T., Fraschetti S., et al. (2015). Seagrass meadows (Posidonia oceanica) distribution and trajectories of change. Sci. Rep. 5, 12505. doi: 10.1038/srep12505
Thomas M. K., Kremer C. T., Klausmeier C. A., Litchman E. (2012). A global pattern of thermal adaptation in marine phytoplankton. Science 338, 1085–1088. doi: 10.1126/science.1224836
Vassallo P., Paoli C., Rovere A., Montefalcone M., Morri C., Nike Bianchi C. (2013). The value of the seagrass Posidonia oceanica: a natural capital assessment. Mar. pollut. Bull. 75, 157–167. doi: 10.1016/j.marpolbul.2013.07.044
Wahid A., Gelani S., Ashraf M., Foolad M. (2007). Heat tolerance in plants: an overview. Environ. Exp. Bot. 61, 199–223. doi: 10.1016/j.envexpbot.2007.05.011
Keywords: dark respiration, extreme climate events, gross photosynthesis, net production, resilience
Citation: Rinaldi A, Martinez M, Badalamenti F, D’Anna G, Mirto S, Marín-Guirao L, Procaccini G and Montalto V (2023) The ontogeny-specific thermal sensitivity of the seagrass Posidonia oceanica. Front. Mar. Sci. 10:1183728. doi: 10.3389/fmars.2023.1183728
Received: 23 March 2023; Accepted: 27 June 2023;
Published: 19 July 2023.
Edited by:
Marco Fusi, Edinburgh Napier University, United KingdomReviewed by:
Marco Munari, University of Padua, ItalyCopyright © 2023 Rinaldi, Martinez, Badalamenti, D’Anna, Mirto, Marín-Guirao, Procaccini and Montalto. This is an open-access article distributed under the terms of the Creative Commons Attribution License (CC BY). The use, distribution or reproduction in other forums is permitted, provided the original author(s) and the copyright owner(s) are credited and that the original publication in this journal is cited, in accordance with accepted academic practice. No use, distribution or reproduction is permitted which does not comply with these terms.
*Correspondence: Valeria Montalto, dmFsZXJpYS5tb250YWx0b0BjbnIuaXQ=
Disclaimer: All claims expressed in this article are solely those of the authors and do not necessarily represent those of their affiliated organizations, or those of the publisher, the editors and the reviewers. Any product that may be evaluated in this article or claim that may be made by its manufacturer is not guaranteed or endorsed by the publisher.
Research integrity at Frontiers
Learn more about the work of our research integrity team to safeguard the quality of each article we publish.