- 1Division of Natural Sciences, Pepperdine University, Malibu, CA, United States
- 2Department of Environmental Studies, Hamilton College, Clinton, NY, United States
- 3Hawai‘i Institute of Marine Biology, University of Hawai‘i at Mānoa, Kaneohe, HI, United States
- 4Daniel K. Inouye Center for Microbial Oceanography: Research and Education, Department of Oceanography and Sea Grant College Program, University of Hawai‘i at Mānoa, Honolulu, HI, United States
Submarine groundwater discharge (SGD) in high volcanic islands can be an important source of freshwater and nutrients to coral reefs. High inorganic nutrient content is generally thought to augment primary production in coastal systems but when this is delivered via a freshwater vector as is the case with SGD in this study, the effects on productivity are unclear. In the current literature, there is limited evidence for a direct association between SGD and primary productivity of reefs. To elucidate the response of primary productivity to SGD, we conducted spatially and temporally explicit in situ benthic chamber experiments on a reef flat along a gradient of SGD. We found significant quadratic relationships between C-uptake and SGD for both phytoplankton and the most abundant macroalga, Gracilaria salicornia, with uptake maxima at SGD-derived salinities of ~21−22 (24.5−26.6 μmol NO3-L−1). These results suggest a physiological tradeoff between salinity tolerance and nutrient availability for reef primary producers. Spatially explicit modeling of reefs with SGD and without SGD indicate reef-scale G. salicornia and phytoplankton C-uptake decreased by 82% and 36% in the absence of SGD, respectively. Thus, nutrient-rich and low salinity SGD has significant effects on algal C-uptake in reef systems.
1 Introduction
Submarine groundwater discharge (SGD), is defined as any flow of water from the seabed or benthos to the coastal ocean, regardless of fluid composition or driving force (Burnett et al., 2006; Moore, 2010). The chemical composition of SGD is geographically unique based on land use, biogeochemical processes in the aquifer and subterranean estuary, precipitation rates, water residence times, and recirculation (Taniguchi et al., 2019). SGD is an important source of terrigenous freshwater and nutrients to coastal waters worldwide (Slomp and Van Cappellen, 2004; Burnett et al., 2006; Luijendijk et al., 2020; Santos et al., 2021). Cho and colleagues (2018) showed that the total SGD-derived dissolved inorganic nitrogen (DIN) and dissolved inorganic phosphorus (DIP) fluxes could be approximately 1.4- and 1.6-fold of the river fluxes to the Indo-Pacific and Atlantic Oceans respectively.
Offshore coral islands which experience tidally modulated SGD input, release nutrients via vertical pore water upwelling, tidal pumping, and temperature-driven convection, which can in turn, lead to sustained productivity within coral reefs (Santos et al., 2010). Tidally dominated SGD inputs can be chronic sources of nutrients to tropical reefs (Nelson et al., 2015; Jiang et al., 2021); this contrasts with fluvial nutrient inputs, which vary greatly with precipitation (Amato et al., 2016; Richardson et al., 2017a; Richardson et al., 2017b). Hence, in coastal areas with tidally dominated SGD inputs, SGD may comprise a significant component of reef nutrient inputs but have highly variable biogeochemistry on small temporal scales (i.e., hours) and spatial scales (i.e., meters) (Swarzenski et al., 2017; Luijendijk et al., 2020; Silbiger et al., 2020).
SGD can have a freshwater component and a recirculated seawater component although in high volcanic islands, the freshwater component is usually large due to high precipitation rates, high shoreline to area ratio, high relief, and immature permeable soil (Moosdorf et al., 2015); thus, SGD input to reefs usually decreases the salinity and increases the nutrient concentration of coastal waters (Burnett et al., 2006; Moore, 2010). The primary producers that inhabit these ephemerally estuarine environments must be able to withstand, grow, and maintain productivity while being exposed to rapid fluctuations in both salinity and nutrients (Santos et al., 2021; Bharathi et al., 2022). All primary producers have physiologically optimized ranges of tolerance for both inorganic nutrients and salinity (Kirst, 1990; Guan et al., 2015; Liu et al., 2016). Although nutrient concentrations have long been known to affect primary productivity on reefs (Nixon et al., 1986; Duarte, 1995; Brandl et al., 2019; Johnson et al., 2020), the effects of salinity on productivity are usually varied and species-specific (Sudhir and Murthy, 2004). The effects of increased nutrients on reef productivity depends on the structure of the benthic community (Yap et al., 1994; Marubini and Davies, 1996; Dizon and Yap, 2003; Fabricius, 2005). Specifically, gross primary productivity (GPP) will be higher in algae-dominated reef communities (Roth et al., 2021) because some fleshy macroalgae can make use of inorganic nutrients on a reef more efficiently and rapidly than zooxanthellae in coral-dominated reef communities (Littler et al., 1991; Hughes et al., 2010; Dailer et al., 2012; Johnson et al., 2020; Vaughan et al., 2021). Experimental factorial studies of salinity pulses and nutrient loading on primary producers show nutrient loading tends to have a long-term effect through complex community interactions (Duarte, 1995; Valiela et al., 1997), while salinity pulsing frequency and intensity has an immediate and direct influence on growth and distribution (Boustany et al., 2015).
Studies have shown that abundance of cyanobacteria, phytoplankton, and macroalgae usually increases with high-nutrient SGD input (Gobler and Boneillo, 2003; Troccoli-Ghinaglia et al., 2010; Waska and Kim, 2011; Peterson et al., 2012; Lee and Kim, 2015; Amato et al., 2016; Lecher and Mackey, 2018; La Valle et al., 2021; Dulai et al., 2023). Specifically, in Hawai‘i studies have found that SGD increases invasive macroalgal abundance, thus altering reef community composition (Amato et al., 2016; La Valle et al., 2019; Dulai et al., 2023). Johnson and Wiegner (2014) showed that coastal primary production and respiration respond differently to surface plumes of SGD over short spatial and temporal scales, with some locations showing net autotrophic conditions and some showing net heterotrophic conditions. In shallow macroalgal-dominated reefs, benthic macroalgae account for the majority of the overall ecosystem productivity (Valiela et al., 1997; Dailer et al., 2012). Hence, we hypothesize that the effect of SGD on the productivity of benthic macroalgae and phytoplankton will be net positive and play an important role in the ecology of reefs and coastal systems with SGD.
Many of the studies measuring production rates, especially in macroalgae, are done in laboratory and mesocosm settings. In situ flux estimates on reef communities are in short supply, mostly because they are difficult to perform, yet these types of studies are critical to improve our understanding of real-time productivity in these algae-dominated coral reefs. Measuring C-uptake rates of primary producers on a reef with SGD characterized by low salinity and high inorganic nutrients gives us species-specific and functional group-specific productivity rates and can shed light on the relationship between productivity and nutrients delivered via a freshwater medium. In this study we measured in situ C-uptake of the main primary producers on an algal-dominated reef with SGD influence using benthic chambers. The experimental design was spatially and temporally explicit in order to capture C-uptake rates across a gradient of SGD input. We then coupled this data with benthic cover data to map and estimate both benthic and water column C-uptake on a reef with SGD and a reef without SGD. This work can elucidate the magnitude of the effect of SGD on both benthic and water column algal productivity of reefs. The effects of high-nutrient SGD on reef primary productivity and reef community composition has important wastewater and land-based pollutant management implications in watersheds with SGD.
2 Materials and methods
2.1 Study site description and experimental design
The study site is Maunalua Bay along the southeast coast of O‘ahu, Hawai‘i. Our specific study area, the reef flat adjacent to Wailupe beach park (Figure 1), has one localized SGD (21.2756°N, 157.7624°W) close to the shoreline (Nelson et al., 2015). The reef flat is dominated by macroalgae. The most abundant algal species was the invasive Gracilaria salicornia, accounting for an average of 13% of the total benthic cover (areas without algal cover included) and approximately 31% of algal cover. The average SGD discharge rate is 20 m3 d−1 m−1 of coast at Wailupe and average nutrient concentrations at the seep were 68 μM NO3− and 2 μM PO43− resulting in groundwater derived nutrient fluxes of 8902 mol NO3− d−1 km−1 shoreline and 238 mol PO43− d−1 km−1 shoreline (Holleman, 2011). SGD is the dominant source of nutrients to the water column and throughout the majority of the year is the only source of terrestrial freshwater (McGowan, 2004).
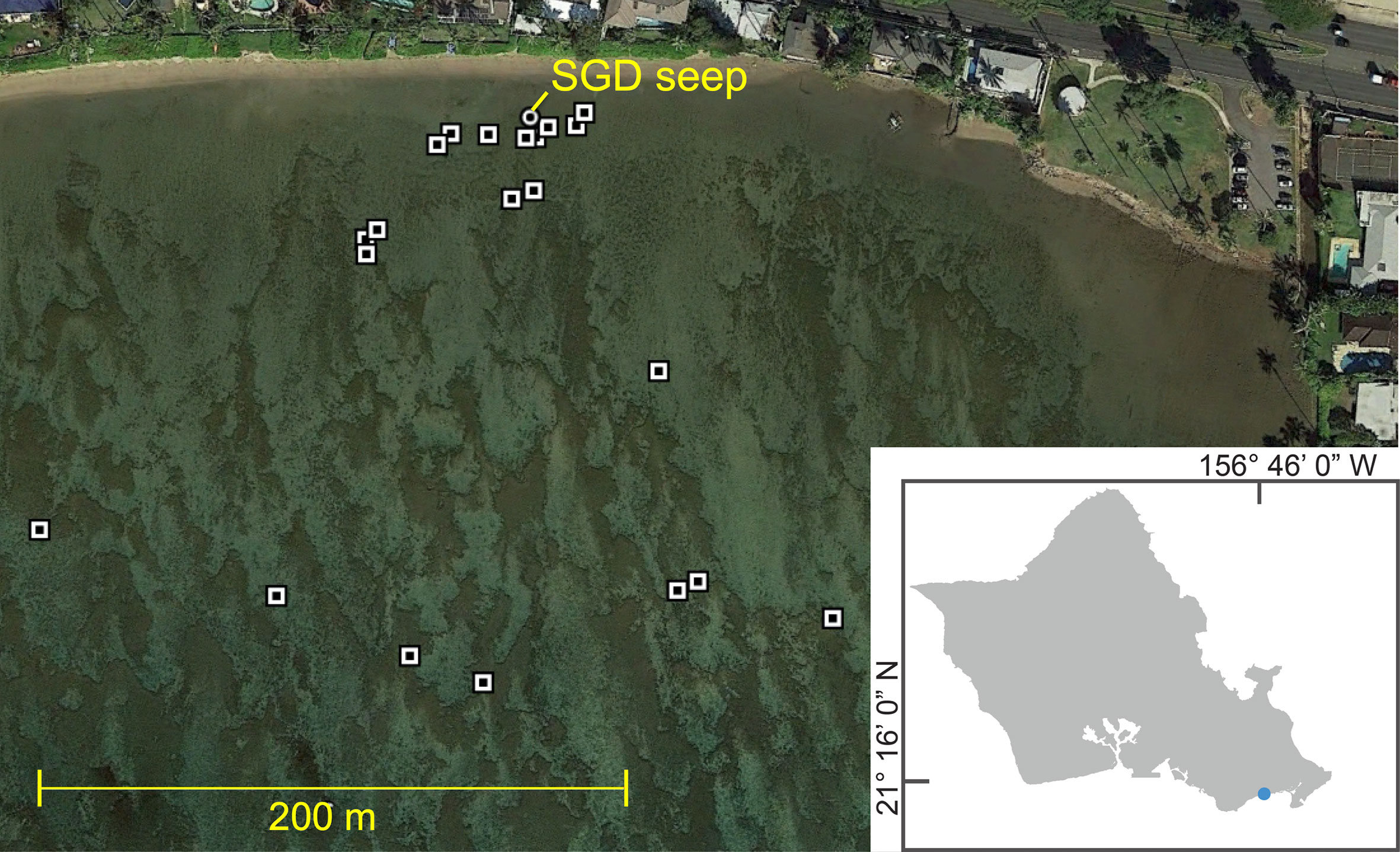
Figure 1 Map of experiment deployments. Exact locations at Wailupe marked by white and black squares; circle shows location of main SGD seep. (Inset) Map of O‘ahu, Hawai‘i, blue dot shows Wailupe’s location.
Experiments were done across space and at different tidal heights (from −0.16 m to 1.5 m MLLW) (Tide Predictions – NOAA Tides & Currents, 2015) in order to capture a range of groundwater input (i.e., different salinities) (Figure 1). All experimental sites were shallower than 2 m at all tide levels. The benthic chamber was sealed using multiple ring-shaped sandbags at the base with additional weighted chains placed on top of the sand bags. The chambers were placed on top of hard substrate covered in macroalgae. The benthic chamber was constructed out of clear 6 mil polypropylene bags (Lehua Greenhouse tarp, 93% PAR transparency) and had a sampling port. Following deployment, benthic chambers were enriched with about 0.3 g of 98 at.% NaH13CO3 to increase total DIC by about 10% (Mateo et al., 2001) and incubated for 1 hour. Each benthic chamber accommodated about 20 L of seawater and encompassed a 0.25 by 0.25 m2 area of reef at its base. Benthic chamber deployments were done across the reef flat in an area 0.11 km2 (440 m offshore by 250 m alongshore) during peak daylight hours (i.e., 9:00−14:00).
2.2 Isotope analyses and numerical procedures
Water samples (1 L) were collected before the beginning of the experiment, right after the NaH13CO3 enrichment, and after the experiment from within the benthic chambers and filtered through a 0.7mm glass fiber filter. Water samples were sent to the Biogeochemical Stable Isotope Facility at the University of Hawai‘i at Mānoa for δ13C analysis of dissolved inorganic carbon (DIC; Torres et al., 2005). Pre-enrichment and post experiment filters were analyzed for N, 15N, C, and 13C content using a Carlo Erba 2500 elemental analyzer coupled with a Finnigan Delta S mass spectrometer (internal error was ±0.05 ‰ with total analytical precision ±0.2 ‰). Ambient samples (i.e., natural abundance samples collected prior to 13C enrichment) of all macroalgal species present in the benthic chamber were picked within 0.3 m of the benthic chamber and all of the macroalgae was gathered from within the benthic chamber at the end of the experiment. Macroalgal samples were separated by species, cleaned of epiphytes and sediment and dried at 60°C over 3 days. The species-specific dried ambient (natural abundance) pre-experimental and post experiment enriched macroalgae was ground, weighed, and analyzed for N, 15N, C, and 13C content in accordance with methods described in Teichberg et al. (2008) and de los Santos et al. (2022).
Isotopic equations are listed in supplemental Table 1 and were derived from equations in Hayes (2004). Macroalgal uptake rates (P) were calculated using equation 1 below, derived from Hama et al. (1983) and used in Mateo et al. (2001). Similar calculations are also specified in Cornelisen and Thomas (2002).
Where ans is the atomic % 13C in the ambient sample’s tissue, ais is the atomic % 13C of the enriched sample tissue, taken after a 1-hour incubation, and aic is the atomic % 13C in the dissolved inorganic carbon in the incubation medium after enrichment. C (mg) is the carbon content of the sample, dw refers to dry weight (g) of the sample, and t (h) is time. Phytoplankton uptake rates were determined using the C-uptake rate equation in Hama et al. (1983).
POC refers to the particulate organic carbon (µg C L−1) in the incubated sample. No correction factor for isotopic discrimination against 13C has been applied to the calculations. C-uptake rates for G. salicornia, phytoplankton, and Acanthophora spicifera were modeled against salinity and fit with quadratic least squares regressions (Figure 2).
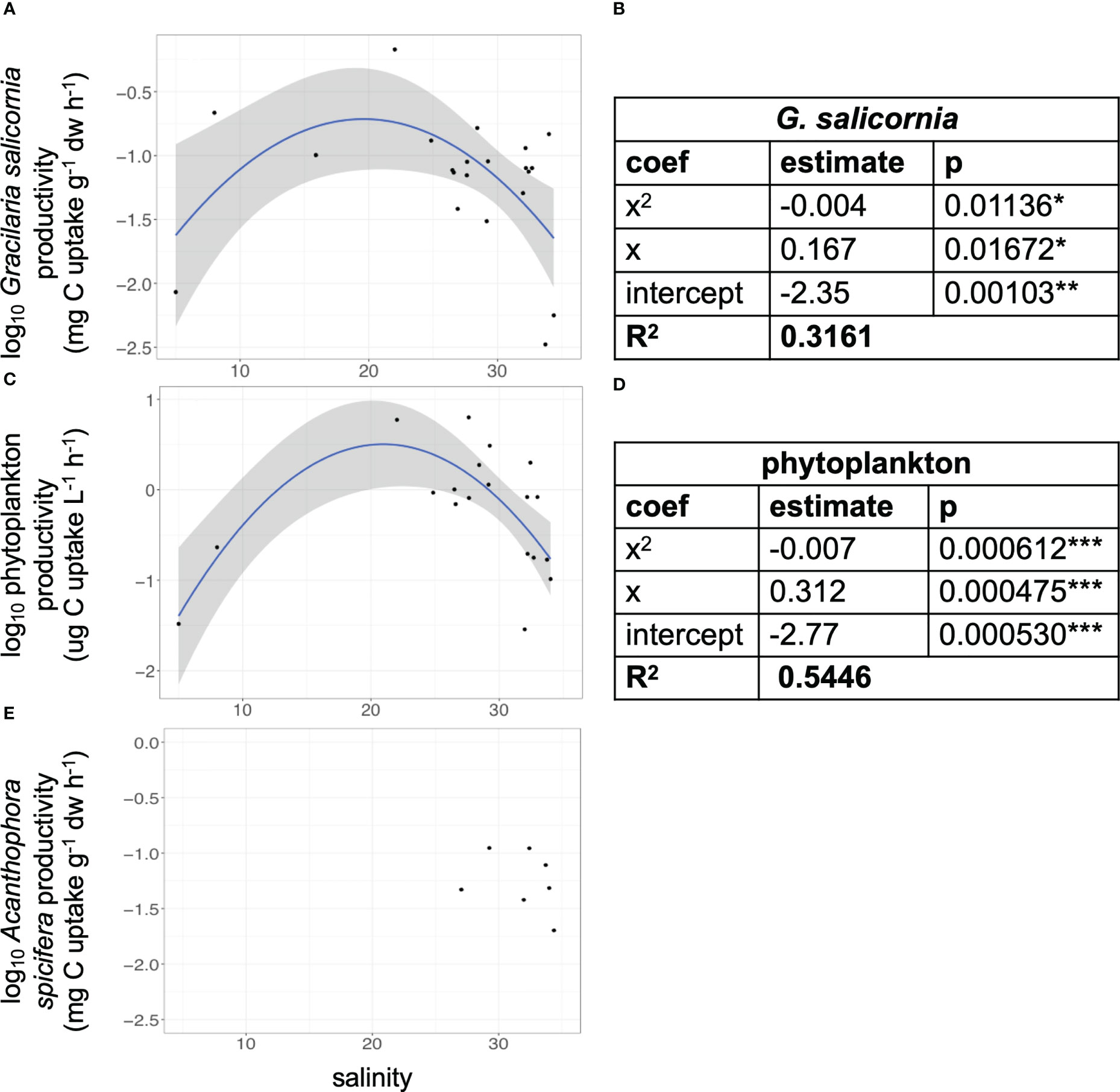
Figure 2 Peak carbon uptake by primary producers at intermediate SGD levels. C-uptake rates modeled against salinity on a reef flat with SGD for (A) G. salicornia, (C) phytoplankton, and (E) A. spicifera. Model statistics for quadratic regressions for (B) G. salicornia and (D) phytoplankton. Quadratic relationships are significant for G. salicornia and phytoplankton C-uptake and salinity (*=p<0.05, **=p<0.01, ***=p<0.001).
2.3 Water chemistry and physical parameter measurements
Salinity, temperature, photosynthetically active radiation (PAR), and current speed were measured using autonomous sensors located inside and outside the benthic chambers. Water samples were taken from within the benthic chamber for dissolved inorganic carbon (DIC) and pH analysis pre-experiment, right after the isotope enrichment, and after the 1-hour incubation. Dissolved inorganic nutrient samples were taken before the incubation and at the end of the experiment (~1 hr).
Salinity and temperature – Two autonomous salinity sensors (Odyssey Temperature and Conductivity loggers, 3 to 60 mS cm−1) and a temperature logger (Onset TidbiT v2 Water Temperature Data Logger) were deployed inside and outside the benthic chambers. The sampling frequency was one measurement per minute for both sensors. Salinity of water samples was measured with a conductivity probe (Orion Star portable meter) to ground truth salinity time series data.
Dissolved inorganic nutrients – Water samples for dissolved inorganic nutrient analysis were filtered through a 0.2 µm previously combusted glass fiber filter. The samples were brought to room temperature, mixed, and analyzed on a Seal Analytical Segmented Flow Injection AutoAnalyzer AA3HR for soluble reactive phosphate (PO43−) ammonium (), nitrate + nitrite (N + N; NO2− + NO3−), and silicate (SiO4) at the SOEST Laboratory for Analytical Biogeochemistry (S-LAB) at the University of Hawai‘i at Mānoa. A filter blank was collected and used to standardize our silicate values.
Dissolved inorganic carbon (DIC) – Water samples for DIC analysis (300 ml) were collected from within the benthic chamber using a 1 L syringe in borosilicate bottles before the experiment, right after the NaH13CO3 enrichment and at the end of the experiment. Samples were brought back to the lab and fixed with 200 μl HgCl2 per 250 ml seawater. Samples were analyzed for DIC using the UIC Coulometer and Marianda VINDTA 3D at the S-LAB at the University of Hawai‘i at Mānoa.
pH – pH on the total scale (pHtot) was measured using a Thermo Scientific Orion Star A329 portable meter with a pH electrode calibrated against a Tris buffer of known pH from the Dickson laboratory at Scripps Institution of Oceanography. Using DIC data, the pH values were corrected for in situ temperature recorded by the temperature loggers at each experimental location using CO2SYS (Lewis and Wallace, 1998). dissociation constants were taken from Uppstrom (1974) and Dickson (1990), while K1 and K2 dissociation constants for carbonic acid were taken from Mehrbach et al. (1973) and refit by Dickson and Millero (1987).
PAR and current – Two autonomous Odyssey Submersible Photosynthetic Active Radiation (PAR) Loggers were deployed about ~15 cm from the benthos; one within and one outside the benthic chamber. The scan rate was one per minute and the detected wavelength was cosine corrected photosynthetic irradiance (400−700 nm). A Nortek Vector 3D Acoustic Velocimeter was deployed outside the chamber at mid-height of the water column (~0.5–1 m depth), set to sample continuously at a rate of 8 Hz, with a nominal velocity range of 0.30 m s−1.
2.4 Distribution maps for carbon uptake
Percent cover of benthic macroalgae was measured on a 400 m by 250 m gridded map of Wailupe (n=73; Figure 3A) using 0.25 × 0.25 m2 quadrats and interpolated via linear interpolation within the boundaries of the data (interp function in package akima in R; Akima and Gebhardt, 2015). All of the biomass was collected from the quadrats, separated by species, rinsed with DI water, and dried for 3 days in a drying over at 60°C. Statistics and plots for linear regressions of species-specific dry weight by percent cover are given in Supplemental Figure 1.
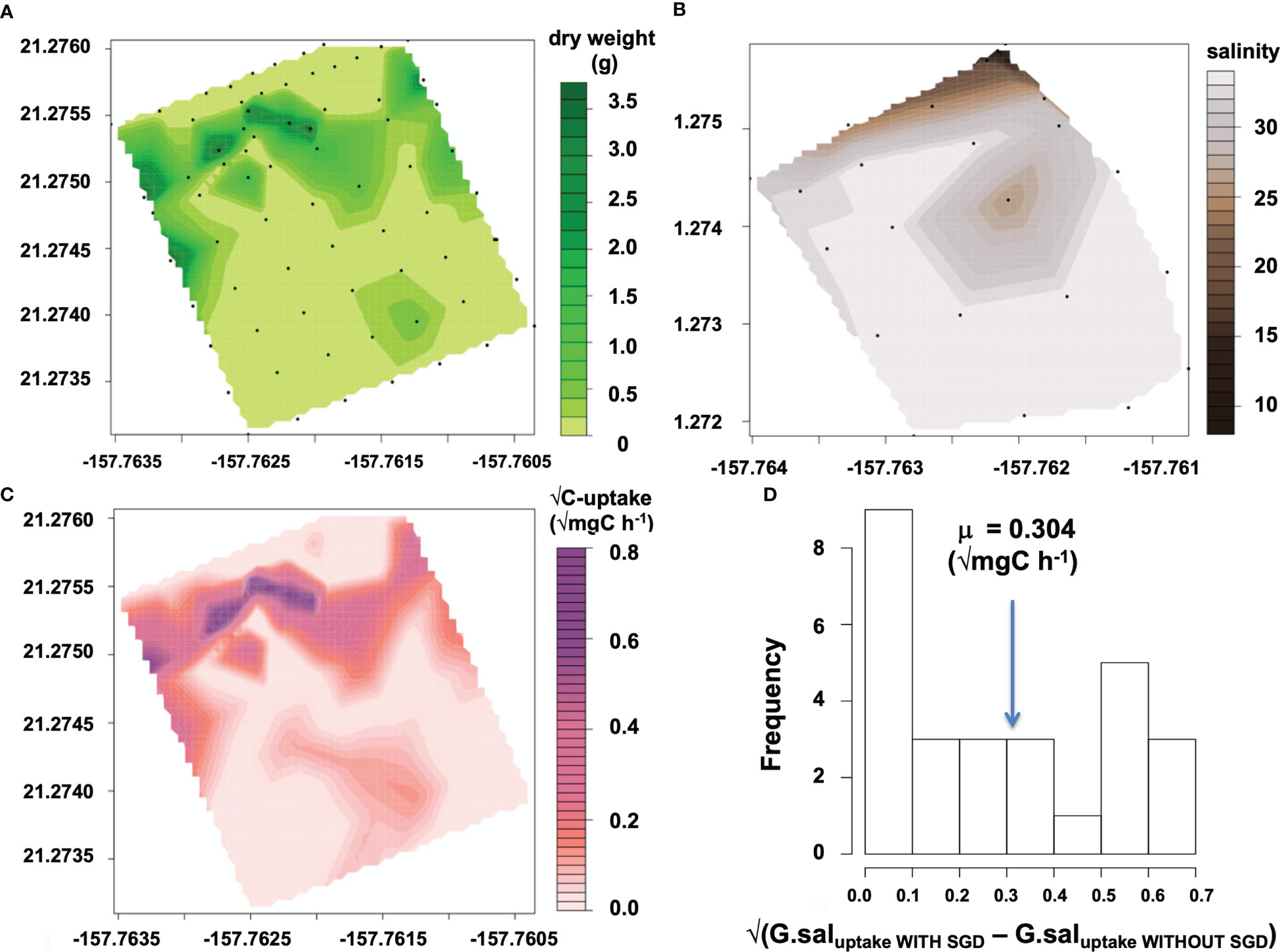
Figure 3 Spatial distribution and modeled C-uptake rates of G. salicornia with respect to SGD inputs on a reef flat. Contour plots shaded by (A) G. salicornia dry weight (g). Black points on maps refer to actual sampling locations. (B) Snapshot of salinity recorded via autonomous sensors at low tide (May 15, 2015). (C) Modeled C-uptake on a reef flat with SGD (mg C h−1). (D) Histogram of the square root transformed differences between G. salicornia C-uptake on a reef with SGD and a reef without SGD. Biomass is assumed to be the same for these reefs for this model. The mean of the square root transformed values is indicated by the blue arrow.
To create C-uptake rate maps of G. salicornia for a reef with SGD, the map of percent cover was converted to biomass using an empirically derived regression model (dw = 0.0392 • (% cover), p=<0.001, F=58.42, r2 = 0.7322; Supplemental Figure 1). The carbon uptake rate maps on a reef without SGD (Figures 4B, D) were calculated using a mean value for carbon uptake rates measured in experiments located at least 250m from the SGD seep and with no SGD influence (ie. >34 salinity) (C-uptake ratenoSGD = 0.0213 mg C g dw−1 h−1 for G. salicornia; 0.0423 mg C g dw−1 h−1 for A. spicifera) multiplied with measured dry weight of either G. salicornia or A. spicifera (dwA.spi(i), g) (Figures 4A, C). The regression model of carbon uptake rates vs. salinity (Figure 2) was multiplied with biomass (Figure 3A) to calculate carbon uptake rates of G. salicornia across the reef with SGD influence (Figure 3C) interpolating a snapshot of salinity values taken by the salinity sensor at a low tide of −0.12 m MLLW (Figure 3B) for each percent cover point. C-uptake at ith benthic survey location (C-uptakeSGD(i), mg C h−1) was calculated by multiplying measured dry weight (dwG.sal(i), g) of G. salicornia by regressed values of C-uptake rate (C-uptake rateG.sal(i), mg C g dw−1 h−1) versus salinity. A. spicifera was only found in benthic experiments located away from the groundwater seep and therefore carbon uptake rates were not calculated for areas with high SGD input.
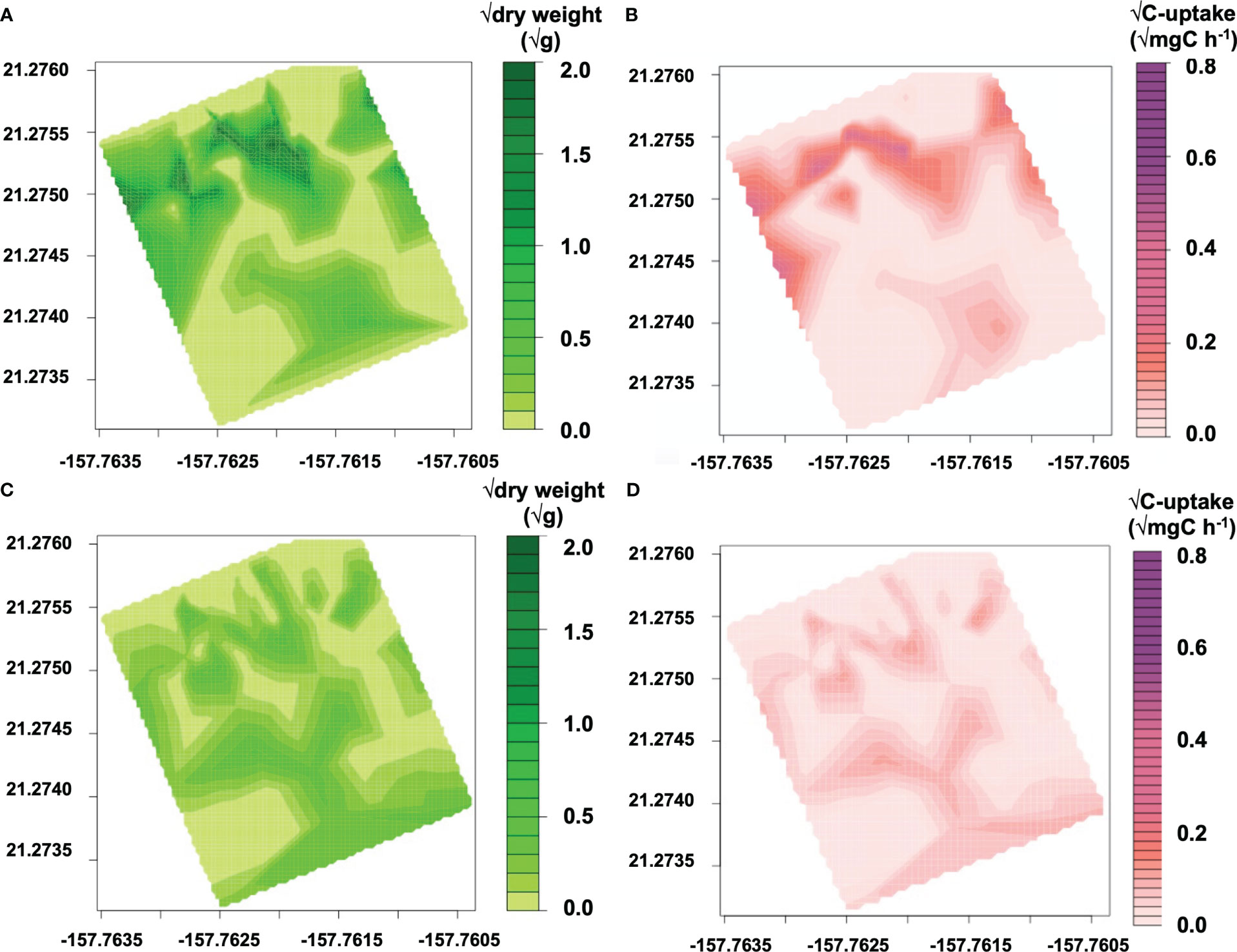
Figure 4 Spatial distributions and modeled C-uptake rates for macroalgal species on a reef without SGD. Contour plots of biomass as dry weight (√g) for (A) G. salicornia and (C) A. spicifera. Contour plots of C-uptake on a reef flat without SGD (mg C h−1) for (B) G. salicornia and (D) A. spicifera.
2.5 Relating benthic carbon uptake and water column carbon uptake
G. salicornia is responsible for the majority of the carbon uptake of the benthic community as it is the most abundant macroalga on the reef. The C-uptake values for G. salicornia on a reef with SGD and on a reef without SGD were given as (mg C h−1 m−2) for each benthic location by dividing the respective C-uptake values by the area of the quadrats used for the benthic surveys (0.0625 m2); the values were then square root transformed and C-uptake values for a reef without SGD were subtracted from the C-uptake values for a reef with SGD (Figure 3D; Supplemental Figure 2). The productivity values were square-root transformed simply for visual purposes, to show the gradient of productivity monochromatically in the maps. Water column productivity on a reef with or without SGD (µg C h−1) was calculated using the regression values for C-uptake rates (µg C L−1 h−1) versus salinity multiplied by water column depth (depth(i), m) at each benthic survey location (Table 1). For this model, we assume that biomass of G. salicornia is the same on this reef and a reef without SGD. A mean C-uptake rate (C-uptake ratenoSGD(i) = 0.692 mg C h−1 L−1) was calculated from experimental data collected about 250m from the SGD seep when there was no SGD influence (i.e., >34 salinity; Table 1) and used to create C-uptake maps on a reef without SGD.
3 Results
3.1 Relationships between productivity and SGD
There were strong linear relationships between salinity and inorganic nutrients (i.e., nitrate and nitrite, total dissolved phosphorus, and silicate) of water samples from varying distances from the localized SGD seeps (Table 2). This shows close to conservative mixing relationships, where the SGD comes in with low salinity (~2) and high nutrient concentrations, and as the SGD mixes with coastal water, the SGD gets diluted. These linear relationships were also found by Richardson and colleagues (2017b). Because salinity, nitrate and nitrite, total dissolved phosphorus, and silicate covary on this reef and we know SGD is the main source of freshwater and nutrients at this site, salinity was used as a proxy for nutrient concentrations and a metric for SGD contribution. Histograms of values for salinity, temperature, DIN, DIP, silicate, DIC, pH, and current speed can be found in Supplemental Figure 2.
We found significant quadratic relationships between C-uptake rates of G. salicornia and phytoplankton with salinity (Table 1; Figure 2). Linear regressions were not found to be significant. A. spicifera only occurred in 7 out of the 22 experimental sites and only in areas that experienced salinities above 27 (Figure 2E), therefore there were insufficient samples to determine a significant model between C-uptake rate and SGD. Quadratic relationships for G. salicornia and phytoplankton peak at approximately 21−22 salinity (24.5−26.6 μmol NO3− L−1 and 0.54–0.58 μmol PO3+4 L−1).
3.2 Effects of SGD on benthic and water column productivity
Maps of G. salicornia and A. spicifera’s biomass and C-uptake on a reef without SGD are shown in Figure 4. Even though uptake rates of each species in areas with no SGD were comparable (mean C-uptake rateG.salicornia = 0.014 ± 0.082 mg C g dw−1 h−1; mean C-uptake rateA. spicifera = 0.0423 ± 0.029 mg C g dw−1 h−1), G. salicornia’s biomass is much greater than that of A. spicifera; therefore, G. salicornia is responsible for the majority of the benthic macroalgal uptake at this site. G. salicornia has also increased in abundance over the last decade from 4.6% in 2003–2004 (McGowan, 2004) to 13% cover according to our benthic surveys done between 2014−2017. G. salicornia is currently the most abundant algal species at this site.
We calculated C-uptake of G. salicornia across the Wailupe reef flat with SGD input (Figure 3). The uptake map (Figure 3C) shows hotspots of uptake where there is high biomass and intermediate salinity. The difference between C-uptake on a reef with SGD and a reef without SGD was calculated for G. salicornia (Figure 3D; Supplemental Figure 3). At low tide, G. salicornia is responsible for a mean additional 0.097 mg C h−1 uptake. C-uptake contribution from the water column and benthos on a reef with SGD are 0.697 mg C m−2 h−1 and 2.72 mg C m−2 h−1, respectively. On a reef with no SGD C-uptake decreases by 32% for the water column and 82% for the benthos (Table 1).
4 Discussion
Our study shows that SGD increases both water column and benthic C-uptake in tropical reef systems. Peak C-uptake of both phytoplankton and G. salicornia occurred in environments with low SGD-derived salinities of ~21.5, and high nutrient concentrations (25.5 μmol NO3− L−1 and 0.54 μmol PO43+ L−1), suggesting a physiological tradeoff between salinity tolerance and nutrient availability for reef primary producers. The water column and benthic contributions to C-uptake on a reef with SGD are approximately 36% and 82% higher than a reef without SGD, respectively. This suggests that SGD increases productivity especially in macroalgae-dominated reefs.
4.1 Macroagal productivity on reefs with SGD
Several studies on macroalgae species in the Gracilaria genus have shown optimal growth rates between 20−30 salinity (Hoyle, 1975; Glenn et al., 1999; Choi et al., 2006). A study by Duarte and colleagues (2010) on the native Hawaiian species Gracilaria coronopofolia showed quadratic relationships between growth and SGD, with maxima at (27 salinity, 7.51 mmol nitrate, 0.15 mmol phosphate). G. salicornia, the main primary producer on this reef, is one of the main invasive species in Hawai‘i. Our work shows that G. salicornia can have high C-uptake rates in areas with SGD (high nutrients and low salinity), which implies that some macroalgae, an invasive species in this case, may outcompete others and thrive in areas with groundwater input.
Wailupe’s benthic community is composed of a variety of macroalgae, zooanthids, and sparse coral. Even considering these major functional groups, whose cover is less than 5% each, G. salicornia is likely responsible for the largest portion of C-uptake on this reef. Our G. salicornia uptake values (2.72 mg C m−2 h−1 on a reef with SGD) account for a small fraction of gross reef productivity, which have been reported to range from 0.33−30 g C m−2 h−1 (Sorokin, 1995; Gattuso et al., 1996; Andréfouët and Payri, 2001). The lack of biodiversity of the benthic community at Wailupe could explain the low productivity at this site. The high abundance of invasive G. salicornia could have created a less productive reef which lacks the variety in functional groups needed to boost this system’s C-uptake (Steneck and Dethier, 1994; Nyström, 2006).
Both the SGD research community and management groups share an interest in the fate of the dissolved inorganic nutrients brought to coastal systems via SGD. Gracilaria species have been well studied for aquaculture purposes and are widely known to have high uptake rates for NH4+, NO3−, and PO33− (Glenn et al., 1999; Yang et al., 2006; Huo et al., 2012). They can remove 13−85% DIN and DIP within 23−45 days (Yang et al., 2006; Huo et al., 2012) in a closed system. Wailupe reef flat has a residence time of approximately 1 day (Wolanski et al., 2009), which suggests that large amounts of DIN and DIP are being exported to the reef crest and other areas offshore.
4.2 Phytoplankton productivity on a reef with SGD
Phytoplankton uptake rates were boosted 32% in reefs with SGD, emphasizing that the effects of SGD on productivity affect the entire reef system. Sugimoto and colleagues (2017) found C-uptake rates of phytoplankton to range from 2−50 µg C l−1 h−1 in a coastal embayment with SGD. Our values fall on the low end of this range, with 1.39 µg C l−1 h−1 on a reef with SGD and decreased by an order of magnitude to 0.136 µg C l−1 h−1 on a reef without SGD. These rates are comparable to those from Alldredge et al. (2016) who used radioisotope incorporation methods on a similar reef flat with SGD in Mo‘orea, French Polynesia to obtain average uptake rates of 0.423 ± 0.276 µg C l−1 h−1. C-uptake values of phytoplankton from Paiko lagoon in Maunalua bay (21.2811°N, −157.7296°W) range from 7−15 mg C m−2 d−1 (K. Peyton, pers. comm.). Our mean C-uptake values are comparable at 10.8 mg C m−2 d−1 on a reef with no SGD and 17.7 mg C m−2 d−1 on a reef with SGD.
4.3 Biogeochemical and temporal effects of SGD
Several studies have shown that high islands with semidiurnal tides, such as the main Hawaiian islands, have high fluxes of SGD with high nutrient levels (Dimova et al., 2012; Moosdorf et al., 2015). On reefs with mostly freshwater SGD that experience semi-diurnal tidal patterns, intermediate salinities can occur up to four times a day, creating a periodicity that could produce consistently elevated productivity on reefs with SGD. Consistent with previous surveys of spatial distribution of SGD at this site (Nelson et al., 2015; Richardson et al., 2017a; Richardson et al., 2017b; Lubarsky et al., 2018), we showed that groundwater can have an effect on productivity up to 200 meters offshore (Figure 1; Supplemental Figure 4); it is important to understand SGD’s full spatial extent in order to create more accurate productivity maps and estimates. Our findings are relevant to any coastal areas with tidally modulated SGD discharging onto an area with high abundance of primary producers, whether in high volcanic islands or karst environments, where the SGD characteristics (i.e., low salinity, low temperature compared to surrounding coastal water, and high in nutrient concentrations) are similar. The effects of SGD with high nutrient concentrations on productivity are likely magnified in oligotrophic waters. These coastal systems with SGD can be hotspots for possible phase shifts as well as restoration sites. These areas should be monitored for changes in community structure and can be targeted by groups engaged in conservation efforts such as local Hawaiian community groups who are currently outplanting limu (algae) maunaea (Gracilaria coronopofolia) as well as other native and endemic species.
In this study, we have shown that SGD is an important source of freshwater and nutrients to reef systems. In this macroalgal-dominated reef, the productivity of both the water column and the main macroalgal species are increased at intermediate SGD-derived salinities. Further research on the benthic communities associated with SGD and its biochemistry is warranted. Understanding the effects of SGD on coastal communities will help direct and prioritize conservation and management efforts. Close attention should be paid to the photosynthetic communities present in coastal areas with SGD as these areas may be hotspots for phase shifts and restoration.
Data availability statement
The datasets presented in this study can be found in online repositories. The names of the repository/repositories and accession number(s) can be found below: https://www.bco-dmo.org/dataset/860764.
Author contributions
FFL, FIT, and CEN were heavily involved in coming up with the research question and designing the study approach. FFL performed all of the research (including all field and lab work). CEN and FIT provided statistical analysis advice, while FFL conducted statistical analyses. FFL wrote the manuscript; CEN and FIT edited the manuscript from its beginning stages through to its submission. JMJ edited the manuscript, conducted literature searches, and helped with the submission process. All authors contributed to the article and approved the submitted version.
Funding
This project was funded in part by NSF DEB 2312723, NSF OCE 1923877, and by a grant/cooperative agreement from the National Oceanic and Atmospheric Administration, Projects A/AS-1, R/SB-14PD (CEN), R/SB-13 (FIMT), R/SB-12 (MJD) and R/SB-11 (HD) sponsored by the University of Hawai‘i Sea Grant College Program, School of Oceanic and Earth Science and Technology, under Institutional Grant No. NA22OAR4170108 and NA14OAR4170071 from NOAA Office of Sea Grant, Department of Commerce.
Acknowledgments
We would like to thank our collaborators M. Donahue, K. Lubarsky, H. Dulai, and C. Richardson for their advice and support throughout the study. Field and lab help was provided by several volunteers; in particular, we would like to thank L. Himes, S. Zummo, J. Boord, M. Henn, G. Perez-Andujar, E. Nalley, J. Zill, A. Dudoit, and M. Brigham.
Conflict of interest
The authors declare that the research was conducted in the absence of any commercial or financial relationships that could be construed as a potential conflict of interest.
Publisher’s note
All claims expressed in this article are solely those of the authors and do not necessarily represent those of their affiliated organizations, or those of the publisher, the editors and the reviewers. Any product that may be evaluated in this article, or claim that may be made by its manufacturer, is not guaranteed or endorsed by the publisher.
Author disclaimer
The views expressed herein are those of the author(s) and do not necessarily reflect the views of NOAA or any of its subagencies.
Supplementary material
The Supplementary Material for this article can be found online at: https://www.frontiersin.org/articles/10.3389/fmars.2023.1178550/full#supplementary-material
Supplementary Table 1 | Important equations and corresponding descriptors.
Supplementary Figure 1 | Dry weight vs. % cover regressions (A) G. salicornia and (B) A. spicifera. Regression statistics for G. salicornia (dry weight = 0.0392 • % cover, p< 0.001, F = 58.4, r2 = 0.732) and for A. spicifera (dry weight = 0.00665 • % cover, p< 0.001, F = 32.9, r2 = 0.571).
Supplementary Figure 2 | Histograms of salinity, temperature, nitrate and nitrite (N + N) concentrations, phosphate (PO43−), ammonium (PO43−), pH, dissolved inorganic carbon (DIC), photosynthetically active radiation (PAR), and mean flow velocity measured for all 22 benthic chamber experiments.
Supplementary Figure 3 | G. salicornia C-uptake with and without SGD. (A) Histograms of square root transformed C-uptake by G. salicornia on a reef without SGD (light gray bars) and with SGD (light green bars, overlap between the two histograms is indicated by the dark gray). (B) Histogram of the square root transformed differences between G. salicornia C-uptake on reef with SGD and without SGD. The mean of the differences is 0.0923 mg C h−1.
Supplementary Figure 4 | C-uptake rates for primary producers partitioned by reef zone and tidal cycle. (A) Conceptual maps of biogeochemical zones (modified from Nelson et al., 2015). Legend shows the colors corresponding to biogeochemical zones. C-uptake rates across tide and zone for (B) G. salicornia, (C) A. spicifera and, (D) phytoplankton.
References
Akima H., Gebhardt A. (2015) Akima: interpolation of irregularly and regularly spaced data. r package version 0.5–12. Available at: https://CRAN.R-project.org/package=akima.
Alldredge A., Carlson C., Moorea Coral Reef LTER (2016). MCR LTER: coral reef: water column: nearshore water profiles, CTD, primary production, and chemistry ongoing since 2005. (Moorea, French Polynesia: UC Santa Barbara).
Amato D. W., Bishop J. M., Glenn C. R., Dulai H., Smith C. M. (2016). Impact of submarine groundwater discharge on marine water quality and reef biota of Maui. PloS One 11, e0165825. doi: 10.1371/journal.pone.0165825
Andréfouët S., Payri C. (2001). Scaling-up carbon and carbonate metabolism of coral reefs using in-situ data and remote sensing. Coral. Reefs 19, 259–269. doi: 10.1007/s003380000117
Bharathi M. D., Venkataramana V., Sarma V. V. S. S. (2022). Phytoplankton community structure is governed by salinity gradient and nutrient composition in the tropical estuarine system. Cont. Shelf Res. 234, 104643. doi: 10.1016/j.csr.2021.104643
Boustany R. G., Michot T. C., Moss R. F. (2015). Effect of nutrients and salinity pulses on biomass and growth of vallisneria americana in lower St johns river, FL, USA. R. Soc Open Sci. 2, 140053. doi: 10.1098/rsos.140053
Brandl S. J., Rasher D. B., Côté I. M., Casey J. M., Darling E. S., Lefcheck J. S., et al. (2019). Coral reef ecosystem functioning: eight core processes and the role of biodiversity. Front. Ecol. Environ. 17, 445–454. doi: 10.1002/fee.2088
Burnett W. C., Dulaiova H., Stringer C. (2006). “Submarine groundwater discharge: its measurement and influence on the coastal zone,” in J. Coastal Res. Proceedings of the 8th International Coastal Symposium (ICS 2004), Vol. I (Winter 2006) Special Issue 39. 35–38.
Cho H.-M., Kim G., Kwon E. Y., Moosdorf N., Garcia-Orellana J., Santos I. R. (2018). Radium tracing nutrient inputs through submarine groundwater discharge in the global ocean. Sci. Rep. 8, 2439. doi: 10.1038/s41598-018-20806-2
Choi H., Kim Y., Kim J., Lee S., Park E., Ryu J., et al. (2006). Effects of temperature and salinity on the growth of Gracilaria verrucosa and g. chorda, with the potential for mariculture in Korea. J. Appl. Phycol. 18, 269–277. doi: 10.1007/s10811-006-9033-y
Cornelisen C. D., Thomas F. I. M. (2002). Ammonium uptake by seagrass epiphytes: isolation of the effects of water velocity using an isotope label. Limnol. Oceanogr. 47, 1223–1229. doi: 10.4319/lo.2002.47.4.1223
Dailer M. L., Smith J. E., Smith C. M. (2012). Responses of bloom forming and non-bloom forming macroalgae to nutrient enrichment in Hawai‘i, USA. Harmful Algae 17, 111–125. doi: 10.1016/j.hal.2012.03.008
de los Santos C. B., Egea L. G., Martins M., Santos R., Masqué P., Peralta G., et al. (2022). Sedimentary organic carbon and nitrogen sequestration across a vertical gradient on a temperate wetland seascape including salt marshes, seagrass meadows and rhizophytic macroalgae beds. Ecosystems. 1–17 . doi: 10.1007/s10021-022-00801-5
Dickson A. G. (1990). Standard potential of the reaction: AgCl(s) + 12H2(g) = ag(s) + HCl(aq), and and the standard acidity constant of the ion HSO4– in synthetic sea water from 273.15 to 318.15 K. J. Chem. Thermodyn. 22, 113–127. doi: 10.1016/0021-9614(90)90074-Z
Dickson A. G., Millero F. J. (1987). A comparison of the equilibrium constants for the dissociation of carbonic acid in seawater media. Deep-Sea Res. 34, 1733–1743. doi: 10.1016/0198-0149(87)90021-5
Dimova N. T., Swarzenski P. W., Dulaiova H., Glenn C. R. (2012). Utilizing multichannel electrical resistivity methods to examine the dynamics of the fresh water–seawater interface in two Hawaiian groundwater systems. J. Geophys. Res. Oceans 117. doi: 10.1029/2011JC007509
Dizon R. M., Yap H. T. (2003). Metabolic changes and compositional shifts in nutrient-enriched tropical reef sediment communities. Sci. Mar. 67, 117–127. doi: 10.3989/scimar.2003.67n2117
Duarte C. M. (1995). Submerged aquatic vegetation in relation to different nutrient regimes. Ophelia 41, 87–112. doi: 10.1080/00785236.1995.10422039
Duarte T. K., Pongkijvorasin S., Roumasset J., Amato D., Burnett K. (2010). Optimal management of a Hawaiian coastal aquifer with nearshore marine ecological interactions. Water Resour. Res. 46. doi: 10.1029/2010WR009094
Dulai H., Smith C. M., Amato D. W., Gibson V., Bremer L. L. (2023). Risk to native marine macroalgae from land-use and climate change-related modifications to groundwater discharge in Hawai‘i. Limnol. Oceanogr. Lett. 8, 141–153. doi: 10.1002/lol2.10232
Fabricius K. E. (2005). Effects of terrestrial runoff on the ecology of corals and coral reefs: review and synthesis. Mar. pollut. Bull. 50, 125–146. doi: 10.1016/j.marpolbul.2004.11.028
Gattuso J. P., Pichon M. R., Delesalle B., Canon C., CopinMontegut G., Pichon M., et al. (1996). Carbon fluxes in coral reefs. i. Lagrangian measurements of community metabolism and resulting air–sea CO2 disequilibrium. Mar. Ecol. Prog. Ser. 145, 109–121. doi: 10.3354/meps145109
Glenn E. P., Moore D., Akutagawa M., Himler A., Walsh T., Nelson S. G. (1999). Correlation between gracilaria parvispora (Rhodophyta) biomass production and water quality factors on a tropical reef in Hawaii. Aquaculture 178, 323–331. doi: 10.1016/S0044-8486(99)00139-8
Gobler C. J., Boneillo G. E. (2003). Impacts of anthropogenically influenced groundwater seepage on water chemistry and phytoplankton dynamics within a coastal marine system. Mar. Ecol. Prog. Ser. 255, 101–114. doi: 10.3354/meps255101
Guan Y., Hohn S., Merico A. (2015). Suitable environmental ranges for potential coral reef habitats in the tropical ocean. PloS One 10, e0128831. doi: 10.1371/journal.pone.0128831
Hama T., Miyazaki T., Ogawa Y., Iwakuma T., Takahashi M., Otsuki A., et al. (1983). Measurement of photosynthetic production of a marine phytoplankton population using a stable 13C isotope. Mar. Biol. 73, 31–36. doi: 10.1007/BF00396282
Hayes J. (2004). An Introduction to Isotopic Calculations. Woods Hole Oceanographic Institution. doi: 10.1575/1912/27058
Holleman K. (2011). Comparison of submarine groundwater discharge, coastal residence times, and rates of primary productivity, manualua bay, Oahu and honokohau harbor, big island, Hawaii, USA. [master’s thesis] (Honolulu, HI: University of Hawai‘i at Mānoa).
Hoyle M. D. (1975). The literature pertinent to the red algal genus gracilaria in Hawaii (University of Hawaii: Marine Agronomy Program).
Hughes T. P., Graham N. A. J., Jackson J. B. C., Mumby P. J., Steneck R. S. (2010). Rising to the challenge of sustaining coral reef resilience. Trends Ecol. Evol. 25, 633–642. doi: 10.1016/j.tree.2010.07.011
Huo Y., Wu H., Chai Z., Xu S., Han F., Dong L., et al. (2012). Bioremediation efficiency of gracilaria verrucosa for an integrated multi-trophic aquaculture system with pseudosciaena crocea in xiangshan harbor, China. Aquaculture 326–329, 99–105. doi: 10.1016/j.aquaculture.2011.11.002
Jiang Z.-P., Lv J., Li Q., Dai M., Kao S.-J., Zheng N., et al. (2021). Tidal-driven submarine groundwater discharge and its influences on the carbonate system of a coastal coral reef in the northern south China Sea. J. Geophys. Res. Oceans 126, e2021JC017203. doi: 10.1029/2021JC017203
Johnson M. D., Fox M. D., Kelly E. L. A., Zgliczynski B. J., Sandin S. A., Smith J. E. (2020). Ecophysiology of coral reef primary producers across an upwelling gradient in the tropical central pacific. PloS One 15, e0228448. doi: 10.1371/journal.pone.0228448
Johnson E. E., Wiegner T. N. (2014). Surface water metabolism potential in groundwater-fed coastal waters of Hawaii island, USA. Estuaries Coast. 37, 712–723. doi: 10.1007/s12237-013-9708-y
Kirst G. O. (1990). Salinity tolerance of eukaryotic marine algae. Annu. Rev. Plant Biol. 41, 21–53. doi: 10.1146/annurev.pp.41.060190.000321
La Valle F. F., Kantar M. B., Nelson C. E. (2021). Coral reef benthic community structure is associated with the spatiotemporal dynamics of submarine groundwater discharge chemistry. Limnol. Oceanogr. 66, 188–200. doi: 10.1002/lno.11596
La Valle F. F., Thomas F. I., Nelson C. E. (2019). Macroalgal biomass, growth rates, and diversity are influenced by submarine groundwater discharge and local hydrodynamics in tropical reefs. Mar. Ecol. Prog. Ser. 621, 51–67. doi: 10.3354/meps12992
Lecher A. L., Mackey K. R. M. (2018). Synthesizing the effects of submarine groundwater discharge on marine biota. Hydrology 5, 60. doi: 10.3390/hydrology5040060
Lee J., Kim G. (2015). Dependence of coastal water pH increases on submarine groundwater discharge off a volcanic island. Estuar. Coast. Shelf Sci. 163, 15–21. doi: 10.1016/j.ecss.2015.05.037
Lewis E. R., Wallace D. W. R. (1998). “Program developed for CO2 system calculations,” (United States: Environmental System Science Data Infrastructure for a Virtual Ecosystem (ESS-DIVE).
Littler M. M., Littler D. S., Titlyanov E. A. (1991). Producers of organic-matter on tropical reefs and their relative dominance. Mar. Biol. 6, 3–14.
Liu X., Xiao W., Landry M. R., Chiang K.-P., Wang L., Huang B. (2016). Responses of phytoplankton communities to environmental variability in the East China Sea. Ecosystems 19, 832–849. doi: 10.1007/s10021-016-9970-5
Lubarsky K. A., Silbiger N. J., Donahue M. J. (2018). Effects of submarine groundwater discharge on coral accretion and bioerosion on two shallow reef flats. Limnol. Oceanogr. 63, 1660–1676. doi: 10.1002/lno.10799
Luijendijk E., Gleeson T., Moosdorf N. (2020). Fresh groundwater discharge insignificant for the world’s oceans but important for coastal ecosystems. Nat. Commun. 11, 1260. doi: 10.1038/s41467-020-15064-8
Marubini F., Davies P. S. (1996). Nitrate increases zooxanthellae population density and reduces skeletogenesis in corals. Mar. Biol. 127, 319–328. doi: 10.1007/BF00942117
Mateo M. A., Renom P., Hemminga M. A., Peene J. (2001). Measurement of seagrass production using the 13C stable isotope compared with classical O2 and 14C methods. Mar. Ecol. Prog. Ser. 223, 157–165. doi: 10.3354/meps223157
McGowan M. P. (2004). Submarine groundwater discharge: freshwater and nutrient input into Hawai‘is coastal zone [master’s thesis] (Honolulu (HI: University of Hawai‘i at Mānoa).
Mehrbach C., Culberson C. H., Hawley J. E., Pytkowicx R. M. (1973). Measurement of the apparent dissociation constants of carbonic acid in seawater at atmospheric Pressure1. Limnol. Oceanogr. 18, 897–907. doi: 10.4319/lo.1973.18.6.0897
Moore W. S. (2010). The effect of submarine groundwater discharge on the ocean. Ann. Rev. Mar. Sci. 2, 59–88. doi: 10.1146/annurev-marine-120308-081019
Moosdorf N., Stieglitz T., Waska H., Dürr H., Hartmann J. (2015). Submarine groundwater discharge from tropical islands: a review. Grundwasser 20, 53–67. doi: 10.1007/s00767-014-0275-3
Nelson C. E., Donahue M. J., Dulaiova H., Goldberg S. J., La Valle F. F., Lubarsky K., et al. (2015). Fluorescent dissolved organic matter as a multivariate biogeochemical tracer of submarine groundwater discharge in coral reef ecosystems. Mar. Chem. 177, 232–243. doi: 10.1016/j.marchem.2015.06.026
Nixon S. W., Oviatt C. A., Frithsen J., Sullivan B. (1986). Nutrients and the productivity of estuarine and coastal marine ecosystems. J. Limnol. Soc South. Afr. 12, 43–71. doi: 10.1080/03779688.1986.9639398
Nyström M. (2006). Redundancy and response diversity of functional groups: implications for the resilience of coral reefs. Ambi 35, 30–35. doi: 10.1579/0044-7447-35.1.30
Peterson B. J., Stubler A. D., Wall C. C., Gobler C. J. (2012). Nitrogen-rich groundwater intrusion affects productivity, but not herbivory, of the tropical seagrass thalassia testudinum. Aquat. Biol. 15, 1–9. doi: 10.3354/ab00413
Richardson C. M., Dulai H., Popp B. N., Ruttenberg K., Fackrell J. (2017b). Submarine groundwater discharge drives biogeochemistry in two Hawaiian reefs. Limnol. Oceanogr. 62, S348–S363. doi: 10.1002/lno.10654
Richardson C. M., Dulai H., Whittier R. B. (2017a). Sources and spatial variability of groundwater-delivered nutrients in Maunalua Bay, O‘ahu, Hawai‘i. J. Hydrol.: Regional Stud. 11, 178–193. doi: 10.1016/j.ejrh.2015.11.006
Roth F., El-Khaled Y. C., Karcher D. B., Rädecker N., Carvalho S., Duarte C. M., et al. (2021). Nutrient pollution enhances productivity and framework dissolution in algae- but not in coral-dominated reef communities. Mar. pollut. Bull. 168, 112444. doi: 10.1016/j.marpolbul.2021.112444
Santos I. R., Chen X., Lecher A. L., Sawyer A. H., Moosdorf N., Rodellas V., et al. (2021). Submarine groundwater discharge impacts on coastal nutrient biogeochemistry. Nat. Rev. Earth Environ. 2, 307–323. doi: 10.1038/s43017-021-00152-0
Santos I. R., Erler D., Tait D., Eyre B. D. (2010). Breathing of a coral cay: tracing tidally driven seawater recirculation in permeable coral reef sediments. J. Geophys. Res. Oceans 115. doi: 10.1029/2010JC006510
Silbiger N. J., Donahue M. J., Lubarsky K. (2020). Submarine groundwater discharge alters coral reef ecosystem metabolism. Proc. R. Soc B. 287, 20202743. doi: 10.1098/rspb.2020.2743
Slomp C. P., Van Cappellen P. (2004). Nutrient inputs to the coastal ocean through submarine groundwater discharge: controls and potential impact. J. Hydrol. 295, 64–86. doi: 10.1016/j.jhydrol.2004.02.018
Steneck R. S., Dethier M. N. A. (1994). Functional group approach to the structure of algal-dominated communities. Oikos 69, 476–498. doi: 10.2307/3545860
Sudhir P., Murthy S. D. S. (2004). Effects of salt stress on basic processes of photosynthesis. Photosynt 42, 481–486. doi: 10.1007/S11099-005-0001-6
Sugimoto R., Kitagawa K., Nishi S., Honda H., Yamada M., Kobayashi S., et al. (2017). Phytoplankton primary productivity around submarine groundwater discharge in nearshore coasts. Mar. Ecol. Prog. Ser. 563, 25–33. doi: 10.3354/meps11980
Swarzenski P. W., Dulai H., Kroeger K. D., Smith C. G., Dimova N., Storlazzi C. D., et al. (2017). Observations of nearshore groundwater discharge: kahekili beach park submarine springs, Maui, Hawaii. J. Hydrol.: Regional Stud. 11, 147–165. doi: 10.1016/j.ejrh.2015.12.056
Taniguchi M., Dulai H., Burnett K. M., Santos I. R., Sugimoto R., Stieglitz T., et al. (2019). Submarine groundwater discharge: updates on its measurement techniques, geophysical drivers, magnitudes, and effects. Front. Environ. Sci. 7. doi: 10.1016/j.csr.2021.104643
Teichberg M., Fox S. E., Aguila C., Olsen Y. S., Valiela I. (2008). Macroalgal responses to experimental nutrient enrichment in shallow coastal waters: growth, internal nutrient pools, and isotopic signatures. Mar. Ecol. Prog. Ser. 368, 117–126. doi: 10.3354/meps07564
Tide Predictions – NOAA Tides & Currents. Available at: https://tidesandcurrents.noaa.gov/noaatidepredictions.html?id=1612340 (Accessed February 27, 2017).
Torres M. E., Mix A. C., Rugh W. D. (2005). Precise δ13C analysis of dissolved inorganic carbon in natural waters using automated headspace sampling and continuous-flow mass spectrometry. Limnol. Oceanogr.: Methods 3 (8), 349–360. doi: 10.4319/lom.2005.3.349
Troccoli-Ghinaglia L., Herrera-Silveira J. A., Comín F. A., Díaz-Ramos J. R. (2010). Phytoplankton community variations in tropical coastal area affected where submarine groundwater occurs. Cont. Shelf Res. 30, 2082–2091. doi: 10.1016/j.csr.2010.10.009
Uppstrom L. R. (1974). The boron/chlorinity ratio of deep-sea water from the pacific ocean. Deep Sea Res. 21, 161–162. doi: 10.1016/0011-7471(74)90074-6
Valiela I., McClelland J., Hauxwell J., Behr P. J., Hersh D., Foreman K. (1997). Macroalgal blooms in shallow estuaries: controls and ecophysiological and ecosystem consequences. Limnol. Oceanogr. 42, 1105–1118. doi: 10.4319/lo.1997.42.5_part_2.1105
Vaughan E. J., Wilson S. K., Howlett S. J., Parravicini V., Williams G. J., Graham N. A. J. (2021). Nitrogen enrichment in macroalgae following mass coral mortality. Coral. Reefs 40, 767–776. doi: 10.1007/s00338-021-02079-w
Waska H., Kim G. (2011). Submarine groundwater discharge (SGD) as a main nutrient source for benthic and water-column primary production in a large intertidal environment of the yellow Sea. J. Sea Res. 65, 103–113. doi: 10.1016/j.seares.2010.08.001
Wolanski E., Martinez J. A., Richmond R. H. (2009). Quantifying the impact of watershed urbanization on a coral reef: maunalua bay, Hawaii. Estuarine Coast. Shelf Sci. 84, 259–268. doi: 10.1016/j.ecss.2009.06.029
Yang Y.-F., Fei X.-G., Song J.-M., Hu H.-Y., Wang G.-C., Chung I. K. (2006). Growth of gracilaria lemaneiformis under different cultivation conditions and its effects on nutrient removal in Chinese coastal waters. Aquaculture 254, 248–255. doi: 10.1016/j.aquaculture.2005.08.029
Keywords: primary productivity, benthic chamber, isotope enrichment, natural abundance, in situ experiment, algae, macroalgae, phytoplankton
Citation: La Valle FF, Jacobs JM, Thomas FI and Nelson CE (2023) Nutrient-rich submarine groundwater discharge increases algal carbon uptake in a tropical reef ecosystem. Front. Mar. Sci. 10:1178550. doi: 10.3389/fmars.2023.1178550
Received: 02 March 2023; Accepted: 02 June 2023;
Published: 20 June 2023.
Edited by:
Wei Jiang, Guangxi University, ChinaReviewed by:
Jianan Liu, East China Normal University, ChinaAndrea Pain, University of Maryland, College Park, United States
Copyright © 2023 La Valle, Jacobs, Thomas and Nelson. This is an open-access article distributed under the terms of the Creative Commons Attribution License (CC BY). The use, distribution or reproduction in other forums is permitted, provided the original author(s) and the copyright owner(s) are credited and that the original publication in this journal is cited, in accordance with accepted academic practice. No use, distribution or reproduction is permitted which does not comply with these terms.
*Correspondence: Florybeth Flores La Valle, ZmxvcnliZXRoLmxhdmFsbGVAcGVwcGVyZGluZS5lZHU=