- 1Ecologia Marina, Centre d’Estudis Avançats de Blanes (CEAB-CSIC), Blanes, Spain
- 2Biodiversitat i Recursos Marins, Universitat de Girona (UdG), Girona, Spain
Active restoration actions are becoming increasingly common for the recovery of degraded ecosystems. However, establishing when an ecosystem is fully restored is rarely achieved, since the recovery of entire communities needs long-term trajectories. The lack of evidence of success is even more severe in marine ecosystems, especially in the context of macroalgal forests, where beyond the vegetation structure and species diversity there is no approximation determining the recovery of the overall functionality. Trait-based ecology facilitates the link between species composition and ecosystem functions and processes. In this study, we used a trait-based approach to assess functional recovery ten years after the start of a restoration action in a marine macroalgal forest. Species and functional diversity were compared among the restored locality, a nearby locality where the expansion of the restoration is naturally occurring, a neighbouring non-restored locality (at a distance of a few meters), and the only two remaining localities dominated by the same structural macroalga that were used as reference (non-perturbed). Species diversity and composition of the restored locality were similar to those found in reference macroalgal forests, while the non-restored and expansion locality showed different species composition and lower species diversity. Functional richness was 4-fold higher in the restored locality than in the non-restored one, even surpassing one reference macroalgal locality. The restored locality showed a greater number of trait categories, especially traits related to higher structural complexity and longer life spans, indicating changes in ecosystem functions and processes. The restoration of a canopy-forming macroalga is the first step to achieving the recovery of an entire macroalgal forest (i.e., associated species and functional diversity). The application of traditional taxonomical indices plus functional parameters provides useful insights into the assessment of the success of restoration actions at the community level, emerging as a promising approach to be replicated and contrasted in other marine and terrestrial ecosystems.
1 Introduction
The impact of humans on natural environments occurs in a wide variety of the world’s ecosystems, mainly derived from changes in land use, pollution, and exploitation of natural resources (Sanderson et al., 2002; Foley et al., 2005; Ellis et al., 2010). These human alterations have led to widespread habitat loss, changes in biodiversity, species invasions and extinctions, and declines in ecosystem condition (Butchart et al., 2010; Ceballos et al., 2015). Consequently, shifts in the functioning of ecosystems have been detected and, in some cases, changes in some ecosystems services that are of value for human societies (Dobson et al., 2006; Cardinale et al., 2012).
Some ecosystems are so degraded that natural recovery is almost impossible in short and medium terms, even when the impacts leading to their collapse have been removed. Human-mediated restoration initiatives are often applied, becoming one of the only tools capable of reverting the loss of an ecosystem (Dobson et al., 1997; Holl & Aide, 2011). Ecological restoration has emerged as a critical measure for the management of heavily degraded ecosystems and is now a global priority (Aronson & Alexander, 2013; Suding et al., 2015). Supporting this idea, the Convention on Biological Diversity has set restoration targets (Secretariat of the Convention on Biological Diversity, 2014), and in 2019 the “United Nation Decade of ecosystem restoration 2021-2030” was declared. In fact, the number of undertaken restoration actions has increased notably over the past decades (Wortley et al., 2013; Carlucci et al., 2020), evolving from mitigation – removing human disturbances to allow for unassisted recovery – to active restoration – direct interventions in an effort to intentionally influence the trajectory of recovery (Simenstad et al., 2006; Elliott et al., 2007).
Some ecosystems have traditionally been more actively restored than others (e.g., terrestrial forests and grasslands) and several years of practice have determined what characterizes successful restoration. In general, vegetation structure, species diversity, and ecosystem functions have been suggested as the three key attributes to assess restoration outcomes (Ruiz-Jaen & Aide, 2005; Wortley et al., 2013). However, while the trajectory of vegetation structure (e.g., vegetation cover, density, and biomass) and species diversity (e.g., richness and abundance) following restoration is well understood, knowledge regarding the recovery effect on ecosystem functions is still limited (Brudvig, 2011; Kollmann et al., 2016). Many evaluations of restoration success first focus on the development of the target species because it is broadly assumed that following its recovery it will add structure to the habitat (Geist & Hawkins, 2016). In doing so, other associated organisms should benefit from the increase in structural complexity and the ecosystem will eventually recover its processes and functions (Geist & Hawkins, 2016). However, recent studies have demonstrated that in some cases either species composition or ecosystem processes have not been fully re-established, pointing to the need to empirically test the relationship between the recovery of a target – usually foundation species – and the processes and functions of the ecosystem (Rey Benayas et al., 2009; Moreno-Mateos et al., 2012; Crouzeilles et al., 2016). In this line, assessment of ecosystem functional recovery should include not only target species viability but also quantifiable properties to describe the community in terms of structure alongside ecosystem functions (Montoya et al., 2012).
Trait-based ecology enables the analysis of the influence of species identity and composition on ecosystem functions and processes (Garnier et al., 2004; McGill et al., 2006). Functional traits are determined by morphological, physiological, and biological characteristics of the species and are considered relevant to influence the environment, affecting ecosystem properties and services (Violle et al., 2007; Díaz et al., 2013). Thus, functional diversity indices based on trait information improve the understanding of the functioning of an ecosystem (Mouillot et al., 2013), and when used together with traditional taxonomic-based indices they can provide a comprehensive evaluation of restoration projects (Cadotte et al., 2011; Montoya et al., 2012; Laughlin, 2014). Indeed, the application of functional trait approaches to ecological restoration is receiving growing attention worldwide (Carlucci et al., 2020), with some examples mainly focused on vegetation (e.g., Piqueray et al., 2015; Engst et al., 2016; Zirbel et al., 2017). To achieve in-depth and thorough assessments of functional recovery, studies should include as many species as possible and a broad combination of traits encompassing different facets of ecological processes. To this effect, comprehensive assessments are still needed for many ecosystems, probably because the required surveys to gather information about different species groups and species traits is challenging and time consuming.
In marine coastal regions, brown seaweeds can create complex ecosystems often described as macroalgal forests, where macroalgae are the major contributors to primary production, providing food and shelter for other organisms, and consequently hosting an important biodiversity hotspot (Steneck et al., 2002; Smale et al., 2013). In the Mediterranean Sea, macroalgal forests are characterized by canopy-forming species of the genus Cystoseira sensu lato (including the genera Cystoseira C. Agardh, Gongolaria Boehmer and Ericaria Stackhouse; Molinari & Guiry, 2020), representing the highest level of complexity. However, several Mediterranean macroalgal forests have been declining over the last decades, such that previously widespread Cystoseira s.l. species have become locally extinct or their populations have been severely reduced and fragmented (e.g., Thibaut et al., 2005; Thibaut et al., 2015; Mariani et al., 2019). The first example of a self-sustaining macroalgal population after restoration in the Mediterranean Sea was reported by Verdura et al. (2018). In March 2011, a single restoring action allowed the recovery of a previously extinct population of the macroalga Gongolaria barbata in the Bay of Maó, Menorca (NW Mediterranean) (Figure 1). The species thrived in the area one hundred years ago, but it became locally extinct following a reduction in water quality related to waste water effluents and harbour operations (Sales et al., 2011). Even when water quality was improved due to management measures involving stopping wastewater dumping, G. barbata populations were unable to recover. The success of the restoring action was first assessed considering the density and size structure distribution of the target species, which showed comparable values to those obtained in the reference population (Verdura et al., 2018) (Figure 1). The presence of fertile individuals and new recruits confirmed that the restored population was self-maintained. More recently, a high-resolution cartography was carried out to describe the expansion patterns of the population, finding an increase of three orders of magnitude in the area occupied by G. barbata since the restoring action took place (Gran et al., 2022).
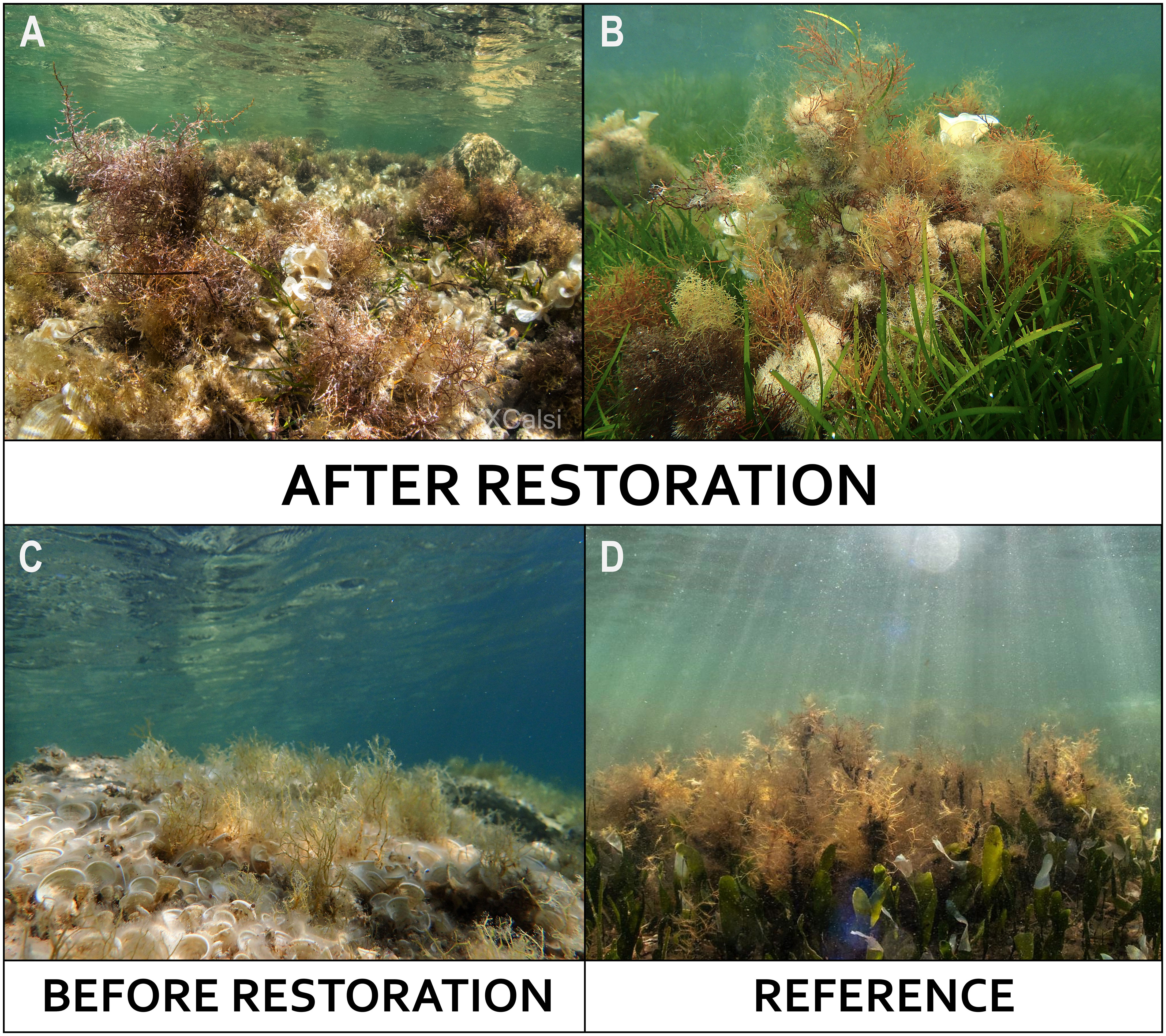
Figure 1 (A, B) Gongolaria barbata locality after the restoring action in the Bay of Maó (Menorca); (C) the “before-the-action” locality sat in the Bay of Maó; and (D) G. barbata habitat in one reference locality, in Cala Rotja. Photo credits: Xavi Calsina (@XCalsi; A), Enric Ballesteros (B), Cristina Galobart (C) and Jordi Boada (D).
The lack of recovery evidence is even more severe in marine ecosystems, since marine restoration science is very young in comparison to its terrestrial counterpart (Elliott et al., 2007; Rey Benayas et al., 2009; Kollmann et al., 2016, but see Saunders et al., 2020), representing only 7% of all restoring actions available in the literature (Blignaut et al., 2013). In the context of macroalgal forests, the few worldwide successful examples of active restoration are focused on the recovery of canopy-forming species (Whitaker et al., 2010; Verdura et al., 2018; Fredriksen et al., 2020; Layton et al., 2020; Cebrian et al., 2021). To our knowledge, only two studies have introduced the measurement of the re-established diversity, addressed by species composition and their abundance (Ling, 2008; Marzinelli et al., 2016). Further, macroalgal restoration projects are in general maintained over time spans of less than 2 years (Eger et al., 2022).
Here, we assess for the first time the recovery of ecosystem functions in a ten-year restored macroalgal forest dominated by Gongolaria barbata. Using both traditional taxonomical indices and a functional trait approach, we test the assumption that after the reintroduction of the primary and structural species the associated biodiversity will recover and, in turn, the associated ecosystem functions and processes. We also hypothesised that the time since the presence of the structural species will influence the outcomes, with longer periods showing better recoveries. To do so, we considered the entire algal species composition and included 14 functional traits related to major aspects of the macroalgal species ecology. The main aim of the present study is to provide evidence of macroalgal taxonomical and functional recovery by describing a valid and solid approach which can be easily replicated and compared among marine and terrestrial ecosystems.
2 Materials and methods
2.1 Localities and sample collection
To study the taxonomical and functional recovery following restoration we have surveyed five different localities: the focus of the initial restoration intervention, with > than 9 years of the structural species presence (hereafter “Restored”); a nearby locality where G. barbata is growing and represents the natural expansion of the restoration, with > than 3 years of the structural species presence (hereafter “Expansion Area”); a non-restored locality placed 100 m from the restored one and where the structural species has not yet arrived, representing the “before-the-action” locality (hereafter, “Non-Restored”); and two reference localities where the specimens used in the restoration come from, Cala Rotja (hereafter, “Reference 1”) and Miami (hereafter, “Reference 2”) (Figure 2). “Reference 1” and “Reference 2” corresponded to the only two remaining G. barbata populations in the Spanish Mediterranean and nearby France (the closest population is found hundreds of km away), and represent mature and well-established G. barbata habitats (Sales & Ballesteros, 2009; Verdura et al., 2018; Mariani et al., 2019). All the studied localities were shallow (0-1 m depth) and sheltered.
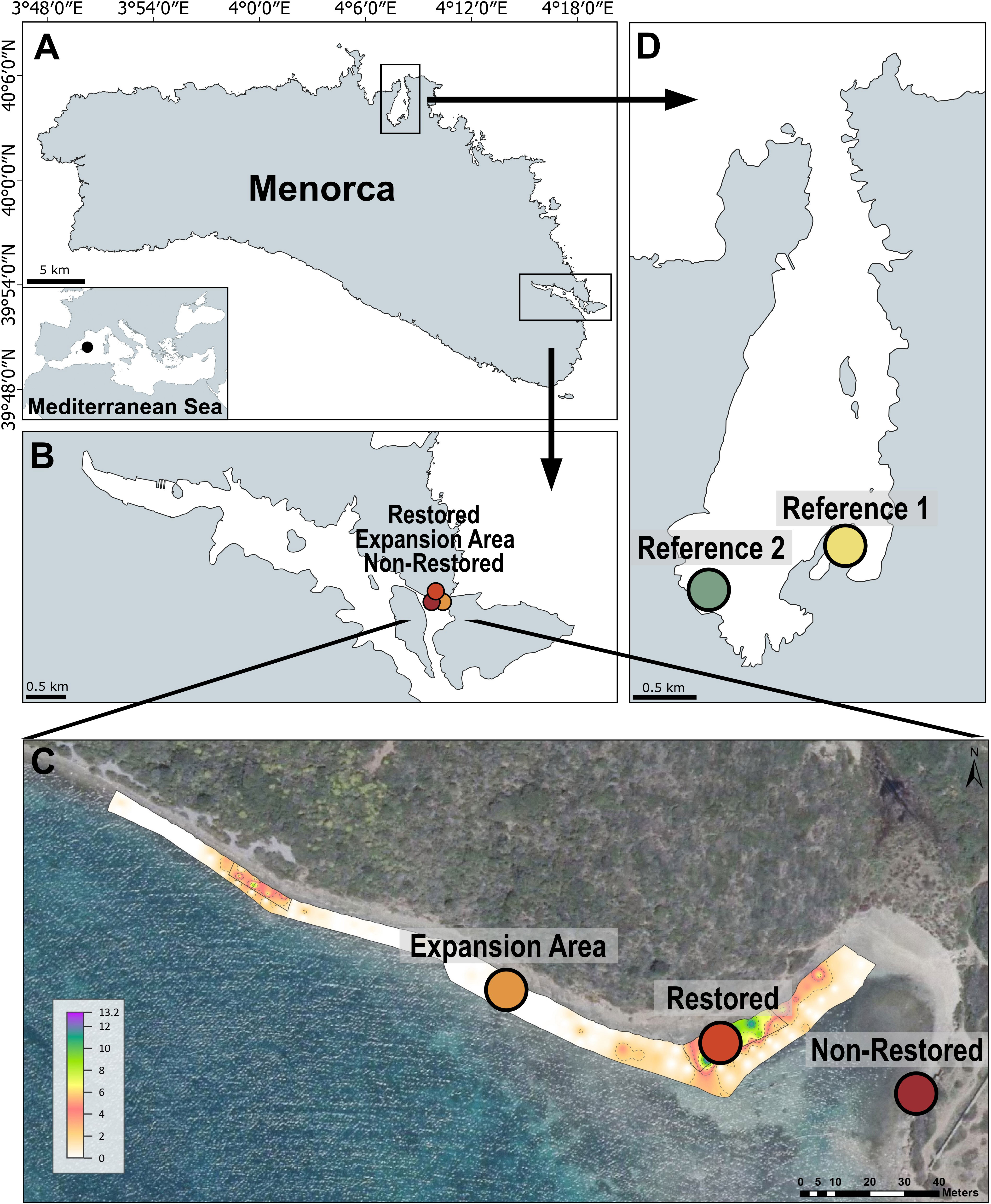
Figure 2 (A) Map of Menorca, NW Mediterranean; (B, C) Restored, Expansion Area and Non-Restored sampling localities in the Bay of Maó (collected 100 m apart); (D) Reference localities of Gongolaria barbata in Fornells Bay. The lower plot (C) was produced by Gran et al. (2022) and represents the abundance of G. barbata restored population, showing the two focal points of the restoration action (delimited by square quadrats) and the expansion area (where G. barbata is naturally growing and expanding after the action). Colours indicate % abundance according to the scale included.
To determine the benthic macroalgal composition, three samples (replicates) were randomly collected at each locality in summer 2019. For each sample, the whole community was removed from an area of 20 x 20 cm with hammer and chisel (Ballesteros, 1986). The sampling area and the number of replicates were based on the information provided by previous studies that defined the minimum sampling area for Mediterranean infra-littoral assemblages dominated by canopy-forming macroalgae (Cinelli et al., 1977; Dhondt & Coppejans, 1977; Boudouresque and Belsher, 1979; Coppejans, 1980; Ballesteros, 1992; Sant et al., 2017). The use of this number of replicates and the area of each sample represents a well-established method across Mediterranean literature (e.g., Mangialajo et al., 2008; Orlando-Bonaca & Rotter, 2018; Piazzi et al., 2018; Sedano et al., 2019). Samples were placed in plastic bags and preserved in 4% seawater-formalin solution for further laboratory identification. Most macroalgal individuals were identified to species level and, whenever this was not possible, to genera level. Species abundances were calculated as dry weights (in g), obtained after 48 hours of drying at 60°C.
2.2 Traditional biodiversity indices
For each sample, we calculated species biomass (B), species richness (S), and species diversity (Shannon-Wiener index, H’). One-way ANOVA with locality (five levels: Non-Restored, Restored, Expansion Area, Reference 1 and Reference 2) as a fixed factor was then performed for each index. We tested for normal distribution of residuals and homogeneity of variances using Shapiro-Wilk and Levene’s tests, respectively, to check the assumptions of the model (all p-values > 0.05). When the ANOVA proved significant results we performed Tukey pairwise-comparisons to test for differences between locality pairs.
To investigate patterns of variation in the species composition among samples and localities, we used a 3D non-metric multidimensional scaling (nMDS) ordination analysis based on Bray-Curtis similarities, with species abundance data previously square-root transformed. To test for differences in Bray-Curtis distances between our samples we used a permutational analysis of variance (PERMANOVA), again with locality (five levels: Non-Restored, Restored, Expansion Area, Reference 1 and Reference 2) as a fixed factor (Anderson, 2017).
2.3 Functional characterization of macroalgal species
Functional diversity was assessed using species trait information. Following the methods described in Teixidó et al. (2018), we considered the traits strictly related to macroalgae and adapted their categorization. We focused on the following 14 traits: morphological form, solitary-colonial life history, maximum longevity, maximum height, maximum width, epibiosis, major photosynthetic pigments, age of reproductive maturity, potential of asexual reproduction, growth rates, physical defences/calcification, and chemical defences. In addition, we added two more traits to better capture species ecological functions: algal biological cycle and type of vegetative (Supplementary Table 1). Trait category values for each species were obtained from Teixidó et al. (2018) or were assigned by team member expertise and the published literature. Hereafter, species sharing the same values in all trait categories were defined as functional entities (FEs, unique combinations of traits).
2.4 Functional diversity
For each locality we calculated the number of species, the number of FE, and the functional richness (Fric). Fric represents the amount of functional space calculated as the volume inside a convex hull, shaped by the position of FEs (Mouillot et al., 2013). The convex hull algorithm determines the most external FEs and joins these points to calculate the volume inside, in a way that the final multidimensional space is the minimum space that includes all the present FEs. To determine the position of each FE, we first used the Gower metric – which allows for mixing different types of variables – to calculate a pairwise distance matrix (Podani & Schmera, 2006). Using the distance matrix, a Principal Coordinate Analysis (PCoA) was computed to build the multidimensional space. The number of axes was selected using the mean squared-deviation index (mSD), which was calculated between FEs initial distances and FEs final distances in the functional space (Maire et al., 2015). Including five PCoA dimensions allowed us to retain 64% of the explained variation and a reasonably low mSD value (0.0082). Last, we obtained the percentage of functional volume that overlaps between pairs of localities (that is, the proportional volume of one locality that is shared with another locality).
All analyses were conducted in R version 4.0.2 (R Development Core Team, 2018).
3 Results
3.1 Traditional biodiversity indices and species composition
A total of 60 macroalgal and one seagrass species were found in the five localities (Supplementary Table 2). Species biomass (B), species richness (S), and species diversity (measured with Shannon-Wiener index, H’) significantly varied among localities (Supplementary Table 3). Species biomass was 3-fold greater in “Restored” than in “Non-Restored” (393 ± 75 and 122 ± 87 g m-2, respectively), while “Expansion Area” showed intermediate values (254 ± 93 g m-2). The biomass of “Restored”, “Reference 1” and “Reference 2” was similar (407 ± 153 and 357 ± 44 g m-2 in “Reference 1” and “Reference 2”, respectively) (Figure 3A). In the same line, mean species richness (S) and species diversity (H’) were also high in “Restored”, with values reaching those found in the richest reference locality (“Reference 1”) (Figures 3B, C). In contrast, “Non-Restored” and “Expansion Area” consistently showed the lowest values of species richness and diversity (Figures 3B, C).
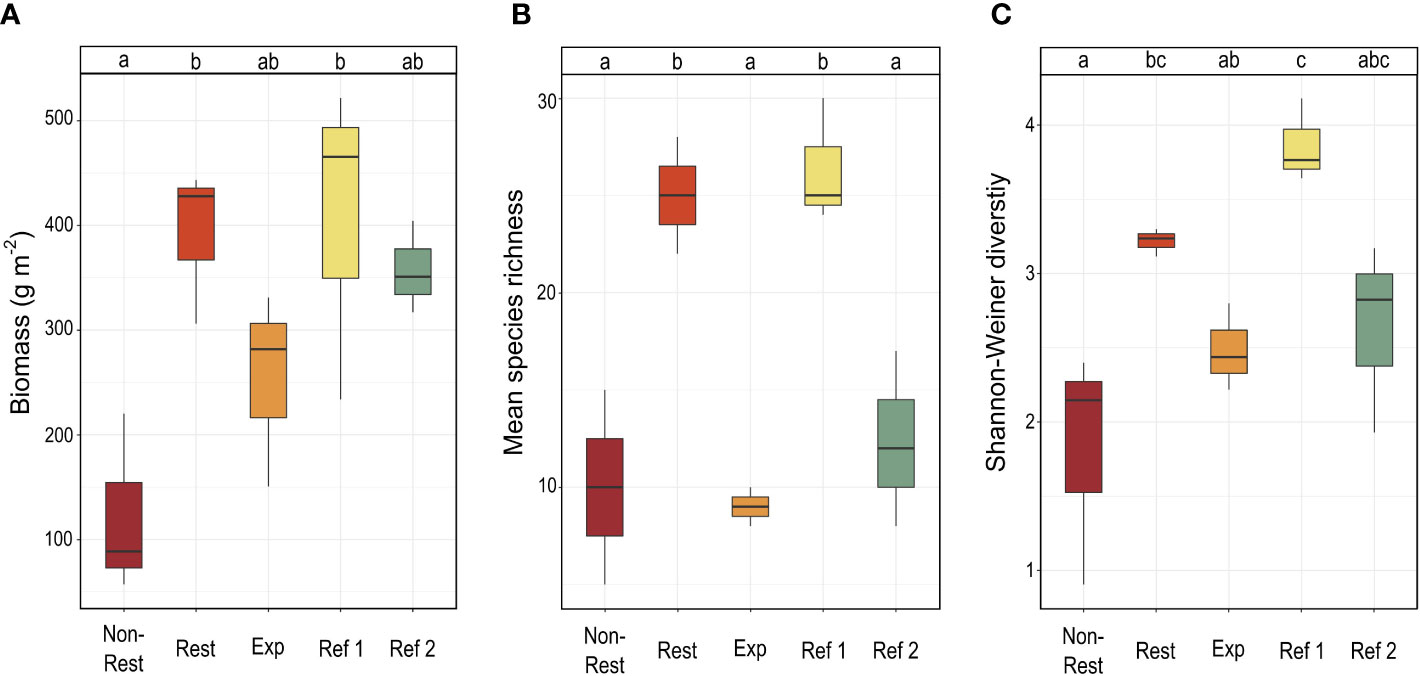
Figure 3 Variability of biomass (A); mean species richness (B); and Shannon-Weiner diversity (C) between localities. Dissimilar letters above boxes indicate significant differences (p-values of Tukey test with 95% confidence intervals). Locality codes: “Non-Restored” (Non-Rest), “Restored” (Rest), “Expansion Area” (Exp), “Reference 1” (Ref 1), “Reference 2” (Ref 2).
Species composition also differed among localities (PERMANOVA: r2 = 0.59, F = 3.61, p-value = 0.001). The nMDS ordination showed that whereas species composition of “Restored” and “Expansion Area” was similar, leading to closer and sometimes overlapping positions in the ordination space, “Non-Restored” (which was collected only few meters apart) was distinctly separated from them (Figure 4). Both “Reference 1” and “Reference 2” also differed from all other localities (“Restored”, “Expansion Area” and “Non-Restored”), thus showing variability in species composition among different G. barbata populations (Figure 4).
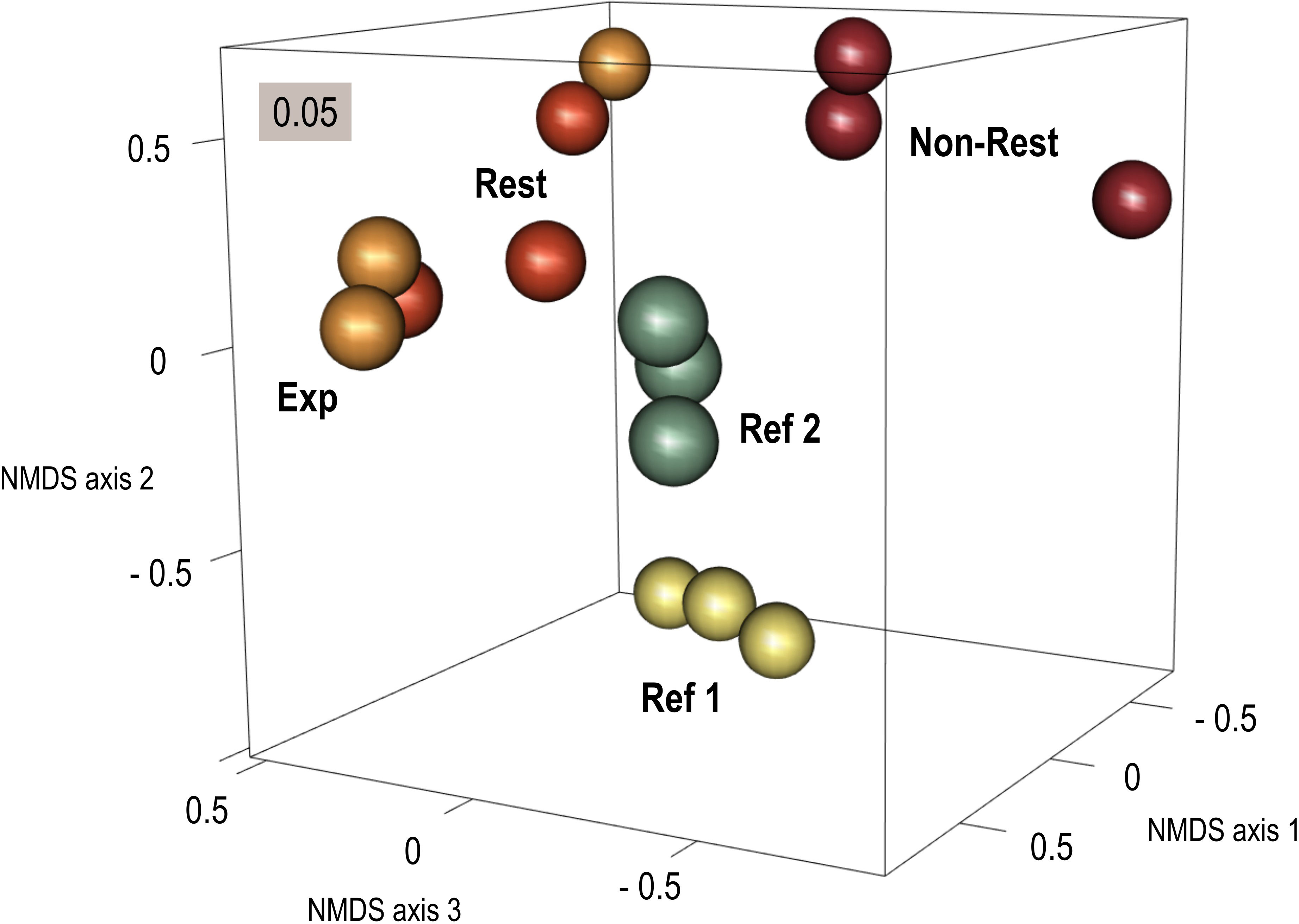
Figure 4 Non-metric multidimensional scaling (nMDS) ordination of species assemblages, based on square-root transformed abundance data (dry weight) and Bray-Curtis dissimilarity. Locality codes: “Non-Restored” (Non-Rest), “Restored” (Rest), “Expansion Area” (Exp), “Reference 1” (Ref 1), “Reference 2” (Ref 2).
3.2 Functional diversity
The total number of identified macroalgal species ranged from 13 in “Expansion Area” to 39 in “Restored” (Figure 5). “Non-Restored” and “Reference 2” showed similar number of species (17 and 19, respectively), while the value found in “Reference 1” was greater and closer to “Restored” (34 and 39, respectively). The high value of species richness in “Restored” is linked to a high number of functional entities (FEs, unique combination of traits). Accordingly, we found 36 FEs in “Restored”, while 13 and 16 FEs in “Expansion Area” and “Non-Restored”, respectively. In addition to the gain of species and FEs, “Restored” showed a 4-fold increase in functional richness (Fric see methods section) when compared to “Non-Restored”, from 0.0016 to 0.0069, respectively (Figure 5). The functional richness in “Expansion Area” did not increase as in “Restored” and was the one with less functional volume (0.0012). For both “Reference 1” and “Reference 2”, the number of species, FEs and functional richness were consistently higher than in “Non-Restored” and “Expansion Area”, but were always lower than in “Restored”. Interestingly, while “Non-Restored” and “Reference 2” presented similar numbers of FEs (16 and 18, respectively), the functional volume that “Reference 2” occupied doubled that of “Non-Restored” (0.0033 and 0.0016, respectively), since the FEs found in “Non-Restored” had similar combination of traits thus leading to closer positions in the functional space (Figure 6). Indeed, the visual representation of FEs and their abundance in the functional space revealed that in “Non-Restored” only two main and similar FEs accounted for the majority of total abundance, whereas in “Restored”, “Expansion Area”, and both reference localities, abundances were distributed among a greater number of FEs (Figure 6). “Restored” and “Expansion Area” showed a similar distribution of FEs, especially when considering the most abundant ones (Figure 6). However, “Restored” supported a high number of non-abundant FEs that were not present in “Expansion Area” (Figure 6).
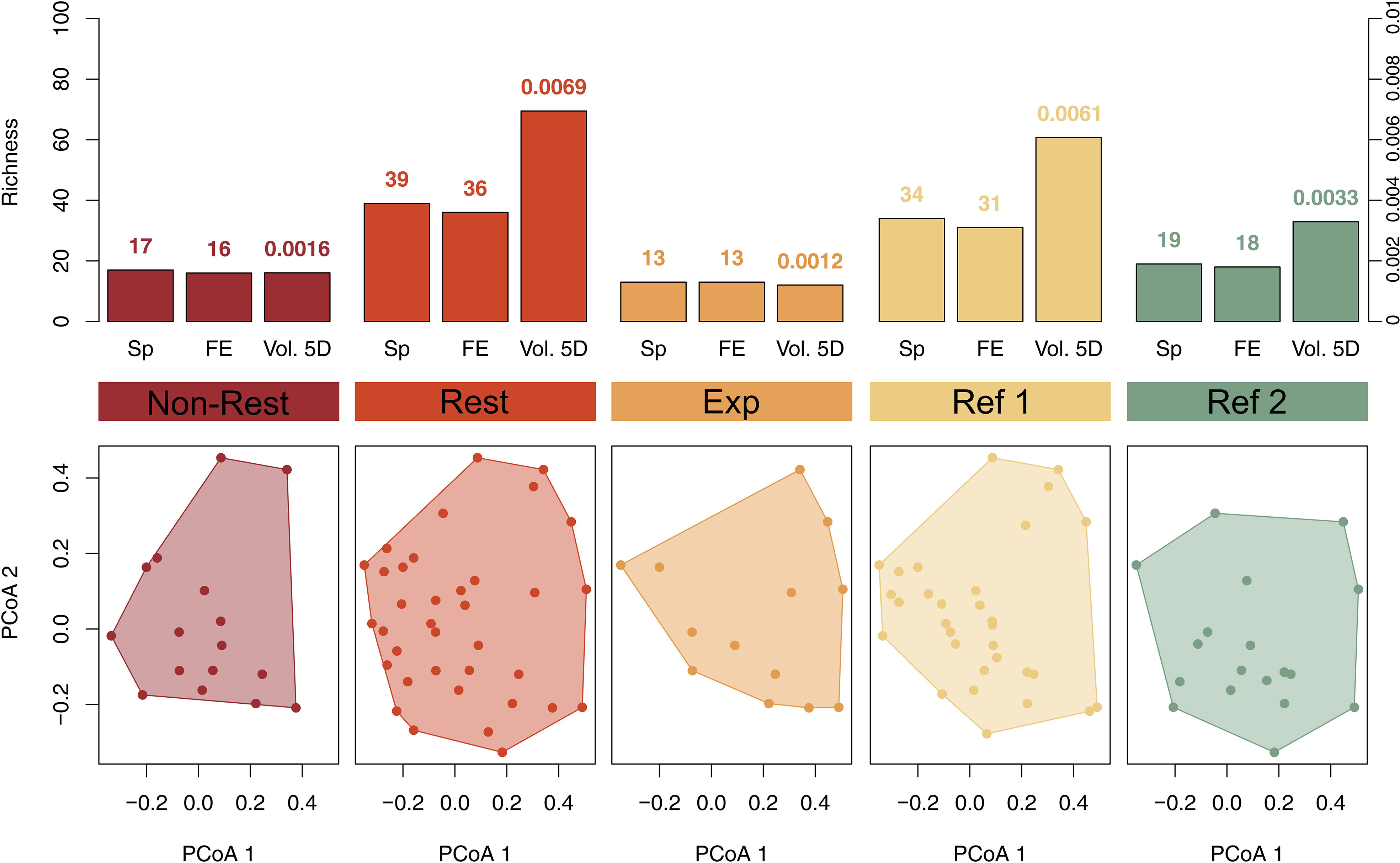
Figure 5 Upper plots: Number of species (Sp) and number of functional entities (FE) in left axis, and functional diversity (Vol. 5D) in right axis, among the five studied localities (values are displayed above corresponding bars). Lower plots: Functional diversity is calculated as the space filled by all the FEs (filled points) present in each locality. The axes (PCoA 1 and PCoA 2) represent the first two dimensions of the 5D space from a Principal Coordinate Analysis (PCoA = computed on functional-trait values). Total number of species = 61; total number of FE = 54.
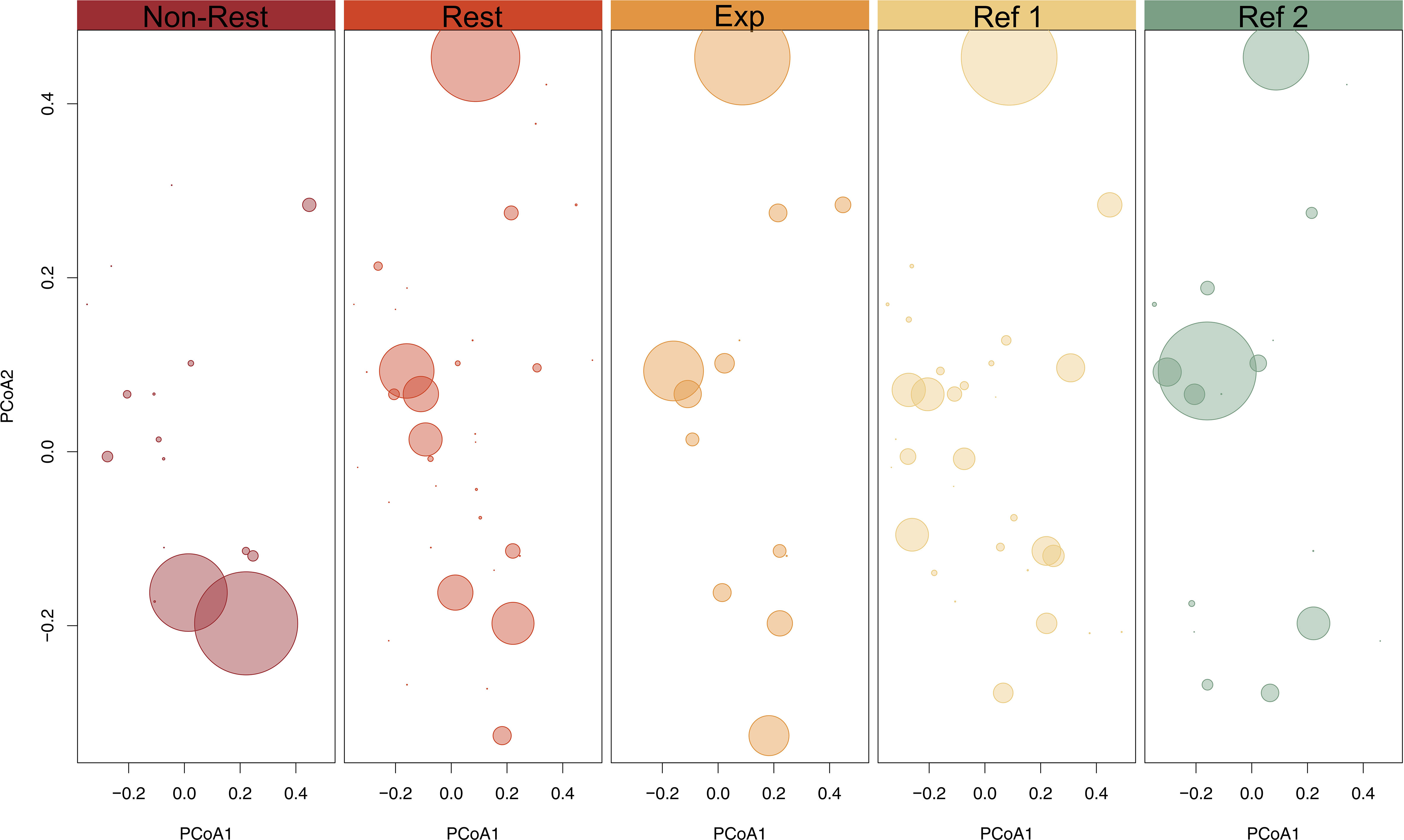
Figure 6 Distribution of FE abundance across the functional space. Each point represents a functional entity and point sizes are proportional to the relative abundance (measured as dry weight).
3.3 Functional traits across localities
The FEs in “Restored”, “Expansion Area”, and both reference localities were characterized by a wide variety of categories within the traits, whereas in “Non-Restored” were more homogenous (Figure 7). After 9 years of the restoration of G. barbata, macroalgal species from “Restored” reached the same varied combination of morphological forms as “Reference 1”, including 8 different morphologies (Figure 7). The “Expansion Area”, representing the area with 3 years of presence of the structural species, also showed 7 different morphologies. The diversity of categories of all the other traits considered in this study was consistently higher for “Restored” and “Reference 1”, which showed categories related to slower life histories, with examples of FEs measuring up to 50 cm, living up to 10-20 years and reaching reproductive maturity mostly between 1 and 2 years old (Figure 7). In contrast, 98% of FEs in “Non-Restored” accounted for only two morphologies (foliose erect/sheets and branched thallus) derived from the macroalgae Padina pavonica and Halopteris scoparia. The predominance of these two species in “Non-Restored” resulted in only one leading category in 9 out of 14 traits: solitary/colonial, height, width, major photosynthetic pigments, age at maturity reproduction, asexual reproduction, growth rates, chemical defences, and biological cycle. Moreover, FEs in “Non-Restored” exhibited categories describing more seasonal life histories, generally with smaller size (5-20 cm), shorter-lived species (1 year as a maximum) and rapid reproductive maturity ages (3-5 months). Overall, both reference localities, “Restored” and “Expansion Area” presented more heterogeneity within traits, with trait categories that were not present in “Non-Restored”, highlighting the higher functional diversity of these assemblages (Figure 7).
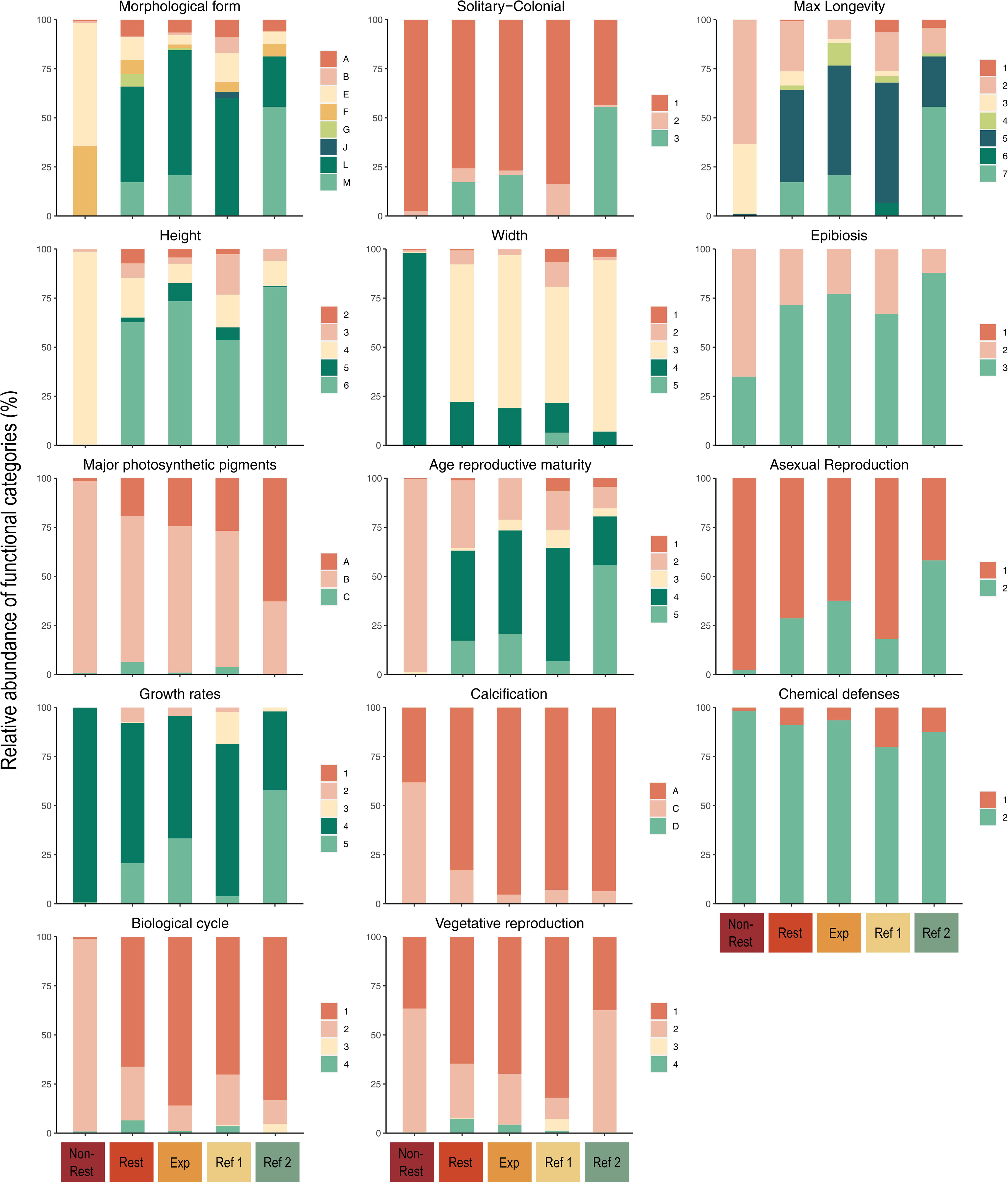
Figure 7 Relative abundances of each trait category in each locality. Trait codes: Morphological form: (A) filaments, (B) stolonial, (C) encrusting, (D) encrusting, leaf-like, with blades, (E) foliose erect thallus, sheets/blades, (F) coarsely branched, (G) articulated, (H) cup-like, (I) massive encrusting, (J) massive-hemispheric, (K) massive-erect, (L) tree-like, (M) grass-like; Solitary-colonial: (1) solitary, (2) gregarious, (3) colonial; Maximum longevity: (1) weeks, (2) 3–11 months, (3) 1 year, (4) 2 years, (5) 5 years, (6) 10–20 years, (7) >20 years; Height: (1) up to 1 mm, (2) 1–10 mm, (3) 10–50 mm, (4) 50–200 mm, (5) 200–500 mm, (6) 500 mm-2000 mm; Width: (1) up to 0.1 mm, (2) 0.1–1 mm, (3) 1–10 mm, (4) 10–50 mm, (5) 50–200 mm, (6) >200 mm; Epibiosis: (1) obligate, (2) facultative, (3) never; Major photosynthetic pigments: (A) Chl a, Chl b, β-carotene, xanthophyll, (B) Chl a, xanthophyll/fucoxanthin, Chl c1 + c2, (C) Chl a, phycocyanin, phycoerythrin; Age reproductive maturity: (1) weeks, (2) 3–5 months, (3) 6–11 months, (4) 1 year, (5) 2 years, (6) 2–5 years, (7) >5 years; Asexual reproduction: (1) no, (2) yes; Growth rates: (1) extreme slow (<1 cm/year), (2) slow (1 cm/year), (3) moderate (>1 cm/year), (4) high (5–10 cm/year), (5) very high (>10 cm/year); Calcification: (A) without, (B) external carbonate, (C) carbonate with discontinuities, (D) continuous carbonate; Chemical defenses: (1) no, (2) yes; Biological cycle: (1) monophasic, (2) diphasic isomorph, (3) diphasic heteromorph, (4) triphasic isomorph, (5) triphasic heteromorph; Vegetative reproduction: (1) without, (2) stolon, (3) fragments, (4) propagules.
4 Discussion
In this study, we provide the first example of recovery of ecosystem functions, under a functional-trait framework, after an active restoration in a macroalgal forest. The patterns in species and functional diversity reported here suggest that restoration is an important strategy in mitigating biodiversity losses and reinstating part of the lost functionality. Due to the limited restoration examples, marine restoration success is often based on short-term periods (1-2 years on average, Bayraktarov et al., 2016; Kollmann et al., 2016, but see Saunders et al., 2020). In terrestrial projects, however, the time elapsed since restoration started has been a key factor to reach similar conditions as those found in reference systems (Martin et al., 2013; Crouzeilles et al., 2016). Considering that the recovery of many marine ecosystems can take up to 15-25 years (Jones & Schmitz, 2009; Borja et al., 2010), the current time span used in most marine restoration activities might be insufficient to reliably evaluate the outputs. To this effect, where short-lived and high-turnover species are the target biological components of an ecosystem, full recovery could be achieved within shorter timescales (see examples in Borja et al., 2010). However, this is not the case of the structural components of macroalgal forests, where some of the foundational species are considered mid-/long-lived seaweeds (see examples in Schiel & Foster, 2006; Smale et al., 2013). Knowledge regarding the dynamics of the target species (e.g., growth rates, turnover, and longevity) should guide restoration efforts, pointing to the importance of long-term monitoring assessments for assemblages constituted of species with long life spans, such as some Mediterranean macroalgal forests (e.g., Ballesteros et al., 2002; Ballesteros et al., 2009; Capdevila et al., 2016).
Also derived from the short-term evaluations, especially in marine ecosystems, restoration success often considers parameters related to the target species’ survival, with common measures of growth or density of the individuals (Bayraktarov et al., 2016; Jacob et al., 2018; Cebrian et al., 2021). In terrestrial ecological restoration, vegetation structure is also the most common quantitative indicator (Ruiz-Jaen & Aide, 2005; Gómez-Aparicio, 2009) together with community measures such as the species richness, the biodiversity indices and the species composition (Gatica-Saavedra et al., 2017; Hughes et al., 2017). In this study, species biomass, mean number of species, and species diversity (Shannon-Weiner index) of the restored locality reached the values of one reference locality (“Reference 1”). The restored locality even surpassed mean values of the other reference locality (“Reference 2”), supporting restoration success. Variations in biodiversity of the two reference localities could be explained by differences in local factors, since a priori similar communities might be subjected to different environmental conditions, resulting in species composition variations (Bernhardt-Römermann et al., 2015; and see examples in Matthews et al., 2009; da Costa et al., 2019). Thus, local environmental factors (e.g., type of substrate or sediment loading) and biotic factors (e.g., grazing pressure) might determine species composition and diversity. For this reason, the inclusion of more than one reference locality in restoration assessments is highly recommendable to account for the variability of natural communities and to describe different, but possible, restoration outcomes (Gann et al., 2019; Prach et al., 2019).
The macroalgal species assemblage of the restored and expansion localities differed from the non-restored locality, and were also different when compared with the macroalgal forests of reference (Figure 8). This trend has also been found in other restoration projects, where restored systems do not strictly follow the expected direction toward references (e.g., Sluis, 2002; Matthews & Spyreas, 2010; Suganuma et al., 2014). Time will determine whether the restored locality will eventually mirror a reference macroalgal forests or it will remain structurally and functionally dissimilar. Recent restoring frameworks and directives suggest that ecosystem restoration should first aim to recover past ecosystems where viable, but where not, a shift in objectives targeting novel but still improved ones should be adopted (Jackson & Hobbs, 2009; Gann et al., 2019). Restored ecosystems may have new combinations of species that have not occurred previously, but that could ensure the maintenance of the structure and functions of the system (Suding & Gross, 2006; Choi et al., 2008). Even so, historical knowledge is highly valuable and should always be used as a guide which accepts multiple trajectories, considers future environmental changes, and seeks for the recovery of ecosystem services (Higgs et al., 2014; Martin, 2017; Gann et al., 2019).
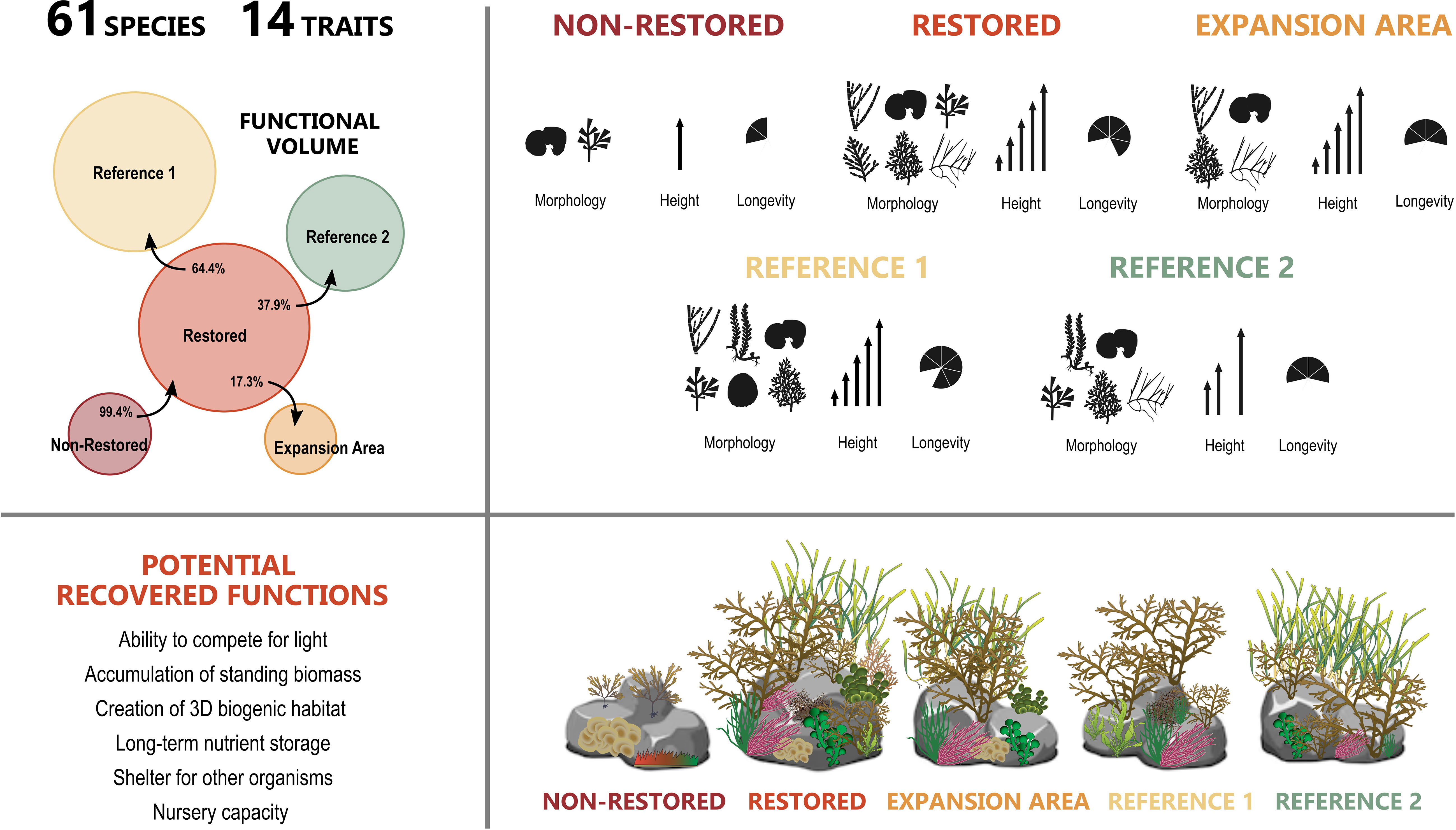
Figure 8 Upper right: Diagram of the functional volume of the studied localities, where circle sizes are proportional to the amount of functional space occupied by each locality. Percentages indicate the proportion of functional volume contained in one locality that is also contained within another locality (e.g., 99.5% of “Non-Restored” volume is shared with “Restored” volume, 37.7% of “Restored” volume is shared with “Reference 2” volume). Upper left: Representation of the variability of trait categories in each locality (three high variable traits were selected as an example and categories with an abundance greater than 2% are represented). Lower right: The potential ecosystem functions recovered in the restored locality. Lower left: Summarised representation of the species diversity in each locality. Graphics were obtained from the Integration and Application Network (ian.umces.edu/media-library).
We also demonstrated an increase of functional richness (Fric) with restoration (Figure 8), indicating that the recovery of macroalgal species filling vacant functional space not occupied prior to restoration, effectively occurred. Interestingly, we observed that the species composition, the distribution and the abundance of FEs and the variability within traits in the area where G. barbata is naturally spreading (“Expansion Area”) is highly similar to those of the restored locality (Figure 8). These similarities are due to the most dominant and abundant species in the restored locality being already present in the area of expansion. Despite this, functional diversity was lower since a high number of less abundant species are still lacking, showing that the recovery of some species and part of the biomass does not directly translate into a full recovery of all other species and their functionality (Figure 8). These results highlight, again, the need of long periods to allow the recovery of the whole community after restoration.
In the restored locality, there were more functional entities (FEs) and they were more distanced in the functional space, deriving from a greater range of categories within each trait (that is, more varied traits). In particular, after restoration, the macroalgal assemblage shifted from having only two main taxa and a small number of trait categories (e.g., two morphological forms, only solitary species, and one category of height and width) to a more heterogeneous assemblage involving six morphologies, the presence of gregarious and colonial taxa, and varied sizes with some large species but also other smaller ones. In addition, the restored assemblage included species with longer life spans and later reproductive maturity. In the same way as terrestrial plants, an increase in species architecture and size suggests a superior ability to compete for light (Reich, 2014) and it is sometimes associated with higher accumulation of standing biomass (de Bello et al., 2010). Similarly, recovering long-lived species along with morphological complexity is likely to increase the creation of three-dimensional biogenic habitats, increasing, for instance, long-term nutrient storage (de Bello et al., 2010; Teixidó et al., 2011) (Figure 8). Adding stable structure and complexity also offers shelter for other organisms and reinstates the nursery capacity of these communities (Ørberg et al., 2018; Fulton et al., 2019). Hence, restoration not only benefited the macroalgal species but also probably lead to further consequences for other groups (i.e., invertebrates and fishes) (Figure 8). Nonetheless, it is important to consider that one process or function is not controlled by a single trait belonging to a specific trophic level (e.g., primary producers, herbivores or carnivores) but by some key traits related to various trophic levels, which are simultaneously involved in the control of multiple functions (de Bello et al., 2010; Pistón et al., 2019). Like the restored locality, both reference macroalgal forests were also characterised by a high variability in the different functional trait categories. A greater range of trait categories in these reference forests could increase the likelihood of some species responding differently to variable conditions and perturbations (e.g., extreme climatic events), which also contributes to assemblage stability and resilience and to the maintenance of long-term ecosystem functioning (Díaz & Cabido, 2001; Cadotte et al., 2011). Last, and of interest, is that the non-restored locality and one reference macroalgal locality (Reference 2) showed similar low diversity values through the application of taxonomical information, the reference macroalgal locality doubled the diversity results when using functional information, underlining the utility and complementarity of these metrics.
Predictable outcomes are a major goal of restoration ecology (Choi, 2007; Rey Benayas et al., 2009; Matthews & Spyreas, 2010), thus studies assessing ecosystem functioning through the lens of trait-based approaches can help to promote more generalizable outcomes (Suding et al., 2008). Even so, few studies have tested these ideas and, to our knowledge, they have never been applied in marine benthic ecosystems. We have demonstrated that if we are dealing with the structural species of an assemblage, a single restoring action can lead to the recovery of the associated biodiversity and, in turn, to the recovery of its functioning. Our study showed that the application of functional metrics together with traditional taxonomical indices provide valuable information for the understanding of macroalgal ecosystem functioning, and this approach has the potential to be extended and replicated in other restoration evaluations.
Data availability statement
The datasets presented in this study can be found in online repositories. The names of the repository/repositories and accession number(s) can be found in the article/Supplementary Material. The R code used to perform all the statistical analyses can be found in Git Hub: https://github.com/cgalobart/Macroalgal-restoration-functional-traits.
Author contributions
EC conceived the ideas; CG and EC collected the data; EB and CG identified the species and defined the traits; CG, RG and EC performed the data analyses; CG and EC drafted the manuscript. All authors contributed to the article and approved the submitted version.
Funding
This research was supported by the Sustainable Blue Economy program (European Union) under the grant agreement – AFRIMED - EASME/EMFF/2017/1.2.1.12/S4/01/SI2.789059, the Spanish Ministry of Science and Innovation under the grant FORESTA - N° PID2020-112985GB-I00, funded by MCIN/AEI/10.13039/501100011033 (European Union), and under the grant FoRescue – N° PCI2022-135070-2 funded by Biodiversa+ (European Biodiversity Partnership). CG was funded by Fundación Tatiana Pérez de Guzmán el Bueno predoctoral fellowship (Medioambiente 2019). RG was supported by an FPI grant (ANIMA – BES-2017-079907).
Acknowledgments
Authors acknowledge the D.G. d’Espais Naturals i Biodiversitat, the D.G. de Pesca i Medi Marí, and the Servei en Recerca i Desenvolupament del Govern de les Illes Balears for providing licenses, and the Jaume Ferrer Marine Station (IEO) for technical and facility support. We specially thank Dra. Maria Elena Cefalì for her support during sample collection. CG, RG, EC are members of the MedRecover Research Group (www.medrecover.org, 2017 SGR 1521) from the Generalitat de Catalunya.
Conflict of interest
The authors declare that the research was conducted in the absence of any commercial or financial relationships that could be construed as a potential conflict of interest.
Publisher’s note
All claims expressed in this article are solely those of the authors and do not necessarily represent those of their affiliated organizations, or those of the publisher, the editors and the reviewers. Any product that may be evaluated in this article, or claim that may be made by its manufacturer, is not guaranteed or endorsed by the publisher.
Supplementary material
The Supplementary Material for this article can be found online at: https://www.frontiersin.org/articles/10.3389/fmars.2023.1176655/full#supplementary-material
References
Anderson M. J. (2017). Permutational multivariate analysis of variance (PERMANOVA). Wiley StatsRef: Statistics Reference Online, 1–15. doi: 10.1002/9781118445112.stat07841
Aronson J., Alexander S. (2013). Ecosystem restoration is now a global priority: time to roll up our sleeves. Restor. Ecol. 21 (3), 293–296. doi: 10.1111/rec.12011
Ballesteros E. (1986). Métodos de análisis estructural en comunidades naturales, en particular del fitobentos. Oecologia Aquatica 8, 117–131.
Ballesteros E. (1992). “Els vegetals i la zonació litoral: especies, comunitats i factors que influeixen en la seva distribució,” in Arxius secció ciències, 101. (Barcelona, SpAain: Institut d’Estudis Catalans), 616. doi: 10.1017/CBO9781107415324.004
Ballesteros E., Garrabou J., Hereu B., Zabala M., Cebrian E., Sala E. (2009). Deep-water stands of Cystoseira zosteroides c. agardh (Fucales, ochrophyta) in the northwestern Mediterranean: insights into assemblage structure and population dynamics. Estuarine Coast. Shelf Sci. 82 (3), 477–484. doi: 10.1016/j.ecss.2009.02.013
Ballesteros E., Hereu B., Zabala M., Alcoverro T., Garrabou J., Sala E. (2002). Rapport mission scandola: cystoseira 2000. Travaux Scientifiques du Parc Naturel Régional la Corse 60, 95–115.
Bayraktarov E., Saunders M. I., Abdullah S., Mills M., Beher J., Possingham H. P., et al. (2016). The cost and feasibility of marine coastal restoration. Ecol. Appl. 26 (4), 1055–1074. doi: 10.1890/15-1077.1
Bernhardt-Römermann M., Baeten L., Craven D., De Frenne P., Hédl R., Lenoir J., et al. (2015). Drivers of temporal changes in temperate forest plant diversity vary across spatial scales. Global Change Biol. 21 (10), 3726–3737. doi: 10.1111/gcb.12993
Blignaut J., Esler K. J., de Wit M. P., Le Maitre D., Milton S. J., Aronson J. (2013). Establishing the links between economic development and the restoration of natural capital. Curr. Opin. Environ. Sustainability 5 (1), 94–101. doi: 10.1016/j.cosust.2012.12.003
Borja Á., Dauer D. M., Elliott M., Simenstad C. A. (2010). Medium- and long-term recovery of estuarine and coastal ecosystems: patterns, rates and restoration effectiveness. Estuaries Coasts 33 (6), 1249–1260. doi: 10.1007/s12237-010-9347-5
Boudouresque C. F., Belsher T. (1979). Le peuplement algal du port de port-vendres: recherches sur l’aire minimale qualitative. Cahiers Biologie Mar. 20, 259–269.
Brudvig L. A. (2011). The restoration of biodiversity: where has research been and where does it need to go? Am. J. Bot. 98 (3), 549–558. doi: 10.3732/ajb.1000285
Butchart S. H. M., Walpole M., Collen B., van Strien A., Scharlemann J. P. W., Almond R. E. A., et al. (2010). Global biodiversity: indicators of recent declines. Science 328 (5982), 1164–1168. doi: 10.1126/science.1187512
Cadotte M. W., Carscadden K., Mirotchnick N. (2011). Beyond species: functional diversity and the maintenance of ecological processes and services. J. Appl. Ecol. 48 (5), 1079–1087. doi: 10.1111/j.1365-2664.2011.02048.x
Capdevila P., Hereu B., Riera J. L., Linares C. (2016). Unravelling the natural dynamics and resilience patterns of underwater Mediterranean forests: insights from the demography of the brown alga Cystoseira zosteroides. J. Ecol. 104 (6), 1799–1808. doi: 10.1111/1365-2745.12625
Cardinale B. J., Duffy J. E., Gonzalez A., Hooper D. U., Perrings C., Venail P., et al. (2012). Biodiversity loss and its impact on humanity. Nature 486 (7401), 59–67. doi: 10.1038/nature11148
Carlucci M. B., Brancalion P. H. S., Rodrigues R. R., Loyola R., Cianciaruso M. V. (2020). Functional traits and ecosystem services in ecological restoration. Restor. Ecol. 28 (6), 1372–1383. doi: 10.1111/rec.13279
Ceballos G., Ehrlich P. R., Barnosky A. D., García A., Pringle R. M., Palmer T. M. (2015). Accelerated modern human-induced species losses: entering the sixth mass extinction. Sci. Adv. 1 (5), 9–13. doi: 10.1126/sciadv.1400253
Cebrian E., Tamburello L., Verdura J., Guarnieri G., Medrano A., Linares C., et al. (2021). A roadmap for the restoration of Mediterranean macroalgal forests. Front. Mar. Sci. 8. doi: 10.3389/fmars.2021.709219
Choi Y. D. (2007). Restoration ecology to the future: a call for new paradigm. Restor. Ecol. 15 (2), 351–353. doi: 10.1111/j.1526-100X.2007.00224.x
Choi Y. D., Temperton V. M., Allen E. B., Grootjans A. P., Halassy M., Hobbs R. J., et al. (2008). Ecological restoration for future sustainability in a changing environment. Ecoscience 15 (1), 53–64. doi: 10.2980/1195-6860(2008)15[53:ERFFSI]2.0.CO;2
Cinelli F., Fresi E., Idato E., Mazzella L. (1977). L'aire minima du phytobenthos dans un peuplement à Cystoseira mediterranea de l'île d'Ischia (Golfe de Naples). Rapport la Comission Internationale pour l’Exploration Scientifique la Mer Méditerranée 24 (4), 113–115.
Coppejans E. (1980). “Phytosociological studies on Mediterranean algal vegetation: rocky surfaces of the photophilic infralittoral zone,” in The shore environment: ecosystems. Systematic association special volume (London: Academic Press), vol. 2., 371–393.
Crouzeilles R., Curran M., Ferreira M. S., Lindenmayer D. B., Grelle C. E. V., Rey Benayas J. M. (2016). A global meta-analysis on the ecological drivers of forest restoration success. Nat. Commun. 7, 11666. doi: 10.1038/ncomms11666
da Costa N., Arnan X., de Paiva Farias R., Leão Barros I. C. (2019). Community responses to fine-scale enviromental conditions: ferns alpha and beta diversity along Brazilian Atlantic forests remnants. Acta Oecologica 101, 103475. doi: 10.1016/j.actao.2019.103475
de Bello F., Lavorel S., Díaz S., Harrington R., Cornelissen J. H. C., Bardgett R. D., et al. (2010). Towards an assessment of multiple ecosystem processes and services via functional traits. Biodiver. Conserv. 19 (10), 2873–2893. doi: 10.1007/s10531-010-9850-9
Dhondt F., Coppejans E. (1977). Résultats d'une étude d'aire minima de peuplements algaux photophiles sur substrat rocheux à port-cros et à banyuls (France). Rapports Procès-Verbaux Des. Réunions la Commission Internationale pour l'Exploration Scientifique la Mer Méditerranée 24 (4), 141–142.
Díaz S., Cabido M. (2001). Vive la différence: plant functional diversity matters to ecosystem processes. Trends Ecol. Evol. 16 (11), 646–655. doi: 10.1016/S0169-5347(01)02283-2
Díaz S., Purvis A., Cornelissen J. H. C., Mace G. M., Donoghue M. J., Ewers R. M., et al. (2013). Functional traits, the phylogeny of function, and ecosystem service vulnerability. Ecol. Evol. 3 (9), 2958–2975. doi: 10.1002/ece3.601
Dobson A. P., Bradshaw A. D., Baker A. J. (1997). Hopes for the future: restoration ecology and conservation biology. Science 277 (5325), 515–522. doi: 10.1126/science.277.5325.515
Dobson A., Lodge D., Alder J., Cumming G. S., Keymer J., McGlade J., et al. (2006). Habitat loss, trophic collapse, and the decline of ecosystem services. Ecology 87 (8), 1915–1924. doi: 10.1890/0012-9658(2006)87[1915:HLTCAT]2.0.CO;2
Eger A. M., Marzinelli E. M., Christie H., Fagerli C. W., Fujita D., Gonzalez A. P., et al. (2022). Global kelp forest restoration: past lessons, present status, and future directions. Biol. Rev. 97 (4), 1449–1475. doi: 10.1111/brv.12850
Elliott M., Burdon D., Hemingway K. L., Apitz S. E. (2007). Estuarine, coastal and marine ecosystem restoration: confusing management and science - a revision of concepts. Estuarine Coast. Shelf Sci. 74 (3), 349–366. doi: 10.1016/j.ecss.2007.05.034
Ellis E. C., Goldewijk K. K., Siebert S., Lightman D., Ramankutty N. (2010). Anthropogenic transformation of the biomes 1700 to 2000. Global Ecol. Biogeogr. 19 (5), 589–606. doi: 10.1111/j.1466-8238.2010.00540.x
Engst K., Baasch A., Erfmeier A., Jandt U., May K., Schmiede R., et al. (2016). Functional community ecology meets restoration ecology: assessing the restoration success of alluvial floodplain meadows with functional traits. J. Appl. Ecol. 53 (3), 751–764. doi: 10.1111/1365-2664.12623
Foley J. A., DeFries R., Asner G. P., Barford C., Bonan G., Carpenter S. R., et al. (2005). Global consequences of land use. Science 309 (5734), 570–574. doi: 10.1126/science.1111772
Fredriksen S., Filbee-Dexter K., Norderhaug K. M., Steen H., Bodvin T., Coleman M. A., et al. (2020). Green gravel: a novel restoration tool to combat kelp forest decline. Sci. Rep. 10, 3983. doi: 10.1038/s41598-020-60553-x
Fulton C. J., Abesamis R. A., Berkström C., Depczynski M., Graham N. A. J., Holmes T. H., et al. (2019). Form and function of tropical macroalgal reefs in the anthropocene. Funct. Ecol. 33 (6), 989–999. doi: 10.1111/1365-2435.13282
Gann G. D., McDonald T., Walder B., Aronson J., Nelson C. R., Jonson J., et al. (2019). International principles and standards for the practice of ecological restoration. second edition. Restor. Ecol. 27 (S1), S1–S46. doi: 10.1111/rec.13035
Garnier E., Cortez J., Billès G., Navas M. L., Roumet C., Debussche M., et al. (2004). Plant functional markers capture ecosystem properties during secondary succession. Ecology 85 (9), 2630–2637. doi: 10.1890/03-0799
Gatica-Saavedra P., Echeverría C., Nelson C. R. (2017). Ecological indicators for assessing ecological success of forest restoration: a world review. Restor. Ecol. 25 (6), 850–857. doi: 10.1111/rec.12586
Geist J., Hawkins S. J. (2016). Habitat recovery and restoration in aquatic ecosystems: current progress and future challenges. Aquat. Conservation: Mar. Freshw. Ecosyst. 26 (5), 942–962. doi: 10.1002/aqc.2702
Gómez-Aparicio L. (2009). The role of plant interactions in the restoration of degraded ecosystems: a meta-analysis across life-forms and ecosystems. J. Ecol. 97 (6), 1202–1214. doi: 10.1111/j.1365-2745.2009.01573.x
Gran A., Movilla J. I., Ballesteros E., Sales M., Bolado I., Galobart C., et al. (2022). Assessing the expansion and success of a restored population of Gongolaria barbata (Stackhouse) kuntze (Fucales, phaeophyceae) using high-precision positioning tools and size distribution frequencies. Mediterr. Mar. Sci. 23 (4), 907–916. doi: 10.12681/mms.30500
Higgs E., Falk D. A., Guerrini A., Hall M., Harris J., Hobbs R. J., et al. (2014). The changing role of history in restoration ecology. Front. Ecol. Environ. 12 (9), 499–506. doi: 10.1890/110267
Holl K. D., Aide T. M. (2011). When and where to actively restore ecosystems? For. Ecol. Manage. 261 (10), 1558–1563. doi: 10.1016/j.foreco.2010.07.004
Hughes A. R., Grabowski J. H., Leslie H. M., Scyphers S., Williams S. L. (2017). Inclusion of biodiversity in habitat restoration policy to facilitate ecosystem recovery. Conserv. Lett. 11 (3), 1–8. doi: 10.1111/conl.12419
Jackson S. T., Hobbs R. J. (2009). Ecological restoration in the light of ecological history. Science 325 (5940), 567–569. doi: 10.1126/science.1172977
Jacob C., Buffard A., Pioch S., Thorin S. (2018). Marine ecosystem restoration and biodiversity offset. Ecol. Eng. 120, 585–594. doi: 10.1016/j.ecoleng.2017.09.007
Jones H. P., Schmitz O. J. (2009). Rapid recovery of damaged ecosystems. PloS One 4 (5), e5653. doi: 10.1371/journal.pone.0005653
Kollmann J., Meyer S. T., Bateman R., Conradi T., Gossner M. M., de Souza Mendonça M., et al. (2016). Integrating ecosystem functions into restoration ecology–recent advances and future directions. Restor. Ecol. 24 (6), 722–730. doi: 10.1111/rec.12422
Laughlin D. C. (2014). Applying trait-based models to achieve functional targets for theory-driven ecological restoration. Ecol. Lett. 17 (7), 771–784. doi: 10.1111/ele.12288
Layton C., Coleman M. A., Marzinelli E. M., Steinberg P. D., Swearer S. E., Vergés A., et al. (2020). Kelp forest restoration in Australia. Front. Mar. Sci. 7. doi: 10.3389/fmars.2020.00074
Ling S. D. (2008). Range expansion of a habitat-modifying species leads to loss of taxonomic diversity: a new and impoverished reef state. Oecologia 156 (4), 883–894. doi: 10.1007/s00442-008-1043-9
Maire E., Grenouillet G., Brosse S., Villéger S. (2015). How many dimensions are needed to accurately assess functional diversity? a pragmatic approach for assessing the quality of functional spaces. Global Ecol. Biogeogr. 24 (6), 728–740. doi: 10.1111/geb.12299
Mangialajo L., Chiantore M., Cattaneo-Vietti R. (2008). Loss of fucoid algae along a gradient of urbanisation, and structure of benthic assemblages. Mar. Ecol. Prog. Ser. 358, 63–74. doi: 10.3354/meps07400
Mariani S., Cefalì M. E., Chappuis E., Terradas M., Pinedo S., Torras X., et al. (2019). Past and present of fucales from shallow and sheltered shores in Catalonia. Regional Stud. Mar. Sci. 32, 100824. doi: 10.1016/j.rsma.2019.100824
Martin D. M. (2017). Ecological restoration should be redefined for the twenty-first century. Restor. Ecol. 25 (5), 668–673. doi: 10.1111/rec.12554
Martin P. A., Newton A. C., Bullock J. M. (2013). Carbon pools recover more quickly than plant biodiversity in tropical secondary forests. Proc. R. Soc. B: Biol. Sci. 280, 20132236. doi: 10.1098/rspb.2013.2236
Marzinelli E. M., Leong M. R., Campbell A. H., Steinberg P. D., Vergés A. (2016). Does restoration of a habitat-forming seaweed restore associated faunal diversity? Restor. Ecol. 24 (1), 81–90. doi: 10.1111/rec.12292
Matthews J. W., Peralta A. L., Flanagan D. N., Baldwin P. M., Soni A., Kent A. D., et al. (2009). Relative influence of landscape vs. local factors on plant community assembly in restored wetlands. Ecol. Appl. 19 (8), 2108–2123. doi: 10.1890/08-1836.1
Matthews J. W., Spyreas G. (2010). Convergence and divergence in plant community trajectories as a framework for monitoring wetland restoration progress. J. Appl. Ecol. 47 (5), 1128–1136. doi: 10.1111/j.1365-2664.2010.01862.x
McGill B. J., Enquist B. J., Weiher E., Westoby M. (2006). Rebuilding community ecology from functional traits. Trends Ecol. Evol. 21 (4), 178–185. doi: 10.1016/j.tree.2006.02.002
Molinari E. A., Guiry M. D. (2020). Reinstatement of the genera Gongolaria boehmer and Ericaria stackhouse (Sargassaceae, phaeophyceae). Notulae Algarum 172, 1–10.
Montoya D., Rogers L., Memmott J. (2012). Emerging perspectives in the restoration of biodiversity-based ecosystem services. Trends Ecol. Evol. 27 (12), 666–672. doi: 10.1016/j.tree.2012.07.004
Moreno-Mateos D., Power M. E., Comín F. A., Yockteng R. (2012). Structural and functional loss in restored wetland ecosystems. PloS Biol. 10 (1), e1001247. doi: 10.1371/journal.pbio.1001247
Mouillot D., Graham N. A. J., Villéger S., Mason N. W. H., Bellwood D. R. (2013). A functional approach reveals community responses to disturbances. Trends Ecol. Evol. 28 (3), 167–177. doi: 10.1016/j.tree.2012.10.004
Ørberg S. B., Krause-Jensen D., Mouritsen K. N., Olesen B., Marbà N., Larsen M. H., et al. (2018). Canopy-forming macroalgae facilitate recolonization of sub-Arctic intertidal fauna and reduce temperature extremes. Front. Mar. Sci. 5. doi: 10.3389/fmars.2018.00332
Orlando-Bonaca M., Rotter A. (2018). Any signs of replacement of canopy-forming algae by turf-forming algae in the northern Adriatic Sea? Ecol. Indic. 87, 272–284. doi: 10.1016/j.ecolind.2017.12.059
Piazzi L., Bonaviri C., Castelli A., Ceccherelli G., Costa G., Curini-Galletti M., et al. (2018). Biodiversity in canopy-forming algae: structure and spatial variability of the Mediterranean Cystoseira assemblages. Estuarine Coast. Shelf Sci. 207, 132–141. doi: 10.1016/j.ecss.2018.04.001
Piqueray J., Ferroni L., Delescaille L. M., Speranza M., Mahy G., Poschlod P. (2015). Response of plant functional traits during the restoration of calcareous grasslands from forest stands. Ecol. Indic. 48, 408–416. doi: 10.1016/j.ecolind.2014.08.039
Pistón N., de Bello F., Dias A. T. C., Götzenberger L., Rosado B. H. P., de Mattos E. A., et al. (2019). Multidimensional ecological analyses demonstrate how interactions between functional traits shape fitness and life history strategies. J. Ecol. 107 (5), 2317–2328. doi: 10.1111/1365-2745.13190
Podani J., Schmera D. (2006). On dendrogram-based measures of functional diversity. Oikos 115 (1), 179–185. doi: 10.1111/j.2006.0030-1299.15048.x
Prach K., Durigan G., Fennessy S., Overbeck G. E., Torezan J. M., Murphy S. D. (2019). A primer on choosing goals and indicators to evaluate ecological restoration success. Restor. Ecol. 27 (5), 917–923. doi: 10.1111/rec.13011
R Development Core Team (2018) R: a language and environment for statistial computing. Available at: https://www.r-project.org/.
Reich P. B. (2014). The world-wide “fast-slow” plant economics spectrum: a traits manifesto. J. Ecol. 102 (2), 275–301. doi: 10.1111/1365-2745.12211
Rey Benayas J. M., Newton A. C., Diaz A., Bullock J. M. (2009). Enhancement of biodiversity and ecosystem services by ecological restoration: a meta-analysis. Science 325 (5944), 1121–1124. doi: 10.1126/science.1172460
Ruiz-Jaen M. C., Aide T. M. (2005). Restoration success: how is it being measured? Restor. Ecol. 13 (3), 569–577. doi: 10.1111/j.1526-100X.2005.00072.x
Sales M., Ballesteros E. (2009). Shallow Cystoseira (Fucales: ochrophyta) assemblages thriving in sheltered areas from menorca (NW mediterranean): relationships with environmental factors and anthropogenic pressures. Estuarine Coast. Shelf Sci. 84 (4), 476–482. doi: 10.1016/j.ecss.2009.07.013
Sales M., Cebrian E., Tomas F., Ballesteros E. (2011). Pollution impacts and recovery potential in three species of the genus Cystoseira (Fucales, heterokontophyta). Estuarine Coast. Shelf Sci. 92 (3), 347–357. doi: 10.1016/j.ecss.2011.01.008
Sanderson E. W., Jaiteh M., Levy M. A., Redford K. H., Wannebo A. V., Woolmer G. (2002). The human footprint and the last of the wild. BioScience 52 (10), 891–904. doi: 10.1641/0006-3568(2002)052[0891:THFATL]2.0.CO;2
Sant N., Chappuis E., Rodríguez-Prieto C., Real M., Ballesteros E. (2017). Cost-benefit of three different methods to study Mediterranean rocky assemblages. Scientia Marina 81 (1), 129–138. doi: 10.3989/scimar.04463.04A
Saunders M. I., Doropoulos C., Bayraktarov E., Babcock R. C., Gorman D., Eger A. M., et al. (2020). Bright spots in coastal marine ecosystem restoration. Curr. Biol. 30 (24), R1500–R1510. doi: 10.1016/j.cub.2020.10.056
Schiel D. R., Foster M. S. (2006). The population biology of large brown seaweeds: ecological consequences of multiphase life histories in dynamic coastal environments. Annu. Rev. Ecol. Evolution Systematics 37, 343–372. doi: 10.1146/annurev.ecolsys.37.091305.110251
Secretariat of the Convention on Biological Diversity (2014). Global biodiversity outlook 4 (Montréal).
Sedano F., Florido M., Rallis I., Espinosa F., Gerovasileiou V. (2019). Comparing sessile benthos on shallow artificial versus natural hard substrates in the Eastern Mediterranean Sea. Mediterr. Mar. Sci. 20 (4), 688–702. doi: 10.12681/mms.17897
Simenstad C., Reed D., Ford M. (2006). When is restoration not? incorporating landscape-scale processes to restore self-sustaining ecosystems in coastal wetland restoration. Ecol. Eng. 26 (1), 27–39. doi: 10.1016/j.ecoleng.2005.09.007
Sluis W. J. (2002). Patterns of species richness and composition in re-created grassland. Restor. Ecol. 10 (4), 677–684. doi: 10.1046/j.1526-100X.2002.01048.x
Smale D. A., Burrows M. T., Moore P., O’Connor N., Hawkins S. J. (2013). Threats and knowledge gaps for ecosystem services provided by kelp forests: a northeast Atlantic perspective. Ecol. Evol. 3 (11), 4016–4038. doi: 10.1002/ece3.774
Steneck R. S., Graham M. H., Bourque B. J., Corbett D., Erlandson J. M., Estes J. A., et al. (2002). Kelp forest ecosystems: biodiversity, stability, resilience and future. Environ. Conserv. 29 (4), 436–459. doi: 10.1017/S0376892902000322
Suding K. N., Gross K. L. (2006). “The dynamic nature of ecological systems: multiple states and restoration trajectories,” in Foundations of restoration ecology. (Washington, DC, USA: Island Press). 190–209.
Suding K., Higgs E., Palmer M., Callicott J. B., Anderson C. B., Baker M., et al. (2015). Committing to ecological restoration. Science 348 (6235), 638–640. doi: 10.1126/science.aaa4216
Suding K., Lavorel S., Chapin F. S., Cornelissen J. H. C., Díaz S., Garnier E., et al. (2008). Scaling environmental change through the community-level: a trait-based response-and-effect framework for plants. Global Change Biol. 14 (5), 1125–1140. doi: 10.1111/j.1365-2486.2008.01557.x
Suganuma M. S., Assis G. B., Durigan G. (2014). Changes in plant species composition and functional traits along the successional trajectory of a restored patch of Atlantic forest. Community Ecol. 15 (1), 27–36. doi: 10.1556/ComEc.15.2014.1.3
Teixidó N., Gambi M. C., Parravacini V., Kroeker K., Micheli F., Villéger S., et al. (2018). Functional biodiversity loss along natural CO2 gradients. Nat. Commun. 9, 5149. doi: 10.1038/s41467-018-07592-1
Teixidó N., Garrabou J., Harmelin J.-G. (2011). Low dynamics, high longevity and persistence of sessile structural species dwelling on Mediterranean coralligenous outcrops. PloS One 6 (8), e23744. doi: 10.1371/journal.pone.0023744
Thibaut T., Blanfuné A., Boudouresque C. F., Verlaque M. (2015). Decline and local extinction of fucales in French Riviera: the harbinger of future extinctions? Mediterr. Mar. Sci. 16 (1), 206–224. doi: 10.12681/mms.1032
Thibaut T., Pinedo S., Torras X., Ballesteros E. (2005). Long-term decline of the populations of fucales (Cystoseira spp. and sargassum spp.) in the albères coast (France, north-western Mediterranean). Mar. pollut. Bull. 50 (12), 1472–1489. doi: 10.1016/j.marpolbul.2005.06.014
Verdura J., Sales M., Ballesteros E., Cefalì M. E., Cebrian E. (2018). Restoration of a canopy-forming alga based on recruitment enhancement: methods and long-term success assessment. Front. Plant Sci. 9. doi: 10.3389/fpls.2018.01832
Violle C., Navas M.-L., Vile D., Kazakou E., Fortunel C., Hummel I., et al. (2007). Let the concept of trait be functional! Oikos 116 (5), 882–892. doi: 10.1111/j.2007.0030-1299.15559.x
Whitaker S. G., Smith J. R., Murray S. N. (2010). Reestablishment of the southern California rocky intertidal brown alga, Silvetia compressa: an experimental investigation of techniques and abiotic and biotic factors that affect restoration success. Restor. Ecol. 18 (s1), 18–26. doi: 10.1111/j.1526-100X.2010.00717.x
Wortley L., Hero J. M., Howes M. (2013). Evaluating ecological restoration success: a review of the literature. Restor. Ecol. 21 (5), 537–543. doi: 10.1111/rec.12028
Keywords: marine restoration, ecosystem functioning, species diversity, functional diversity, functional traits, macroalgal forests
Citation: Galobart C, Ballesteros E, Golo R and Cebrian E (2023) Addressing marine restoration success: evidence of species and functional diversity recovery in a ten-year restored macroalgal forest. Front. Mar. Sci. 10:1176655. doi: 10.3389/fmars.2023.1176655
Received: 28 February 2023; Accepted: 02 May 2023;
Published: 02 June 2023.
Edited by:
Ronald Osinga, Wageningen University and Research, NetherlandsReviewed by:
Dan Smale, Marine Biological Association of the United Kingdom, United KingdomSarah Caronni, University of Milan Bicocca, Italy
Copyright © 2023 Galobart, Ballesteros, Golo and Cebrian. This is an open-access article distributed under the terms of the Creative Commons Attribution License (CC BY). The use, distribution or reproduction in other forums is permitted, provided the original author(s) and the copyright owner(s) are credited and that the original publication in this journal is cited, in accordance with accepted academic practice. No use, distribution or reproduction is permitted which does not comply with these terms.
*Correspondence: Emma Cebrian, ZW1tYUBjZWFiLmNzaWMuZXM=