- 1Dauphin Island Sea Lab, Dauphin Island, AL, United States
- 2School of Marine and Environmental Sciences, University of South Alabama, Mobile, AL, United States
- 3Division of Marine Science, School of Ocean Science and Engineering, University of Southern Mississippi, Stennis Space Center, MS, United States
- 4Department of Oceanography and Coastal Studies, Louisiana State University, Baton Rouge, LA, United States
In the northern Gulf of Mexico (nGoM), the Louisiana Shelf (LS) and Mississippi Bight (MB) subregions are influenced by eutrophication to varying degrees. Despite recognition that dissolved silicon may regulate diatom productivity in the nGoM, there is only one published data set reporting biogenic silica (bSiO2) production rates for each subregion. We report that bSiO2 production rates on the LS and MB are high and appear to be controlled by different nutrients among seasons. Despite exceptional upper trophic level biomass regionally, which suggests significant primary production by diatoms (as in other systems), gross euphotic-zone integrated bSiO2 production rates are lower than major bSiO2 producing regions (e.g. upwelling systems). However, when normalizing to the depth of the euphotic zone, the bSiO2 production rates on the LS are like normalized rates in upwelling systems. We suggest local river-plume influenced hydrography concentrates diatom productivity within shallow euphotic zones, making production more accessible to higher trophic organisms. Comparison of rates between the LS and MB suggest that the fluvial nitrate within the LS stimulates bSiO2 production above that in the MB, which has a smaller watershed and is less eutrophic (relatively). Beyond understanding the factors controlling regional bSiO2 production, these data offer the most comprehensive Si-cycle baseline to date as the LS and MB will likely exchange freely in the mid to late century due to land subsidence of the Mississippi River delta and/or sea-level rise.
Introduction
Diatoms play important roles in coastal biogeochemistry and often dominate the phytoplankton biomass and bloom productivity in these environments. Diatoms are unique in their obligate requirement for silicon, which is required in near unity to nitrogen under the many growth conditions among low- to high-latitude ecosystems (Brzezinski, 1985; Lomas et al., 2019). Widespread use of agricultural fertilizer has increased fluvial nitrogen loading in many watersheds and in some cases has resulted in a doubling of riverine nitrogen input to the coastal ocean (Cloern, 2001). In many eutrophied rivers for nitrogen, dissolved silicon (Si(OH)4) has declined or remained stable, thereby lowering the Si:N ratio below diatoms’ optimum (i.e. <1.0) potentially favoring diatoms blooms to deplete Si(OH)4 before NO3. The decline in Si(OH)4 availability relative to nitrate and phosphate has been linked to shifts from diatom-dominated to flagellate-dominated ecosystems; this so called ‘Si(OH)4 Paradigm’ (Ragueneau et al., 2006) was first proposed for coastal systems by Officer and Ryther (1980) and has been evoked as a potential mechanism for changes in many systems (reviewed by Cloern (2001)). However, the linkage among significant Si:N perturbations and the changing role of diatoms is built largely on standing stock data (e.g. nutrients, phytoplankton abundance), rather than physiological rate data, as the latter data are absent in many systems. Furthermore, testing for an Si:N effect in these systems is challenging given the lack of baseline information prior to the nutrient perturbation.
In the Mississippi River (MR), which discharges onto the Louisiana Shelf (LS) in the northern Gulf of Mexico (nGoM), the annual average Si:N ratio declined from >3 in 1960 to ~1 in 1980. The change was driven by both increasing nitrate and decreasing Si(OH)4 concentrations and has remained around the latter Si:N value to present day (Figure 1). The MR now exhibits strong seasonal oscillations between favorable (>1.0, late summer/autumn) and unfavorable (<1.0, spring) Si:N conditions for diatoms (Figure 1C). This unfavorable period corresponds to the annual peak in MR discharge and elevated primary production rates on the LS from nitrate loading (Lohrenz et al., 1997). The changes in the MR-derived nutrients in the late 20th century coincided with major ecosystem-wide changes in the nGoM, e.g. frequent and intense hypoxia events (Rabalais et al., 2007). On the LS, multiple species from the pennate diatom genera Pseudo-nitzschia numerically dominate the diatom assemblage during periods of high MR flow (Bargu et al., 2016). These results, combined with increasing Pseudo-nitzschia preserved in regional sediments during the late 20th century (Parsons et al., 2002) and the rise of more flagellate harmful algal species on the LS (Dortch et al., 1999), have led to the hypothesis that the Si:N changes in the MR watershed may have reduced the diatom contribution to food web processes in this system (Turner et al., 1998).
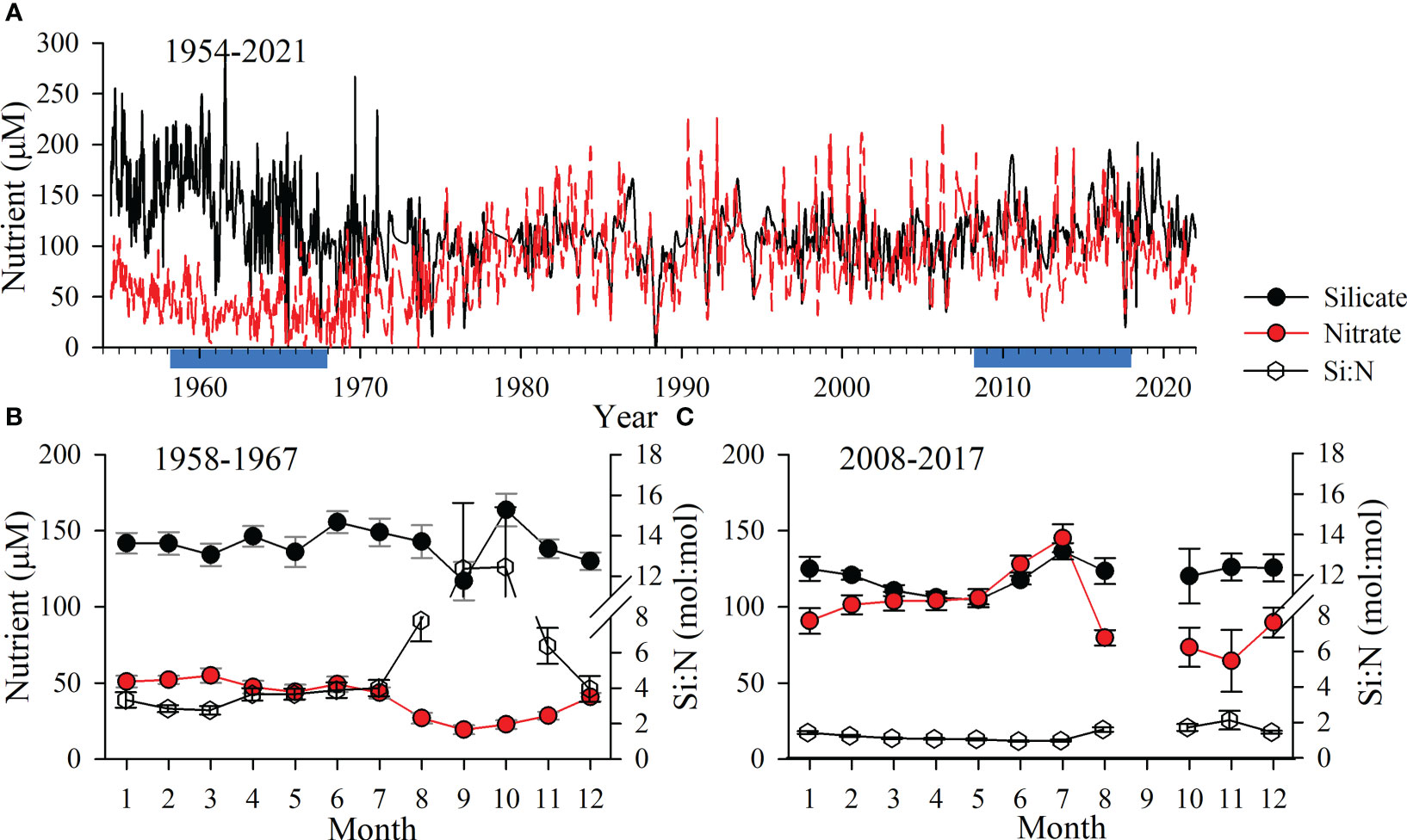
Figure 1 (A) Time series of Mississippi River silicate and nitrate at the United States Geological Survey Station 07373420 in St. Francisville, Louisiana, United States, from August 1954 through December 2021. (B, C) Comparison of the monthly average ( ± standard error) for each nutrient (left scale) and the silicate:nitrate (Si:N) molar ratio (right scale) among 10-year subsets (blue highlights in A) separated by 50 years: 1958 through 1967 (B) and 2008 through 2017 (C).
Biogenic silica (bSiO2) production rates can serve as a proxy for diatom growth as silicon is used to produce their bSiO2 shell. While recent reports have shown the importance of cyanobacteria and Rhizaria in bSiO2 cycling, e.g. (Baines et al., 2012; Biard et al., 2018), diatom biomass is significantly higher on the LS than these other groups. The only published bSiO2 production data in this area was acquired on the LS in the early 1990s (Nelson and Dortch, 1996), approximately a decade after the major MR watershed shift in nutrients stabilized, and this prior work only examined processes (e.g. kinetics of Si(OH)4 uptake by diatoms) at a single depth. There are currently no available euphotic-zone profile data to determine whether diatom Si(OH)4 consumption on the shelf may exceed the delivery of Si(OH)4 from the MR or benthic flux sources, which (if found) would support the hypothesis that Si may exert a strong control over regional diatom productivity, e.g. Dale et al. (2007).
The Mississippi Bight (MB) is located to the east of the LS shelf and MR delta extending to Perdido Bay, Florida (Figure 2). The MB is a river-dominated system fed from the combined rivers of Mobile Bay (Alabama). Analysis of water oxygen isotopic signatures show that MR water did not dominate the freshwater loading to the MB during multiple years in mid 2010s, instead this was driven largely by the combined rivers from the northern domain, especially those discharging through Mobile Bay (Sanial et al., 2019). Mobile Bay has the fourth highest freshwater discharge rate in the United States (Stumpf et al., 1993) but nitrate concentrations rarely exceed 20 µM (Pennock et al., 1999). The MB is also highly productive, being the central habitat range for a variety of economically important fish and invertebrate fauna and is the eastern extension of the so called ‘Fertile Fisheries Crescent’ where, historically, ~70-80% of fisheries landings in the entire GoM occur (Gunter, 1963). Comparison of diatom processes within the water column of the LS and MB allows an assessment of similarities, i.e. both are river plume systems where the main distributaries are separated by ~200 km, and differences occurring due to the watershed sizes and the degree of nutrient loading and/or eutrophication.
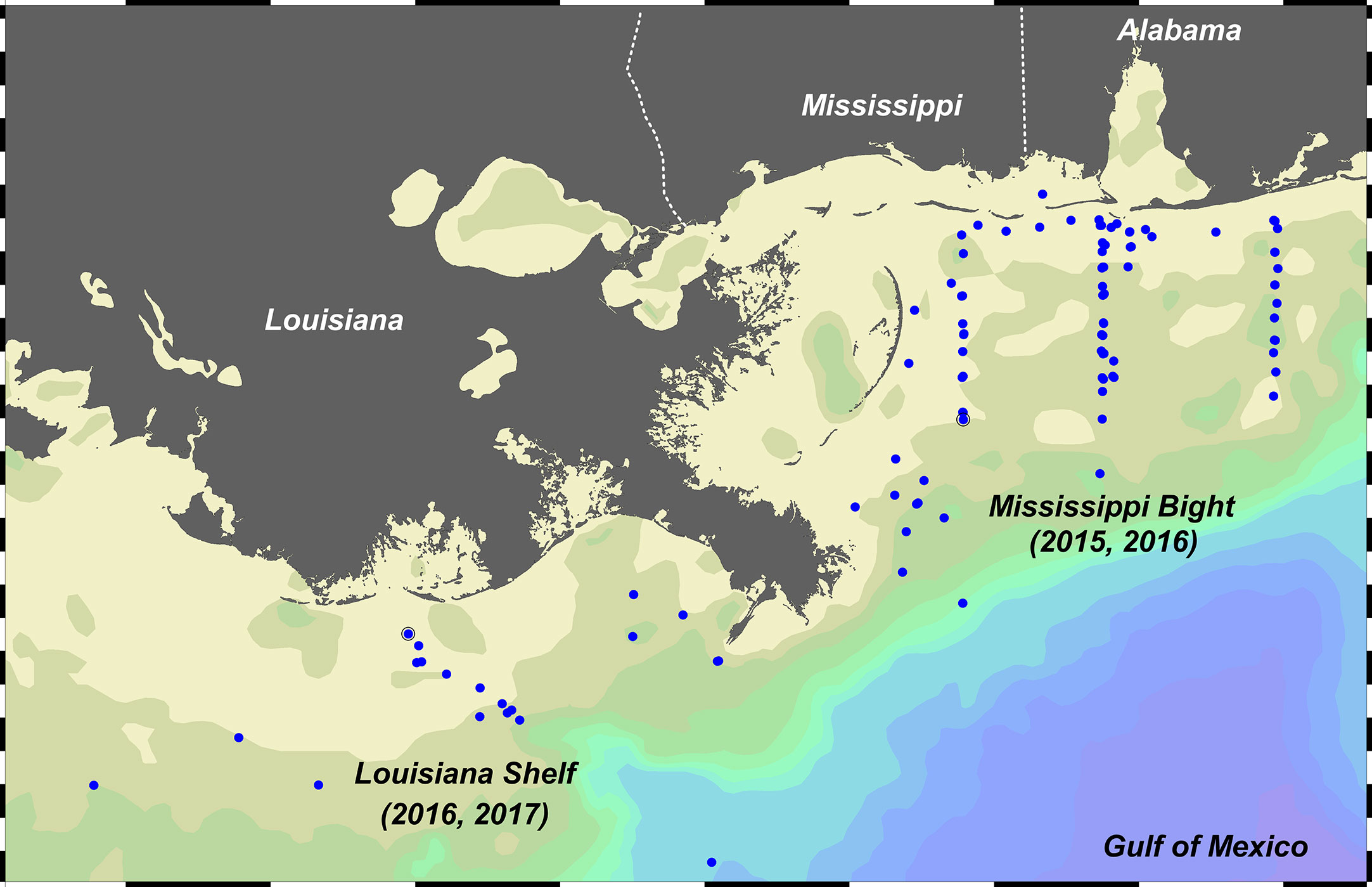
Figure 2 Station map for four cruises in the Louisiana Shelf (LS) and Mississippi Bight (MB). LS cruises were part of the Coastal Louisiana Silicon Cycling (CLASiC) project in August-September 2016 and May 2017 (Pickering et al., 2020, Ghaisas et al., 2021); MB cruises were part of the Consortium for Oil Spill Exposure Pathways in Coastal River-Dominated Ecosystems (CONCORDE) project in October-November 2015 and March-April 2016 (Dzwonkowski et al., 2017; Sanial et al., 2019). Map made using Ocean Data View (Schlitzer, 2016).
Hydrographic processes in both the MB and LS have significantly changed in the last century. Since the 1950s, the river discharge increased 31% and 15% in the MR and Mobile Bay river system, respectively (Milliman et al., 2008), and this trend is expected to increase in the 21st century (Tao et al., 2014). Land subsidence rates in southeastern Louisiana are rapid (Kolker et al., 2011) and there will be pelagic connectivity between the MB and LS domains as the MR delta becomes submerged. Thus, baseline data on diatom biogeochemical processes are required to ground truth models attempting to predict how the MB and LS pelagic ecosystems will alter as their connectivity increases.
The overarching goal of this study was to evaluate whether bSiO2 cycling rates in the LS have significantly changed in the last 30 years and test the hypothesis that Si(OH)4 availability controls diatom productivity. Here, we evaluated seasonal variability between systems and establish baseline rates and stocks. The nGoM is a model region for river-dominated systems which have undergone significant anthropogenic perturbations. The bSiO2 cycling processes in this region may be relevant to many low-latitude river-dominated regions that have undergone, or are presently experiencing, similar stressors.
Methods
Four cruises in the LS and MB were completed as part of the Coastal Louisiana Silicon Cycle (CLASiC) and Consortium for Coastal River-Dominated Ecosystems (CONCORDE) projects, respectively. These programs were independent and had different goals (Greer et al., 2018; Pickering et al., 2020), but similar silicon-cycling rate and ancillary measurements were obtained. The CLASiC cruises were conducted aboard the R/V Pelican during summer (27 August – 6 September 2016) and spring (4 – 13 May 2017), corresponding to the low and high-flow periods of the MR discharge onto the LS, respectively. The two CONCORDE cruises were conducted aboard the R/V Point Sur during autumn (28 October – 7 November 2015) and spring (29 March – 11 April 2016), where the spring cruise corresponded to the annual maximum discharge onto the MB from the Mobile Bay riverine complex and other local rivers.
Water column stations were sampled in salinity zones (CLASiC) and geographic corridors (CONCORDE). Given the dominance of the MR in surface hydrography on the LS, CLASiC cruise stations were targeted to river-influence zones operationally defined by a strong (salinity <25), moderate (salinity 25 – 30), or low riverine signal (salinity >30) in surface salinity. CONCORDE stations were aligned primarily among meridional transects in the west, middle, and east of the MB, with the autumn cruise sampling also in the southern zone near the eastward distributary of the MR and the spring cruise had some stations parallel to the barrier island coast of Alabama in the northern domain. All water column sampling was done using a 12-bottle rosette sampler equipped with 12 L Niskin bottles and a SeaBird SBE911 CTD with a Biospherical photosynthetically active radiation sensor and WET Labs C-Star transmissometer (instruments on both vessels). For CLASiC stations, CTD sampling was conducted ~1 hour after sunrise or in the late afternoon/early evening <2 hours prior to sunset. Samples were either selected at pre-determined depths (standing stocks) or specified light levels (for incubations) normalized to the irradiance available just below the surface (i.e. I0). Specific light levels included 100% (just below surface), 50%, 20%, 5%, and 1% I0. During most CONCORDE stations, sampling was done throughout the night and only surface samples were taken for incubation (i.e. no light depths). Select vertical profiles were conducted, and these hydrocasts were taken in the morning to target the defined light depths as during CLASiC. As in prior studies, e.g. (Lomas et al., 2009; Krause et al., 2021), Niskin samples were pooled into 10 L carboys (covered with opaque bags) and then subsampled for various measurements; the carboy was gently mixed during sampling to keep the contents in suspension.
Subsamples were collected for nutrients and particulate matter. 50 mL of seawater was immediately filtered using a 0.2 µm polycarbonate filter (CLASiC) or ~0.6 µm glass-fiber filter (CONCORDE) for nutrient analyses. Analyses were done as described previously (Dzwonkowski et al., 2017; Pickering et al., 2020) but are briefly discussed here. For Si(OH)4, samples were analyzed using a manual colorimetric method at sea (CLASiC) or on frozen samples (CONCORDE), which were thawed at room temperature for sufficient time to allow Si polymers to break down prior to colorimetric analysis. Filtrate for all other nutrients was immediately frozen. Dissolved inorganic nitrogen (nitrate + nitrite, NO3+NO2; ammonium, NH4) and dissolved soluble reactive phosphorus (SRP) were analyzed using a Skalar autoanalyzer. During CONCORDE, ~500 mL samples were filtered onto 1.2 µm polycarbonate filters for bSiO2 and frozen. In the laboratory, samples were dried and run using a sodium hydroxide digestion (Krause et al., 2009). Samples were collected and stored similarly during CLASiC. However, given the quantity of lithogenic silica in the waters, time-course digestions in sodium carbonate were used. This method is like those used for quantifying bSiO2 in sediments, e.g. Pickering et al. (2020), and can better constrain uncertainty among individual bSiO2 and lithogenic sample pools. Although it is less sensitive, there is sufficient bSiO2 in this system such that detection and quantitation are not issues. Other studies in coastal systems with high and variable lithogenic silica have used modified sodium hydroxide digestions, e.g. Ragueneau et al. (2005), however, we chose the time course to avoid using empirical ratio-based corrections for the lithogenic material.
Additional subsamples were collected to determine rates of bSiO2 production (denoted as ρ). 125 mL or 250 mL polycarbonate bottles (depending on the station biomass) were filled to the brim and received 333 Bq of 32Si(OH)4 (>20 kBq µg Si-1). Bottles were immediately capped, gently inverted to mix the tracer, and placed into mesh bags made of neutral density screening to simulate the percent of incident irradiance (%I0) at the depth of sample collection. The bottles were then incubated for 24 hours in a deck-board incubator cooled by continuously flowing surface seawater. Following incubations, samples were filtered onto 25 mm diameter and 1.2 µm pore size polycarbonate filters, which were then placed onto nylon disc planchettes to dry and covered with a mylar film (secured using a nylon ring). 32Si activity was quantified using gas-flow proportional counting with a GM-25 multicounter (Risø National Laboratory, Technical University of Denmark) after aging the daughter isotope of 32Si, 32P, for seven half-lives (~4 months) to achieve secular equilibrium (Krause et al., 2011). Although some data from the middle MB corridor during autumn 2015 CONCORDE has been published (Dzwonkowski et al., 2017), the remaining data for this cruise, the spring 2016 CONCORDE cruise, and the two CLASiC cruises are original. Blanks were run on filtered seawater samples in which 32Si was added, incubated, and filtered again. Due to the cost of 32Si, only single replicates were done per sample point. Recent observations in the Bering and Chukchi Seas, both high diatom biomass systems like the nGoM, indicated that the coefficient of variation for replicate 32Si data from the averaged 12% and did not change significantly between diatom bloom and non-bloom stations (Krause et al., 2021). Statistical analysis of trends in biomass-normalized bSiO2 production among cruises were done using backward stepwise regression models in SigmaPlot software. Non-parametric comparisons of medians (Mann Whitney U Test) were used to evaluate differences in rates among prior studies and this one.
Results
Physical conditions
In the nGoM, atmospheric forcing (e.g. wind, precipitation) drives physical and biogeochemical variability in shelf waters. Dzwonkowski et al. (2017) observed that the induced storm surge following the passage of post-Hurricane Patricia (fall 2015) over the MB resulted in higher-than-normal and less variable salinity waters (ranging 32.1 to 35.6) drained from the regional estuaries. While most of the MB stations were surface-only, for two rate profiles at shallow stations (bottom depth 18 – 21 m), the irradiance rapidly attenuated and the euphotic zone depths ranged between 7 – 12 m. During the spring MB cruise, temperatures were similarly constrained and ranged from 19.3 to 21.2°C. Due to the high discharge, as expected, surface salinity ranged from 17.9 to 33.5 and laterally increased away from Mobile Bay (Figure 3). The depth of the euphotic zone ranged from 4 – 27 m among five vertical profiles. The two shallowest euphotic zones were associated with the shallowest sites (8 and 12 m) and the euphotic zones were 16 – 27 m (27 – 36 m bottom depth) among the three other sites.
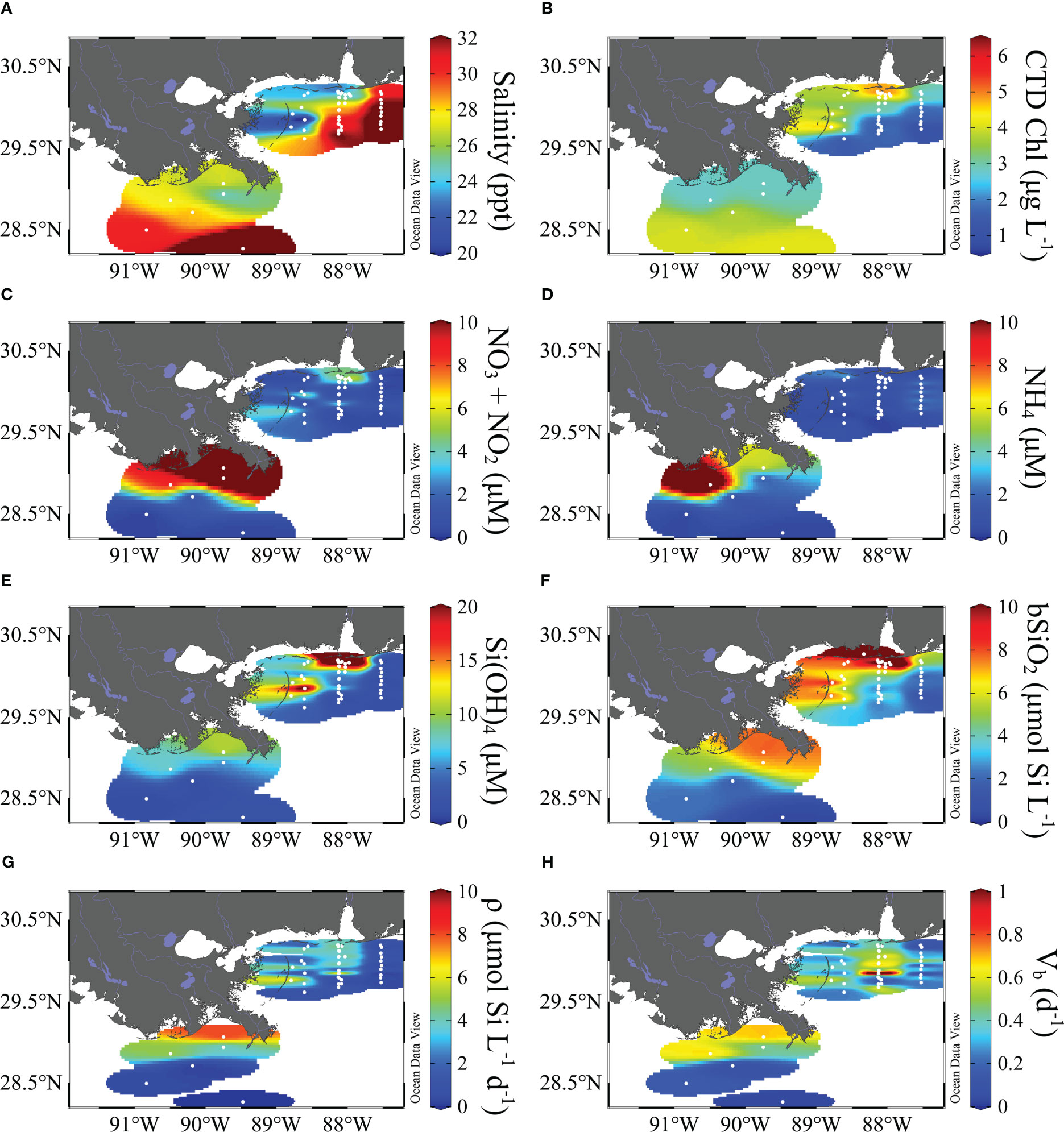
Figure 3 Surface properties for the spring cruises: LS during May 2017 and MB during March-April 2016. Properties include salinity (A), CTD-fluorescence based chlorophyll (B), nitrate+nitrite (C), ammonium (D), silicate (E), biogenic silica standing stock (F), production rate (G) and stock-normalized production rate (H). Units are listed on the plot. Map made using Ocean Data View (Schlitzer, 2016).
The LS physical conditions were similar to MB. Summertime LS surface temperatures were warmest among the four cruises, ranging from 29.0 to 30.3°C. Surface salinities during this cruise ranged from 16.7 to 33.9. All summer stations sampled were between the 20 and 50 m isobaths, and the euphotic zone depths ranged from 5 – 48 m; like the MB, the shallower euphotic zones on the LS were associated with the shallower stations. During the spring LS cruise, the surface temperatures were elevated compared to the spring MB cruise, ranging from 20.9 to 26.0, likely due to the May period LS cruise being later in spring (vs. late March – April for the MB cruise). The surface salinity range sampled was comparable but slightly narrower than the summer cruise, 19.6 to 31.6, although one station was sampled in Mississippi Canyon (~1000 m bottom depth, hereafter referred to as the “deep-water” site) and surface salinity was 36.3 —typical of deeper regional waters outside the direct freshwater influence. The spring-period LS euphotic zones were largely shallower than observed in summer, ranging from 7 – 19 m, and there was no clear relationship with bottom depth.
Dissolved nutrients
During autumn on the MB, dissolved nutrients showed typical ranges as observed in prior studies. NO3+NO2 and NH4 concentrations in the surface averaged 0.46 µM (range: detection limit – 2.1) and 0.33 µM (range: detection limit – 1.3), respectively (Figure 4). SRP was lower and less variable, averaging 0.28 µM (range 0.15 – 0.42). Si(OH)4 concentrations were an order of magnitude higher, averaging 3.51 µM (range: 1.31 – 10.2). The highest concentrations corresponded to surface stations on the southern MB shelf just offshore and eastward of the MR delta. Vertically, there were few differences among stations, unsurprising given the storm passage which thoroughly mixed the water column. During the spring, nutrients in the MB surface concentrations were higher and more variable (Figure 3). NO3+NO2 and NH4 concentrations averaged 2.78 µM (range: detection limit – 13.1) and 0.95 µM (range: detection limit – 3.78), respectively (Figure 4). The average SRP concentration 0.39 µM (range: 0.03 – 3.1) was not as high as inorganic nitrogen forms; however, the dissolved N:P ratios were still below the canonical Redfield-Ketchum-Richards ratio (16:1). Si(OH)4 was also higher than in autumn, averaging 9.33 µM (range 0.42 – 45.3, Figure 4). The highest concentrations for all nutrients were typically observed in the northern domain and a high station in the mid-shelf western domain (except for NH4) just offshore of the Chandeleur Islands (barrier island chain that forms a western boundary for the MB, Figure 3).
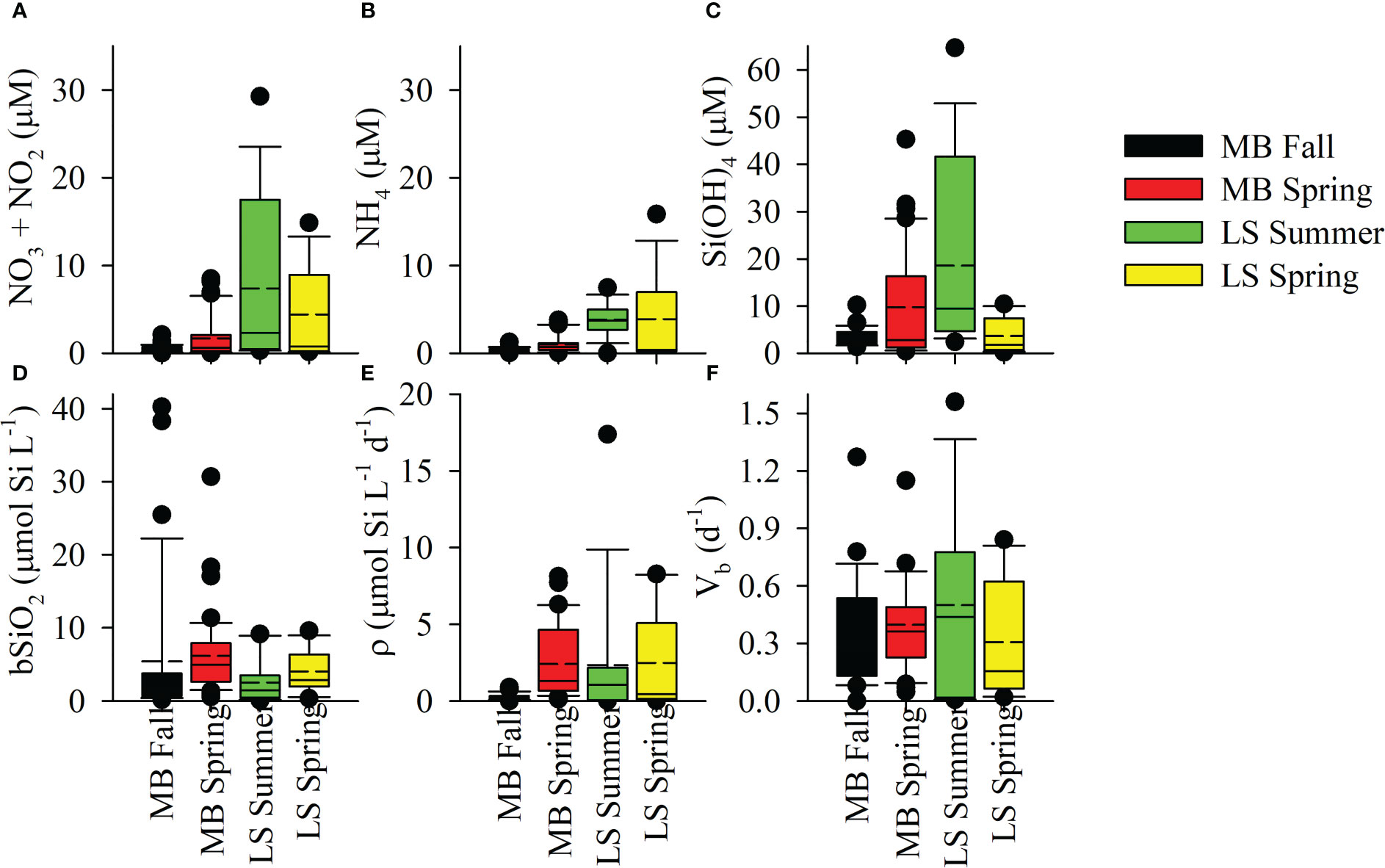
Figure 4 Surface box plots for nitrate+nitrite (A), ammonium (B), silicate (C), biogenic silica standing stock (D), production rate (E) and stock-normalized production rate (F) among all cruises. Box shows median (solid line) and average (dashed line), the box upper/lower boundaries denote the 25 and 75th percentiles with the whiskers denote the 10 and 90th percentiles.
While clear seasonal differences were observed on the LS, there was also a strong difference between nutrient magnitude in the MB and LS. During the LS summer, surface NO3+NO2 and NH4 concentrations averaged 7.40 µM (range: 0.30 – 29.3) and 4.12 µM (range: 1.94 – 7.47), respectively (Figure 4). The average surface SRP was 0.64 µM (range: 0.25 – 2.25). Si(OH)4 concentrations were higher than other nutrients, averaging 18.6 µM (range: 2.40 – 64.7). There was clear spatial variability among nutrients, with the highest concentrations in proximity to the MR outflow (see Dzwonkowski et al. (2018)). During spring, surface NO3+NO2 and NH4 concentrations averaged 5.20 µM (range: 0.17 – 14.9) and 5.59 µM (range: 0.20 – 15.8), respectively (Figures 3, 4). The surface SRP also was lower and less variable, averaging 0.14 µM (range: 0.12 – 0.17). At the deep-water station, surface nutrients were significantly lower than inshore with NO3+NO2, NH4, and SRP concentrations of 0.33, 0.34 and 0.18 µM, in that order (Figures 3, 4). Surface Si(OH)4 concentrations during the spring averaged 4.51 µM (range: 0.17 – 10.4) for the inshore stations, while the surface concentration at the deep-water station was 1.78 µM (Figures 3, 4). There were strong spatial gradients associated with the river proximity, i.e. the highest concentrations observed near the river, except for NH4 where the highest concentration observed in the surface was to the west and inshore (Figure 3).
Biogenic silica stock and production rates
bSiO2 standing stocks in the MB and LS were high but variable. During the autumn MB cruise, surface bSiO2 averaged 5.42 µmol Si L-1 (range: 0.16 – 40.3), while average bSiO2 in the surface was ~20% higher in spring, 6.18 µmol Si L-1 with a lower range (0.55 – 30.7, Figure 4). On the LS, surface bSiO2 were lower, averaging 2.53 µmol Si L-1 (range: 0.06 – 9.14) during the summer cruise. Surface bSiO2 at the shelf stations were elevated during LS spring and averaged 4.42 µmol Si L-1 (range: 1.37 – 9.59, Figures 3, 4). At the deep-water station, surface bSiO2 was lower (0.35 µmol Si L-1), consistent with the lower observed nutrients (Figure 3). At the stations where Si(OH)4 <1 µM, bSiO2 was 2.6 – 7.9 times higher than Si(OH)4, suggesting significant accumulation of diatom biomass. Vertically, bSiO2 in the euphotic zone declined with depth on the MB, although there was considerable variation in the average for the base of the euphotic zone, consistent with the shallow water column depth sampled and therefore the proximity to the sediment/water interface and nepheloid layer (Figure 5). On the LS, bSiO2 increased to a subsurface maximum in the mid-euphotic zone, with surface and deep average concentrations being similar (Figure 5). Between both regions, the average bSiO2 among euphotic zone depths varied by less than a factor of two, much less than variability in rates of production (discussed below).
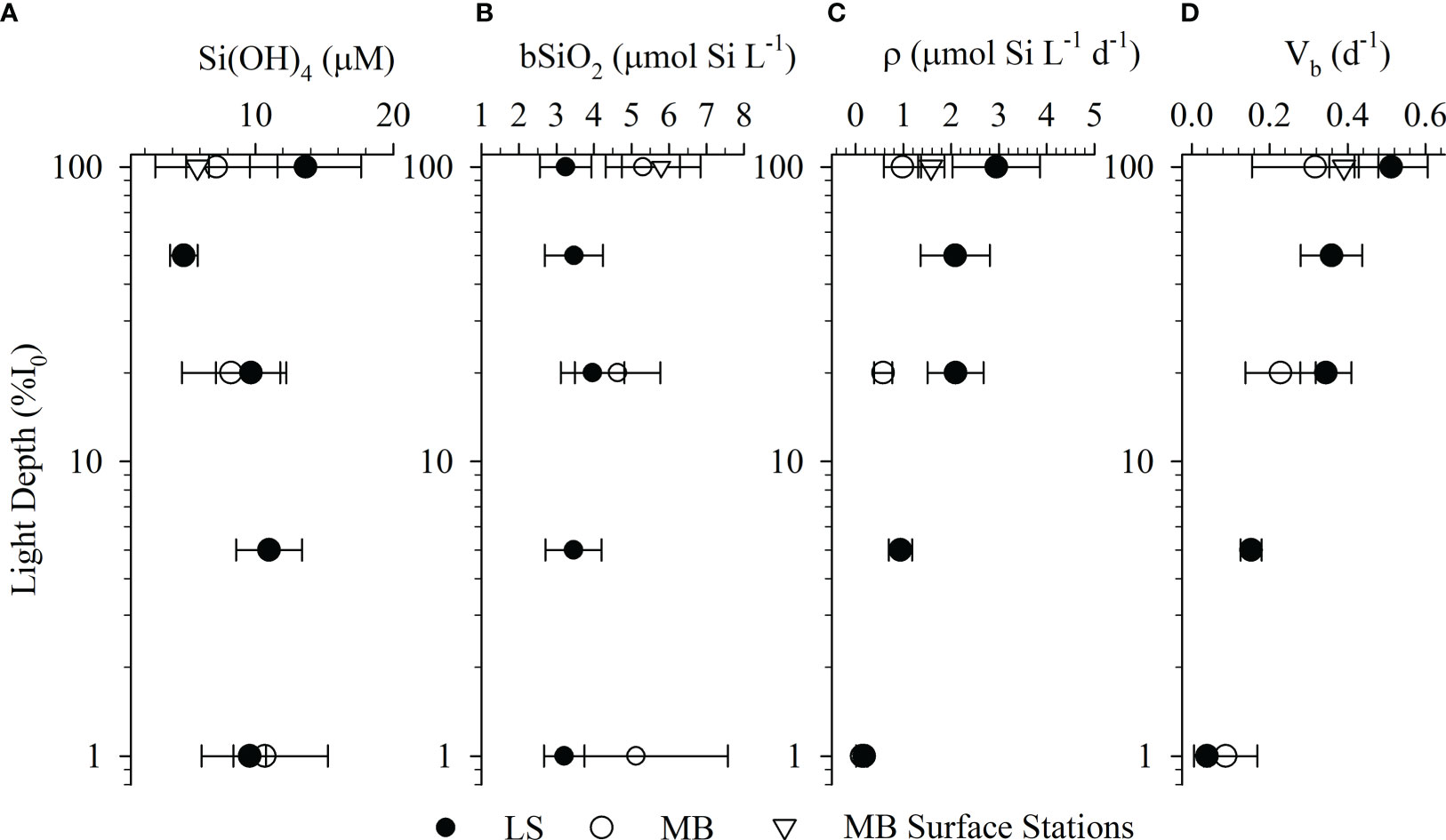
Figure 5 Average ( ± standard error) for silicate (A), biogenic silica standing stock (B), production rate (C), and stock-normalized production rate (D) per irradiance depth (LS and MB) or surface-only stations (MB).
Despite the apparent difference in surface bSiO2 between regions, there was less variation in the euphotic zone integrated stock. ∫bSiO2 in the two autumn MB profile stations were 6.17 and 29.0 mmol Si m-2, this was lower than the ∫bSiO2 among the four spring MB profile stations which averaged 57.1 mmol Si m-2 (range: 46.7 – 70.1, Table 1). On the LS, ∫bSiO2 in the summer was similar to MB in the autumn, averaging 21.9 mmol Si m-2 (range: 3.85 – 48.9, Table 1). However, ∫bSiO2 on the LS during spring was nearly three-fold higher, averaging 64.1 mmol Si m-2 (range: 31.8 – 108.8). At the deep-water station, ∫bSiO2 was 18.7 mmol Si m-2 (Table 1).
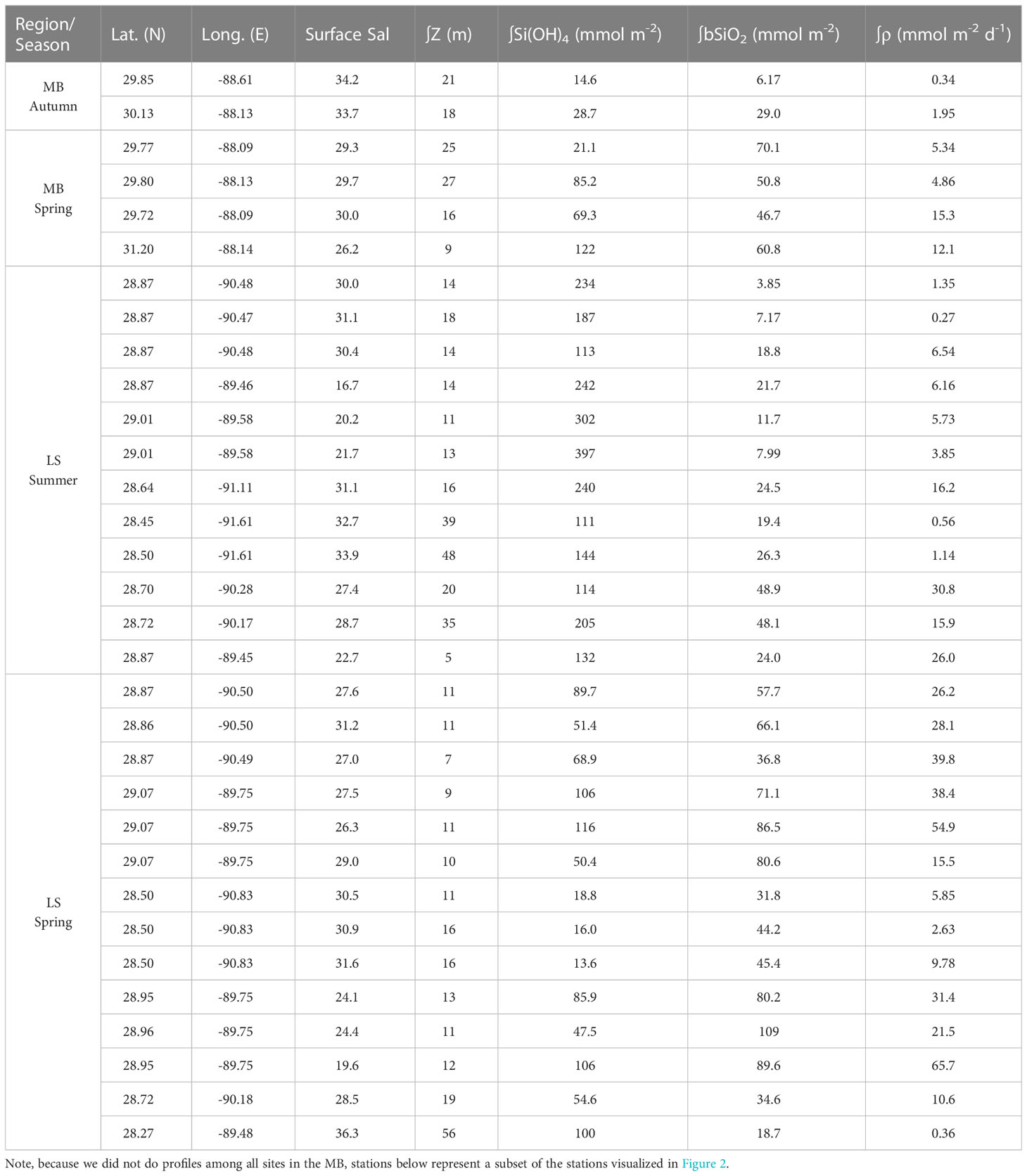
Table 1 Station locations and surface salinity, euphotic zone depth, and integrated Si(OH)4, bSiO2 and ρ among cruises.
The minor disparities in surface bSiO2 between regions were not apparent in the rate of biogenic silica production, ρ, in the surface. During autumn (MB), ρ averaged 0.28 µmol Si L-1 d-1 (range: below detection – 0.89). In the MB spring, surface ρ increased by an order of magnitude compared to the autumn, averaging 2.41 µmol Si L-1 d-1 (range: 0.11 – 8.11, Figure 3). On the LS during summer, the average ρ in the surface, 2.34 µmol Si L-1 d-1, and the range of ρ spanned over four orders of magnitude (range: below detection – 17.4, Figure 4). During spring, surface rates were similar, averaging 2.73 µmol Si L-1 d-1 (range: 0.06 – 8.26, Figure 3) on the shelf, with a low rate in the deep-water station (<0.01 µmol Si L-1 d-1, Figure 3). Vertically, ρ declined with depth in the euphotic zone for both regions (Figure 5); however, there was a disparity at depth, as ρ in the mid euphotic zone on the LS was ~twice the rate at the same light depth on the MB. ∫ρ during the autumn MB cruise stations were 6.17 and 29.0 mmol Si m-2 d-1, this was elevated in the MB during the spring where the average was 9.39 mmol Si m-2 d-1 (range: 4.86 – 15.3, Table 1). On the LS, ∫ρ in the summer and spring averaged 9.54 mmol Si m-2 d-1 (range: 0.27 – 30.8) and 27.0 mmol Si m-2 d-1 (range: 2.63 – 65.7), respectively. In the deep-water station, ∫ρ was 0.36 mmol Si m-2 d-1.
bSiO2 normalized ρ, Vb, suggest many zones had active diatom assemblages (Figure 5). During autumn on the MB, surface Vb averaged 0.36 d-1 (range: below detection to 1.27 d-1), while in the spring, the average Vb was similar 0.40 d-1 (range: 0.05 to 1.27 d-1). These average specific rates imply doubling times approximately 1.5 – 1.9 days. On the LS during summer, surface Vb exceeded the spring values in the MB, averaging 0.50 d-1 (range: <0.05 to 1.56 d-1). The summer surface rate was also higher than the average Vb during the spring for the shelf stations, 0.33 d-1 (range: 0.02 to 0.84 d-1). These LS rates imply doubling times between 1.4 – 2.1 days. At the deep-water station, Vb was low (0.03 d-1) and suggests a doubling time of ~23 days. The similarity in Vb between regions also manifested in vertical trends, as Vb declined precipitously in the lower euphotic zone (Figure 5).
Statistical analysis on the drivers of Vb (to account for biomass differences) were done using surface data. During the autumn on the MB, backward stepwise regression model retained Si(OH)4 and salinity as the best predictors of Vb (Table 2); however, in the spring, the NO3+NO2 alone was retained (Table 2). On the LS (excluding the deep-water site), the model retained Si(OH)4 in both seasons, but in summer and spring, SRP and NO3+NO2 were also retained, respectively, along with salinity in the spring (Table 2).
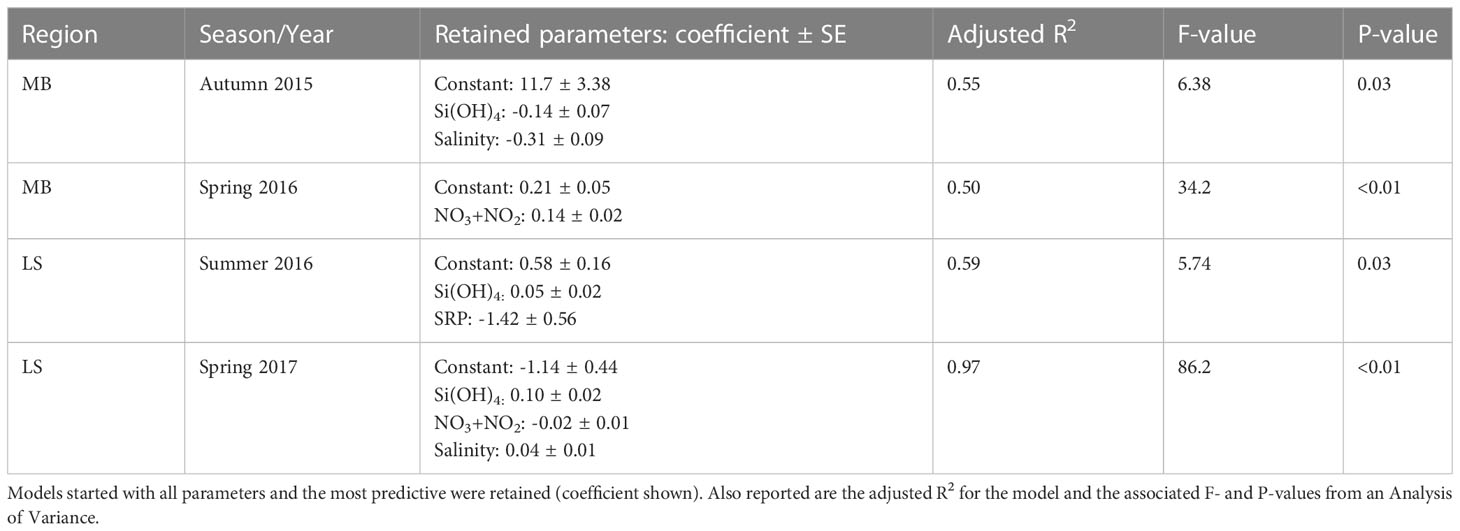
Table 2 Backwards stepwise regression analysis to explore drivers of Vb in the surface waters among stations and cruises.
Discussion
High silica production in the northern Gulf of Mexico: physics and allochthonous factors
River plumes are among the most highly productive ecosystems in the ocean. The nGoM shelf waters have been dubbed the ‘Fertile Fisheries Crescent’ (Gunter, 1963) due to the exceptional productivity of upper trophic level biomass. In many productive fisheries environments (e.g. Monterey Bay, Bering Sea), diatom production can be exceptional (Brzezinski et al., 2003; Krause et al., 2021). To our knowledge, this study reports the first ∫ρ rates within the euphotic zone for the nGoM. Despite the backdrop of a highly productive pelagic ecosystem, the measured ρ on the LS and MB are not among the highest in systems studied to date. Among a survey of 24 studies within many high and low productivity regions, the average ∫ρ rate on the LS shelf was among the upper 33% whereas MB is closer to the median (Figure 6A). ∫ρ in the highest productivity systems (e.g. coastal upwelling zones, Southern Ocean sectors) exceed averages of 50 mmol Si m-2 d-1, whereas the average for the LS during spring was 25 mmol Si m-2 d-1 (Table 1) and for MB was 10 mmol Si m-2 d-1 (Table 1). While the spring cruises were in different years (2016, MB; 2017, LS), the spread of the ∫ρ and the consistently higher values quantified on the LS suggests this may be a consistent difference; we discuss the potential reason(s) for this below (final discussion section). However, the magnitude of diatom production, while enhanced relative to many other systems, is not so exceptionally high as to be a clear factor leading to the stimulation of fisheries production in this region. Therefore, we propose that the unique hydrographic factors working in this system, help to physically concentrate diatom biomass to make it more assessable to higher trophic organisms. Some potential mechanisms are explored below:
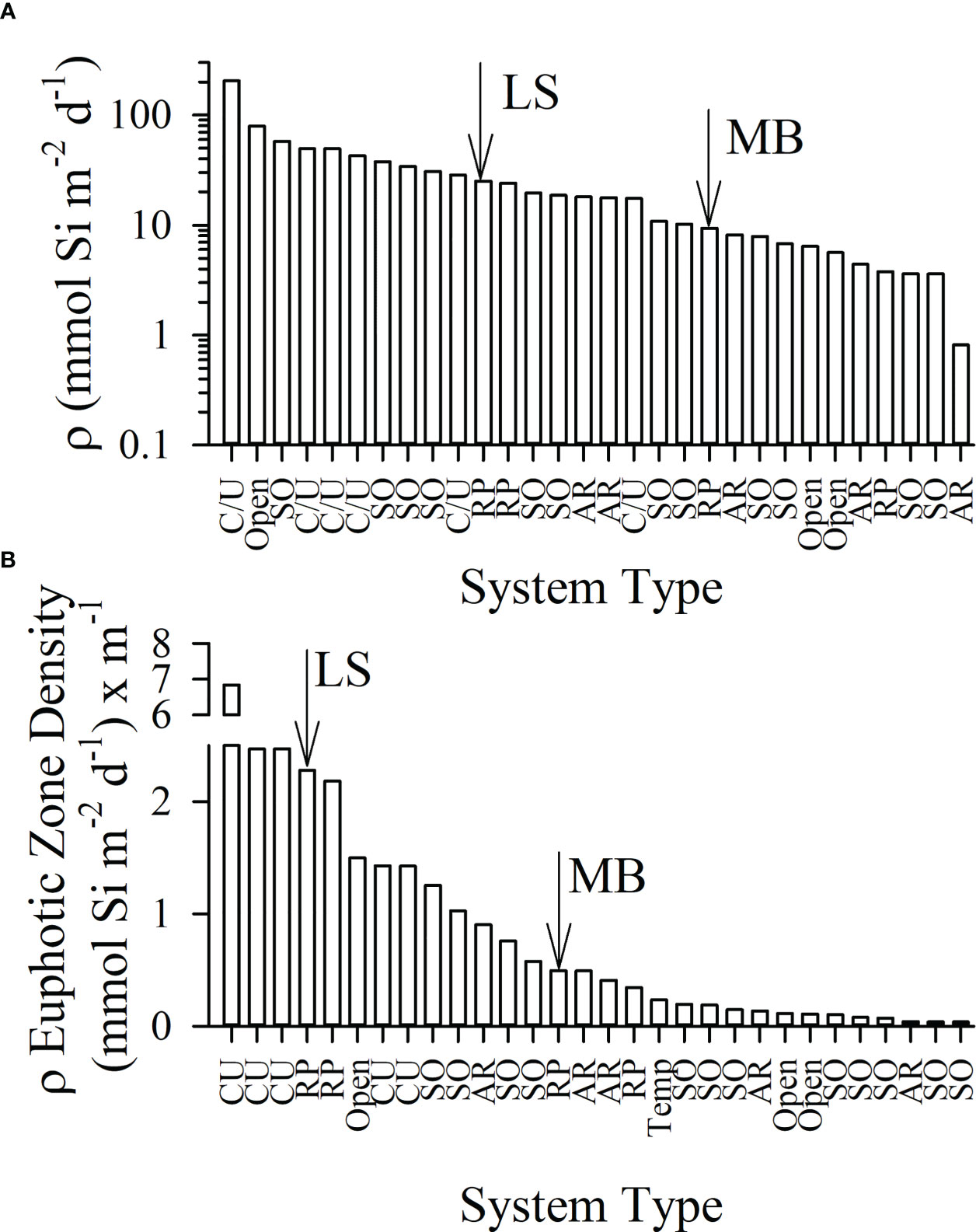
Figure 6 Survey of integrated euphotic zone biogenic silica production rate ranged from highest to lowest (A) among 24 studies and the euphotic-zone depth normalized production (B), termed euphotic zone density (mathematically equivalent single-depth units) for the same studies arranged from high to low. Average values per study are reported from the literature including the following systems: coastal/upwelling (C/U), river plume (RP), open ocean (Open), Southern Ocean (SO), and the Arctic region (RU); studies from mid-ocean gyres (e.g. Hawaii Ocean Time-series, Bermuda Atlantic Time Series study) were omitted due to very low absolute and depth-normalized rates. The average LS and MB value are denoted by the arrows in each plot. Data and original studies are listed in Supplementary Table S1.
Given the river plume forcing, we posit that the high system productivity in the LS and MB can be partially explained by diatom productivity being highly accessible to higher trophic organisms. When normalizing the ∫ρ depth to the depth of the euphotic zone (i.e. mathematically equivalent to volumetric rates, e.g. µmol L-1 d-1 or mmol m-3 d-1), the LS is the 5th highest, and the MB is in the upper 50% for ∫ρ among our survey (Figure 6B). The local river plume forcing facilitates water-column stability (stratification) and thereby allows phytoplankton to consume nutrients and avoid light limitation due to deep mixing. The degree of stratification in this system is extreme, as density differences between the surface and main pycnocline due to salinity are typically much higher (>>1 kg m-3) than open ocean metrics used define mixed layers (e.g. >0.1 kg m-3 differences from the surface). Thus, residence in this stable water column facilitates phytoplankton consumption of nutrients to exhaustion —if other ecological or physical factors are favorable. Indeed, the lowest surface Si(OH)4 observed during the LS spring bloom was 0.17 µM. This concentration is multiple factors lower than Si(OH)4 observed (i.e. ~0.6 – 0.8 µM) in the low-diatom-biomass oligotrophic gyres (Brzezinski et al., 2011; Krause et al., 2017) and is similar to intense diatom blooms observed on the LS (Nelson and Dortch, 1996) or in productive upwelling systems (Nelson et al., 1981; Krause et al., 2015; Closset et al., 2021). We suggest that the LS and MB physics allow for diatom production to be more accessible to higher trophic organisms compared to other systems where the integrated productivity may be higher, but more diffusely distributed within the water column. This is consistent with ideas concerning the importance of spatial aggregations as predictors of higher trophic level variation, e.g. Benoit-Bird and McManus (2012), opposed to typical volumetric abundances. Specifically, the ideas that have emerged in the last decade are not that volume-based rates or standing stock matter for ecological productivity, but instead how aggregated and concentrated production is (or can be at times), as highly concentrated production can be more easily accessed by higher trophic levels.
Beyond basic river-plume stratification dynamics (discussed above), there is regional evidence that small scale and/or ephemeral physical mechanisms in the nGoM may also help efficiently funnel diatom productivity to higher trophic levels. Using an in situ imaging system, Greer et al. (2020) observed a ~2.3 km (lateral extent) thin layer, dominated by the diatom Odontella sp., during July 2016 in the southwestern domain of the MB. Odontella species typically have large cell sizes compared to other diatom genera, especially slender pennate species which are abundant regionally (MacIntyre et al., 2011; Bargu et al., 2016). Greer et al. (2020) suggested the growth and grazing decoupling and motility (i.e. Odontella sp. is not a swimmer) in the thin layer were not major factors contributing to its formation. Specifically, Odontella sp. abundances outside of the thin layer were so low the disparity in abundances within and outside of the layer could not be solely from increased growth rates within the layer; therefore, these authors concluded diatom cells were concentrated by physical processes. Leveraging a high-resolution physical model, Greer et al. (2020) suggested the thin-layer feature was driven by surface convergence and vertical shear. Furthermore, their model outputs suggested that similar convergences occur frequently in the MB region and may contribute to efficient trophic transfer of phytoplankton organic matter to higher trophic levels. Such an idea is consistent with other studies in warm-water regions where standing stocks of phytoplankton may be relatively low but can be enhanced by similar physical mechanisms and fuel efficient passage of producer organic matter to higher trophic level organisms, e.g. Hawaii (McManus et al., 2012).
Despite the potential importance for thin layers regionally, it is unlikely that such dynamics were resolved in our study. Our vertical sampling was limited to four or five depths, which were predetermined based on the irradiance levels required for our deck board incubator. Consequently, it is unlikely that such thin-layer biomass would have been accurately captured in our rates. Specifically, the vertical orientation of the Niskin bottle would likely have sampled a component of such a layer (if any at all). However, this may not matter when trying to quantify the regional ρ. Our bottle incubations clearly captured the vertical extent of euphotic-zone productivity (Figure 5). Such short-duration physical dynamics acting to concentrate (opposed to stimulate) diatom production within a vertical layer would not radically alter the total euphotic zone integrated production, but redistribute (i.e. concentrate) the biomass. Thus, these features may not affect total ρ or annual productivity but could be important to facilitate more efficient transfer of this material up the foodweb.
Comparison of ∫ρ rates with riverine Si(OH)4 delivery show differences in the river effect between systems. Given our vertical sampling was confined to the euphotic zone, we consider stocks and rates in a euphotic-zone integrated context (e.g. one euphotic-zone box opposed to a two-box water column). In the MB, the delivery of riverine Si(OH)4 to the CONCORDE sampling domain (area of the three corridors) would support ~33-40% of the ∫ρ (Table 3); the proportional importance decreases if the entire MB shelf area is considered (i.e. Si in discharged water would be spread over a larger area). This implies that other Si(OH)4 sources are necessary to sustain the production rates of diatoms in the water column. Water-column recycling of bSiO2 can be quantitatively important. Brzezinski et al. (2003) reported that even during blooms in productive systems (e.g. Monterey Bay), 10-20% of ∫ρ was supported by euphotic-zone bSiO2 remineralization. Furthermore, the proportional support of ∫ρ from dissolution increases during non-bloom periods (e.g. 60-70% of ρ) or in regions with warmer water temperatures (e.g. ~30% in a Gulf Stream Warm Core Ring). Given surface temperatures in the MB were ~20°C and 24°C for the spring and autumn cruises, respectively, and the specific dissolution rate for bSiO2 in slightly cooler (~15-20°C) subtropical waters was reported to be ~0.15 d-1 (Brzezinski and Nelson, 1989), such a dissolution rate applied to our measured ∫bSiO2 standing stock would be sufficient to meet 65-100% of the ∫ρ during the MB cruises (Table 1). Note, this would be an upper estimate if most of the bSiO2 standing stock dissolves below the euphotic zone. Furthermore, benthic flux, while not reported for Si(OH)4 in the MB is likely similar to that observed on the LS given the similarity in bSiO2 content in sediments between these regions (Pickering et al., 2020; Kemp et al., 2021). Lehrter et al. (2012) reported benthic Si flux rates ranging from 0.3 – 4.4 mmol Si m-2 d-1 on the LS in spring and summer. This represents a major pool of usable Si but given the shallow euphotic zones and surface layers, such a pool may not be accessible to diatoms until tropical cyclones (e.g. summer) or cold fronts (e.g. autumn) break down this stratification and mix the water column. Taken together, the MB data suggest that over the entirety of the shelf, riverine Si and internal recycling may meet the Si demand for diatoms; however, within this broad area, there are ephemeral conditions where diatoms can exhaust Si faster than replenishment, as has been observed offshore of coastal Alabama in the northern domain of the MB, e.g. MacIntyre et al. (2011).
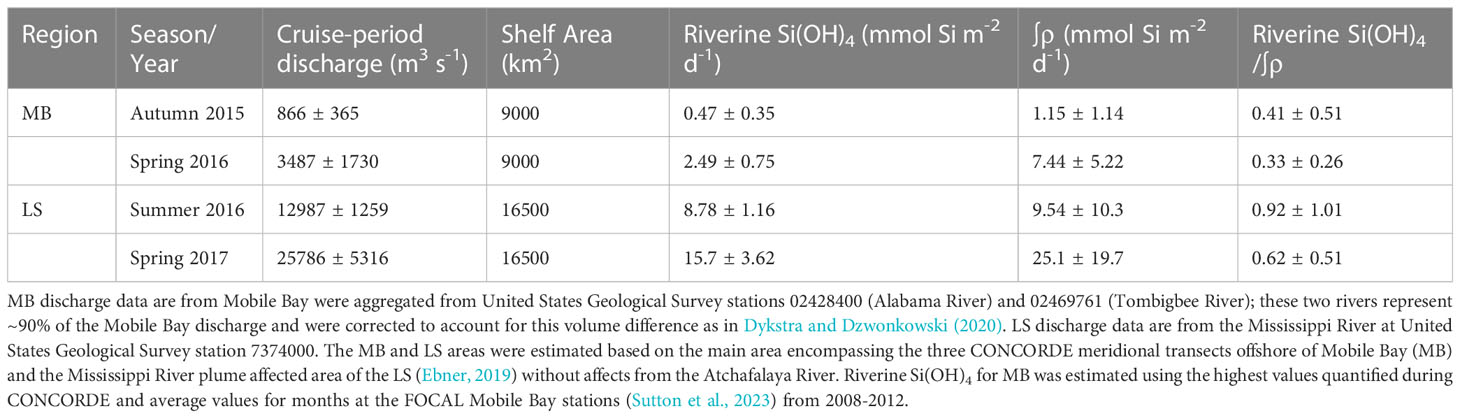
Table 3 Comparison of riverine Si(OH)4 flux relative to ∫ρ (e.g. Table 1) on the MB and LS during each cruise (error is standard deviation).
Unlike the delivery of Si(OH)4 to the MB, the MR delivers an order of magnitude more Si to the LS where it is used in an area less than twice that considered for the MB (Table 3). During the summer and spring, the riverine flux averaged 62% and 92% of the measured ∫ρ. Thus, over the entirety of the shelf, the river flux combined with internal recycling of bSiO2 (discussed above) should have been sufficient to meet the Si demand for diatoms. Furthermore, consistent with calculations by Lehrter et al. (2012), benthic flux of Si could also have been a significant source of Si to help diatom avoid limitation, especially for any diatom production occurring deeper than the main pycnocline. While many studies have suggested that Si limitation may be more prevalent now than before eutrophication (Turner et al., 1998), when considering bulk rates of delivery and shelf-wide production, any limitation by Si is not due to a lack of Si within the integrated system (i.e. upper water column over the shelf area) but must be due to the balance between diatom growth and the Si(OH)4 supply to the euphotic zone. While the Turner et al. (1998) study (among others), inferred diatom Si limitation by nutrient ratios (i.e. Si depleted before N), there is more direct evidence showing that diatoms can be limited by Si(OH)4 on the LS. Nelson and Dortch (1996) reported many instances where Si(OH)4 in the upper water column was <1.5 µM in the summer and <0.4 µM in the spring. We did not observe such low concentrations during the summer, but during the spring on the LS six (of 14) stations had surface Si(OH)4 <1 µM with the lowest concentration of 0.17 µM (Figure 3). Thus, both our study and Nelson and Dortch (1996) demonstrate diatoms can effectively exhaust Si(OH)4 faster than local physical delivery (e.g. mixing) or remineralization scales in the euphotic zone, despite considerable pools of Si which are separated by relatively short vertical scales (e.g. <5 m) —especially compared to gradients in deep-water systems. In the absence of exceptionally concentrated diatom production, which can deplete Si(OH)4 to levels that likely limit diatom growth, e.g. Nelson and Dortch (1996) (and this study), it appears that most of the Si production on the shelf can be met by external Si delivered by the MR with the rest being met by internal recycling or benthic flux (after the exceptional water column stratification is broken down).
Biogenic silica production drivers
While many factors potentially affect the rate of biogenic silica production (i.e. Vb), our analysis focused on a common subset of parameters available for all cruises. The models all explain at least a majority of the variance (i.e. R2 = 0.50 to 0.97), but only one explains nearly all the variance (i.e. LS Spring). Thus, for the other three cruises (LS Summer, MB cruises), there is a quantitatively significant portion of variance which cannot be explained by the variables considered. Despite this, the model results provide an empirical approach to evaluate the factors which best correlated to Vb during these cruises.
A significant number of studies reporting ρ are from systems with relatively invariant salinity. Even in coastal systems with such data, e.g. Bay of Brest (Beucher et al., 2004), salinity ranges are relatively minor (e.g. 32 – 36) compared to the nGoM. During our study, we observed considerable variability in surface salinity during spring (both regions, Figure 3) and summer (LS). For the MB, the effect of the estuarine condition differed between autumn and spring. The autumn cruise occurred after the passage of the remnants from Hurricane Patricia (Dzwonkowski et al., 2017); note, given the lack of historical data, we cannot disentangle the effect of any prior anthropogenic changes that may alter the response following the storm. The storm surge flooded the nGoM estuaries, and as the waters flowed back into the Gulf, the post-storm stratification led to increased rates of primary production, especially among larger cells (e.g. Trichodesmium, diatoms) within 15 km of the Mobile Bay outflow (Dzwonkowski et al., 2017). The regression model for these autumn 2015 data shows that the strongest predictors for Vb were salinity and Si(OH)4 (Table 2). Both parameters had negative coefficients, which means they scaled in the same direction. Si(OH)4 in the waters feeding MB (e.g. Mobile Bay) behaves semi-conservatively, especially compared to other dissolved nutrients, e.g. NO3+NO2, NH4, SRP (Sutton et al., 2023), and thus we would expect Si(OH)4 and salinity to be negatively correlated (i.e. higher Si(OH)4 at lower salinities). Our model instead showed these parameters trended the same direction and Vb was highest when Si(OH)4 and salinity were lower. Such a trend was likely driven by the receding storm surge. Thus, the importance of the salinity effect in this specific season was the ability for the low salinity water to stabilize the water column (which had been well mixed) post storm thereby allowing phytoplankton (e.g. diatoms) to grow; this is consistent with interpretations by Dzwonkowski et al. (2017). During spring in the MB, salinity was not a significant predictor, as Vb was positively associated with NO3+NO2 (Table 2). Such a result is consistent with the differences in the Si:N ratio of waters feeding the MB and LS, where Si:N in the MB is high (>1) and optimal for diatoms compared to the LS (Figure 1, discussed below). In this case, the quantity of NO3+NO2 to the MB was the best predictor of the quantified diatom silica production rate, suggesting a potential stimulatory effect near the plume edge where nutrients are still elevated relative to the offshore waters.
Drivers on the LS differed from those on the MB. The loading of nutrients in the MR watershed increased both N and P in the river over time (Figure 1 for nitrate). Determining what nutrient may limit primary production for the community has important regional implications for management actions in the watershed. For instance, much of the regional regulatory effort to combat hypoxia on the LS focuses on the reduction of N in the river. But while N can limit primary productivity on the LS, e.g. Rabalais et al. (2002) and references therein, there are times when P can be limiting, e.g. Sylvan et al. (2006). The LS cruise effort was more focused on benthic-pelagic coupled processes vs. the cruises in the MB with more fine-scale spatial sampling; therefore, in the LS we have only ~25% of the spatial stations compared to the MB: although unlike the MB, we have full vertical profiles for each occupied station.
The backward stepwise regression model analysis identified that the strongest nutrient predictors for Vb during the summer and spring cruises were NO3+NO2 (spring, negative coefficient), SRP (summer, negative coefficient), silicate (both seasons, positive coefficient), and salinity (spring, positive coefficient). Thus, our data analysis suggests that diatom productivity (Vb) was responding more to NO3+NO2 in the spring and SRP in the summer, while there is a persistent underlying response to Si(OH)4 in both seasons. The latter is expected as Si(OH)4 affects both diatom growth rates and their rate of Si uptake (e.g. nutrient kinetics). The positive correlation between Si(OH)4 and Vb in our statistical analysis infers some degree of kinetic limitation, i.e. Si(OH)4 is suboptimal for uptake (Vb at ambient Si(OH)4 < Vb at non-limiting Si(OH)4) and under these conditions diatoms alter physiology to compensate (McNair et al., 2018). Such kinetic limitation is also consistent with previous LS data from the early 1990s (Nelson and Dortch, 1996).
Model results indicate that the sign of the model coefficients for NO3+NO2 in the spring and SRP in summer are negative. These data infer that Vb, a proxy for diatom growth, is stimulated at lower NO3+NO2 and SRP concentrations than the high-nutrient riverine endmember (if this were the case, then the model coefficient for both would be positive). This is consistent with both remote data and shipboard incubation work showing that primary production and phytoplankton biomass accumulation rates are not highest in the main part of the river discharge zone but downstream, likely due to improved light conditions (reduced turbidity) and stratification which facilitates growth (Lohrenz et al., 1997; Lehrter et al., 2009). Our project results highlight that Si(OH)4 always plays a role in diatom Si uptake (and potentially becomes a growth-limiting factor when concentrations are very low, e.g. <1 µM), but typically diatom activity switches from being more correlated to NO3+NO2 in the spring and SRP in the summer. Such empirical connection of diatom activity to three different nutrients suggests modeling diatom growth in this region over time may be challenging. Identifying the underlying factors affecting these trends (e.g. changes in diatom assemblage, direct evidence of nutrient limitation) should be a priority given diatoms critical role in system productivity.
Has diatom silica production changed due to eutrophication?
As discussed, there have been significant changes to Si(OH)4 and NO3 concentrations in the MR (Figure 1) which feeds the nGoM (Turner and Rabalais, 1991; Turner and Rabalais, 1994a; Turner and Rabalais, 1994b; Turner et al., 2008). These results compelled a United States Environmental Protection Agency Science Advisory Board (Dale et al., 2007) to recommend that:
“. the potential for silicate limitation and its effects on phytoplankton production and composition on the Louisiana-Texas continental shelf should be explored when carrying out experiments on the importance of N and P as limiting factors and when considering nutrient management scenarios.”
The relationship between eutrophication and diatom production could be evaluated by understanding how diatom production rates respond to eutrophied conditions. However, the lack of diatom-specific rate information, facilitated by using isotope-addition methods (e.g. 30Si, 32Si), has hindered understanding the quantity of production attributed to diatoms in this system. While our production rate data set lacks a robust baseline, i.e. one study reporting data on ρ during the early 1990s (Nelson and Dortch, 1996), the comparison between the LS and MB offers potential insight regarding how the system operates now and we can speculate whether this may have changed in the last half century.
Compared to a past study in the nGoM (Nelson and Dortch, 1996), we find no evidence that diatom growth (i.e. Vb is proxy) in the upper depths is significantly different. Working in the same region of the LS during the early 1990s, rates reported by Nelson and Dortch (1996) during the summer and spring fell within the range for our study on the LS during the same seasons (Figure 4) with no significant differences (Mann Whitney U Test (Summer, Spring), U = 25, 47, p = 0.29, 0.88), albeit these earlier data are less variable. Nelson and Dortch (1996) also observed a significant difference between LS rates during spring (lower) and summer (higher), such a trend was not resolved during this present study. With the caveat that Nelson and Dortch (1996) did not report vertically integrated rates, the comparison of our data with those in the early 1990s does not suggest any significant change to the specific rate of diatom bSiO2 production.
Comparison of the ∫ρ data during spring between the MB and LS are consistent with a eutrophication effect suggested in previous studies. There is relatively low nitrate in the Mobile Bay waters, <20 µM (Pennock et al., 1999), compared to the >100 µM concentrations in the MR derived from eutrophication in the watershed (Figure 1). The high nitrate and relative lack of light limitation as the MR discharges onto a deeper continental shelf, potentially facilitates Si(OH)4 uptake while waters are diluted on the LS (consistent with reported non-conservative behavior for Si(OH)4). This situation (i.e. high NO3+NO2 and NO3+NO2>Si(OH)4) does not appear to occur in the MB (consistent with reported conservative behavior for Si(OH)4). During spring, the LS had higher surface concentrations and ranges for NO3+NO2 and NH4 relative to those in the MB, but surface concentrations of Si(OH)4 were higher in the MB than in LS (Figure 3). Such differences are consistent with the well-reported anthropogenic factors in the MR watershed compared to the MB watersheds. For example, the increased nitrogen has been linked to fertilizer use in the MR watershed (Turner et al., 1998). Whereas the reduced Si(OH)4 over time has been attributed to factors like damming (e.g. bSiO2 retained in reservoirs behind dams vs. being carried downstream and dissolving en route), as reported in the Black Sea (Humborg et al., 1997) and other systems (Humborg et al., 2000). In the MB, the watersheds which provide fluvial input onto the shelf ecosystem are much smaller, with comparatively less developed urban and agricultural regions, and have the opposite nutrient trends (i.e. relatively lower NO3+NO2 and higher Si(OH)4) than the MR. Although urbanization is occurring within the MB watershed, the degree of eutrophication is much lower than for the MR (Pennock et al., 1999). Such watershed differences would be expected to affect diatom processes downstream in the coastal domain.
The integrated diatom productivity appears to be significantly higher in the LS compared to the MB during spring, the season we can compare directly. Despite comparable Vb in the MB surface waters, the average ∫ρ was 2.5x higher in the euphotic zone of the LS than the MB. This difference is stark considering the average euphotic zone depth on the LS in spring was ~12 m vs. ~19 m for the MB. While both euphotic zones are relatively shallow compared to deep-water systems, there is likely a vertical zone in both systems where cells switch from nutrient limitation (under high irradiance) to light limitation —sensu Dugdale (1967). Phytoplankton cells can compensate for the lower light by increasing their light harvesting capacity (e.g. increase pigment per cell); however, pigment complexes have higher N requirements relative to P (Geider et al., 1996). Thus, given that the integrated standing stock of NO3+NO2 on the LS in spring was double (~90 mmol m-2) that in the euphotic zone of the MB (~45 mmol m-2, data not shown), the additional NO3+NO2 may have helped diatoms in the lower euphotic zone avoid reducing growth rates due to rapidly attenuating light in the turbid waters. Hence, we posit that eutrophication sets a higher potential for diatom biomass due to higher total N to exploit (if sufficient Si is available) and increases the euphotic-zone ∫ρ via facilitating higher uptake rates at depth (Figure 5).
Due to lack of baseline data for the water column on the effect of eutrophication on diatom production, our interpretation that ρ on the LS has increased in response to eutrophication cannot be tested directly. However, we can glean insights from local sediment records or use experimental approaches (e.g. bioassays) in future studies. For example, on the LS, there has been an increased preservation of diatom valves (especially the genera Pseudo-nitzschia which can have toxic species) in sediments through the end of the 20th century (Parsons et al., 2002). On a similar timescale, albeit not directly synchronized with Pseudo-nitzschia, there have also been increases in sediment bSiO2 (Turner et al., 2008). It is unknown if these trends have continued in the last two decades since these reports. The general trend of increased bSiO2 preservation in sediments after eutrophication, due to increased diatom ρ in the water column, has been observed in limnic systems (Schelske et al., 1987), suggesting some fundamental similarities in the responses of diatoms assemblages to eutrophication among environments. Given the lack of baseline data to assess the general trends in ρ during and before eutrophication in the 20th century, new methods (e.g. silicon stable isotopes) could be employed to fill these temporal gaps. Comparison of trends over time between the LS and MB sediments could provide a means to better understand whether eutrophication has changed diatom silica utilization and production regionally or if similar spatial differences observed in this study reflect general subregional differences driven by the size of the watersheds feeding each site.
Data availability statement
The datasets presented in this study can be found in online repositories. The names of the repository/repositories and accession number(s) can be found below: https://www.bco-dmo.org/project/712667, http://data.gulfresearchinitiative.org (doi: 10.7266/N78050N9), http://data.gulfresearchinitiative.org (doi: 10.7266/N70K2738).
Author contributions
All authors were involved in the collection of samples, sample analyses, archiving data, and assisted with editing the manuscript. The study was conceived by JK with input from KM. The data analysis and initial manuscript was done by JK. All authors contributed to the article and approved the submitted version.
Funding
The Louisiana Shelf work was funded by the United States National Science Foundation Chemical Oceanography (OCE‐1558957 to JK and KM). The Mississippi Bight research was made possible by a grant from the Gulf of Mexico Research Initiative through the CONCORDE Consortium and ACER Consortium programs (both to JK). Data associated with this work are publicly available through the Biological & Chemical Oceanography Data Management Office (https://www.bco-dmo.org/project/712667) and Gulf of Mexico Research Initiative Information & Data Cooperative (GRIIDC) at http://data.gulfresearchinitiative.org (doi: 10.7266/N78050N9, 10.7266/N70K2738).
Acknowledgments
We dedicate this work to the memory of our friend and colleague, Sydney Acton; her technical efforts before, during, and after these four cruises was critical to their success. We also thank the captains, crew, and science parties aboard the R/V Point Sur and R/V Pelican (especially Kevin Martin, Allison Mojzis, Wokil Bam, Neha Ghaisas), Brian Dzwonkowski and Alan Shiller for helpful discussions, and John Perry, Eric Lachenmyer, Grant Lockridge, Yantzee Hintz, and Laura Linn for technical assistance.
Conflict of interest
The authors declare that the research was conducted in the absence of any commercial or financial relationships that could be construed as a potential conflict of interest.
Publisher’s note
All claims expressed in this article are solely those of the authors and do not necessarily represent those of their affiliated organizations, or those of the publisher, the editors and the reviewers. Any product that may be evaluated in this article, or claim that may be made by its manufacturer, is not guaranteed or endorsed by the publisher.
Supplementary material
The Supplementary Material for this article can be found online at: https://www.frontiersin.org/articles/10.3389/fmars.2023.1162685/full#supplementary-material
References
Baines S. B., Twining B. S., Brzezinski M. A., Krause J. W., Vogt S., Assael D., et al. (2012). Significant silicon accumulation by marine picocyanobacteria. Nat. Geosci. 5, 886–891. doi: 10.1038/ngeo1641
Bargu S., Baustian M. M., Rabalais N. N., Del Rio R., Von Korff B., Turner R. E. (2016). Influence of the Mississippi river on pseudo-nitzschia spp. abundance and toxicity in Louisiana coastal waters. Estuaries Coasts 39, 1345–1356. doi: 10.1007/s12237-016-0088-y
Benoit-Bird K. J., McManus M. A. (2012). Bottom-up regulation of a pelagic community through spatial aggregations. Biol. Lett. 8, 813–816. doi: 10.1098/rsbl.2012.0232
Beucher C., Tréguer P., Corvaisier R., Hapette A. M., Elskens M. (2004). Production and dissolution of biosilica, and changing microphytoplankton dominance in the bay of Brest (France). Mar. Ecology-Progress Ser. 267, 57–69. doi: 10.3354/meps267057
Biard T., Krause J. W., Stukel M. R., Ohman M. D. (2018). The significance of giant phaeodarians (Rhizaria) to biogenic silica export in the California current ecosystem. Global Biogeochemical Cycles 32, 987–1004. doi: 10.1029/2018GB005877
Brzezinski M. A. (1985). The Si:C:N ratio of marine diatoms: interspecific variability and the effect of some environmental variables. J. Phycology 21, 347–357. doi: 10.1111/j.0022-3646.1985.00347.x
Brzezinski M. A., Jones J. L., Bidle K. D., Azam F. (2003). The balance between silica production and silica dissolution in the sea: insights from Monterey bay, California, applied to the global data set. Limnology Oceanography 48, 1846–1854. doi: 10.4319/lo.2003.48.5.1846
Brzezinski M. A., Krause J. W., Church M. J., Karl D. M., Li B., Jones J. L., et al. (2011). The annual silica cycle of the north pacific subtropical gyre. Deep-Sea Res. I 58, 988–1001. doi: 10.1016/j.dsr.2011.08.001
Brzezinski M. A., Nelson D. M. (1989). Seasonal changes in the silicon cycle within a gulf stream warm-core ring. Deep-Sea Res. 36, 1009–1030. doi: 10.1016/0198-0149(89)90075-7
Cloern J. E. (2001). Our evolving conceptual model of the coastal eutrophication problem. Mar. Ecol. Prog. Ser. 210, 223–253. doi: 10.3354/meps210223
Closset I., Mc Nair H. M., Brzezinski M. A., Krause J. W., Thamatrakoln K., Jones J. L. (2021). Diatom response to alterations in upwelling and nutrient dynamics associated with climate forcing in the California current system. Limnology Oceanography 66, 1578–1593. doi: 10.1002/lno.11705
Dale V., Bianchi T., Blumberg A., Boynton W., Conley D., Crumpton W., et al. (2007). “Hypoxia in the northern gulf of Mexico: an update by the EPA science advisory board,” in EPA-SAB-08-003 (Washington, DC: EPA Science Advisory Board), 333 pp.
Dortch Q., Parsons M., Rabalais N., Turner R. (1999). What is the threat of harmful algal blooms in Louisiana coastal waters. Recent Res. Coast. Louisiana: Natural System Funct. Response to Hum. Influences 230, 134–144.
Dugdale R. C. (1967). Nutrient limitation in the sea: dynamics, identification, and significance. Limnology Oceanography 12, 685–695. doi: 10.4319/lo.1967.12.4.0685
Dykstra S., Dzwonkowski B. (2020). The propagation of fluvial flood waves through a backwater-estuarine environment. Water Resour. Res. 56, e2019WR025743. doi: 10.1029/2019WR025743
Dzwonkowski B., Fournier S., Reager J. T., Milroy S., Park K., Shiller A. M., et al. (2018). Tracking sea surface salinity and dissolved oxygen on a river-influenced, seasonally stratified shelf, Mississippi bight, northern gulf of Mexico. Continental Shelf Res. 169, 25–33. doi: 10.1016/j.csr.2018.09.009
Dzwonkowski B., Greer A., Briseño-Avena C., Krause J., Soto I., Hernandez F. J., et al. (2017). Estuarine influence on biogeochemical properties of the Alabama shelf during the fall season. Continental Shelf Res. 140, 96–109. doi: 10.1016/j.csr.2017.05.001
Ebner B. (2019). Spatiotemporal variation of benthic silica fluxes in the NGOM shelf (Louisiana State University: Masters).
Geider R. J., MacIntyre H. L., Kana T. M. (1996). A dynamic model of photoadaptation in phytoplankton. Limnology Oceanography 41, 1–15. doi: 10.4319/lo.1996.41.1.0001
Ghaisis N. A., Maiti K., Roy A. (2021). Iron-mediated organic matterpreservation in the Mississippi River influenced shelf sediments. Journal ofGeophysical Research: Biogeosciences 126, e2020JG006089. doi: 10.1029/2020JG006089
Greer A. T., Boyette A. D., Cruz V. J., Cambazoglu M. K., Dzwonkowski B., Chiaverano L. M., et al. (2020). Contrasting fine-scale distributional patterns of zooplankton driven by the formation of a diatom-dominated thin layer. Limnology Oceanography 65, 2236–2258. doi: 10.1002/lno.11450
Greer A., Shiller A., Hofmann E., Wiggert J., Warner S., Parra S., et al. (2018). Functioning of coastal river-dominated ecosystems and implications for oil spill response: from observations to mechanisms and models. Oceanography 31, 90–103. doi: 10.5670/oceanog.2018.302
Humborg C., Conley D. J., Rahm L., Wulff F., Cociasu A., Ittekkot V. (2000). Silicon retention in river basins: far-reaching effects on biogeochemistry and aquatic food webs in coastal marine environments. Ambio 29, 45–50. doi: 10.1579/0044-7447-29.1.45
Humborg C., Ittekkot V., Cociasu A., Vonbodungen B. (1997). Effect of Danube river dam on black Sea biogeochemistry and ecosystem structure. Nature 386, 385–388. doi: 10.1038/386385a0
Kemp E. J., Roseburrough R. R., Elliott E. A., Krause J. W. (2021). Spatial variability of sediment amorphous silica and its reactivity in a northern gulf of Mexico estuary and coastal zone. Gulf Caribbean Res. 32, SC6–SC11. doi: 10.18785/gcr.3201.14
Kolker A. S., Allison M. A., Hameed S. (2011). An evaluation of subsidence rates and sea-level variability in the northern gulf of Mexico. Geophysical Res. Lett. 38, L21404. doi: 10.1029/2011GL049458
Krause J. W., Brzezinski M. A., Baines S. B., Collier J. L., Twining B. S., Ohnemus D. C. (2017). Picoplankton contribution to biogenic silica stocks and production rates in the Sargasso Sea. Global Biogeochemical Cycles 31, 762–774. doi: 10.1002/2017GB005619
Krause J. W., Brzezinski M. A., Goericke R., Landry M. R., Ohman M. D., Stukel M. R., et al. (2015). Variability in diatom contributions to biomass, organic matter production, and export across a frontal gradient in the California current ecosystem. J. Geophysical Research: Oceans 120, 1032–1047. doi: 10.1002/2014JC010472
Krause J. W., Brzezinski M. A., Jones J. L. (2011). Application of low level beta counting of 32Si for the measurement of silica production rates in aquatic environments. Mar. Chem. 127, 40–47. doi: 10.1016/j.marchem.2011.07.001
Krause J. W., Lomas M. W., Danielson S. (2021). Diatom growth, biogenic silica production, and grazing losses to microzooplankton during spring in the northern Bering and chukchi seas. Deep-Sea Res. II 191-192, 104950. doi: 10.1016/j.dsr2.2021.104950
Krause J. W., Nelson D. M., Lomas M. W. (2009). Biogeochemical responses to late-winter storms in the Sargasso Sea, II: increased rates of biogenic silica production and export. Deep-Sea Res. I 56, 861–874. doi: 10.1016/j.dsr.2009.01.002
Lehrter J. C., Beddick D. L. Jr., Devereux R., Yates D. F., Murrell M. C. (2012). Sediment-water fluxes of dissolved inorganic carbon, O2, nutrients, and N2 from the hypoxic region of the Louisiana continental shelf. Biogeochemistry 109, 233–252. doi: 10.1007/s10533-011-9623-x
Lehrter J. C., Murrell M. C., Kurtz J. C. (2009). Interactions between freshwater input, light, and phytoplankton dynamics on the Louisiana continental shelf. Continental Shelf Res. 29, 1861–1872. doi: 10.1016/j.csr.2009.07.001
Lohrenz S. E., Fahnenstiel G. L., Redalje D. G., Lang G. A., Chen X., Dagg M. J. (1997). Variations in primary production of northern gulf of Mexico continental shelf waters linked to nutrient inputs from the Mississippi river. Mar. Ecol. Prog. Ser. 155, 45–54. doi: 10.3354/meps155045
Lomas M. W., Baer S. E., Acton S., Krause J. W. (2019). Pumped up by the cold: elemental quotas and stoichiometry of polar diatoms. Front. Mar. Sci. 6, 286. doi: 10.3389/fmars.2019.00286
Lomas M. W., Lipschultz F., Nelson D. M., Krause J. W., Bates N. R. (2009). Biogeochemical responses to late-winter storms in the Sargasso Sea, I–pulses of primary and new production. Deep-Sea Res. I 56, 843–860. doi: 10.1016/j.dsr.2008.09.002
MacIntyre H. L., Stutes A. L., Smith W. L., Dorsey C. P., Abraham A., Dickey R. W. (2011). Environmental correlates of community composition and toxicity during a bloom of pseudo-nitzschia spp. in the northern gulf of Mexico. J. Plankton Res. 33, 273–295. doi: 10.1093/plankt/fbq146
McManus M. A., Sevadjian J. C., Benoit-Bird K. J., Cheriton O. M., Timmerman A. H., Waluk C. M. (2012). Observations of thin layers in coastal Hawaiian waters. Estuaries coasts 35, 1119–1127. doi: 10.1007/s12237-012-9497-8
McNair H. M., Brzezinski M. A., Krause J. W. (2018). Diatom populations in an upwelling environment decrease silica content to avoid growth limitation. Environ. Microbiol. 20, 4184–4193. doi: 10.1111/1462-2920.14431
Milliman J. D., Farnsworth K., Jones P., Xu K., Smith L. (2008). Climatic and anthropogenic factors affecting river discharge to the global ocean 1951–2000. Global Planetary Change 62, 187–194. doi: 10.1016/j.gloplacha.2008.03.001
Nelson D. M., Dortch Q. (1996). Silicic acid depletion and silicon limitation in the plume of the Mississippi river: evidence from kinetic studies in spring and summer. Mar. Ecology-Progress Ser. 136, 163–178. doi: 10.3354/meps136163
Nelson D. M., Goering J. J., Boisseau D. W. (1981). “Consumption and regeneration of silicic acid in three coastal upwelling systems,” in Coastal upwelling. Ed. Richards F. A. (Washington, D.C.: American Geophysical Union), 242–256.
Officer C. B., Ryther J. H. (1980). The possible importance of silicon in marine eutrophication. Mar. Ecol. Prog. Ser. 3, 83–91. doi: 10.3354/meps003083
Parsons M. L., Dortch Q., Turner R. E. (2002). Sedimentological evidence of an increase in pseudo-nitzschia (Bacillariophyceae) abundance in response to coastal eutrophication. Limnology Oceanography 47, 551–558. doi: 10.4319/lo.2002.47.2.0551
Pennock J. R., Boyer J. N., Herrera-Silveira J. R., Iverson R. L., Whitledge T. E., Mortazavi B., et al. (1999). “Nutrient behavior and phytoplankton production in gulf of Mexico estuaries,” in Biogeochemistry of gulf of Mexico estuaries. Eds. Bianchi T. S., Pennock J. R., Twiley R. R. (New York: John Wiley & Sons), 109–162.
Pickering R. A., Cassarino L., Hendry K. R., Wang X. L., Maiti K., Krause J. W. (2020). Using stable isotopes to disentangle marine sedimentary signals in reactive silicon pools. Geophysical Res. Letters 47, e2020GL087877. doi: 10.1029/2020GL087877
Rabalais N. N., Turner R. E., Dortch Q., Justic D., Bierman V. J., Wiseman W. J. (2002). “Nutrient-enhanced productivity in the northern gulf of Mexico: past, present and future,” in Nutrients and eutrophication in estuaries and coastal waters (Dordrecht: Springer), 39–63.
Rabalais N. N., Turner R. E., Sen Gupta B. K., Platon E., Parsons M. L. (2007). Sediments tell the history of eutrophication and hypoxia in the northern gulf of Mexico. Ecol. Appl. 17, S129–S143. doi: 10.1890/06-0644.1
Ragueneau O., Conley D. J., Leynaert A., Longphuirt S. N., Slomp C. P., Ittekkot V., et al. (2006). “Responses of coastal ecosystems to anthropogenic perturbations of silicon cycling,” in The silicon cycle : human perturbations and impacts on aquatic systems. Eds. Ittekkot V., Unger C., Humborg N., Tac An (Scientific Committee on Problems of the Environment (SCOPE) Series 66), 197–213.
Ragueneau O., Savoye N., Del Amo Y., Cotten J., Tardiveau B., Leynaert A. (2005). A new method for the measurement of biogenic silica in suspended matter of coastal waters: using Si: Al ratios to correct for the mineral interference. Continental Shelf Res. 25, 697–710. doi: 10.1016/j.csr.2004.09.017
Sanial V., Shiller A. M., Joung D., Ho P. (2019). Extent of Mississippi river water in the Mississippi bight and Louisiana shelf based on water isotopes. Estuarine Coast. Shelf Sci. 226, 106196. doi: 10.1016/j.ecss.2019.04.030
Schelske C. L., Conley D. J., Stoermer E. F., Newberry T. L., Campbell C. (1987). “Biogenic silica and phosphorus accumulation in sediments as indices of eutrophication in the laurentian great lakes,” in Paleolimnology IV (Dordrecht: Springer), 79–86.
Schlitzer R. (2016). Ocean data view. Available at: https://odv.awi.ed.
Stumpf R. P., Gelfenbaum G., Pennock J. R. (1993). Nutrient flux and physical stability drive phytoplankton biomass variability along the Alabama shelf [Data set]. Continental Shelf Res. 13, 1281–1301. doi: 10.1016/0278-4343(93)90053-Z
Sutton J. R., Dzwonkowski B., Krause J. W., Hernandez F. J., Graham W. M. (2023). Nutrient flux and physical stability drive phytoplankton biomass variability along the Alabama shelf [Data set] (Dauphin Island Sea Lab, Dauphin Island, AL). doi: 10.57778/MCG4-F330
Sylvan J. B., Dortch Q., Nelson D. M., Brown A. F. M., Morrison W., Ammerman J. W. (2006). Phosphorus limits phytoplankton growth on the Louisiana shelf during the period of hypoxia formation. Environ. Sci. Technol. 40, 7548–7553. doi: 10.1021/es061417t
Tao B., Tian H., Ren W., Yang J., Yang Q., He R., et al. (2014). Increasing Mississippi river discharge throughout the 21st century influenced by changes in climate, land use, and atmospheric CO2. Geophysical Res. Lett. 41, 4978–4986. doi: 10.1002/2014GL060361
Turner R. E., Qureshi N., Rabalais N. N., Dortch Q., Justic D., Shaw R. F., et al. (1998). Fluctuating silicate : nitrate ratios and coastal plankton food webs. Proc. Natl. Acad. Sci. USA 95, 13048–13051. doi: 10.1073/pnas.95.22.13048
Turner R. E., Rabalais N. N. (1991). Changes in Mississippi river water-quality this century. Bioscience 41, 140–147. doi: 10.2307/1311453
Turner R. E., Rabalais N. N. (1994a). “Changes in the Mississippi river nutrient supply and offshore silicate-based phytoplankton community responses,” in Changes in fluxes in estuaries: implications from science to management. Eds. Dyer K. R., Orth R. J. (Fredesnborg: Olsen & Olsen), 485.
Turner R. E., Rabalais N. N. (1994b). Coastal eutrophication near the Mississippi river delta. Nature 368, 619–621. doi: 10.1038/368619a0
Keywords: biogenic silica production, Gulf of Mexico, Louisiana Shelf, Mississippi Bight, eutrophication, diatom
Citation: Krause JW, Boyette AD, Marquez IA, Pickering RA and Maiti K (2023) Drivers of diatom production and the legacy of eutrophication in two river plume regions of the northern Gulf of Mexico. Front. Mar. Sci. 10:1162685. doi: 10.3389/fmars.2023.1162685
Received: 09 February 2023; Accepted: 31 May 2023;
Published: 05 July 2023.
Edited by:
Alex J. Poulton, Heriot-Watt University, United KingdomReviewed by:
Wei Liu, Wenzhou Vocational College of Science and Technology, ChinaArnaud Laurent, Dalhousie University, Canada
Copyright © 2023 Krause, Boyette, Marquez, Pickering and Maiti. This is an open-access article distributed under the terms of the Creative Commons Attribution License (CC BY). The use, distribution or reproduction in other forums is permitted, provided the original author(s) and the copyright owner(s) are credited and that the original publication in this journal is cited, in accordance with accepted academic practice. No use, distribution or reproduction is permitted which does not comply with these terms.
*Correspondence: Jeffrey W. Krause, amtyYXVzZUBkaXNsLmVkdQ==
†Present addresses: Adam D. Boyette, Naval Oceanographic Office, Stennis Space Center, MS, United States
Rebecca A. Pickering, Department of Geology, Lund University, Lund, Sweden