- 1Department of Chemistry, Umeå University (UmU), Umeå, Sweden
- 2Department of Ecology & Environmental Science, UmU, Umeå, Sweden
- 3Umeå Marine Science Centre, UmU, Hörnefors, Sweden
- 4Swedish Environmental Research Institute (IVL), Gothenburg, Sweden
- 5Dean’s Office, Faculty of Medicine, UmU, Umeå, Sweden
Introduction: Thousands of halogenated natural products (HNPs) are generated in the ocean and on land. A subset of these, halomethoxybenzenes (HMBs), are released from both natural and anthropogenic sources. Here we consider: 1. Brominated anisoles (BAs), transformation products of bromophenols. 2. Drosophilin A methyl ether (DAME: 1,2,4,5-tetrachloro-3,6-dimethoxybenzene), a secondary metabolite of terrestrial fungi. 3. Tetrachloroveratrole (TeCV: 1,2,3,4-tetrachloro-5,6-dimethoxybenzene), a lignin byproduct found in bleached kraft mill effluent. 4. Pentachloroanisole (PeCA), a metabolite of the wood preservative pentachlorophenol.
Methods: We examined several ecosystem compartments to determine sources and exchange processes for these HMBs: air, precipitation, rivers, forest fungi and litter, and water from northern Baltic estuaries and offshore. Samples were analyzed for HMBs by capillary gas chromatography – quadrupole mass spectrometry.
Results and discussion: All four types of HMBs were found in air, and BAs, DAME and TeCV were also present in precipitation. BAs and DAME were common in rivers and estuaries, whereas TeCV was low and PeCA was below detection. DAME was identified in several species of fungi and in forest litter; TeCV was occasionally present, but BAs and PeCA were below detection. Concentrations of BAs were higher in estuaries than in rivers or offshore waters, showing that estuaries are hot spots for production. BAs were negatively or not correlated with chlorophyll-a, suggesting contribution by heterotrophic bacteria as well as known production by phytoplankton and macroalgae. DAME was negatively or not correlated with BAs and did not appear to be produced in the estuaries; fungi and forest litter containing fungal mycelia are suggested as sources. HMBs volatilize from sea and land, disperse through the atmosphere, and return via precipitation and rivers. Production and biogeochemical cycles are influenced by climate change and we suggest BAs and DAME for following partitioning and exchange processes.
Highlights:
● Natural HMBs bromoanisoles (BAs) and DAME (drosophin A methyl ether) were measured in ecosystem compartments supplying Baltic estuaries.
● Phytoplankton and macroalgae are known producers of BAs in estuaries and bacteria may contribute.
● DAME is produced by terrestrial fungi, with river transport to estuaries.
● These HMBs are useful for following partitioning and exchange processes.
1 Introduction
Thousands of halogenated natural products (HNPs) are generated in the ocean and on land, and their production and cycling are likely to be impacted by climate change (Bidleman et al., 2020). Halomethoxybenzenes (HMBs) are a subset of HNPs which have both natural and anthropogenic origins (Führer and Ballschmiter, 1998). Dibromo- and tribromoanisoles (DiBA and TriBA) result from bacterial O-methylation of precursor bromophenols (BPs), produced by marine organisms, chlorination of wastewater and industrial activities (Allard et al., 1987; Howe et al., 2005; Koch and Sures, 2018). Drosophilin A methyl ether (DAME: 1,2,4,5-tetrachloro-3,6-dimethoxybenzene), chlorinated anisoles and related compounds are secondary metabolites of terrestrial fungi (DeJong and Field, 1997; Teunissen et al., 1997; Garvie et al., 2015). Tetrachloroveratrole (TeCV: 1,2,3,4-tetrachloro-5,6-dimethoxybenzene) and other chloroveratroles (CVs) are metabolites of the chloroguiaicols formed in bleached kraft mill effluent (Neilson et al., 1983; Neilson et al., 1988; Brownlee et al., 1993). Pentachloroanisole (PeCA) is a metabolite of the wood preservative pentachlorophenol, but may also have unidentified natural sources (Kylin et al., 2017).
BAs and other haloanisoles are undesireable “taste and odor” compounds, arising from the disinfection of drinking water containing halogens (Diaz et al., 2005). They cause “cork taint” in wines (Chatonnet et al., 2004; Alañón et al., 2020), and mustiness in packaged food (Whitfield et al., 1997) and water (Brownlee et al., 1993). On the other hand, BPs are key flavor components in seafood (Chung et al., 2003), and algae are being considered as food additives and in aquaculture to take advantage of BPs and other flavor-enhancing components (Jones et al., 2016; Francezon et al., 2021). The precursor of DAME, drosophilin A (DA = 2,3,5,6-tetrachloro-4-methoxyphenol), has been reported in the meat of wild boar (Sus scrofa), apparently due to consuming fungi (Hiebl et al., 2011). Both DAME and DA have antibiotic activity (Kavanaugh et al., 1952; Teunissen et al., 1997). Neilson et al. (1984) reported threshold toxic concentrations and sublethal effects of several HMBs, including TeCV, on zebrafish (Brachydanio rerio) embryos and larvae. DAME and TriBA have been found in fish from the Laurentian Great Lakes of North America (Renaguli et al., 2020).
In previous papers, we reported BAs (Bidleman et al., 2017a; Bidleman et al., 2017b; Bidleman et al., 2023), DAME, TeCV and PeCA (Bidleman et al., 2023) in air and precipitation samples collected at Råö on the Swedish west coast and at an inland station Pallas in Subarctic Finland. The relative abundance of these compounds in air was ΣBAs (2,4-DiBA + 2,4,6-TriBA) > DAME > TeCV > PeCA at Råö, and DAME > ΣBAs > TeCV > PeCA at Pallas. These spatial patterns were consistent with marine sources for BAs and terrestrial sources for DAME, while the origins of the TeCV and PeCA were unclear. No temporal trends in air were evident for any HMB compound from 2002-2019. Concentrations in precipitation appeared to be controlled by Henry’s law scavenging of the gas-phase compound. A terrestrial source for DAME was suggested by its presence in several species of fungi, gathered from Swedish forests, and by literature reports of DAME in other fungal species worldwide.
Findings of HMBs in air and precipitation of coastal and inland regimes prompted further examination of their transport and exchange between the marine and terrestrial environment. Here for the first time, we report concentrations and linkages of BAs, DAME and TeCV in air, rivers and northern Baltic estuaries and discuss land-sea-air exchange processes. Climate change is producing profound alterations in the Northern Baltic ecosystem, driven by reduced ice cover and greater runoff of terrestrial dissolved organic carbon (DOC), one consequence being a shift from a phytoplankton-based food web to one based on heterotrophic bacteria (Andersson et al., 2015). In addition to supplying food for bacteria (Figueroa et al., 2021), terrestrial DOC sorbs anthropogenic persistent organic pollutants and other compounds of concern (Ripszam et al., 2015), thus influencing their food web bioaccumulation (Andersson et al., 2015). Along with DOC, HMBs provide tracers of land-sea transfer. Because the HMBs studied here are hydrophobic and accumulate in fish (Renaguli et al., 2020), they may be useful for studying bioaccumulation processes in the nearshore zone. As climate-induced changes bring more terrestrial DOC into the northern Baltic, these processes are likely to be even more relevant in the future.
2 Materials and methods
2.1 Air and precipitation sampling
Collection of air and precipitation samples at Pallas (68°0.0 N, 24°13.8 E) and Råö (57°23.622 N, 11°54.852 E) (Figures 1A, B) (Supplementary Material, S1) was done by the Swedish Environmental Research Institute (IVL). Samples were processed in their laboratory and cleaned extracts were sent to Umeå University (UmU) for analysis of HMBs (Bidleman et al., 2023).
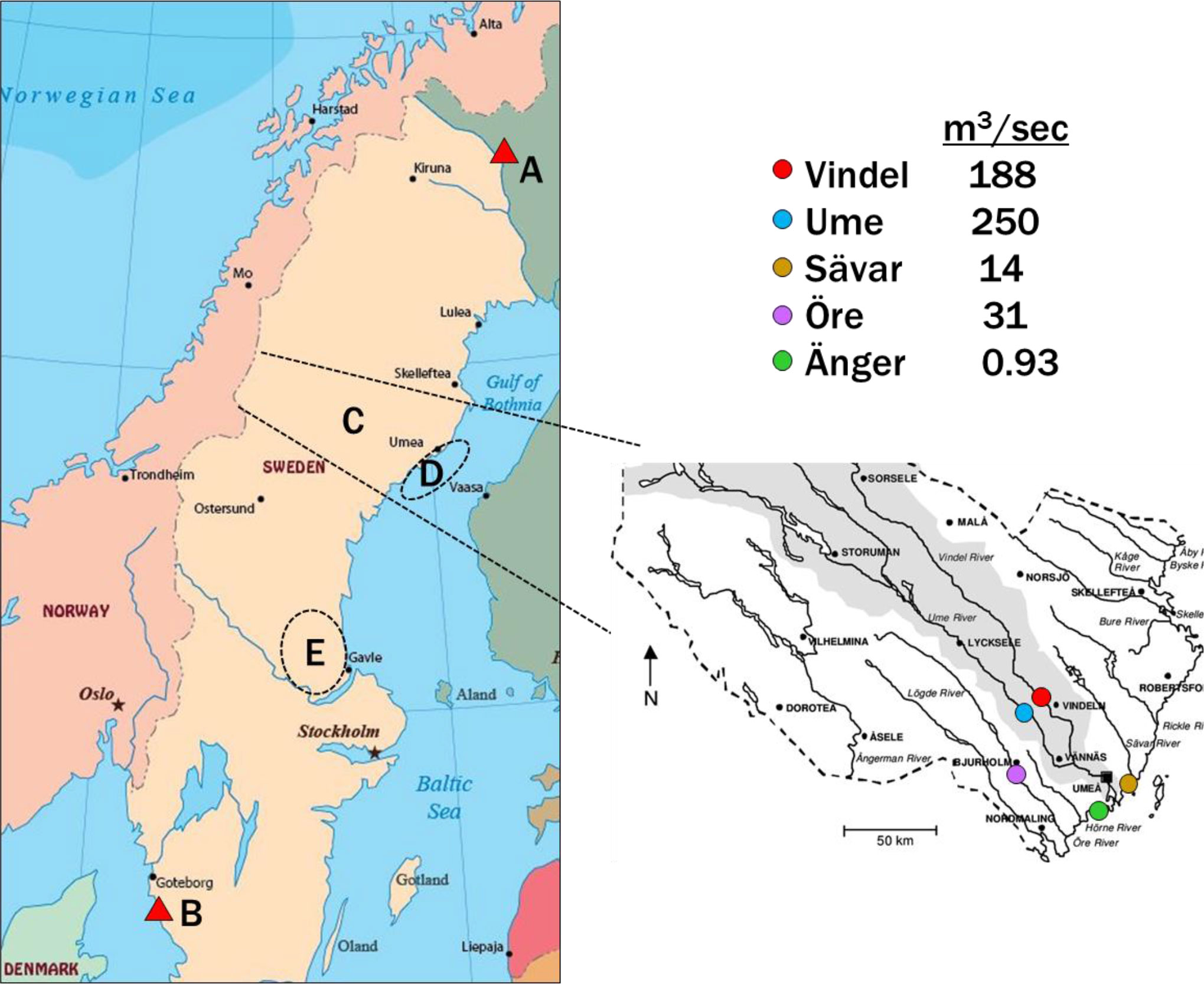
Figure 1 Locations for sample collection. Air at Pallas (A) and Råö (B), rivers (C), estuaries and offshore water (D, “The Quark”) and terrestrial fungi (C, E). The rivers are shown on the side map of Västerbotten County, with their mean flow rates modeled from 2017-2021 (SMHI). Expansion of the estuaries region (D) is shown in Figure S1 and Guo et al. (2022).
2.2 River, estuary and offshore water sampling
River water was collected periodically in Västerbotten County, Sweden, usually soon after the spring flood, but occasionally in fall (S1, Table S1). Five rivers (Vindel, Ume, Sävar, Öre and Änger) were sampled in 2017, 2018, 2019 and 2022, and are shown in Figure 1, Area C, along with their mean flow rates, modeled from 2017-2021 (Swedish Meteorological and Hydrological Institute (SMHI), 2022). Total samples were 5-6 for the first four rivers and 2 for the Änger.
Estuaries Kalvarskatan (KAL, 63°36.072 N, 19°53.140 E) and Ängerån (ÄNG, 63°34.400 N, 19°50.666 E) were sampled during spring-summer in 2018, 2019, 2021 and 2022 (S1, Table S2). The area is shown as in Figure 1D, with more detail in Figure S1. Water was taken from the upper 0.5 m. KAL has no river, whereas ÄNG has a small river (Änger, Figure 1). Both are called “estuaries” throughout this paper. Water samples were collected at two other estuaries which have fresh water inputs intermediate of KAL and ÄNG (Eriksson et al., 2022; Guo et al., 2022): Stadsviken (SVK, 63°33.026 N, 19°47.647 E) and Valviken (VVK, 63°32.468 N, 19°46.725 E), offshore of Hörnefors (HÖRN, 63°33.852 N, 19°56.226 E), in Örefjärden sound (ÖREFJ, 63°31.320 N, 19°48.276 E) and at the island group Holmön (63°47.598 N, 20°52.068 E) in 2018-2019 (Figure S1; Table S4). The Holmön samples were taken offshore of Bergudden Light (HOLBER), and in a shallow strait Sörsundet (HOLSÖR), which separates the two main islands.
Water was collected in 20-L stainless steel cans, 3-5 L was filtered through glass fiber filters and the HMBs were sorbed on solid-phase extraction cartridges (Supplementary S1). Samples were processed within five days of collection to minimize changes in TriBA content (Supplementary S5).
2.3 Phytoplankton sampling
Phytoplankton was collected during bloom periods in May and September 2019 and August 2020 (Supplementary S1). The collections were made at the ÖREFJ station (see above). The phytoplankton in May consisted mainly of diatoms (Skeletonema marinoi, Chaetoceros wighamii), while August and September collections were dominated by the cyanobacterium Aphanizominon flos-aquae. Water samples were taken from within and below the A. flos-aquae blooms.
2.4 Fungi and forest litter sampling
Fungi and coniferous, deciduous or mixed litter were gathered in fall from forests in Västerbotten and Gävleborg Counties, Sweden (Figure 1, Areas C and E), and were frozen until analysis. They were collected and processed as described in Supplementary S1.
2.5 Chemical analysis
Sources of supplies for sample collection and analysis are reported in Supplementary S2. Samples were analyzed for the HMB compounds 2,4-DiBA, 2,4,6-TriBA, DAME, TeCV and PeCA using capillary gas chromatography- quadrupole mass spectrometry (GC-MSD) (Supplementary S3), monitoring the selected ions in Table S5. Quality control information is reported in Supplementary S4. In addition to HMBs, properties measured in estuarine water were temperature (TMP), salinity (SAL), chlorophyll-a (CHL) and dissolved organic carbon (DOC). Methods for these are outlined in Table S6.
2.6 Data analysis
Statistical analysis (regressions, t-tests, etc.) was done using Excel Version 2302 and XLSTAT 2022, and Prism (GraphPad).
3 Results and discussion
3.1 Air and precipitation
Mean concentrations of HMBs in air and precipitation at Råö and Pallas for 2018-2019 are given in Table 1. Monitoring was also conducted in earlier years and all air and precipitation results are reported in Bidleman et al. (2017a); Bidleman et al. (2023). In these studies, only the polyurethane foam (PUF) trap was analyzed for air samples, and corrections were made for incomplete collection due to breakthrough of gas-phase compounds. As noted in Section 1, the order of abundance of HMBs in air at Råö was ΣBAs > DAME > TeCV > PeCA, whereas at Pallas the order was DAME > ΣBAs > TeCA > PeCA. The same abundance order was also found in precipitation, with the exception that PeCA was generally below detection. Mean concentrations of DAME in air at Råö and Pallas were not significantly different (p >0.05, Welch’s t-test of means), whereas precipitation at Pallas showed higher levels of DAME compared to Råö (p = 0.012). Lower abundance of BAs at Pallas can be explained by its remoteness from marine regions (Bidleman et al., 2023).
The apparent enthalpy of surface-to-air exchange (ΔSAH, kJ mol-1) at Råö and Pallas was calculated from Clausius-Clapeyron (CC) plots of log partial pressure in air (Pair/Pa) versus reciprocal temperature:
where R = 8.314 Pa m3 mol-1 K-1 (Bidleman et al., 2023). By comparing observed slopes with those of HMB physicochemical properties (vapor pressure, octanol-air partition coefficient, Henry’s law constant), we found that apparent ΔSAH for TriBA (but not DiBA), DAME and TeCV at Råö were within one standard deviation of property values. At Pallas, ΔSAH was also within one standard deviation of property values for DAME, but not for either BA. Values of ΔSAH that are similar to those of physicochemical properties imply local or regional surface-air exchange (e.g., with soil, vegetation, water) whereas relatively flat slopes of CC plots and low values of ΔSAH are expected for compounds which arrive via long-range transport and show little interaction with local/regional surfaces (Hoff et al., 1998; Wania et al., 1998).
Our interpretation of these observations is that TriBA at Råö is influenced by exchange with coastal seawater, but sources for DiBA may be more dispersed over wider areas of the ocean. Differences between the two BAs might be explained by higher concentrations of TriBA versus DiBA in coastal macroalgae (Bidleman et al., 2023), although we discuss in Section 3.4.1 that BA proportions in water are not necessarily the same as those in macroalgae. BAs have not been reported in Atlantic seawater off the Swedish west coast. DAME may be influenced by exchange with land surfaces around Råö, a conclusion supported by findings of DAME in terrestrial fungi and forest litter (Section 3.3), but a marine contribution is not ruled out, since DAME has been reported in open-ocean seawater (Schreitmüller and Ballschmiter, 1994; Schreitmüller and Ballschmiter, 1995) and offshore water in the northern Baltic (Section 3.5). Being a coastal site, Råö is influenced by alternating land and sea breezes. BAs showed no evidence of local exchange at Pallas, which is expected from its distance from marine sources. However, ΔSAH for DAME at Pallas was suggestive of contributions from the regional (terrestrial) environment (Bidleman et al., 2023).
Mean atmospheric concentrations of HMBs at our stations are compared in Figure 2 with other measurements made in the northern Baltic (Bidleman et al., 2015), Norway (Bohlin-Nizzetto et al., 2021), and the Canadian Arctic (Su et al., 2008; Wong et al., 2011). TriBA concentrations at Pallas and Norway (Svalbard) were similar to each other and lower than those found in the Canadian Archipelago. TeCV concentrations at Pallas were about twice those reported at circumpolar Arctic stations in 2000-2003 (Su et al., 2008). The mean PeCA concentration at Pallas was similar to that reported at Alert, Canada (Wong et al., 2021). DAME has not been reported at other Arctic-Subarctic stations. DAME concentrations of 2-195 pg m-3 have been found at island stations and over the open ocean (Atlas et al., 1986; Wittlinger and Ballschmiter, 1990; Schreitmüller and Ballschmiter, 1994; Schreitmüller and Ballschmiter, 1995).
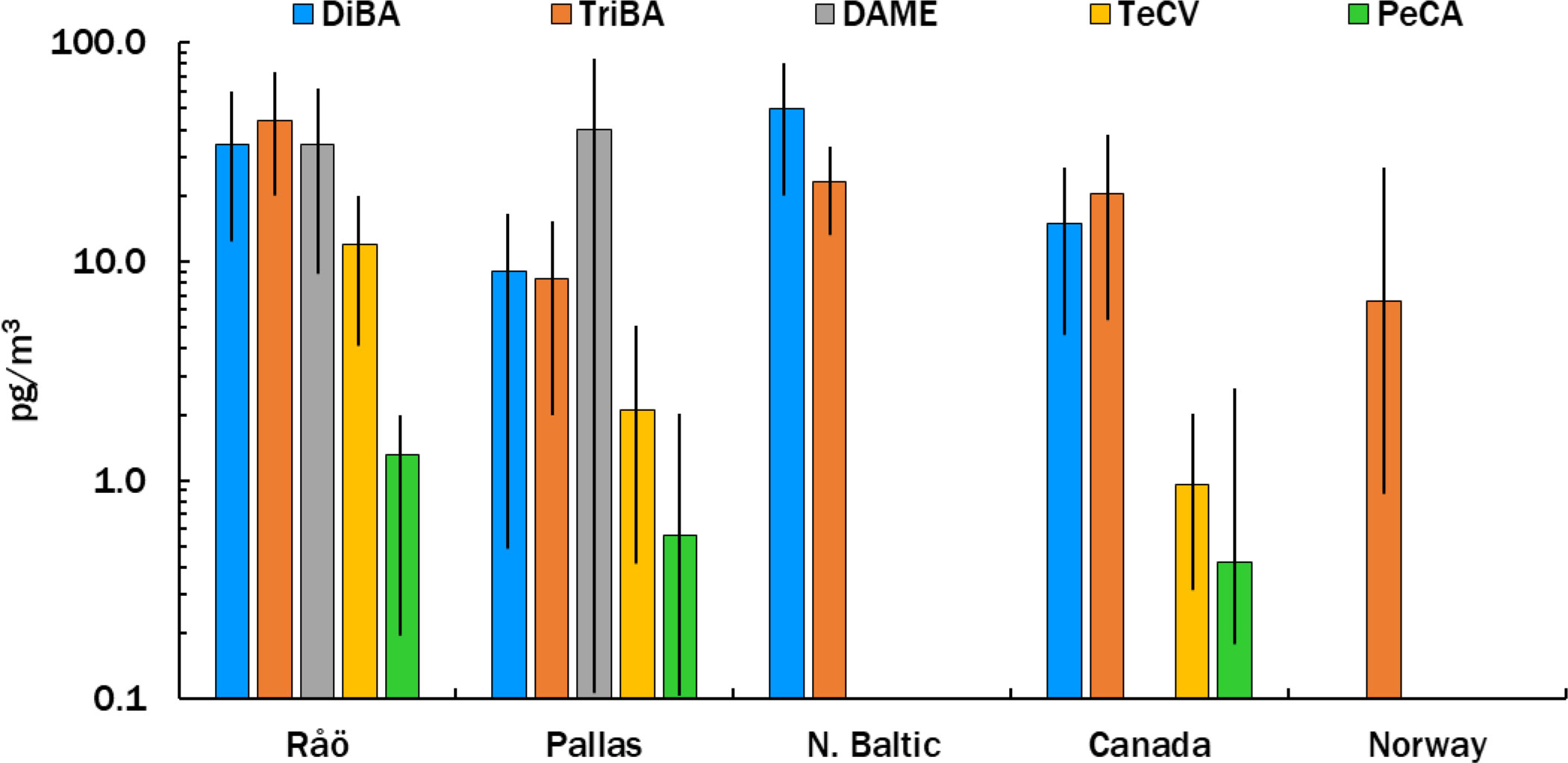
Figure 2 Mean ± SD concentrations of HMBs in air at Råö and Pallas (this study) and the Northern Baltic (Bidleman et al., 2015), compared to reported levels at Canadian (Su et al., 2008; Wong et al., 2011; Wong et al., 2021) and Norwegian (Bohlin-Nizzetto et al., 2021) locations. Variations for PeCA in Canada and TriBA in Norway are shown as ranges.
3.2 Rivers
Mean concentrations of HMBs in rivers are given in Table 2, with details in Table S1. DiBA and TriBA were determined in all samples, DAME and TeCV in only some of them. DAME was the most abundant compound, with a mean concentration over all rivers 5-8 times higher than those of DiBA, TriBA or TeCV. PeCA was generally below detection. No significant correlations were found among the compounds (p >0.05). No differences were evident across the rivers, with the exception that the mean concentration of TriBA in the Öre River (16 ± 5 pg L-1, n=5) was lower than the average of the other four rivers (44 ± 22 pg L-1, n=19) (p<0.001).
Suspected sources of HMBs to the rivers are atmospheric deposition to and drainage from their watersheds. We examined these possibilities by comparing the ratios of mean concentrations of HMBs in rivers (Table S1) to those in precipitation at Råö and Pallas (Table 1). A limitation is that both atmospheric sites are quite distant from the river locations (Figure 1). River/precipitation (R/P) ratios, calculated by assuming mean river concentrations and different scenarios of precipitation contribution, are presented in Table S7. R/P ratios in Scenario 1 assume the Råö precipitation concentrations and are 1.2-3.2 for BAs, 2.0 for TeCV and 9.0 for DAME. Differences in the R/P ratios are obtained if based on precipitation concentrations from Pallas (Scenario 2): 3.1-4.2 for BAs, 6.7 for TeCV and 4.6 for DAME, or averaged precipitation concentrations from both sites (Scenario 3): 1.7-3.5 for BAs, 3.0 for TeCV and 6.1 for DAME. The Västerbotten region (Figure 1C) receives air parcels from all sectors, including from the North Atlantic across Sweden (Newton et al., 2014). Concentrations of BAs at northern Baltic stations, measured in 2011-2012, show the influence of this pathway as well as regional emissions from the Baltic Sea (Bidleman et al., 2015; Bidleman et al., 2017b). Average concentrations of BAs in northern Baltic air, measured in 2011-2012 (23 pg m-3 DiBA, 50 pg m3 TriBA) (Bidleman et al., 2015), are more similar to those at Råö than at Pallas (Figure 2). Based on this consideration, the R/P ratios based on Scenario 1 (precipitation concentrations at Råö) are probably the best of the three estimates of R/P ratios in the Västerbotten region.
An R/P = 1 implies that the precipitation directly drives HMB concentrations in the rivers, whereas R/P >1 suggests additional sources; i.e., runoff from within the watershed. Since R/P >1 for all HMBs and in all scenarios (Table S7), some contribution from within the watershed seems likely. Results from the Scenario 1 calculations suggest greater watershed contribution for DAME than the BAs or TeCV. Atmospheric sources to the watershed are precipitation and atmospheric deposition of gaseous HMBs scavenged by the “forest filter effect” (McLachlan and Horstmann, 1998; Su et al., 2007; Gong et al., 2021). DAME is produced by fungi (Section 3.3) and enters rivers via stream and groundwater drainage from the terrestrial environment, as well as being delivered by air transport from other regions. Transport to the estuaries takes place by rivers and atmospheric deposition.
3.3 Terrestrial fungi and forest litter
Fruiting bodies of terrestrial fungi (n=19) and litter/underlying humus samples (n=6) were screened for DAME and TeCV. Our method has a limit of detection (LOD) of 0.0016 mg kg-1 (S4 Section 4). DAME in four species ranged from 0.3-3.8 mg kg-1 fresh weight (fw): Micromphale perforans (a saprotroph), Stereum hirsutum (a white rot), Thelephora terrestris (an ectomycorrhiza) and Hydnellum mirabile.(an ectomycorrhiza). Four others (Stereum subtomentosum (white rot), Kuehneromyces mutabilis (saprotroph), Cladonia stellaris (lichen), and Jackrogersella multiformis (soft rot, intermediate between white and brown rot) contained 0.015-0.053 mg kg-1 fw. The litter/humus samples, which were presumably permeated with fungal mycelia, contained 0.006-1.6 mg kg-1 fw.
Only a few samples contained detectable levels of TeCV, 0.0033 and 0.039 mg kg-1 fw in M. perforans and T. terrestris, respectively, and 0.0033-0.016 mg kg-1 fw in litter/humus (n=3). The low occurrence of TeCV in fungi and litter is surprising, given its frequency of detection in air and precipitation; however, we note that the number of fungi species screened here is small compared to their diversity. BAs and PeCA were not detected in fungi nor in litter/humus.
The reported range of total chlorine in forest soils of Sweden is 16-458 mg kg-1 dry weight, of which two-thirds or more is organically bound (Svensson et al., 2022). The balance between organic and inorganic chlorine is maintained by rates of chlorination and dechlorination (Svensson et al., 2021). Chlorination of organic matter takes place most actively in the rhizosphere and is carried out by diverse organisms, including bacteria, fungi and vascular plants, as well as by abiotic processes (Öberg and Bastviken, 2012; Montelius et al., 2019; Svensson et al., 2022). DeJong and Field (1997) describe several classes of chlorinated fungal metabolites produced by basidiomycetes: amino acids, anisyls, hydroquinones, orsinols, orsellinate sesquiterpenes and others. We found the chlorinated hydroquinone DAME, and it is likely that the forests are also sources of these other fungal metabolite classes. Some of these have been described as “fungal volatiles” (Dickschat, 2017), implying that they are available for atmospheric transport. Degradation pathways also exist for these compounds; e.g., mineralization and biotransformation in forest soils (DeJong and Field, 1997) and reductive dehalogenation in anaerobic sediments (Milliken et al., 2004), but we did not investigate these processes.
3.4 KAL and ÄNG estuaries
DiBA, TriBA and DAME were the main HMBs found in KAL and ÄNG water. TeCV was identified in a few samples at lower levels and PeCA was below detection. Concentrations are summarized in Table 3 and detailed for each sampling event in Tables S2, along with the other supporting chemical and biological factors listed in Table S6.
3.4.1 Bromoanisoles.
Mean concentrations in KAL were 428 ± 380 pg L-1 DiBA and 294 ± 153 pg L-1 TriBA (n=15), and in ÄNG 281 ± 164 pg L-1 DiBA and 242 ± 106 pg L-1 TriBA (n=5) (Table 3). Means in the northern and southern Baltic in 2011-2013 were 86 ± 51 pg L-1 DiBA and 199 ± 150 pg L-1 TriBA, for a set consisting of mostly open-sea samples (Bidleman et al., 2016). The time courses of BAs in the estuaries over the spring-summer are shown in Figures 3A, B, with fits to the curves in Table S3, where all years have been combined. The pattern for BAs in both estuaries was similar, lower concentrations in May-June, a rise in July-August and falling off in September. This seasonal rise-fall trend was noted for BAs, BPs and their transformation products hydroxylated and methoxylated bromodiphenyl ethers (OH-BDEs, MeO-BDEs) in Baltic macroalgae, amphipods and fish (Dahlgren et al., 2015; Dahlgren et al., 2016).
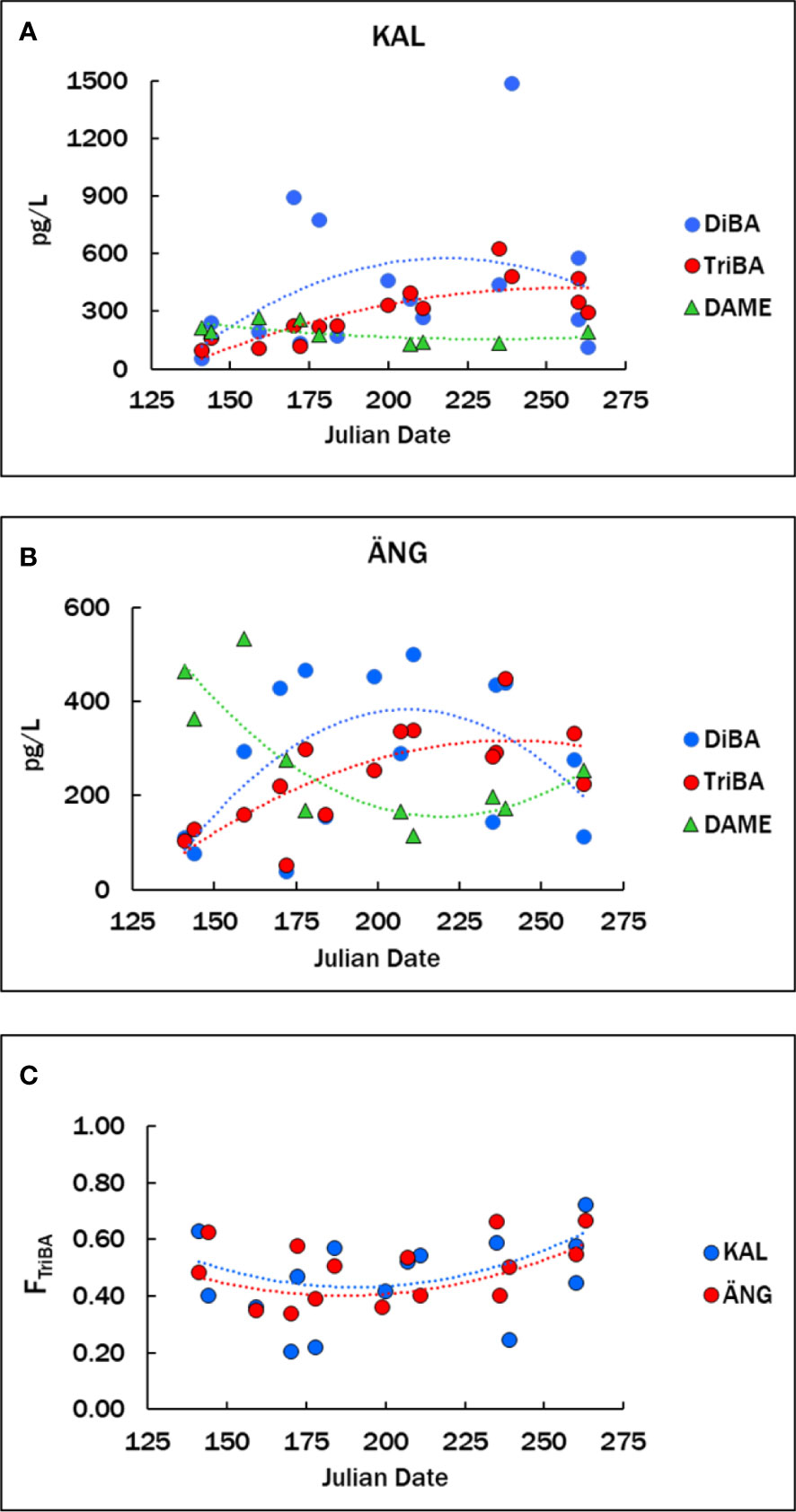
Figure 3 (A, B) Concentrations of BAs and DAME in the KAL and ÄNG estuaries from May to September. (C) Fraction of TriBA, FTriBA = TriBA/(DiBA + TriBA). Data for all years are plotted versus the Julian date of sampling. Numerical data are given in Table S2. Lines are second-order regressions, Y = aX2 + bX + c, with regression parameters in Table S3.
A study in which passive water samples were deployed on the Great Barrier Reef (GBR) from 2007-2013 showed mean concentrations of 450 pg L-1 DiBA and 170 pg L-1 TriBA (Vetter et al., 2018), within the range of those found in KAL and ÄNG. As in KAL, events of high DiBA on the GBR sometimes corresponded with high TriBA, and sometimes not. The precursor BPs were also found on the GBR. At 3840 pg L-1, the mean concentration of DiBP was over eight times the DiBA concentration, whereas the mean for TriBP (21 pg L-1) was lower than that of TriBA. The TriBP concentration was likely lower because of its near-complete dissociation in seawater, resulting in poor collection by the passive sampler (Vetter et al., 2009).
Correlations among the HMB compounds and other chemical and biological factors are summarized in Table S8, where significant relationships (p<0.05) are shown in red, positive correlations are in normal font and negative ones are italicized. DiBA and TriBA were positively and significantly correlated in ÄNG, but not in KAL, where occasional high spikes of DiBA were measured that did not correspond to high TriBA (Figure 3A). SAL and DOC were negatively correlated in both estuaries, more strongly in ÄNG due to delivery of terrestrial dissolved organic matter, which is typical of northern Baltic rivers (Figueroa et al., 2021; Guo et al., 2022). Plots of the BAs and DAME versus SAL, DOC and CHL are shown in Figure 4. TriBA was positively correlated to SAL in both estuaries. A rise in DiBA with SAL was suggested in both estuaries, but the correlations were not significant. DiBA and TriBA were negatively correlated to DOC in ÄNG, but not in KAL, which had a smaller variation in DOC. Both BAs were negatively correlated with CHL in ÄNG (more strongly for DiBA than TriBA) and not correlated with CHL in KAL.
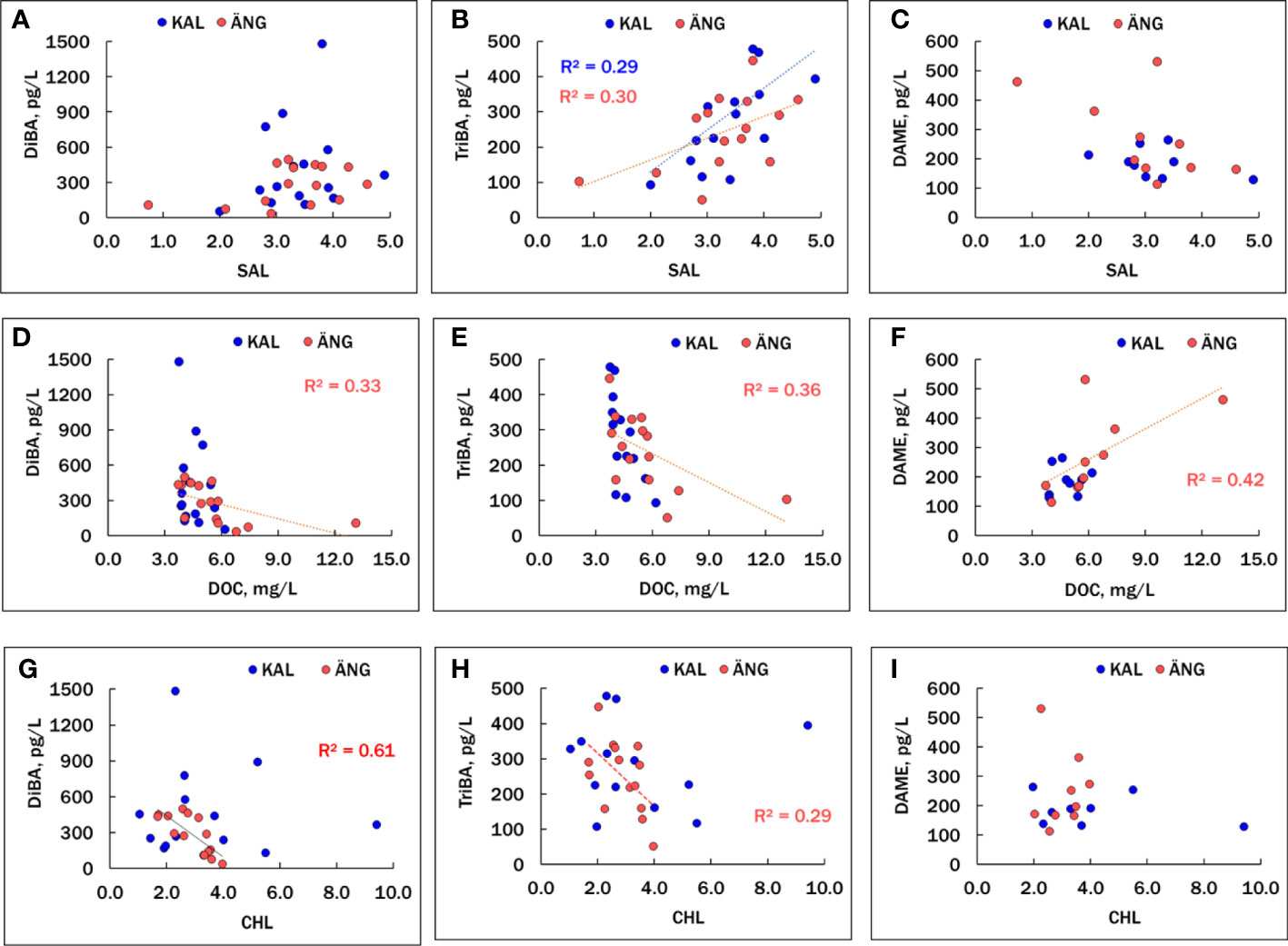
Figure 4 Associations of DiBA (A, D, G), TriBA (B, E, H) and DAME (C, F, I) with variables SAL, DOC and CHL in the KAL and ÄNG estuaries. Only significant (p<0.05) regressions are shown. Correlation coefficients (as r2) are given in Table S8.
Proportions of the two BAs in KAL and ÄNG water were expressed by the fraction FTriBA = TriBA/(DiBA + TriBA), which varied from about 0.2 to 0.7. FTriBA was slightly lower in midsummer than in spring or fall (Figure 3C). Average FTriBA were 0.46 ± 0.16 in KAL and 0.49 ± 0.11 in ÄNG. There were no significant differences between the two estuaries in mean BAs concentrations nor in mean FTriBA (Welch’s t-test, unequal variances, p >0.05). Variations in FTriBA suggest links to production mechanisms. In 16 macroalgae species from the Baltic, and Atlantic coasts of Sweden and Norway, TriBA exceeded DiBA with mean FTriBA = 0.75 ± 0.15 (Bidleman et al., 2019), and TriBA > DiBA in phytoplankton (see below). Thus, these photosynthesizers would be expected to release mainly TriBA to the estuaries, whereas similar abundances of the two BAs in the estuaries suggest additional sources.
Duplicate water samples were collected in September 2019 within and below a bloom of A. flos-aquae and showed the following mean concentrations. Within bloom: DiBA 128 ± 7 pg L-1, TriBA 232 ± 16 pg L-1, below bloom: DiBA 76 ± 23 pg L-1, TriBA 173 ± 13 pg L-1. Although suggestive of higher concentrations within the bloom, neither within-below set is significant. In August 2020, only one set of water samples was taken, with DiBA 49 pg L-1 and TriBA 228 pg L-1 within the bloom, and DiBA 24 pg L-1 and TriBA 198 pg L-1 below. Analysis of the A. flos-aquae for TriBA gave 3.5 ng g-1 fw in 2019 and 0.9 ng g-1 fw in 2020, DiBA in A. flos-aquae was below detection in both years (<0.06 ng g-1 fw). Diatoms (Skeletonema marinoi, Chaetoceros wighamii) collected in May 2019 showed 0.12 ng g-1 fw TriBA and 0.06 ng g-1 fw DiBA, well below the concentrations in A. flos-aquae. TriBA exceeded DiBA in another phytoplankton species, Nodularia spumigena (Löfstrand et al., 2010). Results of this limited study suggest that TriBA is released during A. flos-aquae blooms, either from the alga itself or produced by associated bacteria. The FTriBA in water from these experiments ranged from 0.64-0.89, higher than 0.46-0.49 in KAL and ÄNG (see above), but lower than FTriBA in A. flos-aquae itself (0.94-0.98).
It is not clear whether CHL is a suitable indicator of BAs production by photosynthesizers in general. In the red alga Ceramium tenuicorne, DiBP was positively correlated with CHL but TriBP was not, and DiBP was better correlated to the sum of xanthophylls and carotenoids than to CHL (Lindqvist et al., 2017). We note that TriBA was relative abundant in the fall-collected A. flos-aquae but not in the spring-collected diatoms. Thus, TriBA in water might be more related to CHL in the fall than in spring.
BPs are synthesized by marine algae as secondary metabolites by haloperoxidase enzymes (Koch and Sures, 2018) and production is influenced by environmental stress factors (Dahlgren et al., 2015). Genes for BPs production have been identified in marine gamma-proteobacteria, in particular Pseudoalteromonas spp. (Agarwal et al., 2014; Busch et al., 2019). Several types of microorganisms are capable of generating haloanisoles by O-methylation of precursor halophenols, including bacteria, cyanobacteria and fungi (Neilson et al., 1988; Zhang et al., 2016; Zhou et al., 2021).
In summary, three lines of evidence support bacteria in addition to macroalgae and phytoplankton as sources of BAs to the estuaries: 1. Nearly equal concentrations of DiBA and TriBA were found in water, whereas TriBA > DiBA in macroalgae and phytoplankton. 2. BAs in water showed negative or no correlation with the photosynthetic variable CHL. 3. Production of BPs and other HNPs has been reported in heterotrophic marine bacteria. As noted above, production of BAs is a two-step process, whereby BPs are first formed and then O-methylated to BAs. Interpretation of BA-BP trends in the estuaries would be aided in the future by also determining concentrations of BPs.
3.4.2 DAME and TeCV
The DAME concentrations averaged 189 ± 50 pg L-1 (n=9) in KAL and 271 ± 140 pg L-1 (n=10) in ÄNG (Table 3), with no significant difference in these means (p >0.05). Concentrations in ÄNG were higher in spring, declined over the summer, and rose again in early fall (Figure 3B). Mean DAME concentrations early (up to and including Julian day 200) and later (after Julian day 200) were 221 ± 38 pg L-1 (n=5) and 149 ± 71 pg L-1 (n=4) in KAL (not significant), and 361 ± 145 pg L-1 (n=5) and 180 ± 51 pg L-1 (n=5) in ÄNG (p = 0.058). The suggested higher spring values in ANG probably result from riverine runoff to ÄNG (see below). TeCV concentrations were lower and undetectable in many samples. Means of positive samples for KAL and ÄNG were 27 ± 11 pg L-1 (n=4) and 63 ± 42 pg L-1 (n=3).
Few correlations involving DAME were significant, limited by the smaller number of samples analyzed for this HMB. In ÄNG, a terrestrial-riverine source for DAME was supported by a positive association with DOC (p = 0.043) and a negative (but not significant) relationship to SAL (p = 0.079). One DAME point appears to be an outlier (Figures 4C, F), and if that point is rejected, the correlations of DAME with DOC (positive) and SAL (negative) are stronger (p = 0.00044 and 0.0066, respectively). No significant associations between DAME and DOC, or DAME and SAL, were found in KAL, which has smaller ranges of these independent variables. DAME was negatively correlated with TriBA in ÄNG (p = 0.017) and KAL (p = 0.0075). Rejection of the suspect DAME point in ÄNG led to stronger negative correlations with TriBA (p = 0.012) and DiBA (p = 0.026). These trends suggest that, unlike BAs, DAME is not produced within the estuaries but delivered by terrestrial runoff.
3.5 Other estuaries and offshore waters.
Estuaries VVK and SVK, the strait HOLSÖR, and offshore sites HÖRN, ÖREFJ and HOLBER, were each sampled on 2-3 occasions and results are reported in Tables 4, S4. Concentrations of BAs (VVK and SVK) and DAME (VVK only) were within the ranges of those found in KAL and ÄNG (Table S2). HOLSÖR is a shallow area between two Holmön islands with visible aquatic plants when sampled, and levels of BAs in HOLSÖR were even higher than those in the estuaries (Tables 4, S4). Concentrations of BAs in offshore waters at HÖRN, ÖREFJ and HOLBER (Table 4; Figure 5; Table S4) were lower than those in the estuaries and HOLSÖR, and typical of concentrations reported in the open Baltic (Section 3.4.1). Values of FTriBA, calculated from mean concentrations in Table 4, were 0.56 and 0.54 for VVK and SVK, respectively, slightly higher than FTriBA in KAL and ÄNG (0.46 and 0.49, Section 3.4.1), but still showing similar abundance of the two BAs. Higher abundance of DiBA was found in HOLSÖR (FTriBA = 0.39), while TriBA was more dominant in offshore waters ÖREFJ, HÖRN and HOLBER (FTriBA = 0.60-0.67).
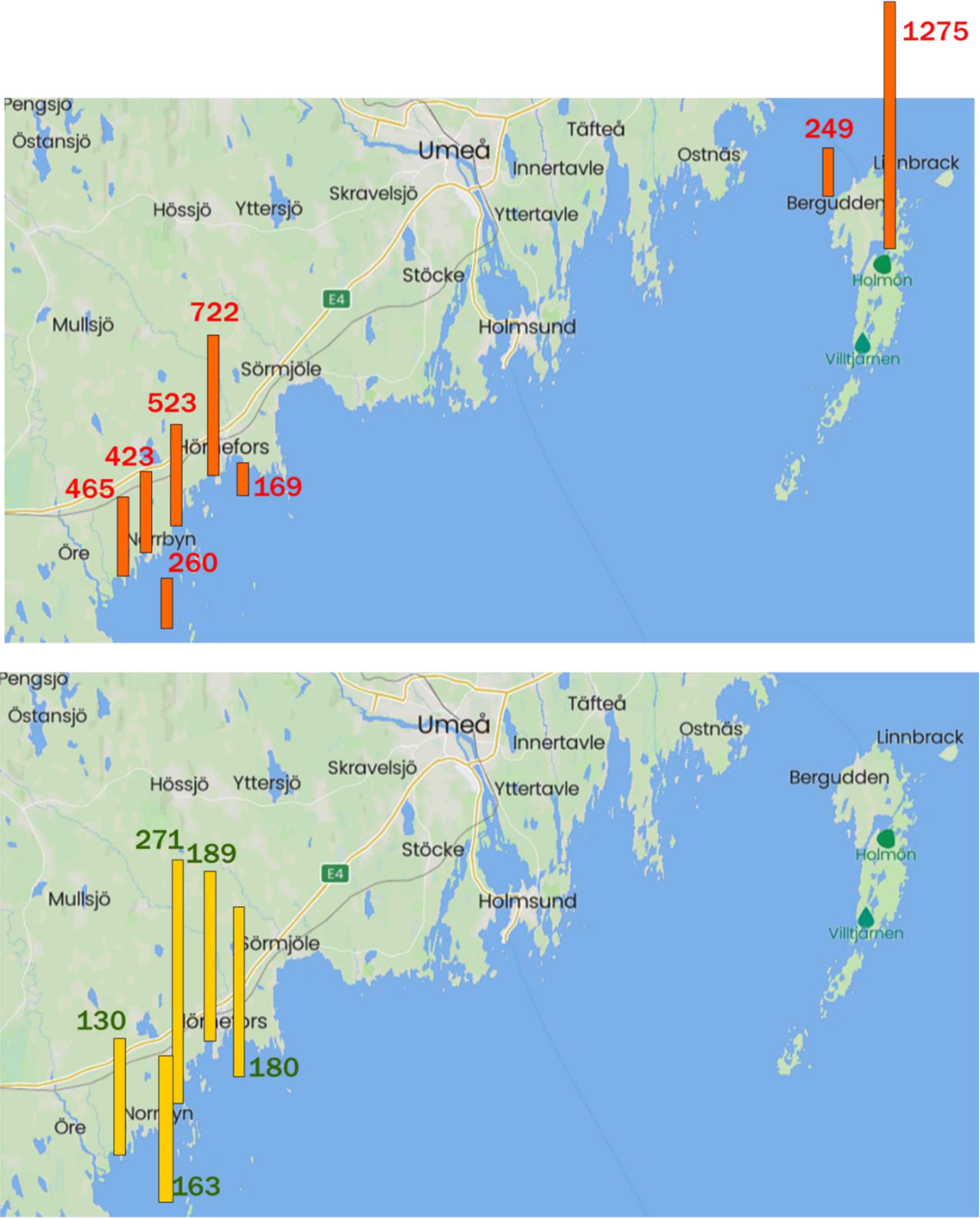
Figure 5 Top: ΣBAs (pg L-1) in KAL, ÄNG, VVK and SVK estuaries and in HOLSÖR compared to offshore stations HÖRN, ÖREFJ and HOLBER. Bottom: DAME (pg L-1) in KAL, ÄNG and VVK estuaries compared to offshore stations HÖRN and ÖREFJ. DAME was not measured at Holmön stations. Data from Tables 3 and S4. Station identifications are shown in Figure S1.
Concentrations of DAME were quite similar in the estuaries and offshore; means 189, 271 and 130 pg L-1 in KAL, ÄNG and VVK, respectively, versus 180 and 163 pg L-1 at HÖRN and ÖREFJ, (Tables 3, 4; Figure 5). This similarity is another indication that DAME is not produced within the estuaries but delivered rather uniformly to coastal waters by riverine runoff and atmospheric deposition. The only other report of DAME in seawater is from a survey in the North and South Atlantic in 1991-1992 (Schreitmüller and Ballschmiter, 1994; Schreitmüller and Ballschmiter, 1995). Concentrations in open-ocean surface water ranged from 1.5-7.7 pg L-1, well below those in our estuaries and offshore waters.
3.6 Atmospheric and riverine loadings versus in situ production of BAs in Bothnian Bay.
Our studies were conducted in estuaries and rivers of the “Quark” between the Bothnian Sea and Bothnian Bay (Area D in Figure 1). The Bothnian Bay has a surface area of 36800 km2 and a catchment area of 260675 km2 (https://en.wikipedia.org/wiki/Bothnian_Bay) and receives loadings of BAs from atmospheric deposition and riverine runoff, as well as from in situ production. In a previous assessment (Bidleman et al., 2015), BAs were judged to be oversaturated in Bothnian Bay surface water with respect to the air (water/air fugacity ratio >1) and the net flux was sea-to-air, removing an estimated ΣBAs = 1360 kg over five-month period from May-September. The reverse process, gaseous deposition of ΣBAs to the Bay, amounted to only 62 kg. Although we sampled only a few of the many rivers flowing into the Bay, we can make a rough estimate of their BAs transport. The annual discharge of eight major rivers into the Bay is 78.5 km3 (https://en.wikipedia.org/wiki/Bothnian_Bay). Scaling to the concentrations and flows of the rivers in Table 2 gives an estimated ΣBAs = 3.3 kg runoff into the Bay over five months. Thus, rivers deliver some BAs to the Bay, but the oversaturated water concentrations are probably maintained by in situ production, which drives the volatilization.
4 Conclusions and implications for climate change influence
The overall picture of HMB transport and exchange can be summed up by “What goes around, comes around”. HMBs volatilize from sea and land, disperse through the atmosphere, and return via precipitation and rivers. This cycle provides a pathway for marine BAs to reach terrestrial ecosystems. BAs have been found in water and larvae of black flies (Simuliidae spp.) from Subarctic streams in Sweden (Kupryianchyk et al., 2018).
Concentrations of BAs in KAL and ÄNG estuaries and two others (VVK and SVK) are higher than those offshore. Negative or no correlations between BAs and CHL in KAL and ÄNG suggest bacterial production in addition to known production by phytoplankton and macroalgae. Rivers also contribute BAs, but their role in Bothnian Bay appears minor relative to atmospheric processes. DAME is found in terrestrial fungi and forest litter and enters estuaries through watershed drainage.
Production and biogeochemical cycles of HNPs are influenced by climate change (Bidleman et al., 2020). The northern Baltic is transitioning from a phytoplankton-based food web to one based on heterotrophic bacteria, driven by higher precipitation and increased runoff of terrestrial dissolved organic matter (Andersson et al., 2015; Figueroa et al., 2016; Andersson et al., 2018; Figueroa et al., 2021). Phytoplankton blooms and are increasing in the southern Baltic, leading to greater eutrophication (Meier et al., 2019). How and where these shifts will affect the distribution of BAs and other HNPs is uncertain, given that macroalgae, phytoplankton and bacteria are all producers. Changes that can be expected over this century have been modeled for a region of the Baltic that encompasses the upper Bothnian Sea, the Quark, and lower Bothnian Bay; i.e. Regions C and D in Figure 1 (Turkia et al., 2022). Increases are predicted for annual filamentous algae and the perennial macroalgae Fucus spp. and Furcellaria lumbricalis. Greater production of heterotrophic bacteria and decreased phytoplankton in the northern Baltic (Andersson et al., 2015; Figueroa et al., 2016; Andersson et al., 2018; Figueroa et al., 2021) operate to augment and diminish BAs, respectively, and the net effect of these opposing processes is unknown. Increased precipitation and terrestrial runoff is likely to bring more DAME into the estuaries, but other climate impacts on DAME production have yet to be determined
BAs, BPs and other HNPs bioaccumulate in Baltic amphipods and fish and show seasonal variations in concentration (Dahlgren et al., 2016), and it is likely that DAME would behave similarly (Renaguli et al., 2020). HMBs might be useful indicators of land-sea interactions, as well as to follow migration of fish between estuaries and offshore.
Data availability statement
The original contributions presented in the study are included in the article/Supplementary Material. Further inquiries can be directed to the corresponding author.
Author contributions
TB, AA, SB and MT contributed to conception and design of the study. Sample collection was done by TB, KA, SB, LE, KH and ON. Chemical analyses were done by TB, SB and KH. MT provided funding and materials support. TB wrote the first draft of the manuscript. All authors contributed to the article and approved the submitted version.
Acknowledgments
This project was supported by funds from the Swedish marine strategic research environment EcoChange (the Swedish Research Council Formas). We thank the staff at the Umeå Marine Sciences Center for assistance in sampling and chemical analyses, Linda Zetterholm (UmU Chemistry) for assistance with river sampling, and Swedish Meteorological and Hydrological Institute (SMHI) for river flow data (Figure 1).
Conflict of interest
The authors declare that the research was conducted in the absence of any commercial or financial relationships that could be construed as a potential conflict of interest.
Publisher’s note
All claims expressed in this article are solely those of the authors and do not necessarily represent those of their affiliated organizations, or those of the publisher, the editors and the reviewers. Any product that may be evaluated in this article, or claim that may be made by its manufacturer, is not guaranteed or endorsed by the publisher.
Supplementary material
The Supplementary Material for this article can be found online at: https://www.frontiersin.org/articles/10.3389/fmars.2023.1161065/full#supplementary-material
References
Agarwal V., El Gamal A. A., Yamanaka K., Poth D., Kersten R. D., Schorn M., et al. (2014). Biosynthesis of polybrominated aromatic organic compounds by marine bacteria. Nat. Chem. Biol. 10, 640–647. doi: 10.1038/nchembio.1564
Alañón M. E., Alarcón M., Díaz-Maroto I. J., Pérez-Coello M. S., Díaz-Maroto M. C. (2020). Corky off-flavor compounds in cork planks at different storage times before processing. influence on the quality of the final stopper. J. Sci. Food. Agric. 101, 4735–4742. doi: 10.1002/jsfa.11119
Allard A.-S., Remberger M., Nielson A. (1987). Bacterial O-methylation of halogen-substituted phenols. Appl. Environ. Microbiol. 53, 839–845. doi: 10.1128/aem.53.4.839-845.1987
Andersson A., Brugel S., Paczkowska J., Rowe O. F., Figueroa D., Kratzer S., et al. (2018). Influence of allochthonous dissolved organic matter on pelagic basal production in a northerly estuary. Estuar. Coast. Shelf Sci. 204, 225–235. doi: 10.1016/j.ecss.2018.02.032
Andersson A., Meier H. E. M., Ripszam M., Rowe O., Wikner J., Haglund P., et al. (2015). Projected future climate change and Baltic Sea ecosystem management. Ambio 44 (suppl. 3), 345–356. doi: 10.1007/s13280-015-0654-8
Atlas E., Sullivan K., Giam C. S. (1986). Widespread occurrence of polyhalogenated aromatic ethers in the marine atmosphere. Atmos. Environ. 20, 1217–1220. doi: 10.1016/0004-6981(86)90156-3
Bidleman T. F., Agosta K., Andersson A., Brorström-Lundén E., Haglund P., Hansson K., et al. (2015). Atmospheric pathways of chlorinated pesticides and natural bromoanisoles in the northern Baltic Sea and its catchment. Ambio 44 (suppl. 3), 472–483. doi: 10.1007/s13280-015-0666-4
Bidleman T. F., Agosta K., Andersson A., Haglund P., Hegmans A., Liljelind P., et al. (2016). Sea-Air exchange of bromoanisoles and methoxylated bromodiphenyl ethers in the northern Baltic. Mar. Pollut. Bull. 112, 58–64. doi: 10.1016/j.marpolbul.2016.08.042
Bidleman T. F., Andersson A., Brorström-Lundén E., Brugel S., Ericson L., Hansson K., et al. (2023). Halomethoxybenzenes in air of the Nordic region. Environ. Sci. Ecotechnol 13, 100209. doi: 10.1016/j.ese.2022.100209
Bidleman T. F., Andersson A., Brugel S., Ericson L., Haglund P., Kupryianchyk D., et al. (2019). Bromoanisoles and methoxylated bromodiphenyl ethers in macroalgae from Nordic coastal regions. Environ. Sci. Proc. Impacts 21, 881–892. doi: 10.1039/C9EM00042A
Bidleman T. F., Andersson A., Haglund P., Tysklind M. (2020). Will climate change influence production and environmental pathways of halogenated natural products? Environ. Sci. Technol. 54, 6468–6485. doi: 10.1021/acs.est.9b07709
Bidleman T. F., Brorström-Lundén E., Hansson K., Laudon H., Nygren O., Tysklind M. (2017a). Atmospheric transport and deposition of bromoanisoles along a temperate to Arctic gradient. Environ. Sci. Technol. 51, 10974–10982. doi: 10.1021/acs.est.7b03218
Bidleman T. F., Laudon H., Nygren O., Svanberg S., Tysklind M. (2017b). Chlorinated pesticides and natural brominated anisoles in air at three northern Baltic stations. Environ. Pollut. 225, 381–389.
Bohlin-Nizzetto P., Aas W., Halvorsen H. L., Nikiforov V., Pfaffhuber K. A. (2021). “Monitoring of environmental contaminants in air and precipitation,” in Annual report 2020. (NILU report 12/2021; Norwegian environment agency m-2060|2021) (Kjeller: Norwegian Institute for Air Research (NILU), 148, ISBN: ISBN: 978-82-425-3039-4. ISSN: 2464-3327.
Brownlee B. G., Macinnis G. A., Noton L. R. (1993). Chlorinated anisoles and veratroles in a Canadian river receiving bleached kraft pulp mill effluent. identification, distribution, and olfactory evaluation. Environ. Sci. Technol. 27, 2450–2455. doi: 10.1021/es00048a021
Busch J., Agarwal V., Schorn M., Machado H., Moore B. S., Rouse G. W., et al. (2019). Diversity and distribution of the bmp gene cluster and its polybrominated products in the genus Pseudoalteromonas. Environ. Microbiol. 21, 1575–1585. doi: 10.1111/1462-2920.14532
Chatonnet P., Bonnet S., Boutou S., Labadie M. D. (2004). Identification and responsibility of 2,4,6,-tribromoanisole in musty, corked odors in wine. J. Agric. Food Chem. 52, 1255–1262. doi: 10.1021/jf030632f
Chung H. Y., Ma W. C. J., Kim J.-S. (2003). Seasonal distribution of bromophenols in selected Hong Kong seafood. J. Agric. Food Chem. 51, 6752–6760. doi: 10.1021/jf034632r
Dahlgren E., Enhus C., Lindqvist D., Eklund B., Asplund L. (2015). Induced production of brominated aromatic compounds in the alga Ceramium tenuicorne. Environ. Sci. Pollut. Res. 22, 18107–18114. doi: 10.1007/s11356-015-4907-7
Dahlgren E., Lindqvist D., Dahlgren H., Asplund L., Lehtilä K. (2016). Trophic transfer of naturally produced brominated aromatic compounds in a Baltic Sea food chain. Chemosphere 144, 1597–1604. doi: 10.1016/j.chemosphere.2015.10.024
DeJong E., Field J. A. (1997). Sulfur tuft and turkey tail: biosynthesis and biodegradation of organohalogens by basidiomycetes. Ann. Rev. Microbiol. 51, 375–414. doi: 10.1146/annurev.micro.51.1.375
Diaz A., Fabrellas C., Ventura F., Galceran M. T. (2005). Determination of the odor threshold concentrations of chlorobrominated anisoles in water. J. Agric. Food Chem. 53, 383–387. doi: 10.1021/jf049582k
Dickschat J. S. (2017). Fungal volatiles – a survey from edible mushrooms to moulds. Nat. Prod. Rep. 34, 310–328. doi: 10.1039/C7NP00003K
Eriksson K. I. A., Thelaus J., Andersson A., Ahlinder J. (2022). Microbial interactions ‒ underexplored links between public health relevant bacteria and protozoa in coastal environments. Front. Microbiol. 13, 877483. doi: 10.3389/fmicb.2022.877483
Figueroa D., Eric Capo E., Lindh M. V., Rowe O. F., Paczkowska J., Pinhassi J., et al. (2021). Terrestrial dissolved organic matter inflow drives temporal dynamics of the bacterial community of a subarctic estuary (northern Baltic Sea). Environ. Microbiol. 23, 4200–4213. doi: 10.1111/1462-2920.15597
Figueroa D., Rowe O. F., Paczkowska J., Legrand C., Andersson A. (2016). Allochthonous carbon - a major driver of bacterioplankton production in the subarctic northern Baltic Sea. Microb. Ecol. 71, 789–801. doi: 10.1007/s00248-015-0714-4
Francezon N., Tremblay A., Mouget J.-L., Pasetto P., Beaulieu L. (2021). Algae as a source of natural flavors in innovative foods. J. Agric. Food Chem. 69, 11753–11772. doi: 10.1021/acs.jafc.1c04409
Führer U., Ballschmiter K. (1998). Bromochloromethoxybenzenes in the marine troposphere of the Atlantic ocean: a group of organohalogens with mixed biogenic and anthropogenic origin. Environ. Sci. Technol. 32, 2208–2215. doi: 10.1021/es970922a
Garvie L. A. J., Wilkins B., Groy P. L., Glaeser J. A. (2015). Substantial production of drosophilin a methyl ether (tetrachloro-1,4-dimethoxybenzene) by the lignicolous basidiomycete Phellinus badius in the heartwood of mesquite (Prosopis juliflora) trees. Sci. Nat. 102, 18. doi: 10.1007/s00114-015-1268-5
Gong P., Xu H., Wang C., Chen Y., Guo L., Wang X. (2021). Persistent organic pollutant cycling in forests. Nat. Rev. Earth Environ. 2, 182–197. doi: 10.1038/s43017-020-00137-5
Guo J., Brugel S., Andersson A., Lau D. C. P. (2022). Spatiotemporal carbon, nitrogen and phosphorus stoichiometry in planktonic food web in a northern coastal area. Estuar. Coast. Shelf Sci. 272, 107903. doi: 10.1016/j.ecss.2022.107903
Hiebl J., Lehnert K., Vetter W. (2011). Identification of a fungi-derived terrestrial halogenated natural product in wild boar (Sus scrofa). J. Agric. Food Chem. 59, 6188–6192. doi: 10.1021/jf201128r
Hoff R. M., Brice K. A., Halsall C. J. (1998). Nonlinearity in the slopes of clausius-clapeyron plots for SVOCs. Environ. Sci. Technol. 32, 1793–1798. doi: 10.1021/es9709740
Howe P. D., Dobson S., Malcolm H. M. (2005). “2,4,6-tribromophenol and other simple bromophenols,” in Concise international chemical assessment document 6 (Geneva: World Health Organisation), 47, ISBN: ISBN 92 4 1560066 9.
Jones B., Smullen R., Carton A. G. (2016). Flavour enhancement of freshwater farmed barramundi (Lates calcarifer), through dietary enrichment with cultivated sea lettuce, Ulva ohnoi. Aquaculture 454, 192–198. doi: 10.1016/j.aquaculture.2015.12.017
Kavanaugh F., Hervey A., Robbins W. J. (1952). Antibiotic substances from basidiomycetes IX. Drosophila subatrata (batsch ex fr.) quel. Proc. Nat. Acad. Sci. (US) 38, 555–560. doi: 10.1073/pnas.38.7.555
Koch C., Sures B. (2018). Environmental concentrations and toxicology of 2,4,6-tribromophenol (TBP). Environ. Pollut. 233, 706–713. doi: 10.1016/j.envpol.2017.10.127
Kupryianchyk D., Giesler R., Bidleman T., Liljelind P., Lau D. C. P., Sponseller R., et al. (2018). Industrial and natural compounds in filter-feeding black fly larvae and water in three tundra streams. Environ. Toxicol. Chem. 37, 3011–3017. doi: 10.1002/etc.4267
Kylin H., Svensson T., Jensen S., Strachan W. M. J., Franich R., Bouwman H. (2017). The trans-continental distributions of pentachlorophenol and pentachloroanisole in pine needles indicate separate origins. Environ. Pollut. 229, 688–695. doi: 10.1016/j.envpol.2017.07.010
Lindqvist D., Dahlgren E., Asplund L. (2017). Biosynthesis of hydroxylated polybrominated diphenyl ethers and the correlation with photosynthetic pigments in the red alga Ceramium tenuicorne. Phytochem. 133, 51–58. doi: 10.1016/j.phytochem.2016.10.009
Löfstrand K., Malmvärn A., Haglund P., Bignert A., Bergman Å., Asplund L. (2010). Brominated phenols, anisoles, and dioxins present in blue mussels from the Swedish coastline. Environ. Sci. Pollut. Res. 17, 1460–1468. doi: 10.1007/s11356-010-0331-1
McLachlan M. S., Horstmann M. (1998). Forests as filters of airborne organic pollutants, a model. Environ. Sci. Technol. 32, 413–420. doi: 10.1021/es970592u
Meier H. E. M., Dieterich C., Eilola K., Gröger M., Höglund A., Radtke H., et al. (2019). Future projections of record-breaking sea surface temperature and cyanobacteria bloom events in the Baltic Sea. Ambio 48, 1362–1376. doi: 10.1007/s13280-019-01235-5
Milliken C. E., Meier G. P., Watts J. E. M., Sowers K. E., May H. D. (2004). Microbial anaerobic demethylation and dechlorination of chlorinated hydroquinone metabolites synthesized by basidiomycete fungi. Appl. Environ. Microbiol. 70, 385–392. doi: 10.1128/AEM.70.1.385-392.2004
Montelius M., Svensson T., Lourino-Cabana B., Thiry Y., Bastviken D. (2019). Radiotracer evidence that the rhizosphere is a hot-spot for chlorination of soil organic matter. Plant-Soil 443, 245–257. doi: 10.1007/s11104-019-04180-0
Neilson A. H., Allard A.-S., Hynning P.Å., Remberger M., Landner L. (1983). Bacterial methylation of chlorinated phenols and guaiacols: formation of veratroles from guaiacols and high molecular weight chlorinated lignin. Appl. Environ. Microbiol. 45, 774–783. doi: 10.1128/aem.45.3.774-783.1983
Neilson A. H., Allard A. -S., Reiland S., Remberger R., Tärnholm A., Viktor T., et al. (1984). Tri- and tetrachloroveratrole, metabolites produced by bacterial O-methylation of tri- and tetrachloroguaiacol: an assessment of their bioconcentration potential and their effects on fish reproduction. Can. J. Fish. Aquat. Sci. 41, 1502–1512.
Neilson A. H., Lindgren C., Hynning P-Å., Remberger M. (1988). Methylation of halogenated phenols and thiophenols by cell extracts of gram-positive and gram-negative bacteria. Appl. Environ. Microbiol. 53, 524–530. doi: 10.1128/aem.54.2.524-530.1988
Newton S., Bidleman T. F., Bergknut M., Racine J., Laudon H., Giesler R., et al. (2014). Atmospheric deposition of persistent organic pollutants and chemicals of emerging concern at two sites in northern Sweden. Environ. Sci. Proc. Impacts 16, 298–305. doi: 10.1039/c3em00590a
Öberg G., Bastviken D. (2012). Transformation of chloride to organic chlorine interrestrial environments: variability, extent, and implications. Crit. Rev. Environ. Sci. Technol. 42, 2526–2545. doi: 10.1080/10643389.2011.592753
Renaguli A., Fernando S., Hopke P. K., Holsen T. M., Crimmins B. S. (2020). Nontargeted screening of halogenated organic compounds in fish fillet tissues from the great lakes. Environ. Sci. Technol. 54, 15035–15045. doi: 10.1021/acs.est.0c05078
Ripszam M., Paczkowska J., Figueira J., Veenaas C., Haglund P. (2015). Dissolved organic carbon quality and sorption of organic pollutants in the Baltic Sea in light of future climate change. Environ. Sci. Technol. 49, 1445–1452. doi: 10.1021/es504437s
Schreitmüller J., Ballschmiter K. (1994). The equilibrium distribution of semivolatile organochlorine compounds between atmosphere and surface water in the Atlantic ocean. Angew. Chem. Int. Eng. Ed. 33, 646–649. doi: 10.1002/anie.199406461
Schreitmüller J., Ballschmiter K. (1995). Air-water equilibrium of hexachlorocyclohexanes and chloromethoxybenzenes in the north and south Atlantic. Environ. Sci. Technol. 29, 207–215. doi: 10.1021/es00001a027
Su Y., Hung H., Blanchard P., Patton G. W., Kallenborn R., Konoplev A., et al. (2008). A circumpolar perspective of atmospheric organochlorine pesticides (OCPs): results from six Arctic monitoring stations in 2000–2003. Atmos. Environ. 42, 4682–4698. doi: 10.1016/j.atmosenv.2008.01.054
Su Y., Wania F., Harner T., Lei Y. D. (2007). Deposition of polybrominated diphenyl ethers, polychlorinated biphenyls, and polycyclic aromatic hydrocarbons to a boreal deciduous forest. Environ. Sci. Technol. 41, 534–540. doi: 10.1021/es0622047
Svensson T., Kylin H., Montelius M., Sandén P., Bastviken D. (2021). Chlorine cycling and the fate of cl in terrestrial environments. Environ. Sci. Pollut. Res. 28, 7691–7709. doi: 10.1007/s11356-020-12144-6
Svensson T., Redon P.-O., Thiry Y., Montelius M., Bastviken D. (2022). Chlorination of soil organic matter: the role of humus type and land use. Sci. Total Environ. 806, 150478. doi: 10.1016/j.scitotenv.2021.150478
Swedish Meteorological and Hydrological Institute (SMHI) (2022) Vattenwebb. Available at: https://www.smhi.se/data/hydrologi/vattenwebb.
Teunissen P. J. M., Swarts H. J., Field J. A. (1997). The de novo production of drosophilin a (tetrachloro-4-methoxyphenol) and drosophilin a methyl ether (tetrachloro-1,4-dimethoxybenzene) by ligninolytic basidiomycetes. Appl. Microbiol. Biotechnol. 47, 695–700. doi: 10.1007/s002530050997
Turkia T., Anderssén E., Lakso E., Saarinen A., Berglund J., Nygård L., et al. (2022). “What will the sea look like in 2120?,” in Future climate and species distribution models for the central gulf of bothnia (European Union: ECOnnect Project final report, Interreg Botnia-Atlantica), 65.
Vetter W., Hasse-Aschoff P., Rosenfelder N., Komarova T., Mueller J. F. (2009). Determination of halogenated natural products in passive samplers deployed along the great barrier reef, Queensland/Australia. Environ. Sci. Technol. 43, 6131–6137. doi: 10.1021/es900928m
Vetter W., Kaserzon S., Gallen C., Knolla S., Gallen M., Hauler C., et al. (2018). Occurrence and concentrations of halogenated natural products derived from seven years of passive water sampling, (2007–2013) at normanby island, great barrier reef, Australia. Mar. Pollut. Bull. 137, 81–90. doi: 10.1016/j.marpolbul.2018.09.032
Wania F., Haugen J.-E., Lei Y. D., Mackay D. (1998). Temperature dependence of atmospheric concentrations of semivolatile organic compounds. Environ. Sci. Technol. 32, 1013–1021. doi: 10.1021/es970856c
Whitfield F. B., Hill J. L., Shaw K. J. (1997). 2,4,6-tribromoanisole: a potential cause of mustiness in packaged food. J. Agric. Food Chem. 45, 889–893. doi: 10.1021/jf960587u
Wittlinger R., Ballschmiter K. (1990). Studies of the global baseline pollution XlII. C6-C14 organohalogens (α-and γ-HCH, HCB, PCB, 4,4'-DDT, 4,4'-DDE, cis- and trans-chlordane, trans-nonachlor, anisols) in the lower troposphere of the southern Indian ocean. Fres. J. Anal. Chem. 336, 193–200. doi: 10.1007/BF00332252
Wong F., Hung H., Dryfhout-Clark H., Aas W., Bohlin-Nizzetto P., Breivik K., et al. (2021). Time trends of persistent organic pollutants (POPs) and chemicals of emerging Arctic concern (CEAC) in Arctic air from 25 years of monitoring. Sci. Total Environ. 775, 145109. doi: 10.1016/j.scitotenv.2021.145109
Wong F., Jantunen L. M., Pućko M., Papakyriakou T., Stern G. A., Bidleman T. F. (2011). Air-water exchange of anthropogenic and natural organohalogens on international polar year (IPY) expeditions in the Canadian Arctic. Environ. Sci. Technol. 45, 876–881. doi: 10.1021/es1018509
Zhang K., Zhang L., Zhang T., Mao M., Fu J. (2016). Study on formation of 2,4,6-trichloroanisole by microbial O-methylation of 2,4,6-trichlorophenol in lake water. Environ. Pollut. 219, 228–234. doi: 10.1016/j.envpol.2016.10.042
Keywords: halogenated natural products, halomethoxybenzenes, Baltic Sea, estuaries, atmospheric and riverine transport
Citation: Bidleman T, Agosta K, Andersson A, Brugel S, Ericson L, Hansson K, Nygren O and Tysklind M (2023) Sources and pathways of halomethoxybenzenes in northern Baltic estuaries. Front. Mar. Sci. 10:1161065. doi: 10.3389/fmars.2023.1161065
Received: 07 February 2023; Accepted: 10 April 2023;
Published: 01 May 2023.
Edited by:
Jonathan Y.S. Leung, University of Adelaide, AustraliaCopyright © 2023 Bidleman, Agosta, Andersson, Brugel, Ericson, Hansson, Nygren and Tysklind. This is an open-access article distributed under the terms of the Creative Commons Attribution License (CC BY). The use, distribution or reproduction in other forums is permitted, provided the original author(s) and the copyright owner(s) are credited and that the original publication in this journal is cited, in accordance with accepted academic practice. No use, distribution or reproduction is permitted which does not comply with these terms.
*Correspondence: Terry Bidleman, dGVycnkuYmlkbGVtYW5AdW11LnNl