- 1Qingdao Institute of Marine Geology, China Geological Survey, Qingdao, China
- 2Laboratory for Marine Geology, Laoshan Laboratory, Qingdao, China
- 3Key Laboratory of Coastal Wetland Biogeosciences, China Geological Survey, Qingdao, China
- 4Tianjin Geological Research and Marine Geology Center, Tianjin North China Geological Exploration Bureau, Tianjin, China
- 5Department of Earth Sciences, Utrecht University, Utrecht, Netherlands
The benthic foraminiferal assemblages are commonly used to indicate different oxygenation conditions. In the last few decades, pore characteristics of the benthic foraminiferal tests from the micro-perspective using high-spatial-resolution analysis have been extensively suggested to indicate redox changes. Based on the whole test of the living shallow-infaunal species Bolivina robusta using a more representative and comprehensive method, we observed a significant negative correlation between the pore density (PD) and bottom dissolved oxygen (DO) concentration, and the average PD was about 36% higher in hypoxic environments (DO<3 mg/l) than in oxic environments (DO>3 mg/l). In terms of reproduction pattern in hypoxic environments, the species seemed to mainly choose the asexual life cycle (74.60%) to get more small generations with larger pore size (PS) (asexual 7 μm vs. sexual 4 μm) and exterior ornamentation (irregular papillae) as their survival strategy. The results provide new insight into the benthic foraminiferal ecology to reconstruct the pale-oceanography and paleo-ecology changes in the East China Sea. Moreover, this study has the potential to be applied in broad regions as an independent proxy by comparison to other widely-distributed benthic foraminiferal species.
1 Introduction
The wide distribution of benthic foraminifera and their rapid response to ecological and environmental changes make them one of the best representatives of seafloor habitats, which are characterized by a combination of different physical, chemical, and biological conditions (Jorissen, 1999; Murray, 2006; Bernhard et al., 2010). The microhabitat preferences of foraminiferal individual taxa may change in response to the changing of pelagic environmental conditions (Jorissen et al., 1995), particularly fluctuations in oxygen levels and food supply (Corliss and Emerson, 1990; Sjoerdsma and van der Zwaan, 1992; Osterman et al., 2008; Farouk et al., 2023), and/or on interactions of competition and predation (Murray, 2006).
From macro-perspective, the conventional benthic foraminiferal assemblages were often used to reconstruct palaeoceanographic and palaeoecological conditions (Kuhnt et al., 2013; Farouk et al., 2023), including but not limited to the changes in oxygenation, whose characteristic taxa are named as low-oxygen foraminiferal assemblages (LOFAs/LOFA) (Bernhard and Bowser, 1999; Brunner et al., 2006; Swarzenski et al., 2008; Wang et al., 2014; Lee et al., 2016). However, existing approaches to using the LOFA and its diversity as a quantitative oxygen indicator still lack reliable calibrations for live faunas and recent data sets (Kuhnt et al., 2013 and references therein). As a possible solution for this, morphological features of benthic foraminiferal tests (ornamentations and pores) observed from the micro-perspective using high-spatial-resolution analysis (with Scanning Electron Microscope, SEM; Atomic Force Microscope, AFM), have been extensively suggested to be related to different oxygenation conditions (Glock et al., 2011; Kuhnt et al., 2013; Kuhnt et al., 2014; Petersen et al., 2016; Rathburn et al., 2018; Giordano et al., 2019; Richirt et al., 2019; Lu et al., 2021).
Field and laboratory observations have shown that the test pores could be used for indicting gas exchanges, and the size and number of pores on benthic foraminifera tend to exhibit a significant change from well-oxygenated to oxygen-depleted environments (Berthold, 1976; Leutenegger and Hansen, 1979; Pérez-Cruz and Machain Castillo, 1990; Moodley and Hess, 1992; Resig and Glenn, 2003; Bernhard et al., 2010; Kuhnt et al., 2013; Kuhnt et al., 2014; Dubicka et al., 2015). Pores also supply a pathway between the exterior and interior chambers for releasing metabolic carbon dioxide (CO2), absorbing solution organic substances for feeding, nitrate respiration, etc. (Berthold, 1976; Glock et al., 2011; Kuhnt et al., 2014; Richirt et al., 2019). The porosity (percentage of surface area covered by pores), which is largely and directly correlated with the pore density (PD) and pore size (PS), indicates a crucial metabolic need of physiology under oxygen-depleted levels (Richirt et al., 2019), i.e., the higher porosity, the higher efficiency of oxygen intake. Specifically, the PD is inversely related to ambient bottom dissolved oxygen (DO) concentration (Glock et al., 2011; Kuhnt et al., 2013; Kuhnt et al., 2014), while the PS has a variable relationship to the DO (Moodley and Hess, 1992). Therefore, the porosity has been widely used as a quantitative proxy to reveal the oxygen conditions, based on the results of selecting partial test areas using manual measurements and/or automated methods with a software package (e.g., software AxioVision 4.7 from ZEISS and software ImageJ) (Kuhnt et al., 2013; Kuhnt et al., 2014; Petersen et al., 2016; Rathburn et al., 2018; Giordano et al., 2019; Richirt et al., 2019; Lu et al., 2021). Though extremely time-consuming, manual measurements have obvious advantages of avoiding problematic pores (e.g., infilled, covered with detrital material, dissolved, and broken by mechanical force), and measuring the offset due to the curvature of the test (Petersen et al., 2016). Automated pore measurement can facilitate processing substantial data rapidly in a short time, but may under- or over-estimated PD/porosity results of inclined, non-perpendicular pores, or pores with similar colors as the surrounding test (Kuhnt et al., 2014; Petersen et al., 2016). Therefore, the advised manual post-treatment of the data is necessary for measurements analyzed using automated approach (Petersen et al., 2016). Moreover, problems may exist for the representativeness of the selected area for most non-flattened species or very large specimens (Petersen et al., 2016).
We have been aware that the foraminiferal porosity is more appropriate than using the PD or PS separately as the hypoxic proxy. However, in the calcification process of the foraminiferal test, a thin calcite layer is being precipitated over the entire test with addition of every new chamber, and the subsequent calcite layers may cause slight changes in the pore characteristics of earlier chambers (Murray, 2006; Glock et al., 2011; Richirt et al., 2019). On the other hand, partial pores are deformed large, filled, or broken in the post-sedimentary process (Petersen et al., 2016). In both cases, the selectivity of statistics for the inconsistent PSs may generate several offsets. Therefore, we had to eliminate the PS and only choose the PD on the relatively flat surface of species Bolivina robusta Brady tests using the manual measurements. The dataset from the whole test of each specimen is more representative and comprehensive than selecting a partial test area to examine the relationship between the PD and DO. Our study aims to develop a reliable, non-destructive, and unlocalized method for quantitatively describing pores in the benthic foraminiferal tests. In the proposed approach, PD could be used to further explore the potential hypoxia as a proxy for the palaeo-environment.
2 Materials and methods
2.1 Study area
The Changjiang (Yangtze River) has a strong influence on the continental shelf through the diluted water (CDW, Changjiang Diluted Water), which extends in the estuaries and adjacent seas throughout the year. Moreover, the CDW triggers stratification and turbid plumes during the flood season (May-October) with 75% of the Changjiang River annual runoff (Dagg et al., 2004; McKee et al., 2004; Burone et al., 2013). Due to the dissolved inorganic nitrogen (DIN) supply (116 Gmol yr−1) (Shen et al., 2006), the primary production (PP) in the CDW plume region may reach as high as 2079 mg C m-3d-1 (Gong et al., 2006). The current system (Figure 1b) in our study area includes the CDW, the Subei coastal current as a branch of the Yellow Sea Coastal Current (YSCC), a perennial northward branch of the Taiwan Warm Current (TWC), and the East China Sea Coastal Current (ECSCC), which interact actively and collectively to govern the hydrodynamics in this region (Wang et al., 2014 and references therein).
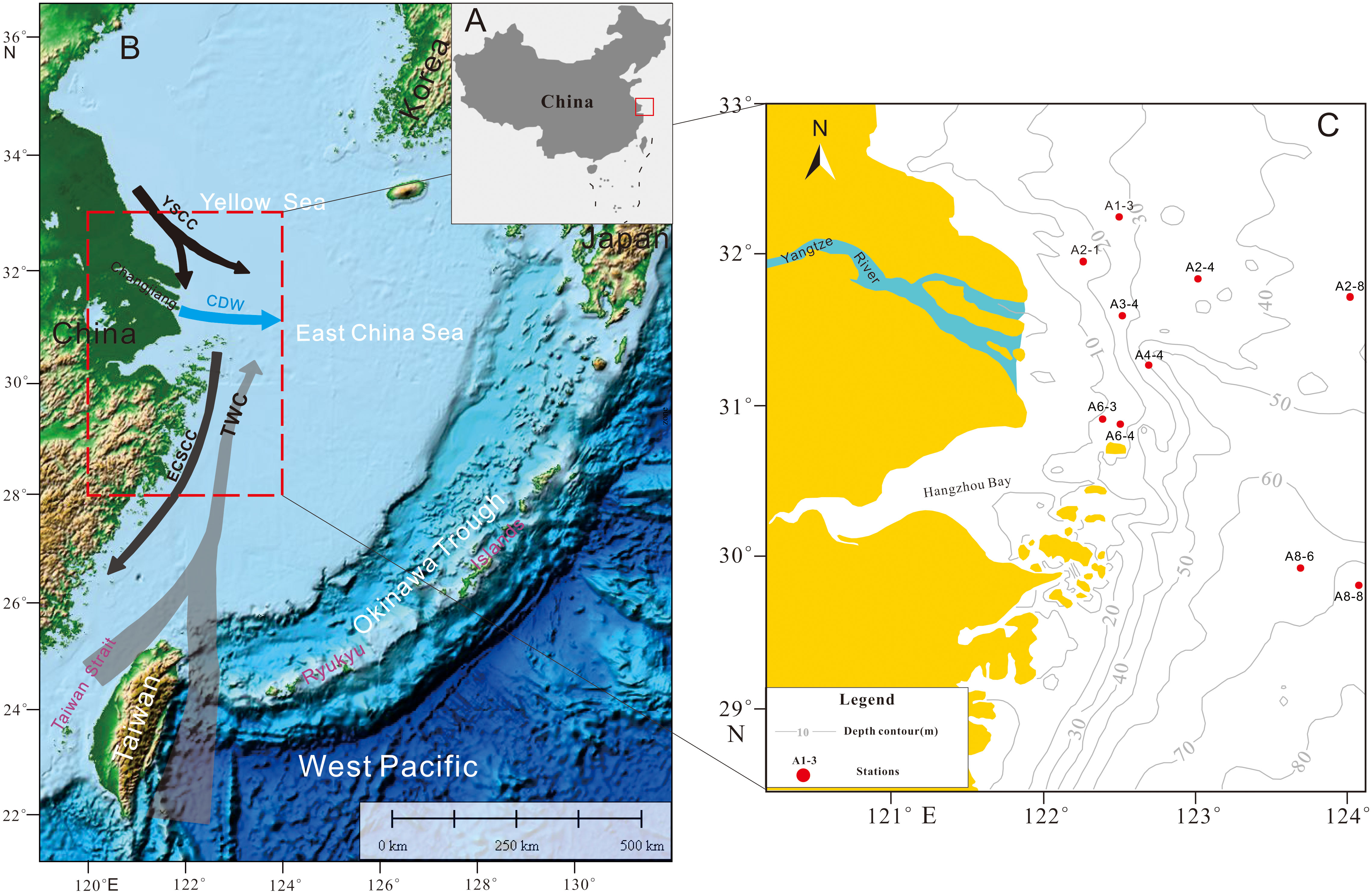
Figure 1 Local currents and sampling stations. (A) is the macroscopic location in China, (B) is the local area, (C) is the distribution of sampling stations. YSCC is Yellow Sea Coastal Current, TWC is Taiwan Warm Current, ECSCC is East China Coastal Current, and CDW is Changjiang (Yangtze River) Diluted Water.
Based on the DO concentrations, four oxygenation levels of microenvironments were categorized, i.e., oxic (DO>3.0 (or 2.0) mg/l), dysoxic (DO within 3.0 (or 2.0) -0.3 mg/l), suboxic (DO within 0.3-0.0 mg/l), and anoxic (DO=0.0 mg/l). The later three levels are collectively known as the hypoxia (Tyson and Pearson, 1991; Diaz, 2001; Wang et al., 2012). Studies have shown that the seasonal hypoxia off the Changjiang estuary in the East China Sea (ECS) (Figures 1a, b) is the largest in the world and is mainly controlled by the current patterns (CDW, branch variations of the TWC and others), increasing nutrients inputs, high PP, density stratification of water column and bottom topography (Chen et al., 2007; Wang, 2009; Wang et al., 2012; Wang et al., 2016; Wu et al., 2021; Zhao et al., 2021; Zhou et al., 2021). The seasonal hypoxia in the Changjiang estuary also influences the local ecosystems (Qian et al., 2017).
2.2 Sampling collection
Surface sediment samples were collected during two cruises off the Changjiang estuary in the East China Sea. Using a stainless steel grab sample from the vessel “Runjiang”, twelve surface samples were collected from six stations (A2-4, A3-4, A6-3, A6-4, A8-6, and A8-8) in June 2015 and four stations(A1-3, A2-1, A2-8, and A4-4) in July 2016 with different bottom dissolved oxygen concentrations (Figure 1c; Table 1). Notably, the sampling cruise in July 2016 was forced to interrupt during July 10-13 when the typhoon Nepartak turned northeast and crossed the Changjiang estuary to the ECS (Wang et al., 2018). Thus, the same stations of A1-3 and A2-8 were collected twice in this cruise, during and after the typhoon Nepartak, respectively (Table 1).
For each sampling station, the uppermost (0-3 cm) sediment was stored in plastic bottle and mixed in a methanol and 2 g L-1 Rose Bengal solutions (Walton, 1952; Oron et al., 2014). The fixing method can stain foraminiferal protoplasm up to a few weeks after their death (Bernhard, 1988). All the samples were kept at temperature ~ 4°C until further treatment. In the laboratory, samples were dried and weighted, and then rinsed over a 63-μm mesh sieve to remove the fine sediments. The residues were oven-dried at temperature <50°C. The living foraminiferal specimens, whose entirely protoplasm was stained or the last one chamber was not unstained in the test (De Stigter et al., 1998), were hand-picked and identified on standard micropaleontological slides under a binocular microscope Zeiss Stemi DV4. Synchronous water depth (WD) and basal water DO concentrations were separately measured via CTD (conductance, temperature, and depth) device and the Winkler titrations method by crews on the vessel.
2.3 SEM analysis
The hand-picked living specimens were mounted on aluminium stubs, sputter coated with gold, and photographed using a Hitachi S-4800 SEM equipment at the Experimental Center in Qingdao Institute of Marine Geology. Except for images that can’t be used (i.e., tests/pores infilled or covered with detrital material, dissolved, or broken, shown in Figures 2–1, 2, 3), the total data of 271 specimens from three analysis times (September 6 and 20, 2016; March 14, 2022) were used in this study (Appendix-Experimental data).
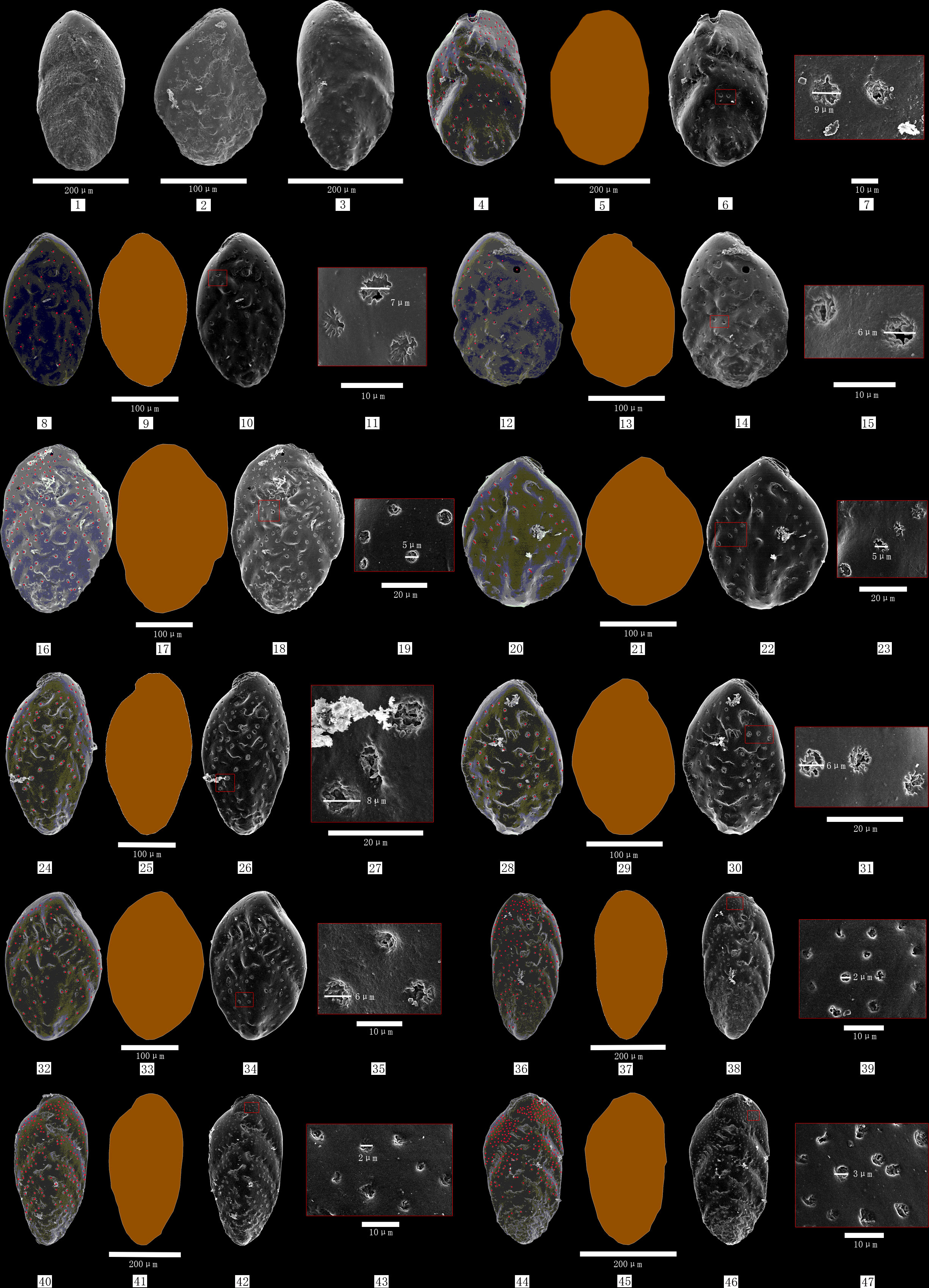
Figure 2 Examples of the deserted tests (1-3), and processes of counting test pores, calculating test area, and estimating enlarged pores’ characteristics (4-47) 1 is a sample covered by detrital material, 2 is a broken test, 3 is a blurry test surface;4-47 are the process of pore counting (red dots), calculation of area (dark brown) and length/width, and estimating enlarged pores’ characteristics (large red rectangle) from the last adjacent photo zone (small red rectangle) of the species B. robusta test in different sampling stations(4-7 are from samples A2-4, 8-11 and 12-15 are from samples A3-4, 16-19 and 20-23 are from samples A6-3; 24-27 and 28-31 are from samples A6-4, 32-35 and 36-39 are from samples A8-6, 40-43 and 44-47 are from samples A8-8).
2.4 Target foraminiferal species selection and measurements
In the proposed investigation method, we selected species B. robusta for three reasons. Firstly, B. robusta has obvious representativeness, because it often dominates the benthic foraminiferal assemblages in the study area, and is also broadly found in almost all samples in this study area. Secondly, the shallow-infaunal B.robusta thrives near the sediment-water interface and exhibit strong morphological adaptions to the wide range of DO concentrations. The living shallow-infaunal B.robusta is therefore more suitable to reveal the surrounding DO changes than deep-infaunal species, which may move to a shallower sediment depth to compensate for the oxygen decrease (Kuhnt et al., 2013; Kuhnt et al., 2014). Thirdly, since the test of B.robusta is biserial and zygomorphous with a relatively flat surface and well-developed pores, the offset in its test surface area is very limited, compared to other genera/species, e.g., Ammonia (Petersen et al., 2016; Rathburn et al., 2018; Giordano et al., 2019; Richirt et al., 2019), Globobulimina turgida (Kuhnt et al., 2014), Bolivina pacifica and Fursenkoina mexicana (Kuhnt et al., 2013). Considering the existence of both sexual and asexual generations of the species B. robusta in sediments, we performed both large-elongate tests with small-microspheric proloculus (sexual reproduction) and small-short tests with large-megalospheric proloculus (asexual reproduction) in the study to explore the preferred reproduction strategy of the species B. robusta under different oxic conditions (Wang et al., 1988; Murray, 2006).
For each living B. robusta test, the pore number (unit: no.) was counted manually. The test surface area (Area, unit: mm2), test length/width ratio (L/W, the ratio of test length to width) and pore size (PS, the diameter of the pores, unit: μm) were calculated following the software CAD. The pore density (PD, pore number per unit area, unit: no./mm2) was calculated as the ratio of pore number to Area. In order to avoid the possible constraint caused by selecting a partial area, we performed the statistical analysis to the entired-individuals unilateral test on SEM images for all measured variables described above.
2.5 Statistical analysis of measured variables
Regression analysis among the PD and DO concentrations and bivariate Pearson Correlation analysis between pairs of variables were performed. According to the oxic levels defined above, all PD data were divided into two groups, i.e., hypoxic (DO concentration < 3mg/L) and oxic (DO concentration ≥ 3mg/L), for the F-test with P (F<= f) values > 0.05 and t-test with P* * (T<= t) < 0.05 through the two-sample analysis of variance (ANOVA) to determine if there was a significant difference. The prediction interval (PI) represents the range within which a predicted PD would fall at a 95% confidence interval (CI) for a given DO concentration. All statistical analyses mentioned above were performed using the software OriginPro 2021.
3 Results
3.1 PD and DO
Our results showed that the PD fluctuated obviously when the DO concentrations varied from above 3.0 mg/l to below 3.0 mg/l, and the micro-morphological features of foraminiferal tests seemed to be linked to the DO concentrations (Figure 3). This is consistent with the proposed threshold value of DO concentrations of 3.0 mg/l between hypoxic- and oxic-microenvironments in previous studies (Tyson and Pearson, 1991; Diaz, 2001; Wang et al., 2012).
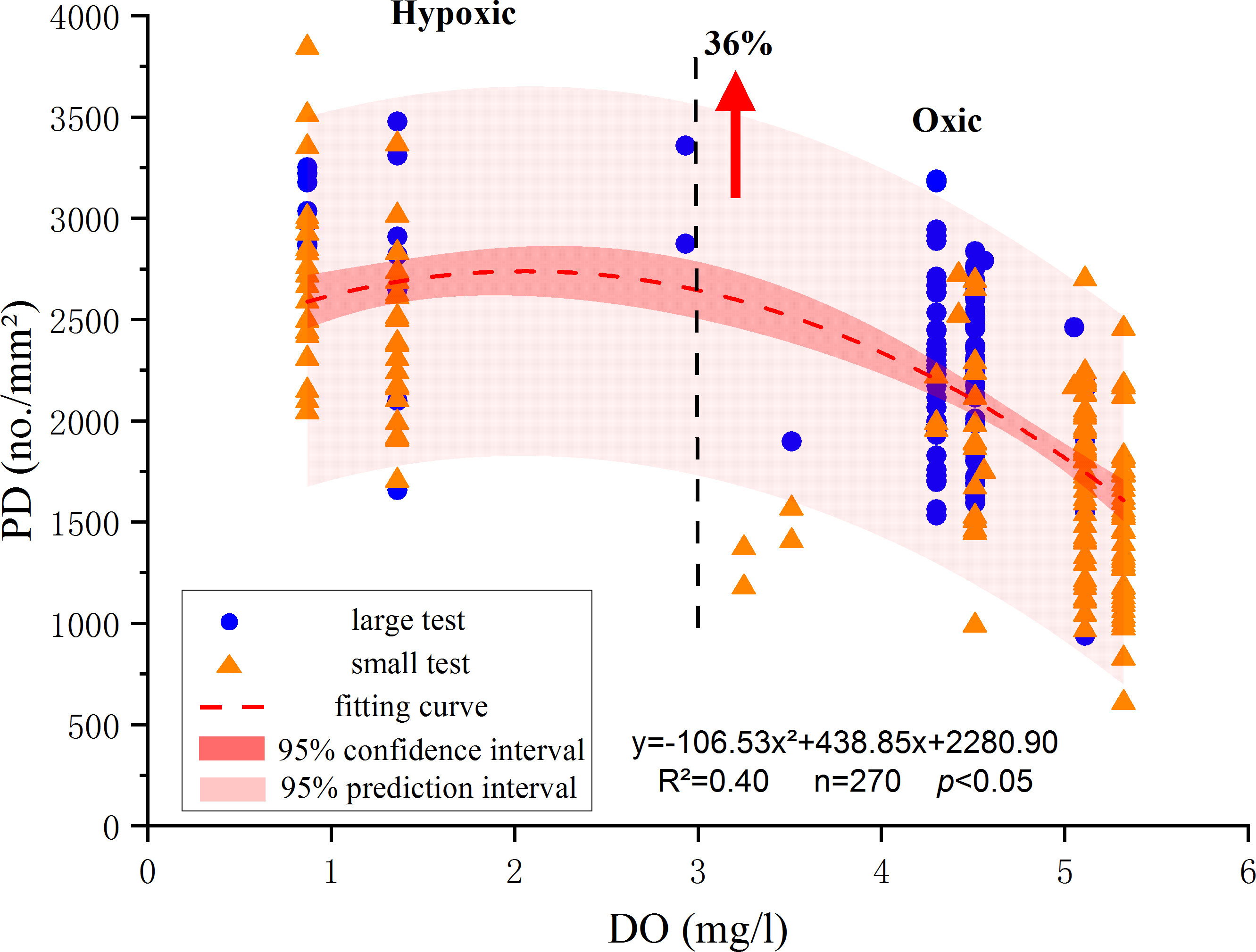
Figure 3 Fitted regression plots of PD and DO concentrations. The blue plots represent the PD from the sexual specimens’ test and the brown triangles represent the PD from the asexual ones. The prediction interval (PI, light red area) represents the range within which a predicted PD would fall at a 95% confidence interval (CI, deep red area) for a given DO concentration. The PI, CI and fitting curve are obtained using both sexual and asexual data.
In the total of 271 test measurements, 63 specimen tests were from the three sampling stations in the hypoxic environment with DO<3 mg/l, with the PD of specimens ranging from 1655 no./mm2 to 3844 no./mm2 (Table 2). The rest 208 specimen tests were from eight sampling stations in the oxic environment with DO>3 mg/l, and their PD values ranged between 608 no./mm2 and 3188 no./mm2 (Table 2).
The PD and DO followed a polynomial regression relationship PD = -106.53 ( ± 19.53) * DO2 + 438.85 ( ± 120.59) * DO + 2280.90 ( ± 139.82) (R2 = 0.40, p<0.05, Figure 3). About 96% of all data were within the 95% PI (light red area in Figure 3). The PI range was larger than the CI range (deep red area in Figure 3) based solely on the fitting of measurements because the PI range took into account the expected random error associated with the predicted PD. Overall, the patterns of the fitting curve of PD versus DO concentrations, as well as the corresponding ranges 95% CI, and 95% PI collectively showed that the PD values of tests of the species B. robusta in the Changjiang estuary increased with the decreases in the DO concentrations.
3.2 Significance of PD difference between oxic and hypoxic groups
The F-test through the two-sample analysis of variance of the oxic and hypoxic groups of PD data showed that P (F<= f) value of 0.18, which is higher than 0.05. Thus, there was no significant difference in the variance between the oxic and hypoxic groups of PD (Table 3), which confirmed the homogeneity of the PD variance of the two groups. On this basis, two-sample t-equal variance hypothesis test could be performed.
The t-test of the two groups of PD data (Table 4) yielded P* * (T<= t) < 0.05, which indicates that the null hypothesis could not be rejected. Thus, at the 0.05 significance level, there was a significant difference between the variance of the two groups. Overall, the PD values of the hypoxic group tended to be higher than that of the oxic group (Figure 3). The average PD of the hypoxic group of 2629 no./mm2 was 36% higher than that of the oxic group (1932 no./mm2).

Table 4 The t-test results of two sets of the PD (superscript * represents one-tail, superscript ** represents two-tail.
3.3 Bivariate Pearson Correlations between variables
The bivariate Pearson Correlation analysis between pairs of variables including WD, DO, Area, pore number, PD, and L/W were performed for the whole dataset, with results shown in Figure 4 and detailed data in the Appendix. Among all measured variables, the Area, PD, and L/W showed a strong positive correlation to the pore number with Pearson Coefficients of 0.94, 0.73, and 0.69 respectively. There is also a positive correlation between Area and L/W (R2 = 0.70, p<0.05).WD showed a positive correlation to the Area with a lower coefficient (R2 = 0.69, p<0.05). In terms of the relationship between DO concentrations and other variables of foraminifers in the correlation matrix (Figure 4F), a significant negative correlation (R2 = -0.57, p<0.05) was observed between the PD and DO concentrations, the pore number showed a less significant negative relationship with DO concentrations (R2 = -0.26, p<0.05), and no significant relationships were observed between other variables and the DO concentrations.
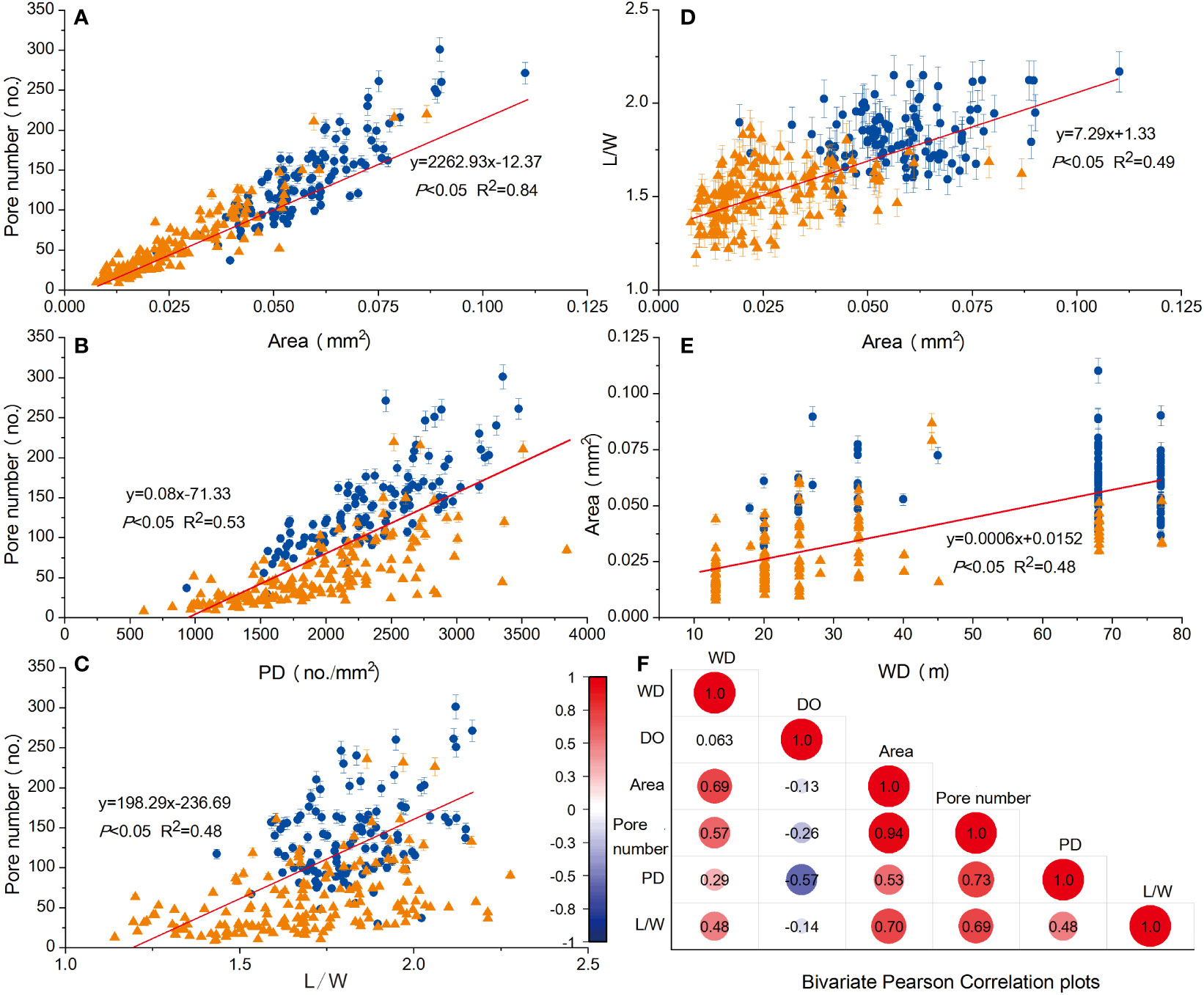
Figure 4 Bivariate scatter plots and Pearson’s Correlation graphs. The blue plots represent the pore density (PD) from the sexual specimens’ test and the brown triangles represent the PD from the asexual ones. The trend lines (red) in (A–D) are obtained using both sexual and asexual data. (A–E) show the positive correlations between Pore number and Area, Pore number and PD, Pore number and L/W, L/W and Area, Area and WD, respectively. (F) is the Bivariate Pearson Correlation plots.
3.4 Characteristics of sexual and asexual generation groups
By further looking into the statistical characteristics of sexual and asexual generation groups (Figure 4), we found that the area of species B. robusta test from sexual generations (blue plots) was mainly confined to 0.0375-0.0900 mm2 (i.e., 37500-90000 μm2), while most of the area of foranimiferal tests from asexual generations (brown triangles) were between 0.0100 and 0.0523 mm2 (i.e., 10000-52300 μm2). Moreover, the pore number of foraminifers from sexual generations was mainly within 70-260, while those of foraminifers from asexual generations were mainly ranged 10-150 (Figures 4A, D). The L/W ratios among the sexual- and asexual generation groups also differed, with higher L/W ratios of sexual generation groups (1.8 on average, ranging 1.4-2.2) than asexual generation groups (1.5 on average, ranging 1.2-1.9) (Figures 4C, D).
3.5 Asexual small-short and sexual large-elongate proportions
Statistics of the number of randomly selected tests in species B. robusta was further analyzed to examine the asexual small-short and sexual large-elongate proportions. The asexual small-short group (large-megalospheric proloculus) with a proportion of 74.60% dominated in the hypoxic environment (DO<3.00 mg/l) (Table 5). The asexual and sexual large-elongate groups (small-microspheric proloculus) accounted for similar proportions on average (55.29% versus 44.71%, respectively) in the oxic environment (DO>3.00 mg/l).
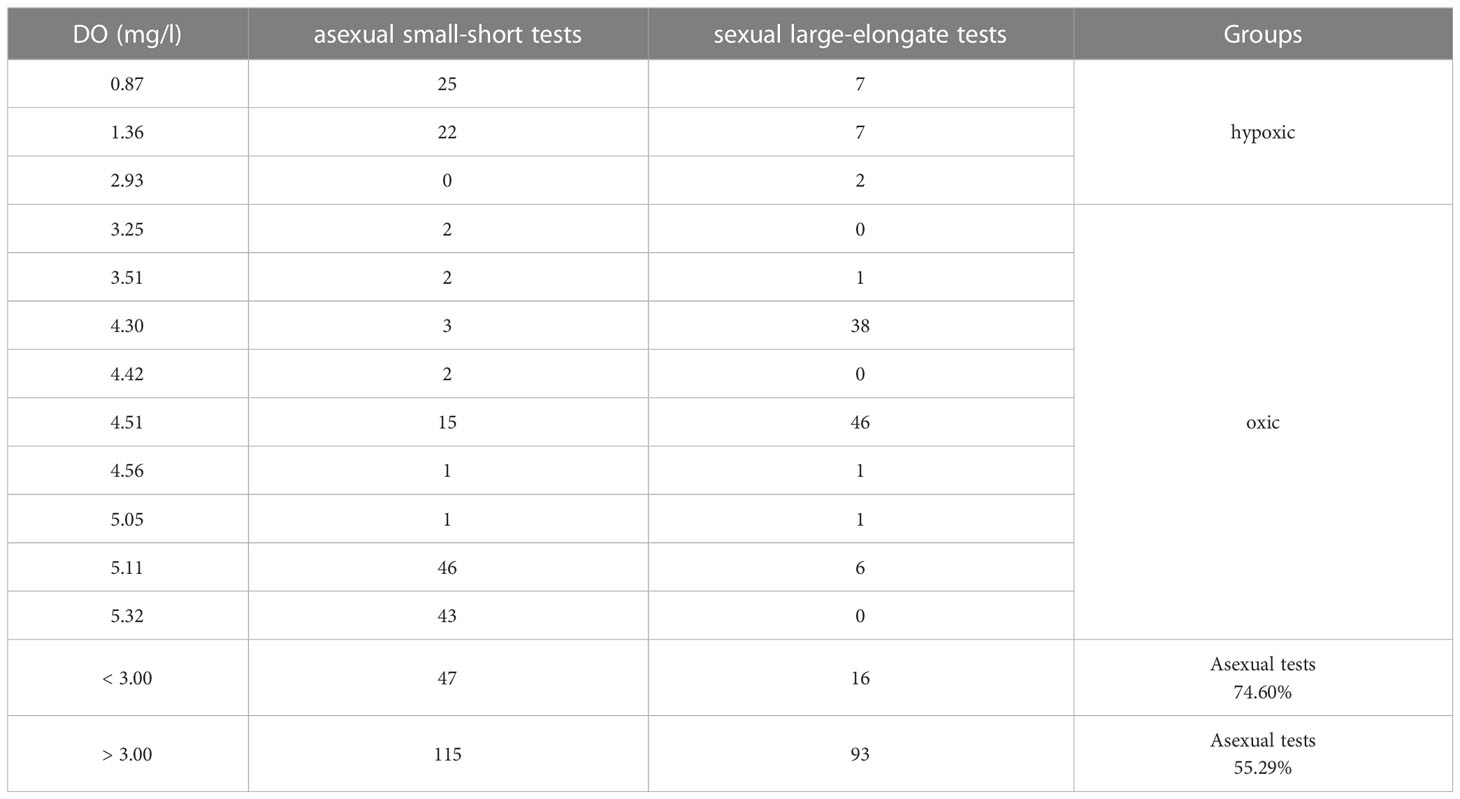
Table 5 The statistics of asexual small-short and sexual large-elongate tests’ numbers in each DO concentration.
4 Discussion
4.1 The strategy of B. robusta survived in the hypoxic environment
In order to understand the interdependent ecological controls on the benthic foraminiferal distribution, the trophic conditions and oxygen concentrations (TROX) conceptual model, which takes into account the organic flux (food), oxygen conditions, and other geochemical changes, was developed by Jorissen et al. (1995) and further improved by van der Zwaan et al. (1999). According to the results of the TROX model, DO seem to be more important than temperature and salinity for the benthic foraminiferal distribution (van der Zwaan et al., 1999), and the foraminiferal survival strategy of low dependence on temperature and salinity may effectively prevent their rapid extinction during the long geo-historical period (e.g., from glacial to interglacial periods). Different groups of benthic foraminiferal species live in different oxygenation environments when other factors are unchanged. In oxygen-depleted or absent anoxic environments where oxygen supply is limited, DO concentrations act as an important controlling factor for benthic foraminifera (van der Zwaan et al., 1999; Murray, 2006; Kuhnt et al., 2013). Lab-culture experiments have shown that though some foraminiferal species can survive hypoxic, anoxic, and/or hydrogen sulphidic conditions for short periods (e.g., several weeks to months), longer exposure (several months to years) of foraminifera to anoxic environments will lead to their death (Murray, 2006; Hein and Geslin, 2012). The DO concentration is a net result of the oxygen production and consumption. Both the oxygen content of bottom water mass and the oxygen consumption due to decomposition of organic matter, mainly influence oxygenation associated with the DO concentration level (Kuhnt et al., 2013). Though the availability of organic matter may influence benthic foraminiferal distribution through the food chain, DO may be a more important factor in the oxygen-depleted environment because of the minor changes in the organic matter flux at a low decomposition rate. Overall, the hypoxic (dysoxic to anoxic) conditions can influence microhabitat selection and migration of benthic foraminifers (Hein and Geslin, 2012).
Since benthic foraminiferal species are both aerobes and facultative anaerobes, they can adapt to different habitats ranging from oxic to hypoxic (and even anoxic) sediments (Murray, 2006; Hein and Geslin, 2012). The aerobic respiration rates of benthic foraminifers are lower than that of other meiofaunal groups probably, which enables the survival of benthic foraminifers in the hypoxic environments using the strategy of lower metabolism rates (Hein and Geslin, 2012). Benthic foraminifera have developed a range of mechanisms and strategies to survive in the hypoxic environments. For example, the shallow-infaunal species near the sediment-water interface have a larger porosity, PD and/or PS through morphological adaptions (Glock et al., 2011; Kuhnt et al., 2013; Kuhnt et al., 2014; Petersen et al., 2016; Rathburn et al., 2018; Lu et al., 2021), while the deep-infaunal species migrate to an optimum oxygenic niches to compensate for oxygen decrease instead of morphological adaptions (Hein and Geslin, 2012; Kuhnt et al., 2013; Kuhnt et al., 2014; Rathburn et al., 2018). The chloroplast-sequestering species tend to have external ornamentations (e.g., toothed fossettes, tubercules, or finger-like flaps) to disarticulate the frustules and tear the cell wall from their algal prey for liberating chloroplasts (Bernhard and Bowser, 1999). Some species accumulate nitrate in the cytoplasm for nitrate respiration (denitrification) as an anaerobic metabolism pathway (Piña-Ochoa et al., 2010; Glock et al., 2011; Hein and Geslin, 2012).
For low-oxygen-tolerant species, the clusters of mitochondria, which are the cell organelles of respiration, often gather near the inner pore terminations, and the cytoplasmic membrane invaginates within the pore plugs to facilitate efficient transport of oxygen. However, the mitochondria clusters are more evenly distributed throughout the cytoplasm for foraminiferal individuals in well-oxygenated environments (Leutenegger and Hansen, 1979; Bernhard et al., 2010). This also confirms that one of the functions of the pores is the entrance channel for oxygen. Pores are important morphological features in hyaline foraminifera. The crucial physiology of foraminifera would supply a possible way to change their functional morphological features, e.g., having higher test porosity by changing PD and/or PS during the transition from oxygenated to oxygen-depleted conditions. The PD may increase to enhance mitochondrial oxygen uptake as oxygen availability decreases, and there may be a minimal PD threshold to enable survival of foraminifera, which explains the uniform PD under higher-oxygen conditions (Figure 3) (Glock et al., 2011). However, higher porosity and thinner specimens can reduce the secretion of calcite and is critically unfriendly to the robustness of test because a thin calcite layer is precipitated over the entire specimen with every new chamber during the calcification process of the foraminiferal test, and the subsequent calcite layers may slightly change the pore characteristics of earlier chambers, particularly for the highly-porous and thin specimens (Glock et al., 2011; Richirt et al., 2019). Despite the possible uncertainties, the significant negative correlation between the PD of shallow-infaunal species B. robusta and DO concentrations observed in this study (Figures 3, 4) is similar to that for the species Bolivina albatrossi (Gary et al., 1989), B. spissa, B. seminude (Glock et al., 2011), B. pacifica (Bernhard et al., 2010; Kuhnt et al., 2013) in the genus Bolivina and some other species like Fursenkoina mexicanna, G.turgida (Kuhnt et al., 2013; Kuhnt et al., 2014). Their increases in PD values enhance their mitochondrial oxygen uptake in response to low-oxygen conditions, with an increase in the average PD of B. robusta by 36% from oxic to hypoxic environments observed in this study (Figure 3). Thus, we speculated that the shallow-infaunal species B. robusta increase their porosity mainly through increasing their PD as their strategy to survive in the hypoxic environment, and their PD changes with DO concentrations followed the relationship expressed in a fitting curve equation (section 3.1, Figure 3).
In addition to serving as an oxygen channel, the functionality of pores of mobile infaunal taxa may also be variable for other processes, e.g., releasing metabolic CO2 (Berthold, 1976), absorption of soluble organic substances for feeding (Glock et al., 2011), nitrate respiration (Glock et al., 2011; Hein and Geslin, 2012; Kuhnt et al., 2014; Richirt et al., 2019). Several studies have shown that nitrate concentration and PD are also correlated for some foraminiferal species (Glock et al., 2011; Kuhnt et al., 2014). If the specimens switch completely to nitrate respiration, nitrate concentration might become a more important factor, particularly when nitrate concentrations decrease and become limited, the PD of foraminifera might increase to optimize nitrate uptake (Glock et al., 2011). The deep-infaunal species can use denitrification as their important metabolic mechanism to survive in anoxic conditions (Piña-Ochoa et al., 2010). However, the epifaunal or shallow-infaunal species typically do not migrate to anoxic sediments and thus do not use nitrate for respiration (Rathburn et al., 2018). Therefore, pores also might not be involved in the denitrification process for epifaunal or shallow infaunal species and their PD values and nitrate concentrations are not correlated (Piña-Ochoa et al., 2010; Kuhnt et al., 2013). Several experiments have observed that the low-oxygen-tolerant species G.turgida can have low PDs and high total nitrate accumulation rates under well-oxygenated conditions, which may be related to the significantly high energy yield of oxygen respiration and its facilitation of high nitrate concentrations with less effort (Koho and Pina-Ochoa, 2012; Kuhnt et al., 2014). However, since the nitrate concentrations are also dependent on its production and consumption through other processes in the biogeochemical cycling and its coupling with oxygen concentration, the reported correlation between nitrate concentrations and foraminifera PD values can be coincidence or subjected to specific conditions/species. This is may be partially the reason why the numbers of pores on the species, B. pacifica, F. mexicanna and G.turgida are more closely related to oxygen availability than to nitrate concentrations (Kuhnt et al., 2013, Kuhnt et al., 2014; Rathburn et al., 2018).
For individual tests, we found that the PD of living B. robusta showed an increasing trend from early to late chambers (Figure 2). Similar trends were also found for the species B. spissa (Glock et al., 2011). Compared with the early chambers generally without pores, the last few large chambers have more pores to meet the needs for sufficient communication between protoplasm and the external environment, e.g. for respiration and/or material exchange (Glock et al., 2011; Rathburn et al., 2018).
4.2 Alternation of asexual and sexual generation groups
Since only sexual reproduction provides genetic novelty from an evolutionary perspective, the benthic foraminifers mainly reproduce by asexual multiple fission tend to evolve more slowly than plankton which entirely adopt sexual reproduction. For the foraminifers with symbionts, asexual reproduction provides a means of transmitting symbionts to the next generation, and the juveniles comprise not only a nucleus but also some cytoplasm with all its organelles. The agamonts from sexual reproduction have to acquire symbionts from the environment (Murray, 2006). This may be the reason for the co-existence of test dimorphism of the species B. robusta with asexual small-short tests (large-megalospheric proloculus) and sexual large-elongate tests (small-microspheric proloculus) in the Changjiang estuarine sediments (Wang et al., 1988; Murray, 2006).
Previous studies have also shown that the average size of foraminiferal specimens living in oxygen-depleted environments is smaller among all the foraminiferal assemblages, possibly because smaller foraminifers can reach reproductive maturity faster under the condition of abundant food (Rathburn and Corliss, 1994; Rathburn et al., 2018) or limited oxygen availability (Jorissen et al., 2007; Koho and Pina-Ochoa, 2012). Anyway, obtaining more small tests through rapid maturation (asexual generations) may also be one of the strategies for foraminifers to multiply and survive in a harsh low-oxygen environment. Despite limited number of tests and data, the statistics of the number of randomly selected tests in living species B. robusta showed the dominance of asexual small-short groups in the hypoxic environment and similar proportions of asexual and sexual groups in the oxic environment, which confirms the reported reproduction strategy mentioned above. This morphological features and relevant reproduction strategy may also be related to the foraminiferal adaption to different habitats ranging from oxic to hypoxic sediments (Murray, 2006; Hein and Geslin, 2012), but more research and data are needed to further study this mechanism.
The sexual generation groups tend to have higher values of tests’ area, L/W ratio and pore number than the asexual generation groups (Figure 4). For individuals with the vague characteristics of proloculus chamber, the big difference in L/W ratio may provide a fast way for quickly distinguishing sexual (L/W>1.8) and asexual tests (L/W<1.5). In this study, both sexual and asexual generation groups tended have higher PD values in the hypoxic environment (Figure 3). Moreover, the asexual individuals generally have higher PS of as high as 7 μm on average (ranging from 5 to 9 μm), whereas the sexual tests’ was only 4 μm on average (ranging from 2 to 8 μm) (Figure 2). Regardless of the increases in PD and/or PS, the benthic foraminiferal specimens can accelerate the efficiency of oxygen uptake through increasing their surface porosity (Petersen et al., 2016; Rathburn et al., 2018; Giordano et al., 2019; Richirt et al., 2019; Lu et al., 2021). However, the foraminiferal individuals cannot indefinitely increase the porosity or decrease the thickness of their tests in an oxygen-depleted bottom environment (Glock et al., 2011; Richirt et al., 2019). For example, the genus Ammonia has an upper threshold of porosity of 30% (Richirt et al., 2019). Furthermore, the pores of sexual generation groups have a rounded and smooth edge, while those of asexual generation groups generally have some irregular papillae protruding into the jagged pores (Figure 2). The special exterior ornamentations of asexual generation groups may enhance their stability when they have higher PS (Richirt et al., 2019), and/or to disarticulate the frustules and tear the cell wall from algal prey for liberating chloroplasts in the process of asexual reproduction (Bernhard and Bowser, 1999). Further research is needed to fully disentangle the functions of this morphological feature.
Overall, in the hypoxic environment, the species B. robusta mainly chooses the asexual life cycle through rapid maturation to get more small generations to multiply the survival chances, and the high PS and complex exterior ornamentations around the pore edges may further enhance survival of asexual specimens. The asexual and sexual generations are similarly importantly in the oxic environment.
5 Conclusions
In this paper, the surface area, ratio of length/width, pore size, and pore number of tests from the living shallow-infaunal species B.robusta in the Changjiang estuary sediment were investigated, and the statistics of the large-elongate groups using the sexual reproduction and small-short groups using the asexual reproduction were described. Instead of selecting a partial test area, a more representative and comprehensive method based on the whole unilateral test for all specimens was used to explore the potential relationship between the foraminiferal PD and DO concentrations.
There was a significant negative correlation between PD and DO, and PD differed significantly in the hypoxic and oxic environments with the average PD in the hypoxic environment 36% higher than that in the oxic environment. The species B. robusta seemed to mainly choose the asexual life cycle with higher PS for reproduction and had irregular exterior ornamentations around the pore edges possibly enhancing their survival in the hypoxic environment.
The results of this study provide new insight into the benthic foraminiferal ecology to reconstruct the pale-oceanography and pale-ecology changes in the East China Sea, and may serve as a reference for comparison to other widely-distributed benthic foraminiferal species (e.g., B. albatrossi, B. spissa, B. pacifica, B. seminude, G. turgida, and F. mexicana) in different world areas. The PD versus DO relationship found this study indicates the potential of foraminiferal PD to be used as an independent proxy for reconstructing paleo-oxygen conditions in broad regions.
Data availability statement
The original contributions presented in the study are included in the article/Supplementary Material. Further inquiries can be directed to the corresponding authors.
Author contributions
FW: Conceptualization, Methodology, Writing- Review and Editing. SY: Methodology, Validation, Formal Analysis, Writing - Original Draft, Supervision. BZ, SG: Investigation, Data Curation. JW: Original Draft, Validation, Supervision. XF, JY, ZN: Methodology, Software. All authors contributed to the article and approved the submitted version.
Funding
This research was jointly supported the National Nature Science Foundation of China (Grant No. U20A20116), Laoshan Laboratory (Grant No. LSKJ202204201), Open Research Fund of Key Laboratory of Coastal Science and Integrated Management and Ministry of Natural Resources (Grant No. 2021COSIMZ003), and China Geology Survey (Grant No. DD20190208, DD20221710).
Acknowledgments
We are grateful to the sampling crews of the vessel “Runjiang”, as well as synchronous measurement (WD and DO) in the field and sample processing in the laboratory by Drs. Xiaoyi Guo, Haiming Nan.
Conflict of interest
The authors declare that the research was conducted in the absence of any commercial or financial relationships that could be construed as a potential conflict of interest.
Publisher’s note
All claims expressed in this article are solely those of the authors and do not necessarily represent those of their affiliated organizations, or those of the publisher, the editors and the reviewers. Any product that may be evaluated in this article, or claim that may be made by its manufacturer, is not guaranteed or endorsed by the publisher.
Supplementary material
The Supplementary Material for this article can be found online at: https://www.frontiersin.org/articles/10.3389/fmars.2023.1159614/full#supplementary-material
References
Bernhard J. M. (1988). Postmortem vital staining in benthic foraminifera: duration and importance in population and distributional studies. J. Foraminifer. Res. 18, 143–146. doi: 10.2113/gsjfr.18.2.143
Bernhard J. M., Bowser S. S. (1999). Benthic foraminifera of dysoxic sediments: chropolast sequestration and functional morphology. Earth Sci. Rev. 46, 149–165. doi: 10.1016/S0012-8252(99)00017-3
Bernhard J. M., Goldstein S. T., Bowser S. S. (2010). An ectobiont-bearing foraminiferal, Bolivina pacifica, that inhabits microxic pore waters: cell-biological and paleoceanographic insights. Environ. Microbiol. 12 (8), 2107–2119. doi: 10.1111/j.1462-2920.2009.02073.x
Berthold W. U. (1976). Ultrastructure and function of wall perforations in patellina corrugate, Williamson, foraminifera. J. Foraminifer. Res. 6 (1), 22–29. doi: 10.2113/gsjfr.6.1.22
Brunner C. A., Beall J. M., Bentley S. J., Furukawa Y. (2006). Hypoxia hotspots in the Mississippi bight. J. Foraminifer. Res. 36, 95–107. doi: 10.2113/36.2.95
Burone L., Ortega L., Franco-Fraguas P., Mahiques M., Garcia-Rodriguez F., Venturini N., et al. (2013). A mutiproxy study between the río de la plata and the adjacent south-western Atlantic inner shelf to assess the sediment footprint of river vs. marine influence. Cont. Shelf Res. 55, 141–154. doi: 10.1016/j.csr.2013.01.003
Chen C. C., Gong G. C., Shiah F. K. (2007). Hypoxia in the East China Sea: one of the largest coastal low-oxygen areas in the world. Mar. Environ. Res. 64, 399–408. doi: 10.1016/j.marenvres.2007.01.007
Corliss B. H., Emerson S. R. (1990). Distribution of rose Bengal stained deep-sea benthic foraminifera from the Nova Scotia continental margin and gulf of Maine. Deep-Sea Res. 37, 381–400. doi: 10.1016/0198-0149(90)90015-N
Dagg M., Benner R., Lohrenz S., Lawrence D. (2004). Transformation of dissolved and particulate materials on continental shelves influenced by large rivers: plume processes. Cont. Shelf Res. 24, 833–858. doi: 10.1016/j.csr.2004.02.003
De Stigter H. C., Jorissen F. J., van der Zwaan G. J. (1998). Bathymetric distribution and microhabitat partitioning of live (Rose Bengal stained) benthic foraminifera along a shelf to deep sea transect in the southern Adriatic Sea. J. Foraminifer. Res. 28, 40–65.
Diaz R. J. (2001). Overview of hypoxia around the world. J. Environ. Qual. 30, 275–281. doi: 10.2134/jeq2001.302275x
Dubicka Z., Zlotnik M., Borszcz T. (2015). Test morphology as a function of behavioral strategies-inferences from benthic foraminifera. Mar. Micropaleontol. 116, 38–49. doi: 10.1016/j.marmicro.2015.01.003
Farouk S., Jain S., Al-Kahtany K., Ahmad F., Abdeldaim A. (2023). Relationship of maastrichtian–thanetian benthic foraminiferal species diversity, palaeooxygenation, and palaeoproductivity in shallow waters of the Western desert, Egypt. Geo-Mar Lett. 43, 4. doi: 10.1007/s00367-023-00745-2
Gary A. C., Healy-Williams N., Ehrlich R. (1989). Water-mass relationships and morphological variability in the benthic foraminifer Bolivina albatrossi Cushman, northern gulf of Mexico. J. Foraminifer. Res. 19, 210–221. doi: 10.2113/gsjfr.19.3.210
Giordano L., Ferraro L., Salvatore M., Oscurato S. L., Maddalena P. (2019). Morphometric analysis on benthic foaminifera through atomic force microscopy. Mar. Micropaleontol. 153, 101775. doi: 10.1016/j.marmicro.2019.101775
Glock N., Eisenhauer A., Milker Y., Liebetrau V., Schönfeld J., Mallon J., et al. (2011). Environmental influences on the pore density of Bolivina spissa (Cushman). J. Foraminifer. Res. 41, 22–32. doi: 10.2113/gsjfr.41.1.22
Gong G. C., Chang J., Chiang K. P., Hsiung T. M., Hung C. C., Duan S. W., et al. (2006). Reduction of primary production and changing of nutrient ratio in the East China Sea: effect of the three gorges dam? geophys. Res. Lett. 33, L07610. doi: 10.1029/2006GL025800
Hein P., Geslin E. (2012). Ecological and biological response of benthic foraminifera under oxygen-depleted conditions: evidence from laboratory approaches. Springer Netherlands 21, 287–303. doi: 10.1007/978-94-007-1896-8_15
Jorissen F. J. (1999). Benthic foraminiferal successions across late quaternary Mediterranean sapropels. Mar. Geol. 153, 91–101. doi: 10.1016/S0025-3227(98)00088-7
Jorissen F. J., Fontanier C., Thomas E. (2007). Paleoceanographical proxies based on deep-sea benthic foraminiferal assemblage characteristics. Develop. Mar. Geol. 1, 263–325. doi: 10.1016/S1572-5480(07)01012-3
Jorissen F. J., Stigter H. C., Widmark J. G. W. (1995). A conceptual model explaining benthic foraminiferal microhabitats. Mar. Micropaleontol. 26, 3–15. doi: 10.1016/0377-8398(95)00047-X
Koho C., Pina-Ochoa E. (2012). Benthic foraminifera: inhabitants of low oxygen environments. Springer Netherlands 21, 251–285. doi: 10.1007/978-94-007-1896-8_14
Kuhnt T., Friedrich O., Schmiedl G., Milke R. Y., Mackensen A., Luckge A. (2013). Relationship between the pore density in benthic foraminifera and bottom-water oxygen content. Deep-Sea Res. Pt. I. 176, 85–95. doi: 10.1016/j.dsr.2012.11.013
Kuhnt T., Schiebel R., Schmiedl G., Milker Y., Mackensen A., Friedrich O. (2014). Automated and manual analyses of the pore density-to-oxygen relationship in Globobulimina turgida (Bailey). J. Foraminiferal Res. 44, 5–16. doi: 10.2113/gsjfr.44.1.5
Lee Y. G., Jeong D. U., Lee J. S., Choi Y. H., Lee M. O. (2016). Effect of hypoxia caused by mussel farming on benthic foraminifera in semi-closed gamak bay, south Korea. Mar. Pollut. Bull. 109, 566–581. doi: 10.1016/j.marpolbul.2016.01.024
Leutenegger S., Hansen H. J. (1979). Ultrastructural and radiotracer studies of pore function in foraminifera. Mar. Biol. 54, 11. doi: 10.1007/BF00387046
Lu W. Y., Barbosa C. F., Rathburn A. E., Xavier P., da M., Cruz A. P. S., et al. (2021). Proxies for paleo-oxygenation: a downcore comparison between benthic foraminiferal surface porosity and I/Ca. Palaeogeogr. Palaeocl. 579, 110588. doi: 10.1016/j.palaeo.2021.110588
McKee B. A., Aller R. C., Allison M. A., Bianchi T. S., Kineke G. C. (2004). Transport and transformation of dissolved and particulate materials on continental margins influenced by major rivers: benthic boundary layer and seabed processes. Cont. Shelf Res. 24, 899–926. doi: 10.1016/j.csr.2004.02.009
Moodley L., Hess C. (1992). Tolerance of infaunal benthic foraminifera for low and high oxygen concentrations. Biol. Bulletin. 183, 94–98. doi: 10.2307/1542410
Murray J. W. (2006). Ecology and application of benthic foraminifera (Cambridge University Press), 1–386.
Oron S., Angel D., Tchernov B. G., Merkado G., Kiflawi M., Abramovich S. (2014). Benthic foraminiferal response to the removal of aquaculture fish cages in the gulf of aqaba-eilat, red Sea. Mar. Micropaleontol. 107, 8–17. doi: 10.1016/j.marmicro.2014.01.003
Osterman L. E., Poore R. Z., Swarzenski P. W. (2008). The last 1000 years of natural and anthropogenic low-oxygen bottom water on the Louisiana shelf, gulf of Mexico. Mar. Micropaleontol. 66, 291–303. doi: 10.1016/j.marmicro.2007.10.005
Pérez-Cruz L., Machain Castillo M. L. (1990). Benthic foraminifera of the oxygen minimum zone, continental shelf of the gulf of tehuantepec, Mexico. J. Foraminiferal Res. 20 (4), 312–325. doi: 10.2113/gsjfr.20.4.312
Petersen J., Riedel B., Barras C., Pays O., Guihéneuf A., Mabilleau G., et al. (2016). Improved methodology for measuring pore patterns in the benthic foraminiferal genus Ammonia. Mar. Micropaleontol. 128, 1–13. doi: 10.1016/j.marmicro.2016.08.001
Piña-Ochoa E., Høgslund S., Geslin E., Cedhagen T., Revsbech N. P., Nielsen L. P., et al. (2010). Widespread occurrence of nitrate storage and denitrification among foraminifera and gromiids. Proc. Natl. Acad. Sci. 107, 1148–1153. doi: 10.1073/pnas.0908440107
Qian W., Dai M. H., Xu M., Kao S. J., Du C. J., Liu J. W., et al. (2017). Non-local drivers of the summer hypoxia in the East China Sea off the changjiang estuary. Estuar. Coast. Shelf S. 198, 393–399. doi: 10.1016/j.ecss.2016.08.032
Rathburn A. E., Corliss B. H. (1994). The ecology of living (Stained) deep-sea benthic foraminifera from the sulu Sea. Paleoceanography 9, 87–150. doi: 10.1029/93PA02327
Rathburn A. E., Willingham J., Ziebis W., Burkett A. M., Corliss B. H. (2018). A new biological proxy for deep-sea paleo-oxygen: pores of epifaunal benthic foraminifera. Sci. Rep. 8, 9456. doi: 10.1038/s41598-018-27793-4
Resig J. M., Glenn C. R. (2003). Sieve plates and habitat adaptation in the foraminifer planulina ornata. Pacific Sci. 57 (1), 103–110. doi: 10.1353/psc.2003.0007
Richirt J., Champmartin S., Schweizer M., Mouret A., Petersen J., Ambari A., et al. (2019). Scaling laws explain foraminiferal pore patterns. Sci. Rep. 9, 1–11. doi: 10.1038/s41598-019-45617-x
Shen H. T., Huang Q. H., Liu X. C. (2006) LOICZ budgets for estuaries. Available at: http://data.ecology.su.se/mnode/Asia/China/yangtze/yangtze.htm.
Sjoerdsma P. G., van der Zwaan G. J. (1992). Simulating the effect of changing organic flux and oxygen conent on the distribution of benthic foraminifera. Mar. Micropaleontol. 19, 163–180. doi: 10.1016/0377-8398(92)90027-H
Swarzenski P. W., Campbell P. L., Osterman L. E., Poore R. Z. (2008). A 1000-year sediment record of recurring hypoxia off the Mississippi river: the potential role of terrestrially derived organic matter inputs. Mar. Chem. 109, 130–142. doi: 10.1016/j.marchem.2008.01.003
Tyson R. V., Pearson T. H. (1991). Modern and ancient continental shelf anoxia: an overview. Geological Soc. London Special Publicat. 58, 1–24. doi: 10.1144/GSL.SP.1991.058.01.01
van der Zwaan G. J., Duijnstee I. A. P., den Dulk M., Ernst S. R., Jannink N. T., Kouwenhoven T. J. (1999). Benthic foraminifers: proxies or problems? a review of paleocological concepts. Earth Sci. Rev. 46, 213–236. doi: 10.1016/S0012-8252(99)00011-2
Walton W. R. (1952). Techniques for recognition of living foraminifera. Contribut. Cushman Foundation Foraminiferal Res. 3, 56–60.
Wang B. D. (2009). Hydromorphological mechanisms leading to hypoxia off the changjiang estuary. Mar. Environ. Res. 67, 53–58. doi: 10.1016/j.marenvres.2008.11.001
Wang H. J., Dai M. H., Liu J. W., Kao S. J., Zhang C., Cai W. J., et al. (2016). Eutrophication-driven hypoxia in the East China Sea off the changjiang estuary. Environ. Sci. Technol. 50, 2255–2263. doi: 10.1021/acs.est.5b06211
Wang F. F., Liu J., Qiu J. D., Wang H. (2014). Historical evolution of hypoxia in the East China Sea off the changjiang (Yangtze river) estuary for the last ~13,000 years: evidence from the benthic foraminiferal community. Cont. Shelf Res. 90, 151–162. doi: 10.1016/j.csr.2014.02.013
Wang B., Wei Q., Chen J., Xie L. (2012). Annual cycle of hypoxia off the changjiang (Yangtze river) estuary. Mar. Environ. Res. 77, 1–5. doi: 10.1016/j.marenvres.2011.12.007
Wang F. F., Yu Z. G., Xu B. C., Liu J., Guo X. Y., Nan H. M. (2018). Nepartak typhoon influenced bottom sediments from the Yangtze river estuary and adjacent East China Sea-foraminiferal evidence. Geochem. Geophy. Geosy. 19 (4), 1049–1063. doi: 10.1002/2017GC007413
Wang P. X., Zhang J. J., Zhao Q. H., Min Q. B., Bian Y. H., Zheng L. F., et al. (1988). Foraminifera and ostracoda in bottom sediments of the East China Sea Vol. 147 (China Ocean Press).
Wu L. N., Wei Q. S., Ran X. B., Sun J. C., Wang B. D. (2021). Dissolved oxygen distributions, hypoxic and acidification characteristics, and their controlling factors in the southern yellow Sea and off the changjiang estuary. China Environ. Sci. 41 (3), 1311–1324. doi: 10.19674/j.cnki.issn1000-6923.20201105.001
Zhao Y. Q., Zhou L., Zhao N., Sheng H., Wang Y. P. (2021). The response of sedimentary records in the mud area of the changjiang estuary to hypoxia in the last 300 years. Mar. Sci. Bulletin. 40 (1), 70–83. doi: 10.11840/j.issn.1001-6392.2021.01.009
Keywords: East China Sea, benthic foraminifera, pore density, hypoxia, alternation of generations, proxy of environmental conditions
Citation: Wang F, Yang S, Zhai B, Gong S, Wang J, Fu X, Yi J and Ning Z (2023) Pore density of the benthic foraminiferal test responded to the hypoxia off the Changjiang estuary in the East China Sea. Front. Mar. Sci. 10:1159614. doi: 10.3389/fmars.2023.1159614
Received: 06 February 2023; Accepted: 03 May 2023;
Published: 18 May 2023.
Edited by:
Sherif Farouk, Egyptian Petroleum Research Institute, EgyptCopyright © 2023 Wang, Yang, Zhai, Gong, Wang, Fu, Yi and Ning. This is an open-access article distributed under the terms of the Creative Commons Attribution License (CC BY). The use, distribution or reproduction in other forums is permitted, provided the original author(s) and the copyright owner(s) are credited and that the original publication in this journal is cited, in accordance with accepted academic practice. No use, distribution or reproduction is permitted which does not comply with these terms.
*Correspondence: Feifei Wang, ZmZ3YW5nMTIzQDE2My5jb20=; Shixiong Yang, eXN4XzY2NkAxNjMuY29t