- 1Fisheries College, Guangdong Ocean University, Zhanjiang, China
- 2Guangdong Provincial Key Laboratory of Aquatic Animal Disease Control and Healthy Culture, Guangdong Ocean University, Zhanjiang, China
As the global ocean continues to experience the consequences of an increase in the frequency and intensity of heat waves, the trend is expected to persist into the 21st century, with a projected tripling of heat waves by 2040. This phenomenon poses a significant threat to marine ecosystems and the survival of marine organisms, including the ecologically and economically vital bivalves. Bivalves are vulnerable to harm from heat stress at various levels of biological organization, and their growth can be negatively impacted by high temperatures, potentially leading to mass mortalities and posing a threat to ecosystem quality and food security. In light of these concerns, this review aims to provide a comprehensive examination of the effects of heat stress on bivalves. It summarizes the physiological and biochemical changes that bivalves undergo in response to extreme heat events and offers an overview of the strategies they employ to mitigate their impacts. A better understanding of the underlying mechanisms of bivalve responses to heat stress is crucial in order to fully appreciate the impact of these events on these organisms. This review synthesizes the current knowledge on heat stress in bivalves and highlights the importance of further research in this area. By providing a comprehensive overview of the physiological and biochemical changes that bivalves experience during heat stress and the strategies they use to mitigate its impact, this review aims to support the development of more effective approaches to minimize heat stress in bivalves.
1 Introduction
Projections indicate an increase in the frequency and severity of heat waves (Figure 1) during the contemporary era (Perkins-Kirkpatrick et al., 2022). Although multiple definitions exist, heat waves are typically defined as extended periods with temperatures significantly higher than average (Perkins and Alexander, 2013). A trend of rising record-breaking temperatures in months refers to the average temperatures observed in a particular month over a given period of time. For instance, January temperatures in a particular location would refer to the average temperature recorded for that month over a given period of time, which has been observed since the late 19th-early 20th century, with the number of such months increasing five-fold (Coumou and Robinson, 2013; Coumou et al., 2013). Extreme heat events are a manifestation of global warming, as rising temperatures increase the frequency, duration, and intensity of these extreme heat events (Coumou and Robinson, 2013; Dulière et al., 2013). Life on Earth has evolved with optimal temperatures for certain tasks, known as their thermal niche (Bennett et al., 2021). Exceeding these temperature limits can result in declines in performance and even mortality (Smith et al., 2021). Global warming is causing the Earth’s atmosphere to warm up, which in turn is leading to an increase in ocean temperatures. According to the IPCC, the average global ocean temperature is projected to increase by 0.2°C to 0.5°C by the end of the 21st century under a low-emissions scenario and 1.8°C to 4.4°C under a high-emissions scenario. However, the actual temperature rise will depend on the extent to which greenhouse gas emissions are reduced and the effectiveness of mitigation efforts to limit global warming (Alfonso et al., 2021; IPCC,2021).
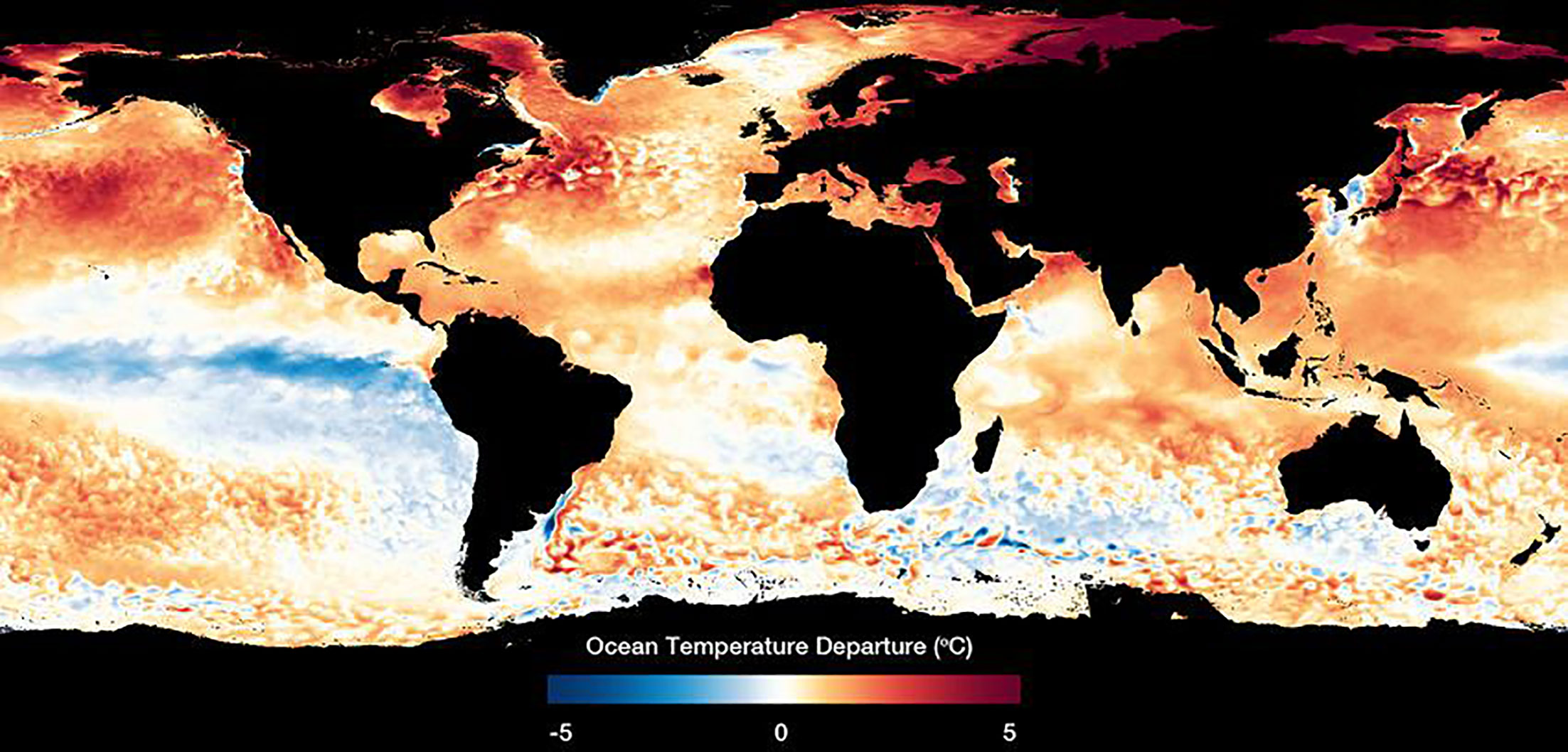
Figure 1 Sea surface temperature anomalies were measured in September 2020 (IUCN, 2021).
As our planet continues to warm, many organisms are being pushed beyond their upper-temperature thresholds with increasing frequency and intensity (Smith et al., 2021). Warming-induced responses have been identified at multiple levels, from individual genes to entire ecosystems, and profoundly impact human societies. The compounding effects of climate change and natural disasters have the potential to trigger dramatic ecological transformations. Heat stress, a consequence of thermal disequilibrium, can have detrimental effects on organisms (Oliver et al., 2018). Factors that contribute to this disequilibrium include regional climate conditions as well as a range of observable factors, such as thermal irradiance, temperature, relative humidity, movement, species, genetic lineage, sex, age, enzyme production, and thermoregulation ability (Benestad et al., 2005; Rostagno et al., 2020).
Heat stress is a widely researched topic in both animal and plant biology. Its financial impact on the livestock and plant agriculture industries has made it a focus of study. However, the bivalve aquaculture industry, a rapidly growing sector in the global food economy, remains under-explored in the context of heat stress. Bivalves, including oysters, mussels, and clams, are a significant source of animal protein and contribute 14-16% of the average protein consumed by humans. The industry is dominated by Asia, which accounts for 92.27% of the world’s total aquaculture output and 21.42% of the mollusk aquaculture output. The impact of heat stress on bivalves, from larvae to juveniles and adults, has been extensively documented and is a leading cause of global mass mortality in farmed bivalves (Leverone et al., 2007; Vohmann et al., 2009; Malham et al., 2012; Defeo et al., 2013; Yurimoto et al., 2014; McFarland et al., 2016; Ortega et al., 2016). These trends are expected to intensify with climate change and the growing demand for bivalves. Bivalves play a crucial role in marine ecosystems, providing biomass for predators, a substrate for epibionts, topographic relief, and serving as a source of calcium carbonate (Dame, 2011; Glynn and Enochs, 2011). Given the ecological significance of bivalves and the importance of the bivalve aquaculture industry, it is imperative to conduct comprehensive studies on the effects of heat stress on these species and explore methods for mitigating their impacts.
Bivalves’ mortality and stress thresholds can vary significantly based on species, environmental conditions, and geographic location (Thomas and Bacher, 2018). Generally, bivalves are highly sensitive to temperature changes, and even minor deviations from their ideal temperature range can have adverse impacts on their physiology and behavior. High temperatures are usually more detrimental to bivalves than low temperatures, with some species experiencing mortality at temperatures exceeding 30°C (86°F), although lower temperatures can also result in mortality if the exposure is prolonged (Seuront et al, 2019) Bivalves can also experience stress when subjected to temperatures outside of their preferred range, even if such temperatures do not cause mortality. Research indicates that some bivalve species may experience stress when exposed to temperatures above 25°C (77°F) (Masanja et al, 2022) or below 10°C (50°F) (Boroda et al, 2020), although the precise threshold can vary depending on the species and environmental conditions.
Heat stress in bivalves can manifest as either acute or chronic forms, with acute stress resulting from sudden increases in environmental temperature and chronic stress arising from extended exposure to high temperatures. The impacts of heat stress on bivalve welfare are well documented, including cellular damage and oxidative stress, leading to impaired immune function, elevated inflammation, shifts in microbial populations, and reduced growth (Aagesen and Häse, 2014; Rahman et al., 2019; Rahman and Rahman, 2021). To develop effective heat stress mitigation strategies, it is crucial to establish accurate methods for assessing heat stress in bivalves. With global temperatures projected to rise by 1.5°C over the next two decades (IPCC, 2021), innovative approaches are needed to minimize heat stress. This review aims to provide a comprehensive overview of the underlying mechanisms of heat stress in bivalves, utilizing these organisms as models due to their vulnerability to heat stress-related mortality events. Evidence suggests that heat stress is not a simple physiological response to high temperatures but encompasses physiological and molecular-level responses. The review also explores techniques for mitigating heat stress, which have potential applications for bivalve aquaculture and the broader aquaculture sector.
2 Marine heat extremes
Marine heat extremes, characterized by sustained elevations in seawater temperatures (Figure 2), have become more frequent and intense in recent years (Frölicher et al., 2018; Oliver et al., 2018). This trend’s root cause is long-term anthropogenic change, which has led to a steady rise in ocean temperatures (Frölicher et al., 2018). These marine heat extremes have devastatingly impacted ecosystems, maritime industries, and human activities, leading to mass mortality events and other adverse effects (Frölicher et al., 2018; Smith et al., 2022). In particular, sessile species, such as bivalves, coral, algae, and sponges, are particularly vulnerable to acute temperature stress as they cannot escape extreme climatic conditions (Smith et al., 2022). This has caused a change in bivalve ecosystems in combination with long-term warming (Smith et al., 2022).
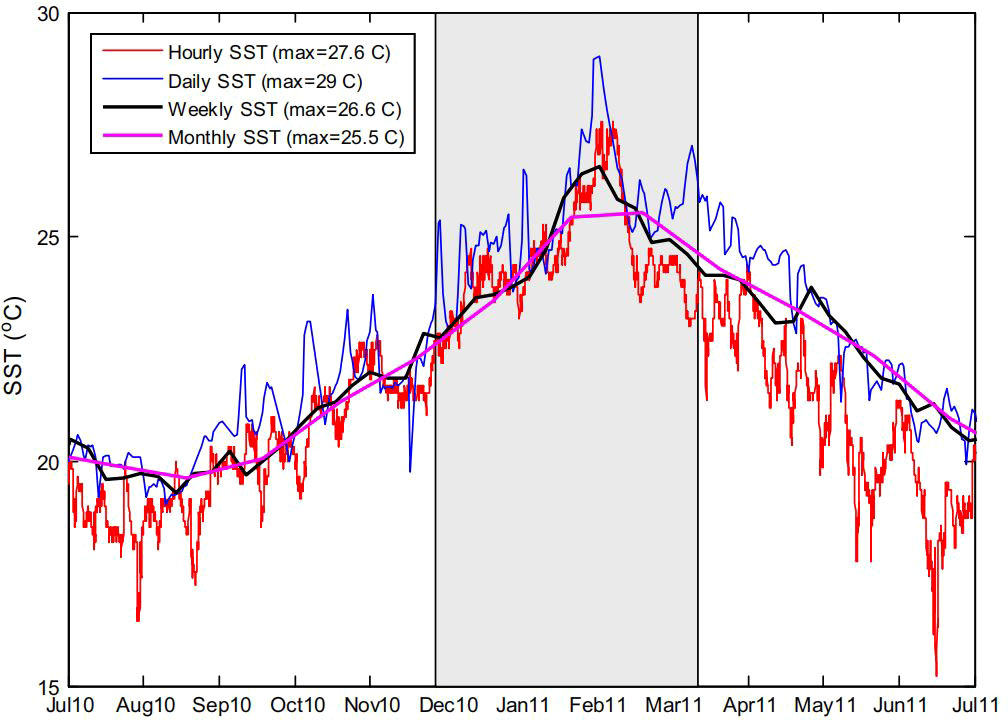
Figure 2 A hierarchical approach to defining marine heatwaves This figure caption displays the temperature variations over a twelve-month period around the Ningaloo Niño marine heatwave of 2011. The shaded area denotes the months from December 2011 to April 2012, off the coast of Western Australia. The data were obtained from four distinct sources: weekly and monthly Reynolds SST measurements (29.5-30.5S; 114.5-115.5E), daily satellite SST (29.5-30.5S; 114.5-115.5E), and an hourly in-situ logger from Jurien Bay (30 18.5S; 114 58.3E). These results were reported in a study by Hobday et al. (2016).
To provide a comprehensive overview of the impact of marine heat extremes on bivalve species, we conducted a literature review on heat stress and bivalves. We utilized multiple databases, including Google Scholar, Research Gate, Semantic Scholar, Science Direct, Springer, and Web of Science. We searched for relevant keywords such as “heat stress,” “bivalve,” “marine heat stress,” “heat stress tolerance,” and “heatwave acclimation.” Our selection criteria were based on the relevance of the studies to our research questions from published articles from 2000-2022. The results of our literature review draw from a range of studies, including heat exposure tests in the lab and similar studies on other organisms. To ensure the validity of our conclusions, we established relevant comparisons and incorporated relevant literature. This review provides a comprehensive understanding of the impact of marine heat extremes on bivalve species and highlights the importance of further research in this area.
3 How heat stress affects bivalves
Heat stress is expected to become long-lasting and more frequent, affecting coastal habitats, particularly intertidal species living in the vicinity of their temperature tolerance limits. Species and communities of bivalves exhibit acclimation abilities, which are impacted by their thermal histories and frequencies of temperature changes (Peck et al., 2002; Xu et al., 2021; Xu et al., 2022). A temperature increase in each organism’s tolerance limits can speed up various biological processes, including metabolism, development, filtration rate, and absorption efficiency. Similarly, many bivalves exhibit the capacity to adapt over long periods, but at a cost. On the other hand, acute and rapid temperature increases may cause more substantial stress, resulting in sublethal and fatal effects (Gosling, 2015; Macho et al., 2016; He et al., 2021; Xu et al., 2021).
3.1 Heat stress effects on behavior
Bivalves elicit behavioral reactions to preserve core body homeostasis as the first line of defense. Whether it is cold or heat stress, maintaining homeostasis requires balancing heat production and heat loss (Table 1). Thermal stress frequently causes behavioral responses and, in exceptional circumstances, may prove fatal (Verdelhos et al., 2015). Ecological processes and connections between species are impacted due to bivalve behavior changes induced by heat stress (Nagelkerken and Munday, 2016). One observed behavior change was by surfacing during heatwaves; a prime example, Cerastoderma edule (Rahman et al., 2019) residing in water pools subjected to the warmth of the sun can surface after the high tide or gradually during the low tide, depending on how the sediment surface is laid out. When exposed to the same level of heat stress, cockles travel in opposite directions: upward in sediment pools beneath the surface and downwards in well-drained sediments (Malham et al, 2012; Frölicher et al., 2018; Zhou et al, 2022). Another behavior pattern is observed in mussels as it is common to see groups of mussels forming complicated beds of mussels with their byssal connection threads (Manríquez et al., 2021). For juvenile and adult mussels with many other species, this intricate bed structure serves as a moist and cool microhabitat (Suchanek, 1978; Miller and Dowd, 2019). Mussel byssal thread attachment is also known to be weaker in higher water temperatures, causing dropoff from rope-grownn mussel farms,heatwave-induced bioturbation has a more significant impact on cohesive muddy systems than on non-cohesive sandy systems hence sediment type will have a significant impact on its magnitude at the landscape level (Li et al., 2017; Soissons et al., 2019); this results in behavioral reactions to thermal stress in infauna bivalves, such as digging away to keep away from the hot surface (Sakurai et al., 1996; Verdelhos et al., 2015).
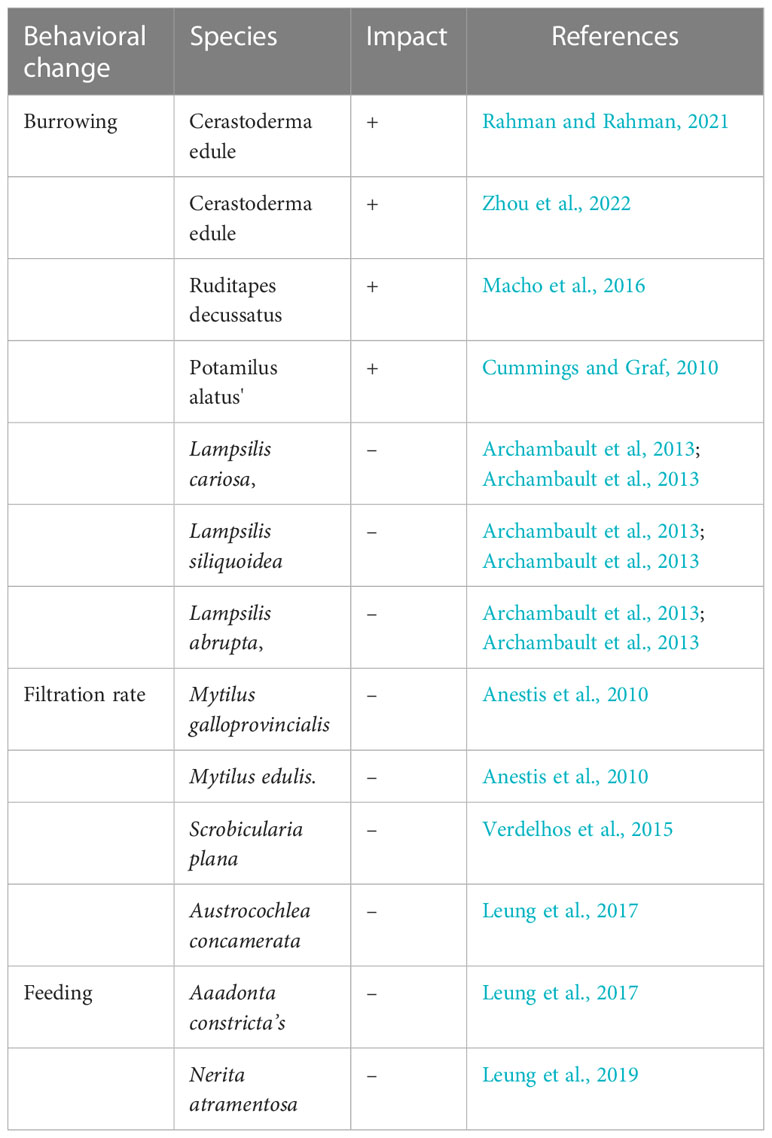
Table 1 Behavioral changes in bivalves in response to heat stress (+ representing increase, and – representing decrease).
Burrowing depth can be observed due to heat, but siphons continue to inhale heated water for respiration and filtration if the organism remains active (Macho et al., 2016). Burrowing deeper or for a more extended period to avoid the stressor is a behavioral response that can occur when exposed to rapidly increasing water temperatures (Bertolini and Pastres, 2021). Burrowing depths vary among species. Ruditapes decussatus, for example, burrows down to 8 centimeters, while Ruditapes philippinarum prefers shallower depths of 3 centimeters (Macho et al., 2016). It came to our attention that the heat affected the burrowing habits of the Potamilus alatus, such as the likelihood related to burrowing and the delay in first foot extension (Cummings and Graf, 2010). Researchers realized mussel burrowing was negatively affected by rising temperatures in species such as Lampsilis cariosa, Lampsilis abrupta, and Lampsilis siliquoidea (Archambault et al., 2013).
Bivalve behavior changes to keep their valves partially open, affecting the volume of water they filter and, thus, the quantity of food they absorb (Anestis et al., 2007). When the temperature is > 25°C, filtration reduces significantly in the bivalves Mytilus galloprovincialis and Mytilus edulis (Anestis et al., 2010), - M. galloprovincialis acclimated to 24°C, showed longer valve closure times (Galimany et al., 2011). Robson, 2008 observed valve gaping behavior in mussels Scrobicularia plana and Cerastoderma edule were observed to valve-gape during heat exposure to deter predators and feed (Verdelhos et al., 2015). When temperatures reach critical levels, certain bivalve species may be able to cope with these stressors for a short time through behavioral mechanisms.
3.2 Influence of heat stress on bivalve growth
Both biotic and abiotic factors influence the growth and size of organisms. Among the abiotic factors, temperature plays a critical role. It is responsible for one of the well-established responses known as the temperature-size rule, where body mass declines as temperatures rise (Daufresne et al., 2009). However, the effects of warming temperatures on organisms are complex, and when optimal temperature ranges are exceeded, they can result in reduced growth (Ferreira-Rodríguez et al., 2018; Xu et al., 2023b).
Temperature also has significant impacts on the growth, spawning, and development of bivalve larvae such as Mercenaria mercenaria and Argopecten irradians (Eversole, 2001; Eversole, 2001; Barber and Blake, 2006; Tomasetti et al., 2023). Successful larval spawning and growth of bivalves depend critically on the optimal temperature range of 17-24°C, which has been identified for several species, such as Olympia oysters and Mactra chinensis (Lawlor and Arellano, 2020; Jwa and Hong, 2022). However, the optimal growth of bivalves calculated from the most common bivalve, which are oysters, clams, scallops, and mussels, occurs at an average temperature of 18°C, with a range of 12-24°C; studies also found that there was a significant error associated with the average, with a standard deviation of 2°C, higher temperatures can slow growth and increase mortality rates (Fritz et al., 2001; Rico-Villa et al., 2009; Acquafredda et al., 2019; Arrieche et al., 2020). Heatwaves can cause significant physiological stress in marine organisms, leading to differences in growth and even declines in population.
For instance, a rapid temperature increase caused a significant decline in the stock of Ruditapes philippinarum (Ponti et al., 2017; Sampaio et al., 2018). Similarly, Ostrea edulis experiences a decrease in individual biomass gains when exposed to heat waves, and extreme heat reduces the growth and shell strength of M. gigas (Mackenzie et al., 2014; Leung et al., 2017; Lemasson et al., 2018; Gilson et al., 2021). Scallop shell length decreased by 2% under 2-3°C warmer temperatures, leading to significant changes in growth and flesh quality (Fernández-reiriz et al., 2011; Hart, 2014; Johnston et al., 2021).
The average heat stress duration that can elicit an appreciable effect on bivalve growth can vary depending on the species of bivalve, the intensity and duration of the heat stress, and other factors salinity, nutrient availability, and human activities (Roegner and Mann, 1995; Roegner and Mann, 1995).
3.3 How heat stress impacts bivalves’ energy metabolism
Stability in the energy balance is essential for adapting to stressful situations and developing a tolerance for them (Parsons, 2005; Sokolova et al., 2012). Sample investigations have shown the value of energy homeostasis as a measurement method (Shin et al., 2009; Blevins et al., 2012), including many stressors’ effects on marine species at several levels of biology (Sokolova et al., 2012) and importantly, for establishing a direct connection between the fitness of individuals and the effects on populations and ecosystems (Goodchild et al., 2019). The capacity for continued existence and productive behavior, as well as to adapt to and tolerate stress, is closely linked to its energy metabolism. There will always be a limit to an organism’s ability to gain and use energy and store it metabolically (Sokolova, 2013). Effective energy management is crucial for maintaining an organism’s overall health. To this end, regulating the amount of energy expended and its allocation is paramount. In fact, exposure to environmental stress, such as thermal stress, can result in increased energy demands, which can severely impact an organism’s energy balance, as previously demonstrated by Sokolova et al. (2012) and Chovatiya and Medzhitov, 2014. The impact of thermal stress on behavior is particularly noticeable in species with high metabolic activity and mobility. This stress can put a strain on energy-related processes such as acquisition, conversion, and conservation systems, as observed by Kooijman and Kooijman (2010). This is evident in the upregulation of lactate dehydrogenase (LDH), which means the increase in the production of LDH by cells in Littorina saxatilis, one of the critical enzymes of anaerobic metabolism (Hochachka, 1973; Sokolova and Pörtner, 2001). Similar experimentation in Pinctada fucata and Dosinia corrugata with heat stress reduced lactate dehydrogenase activity, which refers to a reduction in the enzyme’s catalytic activity, which is intimately linked to cell metabolism (Nie et al., 2018; Zhang et al., 2022a). R. philippinarum enzymes Na+/K+-ATPase (NKA), Ca2+/Mg2+-ATPase (CMA), and Total-ATPase (T-ATP), which are involved in energy metabolism, responded differently to heatwaves. For example, R. philippinarum activity of NKA under the first heatwave exposure significantly depressed NKA; however, following the second exposure, NKA activity increased significantly; compared with NKA, contrasting responses of CMA to heatwaves were observed (He et al., 2022b). During heatwave experiments on clams, respiratory rates and levels of proteins and triglycerides were almost twice as high during exposure to elevated temperatures compared to the control temperature (Falfushynska et al., 2019; Carneiro et al., 2020). Triglycerides may be employed in the bivalve to store energy for metabolic maintenance during periods of limited thermal stress (Gabbott, 1976; Fraser et al., 2002; Pernet et al., 2007a) because biochemical synthesis is an energy-intensive physiological process (Pernet et al., 2007b). When exposed to heatwave simulations, bivalves were able to slow down the increase in filtration and respiratory rates, which helped them cope with the additional energy demand that could not be met through respiration alone. This suggests that bivalves use filtration to mitigate the impact of temperature changes (Bayne, 1993; Kittner and Riisgård, 2005).
The metabolic rate of bivalves continues to rise in response to increasingly demanding environmental conditions, such as those caused by rising temperatures (Kordas et al., 2011; Thomas and Bacher, 2018). The activation of molecular defense systems necessitates a significant amount of metabolic energy (Abele et al., 2004; Somero, 2020); hence, the elevation of aerobic metabolism is necessary during heat waves. Nevertheless, marine species may also be able to down-regulate their aerobic metabolism to save energy and not use molecular defense systems, despite possible cellular harm (Strahl, 2011; González et al., 2015; Xu et al., 2023b), whereas other findings show that when there is a heatwave, aerobic metabolism increases quickly (Peck et al., 2002; Stevens and Gobler, 2018). Acute exposure of Pinctada maxima to heatwaves reduced chaperone-mediated autophagy, Na/K-ATPase, and T-ATP activities, indicating a metabolism decline (Yao and Somero, 2013). When the bivalve’s body’s temperature reaches a crucial point for survival, metabolic depression is a mechanism to conserve energy (Storey and Storey, 1990). It involves alterations to the body’s biochemistry and metabolism (Nie et al., 2018). The synthesis of proteins is an energy-intensive process for the cell; thus, these metabolic changes try to conserve energy and adjust to the obstacles presented by higher temperatures. (Carneiro et al., 2021).
Bivalves obtain only a small amount of energy at low temperatures, whereas they use more than they gain (Sokołowski and Brulińska, 2018). Alternatively, the temperature has been demonstrated to affect metabolic rates in different bivalves, including Pinctada margaritifera (Aldridge, 1999; Hicks and McMahon, 2002; Le Moullac et al., 2007). It takes much energy to activate transcription, synthesize heat-shock proteins, and group with HSP chaperoned ATP-dependent throughout the heat-shock response. Research by Hawkins and Bayne (1985) showed that Mytilus edulis uses 20%–25% of its total energy budget in the production of the heat shock protein; similar research demonstrated that as temperatures were increased progressively >7°C, Laternula elliptica metabolism increased to new levels (Peck et al., 2002). The Nacella concinna showed different peaks of temperature-induced changes in metabolic rate, followed by partial acclimatization (Pörtner et al., 2007; Kronschnabel, 2020). Some species have a lower average metabolism level than temperate or tropical species. This indicates that some marine species are more efficient with their use of energy because of being impacted by heat.
3.4 Immunity challenged upon exposure to heat stress
Pathogen development, disease dissemination, and host vulnerability in bivalves have increased because of climate change in the last century (Williams et al., 2021). Because of the rising temperature, parasites are becoming more prevalent in bivalves. Numerous environmental elements, such as rapid increases in temperature, affect the immune system’s ability to function effectively (Parkin and Cohen, 2001), harm immunity, and increase the frequency of illnesses (Gestal et al., 2008). Bivalves’ resistance to parasites and other pathogens may be lowered by high temperatures, reducing their enzymatic and phagocytic activities (Longshaw and Malham, 2013; Thieltges et al., 2006). Infections can increase mortality in two ways: directly and indirectly. The neuroendocrine system responds quickly to extrinsic influences and reduces preliminary stress by modulating immune processes to maintain thermal stress (Liu et al., 2018; Joyce et al., 2018). Regarding bivalves, innate immunity is the primary line of defense (Zimmerman et al., 2010). Transcriptional analysis shows that heat stress affects gene expression in monooxygenase, neuropeptide Y receptor, steroid hormone-mediated signaling, and N-acetyl transferase. (Liu et al., 2017a). Additionally, the neuroendocrine system can regulate immunity in marine bivalves by synthesizing immune-related enzymes, as exemplified in the scallop Chlamys farreri; acute heat stress increases superoxide dismutase activity, which reverses by blocking the adrenergic receptor binding activity (Zhang et al., 2014).
Bivalves’ principal defense mechanism against pathogens is hemocytes (Nguyen and Alfaro, 2020). Proteins and the circulation of hemocytes all play a role in bivalves to withstand both bacterial and temperature stress (Rahman et al., 2019). Vibrio harvey induced hemocyte expression was significantly enhanced in bivalves by the sudden increase in temperature (Lei et al., 2016). Recognition, phagocytosis, and removal of foreign organisms by enzymatic or oxidative breakdown are the functions of the hemocytes (Castillo et al., 2015). Phagocytic hemocytes do this in the organism’s blood and hemolymph (Hégaret et al., 2003). Lysosomal membrane integrity and phagocytosis impair Mytilus galloprovincialis during elevated temperature (Parisi et al., 2017). Mytilus coruscus’ hemocyte, phagocytosis rate, and lysosomal content were all drastically reduced, triggered by chronic exposure to high temperatures (Wu et al., 2016). The findings of previous studies align with the current research, which observed an increase in the number of hemocytes in several bivalve species, including Crassostrea gigas (Rahman et al., 2019), Mytilus galloprovincialis (Yao and Somero, 2012), in response to rising water temperatures. This increase in hemocyte count may be due to cell mobilization from tissues into the hemolymph (Matozzo and Marin, 2011). However, exposure to elevated temperatures can also negatively impact bivalve immunity by affecting various hemocyte parameters, including number, motility, viability, adhesive capability, phagocytic ability, membrane permeability, and intracellular enzyme activities (Fisher, 1988; Monari et al., 2007). This can lead to a weakened immune system in bivalves. Studies have shown that a thermal gradient is associated with an increased proportion of granulocytes and higher mortality of hyalinocytes in species such as Crassostrea virginica (Chu and La Peyre, 1993), Ruditapes philippinarum (Flye-Sainte-Marie et al., 2009), Chlamys farreri (Lin et al., 2012), and Pinctada fucata martensii (Li et al., 2015). On the other hand, low values of hemocytes have been observed at high water temperatures (Table 2). A correlation between rising temperatures and a reduction in the percentage of granulocytes was also identified in Spisula solidissima (Hornstein et al., 2018), Unio pictorum (Beggel et al., 2017), and Melemaea virgata (Hong et al., 2021). In order to understand the impacts of heat stress on bivalve immunity, further research is needed to examine the functional characteristics of the immune system in bivalves under these conditions (Gestal et al, 2008; Allam and Pales Espinosa, 2016). This will aid in establishing valid criteria for assessing the effects of heat stress on bivalves.
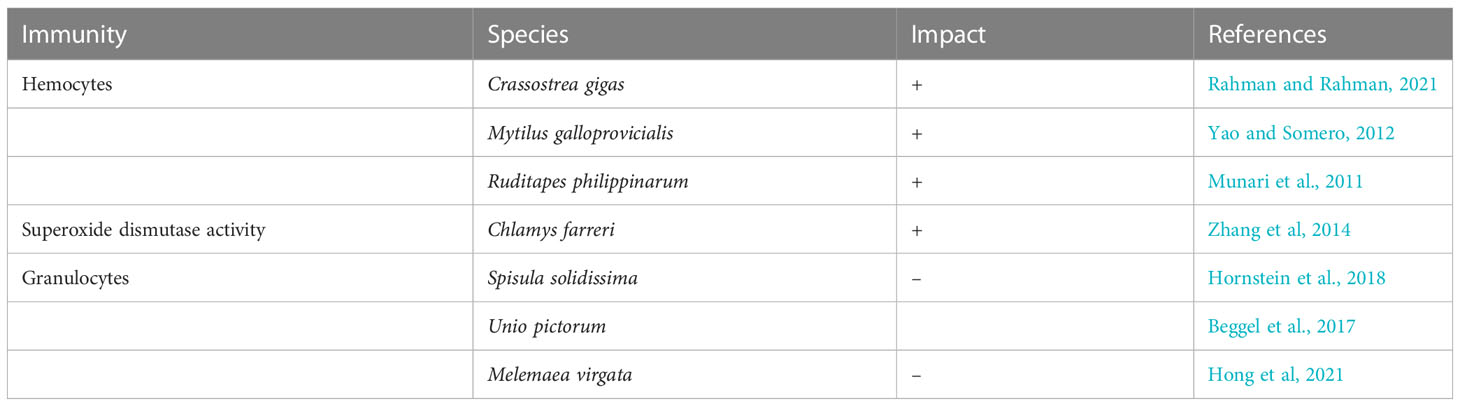
Table 2 Immunity impacted by heat stress (+ representing upregulation, and – representing downregulation).
3.5 Molecular response on bivalves due to heat stress
Heat stress can trigger changes at the molecular level and responses at the gene level in bivalves. One known molecular response to heat stress is the over-expression of Heat Shock Proteins (HSPs), which can have adverse effects. When heat-shock reactions occur, the synthesis of almost all other proteins is suppressed simultaneously, suggesting that HSP production might come at a high energetic cost since they can make up a significant proportion of cellular protein during stress (Ferreira-Rodríguez et al., 2018). Studies have shown that exposure to heat stress can lead to upregulation patterns of specific HSPs in bivalves. For example, following encounters with heatwaves, upregulation patterns of HSP90 were observed in Pinctada maxima after six hours of exposure (Masanja et al.,2022). Similarly, in Laternula elliptica, treatment with acute temperatures for 12 hours led to HSP90 upregulation patterns (Kim et al., 2009). Additionally, HSP70 was broadly expressed in gill tissues of various bivalve species, including Laternula elliptica, Ruditapes philippinarum, Chlamys nobilis, and Pinctada maxima, during exposure to heat stress lasting from six to 24 hours (Park et al, 2007; Nie et al., 2017; Cheng et al., 2019; Xu et al., 2021). Moreover, upregulation patterns of HSP69 have been observed in bivalves’ gill tissues in response to heat stress after 24 hours of exposure (Roberts et al., 1997; Chapple et al., 1998; Kregel, 2002; Piano et al., 2004; Hofmann, 2005).
Organisms have evolved various mechanisms to counteract oxidative damage, including enzymatic and non-enzymatic antioxidant defense systems. However, enzyme inhibition can result in oxidative damage to cells (Birben et al., 2012). These enzymatic antioxidants indicate whether organisms can or cannot cope with cellular damage caused by extreme heat stress episodes or other stressors (Fordyce et al., 2019). Integrating temperature-sensitive enzymes provides important process-based information concerning heat stress impacts on bivalves. Glutathione (GSH) is an essential antioxidant that inhibits ROS-induced mitochondrial dysfunction. The activity of antioxidant enzymes, including adenosine triphosphate (ATP) syntHäse (ACP), adenylate kinase (AKP), and glutathione (GSH), was found to increase significantly in the bivalve mollusk Pinctada maxima with an increase in seawater temperature (Xu et al., 2021). On the other hand, increased levels of malondialdehyde were observed with decreased ACP, AKP, and GSH activities (Zhang et al., 2020). In response to acute heat stress, such as exposure to temperatures of 28°C and 32°C in Pteria penguin, Pinctada fucata, and Perna viridis, the activity of plasma catalase (CAT), glutathione peroxidase (GSH-Px), and superoxide dismutase (SOD) was significantly enhanced (Wang et al, 2018b; Gu et al., 2020; Wei et al., 2021).
However, when Pinctada maxima were subjected to intense heat stress at 36°C, its CAT, GSH-Px, and SOD activities decreased significantly (He et al., 2022b; Zhang et al., 2022b). Rahman et al. (2020) suggests that temperatures exceeding the body’s thermal threshold can cause a total or partial shutdown of the antioxidant defense system. In exceptional cases, Perna viridis and Nacella concinna can exhibit enhanced antioxidant enzyme activities when exposed to temperatures higher than 36°C (Abele et al., 1998; Lam, 2009). In numerous studies, protein synthesis has been inhibited by high temperatures (Lindquist, 1986; Liu et al., 2013). Temperature exposure might cause ribosomal subregion destabilization, causing such inhibitory activity. (Liu et al., 2013). Various investigations have found that the action of several ribosomal protein genes is increased in Sinonovacula constricta, Crassostrea gigas, and Ruditapes philippinarum (Quinn et al., 2011; Lim et al., 2016; Nie et al., 2016). Heat-induced ribosomal protein mRNA transcript upregulation in bivalves may minimize the consequences of thermal stress, implying a unique endeavor to defend ribosome function, which could be an adaptive response to heat stress. Genes involved in various physiological processes were downregulated after heat stress was imposed on clams. Tumor necrosis factor receptor binding (TNF-BP) gene that plays a role in the regulation of the immune system tyrosinase (TYR) involved in energy metabolism, specifically glycolysis and gluconeogenesis, ketohexokinase (KHK)involved specifically in the formation of shell matrix proteins, carbonic anhydrase (CA) chitinase (CAS), both involved in biomineralization, specifically in the formation of shell matrix proteins, and phosphotransferase (PT) involved in energy metabolism were all considerably downregulated (He et al, 2023) (Table 3).
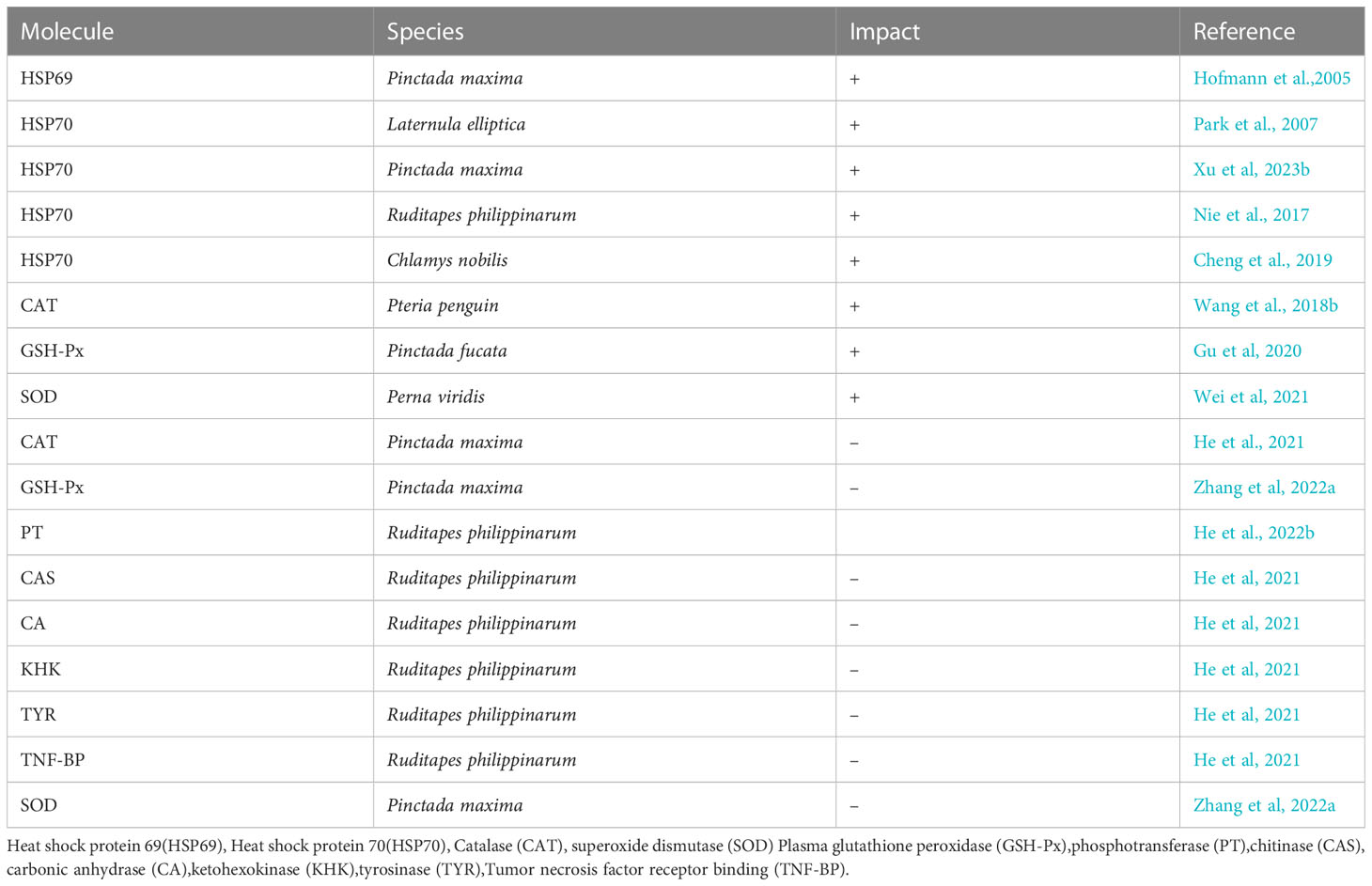
Table 3 Heat stress impacts bivalves’ molecular activity (+ representing upregulation and – representing downregulation).
4 Mechanisms of heat stress tolerance
4.1 Avoidance/burrowing behavior
Due to the increased risk of being dislodged or attacked by predators, bivalves may resort to surface behaviors during heat waves. For example, cockles that live in emerging sediments come to the surface when high tide arrives, while cockles that live in water pools gradually come to the surface during low tide during heat waves (Zhou et al., 2022). For benthic species, the only way to “escape” heat stress is to go to cooler areas by changing their burrowing habits or taking advantage of natural geomorphologic tidal-flat characteristics (Reise, 2012). Different bivalves engage in a wide variety of daring evasion strategies. Foot, shell, and siphons help bivalves burrow; they work sequentially, causing downward movement. Sea scallops, for instance, exhibit remarkable stress-relieving behavior. When threatened, scallops clamp their shell’s valves together to create forward momentum and escape the danger. The scallop will eventually fall to the water floor after being clapped multiple times. As a method of relieving tension, swimming is an obvious survival asset for scallops. Burst swimming, the sort of swimming used by scallops during escape behavior, is a stereotypical escape response (Guderley and Tremblay, 2013). The phasic muscle, a subset of the adductor muscle, is recruited for explosive swimming. Acidifying the intracellular environment due to the anaerobic synthesis of arginine phosphate and glycolytic ATP causes muscular contraction. The scallop recovers thanks to mitochondrial aerobic ATP synthesis (Guderley et al., 1995). Most bivalves can survive by burrowing either just below the surface or deep into the sediment. A cockle (Cerastoderma edule) is a type of suspension feeder that lives in a shallow burrow. Superior articulation teeth on the hinges, extensive shell sculpture (ribs), a broad foot, and small siphons are characteristic of bivalves with this lifestyle (Burdon et al, 2014). Razor shells (Solen and Ensis spp.) are extended for a rapid descent. When hot, they may burrow quicker. By surfacing during heat waves, bivalves risk dislodging and predators. Extreme temperature changes from floods and drying make acclimation problematic (Munari et al., 2011; Gomes and Bernardino, 2020).
4.2 Phenotypic flexibility
An individual’s ability to adapt through phenotypic flexibility permits them to sustain performance and fitness in unpredictable conditions. This is the quickest and most crucial reaction to change for long-lived organisms with slow generation periods and low genetic flexibility (Somero et al., 2012). In a rapidly changing environment, individuals showing consistent but reversible changes in behavior, physiology, and morphology may be more likely to be selected. Multiple studies show that adults can undergo substantial but reversible phenotypic changes, most notably in the size of their organ systems with their metabolic requirements (King et al., 2018; Wanninger and Wollesen, 2019). Reduced reproductive potential phenotypes are more susceptible to reversible phenotypic change than other species. Phenotypic plasticity can be obtained through variations in phenotype, and these variations are diagnostic of species with similar phenotypes but less plasticity. An organism’s energy can be used for various purposes, such as maintaining the body, growth, and reproduction. However, how much of that energy goes towards each of these purposes can differ from organism to organism and even within the same organism at different stages of its life (Stearns et al., 1992). Researchers looked at the morphological differences between wedge clam Donax serra populations living along two different temperature coasts. In the cooler north, where recruits are denser, clams weighed in at 8 grams, were more spherical, and had a higher body density than their warmer southern counterparts, who weighed in at 2.4-2.9 grams (Soares et al., 1998).
In another study, Thomas et al. () focused on three benthic species: the Pacific oyster Magallana gigas and two Mytilidae species, Mytilus edulis, and Mytilus galloprovincialis. These species exhibit contrasting biogeographic patterns, with M. edulis being a species from cold temperate areas distributed in the North-East Atlantic from northern Europe to the northeastern Spanish coast, while M. galloprovincialis is a Mediterranean species found as far north as the Scottish islands. M. gigas was introduced to French coasts in the late s (Troost, 2010; Thomas et al, 2016 ) The three species also display distinct physiological thermal tolerances. M. edulis and M. galloprovincialis have similar low-temperature thresholds (TL) of 4.4°C and 4.9°C, respectively, but exhibit different high-temperature thresholds (TH) of 22°C and 27°C, respectively. In contrast, M. gigas has a high-temperature threshold of 32°C, with a large tolerance range of 29°C. These findings highlight the variability in thermal tolerance among the studied species and may have important implications for their distribution and persistence in the face of climate change.
In another recent study conducted by Bahkmet et al.,, the physiological characteristics of two different species of mussels in the White Sea were compared. Specifically, the invasive blue mussel species Mytilus trossulus and the native Mytilus edulis were examined. Over the course of two years, Bakhmet et al, 2022 conducted in situ monitoring of heart activity for both species and their hybrids. They also conducted a laboratory experiment to test the thermal tolerance of these species. Interestingly, the results showed that the heart rate and variability of the invasive species and hybrids were greater than those of the native species. However, in the laboratory experiment, the native M. edulis exhibited a higher thermal tolerance of 10-17°C compared to M. trossulus. These findings suggest that M. edulis has a greater physiological potential than M. trossulus when it comes to adapting to increased temperatures. This is similar study to the study by Li et al. (2017) study, which explored the underlying mechanisms of adaptive divergence in two oyster subspecies and assesses their evolutionary potential of phenotypic plasticity. As a result, in a warming climate, the native species may have a competitive advantage over the invasive species due to their higher level of phenotypic plasticity.
4.3 Molecular mechanisms
4.3.1 Oxidative stress and antioxidants
The high temperatures experienced by bivalve mollusks trigger oxidative stress, generating reactive oxygen species (ROS) (Wang et al, 2018b). Recent studies have revealed that bivalves are protected from the adverse effects of heat stress through an increase in antioxidant activity (Wang et al, 2018b; He et al., 2022a). In response to heat, the elevation of superoxide dismutase (SOD) expression in manila clams highlights that the activity of antioxidant enzymes is temperature-sensitive and that their activation occurs at varying temperatures (He et al., 2022b). Anestis et al. (2007) found that superoxide dismutase (SOD), catalase (CAT), and adenosine triphosphate reductase (GR) activities all increased at 20°C after two weeks of acclimation to 18°C, demonstrating that bivalves employ this as a mitigation measure against heat stress. Manduzio et al. (2005) observed a reduction in ROS production in Mytilus edulis and Mytilus galloprovincialis, where ascorbic acid (AsA) and glutathione (GSH) synthesis were enhanced. Yin et al. (2017) reported that after being subjected to 30°C of heat, ROS production increased by 5.8, and Ruditapes philippinarum was observed to increase activities of ascorbate peroxidase (APX), CAT, and glutathione S-transferase (GST), all of which contributed to heat stress tolerance. Cells enhance the activity of CAT, APX, SOD, GR, and peroxidase (POX) to mitigate heat stress in bivalves (Buttemer et al., 2010).
4.3.2 Heat shock proteins
Bivalves respond to heat stress by producing heat shock proteins (HSPs), which play a crucial role in mitigating the impact of heat stress on their efficiency, as shown in Figure 3. Heat stress can result from sudden or gradual temperature increases, as noted by Xu et al. (2021). HSPs are upregulated in heat-stressed bivalves, plants, humans, and microbes, as highlighted in Fabbri et al. (2008). Interestingly, bivalves produce HSPs not only in the adult stage but also juvenile when exposed to intense heat, as Jong et al. (2008) observed. Furthermore, the expression of HSP70 was found to be upregulated in Pinctada maxima at high ambient temperatures, contributing to the bivalve’s heat tolerance, according to Xu et al. (2023b). In Pinctada maxima, HSP-70 and HSP-90 expression in the gill tissue rises to mitigate the impact of heat stress, as noted by Xu et al. (2021) and Masanja et al. (2022). Moreover, HSPs are present in higher quantities in heat-resistant lines, and they play a crucial role in reducing heat stress beyond just preventing protein denaturation, as pointed out by Fabbri et al. (2008) and Masanja et al. (2022). The production of HSPs is not limited to any particular stage of bivalve development, and their expression can be upregulated in response to heat stress.
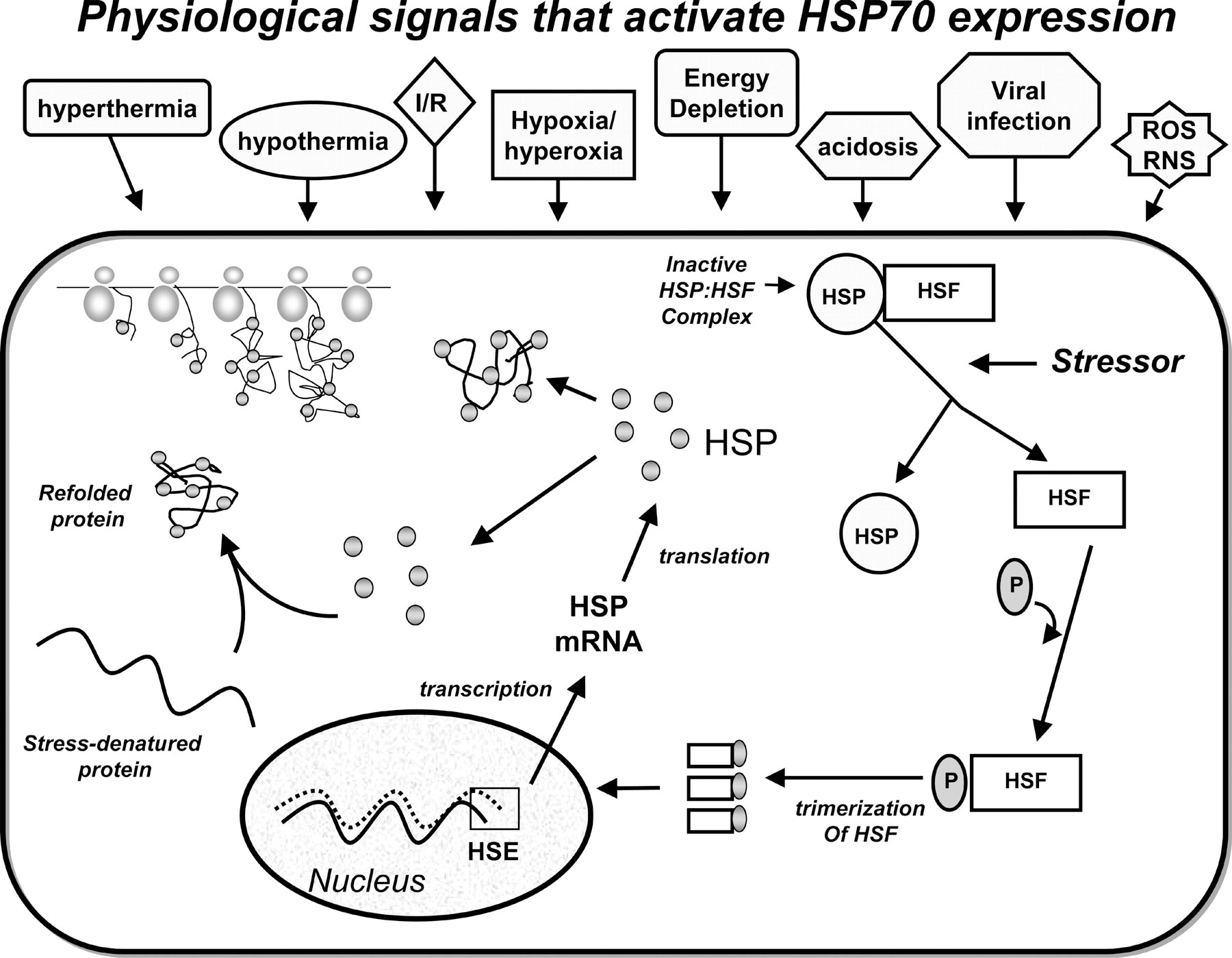
Figure 3 Physiological stimuli that induce HSPs. Heat shock proteins defend cells by assembling, transporting, and repairing newly produced and stress-damaged proteins. ischemia-reperfusion (Kregel et al.,2002).
5 Mitigation measures to consider for future heat stress tolerance
5.1 Conventional breeding strategies
Conventional breeding practices, including selection and hybridization, have been found to reduce genetic diversity in domesticated and selected traits (Hollenbeck and Johnston, 2018). This highlights the importance of separating wild and cultured bivalves’ genetic variability to enhance their performance under stress. A crucial step in achieving this is to better understand genetic variation in different species. For instance, recent studies have provided outstandingly detailed descriptions of genetic variation in coral species, such as Goniocorella Dumosa, Madrepora oculate, and Solenosmilia variabilis, and their response to temperature variations (Zeng et al., 2020).
5.1.1 Selection
Selection in genetics refers to the process by which certain traits become more or less common in a population over time due to the effects of natural selection (Schluter and Rieseberg, 2022). Natural selection is a mechanism of evolution that occurs when individuals with heritable traits that help them survive and reproduce better than others in their environment pass those traits on to their offspring (Schluter and Rieseberg, 2022). One common breeding method is mass selection, in which individuals are selected from a population based on their performance on a single trait (Zhong et al., 2016). For example, individuals with the highest growth rates may be selected to produce the next generation of oysters (Zhong et al., 2016). Mass selection can be effective in improving a single trait, but it can lead to inbreeding and a loss of genetic diversity (Zhang et al., 2019). Another common breeding method is family selection, in which individuals are selected from families based on their relatives’ performance. For example, oysters from families with high growth rates may be selected to produce the next generation of oysters (Gjedrem and Rye, 2016). Family selection can be more effective than mass selection in improving multiple traits, but it is more time-consuming and expensive (O’Connor, 2019).
Several challenges are associated with selection breeding in bivalves (Gjedrem and Rye, 2016). Bivalves have a long generation time, which means that it takes several years to produce a new generation of offspring (Moss et al., 2016). This makes it difficult to select desirable traits and evaluate the success of breeding programs. Bivalve populations are often heterogeneous, meaning that individuals within a population vary widely in their genetic makeup (Virgin et al, 2019). This makes it difficult to identify and select desirable genes. Bivalves are highly sensitive to environmental factors, such as water temperature, salinity, and food availability (Muhammad et al., 2020). This means that the expression of desirable traits can be affected by environmental conditions, making it difficult to predict the performance of offspring. Genetic drift is the random change in the frequency of genes in a population (Jorde et al., 1999). This can occur due to small population sizes or the founder effect, when a new population is established from a small number of individuals. Genetic drift can lead to the loss of desirable genes from a population, making it difficult to improve the performance of bivalves through breeding (Hedgecock and Pan, 2021). Despite these challenges, selection breeding can be an effective tool for improving the performance of bivalves. By carefully selecting desirable traits and managing environmental factors, it is possible to produce bivalves that are more productive, more resistant to disease, and better able to tolerate environmental stressors.
5.1.2 Genetic variation
Genetic variation is a key factor for the adaptation and survival of bivalves in changing environments. Genetic variation can play a role in improving heat stress regulation in bivalves. By having a diverse gene pool, populations of bivalves may have a greater chance of possessing genetic traits that confer resistance to heat stress. For example, Pinctada margaritifera inhabit contrasting thermal regimes (Le Luyer et al, 2022). It was revealed that genetic variation across P.margaritifera populations overlaps with gene expression and involves the mitochondrial respiration machinery, a central physiological process that contributes to species’ thermal sensitivity and their distribution ranges (Le Luyer et al, 2022). Furthermore, it was discovered that fatty acid remodeling, a metabolic pathway that modulates membrane fluidity and cellular functions, was influenced by genetic and environmental factors in this species (Le Luyer et al, 2022).
Furthermore, in 2017, Mathiesen et al. (2017) conducted a study to explore the impact of thermal stress on genetic variation, which included two bivalve species, Mytilus edulis, and Mytilus trossulus. The findings revealed that temperature variation had an effect on the expression of genetic variation in only one-third of the cases, primarily in a single clonal species. Moreover, the effects were species-specific, with genetic variation increasing or decreasing under stress depending on the trait. Notably, the M. edulis populations’ genetic structure displayed a marked separation between the samples from each side of the Atlantic, with Icelandic M. edulis appearing as a mixture of the two gene pools. This divergence of West and East Atlantic populations clarifies how M. edulis managed to survive in a West Atlantic glacial refugium. A study by Truebano et al. (2010) used a cDNA microarray to study the transcriptional response of the Antarctic bivalve Laternula elliptica to heat stress. They found that genes involved in the cellular component movement, microtubule-based processes, intracellular signal transduction, and catabolic and metabolic processes were differentially expressed under heat stress conditions. This suggests that these genes may play a role in enhancing the thermal tolerance of this cold-adapted species. Similarly, microRNAs, which are small noncoding RNAs that regulate gene expression at the post-transcriptional level, have been shown to modulate the stress response in various bivalve species. By manipulating these heat-tolerant genes and microRNAs, it may be possible to improve the survival and performance of bivalves under warming seawater temperatures. However, tolerance levels among species are difficult to quantify, as field experiments cannot be repeated with the same exactness due to the variable and unpredictable nature of their surroundings (Deng et al., 2005; Charlesworth and Willis, 2009; Camara and Symonds, 2014).
5.1.3 Hybridization
Hybridization is another conventional breeding practice that can produce new varieties of animals and plants with improved traits and adaptation to environmental stress (Chan et al., 2019). One of the strategies to enhance the heat tolerance of bivalves is to use intraspecific hybridization, which is the crossbreeding of individuals from different populations or lines within the same species. Intraspecific hybridization can create heterosis or hybrid vigor, which is the phenomenon of the superior performance of hybrids compared to their parents. For example, a study by Zheng et al. (2022) showed that intraspecific hybridization of noble scallop Chlamys nobilis, an economically and ecologically important bivalve species, resulted in higher hatching rate, survival rate, growth rate, and carotenoid content than inbred scallops under both ambient and acidified seawater conditions. Carotenoids are pigments that can protect bivalves from oxidative stress caused by high temperatures (Borodina, 2018). It is suggested that hybridization enhanced the expression of genes related to signal transduction, DNA replication, and post-translational modification, which may contribute to the heterosis observed in hybrid scallops (Zheng et al., 2022). Another example of intraspecific hybridization in bivalves is the crossbreeding of different strains of Pacific oyster Crassostrea gigas; Meng et al. (2021) reported that hybrid oysters exhibited higher growth rates, survival rates, shell strength, and disease resistance than purebred oysters under various environmental conditions, including high temperatures. Hybridization also increased oysters’ genetic diversity and heterozygosity, which may enhance their adaptive potential to changing environments (Meng et al., 2021). Hybridization is a promising technique to improve the heat tolerance of bivalve species, as it can generate hybrids with superior traits and performance than their parents. However, hybridization also poses some challenges and risks, such as the loss of local adaptation, genetic introgression, and outbreeding depression. Therefore, careful selection of parental stocks, monitoring of hybrid performance, and evaluation of ecological impacts are necessary to ensure the success and sustainability of hybridization programs in bivalve breeding.
Conventional breeding practices in bivalves aim to enhance genetic diversity, improve the growth rate, disease resistance, and other desirable traits in cultured populations. Significant progress has been made in bivalve breeding programs, including the development of broodstock management, genetic markers, and selective breeding methods (Nascimento‐Schulze et al., 2021). However, conventional breeding practices in bivalves are facing several challenges, including limited genetic variation, difficulties in accurate phenotyping, and the long generation times of bivalve species. Several strategies have been proposed to overcome these challenges to enhance the effectiveness of conventional breeding practices in bivalve species. These strategies include incorporating genomic selection and high-throughput phenotyping methods, establishing breeding programs that integrate hatchery and field-based methods, and using transgenic technologies to enhance desirable traits in bivalve species (Robledo et al., 2017).
Tolerance to heat stress also varies greatly between developmental stages due to the unique demands of each stage. Therefore, bivalves need to be tested for heat tolerance at every developmental stage, and different stages of development need to be analyzed for genetic tolerance variance. Breeding for physiological traits is essential for creating heat-tolerant cultivars that do not compromise yield, and for adaptation to heat stress, breeding must prioritize canopy structure. In conclusion, understanding the genetic variation in heat tolerance among bivalve species is crucial for developing effective breeding strategies for creating heat-tolerant cultivars.
5.2 Omics approaches in developing heat stress tolerance
Bivalves exhibit complex responses to heat stress, which are only beginning to be understood through omics techniques. These methods have enabled us to identify various heat-stress genes through gene expression analysis and genetic screening in different bivalve species. Among the regulators of post-transcriptional gene expression, microRNAs have been found to play a crucial role in bivalve heat stress resistance (Chinnusamy et al., 2007; Abo-Al-Ela and Faggio, 2021). Research has explored microRNAs’ impact on bivalves’ heat stress tolerance. For instance, the expression of cgi-miR-1984 was significantly upregulated, while scaffold631_909 was downregulated in heat-stressed oysters, suggesting their role as key mediators in maintaining physiological function during heat stress (Zhou et al.,2014). Moreover, studies have shown that stress-downregulated microRNAs contribute to heat tolerance by reducing the accumulation of targeted RNAs, whereas stress-upregulated microRNAs fail to target specified RNAs and do not contribute to tolerance (Bhaskaran and Mohan, 2014; Rosani et al., 2016; Abo-Al-Ela and Faggio, 2021; Rosani et al., 2021). In oyster hemocytes, this mechanism was observed when exposure to Vibrio splendidus led to the upregulation of cgi-miR-1984 and downregulation of scaffold631_909. The presence of unique miRNAs, such as miR-1984, in mollusk hemocytes, highlights their essential role in maintaining physiological function under heat stress conditions (Zhou et al., 2014; Zhao et al., 2016). The role of microRNAs in promoting cell tolerance, maintaining transcriptome homeostasis, and influencing developmental plasticity is increasingly being recognized. Additionally, microRNAs have been identified as potential tools for genetic engineering, which could aid in the creation of heat-stress-tolerant cells, as illustrated in Figure 4. Recent advances in technology, such as microarray and transcriptome analysis, have enabled researchers to investigate gene expression patterns in response to heat stress. Notably, Abo-Al-Ela et al. (2021) discovered that heat shock proteins (HSPs) are expressed in conditions beyond heat stress, demonstrating the complexity of gene regulation under stress.
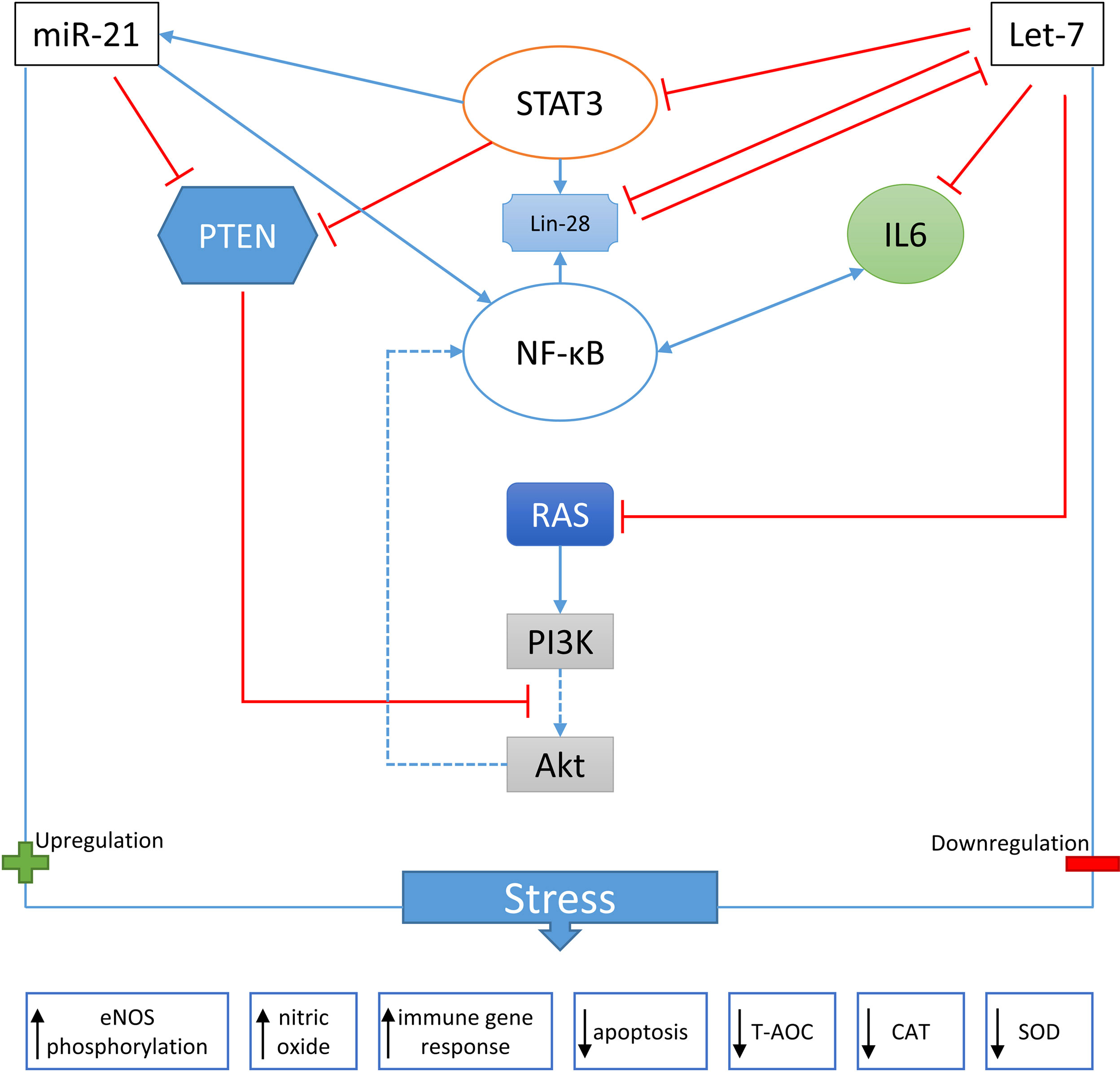
Figure 4 Bivalve mollusks stress-regulate miR-21 and let-7. This information was gathered from mammalian investigations. CAT: catalase; IL6: interleukin 6; NF-B: nuclear factor kappa light-chain enhancer of activated B cells; PI3K: phosphatidylinositol 3′-kinase; PTEN: phosphatase and TENsin homolog or phosphatidylinositol-3,4,5-triphosphate. (H.G. Abo-Al-Ela and C. Faggio,2021).
Transcriptome analysis is a technique that measures the expression levels of all the genes in a cell or tissue under different conditions (Lowe et al, 2017). By comparing the transcriptomes of heat-tolerant and heat-sensitive bivalves or of bivalves exposed to different temperatures, researchers can discover the genes and pathways that are responsive to heat stress and confer thermotolerance. For example, one study compared the transcriptomes of two oyster species, Crassostrea gigas, and Crassostrea angulata, which differ in their thermal tolerance (Ghaffari et al, 2019). The study found that C. angulata had higher expression levels of genes related to heat shock proteins (HSPs), antioxidant enzymes, immune response, apoptosis, and calcium signaling than C. gigas under heat stress. These genes may help C. angulata cope with heat-induced oxidative damage, inflammation, cell death, and calcium imbalance (Ghaffari et al, 2019). Transcriptome analysis can also reveal the transcription factors (TFs) that regulate the expression of heat stress-responsive genes in bivalves (Zhou et al, 2019) TFs are proteins that bind to specific DNA sequences and control gene transcription (Inukai et al., 2017). Transcriptome analysis is a powerful tool to develop heat stress tolerance in bivalves by uncovering the molecular mechanisms of thermotolerance and providing potential targets for genetic improvement or biotechnological applications.
6 Knowledge gaps
Elevated temperatures can accelerate various biological processes, including metabolism, development, filtration rate, and absorption efficiency, and some bivalves can adapt over long periods, albeit at a cost (Xu et al., 2021). Conversely, rapid and acute temperature increases can cause severe stress and result in sublethal and fatal effects (Gosling, 2015; Macho et al., 2016; He et al., 2021; Xu et al., 2021). This high mortality has been observed worldwide in inter-tidal bivalve fishing beds (He et al., 2021; Xu et al., 2021). Studying heat stress effects on marine bivalves can help fill the information gaps at various cellular levels. Hence, it is crucial to understand the extent to which marine bivalves can adapt to heat stress and the costs involved. In the coming years, the following points should be taken into consideration:
I. Evaluating the effects of heat stress in marine bivalves is crucial to understanding the impacts of environmental changes on this species. To understand heat stress comprehensively, measuring the stage-specific thermal limit and identifying the most vulnerable times for heat stress in these organisms is essential. This involves collecting data at various life history stages and under different stressors, as the life cycles of marine bivalves are complex, including planktonic larval periods and benthic juvenile and adult stages (Lacroix et al., 2015; Boutet et al., 2022).
II. Exposure to severe heat stress can cause larval dysfunction, potentially leading to crossover effects on juvenile development and adult strength and conditioning (Lacroix et al., 2015; Zhao et al., 2020; Boutet et al., 2022). Determining the persistence and severity of these carryover effects over time is crucial for understanding the resilience of marine bivalves to increasing heat stress incidents.
III. Marine bivalves may display resilience to environmental stressors through trans-generational plasticity, where offspring display phenotypic changes in response to the stress experienced by their parents (Moore et al., 2015; Zhao et al., 2020). Further research is necessary to explore the potential of marine bivalves to adapt to heat stress through this mechanism. Additionally, more research is needed to gain a deeper understanding of the physiology of these organisms, including their ability to adapt to cold extremes (Masanja et al, 2023)microplastics (Mkuye et al., 2022), the allocation of energy under different nitrite and ammonia concentrations (Nkuba et al., 2021), and how their behavior and growth rate may be impacted by increased frequency and intensity of heat stress.
7 Summary and outlook
The impact of heat stress on the aquaculture industry is becoming a growing concern worldwide. Bivalves’ development, appearance(shell), and function can be altered due to exposure to high temperatures, resulting in developmental and growth difficulties. Additionally, heat stress can induce oxidative damage in these species. Bivalves have evolved physiological, biochemical, and structural adaptations to cope with elevated temperatures. However, data from fragmented gene pools may not accurately reflect the bivalves’ tolerance to heat stress. Hence, evaluating heat-tolerant wild relatives, accessions, and genetic resources is of utmost importance. Contemporary methods such as genetic engineering, molecular markers, genomics, and quantitative trait loci (QTLs) can aid in a deeper understanding of the complex features of bivalves and their ability to withstand heat stress.
Author contributions
We hereby declare the credit author statement as follows: FM: Writing – original draft, Data curation, Investigation, Validation. KY: Data curation, Writing – review and editing, Validation. YX: Writing – review and editing, Validation, Data curation GH: Data curation, Writing – review and editing, Validation. XL: Writing – review and editing, Validation, Data curation XX: Data curation, Writing – review and editing, Validation. JX: Writing – review and editing, Validation, Data curation LX: Writing – review and editing, Validation. RM: Writing – review and editing. YD: Conceptualization, Resources. LZ: Conceptualization, Writing-original draft preparation, Project administration Kind regards, LZ on behalf of all other co-authors. All authors contributed to the article and approved the submitted version.
Funding
The present study has been made possible by research grants from the National Science Foundation of China (42076121, M-0163, 42211530423), the Guangdong Zhujiang Talents Program (2021QN02H665), the Department of Education of Guangdong Province (2020KTSCX050 and 2022ZDZX4012), the earmarked fund for Modern Agro-industry Technology Research System (CARS-49), and the program for Scientific Research Start-up Funds of Guangdong Ocean University.
Conflict of interest
The authors declare that the research was conducted in the absence of any commercial or financial relationships that could be construed as a potential conflict of interest.
Publisher’s note
All claims expressed in this article are solely those of the authors and do not necessarily represent those of their affiliated organizations, or those of the publisher, the editors and the reviewers. Any product that may be evaluated in this article, or claim that may be made by its manufacturer, is not guaranteed or endorsed by the publisher.
References
Aagesen A. M., Häse C. C. (2014). Seasonal effects of heat shock on bacterial populations, including artificial vibrio parahaemolyticus exposure, in the pacific oyster, crassostrea gigas. Food Microbiol. 38, 93–103. doi: 10.1016/j.fm.2013.08.008
Abele D., Burlando B., Viarengo A., Pörtner H. O. (1998). Exposure to elevated temperatures and hydrogen peroxide elicits oxidative stress and antioxidant response in the Antarctic intertidal limpet Nacella concinna. Comp. Biochem. Physiol. Part B: Biochem. Mol. Biol. 120 (2), 425–435. doi: 10.1016/S0305-0491(98)10028-7
Abele D., Puntarulo S. (2004). Formation of reactive species and induction of antioxidant defence systems in polar and temperate marine invertebrates and fish. Comp. Biochem. Physiol. Part A: Mol. Integr. Physiol. 138 (4), 405–415. doi: 10.1016/j.cbpb.2004.05.013
Abo-Al-Ela H. G., Faggio C. (2021). MicroRNA-mediated stress response in bivalve species. Ecotoxicology Environ. Saf. 208, 111442. doi: 10.1016/j.ecoenv.2020.111442
Acquafredda M. P., Munroe D. M., Ragone Calvo L. M., De Luca M. (2019). The effect of rearing temperature on the survival and growth of early juvenile Atlantic surfclams (Spisula solidissima). Aquaculture Rep. 13, 100176. doi: 10.1016/j.aqrep.2018.100176
Aldridge D. C. (1999). The morphology, growth, and reproduction of Unionidae (Bivalvia) in a fenland waterway. J. molluskan Stud. 65 (1), 47–60. doi: 10.1093/mollus/65.1.47
Alfonso S., Gesto M., Sadoul B. (2021). Temperature increase and its effects on fish stress physiology in the context of global warming. J. fish Biol. 98 (6), 1496–1508. doi: 10.1111/jfb.14599
Allam B., Pales Espinosa E. (2016). Bivalve immunity and response to infections: are we looking at the right place? Fish Shellfish Immunol. 53, 4–12. doi: 10.1016/j.fsi.2016.03.037
Anestis A., Lazou A., Pörtner H. O., Michaelidis B. (2007). Behavioral, metabolic, and molecular stress responses of marine bivalve Mytilus galloprovincialis during long-term acclimation at increasing ambient temperature. Am. J. Physiology-Regulatory Integrative Comp. Physiol. 293, 911–921. doi: 10.1152/ajpregu.00124.2007
Anestis A., Pörtner H. O., Karagiannis D., Angelidis P., Staikou A., Michaelidis B. (2010). Response of Mytilus galloprovincialis (L.) to increasing seawater temperature and to marteliosis: metabolic and physiological parameters. Comp. Biochem. Physiol. Part A: Mol. Integr. Physiol. 156 (1), 57–66. doi: 10.1016/j.cbpa.2009.12.018
Archambault J. M., Gregory Cope W., Kwak T. J. (2013). Burrowing, byssus, and biomarkers: behavioral and physiological indicators of sublethal thermal stress in freshwater mussels (Unionidae). Mar. Freshw. Behav. Physiol. 46 (4), 229–250. doi: 10.1080/10236244.2013.805891
Arrieche D., Maeda-Martínez A. N., Acosta-Balbás V., Freites L., Acosta-Salmón H., Lodeiros-Seijo C. (2020). Optimum temperature for growth of an invasive green mussel perna viridis population from Venezuela, determined in an open-flow system. Aquaculture Rep. 16, 100284. doi: 10.1016/j.aqrep.2020.100284
Bakhmet I., Aristov D., Marchenko J., Nikolaev K. (2022). Handling the heat: changes in the heart rate of two congeneric blue mussel species and their hybrids in response to water temperature. J. Sea Res. 185, 102218. doi: 10.1016/j.seares.2022.102218
Barber B. J., Blake N. J. (2006). “Reproductive physiology,” in Developments in aquaculture and fisheries science, vol. Vol. 35. (Elsevier), 357–416.
Bayne B. L. (1993). “Feeding physiology of bivalves: time-dependence and compensation for changes in food availability,” in Bivalve filter feeders (Berlin, Heidelberg: Springer), 1–24.
Beggel S., Hinzmann M., Machado J., Geist J. (2017). Combined impact of acute exposure to ammonia and temperature stress on the freshwater mussel unio pictorum. Water 9, 3–5. doi: 10.3390/w9070455
Benestad R. E. (2005). A review of the solar cycle length estimates. Geophys. Res. Lett. 32 (15). doi: 10.1029/2005gl023621
Bennett J. M., Sunday J., Calosi P., Villalobos F., Martínez B., Molina-Venegas R., et al. (2021). The evolution of critical thermal limits of life on earth. Nat. Commun. 12 (1), 1198. doi: 10.1038/s41467-021-21263-8
Bertolini C., Pastres R. (2021). Tolerance landscapes can be used to predict species-specific responses to climate change beyond the marine heatwave concept: using tolerance landscape models for an ecologically meaningful classification of extreme climate events. Estuarine Coast. Shelf Sci. 252, 107284. doi: 10.1016/j.ecss.2021.107284
Bhaskaran M., Mohan M. (2014). MicroRNAs: history, biogenesis, and their evolving role in animal development and disease. Veterinary Pathol. 51 (4), 759–774. doi: 10.1177/0300985813502820
Birben E., Sahiner U. M., Sackesen C., Erzurum S., Kalayci O. (2012). Oxidative stress and antioxidant defense. World Allergy Organ. J. 5 (1), 9–19. doi: 10.1097/WOX.0b013e3182439613
Blevins J. E., Moralejo D. H., Wolden-Hanson T. H., Thatcher B. S., Ho J. M., Kaiyala K. J., et al. (2012). Integrative and translational physiology: integrative aspects of energy homeostasis and metabolic diseases: alterations in activity and energy expenditure contribute to lean phenotype in Fischer 344 rats lacking the cholecystokinin-1 receptor gene. Am. J. Physiology-Regulatory Integrative Comp. Physiol. 303 (12), R1231. doi: 10.1152/ajpregu.00393.2012
Boroda A. V., Kipryushina Y. O., Odintsova N. A. (2020). The effects of cold stress on mytilus species in the natural environment. Cell Stress chaperones 25 (6), 821–832. doi: 10.1007/s12192-020-01109-w
Borodina A. V. (2018). Carotenoids in the gonads of the bivalved mollusk Anadara kagoshimensis (Tokunag). J. Evolutionary Biochem. Physiol. 54 (4), 267–272. doi: 10.1134/s0022093018040026
Boutet I., Lacroix C., Devin S., Tanguy A., Moraga D., Auffret M. (2022). Does the environmental history of mussels have an effect on the physiological response to additional stress under experimental condition? Sci. Total Environ. 806, 149925. doi: 10.1016/j.scitotenv.2021.149925
Burdon D., Callaway R., Elliott M., Smith T., Wither A. (2014). Mass mortalities in bivalve populations: a review of the edible cockle cerastoderma edule (L.). Estuarine Coast. Shelf Sci. 150, 271–280. doi: 10.1016/j.ecss.2014.04.011
Buttemer W. A., Abele D., Costantini D. (2010). From bivalves to birds: oxidative stress and longevity. Funct. Ecol. 24, 971–983. doi: 10.1111/j.1365-2435.2010.01740.x
Camara M. D., Symonds J. E. (2014). Genetic improvement of new Zealand aquaculture species: programs, progress, and prospects. New Z. J. Mar. Freshw. Res. 48 (3), 466–491. doi: 10.1080/00288330.2014.932291
Carneiro A. P., Soares C. H. L., Manso P. R. J., Pagliosa P. R. (2020). Impact of marine heat waves and cold spell events on the bivalve Anomalocardia flexuosa: a seasonal comparison. Mar. Environ. Res. 156, 104898. doi: 10.1016/j.marenvres.2020.104898
Carneiro A. P., Soares C. H. L., Pagliosa P. R. (2021). Does the environmental condition affect the tolerance of the bivalve Anomalocardia flexuosa to different intensities and durations of marine heatwaves? Mar. pollut. Bull. 168, 112410. doi: 10.1016/j.marpolbul.2021.112410
Castillo M. G., Salazar K. A., Joffe N. R. (2015). The immune response of cephalopods from head to foot. Fish Shellfish Immunol. 46 (1), 145–160. doi: 10.1016/j.fsi.2015.05.029
Chan W. Y., Hoffmann A. A., Oppen M. J. H. (2019). Hybridization as a conservation management tool. Conserv. Lett. 12 (5). doi: 10.1111/conl.12652
Chapple J. P., Smerdon G. R., Berry R. J., Hawkins A. J. (1998). Seasonal changes in stress-70 protein levels reflect thermal tolerance in the marine bivalve Mytilus edulis l. J. Exp. Mar. Biol. Ecol. 229 (1), 53–68. doi: 10.1016/S0022-0981(98)00040-9
Charlesworth D., Willis J. H. (2009). The genetics of inbreeding depression. Nat. Rev. Genet. 10 (11), 783–796. doi: 10.1038/nrg2664
Cheng J., Li H., Huang Z., Zhang F., Bao L., Li Y., et al. (2019). Expression analysis of the heat shock protein genes and cellular reaction in dojo loach (Misgurnus anguillicaudatus) under the different pathogenic invasion. Fish Shellfish Immunol. 95, 506–513. doi: 10.1016/j.fsi.2019.10.073
Chovatiya R., Medzhitov R. (2014). Stress, inflammation, and defense of homeostasis. Mol. Cell 54 (2), 281–288. doi: 10.1016/j.molcel.2014.03.030
Chu F., La Peyre J. (1993). Perkinsus marinus susceptibility and defense-related activities in Eastern oysters Crassostrea virginica temperature effects. Dis. Aquat Organ 16, 223–234. doi: 10.3354/dao016223
Coumou D., Robinson A. (2013). Historic and future increase in the global land area affected by monthly heat extremes. Environ. Res. Lett. 8 (3), 34018. doi: 10.1088/1748-9326/8/3/034018
Coumou D., Robinson A., Rahmstorf S. (2013). Global increase in record-breaking monthly-mean temperatures. Climatic Change 118 (3–4), 771–782. doi: 10.1007/s10584-012-0668-1
Cummings K. S., Graf D. L. (2010). “Mollusca: Bivalvia,” in Ecology and classification of north American freshwater invertebrates (Academic Press), 309–384.
Dame R. F. (2011). Ecology of marine bivalves: An ecosystem approach, second edition (2nd ed.) (CRC Press). doi: 10.1201/b11220
Data C. (2009). Guidelines on analysis of extremes in a changing climate in support of informed decisions for adaptation (World Meteorological Organization).
Daufresne M., Lengfellner K., Sommer U. (2009). Global warming benefits the small in aquatic ecosystems. PNAS 106 (31), 12788–12793. doi: 10.1073/pnas.0902080106
de Almeida E. A., Bainy A. C. D., de Melo Loureiro A. P., Martinez G. R., Miyamoto S., Onuki J., et al. (2007). Oxidative stress in perna perna and other bivalves as indicators of environmental stress in the Brazilian marine environment: antioxidants, lipid peroxidation, and DNA damage. Comp. Biochem. Physiol. Part A: Mol. Integr. Physiol. 146 (4), 588–600. doi: 10.1016/j.cbpa.2006.02.040
Defeo O., Castrejón M., Ortega L., Kuhn A. M., Gutiérrez N. L., Castilla J. C. (2013). Impacts of climate variability on Latin American small-scale fisheries. Ecol. Soc. 18, 30. doi: 10.5751/ES-05971-180430
Deng Y., Liu X., Zhang G., Guo X. (2005). Inbreeding depression and maternal effects on early performance of pacific abalone. North Am. J. Aquaculture 67 (3), 231–236. doi: 10.1577/A04-021.1
Dulière V., Zhang Y., Salathé E. P. (2013). Changes in twentieth-century extreme temperature and precipitation over the western united states based on observations and regional climate model simulations*. J. Climate 26 (21), 8556–8575. doi: 10.1175/jcli-d-12-00818.1
Eversole A. G. (2001). Chapter 5 Reproduction in Mercenaria mercenaria. Biology of the Hard Clam, 221–260. doi: 10.1016/s0167-9309(01)80033-8
Fabbri E., Valbonesi P., Franzellitti S. (2008). HSP expression in bivalves. ISJ-Invertebrate Survival J. 5, 135–161.
Falfushynska H., Sokolov E. P., Haider F., Oppermann C., Kragl U., Ruth W., et al. (2019). Effects of a common pharmaceutical, atorvastatin, on energy metabolism and detoxification mechanisms of a marine bivalve Mytilus edulis. Aquat. toxicToxicology, 47–61. doi: 10.1016/j.aquatox.2018.12.022
Falfushynska H. I., Wu F., Ye F., Kasianchuk N., Dutta J., Dobretsov S., et al. (2019). The effects of ZnO nanostructures of different morphology on bioenergetics and stress response biomarkers of the blue mussels Mytilus edulis. Sci. Total Environ. 694, 133717. doi: 10.1016/j.scitotenv.2019.133717
Fernández-reiriz M. J., Pérez-Camacho A., Peteiro L. G., Labarta U. (2011). Growth and kinetics of lipids and fatty acids of the clam Venerupis pullastra during larval development and postlarvae. Aquaculture Nutr. 17 (1), 13–23. doi: 10.1111/j.1365-2095.2009.00701.x
Ferreira-Rodríguez N., Fernández I., Cancela M. L., Pardo I. (2018). Multibiomarker response shows how native and non-native freshwater bivalves differentially cope with heat-wave events. Aquat. Conserv: Mar. Freshw. Ecosyst. 28, 934–943. doi: 10.1002/aqc.2884
Fisher W. S. (1988). Environmental influence on bivalve hemocyte function. Am. Fish Soc. Spec Publ 18, 225–237.
Flye-Sainte-Marie J., Soudant P., Lambert C., Le Goïc N., Goncalvez M., Travers M.-A., et al. (2009). Variability of the hemocyte parameters of Ruditapes philippinarum in the field during an annual cycle. J. Exp. Mar. Bio Ecol. 377, 1–11. doi: 10.1016/j.jembe.2009.06.003
Fordyce A. J., Ainsworth T. D., Heron S. F., Leggat W. (2019). Marine heatwave hotspots in coral reef environments: physical drivers, ecophysiological outcomes, and impact upon structural complexity. Front. Mar. Sci. 6, 498. doi: 10.3389/fmars.2019.00498
Fraser K. P., Clarke A., Peck L. S. (2002). Low-temperature protein metabolism: seasonal changes in protein synthesis and RNA dynamics in the Antarctic limpet Nacella concinna strebel 1908. J. Exp. Biol. 205 (19), 3077–3086. doi: 10.1242/jeb.205.19.3077
Fritz L. W. (2001). “Shell structure and age determination,” in Developments in aquaculture and fisheries science, vol. Vol. 31. (Elsevier), 53–76.
Fritz L. W. (2001). Chapter 2 shell structure and age determination. Biol. Hard Clam 53–76. doi: 10.1016/s0167-9309(01)80030-2
Frölicher T. L., Fischer E. M., Gruber N. (2018). Marine heatwaves under global warming. Nature 560 (7718), 360–364. doi: 10.1038/s41586-018-0383-9
Gabbott P. A. (1976). Energy metabolism. In Bayne B. L. Ed. Marine mussels: Their ecology and physiology (Cambridge University Press), 293–355.
Galimany E., Ramón M., Ibarrola I. (2011). Feeding behavior of the mussel mytilus galloprovincialis (L.) in a mediterranean estuary: A field study. Aquaculture 314 (1–4), 236–243. doi: 10.1016/j.aquaculture.2011.01.035
Gestal C., Roch P., Renault T., Pallavicini A., Paillard C., Novoa B., et al. (2008). Study of diseases and the immune system of bivalves using molecular biology and genomics. Rev. Fisheries Sci. 16 (sup1), 133–156. doi: 10.1080/10641260802325518
Ghaffari H., Wang W., Li A., Zhang G., Li L. (2019). Thermotolerance divergence revealed by the physiological and molecular responses in two oyster subspecies of crassostrea gigas in China. Front. Physiol 10. doi: 10.3389/fphys.2019.01137
Gilson A. R., Coughlan N. E., Dick J. T., Kregting L. (2021). Marine heat heatwaveserentially affect functioning of native (Ostrea edulis) and invasive (Crassostrea [Magallana] gigas) oysters in tidal pools. Mar. Environ. Res. 172, 105497. doi: 10.1016/j.marenvres.2021.105497
Gjedrem T., Rye M. (2016). Selection response in fish and shellfish: a review. Rev. Aquaculture 10 (1), 168–179. doi: 10.1111/raq.12154
Glynn P. W., Enochs I. C. (2011). Invertebrates and their roles in coral reef ecosystems. Coral reefs: an ecosystem transition, 273–325. doi: 10.1007/978-94-007-0114-4_18
González P. M., Malanga G., Puntarulo S. (2015). Cellular oxidant/antioxidant network: update on the environmental effects over marine organisms. Open Mar. Biol. J. 9 (1), 1–13. doi: 10.2174/1874450801509010001
Gomes L. E. de O., Bernardino A. F. (2020). Drought effects on tropical estuarine benthic assemblages in eastern brazil. Sci. Total Environ. 703, 135490. doi: 10.1016/j.scitotenv.2019.135490
Goodchild C. G., Simpson A. M., Minghetti M., DuRant S. E. (2019). Bioenergetics-adverse outcome pathway: linking organismal and suborganismal energetic endpoints to adverse outcomes. Environ. Toxicol. Chem. 38 (1), 27–45. doi: 10.1002/etc.4280
Gu Z., Wei H., Cheng F., Wang A., Liu C. (2020). Effects of air exposure time and temperature on physiological energetics and oxidative stress of winged pearl oyster (Pteria penguin). Aquaculture Rep. 17, 100384. doi: 10.1016/j.aqrep.2020.100384
Guderley H. E., Rojas F. M., Nusetti O. A. (1995). Metabolic specialization of mitochondria from scallop phasic muscles. Mar. Biol. 122 (3), 409–416. doi: 10.1007/BF00350873
Guderley H. E., Tremblay I. (2013). Escape responses by jet propulsion in scallops. Can. J. zoology 91 (6), 420–430. doi: 10.1139/cjz-2013-0004
Hart A. M. (2014). 3.6 the effect of the 2011 marine heat wave on roe’s abalone (Haliotis roei) stocks in Western Australia by a. hart. the marine heat wave off Western Australia during the summer of 2010/11–2 years on. 12.
Hawkins A. J. S., Bayne B. L. (1985). Seasonal variation in the relative utilization of carbon and nitrogen by the mussel Mytilus edulis: budgets, conversion efficiencies, and maintenance requirements. Mar. Ecol. Prog. series. Oldendorf 25 (2), 181–188. doi: 10.3354/meps025181
He G., Liu X., Xu Y., Liang J., Deng Y., Zhang Y., et al. (2021). Repeated exposure to simulated marine heat wheat waves the thermal tolerance in pearl oysters. Aquat. Toxicol. 239, 105959. doi: 10.1016/j.aquatox.2021.105959
He G., Peng Y., Liu X., Liu Y., Liang J., Xu X., et al. (2022b). Post-responses of intertidal bivalves to recurrent heatwaves. Mar. pollut. Bull. 184, 114223. doi: 10.1016/j.marpolbul.2022.114223
He G., Xiong X., Peng Y., Yang C., Xu Y., Liu X., et al. (2023). Transcriptomic responses reveal impaired physiological performance of the pearl oyster following repeated exposure to marine heatwaves. Sci. Total Environ. 854, 158726. doi: 10.1016/j.scitotenv.2022.158726
He G., Zou J., Liu X., Liang F., Liang J., Yang K., et al. (2022a). Assessing the impact of atmospheric heatwaves on intertidal clams. Sci. Total Environ. 841, 156744. doi: 10.1016/j.scitotenv.2022.156744
Hedgecock D., Pan F. T. C. (2021). Genetic divergence of selected and wild populations of pacific oysters (Crassostrea gigas) on the West coast of north America. Aquaculture 530, 735737. doi: 10.1016/j.aquaculture.2020.735737
Hégaret H., Wikfors G. H., Soudant P. (2003). Flow cytometric analysis of hemocytes from eastern oysters, crassostrea virginica, subjected to a sudden temperature elevation: II. hemocyte functions: aggregation, viability, phagocytosis, and respiratory burst. J. Exp. Mar. Biol. Ecol. 293 (2), 249–265. doi: 10.1016/s0022-0981(03)00235-1
Hicks D. W., McMahon R. F. (2002). Respiratory responses to temperature and hypoxia in the nonindigenous brown mussel, Perna perna (Bivalvia: mytilidae), from the gulf of Mexico. J. Exp. Mar. Biol. Ecol. 277 (1), 61–78. doi: 10.1016/S0022-0981(02)00276-9
Hobday A. J., Alexander L. V., Perkins S. E., Smale D. A., Straub S. C., Oliver E. C. J., et al. (2016). A hierarchical approach to defining marine heatwaves. Prog. Oceanography 141, 227–238. doi: 10.1016/j.pocean.2015.12.014
Hochachka P. W. (1973). “Basic strategies and mechanisms of enzyme adaptation to temperature,” in Effects of temperature on ectothermic organisms (Berlin, Heidelberg: Springer), 69–81.
Hofmann G. E. (2005). Patterns of hsp gene expression in ectothermic marine organisms on small to large biogeographic scales. Integr. Comp. Biol. 45 (2), 247–255. doi: 10.1093/icb/45.2.247
Hollenbeck C. M., Johnston I. A. (2018). Genomic tools and selective breeding in molluscs. Front. Genet. 9. doi: 10.3389/gene.2018.00253
Hong H.-K., Kim C. W., Kim J.-H., Kajino N., Choi K.-S. (2021). Effect of extreme heatwaves on the mortality and cellular immune responses of purplish bifurcate mussel Mytilisepta virgata (Wiegman) (Septifer virgatus) in indoor mesocosm experiments. Front. Mar. Sci. 8. doi: 10.3389/fmars.2021.794168
Hornstein J., Pales Espinosa E., Cerrato R. M., Lwiza K. M. M., Allam B. (2018). The influence of temperature stress on the physiology of the Atlantic surf clam, Spisula solidissima. Comp. Biochem. Physiol. A 222, 66–73. doi: 10.1016/j.cbpa.2018.04.011
Inukai S., Kock K. H., Bulyk M. L. (2017). Transcription factor-DNA binding: beyond binding site motifs. Curr. Opin. Genet. Dev. 43, 110–119. doi: 10.1016/j.gde.2017.02.007
Johnston D. J., Yeoh D. E., Harris D. C. (2021). Environmental drivers of commercial blue swimmer crab (Portunus armatus) catch rates in Western Australian fisheries. Fisheries Res. 235, 105827. doi: 10.1016/j.fishres.2020.105827
Jong L. D., Moreau X., Thiéry A. (2008). Expression of heat shock proteins as biomarker tool in aquatic invertebrates: actual knowledge and ongoing developments for the early detection of environmental changes and ecological risks. Emma Morel and Camille Vincent. Heat-Shock Proteins: New Research, 20, Nova Publishers, pp.375–392.
Jorde P. E., Palm S., Ryman N. (1999). Estimating genetic drift and effective population size from temporal shifts in dominant gene marker frequencies. Mol. Ecol. 8 (7), 1171–1178. doi: 10.1046/j.1365-294x.1999.00676.x
Joyce A., Vogeler S. (2018). Molluscan bivalve settlement and metamorphosis: neuroendocrine inducers and morphogenetic responses. Aquaculture 487, 64–82. doi: 10.1016/j.aquaculture.2018.01.002
Jwa M. S., Hong C. Y. (2022). Investigating the link between microalgal nutrition and the environment in hen clam (Mactra chinensis) larvae growth and survival. Appl. Sci. 12 (3), 1367. doi: 10.3390/app12031367
Kim M., Ahn I. Y., Kim H., Cheon J., Park H. (2009). Molecular characterization and induction of heat shock protein 90 in the Antarctic bivalve Laternula elliptica. Cell Stress Chaperones 14 (4), 363–370. doi: 10.1007/s12192-008-0090-9
King N. G., McKeown N. J., Smale D. A., Moore P. J. (2018). The importance of phenotypic plasticity and local adaptation in driving intraspecific variability in thermal niches of marine macrophytes. Ecography 41, 1469–1484. doi: 10.1111/ecog.03186
Kittner C., Riisgård H. U. (2005). Effect of temperature on filtration rate in the mussel mytilus edulis: no evidence for temperature compensation. Mar. Ecol. Prog. Ser. 305, 147–152. doi: 10.3354/meps305147
Kooijman B., Kooijman S. A. L. M. (2010). Dynamic energy budget theory for metabolic organization (Cambridge university press).
Kordas R. L., Harley C. D., O’Connor M. I. (2011). Community ecology in a warming world: the influence of temperature on interspecific interactions in marine systems. J. Exp. Mar. Biol. Ecol. 400 (1–2), 218–226. doi: 10.1016/j.jembe.2011.02.029
Kregel K. C. (2002). Invited review: heat shock proteins: modifying factors in physiological stress responses and acquired thermotolerance. J. Appl. Physiol. 92 (5), 2177–2186. doi: 10.1152/japplphysiol.01267.2001
Kronschnabel A. (2020). Tenacity of the Antarctic limpet nacella concinna after long-term acclimation to warming.
Lacroix C., Richard G., Seguineau C., Guyomarch J., Moraga D., Auffret M. (2015). Active and passive biomonitoring suggest metabolic adaptation in blue mussels (Mytilus spp.) chronically exposed to a moderate contamination in Brest harbor (France). Aquat. Toxicol. 162, 126–137. doi: 10.1016/j.aquatox.2015.03.008
Lam P. K. (2009). Use of biomarkers in environmental monitoring. Ocean Coast. Manage. 52 (7), 348–354. doi: 10.1016/j.ocecoaman.2009.04.010
Lawlor J. A., Arellano S. M. (2020). Temperature and salinity, not acidification, predict near-future larval growth and larval habitat suitability of Olympia oysters in the salish Sea. Sci. Rep. 10 (1), 1–15. doi: 10.1038/s41598-020-69568-w
Lei Q. N., Wu Y. Y., Liang H. Y., Wang Z. X., Zheng Z., Deng Y. W. (2016). Molecular cloning and expression analysis of heat shock protein 20 (HSP20) from the pearl oyster Pinctada martensii. Genet. Mol. Res. 15 (2). doi: 10.4238/gmr.15028799
Le Luyer J., Monaco C. J., Milhade L., Reisser C., Soyez C., Raapoto H., et al. (2022). Gene expression plasticity, genetic variation and fatty acid remodelling in divergent populations of a tropical bivalve species. J. Anim. Ecol. 91 (6), 1196–1208. doi: 10.1111/1365-2656.13706
Lemasson A. J., Hall-Spencer J. M., Fletcher S., Provstgaard-Morys S., Knights A. M. (2018). Indications of future performance of native and non-native adult oysters under acidification and warming. Mar. Environ. Res. 142, 178–189. doi: 10.1016/j.marenvres.2018.10.003
Leverone J. R., Shumway S. E., Blake N. J. (2007). Comparative effects of the toxic dinoflagellate karenia brevis on clearance rates in juveniles of four bivalve molluscs from florida, USA. Toxicon 49 (5), 634–645. doi: 10.1016/j.toxicon.2006.11.003
Le Moullac G., Quéau I., Le Souchu P., Pouvreau S., Moal J., René Le Coz J., et al. (2007). Metabolic adjustments in the oyster Crassostrea gigas according to oxygen level and temperature. Mar. Biol. Res. 3 (5), 357–366. doi: 10.1080/17451000701635128
Leung J. Y., Connell S. D., Nagelkerken I., Russell B. D. (2017). Impacts of near-future ocean acidification and warming on the shell mechanical and geochemical properties of gastropods from intertidal to subtidal zones. Environ. Sci. Technol. 51 (21), 12097–12103. doi: 10.1021/acs.est.7b02359
Leung J. Y., Russell B. D., Connell S. D. (2019). Adaptive responses of marine gastropods to heatwaves. One Earth 1 (3), 374–381. doi: 10.1016/j.oneear.2019.10.025
Li A., Li L., Song K., Wang W., Zhang G. (2017). Temperature, energy metabolism, and adaptive divergence in two oyster subspecies. Ecol. Evol. 7 (16), 6151–6162. doi: 10.1002/ece3.3085
Li S., Liu Y., Liu C., Huang J., Zheng G., Xie L., et al. (2015). Morphology and classification of hemocytes in Pinctada fucata and their responses to ocean acidification and warming. Fish Shellfish Immunol. 45, 194–202. doi: 10.1016/j.fsi.2015.04.006
Li T., Shao M. A., Jia Y. (2017). Characteristics of soil evaporation and temperature under aggregate mulches created by burrowing ants (Camponotus japonicus). Soil Sci. Soc. America J. 81 (2), 259–267. doi: 10.2136/sssaj2016.08.0259
Lim H. J., Kim B. M., Hwang I. J., Lee J. S., Choi I. Y., Kim Y. J., et al. (2016). Thermal stress induces a distinct transcriptome profile in the pacific oyster Crassostrea gigas. comparative biochemistry and physiology part d. Genomics Proteomics 19, 62–70. doi: 10.1016/j.cbd.2016.06.006
Lin T., Zhou K., Lai Q., Yao Z., Li Z., Xing J. (2012). Seasonal variations of water temperature, food availability, size, and reproduction on the hemocyte parameters in the scallop. Chlamys farreri. J. Shellfish Res. 31, 663–670. doi: 10.2983/035.031.0309
Lindquist S. (1986). The heat-shock response. Annu. Rev. Biochem. 55 (1), 1151–1191. doi: 10.1146/annurev.bi.55.070186.005443
Liu X., Ji C., Zhao J., Wu H. (2013). Differential metabolic responses of clam Ruditapes philippinarum to Vibrio anguillarum and Vibrio splendidus challenges. Fish shellfish Immunol. 35 (6), 2001–2007. doi: 10.1016/j.fsi.2013.09.014
Liu Z., Li M., Yi Q., Wang L., Song L. (2018). The neuroendocrine-immune regulation in response to environmental stress in marine bivalves. Front. Physiol. 9, 1456. doi: 10.3389/fphys.2018.01456
Liu Z., Wang L., Zhou Z., Liu Y., Dong M., Wang W., et al. (2017a). Transcriptomic analysis of oyster Crassostrea gigas larvae illustrates the response patterns regulated by catecholaminergic system upon acute heat and bacterial stress. Dev. Comp. Immunol 73, 52–60. doi: 10.1016/j.dci.2017.03.005
Longshaw M., Malham S. K. (2013). A review of the infectious agents, parasites, pathogens and commensals of european cockles (Cerastoderma edule and c. glaucum) – ERRATUM. J. Mar. Biol. Assoc. United Kingdom 93 (4), 1141–1141. doi: 10.1017/s0025315413000167
Lowe R., Shirley N., Bleackley M., Dolan S., Shafee T. (2017). Transcriptomics technologies. PloS Comput. Biol. 13 (5), e1005457. doi: 10.1371/journal.pcbi.1005457
Macho G., Woodin S. A., Wethey D. S., Vázquez E. (2016). Impacts of sublethal and lethal high temperatures on clams exploited in European fisheries. J. Shellfish Res. 35 (2), 405–419. doi: 10.2983/035.035.0215
Mackenzie C. L., Lynch S. A., Culloty S. C., Malham S. K. (2014). Future oceanic warming and acidification alter immune response and disease status in a commercial shellfish species, Mytilus edulis l. PloS One 9 (6), e99712. doi: 10.1371/journal.pone.0099712
Malham S. K., Hutchinson T. H., Longshaw M. (2012). A review of the biology of European cockles (Cerastoderma spp.). J. Mar. Biol. Assoc. United Kingdom 92 (7), 1563–1577. doi: 10.1017/S0025315412000355
Manduzio H., Rocher B., Durand F., Galap C., Leboulenger F. (2005). The point about oxidative stress in molluscs. Invertebrate Survival J.
Manríquez P. H., Jara M. E., González C. P., Seguel M. E., Domenici P., Watson S. A., et al. (2021). The combined effects of climate change stressors and predatory cues on a mussel species. Sci. Total Environ. 776, 145916. doi: 10.1016/j.scitotenv.2021.145916
Masanja F., Xu Y., He G., Liang F., Liu X., Yang K., et al. (2022). Exploring HSP90 as a biomarker for marine heatwaves in Pinctada maxima. Front. Mar. Sci. 9, 913920. doi: 10.3389/fmars.2022.913920
Masanja F., Xu Y., Yang K., Mkuye R., Deng Y., Zhao L. (2023). Surviving the cold: a review of the effects of cold spells on bivalves and mitigation measures. Front. Mar. Sci. 10. doi: 10.3389/fmars.2023.1158649
Mathiesen S. S., Thyrring J., Hemmer-Hansen J., Berge J., Sukhotin A., Leopold P., et al. (2017). Genetic diversity and connectivity within mytilus spp. in the subarctic and Arctic. Evol. Appl. 10, 39–55. doi: 10.1111/eva.12415
Matozzo V., Marin M. G. (2011). Bivalve immune responses and climate changes: is there a relationship? Invertebrate survival J. 8 (1), 70–77.
McFarland K., Jean F., Thebault J., Volety A. K. (2016). Potential impacts of blooms of the toxic dinoflagellate Karenia brevis on the growth, survival and juvenile recruitment of the non-native green mussel Perna viridis in southeastern united states. Toxicon 109, 94–102. doi: 10.1016/j.toxicon.2015.11.017
Meng L., Li Q., Xu C., Liu S., Kong L., Yu H. (2021). Hybridization improved stress resistance in the pacific oyster: evidence from physiological and immune responses. Aquaculture 545, 737227. doi: 10.1016/j.aquaculture.2021.737227
Miller L. P., Dowd W. W. (2019). Repeatable patterns of small-scale spatial variation in intertidal mussel beds and their implications for responses to climate change. Comp. Biochem. Physiol. Part A: Mol. Integr. Physiol. 236, 110516. doi: 10.1016/j.cbpa.2019.06.016
Mkuye R., Gong S., Zhao L., Masanja F., Ndandala C., Bubelwa E., et al. (2022). Effects of microplastics on physiological performance of marine bivalves, potential impacts, and enlightening the future based on a comparative study. Sci. Total Environ. 838, 155933. doi: 10.1016/j.scitotenv.2022.155933
Monari M., Matozzo V., Foschi J., Cattani O., Serrazanetti G. P., Marin M. G. (2007). Effects of high temperatures on functional responses of haemocytes in the clam chamelea gallina. Fish Shellfish Immunol. 22, 98–114. doi: 10.1016/j.fsi.2006.03.016
Moore S., Zhang X., Mudge A., Rowe J. H., Topping J. F., Liu J., et al. (2015). Spatiotemporal modelling of hormonal crosstalk explains the level and patterning of hormones and gene expression in Arabidopsis thaliana wild-type and mutant roots. New Phytol. 207 (4), 1110–1122. doi: 10.1111/nph.13421
Moss D. K., Ivany L. C., Judd E. J., Cummings P. W., Bearden C. E., Kim W.-J., et al. (2016). Lifespan, growth rate, and body size across latitude in marine bivalvia, with implications for phanerozoic evolution. Proc. R. Soc. B: Biol. Sci. 283 (1836), 20161364. doi: 10.1098/rspb.2016.1364
Muhammad G., Atsumi T., Komaru A. (2020). The influence of water temperature, salinity and food availability on nacre deposition rates in shells and pearls of Japanese and hybrid pearl oyster, Pinctada fucata. Aquaculture 528, 735512. doi: 10.1016/j.aquaculture.2020.735512
Munari M., Matozzo V., Marin M. G. (2011). Combined effects of temperature and salinity on functional responses of haemocytes and survival in air of the clam ruditapes philippinarum. Fish Shellfish Immunol. 30 (4-5), 1024–1030. doi: 10.1016/j.fsi.2011.01.025
Nagelkerken I., Munday P. L. (2016). Animal behaviour shapes the ecological effects of ocean acidification and warming: moving from individual to community-level responses. Global Change Biol. 22 (3), 974–989. doi: 10.1111/gcb.13167
Nascimento-Schulze J. C., Bean T. P., Houston R. D., Santos E. M., Sanders M. B., Lewis C., et al. (2021). Optimizing hatchery practices for genetic improvement of marine bivalves. Rev. Aquaculture 13 (4), 2289–2304. doi: 10.1111/raq.12568
Nguyen T. V., Alfaro A. C. (2020). Applications of omics to investigate responses of bivalve haemocytes to pathogen infections and environmental stress. Aquaculture 518, 734488. doi: 10.1016/j.aquaculture.2019.734488
Nie H., Chen P., Huo Z., Chen Y., Hou X., Yang F., et al. (2017). Effects of temperature and salinity on oxygen consumption and ammonia excretion in different colour strains of the Manila clam, Ruditapes philippinarum. Aquaculture Res. 48 (6), 2778–2786. doi: 10.1111/are.13111
Nie H., Jiang L., Huo Z., Liu L., Yang F., Yan X. (2016). Transcriptomic responses to low-temperature stress in the Manila clam, ruditapes philippinarum. Fish Shellfish Immunol. 55, 358–366. doi: 10.1016/j.fsi.2016.06.008
Nie H., Liu L., Wang H., Huo Z., Yan X. (2018). Stress levels over time in Ruditapes philippinarum: the effects of hypoxia and cold stress on hsp70 gene expression. Aquaculture Rep. 12, 1–4. doi: 10.1016/j.aqrep.2018.08.003
Nkuba A. C., Mahasri G., Lastuti N. D. R., Mwendolwa A. A. (2021). Correlation of nitrite and ammonia with prevalence of Enterocytozoon hepatopenaei (EHP) in shrimp (Litopenaeus vannamei) on several super-intensive ponds in East Java, Indonesia. Jurnal Ilmiah Perikanan dan Kelautan 13 (1), 58–67. doi: 10.20473/jipk.v13i1.24430
O’Connor K. (2019). Selection strategies to improve yield in macadamia using component traits and genomics. doi: 10.14264/uql.2019.862
Oliver E. C. J., Donat M. G., Burrows M. T., Moore P. J., Smale D. A., Alexander L. V., et al. (2018). Longer and more frequent marine heatwaves over the past century. Nat. Commun. 9 (1), 1324. doi: 10.1038/s41467-018-03732-9
Ortega L., Celentano E., Delgado E., Defeo O. (2016). Climate change influences on abundance, individual size, and body abnormalities in a sandy beach clam. Mar. Ecol. Prog. Ser. 545, 203–213. doi: 10.3354/meps11643
Parisi M. G., Mauro M., Sarà G., Cammarata M. (2017). Temperature increases, hypoxia, and changes in food availability affect immunological biomarkers in the marine mussel mytilus galloprovincialis. J. Comp. Physiol. B 187 (8), 1117–1126. doi: 10.1007/s00360-017-1089-2
Park H., Ahn I. Y., Lee H. E. (2007). Expression of heat shock protein 70 in the thermally stressed Antarctic clam Laternula elliptica. Cell Stress chaperones 12 (3), 275. doi: 10.1379/CSC-271.1
Parsons P. A. (2005). Environments and evolution: interactions between stress, resource inadequacy, and energetic efficiency. Biol. Rev. 80 (4), 589–610. doi: 10.1017/S1464793105006822
Peck L. S., Pörtner H. O., Hardewig I. (2002). Metabolic demand, oxygen supply, and critical temperatures in the Antarctic bivalve Laternula elliptica. Physiol. Biochem. Zoology 75 (2), 123–133. doi: 10.1086/340990
Perkins S. E., Alexander L. V. (2013). On the measurement of heat waves. J. Clim 26, 4500–4517. doi: 10.1175/JCLI-D-12-00383.1
Perkins-Kirkpatrick S. E., Stone D. A., Mitchell D. M., Rosier S., King A. D., Lo Y. E., et al. (2022). On the attribution of the impacts of extreme weather events to anthropogenic climate change. Environ. Res. Lett. 17 (2), 024009. doi: 10.1088/1748-9326/ac44c8
Pernet F., Gauthier-Clerc S., Mayrand É. (2007a). Change in lipid composition in eastern oyster (Crassostrea virginica gmelin) exposed to constant or fluctuating temperature regimes. Comp. Biochem. Physiol. Part B: Biochem. Mol. Biol. 147 (3), 557–565. doi: 10.1016/j.cbpb.2007.03.009
Pernet F., Tremblay R., Comeau L., Guderley H. (2007b). Temperature adaptation in two bivalve species from different thermal habitats: energetics and remodelling of membrane lipids. J. Exp. Biol. 210 (17), 2999–3014. doi: 10.1242/jeb.006007
Piano A., Valbonesi P., Fabbri E. (2004). Expression of cytoprotective proteins, heat shock protein, 70and metallothioneins in tissues of Ostrea edulis exposed to heat and heavy metals. Cell Stress chaperones 9 (2), 134. doi: 10.1379/483.1
Ponti M., Castellini A., Ragazzoni A., Gamba E., Ceccherelli V. U., Abbiati M. (2017). Decline of the Manila clams stock in the northern Adriatic lagoons: a survey on ecological and socio-economic aspects. Acta Adriatica 58 (1), 89–103. doi: 10.32582/aa.58.1.7
Pörtner H. O., Peck L., Somero G. (2007). Thermal limits and adaptation in marine Antarctic ectotherms: an integrative view. Philos. Trans. R. Soc. B: Biol. Sci. 362 (1488), 2233–2258. doi: 10.1098/rstb.2006.1947
Quinn N. L., McGowan C. R., Cooper G. A., Koop B. F., Davidson W. S. (2011). Ribosomal genes and heat shock proteins as putative markers for chronic, sublethal heat stress in Arctic charr: applications for aquaculture and wild fish. Physiol. Genomics 43 (18), 1056–1064. doi: 10.1152/physiolgenomics.00090.2011
Rahman M. A., Henderson S., Miller-Ezzy P., Li X. X., Qin J. G. (2019). Immune response to temperature stress in three bivalve species: pacific oyster Crassostrea gigas, Mediterranean mussel Mytilus galloprovincialis, and mud cockle Katelysia rhytiphora. Fish shellfish Immunol. 86, 868–874. doi: 10.1016/j.fsi.2018.12.017
Rahman M. A., Henderson S., Miller-Ezzy P. A., Li X. X., Qin J. G. (2020). Analysis of the seasonal impact of three marine bivalves on seston particles in water column. J. Exp. Mar. Biol. Ecol. 522, 151251. doi: 10.1016/j.jembe.2019.151251
Rahman M. S., Rahman M. S. (2021). Effects of elevated temperature on prooxidant-antioxidant homeostasis and redox status in the American oyster: signaling pathways of cellular apoptosis during heat stress. Environ. Res. 196, 110428. doi: 10.1016/j.envres.2020.110428
Reise K. (2012). Tidal flat ecology: an experimental approach to species interactions Vol. Vol. 54 (Springer Science & Business Media).
Rico-Villa B., Pouvreau S., Robert R. (2009). Influence of food density and temperature on ingestion, growth and settlement of pacific oyster larvae, crassostrea gigas. Aquaculture 287 (3–4), 395–401. doi: 10.1016/j.aquaculture.2008.10.054
Roberts D. A., Hofmann G. E., Somero G. N. (1997). Heat-shock protein expression in mytilus californianus acclimatization (seasonal and tidal-height comparisons) and acclimation effects. Biol. Bull. 192 (2), 309–320. doi: 10.2307/1542724
Robledo D., Palaiokostas C., Bargelloni L., Martínez P., Houston R. (2017). Applications of genotyping by sequencing in aquaculture breeding and genetics. Rev. Aquaculture 10 (3), 670–682. doi: 10.1111/raq.12193
Robson A. A. (2008). Gaping at environmental variability: how do bivalves react to changing circumstance (Swansea University).
Roegner G. C., Mann R. L. (1995). Early recruitment and growth of the American oyster crassostrea virginica (Bivalvia: ostreidae) with respect to tidal zonation and season. Mar. Ecol. Prog. Ser. 117, 91–101. doi: 10.3354/meps117091
Rosani U., Bortoletto E., Bai C.-M., Novoa B., Figueras A., Venier P., et al. (2021). Digging into bivalve miRNAomes: between conservation and innovation phil. Philos Trans A Math Phys Eng Sci 376 (1825), 20200165. doi: 10.1098/rstb.2020.0165
Rosani U., Pallavicini A., Venier P. (2016). The miRNA biogenesis in marine bivalves. PeerJ 4, e1763. doi: 10.7717/peerj.1763
Rostagno M. H. (2020). Effects of heat stress on the gut health of poultry. J. Anim. Sci. 98 (4). doi: 10.1093/jas/skaa090
Sakurai I., Seto M., Nakao S. (1996). Effects of water temperature, salinity, and substrata on burrowing behaviors of the three bivalves, Pseudocardium sachalinensis, mactra chinensis, and Ruditapes philipinarum. Nippon Suisan Gakkaishi 62 (6), 878–885. doi: 10.2331/suisan.62.878
Sampaio E., Lopes A. R., Francisco S., Paula J. R., Pimentel M., Maulvault A. L., et al. (2018). Ocean acidification dampens physiological stress response to warming and contamination in a commercially-important fish (Argyrosomus regius). Sci. Total Environ. 618, 388–398. doi: 10.1016/j.scitotenv.2017.11.059
Schluter D., Rieseberg L. H. (2022). Three problems in the genetics of speciation by selection. Proc. Natl. Acad. Sci. 119 (30). doi: 10.1073/pnas.2122153119
Seuront L., Nicastro K. R., Zardi G. I., Goberville E. (2019). Decreased thermal tolerance under recurrent heat stress conditions explains summer mass mortality of the blue mussel mytilus edulis. Sci. Rep. 9 (1), 17498. doi: 10.1038/s41598-019-53580-w
Shin A. C., Zheng H., Berthoud H. R. (2009). An expanded view of energy homeostasis: neural integration of metabolic, cognitive, and emotional drives to eat. Physiol. Behav. 97 (5), 572–580. doi: 10.1016/j.physbeh.2009.02.010
Smith K. E., Burrows M. T., Hobday A. J., King N. G., Moore P. J., Sen Gupta A., et al. (2022). Biological impacts of marine heatwaves. Annu. Rev. Mar. Sci. 15 (1), 119–145. doi: 10.1146/annurev-marine-032122-121437
Smith K. E., Burrows M. T., Hobday A. J., Sen Gupta A., Moore P. J., Thomsen M., et al. (2021). Socioeconomic impacts of marine heatwaves: global issues and opportunities. Science 374 (6566), eabj3593. doi: 10.1126/science.abj3593
Soares A. G., Callahan R. K., De ruyck A. M. C. (1998). Microevolution and phenotypic plasticity in donax serra röding (Bivalvia: donacidae) on high energy sandy beaches. J. molluscan Stud. 64 (4), 407–421. doi: 10.1093/mollus/64.4.407
Soissons L. M., da Conceicao T. G., Bastiaan J., van Dalen J., Ysebaert T., Herman P. M., et al. (2019). Sandification vs. muddification of tidal flats by benthic organisms: a flume study. Estuarine Coast. Shelf Sci. 228, 106355. doi: 10.1016/j.ecss.2019.106355
Sokolova I. M. (2013). Energy-limited tolerance to stress as a conceptual framework to integrate the effects of multiple stressors. Integr. Comp. Biol. 53 (4), 597–608. doi: 10.1093/icb/ict028
Sokolova I. M., Frederich M., Bagwe R., Lannig G., Sukhotin A. A. (2012). Energy homeostasis as an integrative tool for assessing limits of environmental stress tolerance in aquatic invertebrates. Mar. Environ. Res. 79, 1–15. doi: 10.1016/j.marenvres.2012.04.003
Sokolova I. M., Pörtner H. O. (2001). Physiological adaptations to high intertidal life involve improved water conservation abilities and metabolic rate depression in Littorina saxatilis. Mar. Ecol. Prog. Ser. 224, 171–186. doi: 10.3354/meps224171
Sokołowski A., Brulińska D. (2018). The effects of low seawater pH on energy storage and heat shock protein 70 expression in a bivalve Limecola balthica. Mar. Environ. Res. 140, 289–298. doi: 10.1016/j.marenvres.2018.06.018
Somero G. N. (2020). The cellular stress response and temperature: function, regulation, and evolution. J. Exp. Zoology Part A: Ecol. Integr. Physiol. 333 (6), 379–397. doi: 10.1002/jez.2344
Stevens A. M., Gobler C. J. (2018). Interactive effects of acidification, hypoxia, and thermal stress on growth, respiration, and survival of four north Atlantic bivalves. Mar. Ecol. Prog. Ser. 604, 143–161. doi: 10.3354/meps12725
Storey K. B., Storey J. M. (1990). Metabolic rate depression and biochemical adaptation in anaerobiosis, hibernation and estivation. Q. Rev. Biol. 65 (2), 145–174. doi: 10.1086/416717
Strahl J. (2011). Life strategies in the long-lived bivalve arctica islandica on a latitudinal climate gradient–environmental constraints and evolutionary adaptations (University of Bremen).
Suchanek T. H. (1978). The ecology of Mytilus edulis l. @ in exposed rocky intertidal communities. J. Exp. Mar. Biol. Ecol. 31 (1), 105–120. doi: 10.1016/0022-0981(78)90139-9
Thieltges D. W., Krakau M., Andresen H., Fottner S., Reise K. (2006). Macroparasite community in molluscs of a tidal basin in the wadden sea. Helgoland Mar. Res. 60 (4), 307–316. doi: 10.1007/s10152-006-0046-3
Thomas Y., Bacher C. (2018). Assessing the sensitivity of bivalve populations to global warming using an individual-based modelling approach. Global Change Biol. 24 (10), 4581–4597. doi: 10.1111/gcb.14402
Thomas Y., Pouvreau S., Alunno -Bruscia M., Barillé L., Gohin F., Bryère P., et al. (2016). Global change and climate -driven invasion of the pacific oyster (Crassostrea gigas) along European coasts: a bioenergetics modelling approach. J. Biogeography 43 (3), 568–5579. doi: 10.1111/jbi.12665
Tomasetti S. J., Hallinan B. D., Tettelbach S. T., Volkenborn N., Doherty O. W., Allam B., et al. (2023). Warming and hypoxia reduce the performance and survival of northern bay scallops (Argopecten irradians irradians) amid a fishery collapse. Global Change Biol. 29, 2092–2107. doi: 10.1111/gcb.16575
Troost K. (2010). Causes and effects of a highly successful marine invasion: case -study of the introduced pacific oyster crassostrea gigas in continental NW European estuaries. J. Sea Res. 64 (3), 145–1165. doi: 10.1016/j.seares.2010.02.004
Truebano M., Burns G., Thorne M. A. S., Hillyard G., Peck L. S., Skibinski D. O. F., et al. (2010). Transcriptional response to heat stress in the Antarctic bivalve laternula elliptica. J. Exp. Mar. Biol. Ecol. 391, 65–72. doi: 10.1016/j.jembe.2010.06.011
Verdelhos T., Marques J. C., Anastácio P. (2015). Behavioral and mortality responses of the bivalves Scrobicularia plana and Cerastoderma edule to temperature, as indicator of climate change’s potential impacts. Ecol. Indic. 58, 95–103. doi: 10.1016/j.ecolind.2015.05.042
Virgin S. D. S., Lyons K. J., Barbeau M. A. (2019). Microhabitat heterogeneity and population ecology of ribbed mussels (Geukensia demissa) near their northern range limit (Maritime Canada). Estuaries Coasts 42 (6), 1541–1557. doi: 10.1007/s12237-019-00598-y
Vohmann A., Borcherding J., Kureek A. (2009). Strong body mass decrease of the invasive clam Corbicula fluminea during summer. Biol. Invasions 12, 53–64. doi: 10.1007/s10530-009-9429-0
Wang J., Dong B., Yu Z. X., Yao C. L. (2018a). The impact of acute thermal stress on green mussel Perna viridis: oxidative damage and responses. Comp. Biochem. Physiol. Part A: Mol. Integr. Physiol. 222, 7–15. doi: 10.1016/j.cbpa.2018.04.001
Wang J., Ren R.-m., Yao C.-L. (2018b). Oxidative stress responses of mytilus galloprovincialis to acute cold and heat during air exposure. J. Molluscan Stud. 84 (3), 285–292. doi: 10.1093/mollus/eyy027
Wanninger A., Wollesen T. (2019). The evolution of molluscs. Biol. Rev. Cambridge Philos. Soc. 94 (1), 102–115. doi: 10.1111/brv.12439
Wei H., Deng Z., Zhao W., Liao M., Li Y., Yu G., et al. (2021). Effects of air exposure at different temperatures in selected breeding lines of Pinctada fucata with different shell color. Aquaculture Rep. 21, 100879. doi: 10.1016/j.aqrep.2021.100879
Williams P. C., Bartlett A. W., Howard-Jones A., McMullan B., Khatami A., Britton P. N., et al. (2021). Impact of climate change and biodiversity collapse on the global emergence and spread of infectious diseases. J. paediatrics Child Health 57 (11), 1811–1818. doi: 10.1111/jpc.15681
Wu F., Lu W., Shang Y., Kong H., Li L., Sui Y., et al. (2016). Combined effects of seawater acidification and high temperature on hemocyte parameters in the thick shell mussel mytilus coruscus. Fish Shellfish Immunol. 56, 554–562. doi: 10.1016/j.fsi.2016.08.012
Xu X., Tong Y., Deng Y., Zhao L. (2023a). Impacts of marine heatwaves on byssus production in highly invasive fouling mussels. Mar. Environ. Res. 184, 105871. doi: 10.1016/j.marenvres.2022.105871
Xu Y., Zhang Y., Liang J., He G., Liu X., Zheng Z., et al. (2021). Impacts of marine heatwaves on pearl oysters are alleviated following repeated exposure. Mar. pollut. Bull. 173, 112932. doi: 10.1016/j.marpolbul.2021.112932
Xu X., Zhang X., Peng J., Deng Y., Liu Y., Jiang L., et al. (2023b). Survival and physiological energetics of highly invasive mussels exposed to heatwaves. Mar. Environ. Res. 187, 105948. doi: 10.1016/j.marenvres.2023.105948
Xu Y., Zhao L., Jian L., He G., Liu X., Yang K., et al. (2022). Responses of pearl oysters to marine heatwaves as indicated by HSP70. Front. Mar. Sci. 9. doi: 10.3389/fmars.2022.847585
Yao C. L., Somero G. N. (2012). The impact of acute temperature stress on hemocytes of invasive and native mussels (Mytilus galloprovincialis and Mytilus californianus): DNA damage, membrane integrity, apoptosis, and signaling pathways. J. Exp. Biol. 215 (24), 4267–4277. doi: 10.1242/jeb.073577
Yao C. L., Somero G. N. (2013). Thermal stress and cellular signaling processes in hemocytes of native (Mytilus californianus) and invasive (M. galloprovincialis) mussels: cell cycle regulation and DNA repair. Comp. Biochem. Physiol. Part A: Mol. Integr. Physiol. 165 (2), 159–168. doi: 10.1016/j.cbpa.2013.02.024
Yin X., Chen P., Chen H., Jin W., Yan X. (2017). Physiological performance of the intertidal Manila clam (Ruditapes philippinarum) to long-term daily rhythms of air exposure. Sci. Rep. 7, 41648. doi: 10.1038/srep41648
Yurimoto T., Kassim F. M., Fuseya R., Man A. (2014). Mass mortality event of the blood cockle, Anadara granosa, in aquaculture ground along selangor coast, peninsular Malaysia. Int. Aquat Res. 6, 177–186. doi: 10.1007/s40071-014-0077-3
Zeng J., Huynh N., Phelps B., King-Jones K. (2020). Snail synchronizes endocycling in a TOR-dependent manner to coordinate entry and escape from endoreplication pausing during the drosophila critical weight checkpoint. PloS Biol. 18 (2), e3000609. doi: 10.1371/journal.pbio.3000609
Zhang H., Jia H., Xiong P., Yao G., He M. (2022a). Transcriptome and enzyme activity analyses of tolerance mechanisms in pearl oyster Pinctada fucata under high-temperature stress. Aquaculture 550, 737888. doi: 10.1016/j.aquaculture.2022.737888
Zhang J., Li Q., Xu C., Han Z. (2019). Response to selection for growth in three selected strains of the pacific oyster Crassostrea gigas. Aquaculture 503, 34–39. doi: 10.1016/j.aquaculture.2018.12.076
Zhang W. Y., Storey K. B., Dong Y. W. (2020). Adaptations to the mudflat: insights from physiological and transcriptional responses to thermal stress in a burrowing bivalve Sinonovacula constricta. Sci. Total Environ. 710, 136280. doi: 10.1016/j.scitotenv.2019.136280
Zhang H., Tan K., Deng H., Zheng H. (2022b). Characterization of the CnMnSOD in response to lower temperature stress in noble scallop Chlamys nobilis with different carotenoids content. Aquaculture Res. 53 (6), 2448–2456. doi: 10.1111/are.15762
Zhang H., Zhou Z., Yue F., Wang L., Yang C., Wang M., et al. (2014). The modulation of catecholamines on immune response of scallop Chlamys farreri under heat stress. Gen. Comp. Endocrinol. 195, 116–124. doi: 10.1016/j.ygcen.2013.11.006
Zhao L., Shirai K., Tanaka K., Milano S., Higuchi T., Murakami-Sugihara N., et al. (2020). A review of transgenerational effects of ocean acidification on marine bivalves and their implications for sclerochronology. Estuar. Coast. Shelf Sci. 235, 106620. doi: 10.1016/j.ecss.2020.106620
Zhao X., Yu H., Kong L., Liu S., Li Q. (2016). High throughput sequencing of small RNAs transcriptomes in two Crassostrea oysters identifies microRNAs involved in osmotic stress response. Sci. Rep. 6 (1), 22687. doi: 10.1038/srep22687
Zheng H., Tan K., Zhang H., Ma H., Li S., Zheng H. (2022). Intraspecific hybridization as a mitigation strategy of ocean acidification in marine bivalve noble scallop Chlamys nobilis. Sci. Total Environ. 832, 154736. doi: 10.1016/j.scitotenv.2022.154736
Zhong X., Feng D., Yu H., Kong L., Li Q. (2016). Genetic variation and breeding signature in mass selection lines of the pacific oyster (Crassostrea gigas) assessed by SNP markers. PloS One 11 (3), e0150868. doi: 10.1371/journal.pone.0150868
Zhou Z., Bouma T. J., Fivash G. S., Ysebaert T., van IJzerloo L., van Dalen J., et al. (2022). Thermal stress affects bioturbators’ burrowing behavior: a mesocosm experiment on common cockles (Cerastoderma edule). Sci. Total Environ. 824, 153621. doi: 10.1016/j.scitotenv.2022.153621
Zhou L., Liu Z., Dong Y., Sun X., Wu B., Yu T., et al. (2019). Transcriptomics analysis revealing candidate genes and networks for sex differentiation of yesso scallop (Patinopecten yessoensis). BMC Genomics 20 (1), 671. doi: 10.1186/s12864-019-6021-6
Zhou Z., Wang L., Song L., Liu R., Zhang H., Huang M., et al. (2014). The identification and characteristics of immune-related microRNAs in haemocytes of oyster crassostrea gigas. PloS One 9 (2), e88397. doi: 10.1371/journal.pone.0088397
Keywords: climate change, extreme weather event, physiology, bivalve, heat stress
Citation: Masanja F, Yang K, Xu Y, He G, Liu X, Xu X, Xiaoyan J, Xin L, Mkuye R, Deng Y and Zhao L (2023) Impacts of marine heat extremes on bivalves. Front. Mar. Sci. 10:1159261. doi: 10.3389/fmars.2023.1159261
Received: 05 February 2023; Accepted: 19 May 2023;
Published: 08 June 2023.
Edited by:
Carter Newell, Pemaquid Mussel Farms, United StatesCopyright © 2023 Masanja, Yang, Xu, He, Liu, Xu, Xiaoyan, Xin, Mkuye, Deng and Zhao. This is an open-access article distributed under the terms of the Creative Commons Attribution License (CC BY). The use, distribution or reproduction in other forums is permitted, provided the original author(s) and the copyright owner(s) are credited and that the original publication in this journal is cited, in accordance with accepted academic practice. No use, distribution or reproduction is permitted which does not comply with these terms.
*Correspondence: Liqiang Zhao, bHpoYW9AZ2RvdS5lZHUuY24=