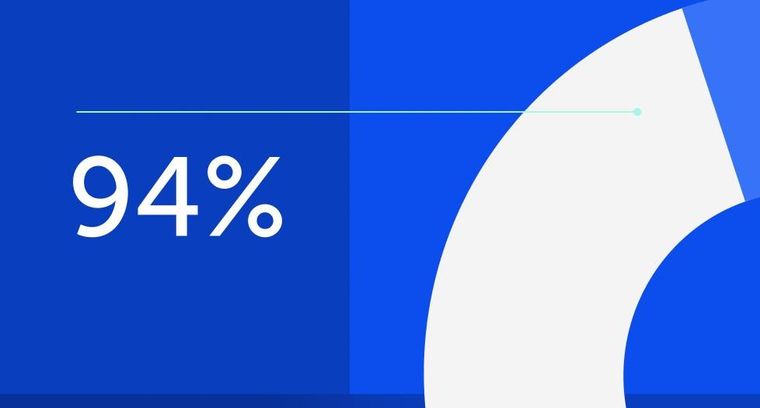
94% of researchers rate our articles as excellent or good
Learn more about the work of our research integrity team to safeguard the quality of each article we publish.
Find out more
ORIGINAL RESEARCH article
Front. Mar. Sci., 28 February 2023
Sec. Aquatic Physiology
Volume 10 - 2023 | https://doi.org/10.3389/fmars.2023.1151605
This article is part of the Research TopicCephalopods in the Anthropocene: Multiple Challenges in a Changing OceanView all 16 articles
Anthropogenic activity and its associated sounds have been shown to incur adverse effects on the behaviour and physiology of a wide range of aquatic taxa, from marine mammals to fishes. Yet, little is known about how invertebrates detect and respond to anthropogenic sound. The hummingbird bobtail squid (Euprymna berryi) has a short lifespan (< 6 months), grows to sexual maturity around 90 days post hatching and its small size (< 5 cm mantle length) makes the species an ideal candidate to examine potential effects of sound exposure under laboratory conditions. Hearing and behavioural observations were made before, during and after 15 minutes of vessel sound playback, and aural sensitivity curves were determined using auditory evoked potentials. A significant decrease in relative ventilation rate was observed during and post sound exposure. Auditory sensitivity before and after vessel sound exposure was also examined for three different ages: juveniles, mid- and late adults. Baseline audiograms indicated that there was a decrease in aural sensitivity with age. All three age groups showed similar, significantly decreased hearing sensitivity following sound exposure, however auditory sensitivity recovered within two hours. Globally, anthropogenic sounds have become louder and more persistent, therefore there may be limited time for these animals to recover from sound exposure. Given their ecological and economic importance, cephalopods should be considered in management and policy on underwater noise owing to potential adverse effects of anthropogenic sound on behaviour and physiology.
Underwater sound is used by aquatic life to navigate their environment, to find suitable habitats, locate food and avoid predators, as well as communicate with conspecifics. In recent years, animals have been exposed to increasing amounts of anthropogenic sound which may negatively affect their behaviour (e.g., foraging, movements, predator/prey interactions and mating (Myrberg, 1990; Shannon et al., 2016; Erbe et al., 2018) and physiology (e.g., heart rate, oxygen consumption and hearing)(Williams et al., 2015; de Soto and Kight, 2016) . However, most bioacoustics research has focussed on marine mammals and fishes, while the extent to which sound produced by human activity might be affecting invertebrates has been largely overlooked (Hawkins et al., 2015; Popper and Hawkins, 2018).
Ecologically, cephalopods use sound as they occupy many of the same niches as other acoustically sensitive fishes. Shipping, geophysical activity and construction all produce low frequency (< 1000 Hz) sound, which may overlap the hearing sensitivity of cephalopods, contributing to masking of biologically relevant stimuli or inducing acoustic trauma (Mooney et al., 2010) . Behavioural reactions to a sound stimulus can range from exhibiting a momentary awareness of the sound, to small movements, or escape responses (Hawkins et al., 2015). Cephalopods occupy a key position in the food web (Boyle and Rodhouse, 2008: and if their population declines or migrates, for example to avoid a noisier environment, disruptive effects on the trophic structure potentially could occur (e.g., Tyack et al., 2011). Sound exposures may also induce physiological damage and more severe impairments to function and fitness such as permanent or temporary hearing loss (Hawkins et al., 2015).
Cephalopods possess hair filled statocysts that regulate equilibrium and balance, and these organs have been associated with sound detection (Offutt, 1970; Budelmann, 1990; Williamson, 1995). Each statocyst contains three lobes positioned in the x, y and z planes, which contain heavily innervated hair cells coupled to the statolith, a dense particle within the statocyst (Budelmann, 1990; André et al., 2011). During underwater sound wave propagation, regions of compression and rarefaction generated by local particle motion are produced in conjunction with pressure fluctuations. The statocyst detects sound through the differential displacement of the heavier statolith in contrast to the surrounding tissues and endolymph (Budelmann, 1992). The statocyst is further divided into a macula that aids orientation, and a crista-cupula that acts as an angular accelerometer (Budelmann, 1990; Mooney et al., 2010).
Auditory evoked potentials (AEPs) have been employed to investigate the auditory responses of animals that are challenging to study via conventional psychophysical experiments, such as fishes and invertebrates (Higgs and Radford, 2016; Sisneros et al., 2016). AEPs reflect synchronous neural activity as afferent responses are conducted from the auditory end organ to higher centres (Burkhard et al., 2007). It was recently determined that adult cephalopods can detect low frequency vibrations using the AEP technique. Sepiotethis lessoniana (bigfin reef squid) and Octopus sp. (common octopus) were sensitive to frequencies between 400 and 1600 Hz (Hu et al., 2009), while Dorytheuthis pealeii (longfin squid) showed auditory sensitivity to frequencies between 30 and 500 Hz, with the lowest threshold between 100 and 200 Hz (Mooney et al., 2010). A behavioural study on D. pealeii also showed sensitivity to 200 - 400 Hz sound (Mooney et al., 2016). The modality and frequency range of hearing suggests that squid probably detect acoustic particle motion stimuli from both predators and prey, as well as low frequency environmental sound signatures that may aid navigation (Mooney et al., 2010). However, these studies focussed solely on adults, and it is unknown to what extent newly hatched and juvenile cephalopods use sound. In other marine invertebrates, juveniles are often hypersensitive to external stimuli or stressors. For larval crustaceans, sound plays an important role for juveniles to orientate and settle on suitable reef habitat (Montgomery et al., 2006; Stanley et al., 2012). Squid statocysts are fully formed in hatchlings (Hanlon et al., 1983) indicating that cephalopods may have the capacity for sound sensitivity from an early age.
The hummingbird bobtail squid (Euprymna berryi) has a short lifespan (< 6 months), grows to sexual maturity around 90 days post hatching and its small size (< 5 cm mantle length) makes the species an ideal candidate for examining the ontogeny of cephalopod hearing. Additionally, the benthic E. berryi inhabit shallow waters (< 20 m) throughout the Indo-Pacific, preferring sandy or fine sediment substrate where they bury themselves during the day to avoid predation. The benthic lifestyle may make E. berryi more susceptible to the negative effects of sound exposure than mobile species that can swim away from the source, especially as anthropogenic sounds often peak during daylight hours.
The aims of this study were to determine, under laboratory conditions, the auditory sensitivity of the hummingbird bobtail squid (E. berryi) and to investigate the potential impacts of noise on the behaviour and auditory sensitivity of different life stages.
Hummingbird bobtail squid (E. berryi) were cultured at the Marine Biological Laboratory’s Cephalopod Breeding Centre in Woods Hole, MA to three different ages [juvenile 45 – 60 days (n = 16); mid adult 90 – 100 days (n = 15); late adult 125 – 140 days (n = 14)] for the experiments (Table 1). These age groups were chosen to reflect pre and post sexual maturity (juvenile and mid adult) as well as potential senescence (late adult).
Table 1 Number of animals used for the behavioural and hearing experiments (baseline; noise exposure; recovery).
All squid were housed in tanks on a semi-open system where natural seawater was conditioned (mechanically, chemically, and biologically filtered) and maintained at 24°C, 8.5 pH, 32 ppt salinity, 0 ppm ammonia/nitrite and< 10 ppm nitrate. Each age group was housed separately in holding tanks that measured 21.5 cm x 11.0 cm for juveniles, 45.5 cm x 23.5 cm for mid-adults and 44.0 cm x 76.0 cm for late adults. The sound pressure level in the holding tanks was recorded using a hydrophone (Soundtrap300; Ocean Instruments, NZ, sampling rate 24 kHz), and the median root mean squared sound pressure level was 80 dB re. 1µPa (10 Hz – 12 kHz).
All experiments were conducted between June 1st and September 2nd, 2022, in a rectangular fibreglass experimental tank (56 x 40 x 30 cm). The experimental tank was enclosed within an acoustic isolation box (76 x 68 x 66 cm) covered on all sides with 2.2 cm thick insulating foam panels to reduce background sound and placed on a vibration isolation table (TMS Vibration Control 63-543 = 122 x 76 cm) to minimise vibrations. All trials were conducted during the daytime, and squid were tested individually for each trial.
This animal study was reviewed and approved by the Institutional Animal Care and Use Committee at the Marine Biological Laboratory, Woods Hole, MA, USA protocol number 22-13F, in compliance with the EU Directive 2010/63/EU on cephalopod use and AAALAC guidelines on the care and welfare of cephalopods (Fiorito et al., 2014, 2015; Lopes et al., 2017).
To assess changes in behaviour in relation to sound, video recordings were taken of unanaesthetised baseline and sound exposed individuals (Table 1). On the day of the experiment, individual squid were hand-transferred from their holding tanks into the experimental tank and placed inside a soft mesh arena (10 x 10 x 4 cm) where individuals remained in the centre of the tank. Squid were allowed to acclimate to the experimental tank for five minutes (preliminary trials showed this time was sufficient as squid remained quiescent). A high-resolution camera (PixeLINK PL-E533CU, Pixelink, Ontario, Canada) was positioned 28 cm above the experimental tank to observe behaviour under an infrared light (850 nm, S75, SmartVision) with viewing field encompassing the entire 10 x 10 cm experimental arena (Figure 1). PixelLinkCapture software (PixeLINK, Ontario, Canada) was used to capture video recordings at 19 frames per second at a resolution of 1980 x 1020 pixels.
Figure 1 AEP experimental tank setup (not to scale). 1: Experimental tank; 2: Underwater speaker (Lubell Labs UW-30); 3. Mesh sling with animal suspended 4cm below water surface; 4: Hydrophone (B&K 8103); 5. Ground for experimental tank; 6. Recording and reference electrodes; 7. Headstage (Dagan); 8. Camera (Thorlabs); 9. IR light; 10. Acoustic isolation chamber; 11. Vibration isolation table (TMS Vibration Control); 12. Sump tank; 13. Pump and associated tubing; 14. Bubbler; 15. Heater.
Baseline individuals were observed via video recordings for 15 minutes with no sound exposure. Sound exposed animals were recorded for three different 15-minute sessions before, during and after presentation of vessel sound. Vessel sound [sound pressure (re 1 µPa) = 150 dB; particle acceleration (re. 1 ms-2) = -8.6 dB (both measured between 100 and 1000 Hz)] was emitted from the underwater speaker (UW30; Lubell Labs Inc, USA) submerged in the centre of the experimental tank, 16 cm below the squid. Sound exposure level was limited by the maximum capacity of the underwater speaker however, the exposure level was deemed suitable given that vessel source levels have been documented up to 195 dB in the field (McKenna et al., 2013). The exposure duration was also designed to replicate exposure to anthropogenic sound in the natural environment. Audio files of the vessel [an idling 15 m research vessel (Detroit Diesel 12 V-71 engine; power output: 7 – 1193 kW; single screw)] sound used for exposure experiments were recorded on a hydrophone (Soundtrap 300; Ocean Instruments, NZ). The hydrophone was positioned midwater (water depth 2 m) ca. 1 m away from the propellor of the research vessel while it was idling dockside (Mackiewicz et al., 2021).
Behaviours of the mid- and late-adult animals (baseline and sound exposure individuals) were observed by the author via the video recordings post hoc. No jetting, inking or colour change was observed from any of the animals. The number of breaths per minute was recorded using a manual hand-held counter (respiration rates provided in Supplementary Tables 1, 2). Individual’s ventilation rate (breaths per minute) was divided by the average value of the 15 minutes of its pre baseline counts to provide a relative value for comparing between the different age groups and different exposure conditions (before, during and after sound exposure). Juveniles underwent the same experimental sound exposure treatments. However, due to equipment availability, video was not recorded for juvenile behavioural observations.
Immediately after the initial behavioural observations, auditory evoked potential (AEP) recordings were used to measure the hearing sensitivity of the baseline and sound exposed individuals across the same three different age groups (juvenile, mid-adult, and late-adult; Table 1). To examine potential recovery of auditory sensitivity, a subset of the sound exposed individuals were re-tested 2 hours post sound exposure. All AEP recordings were performed in the same rectangular fiberglass experimental tank used for behavioural observations (Figure 1).
Following behavioural observations, squid were anesthetised by immersion in a bath of MgCl2 solution [7.5% (75 g dissolved in 1 L distilled water) mixed with home tank sea water in a ratio of 1:3 MgCl2: sea water]. MgCl2 has been identified to have no effect on cephalopod evoked responses and allows the animals to continue breathing (Messenger et al., 1985; Preuss and Budelmann, 1995; Mooney et al., 2010). State of anesthesia was confirmed once the individual stopped responding to a physical stimulus (a gentle pinch with a pair of blunt forceps on the mantle). Additional signs of anesthesia included slowed respiratory rate, pale colour, and loss of sucker attachment.
Once sedated, animals were moved from the anaesthetic bath back into the experimental tank. Inside the experimental tank, the individual was supported by a nylon mesh sling 16 cm directly above the underwater speaker at a fixed position of 4 cm water depth. Briefly, the AEP procedure entailed placing two subdermal electrodes (Rochester Electro-Medical Inc., USA) just under the skin of the animal following the methodology of Mooney et al., 2010. The recording electrode was placed at the posterior margin of the head, medial to the statocyst, using a micromanipulator (World Precision Instruments; M3301-M3). The reference electrode was inserted in the muscle of the cephalopod’s body, at least 1 cm away from the head. Only the tips of the electrodes were uncoated to allow for evoked potential recordings from the desired location. The rest of the electrode and wire leads were waterproofed to allow for recording underwater.
Pure tone stimuli (50 ms duration; 500 repetitions; 3 ms silence between repetitions) between 100 and 1000 Hz were presented to the animals via an underwater speaker in the experimental tank; the sound output was monitored in real time using an oscilloscope (Tetronix, USA). Individuals were tested in 100 Hz increments to produce an audiogram. For the sound stimulus, a programmable attenuator (CED 3505; Cambridge Electronics Design, UK) and biological amplifier (AS-35; Accusonic Corp, Canada) controlled sound amplitude in 3 dB re. 1 µPa steps up to the maximum sound level of the underwater speaker (150 dB re. 1 µPa sound pressure level, -8.6 dB re. 1 ms-2 particle acceleration).
It is important to note that the difference between sound pressure and particle motion cannot be easily predicted in small tanks (Nedelec et al., 2016; Sisneros et al., 2016; Jézéquel et al., 2022). Therefore, prior to the start of each AEP trial, particle motion and sound pressure were calibrated at the position normally occupied by the experimental animal to allow audiograms to be produced for the two measures. Particle motion was measured using a tri-axial accelerometer (PCB Piezotronics Inc., USA; sensitivity: X = 10.47 mV ms-1; Y = 10.35 mV ms-1; Z = 10.29 mV ms-1, frequency response 0.5 – 2000 Hz), modified to be neutrally buoyant using a polystyrene float to counterbalance the weight of the device, connected to a signal conditioner (482C; PCB Piezotronics Inc., USA). The accelerometer was placed such that its x-axis corresponded to the anterior-posterior, the y-axis to the left-right, and the z-axis to the dorsal-ventral positions. For a given frequency, particle acceleration measurements were made across the corresponding sound intensity range throughout the attenuation range. Sound pressure was measured using a hydrophone (8103; Bruel and Kjaer, Denmark) connected to an amplifier (Nexus Conditioning Amplifier 2692-01s; Bruel and Kjaer, Denmark). All data were recorded using Power Lab data acquisition system and analysed offline as the voltage root mean square (Vrms) using LabChart software (Version 8, AD Instruments, USA).
Vrms values measured with the hydrophone were converted into dBrms (Equation 1).
Vrms values for each axis (X, Y and Z) of the particle accelerometer were calibrated to the sensitivity of the accelerometer and used to calculate the magnitude of particle acceleration in dB scale (equation 2) (Vetter et al., 2015, 2018; Nissen et al., 2019).
During the AEP recordings, signals from the implanted electrodes were amplified with a headstage (gain = 10 x) connected to an extracellular amplifier (gain = 100 x; EX1; Dagan Corporation, Minneapolis, MN, USA), with a 0.05 to 10.0 kHz band pass filter. A data acquisition system (micro3, 1401; Cambridge Electronic Design Ltd., UK) administered sound stimuli and a custom Spike 2 script (Cambridge Electronic Design; UK) collected and averaged responses.
The presence of an AEP was initially assessed visually by observation of the characteristic double frequency AEP wave (Maruska et al., 2007). Secondly, the presence of an AEP was verified quantitatively by fast Fourier transform (FFT) power spectrum analysis (1024 pt, Hanning window) of the averaged waveform response. The auditory threshold was defined as the lowest sound intensity that elicited an observable and repeatable AEP, with the presence of a significant peak (FFT level ≥ 0.001 µV) at the second harmonic of the stimulation frequency (Egner and Mann, 2005; Higgs and Radford, 2016; Bhandiwad et al., 2017) because of the opposed orientation of the hair cells (Budelmann, 1990; Ladich and Fay, 2013; Sisneros et al., 2016) (Figure 2).
Figure 2 Auditory evoked potential (AEP) response for (A) juvenile; (B) mid-adult and (C) late-adult hummingbird bobtail squid (E. berryi) to (D) the 300 Hz signal presented. Each panel (A–C) displays the average AEP trace (500 repetitions) for the indicated sound pressure level (dB re. 1 µPa) on the left and the fast Fourier transformation (FFT) on the right. Please note the y axis for the FFT data varies. FFT peaks are twice the stimulus frequency (600 Hz) owing to the opposed orientation of the hair cells. AEP thresholds (0.005 µV) for each age group are highlighted in red.
At the conclusion of each AEP experiment, individuals were euthanised with an overdose of MgCl2 solution (1:1 dilution of 7.5% MgCl2 in home tank seawater). Individuals remained in the solution for a minimum of 10 minutes following the cessation of respiration. A recently euthanised squid was used as a control animal to ensure all detected waveforms from the anesthetised squid were biological in origin.
All statistical analyses were performed using Sigmaplot software (Version 14). Ventilation rate data was calculated as a percentage, to determine the effects of sound exposure on the observed ventilation rates of squid; the data was arcsine transformed and analysed using a Kruskal-Wallis one-way ANOVA on ranks. A Tukey post hoc test determined significant differences between time periods for each age group (α = 0.05).
Auditory evoked potential data passed normality (Shapiro-Wilk P > 0.05) and equal variance (Brown-Forsythe) testing therefore all sensitivity data are reported as mean ± 1 SD. To determine whether there was a difference in baseline auditory sensitivity between juvenile, mid-adult and late-adult squid, a two-way analysis of variance (ANOVA) with frequency (Hz) and age as factors, and sensitivity measurements [either sound pressure level (SPL) or particle acceleration level (PAL)] as the dependent variable was performed. A Holm-Sidak post hoc test determined significant differences between ages for each frequency (α = 0.05).
Additionally, to determine the effects of sound exposure and recovery on the auditory sensitivity of squid, a two-way repeated measure ANOVA with frequency (Hz) and time (baseline, sound exposure or recovery) as factors, and sensitivity measurements (either sound pressure level or particle acceleration level) as the dependent variable was performed. A Holm-Sidak post hoc test determined significant sensitivity shifts from baseline for each frequency (α = 0.05).
Background sound levels were significantly lower, across all frequencies, than the sound exposure playback (Figures 3A, B). For example, at 300 Hz, the background sound power spectral density was 68 dB re 1 µPa2 Hz-1 compared to 118 dB re 1 µPa2 for sound exposure.
Figure 3 (A) Power spectral density (dB re. 1 µPa2 Hz-1) curves versus frequency for the background sound of the experimental tank (black) and sound exposure (red). (B) Spectrogram for the sound exposure trial versus time. (C) Box plots (maximum, 75%, median, 25%, minimum) for relative ventilation rates (breaths min-1/average pre) of the mid adult (light blue) and late adult (dark blue) versus sound treatment (before; sound exposure, after), asterisk indicates significant difference to before.
The ventilation rates of mid- and late-adult squid were observed before, during and after sound exposure. There was a significant decrease in the relative ventilation rate both during and after sound exposure compared to before levels for the two age groups tested (mid adult: one-way ANOVA, H = 80.681, d.f. = 2, P< 0.001; late adult: one-way ANOVA, H = 30.735, d.f. = 2, P< 0.001) (Figure 3C). No significant difference was found between the two ages groups observed (Figure 3C). Additionally, there was no significant difference in the relative ventilation rates between individuals within each age group (Supplementary Figure 1).
Bobtail squid responded to all tested frequencies between 100 and 1000 Hz, and all age groups showed greatest auditory sensitivity between 300 and 500 Hz. Sensitivity decreased up to 1000 Hz and AEPs above 1000 Hz were not detectable at the maximum sound pressure levels (150 dB re. 1 µPa). There was a significant difference in both PAL (two-way ANOVA, d.f. = 2, F = 9.593, P = 0.002) and SPL (two-way ANOVA, d.f. = 2, F = 5.011, P = 0.020) auditory sensitivity curves when the different age groups were compared (Figure 4). Post-hoc tests (Holm-Sidak, p< 0.05) showed significant differences in PAL were observed at 200, 300, 400, 900 and 1000 Hz (Figure 4A). For example, at 300 Hz, juvenile squid were most sensitive (-32.5 ± 1.0 dB re. 1ms-2), followed by mid-adult (-21.2 ± 1.9 dB re. 1ms-2) and late adult (-14.5 ± 4.6 dB re. 1ms-2). Significant differences were also observed between the different ages at 200 and 300 Hz when thresholds were measured in SPL (Figure 4B). For example, at 300 Hz, mid-adults were most sensitive (SPL: 135.3 ± 2.9 dB re. 1 µPa) followed by juveniles (SPL: 140.3 ± 1.2 dB re. 1 µPa) and late-adults (141.0 ± 2.9 dB re. 1 µPa). Individual SPL and PAL threshold values for all frequencies and ages tested are provided in Supplementary Tables 3, 4.
Figure 4 The thresholds of (A) particle acceleration level (dB re. 1 ms-2) and (B) sound pressure level (dB re. 1 µPa) needed to evoke an AEP response plotted against frequency (Hz) for three different aged hummingbird bobtail squid (E. berryi). Data are plotted as mean ± SD. Statistical difference in sensitivity at that frequency indicated by circles (O) between juveniles and mid adults, triangles (Δ) between juvenile and late adult, and asterisks (*) between mid adult and late adults.
Following 15 minutes of vessel sound exposure, tone pips of higher PALs were needed to evoke a response in juvenile and late adults (Figure 5). This also corresponded to similar, higher SPLs for all age groups post sound-exposure. Juveniles exhibited a significant decrease in auditory sensitivity following sound exposure at 400, 500, 600 and 800 Hz in both SPL and PAL sensitivity audiograms (Figures 5A, B); whereas mid-adults had a significant decrease in auditory sensitivity for SPL audiograms at 200, 300, 400 and 500 Hz but no significant difference in PAL audiograms (Figures 5C, D). Late-adults had significant differences in auditory sensitivity for PAL at 200 Hz and for SPL at 400, 500 and 600 Hz (Figures 5E, F).
Figure 5 The thresholds of (A, C, E) particle acceleration level (dB re. 1 ms-2) and (B, D, F) sound pressure level (dB re. 1 µPa) needed to evoke an AEP response plotted against frequency (Hz) for three different aged hummingbird bobtail squid (E. berryi) groups (A, B) juveniles; (C, D) mid-adult and (E, F) late adult. Data are plotted as mean ± SD. Statistical difference (Holm-Sidak< 0.05) in sensitivity at that frequency indicated by asterisks (*) between sound exposure and baseline, and triangles (Δ) between sound exposure and recovery.
Juvenile and mid-adult bobtail squid were also tested following a two-hour recovery period after sound exposure. There was no significant difference between the baseline and recovery auditory sensitivity curves (for both SPL and PAL) across all frequencies tested (Figures 5A–D).
Human activity, such as recreational and commercial shipping, often heavily utilizes the areas where many species of cephalopod, including the hummingbird bobtail squid (E. berryi), inhabit. In this study, auditory evoked potentials showed that E. berryi were sensitive between 100 – 1000 Hz to both particle motion and sound pressure stimuli, with greatest sensitivity observed in response to 300 – 500 Hz. This low frequency sensitivity matches the frequency range of both abiotic and biotic sound sources of shallow water marine environments (McKenna et al., 2021), as well as the low frequency range of vessel noise (Duarte et al., 2021). In this study exposure to short duration (fifteen minutes) and high intensity (150 dB re. 1 µPa sound pressure level, -8.6 dB re. 1 ms-2 particle acceleration) vessel sound was found to have significant effects on both the behaviour and auditory physiology of hummingbird bobtail squid.
For all age groups tested, auditory sensitivity was impaired following exposure to vessel sound, with a higher sound level needed to evoke a response. A change in auditory sensitivity has potential implications for cephalopods because it may reduce their ability to assess their environment, find suitable settlement habitat, avoid predators, or detect prey. Importantly, we noted that exposure to short term vessel sound caused temporary hearing loss (or temporary threshold shift – TTS) because, auditory sensitivity returned to pre-exposure baseline levels following a two-hour recovery period. The number of individuals retested following the recovery period was limited owing to the number of animals available. Yet, the data collected provides preliminary evidence that vessel sound exposure did not cause a permanent threshold shift (PTS) where sensory hair cells were damaged beyond repair. Acoustic trauma, including lesions, hair cell loss and neuron swelling, have been reported in other cephalopod species (Loligo vulgaris, Sepia officinalis, Octopus vulgaris and Illex coindetti) following two hours of sound exposure (50 – 400 Hz, 157 ± 5 dB re 1µPa, although the particle acceleration levels were not noted) (André et al., 2011). A range of exposure durations and sound exposure levels should be tested to investigate when PTS occurs in cephalopods. Fishes can repair and replace damaged hair cells following exposure to intense sounds or ototoxic drugs (Lombarte et al., 1993; Lombarte and Popper, 1994) therefore, potential regenerative mechanisms in cephalopod models also needs to be explored.
Sound exposure had a significant effect on behaviour because the relative ventilation rate of mid and late adults significantly decreased both during and post sound exposure compared to baseline rates. Such a response could be a potential defence mechanism to avoid detection by predators (Mader et al., 2010). Anti-predator defence has been identified in many animals (Sih, 1987; Kavaliers and Choleris, 2001; Alcock, 2005), including the webfoot octopus (Octopus ocellatus) that suppressed its respiration when exposed to 50, 100 and 150 Hz tones (Kaifu et al., 2007). Alternatively, startle responses have been observed in cephalopods exposed to rapid-onset air gun sounds (147 - 151 dB re 1 µPa sound exposure level) (Fewtrell and McCauley, 2012). Body pattern changes, inking, jetting and startle responses (Jones et al., 2020) and fewer prey captures (Jones et al., 2021) were also observed in longfin squid exposed to impulsive pile driving sound (190 – 194 dB re 1 µPa). Sustained reductions in feeding behaviour due to anthropogenic stressors could lead to reduced survival, especially in regions with patchy prey distributions or limited prey abundance (Jones et al., 2021). However, behavioural impacts, including alarm jetting, may be short lived and have minimal impacts on squid energetics (Cones et al., 2022). It is also important to consider the effects of noise exposure on cephalopods in the context of their natural environment. The soundscape of the Indo-Pacific, where E.berryi lives, includes sounds from fishes, marine mammals (such as the Indo-Pacific dolphin, Sousa chinensis) and anthropogenic activity. For example, a recent study found that vessel sound was present in up to 30% of recordings taken at ten shallow water locations (Xu et al., 2020). E.berryi buries in the sand during the day and emerges at night to feed. This lifestyle means that they may be exposed to anthropogenic sound regularly and repeatably throughout the daytime, compared to open water species that could leave the area during exposure. More soundscape studies are needed to confirm the sound levels that different species are exposed to as well as spatial and temporal trends in anthropogenic noise exposure. While there is some evidence of habituation to acoustic stimuli in cephalopods (Samson et al., 2016; Cones et al., 2022), most behavioural experiments on the effect of noise on aquatic life have been conducted in the laboratory where conditions can be controlled, and individuals observed. Yet, the caveats of working in a laboratory environment must be noted, as small experimental tanks have complex acoustic fields with overlapping reflection and refraction (Jézéquel et al., 2022). The sound exposure methodology used in this study was chosen to alleviate some of the complications of small tanks by placing the squid in a small arena within the experimental tank and calibrating at the location of the animal. In other studies, sound exposure chambers have been used to control the relative magnitudes of particle motion and sound pressure by placing sound projectors at either end of a steel tube (Martin and Rogers, 2008; Halvorsen et al., 2012) . It is recommended that future research efforts strive to conduct experiments in the wild because individuals may behave differently depending upon location, water temperature, physiological state, motivation, age, body size and previous exposure (Popper et al., 2022). Furthermore, cephalopods inhabit shallow waters to deep oceans (Boyle and Rodhouse, 2008), and will be subject to different sound exposure levels from anthropogenic activity depending on sound propagation properties, bolstering the need to investigate behavioural and physiological effects using field experiments at a range of locations and water depths.
To the authors’ knowledge, this is the first study that compared auditory thresholds between different ages of a cephalopod species. The different age groups behaved similarly, remaining quiescent in the daytime during the experiments and increasing activity at night or when feeding in their holding tanks. Both mid and late adults were observed to have a significant decrease in ventilation rate with anthropogenic sound exposure. In terms of AEPs, for particle acceleration there was a loss of sensitivity with age and for sound pressure juveniles and mid adults were more sensitive than late adults. To extend this study, it would be interesting to test the auditory sensitivity of recently hatched individuals (< 30 days old) and determine if they are more sensitive than juveniles. Additionally, cephalopods undergo senescence and can exhibit physiological and behavioural changes, including a loss of coordination and a reduction in feeding (Roumbedakis and Guerra, 2019) . The late-adults used in this study were 125 – 140 days old, and older individuals (up to 180 days) could be tested to examine whether further loss of auditory sensitivity occurs with age. Furthermore, microscopy techniques may be able to establish if there is evidence of degradation in the sensory hair cells of the statoliths during senescence.
The auditory sensitivity data collected on E.berryi in this study aligns with previous behavioural and physiological results that the cephalopod statocyst detects low frequency sound (Mooney et al., 2010; Samson et al., 2016. Continued research focussed on understanding how animals detect and use particle motion is critical to the future evaluation and regulation of sound in the natural environment. It is likely that substrate born vibrations, are also important to these cephalopods and certainly to other invertebrates. Addressing how these squid perceive and utilize this cue could greatly expand our understanding of their sensory ecology, and how they may be impacted by noise. Substrate-borne vibrations can travel great distances with little attenuation, allowing for potentially improved sensitivity and a wider range of noise-based impairments. In this study, frequencies< 100 Hz were not tested owing to the limitations of the use of an underwater speaker as the sound source. Yet cephalopods likely detect infrasound (< 20 Hz) (Packard et al., 1990) and given that many anthropogenic noise sources persist into these lower frequencies, squid sound sensitivity to infrasound should be examined, as particle motion at these frequencies may propagate through both the water and sediment (Popper and Hawkins, 2018).
This study expands knowledge on cephalopod hearing highlighting that exposure to short duration, high intensity anthropogenic sound can cause significant shifts in the behaviour and physiology of E.berryi. There was some evidence that the reduction in auditory sensitivity was a temporary threshold shift however, human produced sounds have become louder and more persistent and there may be limited time for these animals to recover from noise exposure. Understanding baseline sensitivity and potential physiological effects of noise on cephalopods is an important step towards establishing guidelines for management and policy to protect cephalopods from noise pollution. Auditory thresholds for marine mammals and fishes have been established and are regularly used in environmental impact assessments globally (Popper et al., 2014; National Marine Fisheries Service, 2018) . Now, guidelines based on both sound pressure and particle motion are needed to support the inclusion of cephalopods within management and policy because anthropogenic activities and associated sound levels in the ocean are increasing, while the role sound plays in cephalopod life history is only just beginning to be understood.
The original contributions presented in the study are included in the article/Supplementary Material. Further inquiries can be directed to the corresponding author.
The animal study was reviewed and approved by Institutional Animal Care and Use Committee at the Marine Biological Laboratory, Woods Hole, MA, USA.
RP: Conceptualization, Methodology, Formal Analysis, Visualization, Writing - original draft. TM: Conceptualization, Methodology, Writing - review and editing. AM: Conceptualization, Resources, Writing - review and editing. All authors contributed to the article and approved the submitted version.
This research and publication were funded in part by the Kavli Institution and The Grass Foundation, via a Grass Fellowship awarded to RP.
Special thanks to Dr Catherine Carr, Dr Elva Diaz, Dr Laura Cocas and Dr Angie Michaiel for their support during this research. We would also like to thank the Marine Biological Laboratory’s Cephalopod Culture Program and all the Marine Resources Centre staff, especially Daniel Calzarette, Bret Grasse, Taylor Sakmar and Miranda Vogt for providing cephalopod care, and Lisa Abbo for veterinary advice on the project. We would like to dedicate this manuscript to the memory of David Remsen, director of the Marine Resources Centre.
The authors declare that the research was conducted in the absence of any commercial or financial relationships that could be construed as a potential conflict of interest.
All claims expressed in this article are solely those of the authors and do not necessarily represent those of their affiliated organizations, or those of the publisher, the editors and the reviewers. Any product that may be evaluated in this article, or claim that may be made by its manufacturer, is not guaranteed or endorsed by the publisher.
The Supplementary Material for this article can be found online at: https://www.frontiersin.org/articles/10.3389/fmars.2023.1151605/full#supplementary-material
Alcock J. (2005). Animal behaviour: An evolutionary approach. 8th ed (Sunderland, MA, USA: Sinauer Associates).
André M., Solé M., Lenoir M., Durfort M., Quero C., Mas A., et al. (2011). Low-frequency sounds induce acoustic trauma in cephalopods. Front. Ecol. Environ. 9, 489–493. doi: 10.1890/100124
Bhandiwad A. A., Whitchurch E. A., Colleye O., Zeddies D. G., Sisneros J. A. (2017). Seasonal plasticity of auditory saccular sensitivity in “sneaker” type II male plainfin midshipman fish, porichthys notatus. J. Comp. Physiol. A 203, 211–222. doi: 10.1007/s00359-017-1157-9
Boyle P., Rodhouse P. (2008). “Population ecology,” in Cephalopods: Ecology and fisheries (Oxford, UK: Blackwell Publishing), 205–222.
Budelmann B. U. (1990). “The statocyst of squid,” in Squid as experimental animals. Eds. Gilbert D. L., Adelman W. J., Arnold J. M. (London: Plenum Press), 421–439.
Budelmann B. U. (1992). “Hearing in non-arthropod invertebrates,” in The evolutionary biology of hearing. Eds. Webster D. B., Fay R. A., Popper A. N. (New York: Springer Verlag).
Burkhard R. F., Eggermont J. J., Don M. (2007). Auditory evoked potentials: Basic principles and clinical applications (Philadephia: Lippincott, Williams and Wilkins).
Cones S. F., Jézéquel Y., Ferguson S., Aoki N., Mooney T. A. (2022). Pile driving noise induces transient gait disruptions in the longfin squid (Doryteuthis pealeii). Front. Mar. Sci. 9. doi: 10.3389/fmars.2022.1070290
de Soto N. A., Kight C. (2016). “Physiological effects of noise on aquatic animals,” in Stressors in the marine environment (Oxford, UK: Oxford University Press), 135–158.
Duarte C. M., Chapuis L., Collin S. P., Costa D. P., Devassy R. P., Eguiluz V. M., et al. (2021). The soundscape of the anthropocene ocean. Science 371, eaba4658. doi: 10.1126/science.aba4658
Egner S. A., Mann D. A. (2005). Auditory sensitivity of sergeant major damselfish abudefduf saxatilis from post-settlement juvenile to adult. Mar. Ecol. Prog. Ser. 285, 213–222. doi: 10.3354/meps285213
Erbe C., Dunlop R., Dolman S. (2018). “Effects of noise on marine mammals,” in Effects of anthropogenic noise on animals. Eds. Slabbekoorn H., Dooling R. J., Popper A. N., Fay R. R. (New York, NY: Springer New York), 277–309. doi: 10.1007/978-1-4939-8574-6_10
Fewtrell J. L., McCauley R. D. (2012). Impact of air gun noise on the behaviour of marine fish and squid. Mar. pollut. Bull. 64, 984–993. doi: 10.1016/j.marpolbul.2012.02.009
Fiorito G., Affuso A., Anderson D. B., Basil J., Bonnaud L., Botta G., et al. (2014). Cephalopods in neuroscience: regulations, research and the 3Rs. Invertebrate Neurosci. 14, 13–36. doi: 10.1007/s10158-013-0165-x
Fiorito G., Affuso A., Basil J., Cole A., de Girolamo P., D’Angelo L., et al. (2015). Guidelines for the care and welfare of cephalopods in research –a consensus based on an initiative by CephRes, FELASA and the Boyd group. Lab. Anim. 49, 1–90. doi: 10.1177/0023677215580006
Halvorsen M. B., Casper B. M., Woodley C. M., Carlson T. J., Popper A. N. (2012). Threshold for onset of injury in Chinook salmon from exposure to impulsive pile driving sounds. PloS One 7, e38968. doi: 10.1371/journal.pone.0038968
Hanlon R. T., Hixon R. F., Hulet W. H. (1983). Survival, growth, and behavior of the loligind squids (Loligo pealei, lolliguncula brevis) (Mollusca: cephalopoda) in closed sea water systems. Biol. Bull. 165, 637–685. doi: 10.2307/1541470
Hawkins A. D., Pembroke A. E., Popper A. N. (2015). Information gaps in understanding the effects of noise on fishes and invertebrates. Rev. Fish Biol. Fish 25, 39–64. doi: 10.1007/s11160-014-9369-3
Higgs D. M., Radford C. A. (2016). “The potential overlapping roles of the ear and lateral line in driving a’coustic’ responses,” in Fish hearing and bioacoustics: An anthology in honor of Arthur n. popper and Richard r. fay. Ed. Sisneros J. A. (Cham: Springer International Publishing), 255–270.
Hu M. Y., Yan H. Y., Chung W.-S., Shiao J.-C., Hwang P.-P. (2009). Acoustically evoked potentials in two cephalopods inferred using the auditory brainstem response (ABR) approach. Comp. Biochem. Physiol. A Mol. Integr. Physiol. 153, 278–283. doi: 10.1016/j.cbpa.2009.02.040
Jézéquel Y., Bonnel J., Aoki N., Mooney T. A. (2022). Tank acoustics substantially distort broadband sounds produced by marine crustaceans. J. Acoust. Soc. Am. 152, 3747–3755. doi: 10.1121/10.0016613
Jones I. T., Peyla J. F., Clark H., Song Z., Stanley J. A., Mooney T. A. (2021). Changes in feeding behavior of longfin squid (Doryteuthis pealeii) during laboratory exposure to pile driving noise. Mar. Environ. Res. 165, 105250. doi: 10.1016/j.marenvres.2020.105250
Jones I. T., Stanley J. A., Mooney T. A. (2020). Impulsive pile driving noise elicits alarm responses in squid (Doryteuthis pealeii). Mar. pollut. Bull. 150, 110792. doi: 10.1016/j.marpolbul.2019.110792
Kaifu K., Segawa S., Tsuchiya K. (2007). Behavioral responses to underwater sound in the small benthic octopus octopus ocellatus. J. Mar. Acoust. Soc. Jpn. 34, 266–273. doi: 10.3135/jmasj.34.266
Kavaliers M., Choleris E. (2001). Antipredator responses and defensive behavior: ecological and ethological approaches for the neurosciences. Neurosci. Biobehav. Rev. 25, 577–586. doi: 10.1016/S0149-7634(01)00042-2
Ladich F., Fay R. R. (2013). Auditory evoked potential audiometry in fish. Rev. Fish Biol. Fish 23, 317–364. doi: 10.1007/s11160-012-9297-z
Lombarte A., Popper A. N. (1994). Quantitative analyses of postembryonic hair cell addition in the otolithic endorgans of the inner ear of the european hake, merluccius merluccius (gadiformes, teleostei). J. Comp. Neurol. 345, 419–428. doi: 10.1002/cne.903450308
Lombarte A., Yan H. Y., Popper A. N., Chang J. S., Platt C. (1993). Damage and regeneration of hair cell ciliary bundles in a fish ear following treatment with gentamicin. Hear. Res. 64, 166–174. doi: 10.1016/0378-5955(93)90002-I
Lopes V. M., Sampaio E., Roumbedakis K., Tanaka N. K., Carulla L., Gambús G., et al. (2017). Cephalopod biology and care, a COST FA1301 (CephsInAction) training school: anaesthesia and scientific procedures. Invertebrate Neurosci. 17, 8. doi: 10.1007/s10158-017-0200-4
Mackiewicz A. G., Putland R. L., Mensinger A. F. (2021). Effects of vessel sound on oyster toadfish opsanus tau calling behavior. Mar. Ecol. Prog. Ser. 662, 115–124. doi: 10.3354/meps13634
Mader T. L., Johnson L. J., Gaughan J. B. (2010). A comprehensive index for assessing environmental stress in animals1. J. Anim. Sci. 88, 2153–2165. doi: 10.2527/jas.2009-2586
Martin J. S., Rogers P. H. (2008). Sound exposure chamber for assessing the effects of high-intensity sound on fish. Bioacoustics 17, 331–333. doi: 10.1080/09524622.2008.9753866
Maruska K. P., Boyle K. S., Dewan L. R., Tricas T. C. (2007). Sound production and spectral hearing sensitivity in the Hawaiian sergeant damselfish, abudefduf abdominalis. J. Exp. Biol. 210, 3990–4004. doi: 10.1242/jeb.004390
McKenna M. F., Wiggins S. M., and Hildebrand J. A (2013). Relationship between container ship underwater noise levels and ship design, operational and oceanographic conditions. Sci. Rep. 3, 1760. doi: 10.1038/srep01760
McKenna M. F., Baumann-Pickering S., Kok A. C. M., Oestreich W. K., Adams J. D., Barkowski J., et al. (2021). Advancing the interpretation of shallow water marine soundscapes. Front. Mar. Sci. 8. doi: 10.3389/fmars.2021.719258
Messenger J. B., Nixon M., Ryan K. P. (1985). Magnesium chloride as an anaesthetic for cephalopods. Comp. Biochem. Physiol. Part C.: Comp. Pharmacol. 82, 203–205. doi: 10.1016/0742-8413(85)90230-0
Montgomery J. C., Jeffs A., Simpson S. D., Meekan M., Tindle C. (2006). Sound as an orientation cue for the pelagic larvae of reef fishes and decapod crustaceans. Adv. Marine Biol. 51, 143–196. doi: 10.1016/S0065-2881(06)51003-X
Mooney T. A., Hanlon R. T., Christensen-Dalsgaard J., Madsen P. T., Ketten D. R., Nachtigall P. E. (2010). Sound detection by the longfin squid (Loligo pealeii) studied with auditory evoked potentials: sensitivity to low-frequency particle motion and not pressure. J. Exp. Biol. 213, 3748–3759. doi: 10.1242/jeb.048348
Mooney T. A., Samson J. E., Schlunk A. D., Zacarias S. (2016). Loudness-dependent behavioral responses and habituation to sound by the longfin squid (Doryteuthis pealeii). J. Comp. Physiol. A 202, 489–501. doi: 10.1007/s00359-016-1092-1
Myrberg A. A. (1990). The effects of man-made noise on the behavior of marine animals. Environ. Int. 16, 575–586. doi: 10.1016/0160-4120(90)90028-5
National Marine Fisheries Service (2018). 2018 revision to: Technical guidance for assessing the effects of anthropogenic sound on marine mammal hearing (Version 2.0) - underwater thresholds for onset of permanent and temporary threshold shifts. (NOAA Technical Memorandum NMFS-OPR-59, National Oceanic and Atmospheric Administration (NOAA), US Department of Commerce)
Nedelec S. L., Campbell J., Radford A. N., Simpson S. D., Merchant N. D. (2016). Particle motion: the missing link in underwater acoustic ecology. Methods Ecol. Evol. 7, 836–842. doi: 10.1111/2041-210X.12544
Nissen A. C., Vetter B. J., Rogers L. S., Mensinger A. F. (2019). Impacts of broadband sound on silver (Hypophthalmichthys molitrix) and bighead (H. nobilis) carp hearing thresholds determined using auditory evoked potential audiometry. Fish Physiol. Biochem. 45, 1683–1695. doi: 10.1007/s10695-019-00657-y
Offutt G. C. (1970). Acoustic stimulus perception by the American lobsterHomarus americanus (Decapoda). Experientia 26, 1276–1278. doi: 10.1007/BF01898016
Packard A., Karlsen H. E., Sand O. (1990). Low frequency hearing in cephalopods. J. Comp. Physiol. A 166, 501–505. doi: 10.1007/BF00192020
Popper A. N., Hawkins A. D. (2018). The importance of particle motion to fishes and invertebrates. J. Acoust. Soc. Am. 143, 470–488. doi: 10.1121/1.5021594
Popper A. N., Hawkins A. D., Fay R. R., Mann D. A., Bartol S., Carlson T. J., et al. (2014). ASA S3/SC1.4 TR-2014 sound exposure guidelines for fishes and Sea turtles: A technical report prepared by ANSI-accredited standards committee S3/SC1 and registered with ANSI (Cham: Springer International Publishing). doi: 10.1007/978-3-319-06659-2
Popper A. N., Hice-Dunton L., Jenkins E., Higgs D. M., Krebs J., Mooney A., et al. (2022). Offshore wind energy development: Research priorities for sound and vibration effects on fishes and aquatic invertebrates. J. Acoust. Soc. Am. 151, 205–215. doi: 10.1121/10.0009237
Preuss T., Budelmann B. U. (1995). Proprioceptive hair cells on the neck of the squid lolliguncula brevis: a sense organ in cephalopods for the control of head-to-body position. Philos. Trans. R. Soc. Lond. B. Biol. Sci. 349, 153–178. doi: 10.1098/rstb.1995.0101
Roumbedakis K., Guerra Á. (2019). “Cephalopod senescence and parasitology,” in Handbook of pathogens and diseases in cephalopods. Eds. Gestal C., Pascual S., Guerra Á., Fiorito G., Vieites J. M. (Cham: Springer International Publishing), 207–211. doi: 10.1007/978-3-030-11330-8_16
Samson J. E., Mooney T. A., Gussekloo S. W. S., Hanlon R. T. (2016). “A brief review of cephalopod behavioral responses to sound,” in The effects of noise on aquatic life II. Eds. Popper A. N., Hawkins A. (New York, NY: Springer New York), 969–975.
Shannon G., McKenna M. F., Angeloni L. M., Crooks K. R., Fristrup K. M., Brown E., et al. (2016). A synthesis of two decades of research documenting the effects of noise on wildlife. Biol. Rev. 91, 982–1005. doi: 10.1111/brv.12207
Sih A. (1987). “Predators and prey lifestyles: an evolutionary and ecological overview,” in Predation direct and indirect impacts on aquatic communities. Eds. Kerfoot W. C., Sih A. (Hanover and London: University Press of New England), 203–224.
Sisneros J. A., Popper A. N., Hawkins A. D., Fay R. R. (2016). “Auditory evoked potential audiograms compared with behavioral audiograms in aquatic animals,” in The effects of noise on aquatic life II. Eds. Popper A. N., Hawkins A. (New York, NY: Springer New York), 1049–1056.
Stanley J. A., Radford C. A., Jeffs A. G. (2012). Location, location, location: finding a suitable home among the noise. Proc. R. Soc. B.: Biol. Sci. 279, 3622–3631. doi: 10.1098/rspb.2012.0697
Tyack P. L., Zimmer W. M. X., Moretti D., Southall B. L., Claridge D. E., Durban J. W., et al. (2011). Beaked whales respond to simulated and actual navy sonar. PloS One 6, e17009. doi: 10.1371/journal.pone.0017009
Vetter B. J., Brey M. K., Mensinger A. F. (2018). Reexamining the frequency range of hearing in silver (Hypophthalmichthys molitrix) and bighead (H. nobilis) carp. PloS One 13, e0192561. doi: 10.1371/journal.pone.0192561
Vetter B. J., Cupp A. R., Fredricks K. T., Gaikowski M. P., Mensinger A. F. (2015). Acoustical deterrence of silver carp (Hypophthalmichthys molitrix). Biol. Invasions 17, 3383–3392. doi: 10.1007/s10530-015-0964-6
Williams R., Wright A. J., Ashe E., Blight L. K., Bruintjes R., Canessa R., et al. (2015). Impacts of anthropogenic noise on marine life: Publication patterns, new discoveries, and future directions in research and management. Ocean Coast. Manag. 115, 17–24. doi: 10.1016/j.ocecoaman.2015.05.021
Williamson R. (1995). “The statocysts of cephalopods,” in Cephalopod neurobiology: neuroscience studies in squid, octopus and cuttlefish. Eds. Abbott N. J., Williamson R., Maddock L. (Oxford: Oxford University Press).
Keywords: cephalopods, hearing, underwater sound, noise pollution, threshold shift, Euprymna berryi
Citation: Putland RL, Mooney TA and Mensinger AF (2023) Vessel sound causes hearing loss for hummingbird bobtail squid (Euprymna berryi). Front. Mar. Sci. 10:1151605. doi: 10.3389/fmars.2023.1151605
Received: 26 January 2023; Accepted: 16 February 2023;
Published: 28 February 2023.
Edited by:
Roger Villanueva, Institute of Marine Sciences (CSIC), SpainReviewed by:
Michael L. Fine, Virginia Commonwealth University, United StatesCopyright © 2023 Putland, Mooney and Mensinger. This is an open-access article distributed under the terms of the Creative Commons Attribution License (CC BY). The use, distribution or reproduction in other forums is permitted, provided the original author(s) and the copyright owner(s) are credited and that the original publication in this journal is cited, in accordance with accepted academic practice. No use, distribution or reproduction is permitted which does not comply with these terms.
*Correspondence: Rosalyn L. Putland, cm9zYWx5bi5wdXRsYW5kQGNlZmFzLmdvdi51aw==
Disclaimer: All claims expressed in this article are solely those of the authors and do not necessarily represent those of their affiliated organizations, or those of the publisher, the editors and the reviewers. Any product that may be evaluated in this article or claim that may be made by its manufacturer is not guaranteed or endorsed by the publisher.
Research integrity at Frontiers
Learn more about the work of our research integrity team to safeguard the quality of each article we publish.