- 1Pathogen Transmission and Disease Research Group, Institute of Marine Research, Bergen, Norway
- 2Fish Heath Research Group, Norwegian Veterinary Institute, Ås, Norway
Piscine orthoreovirus -1 (PRV-1) causes the disease heart and skeletal muscle inflammation (HSMI) in farmed Atlantic salmon, and the virus has been detected in wild anadromous Atlantic salmon and brown trout. However, the infection prevalence, viral kinetics, and disease severity in different life stages of Atlantic salmon and brown trout are unknown. The current study aimed to evaluate and compare susceptibility to PRV-1 infection and development of HSMI in different life stages of anadromous Atlantic salmon (Salmo salar) and brown trout (Salmo trutta). We challenged Atlantic salmon and brown trout fry, parr, and post-smolts with PRV-1 by bath, cohabitation, or IP injection. The kinetics of viral infection and disease development were evaluated by RT-qPCR, in situ hybridization, and histology. Our results indicated that PRV-1 infection prevalence and viral kinetics depend on the developmental stage and challenge method in both Atlantic salmon and brown trout. All developmental stages of Atlantic salmon and brown trout can be infected with PRV-1. However, brown trout showed a lower infection prevalence, with positive cases exhibiting only mild infections without any pathological changes in the target organs, while all life stages of Atlantic salmon developed heart lesions characteristic of HSMI. These results strongly suggest that brown trout are less susceptible to PRV-1 infection than Atlantic salmon and further confirm the species-specific susceptibility and disease development for PRV-1 infection.
1 Introduction
Heart and skeletal muscle inflammation (HSMI) is caused by piscine orthoreovirus (PRV) subtype 1, a species of the genus Orthoreovirus (Wessel et al., 2017). PRV is a non-enveloped, icosahedral virus with an outer and inner capsid layer of 70-80 nm size (Finstad et al., 2014). It is a double-stranded RNA virus with 10 genomic dsRNA segments classified based on the size of the segments (Markussen et al., 2013). HSMI was first reported in farmed Atlantic salmon in Norway in 1999 (Kongtorp et al., 2004a). Since then, the number of outbreaks has risen markedly. In 2021, 188 cases were reported from Atlantic salmon aquaculture farms all along the Norwegian coast (Sommerset et al., 2021). HSMI was listed as a notifiable disease in the Norwegian national list of fish diseases in 2008. However, due to the ubiquitous presence of the virus and many subclinical cases, PRV was removed from the notifiable list in 2014. This caused a subsequent drop in the number of registered cases. Recent data indicates that HSMI outbreaks are a frequent occurrence in Norwegian fish farms (Sommerset et al., 2021).
Different PRV subtypes/genotypes cause species-specific diseases in salmonids. PRV-1 causes HSMI in Atlantic salmon (Wessel et al., 2017), while PRV-2 causes erythrocytic inclusion body syndrome (EIBS) in Japanese coho salmon (Onchorhynchus kisutchi) (Takano et al., 2016), and PRV-3 causes HSMI-like-disease in rainbow trout (Onchorhynchus mykiss) (Vendramin et al., 2019). Differences in virulence have also been observed within PRV-1 genetic variants. The PRV-1a genotype, considered the ancestral strain, causes only mild heart lesions in Atlantic salmon. Whereas PRV-1b is the genotype mainly associated with HSMI outbreaks in farmed Atlantic salmon in Norway (Wessel et al., 2020).
HSMI is mainly observed during Atlantic salmon grow-out in sea cages. Only a few cases of disease have been reported from broodstock farms and hatcheries (Sommerset et al., 2020), but asymptomatic PRV infection is common. Horizontal transmission is the major mode of spread between cages and farms, and important risk factors for the transmission of HSMI includes previous disease outbreak in the farm and the geographical distance between adjacent farms (Aldrin et al., 2010). Diseased fish show clinical signs like anorexia, lethargy, abnormal swimming, increase in mortality and the gross pathology includes a pale heart, pale or yellowish liver, swollen spleen, and loose muscular layer (Kongtorp et al., 2004b). PRV-1 infects and replicates within viral factories in the cytoplasm of red blood cells (Haatveit et al., 2016), which causes a transient reduction in hemoglobin levels. However, hemolytic anaemia is not observed in PRV-1 infected Atlantic salmon (Lund et al., 2017). Erythrocytes are the primary site of replication during the early phase of infection beforeits spread and infection of the heart, skeletal muscle, and other organs (Wessel et al., 2015). The classical histopathological changes include epicarditis, myocarditis in spongy and compact myocardium of heart ventricle, extensive infiltration of mononuclear inflammatory cells and necrosis of red skeletal muscle. Severely infected fish suffer circulatory disturbances and respiratory impairment leading to heart failure and death (Kongtorp et al., 2004b).
HSMI outbreaks have also been reported in Atlantic salmon hatcheries and broodfish facilities in Norway (Sommerset et al., 2021). Despite high viral loads and the presence of histopathological changes in PRV-1 infected hearts, morbidity and mortality are not common in Atlantic salmon pre-smolts (Lovoll et al., 2012). After seawater transfer, clinical HSMI manifests, correlating with increased viral load, high morbidity, and mortality of up to 20% (Kongtorp et al., 2004b). PRV-1 infection has been shown to cause disease after experimental infection in freshwater (Kongtorp et al., 2004b). Atlantic salmon in the freshwater stage are susceptible to various viruses like infectious pancreatic necrosis virus (IPNV), salmon gill poxvirus, salmonid alphavirus (SAV), piscine myocarditis virus (PMCV) and infectious salmon anaemia virus (ISAV) (Raynard et al., 2001; Bratland and Nylund, 2009; Wiik-Nielsen et al., 2012; Mutoloki et al., 2016; Gjessing et al., 2017). Infections contracted in freshwater stages could contribute to or cause viral disease outbreaks in seawater. Hence, the production of robust, healthy, and pathogen-free smolts are pre-requisites for preventing disease outbreaks during the seawater phase. Prevention of virus entry through strict biosecurity and sanitary measures is one of the main strategies for hatcheries to produce healthy fish.
Salmon post-smolts are vulnerable to many infectious diseases when they are transferred to seawater owing to significant biological, management, and environmental stress factors such as smoltification, sea lice treatment, handling, and transport. A multitude of predisposing risk factors such as proximity to other infected farms, increased cohort lifespan at sea, and previous HSMI outbreaks in the farming environment, also contribute to the increased infection pressure, disease development, morbidity, and mortality (Kristoffersen et al., 2013). Many viral disease outbreaks and the onset of clinical signs typically occur during the first year after seawater transfer. Longitudinal studies in Atlantic salmon farms have shown that HSMI can occur as either a clinical or subclinical outbreak as early as 14 days after seawater transfer, and can last for up to 4 months (Kongtorp et al., 2006). As horizontal transmission is the main route for the spread of PRV-1, viral transmission can occur between farmed and wild salmon, and vice versa. PRV-1 has been detected in wild Atlantic salmon and returning anadromous brown trout in Norwegian rivers (Garseth et al., 2013a; Garseth et al., 2013b). PRV-1 has also been detected in brown trout caught from farming and non-farming regions in Norway (Madhun et al., 2016). Interestingly, PRV infection has not been detected in non-migrating landlocked wild Atlantic salmon and brown trout (Garseth and Biering, 2018). PRV infection has been associated with other diseases such as proliferative darkening syndrome (PDS) in brown trout (Kuehn et al., 2018) and jaundice syndrome in chinook salmon (Di Cicco et al., 2018). Disease outbreaks in farmed Atlantic salmon can lead to elevated infection pressure, mediate pathogen/disease transmission to other susceptible salmonid populations in the wild (Mordecai et al., 2021).
Virus transmission is a complex process that depends on several virus, host, and environmental factors. Once the virus successfully enters the permissive cells, it may replicate and spread to other cells. In resistant fish, strong immune responses can halt or suppress virus replication and prevent the spread of infection. Conversely, susceptible fish may mount a weaker or slower immune response, allowing the virus to replicate and spread, eventually leading to clinical disease (Louten, 2016). Thus, a better understanding of the survival, transmission, and virulence of viruses in all life stages is critical for assessment of their inherent risk for negative impacts for both farmed and wild salmonids, as well as to guide the development of preventive measures.
In the present study, we report on PRV-1 infection and disease dynamics in fry and parr in freshwater and post-smolts in seawater, for both Atlantic salmon and brown trout. We have compared bath and cohabitation challenge methods and compared the differences in growth rate, viral load, infection dynamics, mortality, and histological changes during the experimental period. Our results provide valuable insight into the susceptibility to and consequences of PRV-1 infection in different life stages of Atlantic salmon and brown trout.
2 Materials and methods
2.1 Fish
Both Atlantic salmon (Salmo salar) and brown trout (Salmo trutta) used in the experiment were hatched and reared following standard protocols at the Institute of Marine Research (IMR) Matre, Western Norway. Prior to the experimental disease challenges, the fish were transferred to IMR’s disease challenge facility in Bergen, Western Norway. The Atlantic salmon broodfish originated from wild Atlantic salmon from the river Etne, and the trout originated from anadromous wild brown trout (sea trout) caught in the river Matre. Salmon and trout used in Experiment 1 and 2 were from the same production batches, whereas the post-smolt used in Experiment 3 were from a different production batch. An overview of experimental groups is given in Table 1. Prior to the start of experiments, both salmon and trout were tested for infections by TaqMan RT-qPCR assay and found negative for the presence of salmonid alphavirus (SAV) (Hodneland and Endresen, 2006), infectious pancreatic necrosis virus (IPNV) (Nylund et al., 2011, piscine myocarditis virus (PMCV) (Lovoll et al., 2010), and piscine orthoreovirus-1 (PRV-1) (Wessel et al., 2015). Fish were anaesthetized using 60 mg/L Benzocaine (Apotekproduksjon AS) before handling and euthanized with an overdose of Benzocaine (160 mg/L) before sampling. All the experimental procedures were reviewed and approved by the Norwegian animal research authority prior to start of challenge experiments (FOTS ID: 15111 and 11260).
2.2 Experimental setup and sampling
2.2.1 Experiment 1: Fry
The salmon and trout fry, and the salmon parr used as shedder fish, were transported to Bergen from Matre and acclimatized in freshwater for 3 weeks prior to the start of challenge experiment. Shedders were fed twice daily, with Nutra Olympic (Skretting), and the salmon and trout fry were fed four times a day with Nutra Sprint (Skretting). At the start of the challenge, the average weight of the salmon shedder fish was 17.95 ± 2.87 g (mean ± SD). The salmon and trout fry were 0.38 ± 0.07 g and 0.54 ± 0.23 g, respectively. The water temperature ranged from 10°C to 12°C during the experimental period. The fish were reared under continuous light throughout the experimental period.
Salmon and trout fry were infected with PRV-1 by bath and modified cohabitation challenge (Figure 1). The virus inoculum for the challenge originated from the salmon shedders which were intraperitoneal (i.p.) injected with 50 µl PRV-1 infectious material (Ct 18.0 diluted 1:5), four weeks prior to the start of virus challenge. The virus inoculum for the i.p. challenge was prepared from a PRV-1 infected blood cell pellet (PRV-1b isolate NOR2012-V3621) as previously described (Wessel et al., 2017). PRV-1 infection in the shedders was confirmed one week prior to the fry challenge by qPCR-analysis, giving an average Ct-value in heart and spleen of the tested shedders, of 20.2 and 18.9, respectively.
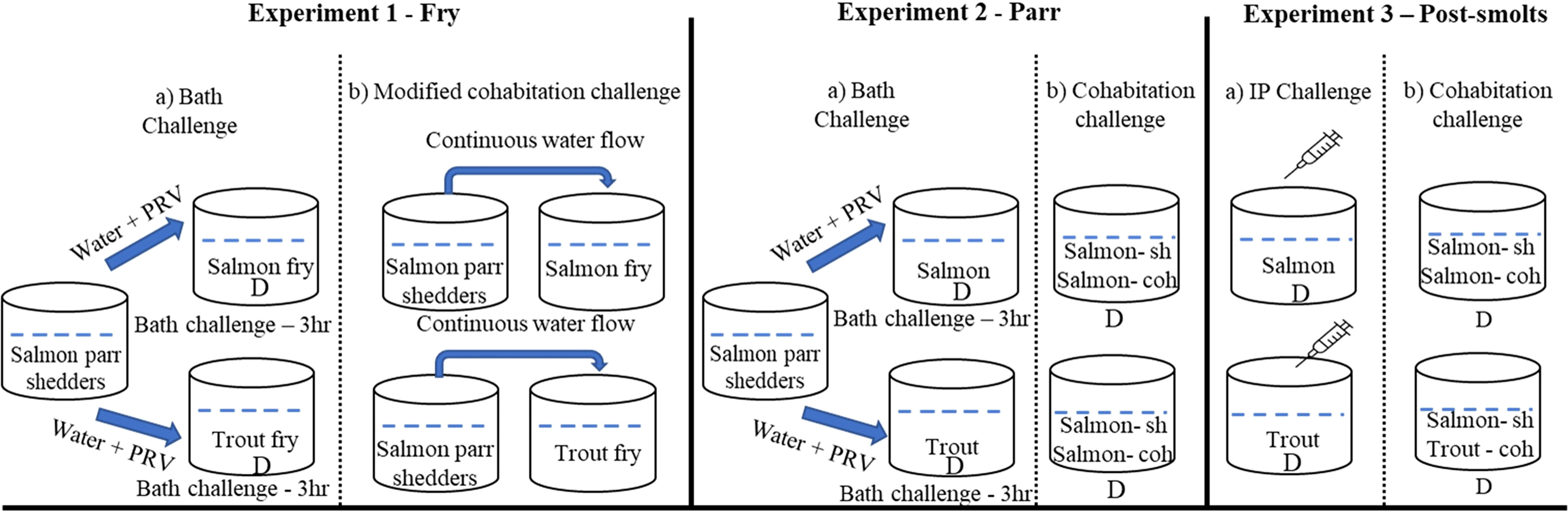
Figure 1 Experimental setup and challenge methods used in the study. *D denotes tanks in duplicates.
Bath challenge: On the day of challenge (0 wpi) water flow in the shedder fish (n=200) tank was stopped for four hours to increase the number of viral particles in the water. The water was aerated continuously and oxygen levels in the water were monitored to ensure fish welfare. After 4 hours, 30 L virus containing water from the shedder tank was transferred to two different basins, one for salmon and one for trout. Salmon (n=440) and trout (n=440). The fry was then transferred into the virus containing water and exposed for a period of 3 hours. The water was aerated during the entire 3-hour challenge period, and oxygen levels in the water were monitored. After incubation, the bath-challenged fry was divided into 2 separate 250 L experimental tanks per fish species, with either 220 salmon fry or 220 trout fry in each tank (stocking density - 0.3344 kg m-3).
Modified cohabitation challenge: The size difference between shedders (17.95 ± 2.87 g) and fry (0.38 ± 0.07 g) was large. Hence, to avoid predation and loss of naïve fry due to cannibalism, the cohabitation method was modified. Our previous experience has shown that using net cages inside the shedder fish tanks adversely affects the water flow and the welfare of shedder fish. Thus, we decided not to have the shedders and fry in the same tank as in a usual cohabitation challenge model. A modified cohabitation model was therefore used, with shedders and fry kept in different tanks, and water pumped from the shedder tanks to the fry tanks. One shedder tank was supplying one fry tank. The 200 shedders used in the bath challenge were distributed into two 250 L tanks (n=100). Salmon (n=440) and trout (n=440) fry were transferred into two 250 L tanks adjacent to the shedder tanks, one tank for each species. Throughout the experimental period, inflow for the shedder tank was set to approximately 750 L h-1, and the water flow from the shedder tanks into the fry tanks was approximately 350 L h-1.
From each tank, at each sampling time-point, 8 fish were sampled for qPCR and 8 for histology. The “organ package” containing all the internal organs cut from esophagus to anus were used for molecular analysis and whole fish were formalin fixed for histopathological analyses for all groups at 0-, 2-, 5-, 7-, 9- and 12-week post infection (wpi).
2.2.2 Experiment 2: Parr
The salmon parr to be used as shedder fish and the salmon and trout parr were transported from Matre to Bergen and acclimatized for 4 weeks until start of the challenge. The fish were fed four times a day with Nutra Olympic (Skretting). At the start of the challenge, the average weight of the salmon and trout parr were 7.2 ± 2.4 g and 8.6 ± 2.0 g, respectively. The water temperature varied from approximately 13°C in August, to 14°C in September and 11°C in October. The fish were maintained on a 12:12hr light dark regime throughout the experimental period.
Like experiment 1, the virus inoculum for both bath and cohabitation challenge originated from the salmon shedders which were i.p. injected with 50µl of the same PRV-1b infectious material as used in experiment 1, four weeks before the start of the parr challenge. PRV-1 infection in the shedders was confirmed one week prior to the parr challenge by qPCR analysis. The average Ct-value in blood and spleen of the tested shedders was 11.8 and 17.3, respectively.
Bath challenge: On the day of challenge, the water flow in the shedder fish tank was stopped for 3 hours. The water was aerated and oxygen levels in the water were monitored to ensure fish welfare. Then, 30 L of water from the shedder tank was transferred into each of four smaller basins. 140 trout were then added into the first two basins (70 fish per basin), and 140 salmon were added into the remaining two (also 70 fish per basin). The fish were exposed to PRV-1-containing water for 3 hours. The water was aerated during the bath challenge and the oxygen levels in the water were monitored. Following exposure, the fish were transferred into four 250 L tanks (stocking density – 2 kg m-3) for the remainder of the experimental period.
Cohabitation challenge: The experiment was set up in four tanks by transferring 30 salmon shedders (at 4 wpi) into four different 250 L experimental tanks, followed by transfer of 70 trout into two of these tanks and 70 salmon to the last two cohabitant challenge tanks. Shedder salmon were adipose fin clipped to aid identification during the sampling. Eight fish from each tank were sampled for qPCR/histology. Sampling of spleen for qPCR and heart for histopathological analyses were carried out for all groups at 0-, 2-, 5-, 7-, 9- and 12-wpi.
2.2.3 Experiment 3: Post-smolt
Pre-smolts were transported from Matre to Bergen and acclimatized in freshwater for three weeks before the challenge. The fish were subjected to continuous light (24:0 L:D) and smoltification was completed by gradually increasing the water salinity to 30 ppt over a period of two weeks. The fish were fed once daily with Feed Supreme (Skretting). At the start of the experiment, the average weight of the salmon and trout were 53.2 ± 10.2 g and 47.0 ± 7.4 g, respectively. The water temperature varied between 10-12°C throughout the experiment. After smoltification, the fish were maintained on a 12/12 light dark regime throughout the experimental period.
The i.p. challenge for the experimental groups of both salmon and trout was set up using the same procedure as previously described for creating the salmon shedder group for the cohabitation experiment. Briefly, 80 salmon and 80 trout were i.p. injected with 50 µl of the PRV-1 infectious material. The fish were then transferred into four different 250 L tanks, two with trout (n=40) and two with salmon (n=40) (stocking density – 8.5 kg m-3).
The cohabitation challenge was set up by transferring infected salmon shedders (at 4 wpi) into each of four tanks (n=30), and then adding trout to two of the tanks (n=30), and naïve salmon to the last two (n=30). Shedder salmon were adipose fin clipped to further aid identification of shedders/species during the experiment. Eight fish from each tank were sampled for qPCR/histology. Sampling of spleen for qPCR and heart for histopathological analyses were carried out for all groups at 0-, 2-, 4-, 8- and 10-wpi.
2.3 RNA extraction and RT-qPCR
Total RNA was extracted from spleen tissue (parr and post smolt) and the organ package (fry) with Promega Reliaprep simplyRNA HT 384 (Nerliens) on a Biomek 4000 Laboratory Automated Workstation (Beckman Coulter) according to the manufacturer’s instructions and quantified using a NanoDrop™-1000 spectrophotometer (Thermo Fisher Scientific). The RNA samples were normalized to a concentration of 50 ng/µL using the Biomek 4000 Laboratory Automated Workstation (Beckman Coulter).
The quantitative real-time RT-qPCR was conducted using the assay designed for targeting the PRV-1 segment S1 gene (Fwd TGCGTCCTGCGTATGGCACC, Rev GGCTGGCATGCCCGAATAGCA and Probe FAM-ATCACAACGCCTACCT–MGBNFQ) (primer efficiency – 97.4%) (Wessel et al., 2015). The sample and standard mixture was prepared using AgPath-ID One Step RT-PCR reagents (Thermo Fisher Scientific) according to the manufacturer’s instructions with 4 µL of diluted RNA at a total of 200ng in a reaction mix containing 400 nM of forward primer, 400 nM of reverse primer, and 160 nM of probe in a total volume of 10 µl on a 384 well-plate. The Atlantic salmon elongation factor α 1 gene (ELF) (Fwd CCCCTCCAGGACGTTTACAAA, Rev CACACGGCCCACAGGTACA and Probe FAM-ATCGGTGGTATTGGAAC–MGBNFQ) (primer efficiency - 100%) was used as endogenous control (Olsvik et al., 2005). For the qPCR assay, amplification and fluorescence detection were performed by a QuantStudio™ 5 Real-Time PCR System (Applied Biosystems) for 40 cycles with a threshold value of 0.2 applied to all samples.
2.4 Histopathology
Histopathological changes in the hearts of PRV-1 infected Atlantic salmon and brown trout fry, parr and post smolts were examined. For histology, whole fry (tail and fins removed) or isolated hearts from parr and post smolts were fixed in formalin (Sigma-Aldrich) for 48 hrs and then transferred to 70% ethanol. After dehydration in a graded ethanol series and clearing with xylene, the tissues were embedded in histowax. Sections were cut at 3µm thickness and stained using Shandon instant hematoxylin (Thermo Scientific), erythrosine and saffron (Waldeck) (HES) staining. The sections were scanned using the NanoZoomer S60 digital slide scanner (Hamamatsu Photonics, United Kingdom) and the images were viewed using the software NanoZoomer Digital Pathology NDP.view2 (Hamamatsu Photonics). Selected sections were photographed using Leica DMRBE microscope mounted with a SPOT Insight camera. The histopathology visual analog scoring for parr and post smolt heart was done as described previously with some modifications (Finstad et al., 2012). The heart ventricle sections of parr and post smolts were divided into four quarters, scored separately for epicardium and myocardium, and the average score was calculated for each fish. For the fry, the pathological changes in epicardium and myocardium of the whole heart were scored separately as described (Wessel et al., 2017).
2.5 In situ hybridization
RNAscope in situ hybridization of PRV-1 using the RNAscope 2.5 HD manual detection red kit (Advanced Cell Diagnostics, Newark, CA, USA) was carried out as described previously (Dhamotharan et al., 2020). The probe targeting PRV-1 segment L3 region 415-1379 (relative to ORF start) (KY429945) (catalogue number 537451) was used for ISH. A probe targeting Salmo salar peptidylprolyl isomerase B (ppib) (PPIB) mRNA (catalogue number 494421) was used as a positive control, and a probe against the Bacillus subtilis strain SMY dihydrodipicolinate reductase (dapB) gene (catalogue number 310043) was used as negative control. Formalin fixed paraffin embedded (FFPE) sections (n=2) from salmon (7-, 9-, and 12-wpi) and trout (7- and 9-wpi) bath challenge were stained for PRV-1. In brief, the sections were baked at 60°C for 60 min and deparaffinized in two changes of xylene for 5 minutes each and two changes of absolute ethanol for 1 minute each with agitation. The slides were air-dried for 5 minutes at RT. The sections were incubated with 3% RNAscope hydrogen peroxide for 10 min at room temperature and then boiled for 15 min in RNAscope 1x target retrieval reagent at 95-99°C. The slides were rinsed with distilled water and transferred to absolute ethanol for 3 minutes, followed by drying at 60°C. A hydrophobic barrier was drawn around each section using an Immedge™ barrier pen (Vector Laboratories) and dried overnight at RT.
The sections were then treated with RNAscope protease plus by incubating at 40°C in a humid hybridization oven for 15 minutes. Appropriate probes were added to the sections and hybridized for 2 hrs at 40°C, followed by washing in two changes of RNAscope 1X wash buffer for 2 min with agitation. The sections were hybridized with RNAscope 2.5 AMP 1 to AMP 6 according to the manufacturer’s recommendations for time and temperature. The staining was done with a mixture of RNAscope 2.5 Fast Red – A and Fast Red – B for 10 minutes in a dark room at RT. The slides were counterstained with 50% Gill’s hematoxylin I for 2 minutes, followed by bluing with distilled water containing 0.02% ammonia. The slides were dried at 60°C for 15 minutes, dipped in fresh xylene before mounting with EcoMount (Biocare).
2.6 Statistical analysis
The data arrangement, visualization, and statistical analyses were performed in R (R core team, 2013). The mean ± standard deviation (SD) of qPCR Ct value from positive samples were described in Tables 2–4, and statistically different infection prevalence between salmon and trout in each time point and the developmental stage was regarded as significant when the P-value was less than 0.05 by the Chi-square analysis using a “gmodels” library in R (Warnes et al., 2015). The raincloud plot was employed to present the individual Ct value with different time points, species, and challenge methods in Figures 2–4 using “cowplot”, “ggplot2”, and “tidyverse” libraries in R (Allen et al., 2019). Welch’s two-sample t-test was implemented to compare viral load (Ct value) and growth performance between Atlantic salmon and brown trout in the same challenge group at the same time point, and Tukey HSD one-way analysis of variance (ANOVA) test was carried out for qPCR Ct value from PRV positive salmon or trout among the different sampling time point using the “haven” library (Wickham and Miller, 2017).
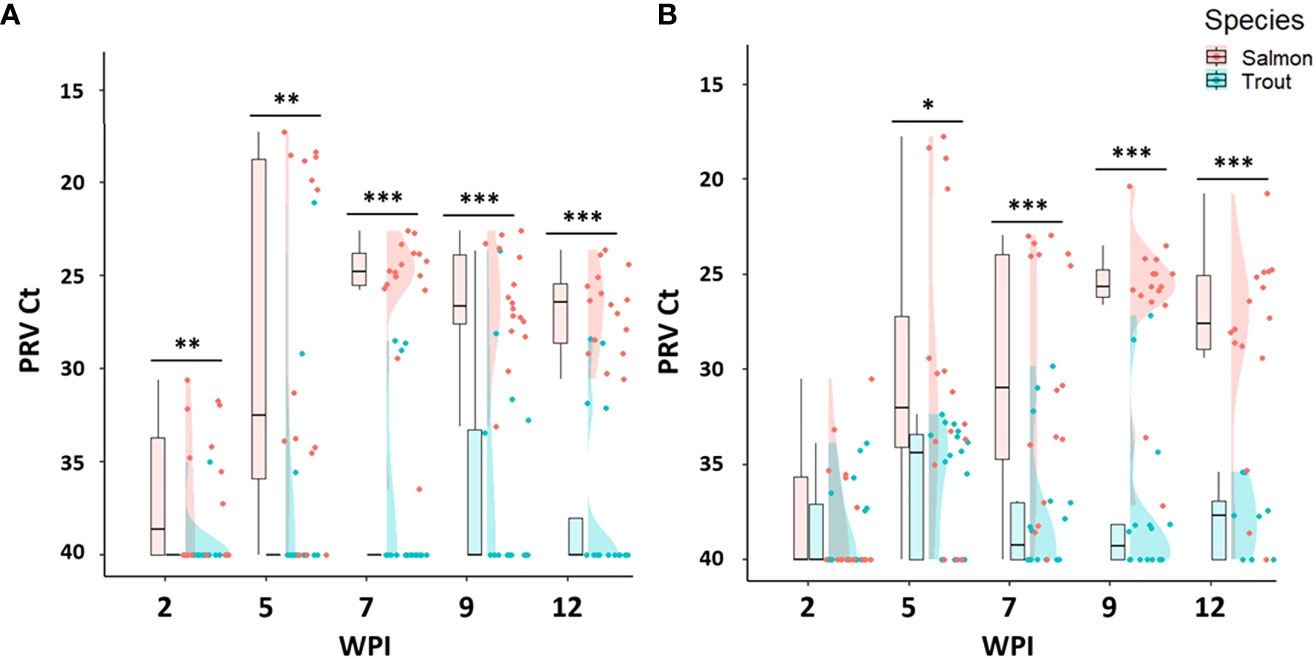
Figure 2 Raincloud plots comparing PRV-1 viral load between salmon and trout during the fry (A) bath, and (B) cohabitation challenge. Asterisk (* P < 0.05; ** P < 0.01; *** P < 0.001) indicate significant differences based on Welch’s two sample t-test.
3 Results
3.1 PRV-1 infection susceptibility of fry
3.1.1 Atlantic salmon
Both the bath and cohabitation challenge methods produced 100% infection prevalence as well as similar viral loads in Atlantic salmon fry (Table 2). At 2 wpi, the infection prevalence was 50% in the bath challenge and 38% in the cohabitation challenge. The infection prevalence increased during the following weeks and reached 100% at 7 wpi in the bath challenge and at 9 wpi in the cohabitation challenge (Table 2). The viral load in bath challenge peaked at 5 wpi (mean Ct 25.0 ± 7.6) and thereafter maintained at the same level (Figure 2A). Similarly, the viral load of infected fish in the cohabitation challenge peaked at 9 wpi (Figure 2B).
3.1.2 Brown trout
Both the bath and cohabitation challenge methods failed to reach 100% infection prevalence in brown trout fry (Table 2). Both methods produced significantly lower viral loads in brown trout fry compared to salmon (Figures 2A, B). In the bath challenge, the highest infection prevalence was 31% at 9 wpi. The cohabitation challenge consistently had higher infection prevalence at all the sampling points than the bath challenge, achieving the highest infection prevalence of 69% at 5 wpi (Table 2). However, the mean viral load in the bath challenge was higher than the cohabitation challenge at all sampling time points. The highest average Ct values of 28.6 ±7 and 33.7±1 were observed at 5 wpi after the bath and cohabitation challenges, respectively (Figures 2A, B).
3.2 PRV-1 infection susceptibility of parr
3.2.1 Atlantic salmon
Both the bath and cohabitation challenge methods failed to reach 100% infection prevalence in Atlantic salmon parr (Table 3 and Figure 3). The bath challenge resulted in no detectable PRV-1 infection except one fish with Ct 37.8 at 12wpi. In the cohabitation challenge, the infection prevalence differed between the replicate tanks, with 25% in tank 1 and 100% in the second tank giving an average infection prevalence of 63% at 7 wpi in salmon parr. Nevertheless, the infected fish had high viral loads, achieving a peak at 7 wpi (mean Ct 23.8 ± 5.3) which was maintained at similar levels up to the last sampling time point (12 wpi) without any significant differences between successive sampling intervals (Figure 3).
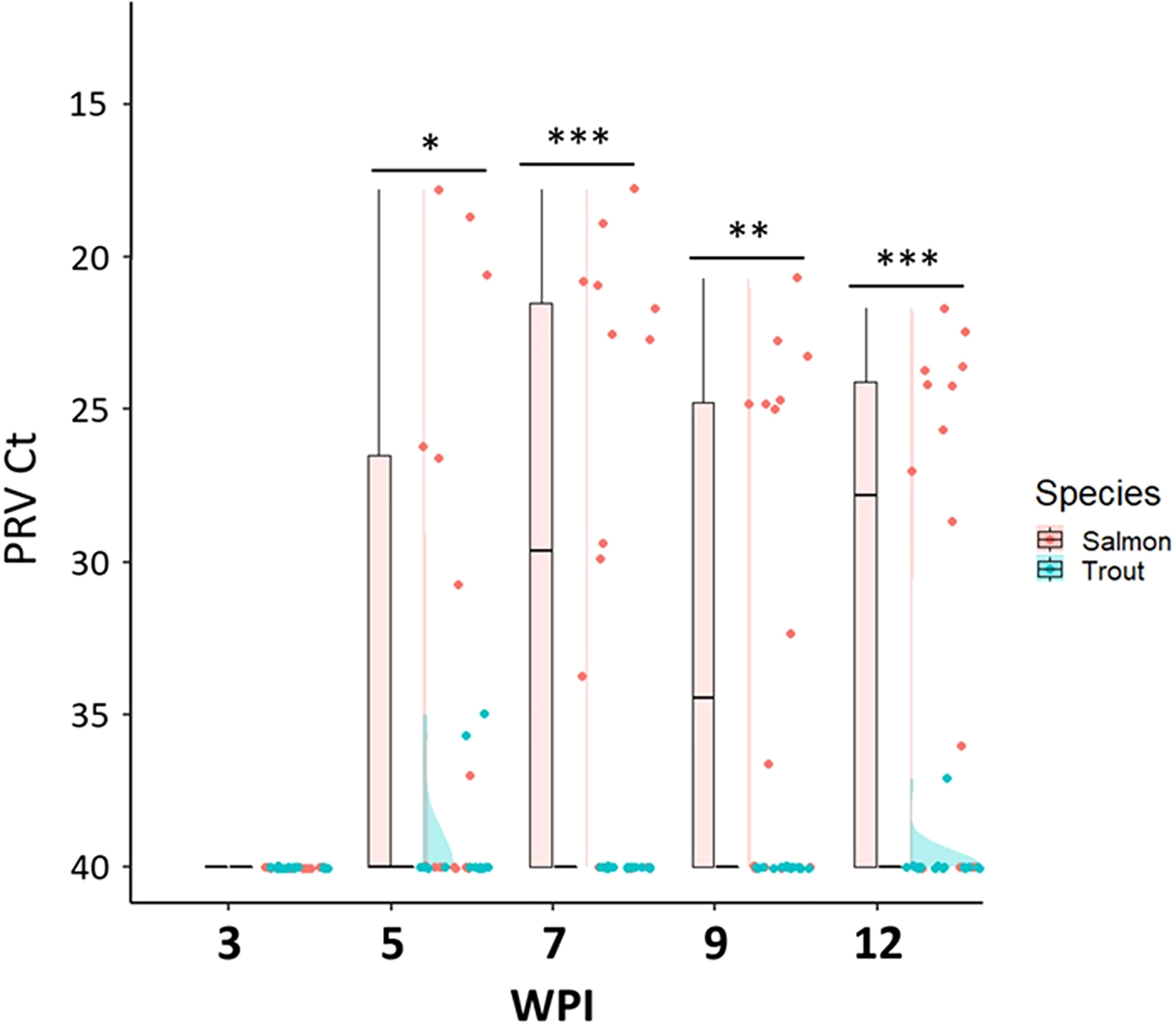
Figure 3 Raincloud plots comparing the PRV-1 viral load in salmon and trout parr after the cohabitation challenge. Asterisk (* P < 0.05; ** P < 0.01; *** P < 0.001) indicate significant differences based on Welch’s two sample t-test.
3.2.2 Brown trout
The cohabitation challenge resulted in a maximum infection prevalence of 13% at 5 wpi while the bath challenge resulted in no detectable PRV-1 infection in brown trout parr (Table 3). Two PRV-1 positive fish had very low viral loads, Ct 35.7, 35.0 at 5 wpi and one PRV-1 positive fish at 12 wpi, Ct 37.1 in the cohabitation experiment, all other trout parr were PRV-1-negative (Figure 3).
3.3 PRV-1 infection susceptibility of post-smolts
3.3.1 Atlantic salmon
Both the cohabitation and IP challenge resulted in 100% infection of salmon post-smolts with PRV-1 (Table 4). In the cohabitation challenge, the viral load peaked at 8 wpi (mean Ct value 20.3 ± 4.9) but had significantly decreased by week 19 (Figure 4A). In the IP challenge, the infection prevalence reached 100% at 2 wpi, six weeks earlier than the cohabitation challenge (Table 4). The viral load of PRV-1 positive IP challenged salmon peaked at 4 wpi (mean Ct 19.8 ± 1.5), four weeks earlier than the cohabitation challenge and reduced significantly (p <0.0001) afterwards (Figure 4B). In the cohabitation challenge, virus infection had not cleared by 12wpi, with all samples still positive for PRV-1 (mean viral load 28.2 ± 1.0). In IP challenge, four tested fish at 19 wpi had a mean Ct value of 33.5 ± 3.
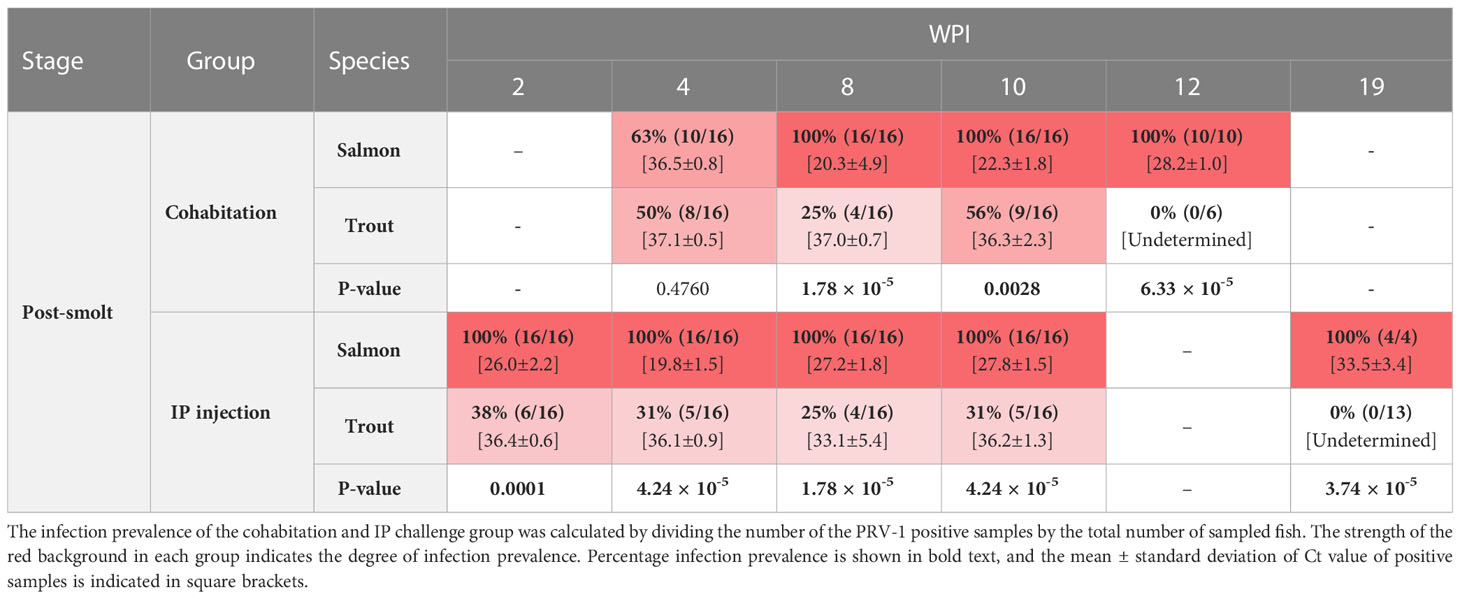
Table 4 The prevalence of PRV -1 positive salmon and trout post-smolt at 2, 4, 8, 10, and 12 or 19 WPI.
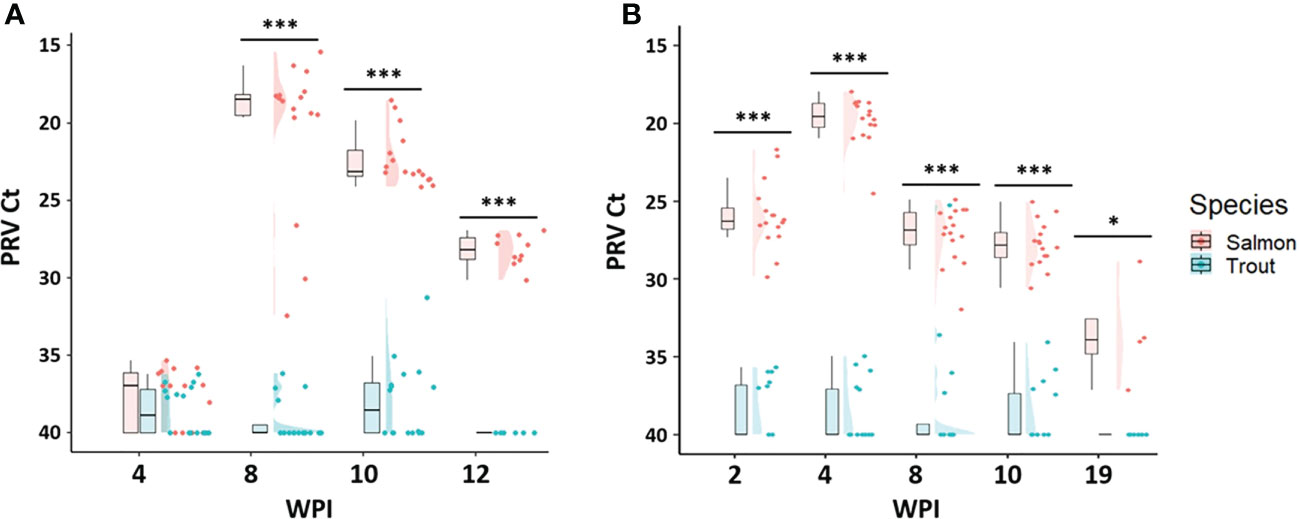
Figure 4 Raincloud plots for comparison of PRV-1 viral RNA load between salmon and brown trout in post-smolt stage after (A) cohabitation, and (B) IP challenge Asterisk (* P < 0.05; *** P < 0.001) indicate significant differences based on Welch’s two sample t-test. At 19 WPI 4 remaining fish were tested for viral load.
3.3.2 Brown trout
Both the cohabitation and IP challenge methods failed to reach 100% infection in brown trout post-smolts (Table 4 and Figures 4A, B). The maximum infection prevalence was 56% for the cohabitation challenge and 38% for the IP challenge. The cohabitation challenge resulted in a higher infection prevalence and showed lower levels of viral RNA, with averages above Ct 36. Similarly, all the infected fish in the IP challenge had Ct values above 33, except for one fish at 8 wpi which had a Ct value of 25.3.
3.4 Histopathology
PRV-1 infected salmon fry, parr and post-smolts all developed histopathological changes characteristic of PRV-1 infection, such as epicarditis and myocarditis in both the compact and spongy myocardium of the heart (Figures 5–7). In contrast, none of the PRV-1 infected brown trout showed histopathological changes at any the life stage or in any challenge method (Figures S1, S2).
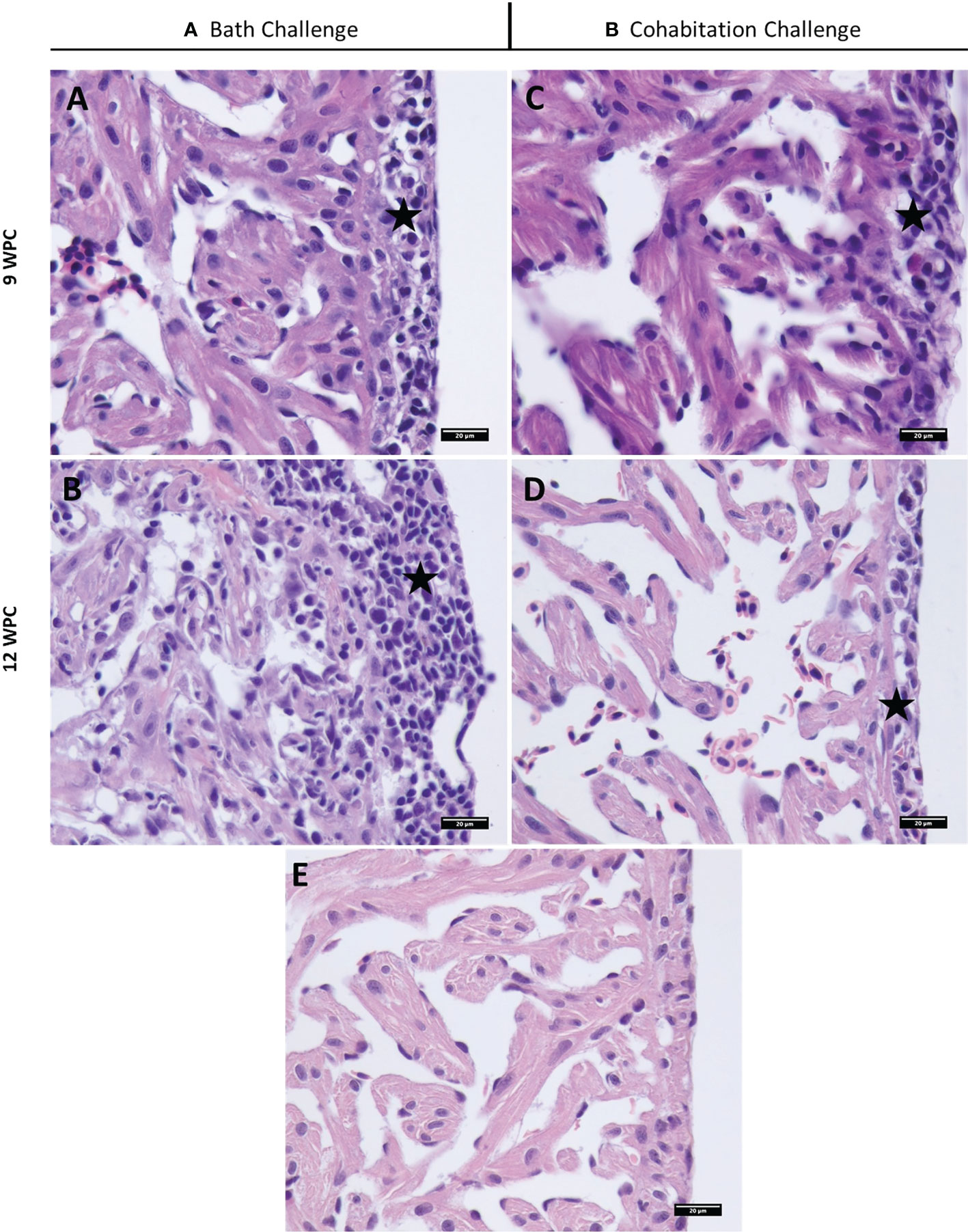
Figure 5 Histopathological changes in PRV-1 infected Atlantic salmon fry heart (A, B). Bath challenged fry heart ventricle displaying epicarditis at 9 and 12 WPI and inflammation in myocardium typical of HSMI (C, D). Cohabitation challenged fish showing mild inflammation mainly in epicardium at 9 and 12 WPI. (E) Noninfected control salmon heart at 12 WPC. Stars highlight epicarditis.
In salmon fry, heart lesions were observed in both epicardium and myocardium after bath challenge starting from 7 wpi and peaking at 12 wpi (Figure 5 and Table S1A). Cardiomyocyte necrosis and degeneration was also observed in some of the fish. The cohabitation challenge method resulted in mild heart lesions at 9 and 12 wpi in salmon fry (Figure 5).
In salmon parr, severe heart lesions were observed in the cohabitation challenge at 9 and 12 wpi (Figures 6A, B, and Table S1B). In the replicate cohabitation tank, which had a lower prevalence and viral load, correspondingly milder, or no pathological changes were observed. Heart lesions were characterized by infiltration of inflammatory cells in diffuse multilayers throughout the epicardium and multifocal to diffuse inflammation in both compact and spongy myocardium. Necrotic degeneration and vacuolations were observed in the myocardium of some of the severely infected fish.
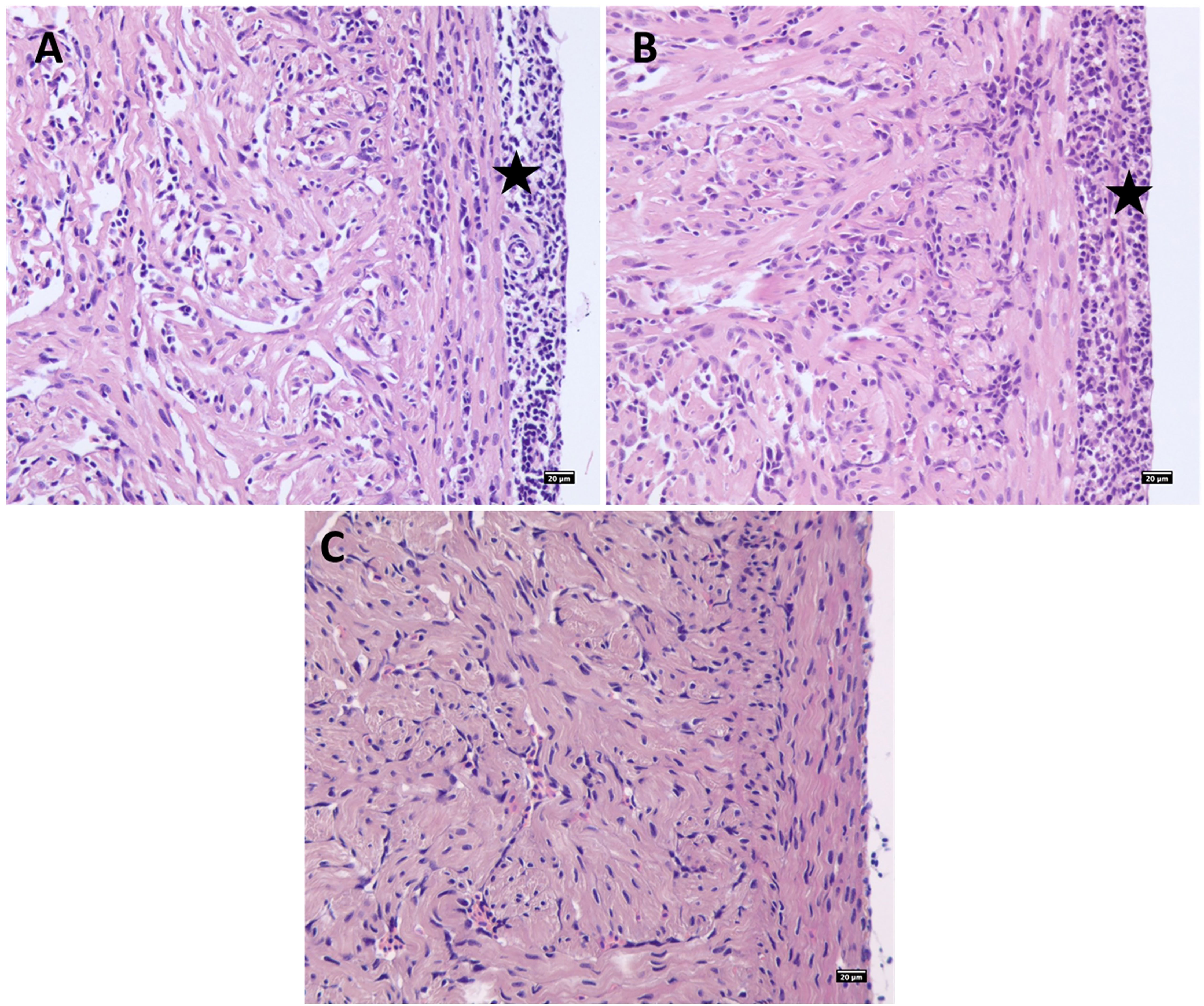
Figure 6 PRV-1 cohabitation challenged Atlantic salmon parr heart ventricle showing severe epicarditis and myocarditis in the compact and spongy myocardium at (A) 9 WPI and (B) 12 WPI. (C) Noninfected control salmon parr heart at 12 WPC. Stars highlight epicarditis.
In salmon post-smolts, severe inflammatory changes such as infiltration of inflammatory cells, necrosis, vacuolation and degeneration of cardiomyocytes were seen in the heart ventricle in the cohabitation challenge at 10 wpi (Figure 7A and Table S1C). The peak in heart pathology was observed 2 weeks after the peak in viral load in thew cohabitation challenge (score - epi – 1.7 ± 1.2, myo 1.9 ± 1.3). At 8 wpi, IP challenged salmon post-smolts showed mild to moderate pathological changes in heart tissues (Figure 7B and Table S1C).
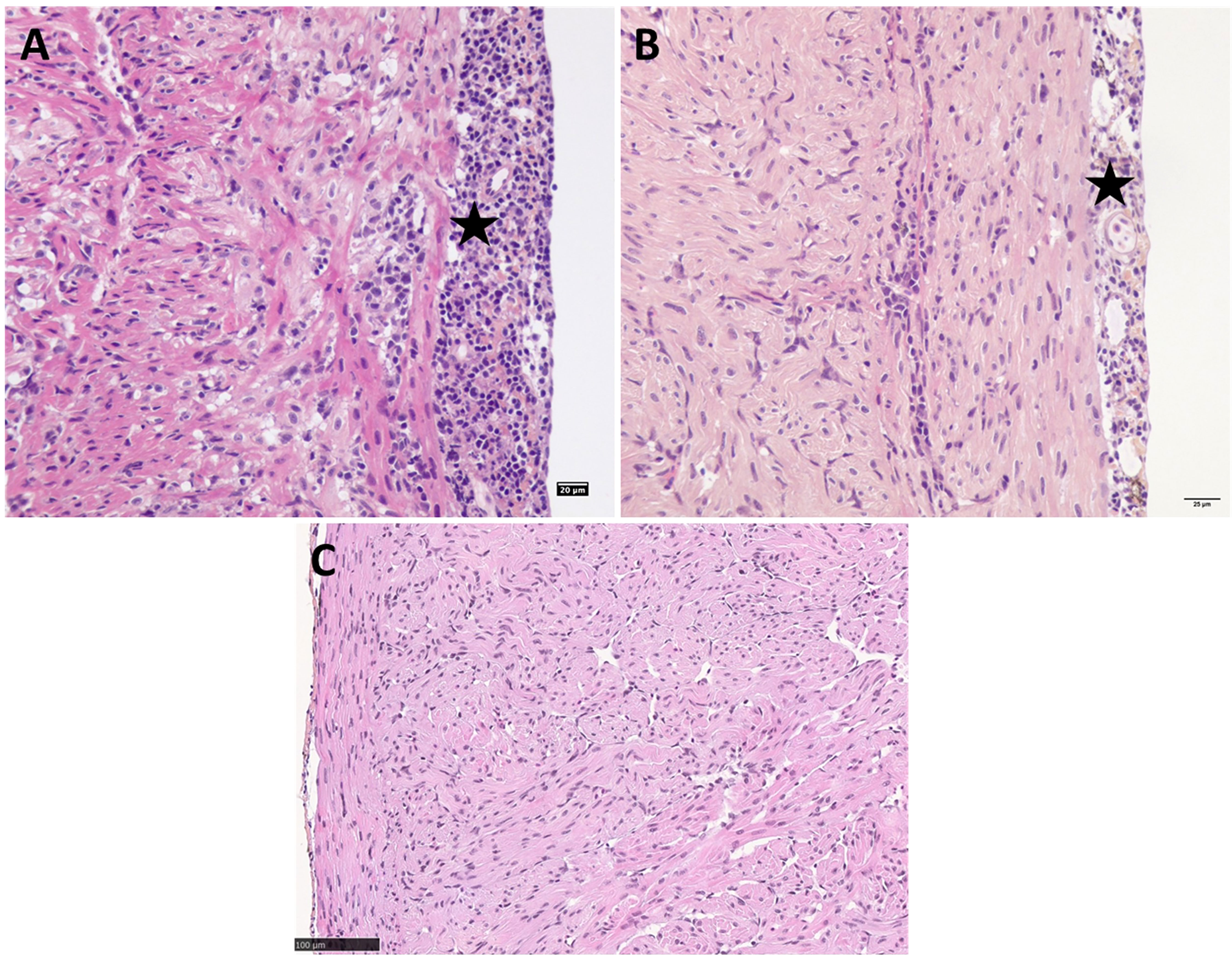
Figure 7 PRV-1 challenged Atlantic salmon post-smolts heart ventricle showing severe epicarditis and myocarditis in the compact and spongy myocardium (A) Cohabitation challenge at 10 WPI (B) IP challenge at 8 WPI. (C) Noninfected control Atlantic salmon post-smolt heart ventricle at 8 WPC. Stars highlight epicarditis.
3.5 In situ hybridization of PRV-1 in fry
In situ staining of PRV-1 bath challenged salmon whole fry at 7, 9, and 12 wpi revealed the temporal and spatial infection profile of PRV-1 in different tissues (Figures 8A–I, 9A–D, S3A–D). Trout fry were negative for PRV-1 staining at 7 and 9 wpi (data not shown). However, PRV-1 positive staining was observed in erythrocytes in bath challenged salmon at 7, 9 and 12 wpi which reflected well with the detection of viral load by qPCR. The erythrocytes in brain, gills, pancreas, eye, liver, and kidney were also positive for PRV-1 at 7 wpi. In head kidney, melanomacrophages were heavily stained for PRV-1 (Figure 8D). At 7 wpi, the salmon fry hearts without noticeable pathology showed heavy PRV-1 staining in cardiomyocytes (Figure 8B). Hepatocytes were positive for PRV-1 staining at 7 wpi (Figure 8C) coinciding with cardiomyocyte staining. At 9 wpi, PRV-1 positive staining was observed in cardiomyocytes and erythrocytes in heart ventricle, hepatocytes and erythrocytes in liver, and in kidney, both in erythrocytes and melanomacrophages (Figure S3). At 12 wpi, the cardiomyocytes were negative for the virus, but the pathological changes were still noticeable (Figure 9A). Erythrocytes in the heart ventricle (Figure 9B) and liver (Figure 9C), both erythrocytes and melanomacrophages in head kidney (Figure 9D) were still positive for PRV-1 at 12 wpi.
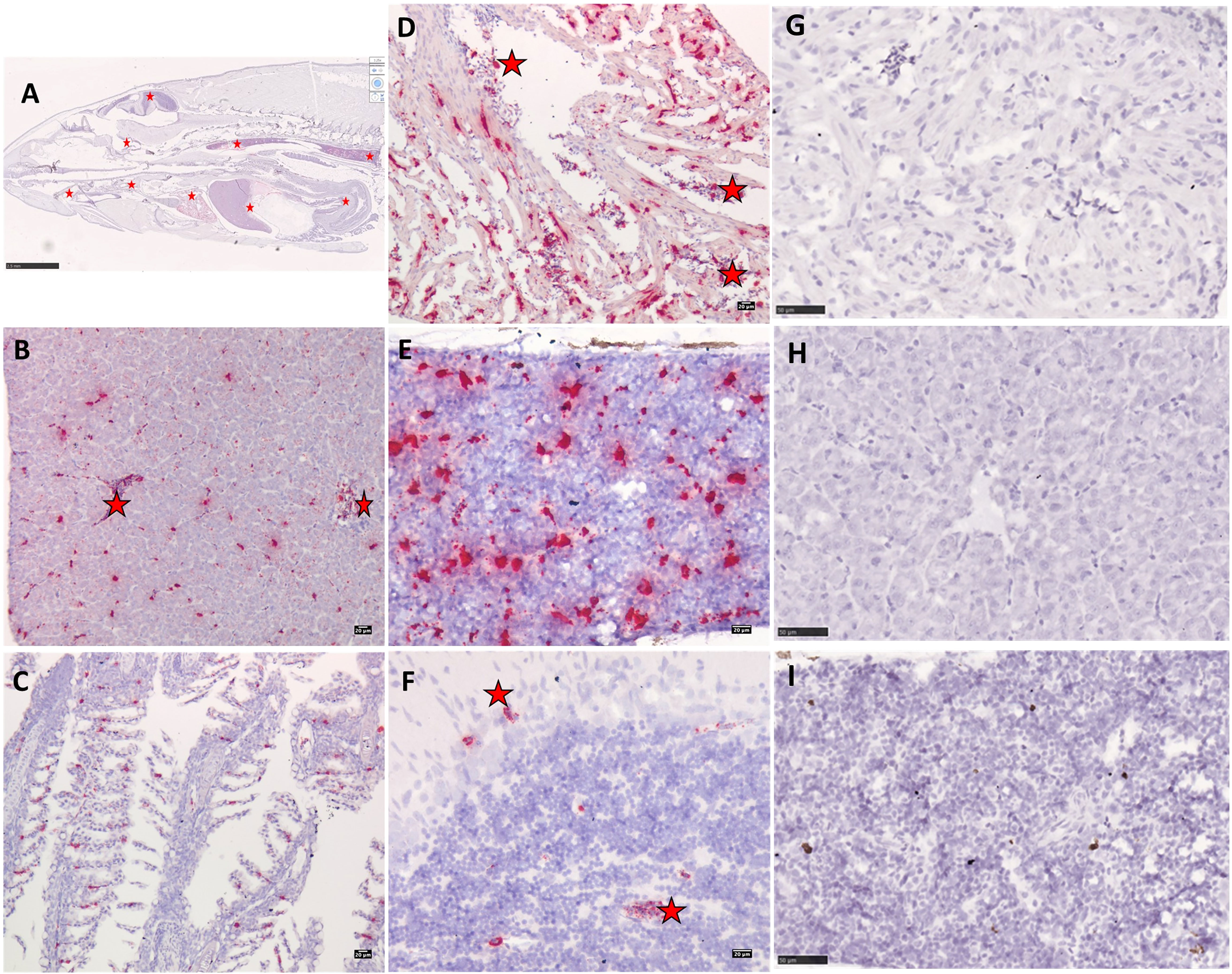
Figure 8 Micrographs showing PRV-1 in situ staining of bath challenged Atlantic salmon (A) whole fry at 7WPI. (D) PRV-1 positive staining in heart ventricle cardiomyocytes and erythrocytes. (B) Liver showing positive staining in erythrocytes and hepatocytes. (E) In head kidney, PRV-1 staining is observed mainly in melanomacrophages. (C, F) PRV-1 positive erythrocytes in gills and brain. PRV-1 bath challenged Atlantic salmon tissues stained with negative control ISH probes (G) heart ventricle (H) liver (I) head kidney. PRV-1 positive staining in erythrocytes in different organs highlighted in red stars.
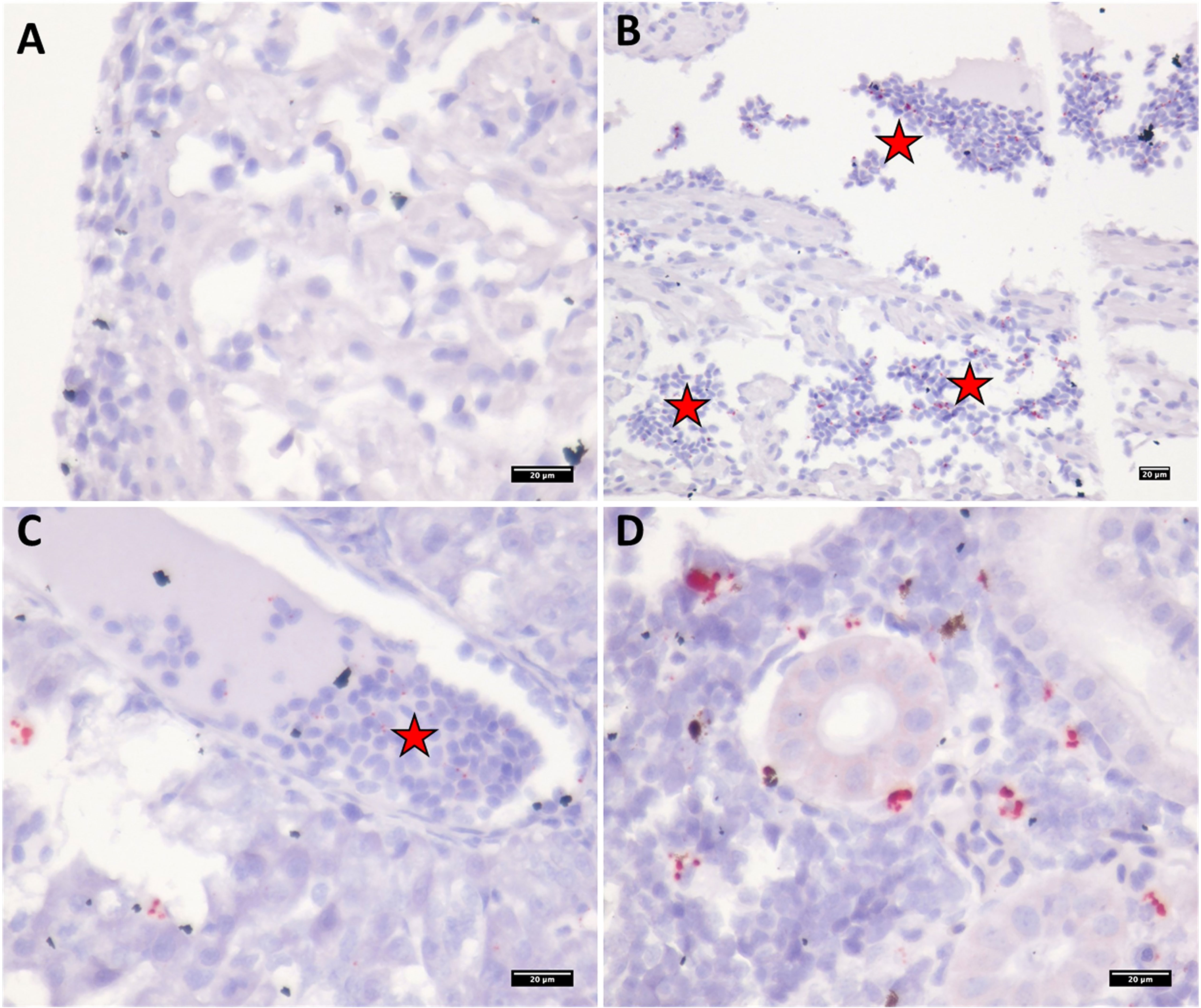
Figure 9 Micrographs showing PRV-1 in situ staining of bath challenged Atlantic salmon tissues at 12WPI. (A) no staining in epicardium or cardiomyocytes (B) PRV-1 positive staining in erythrocytes in heart ventricle (C) Liver showing positive staining in erythrocytes. (D) In kidney, PRV-1 staining is observed in erythrocytes and melanomacrophages. PRV-1 positive staining in erythrocytes in different organs highlighted in red stars.
3.6 Growth performance
The growth performance at the fry, parr and post smolt stages of salmon and trout was compared between the control and cohabitation groups (Figures 10A–C). At the fry stage, both salmon and trout PRV-1 infected groups had a consistently and significantly lower weight gain than fish in the control groups for most of the sampling time points (Figure 10A). There were no significant differences in weight between control and infected salmon post smolts. There were no significant differences between the control and cohabitation groups in the parr and post-smolt stages of trout except at 4 and 10 wpi in the post smolt (Figure 10C) where the infected fish appeared to perform better than the control fish.
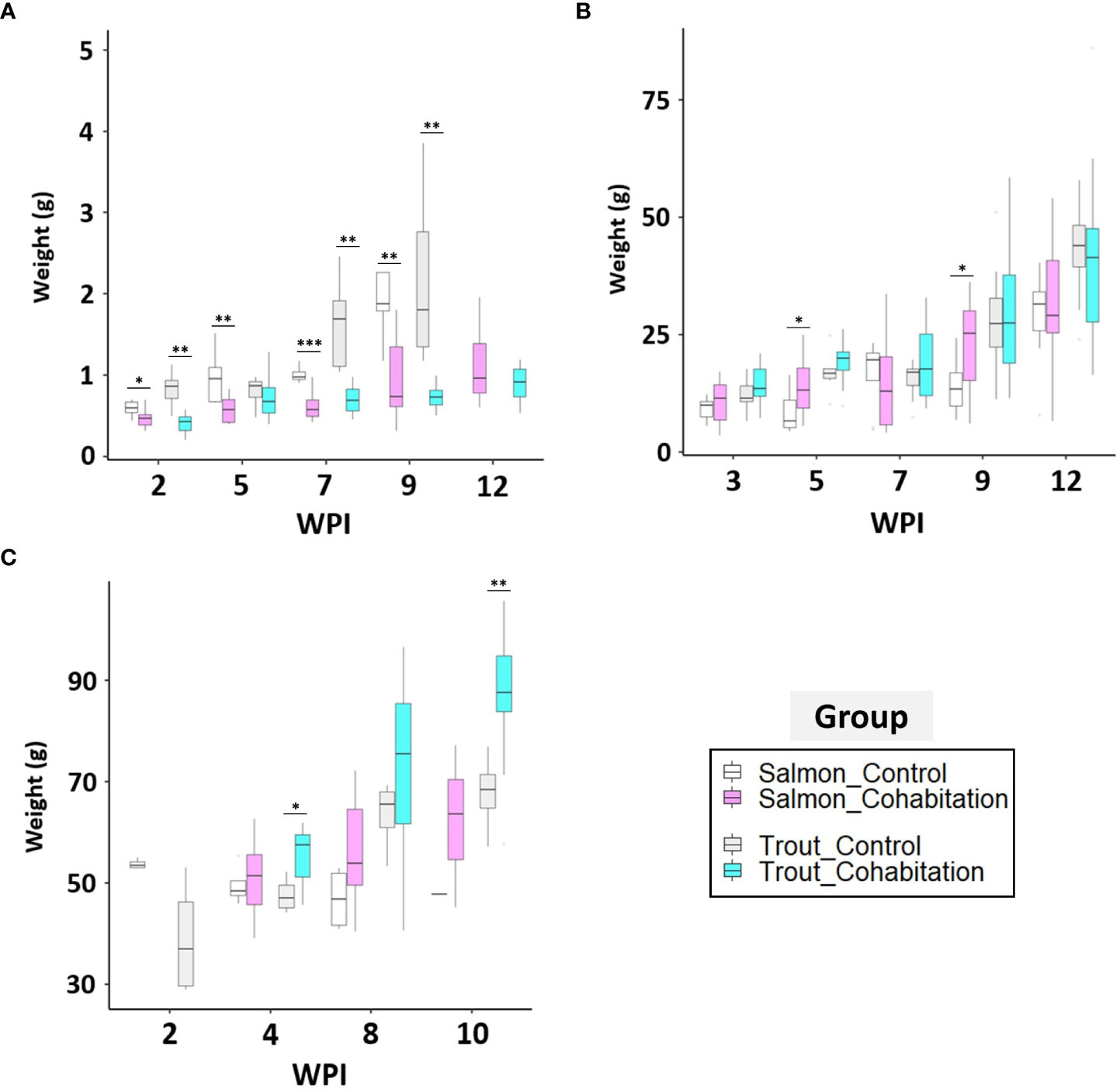
Figure 10 Box and whisker plots for the weight of the control and PRV-1 challenged groups. The box indicates median weight, interquartile range (IQR) and the whiskers indicates ranges of plus or minus 1.5 times IQR. The comparison between control and cohabitation (A; fry, B; parr, and C; post-smolt) for salmon and brown trout. Asterisk (* P < 0.05; ** P < 0.01; *** P < 0.001) indicate significant differences between same species of control and cohabitated group based on Welch’s two sample t-test.
4 Discussion
Since Atlantic salmon and brown trout are anadromous fish that spend part of their life in freshwater and part in seawater, their physiological, behavioral, and morphological characteristics are altered during the different developmental stages (Mccormick et al., 1998). These differences may affect the interaction between the host and pathogen, and ultimately affect the virulence and/or pathological outcome. In salmon farming, HSMI outbreaks occur after seawater transfer, and accordingly, most studies of PRV-1 infection have been carried out with post-smolts (Finstad et al., 2014; Wessel et al., 2017). However, PRV-1 infection at the freshwater stage has also been reported in 36% of cohorts in one of the longitudinal studies following an Atlantic salmon production cycle (Lovoll et al., 2012). Despite this, information on PRV-1 susceptibility and disease development during pre-smolt stages (fry and parr) is limited. Likewise, the susceptibility of trout at different developmental stages to PRV-1 infection has not been verified yet. In the wild, salmon and trout share partially overlapping ecological niches (Nevoux et al., 2019). Given the high likelihood of PRV exposure between different salmonid species, and possibility of transmission to fry and parr in rivers from infected escapees from fish farms, studies on the susceptibility and virulence of PRV-1 in the fry, parr and post smolt stages of different salmonid species is necessary. The Atlantic salmon used in Norway originates from different selective breeding programs and may have varying genetic backgrounds with varying disease susceptibility. In this study, we used wild-caught salmon and trout to produce fry, parr and post-smolts. Hence, to advance the knowledge on these topics, this study evaluated and compared the susceptibility and infection dynamics of brown trout and Atlantic salmon fry, parr and post smolts, as well as observed the mortality, pathology and growth performance following PRV-1 infection.
The results showed that the PRV-1 infection prevalence and viral kinetics depended on the developmental stages and challenge method. Our results suggest that Atlantic salmon appears to be the primary host and most susceptible to PRV-1 infection. All three PRV-1 challenge methods used in this study suggested that brown trout are less susceptible to disease by the tested PRV-1 genotype as suggested by the general trend of lower prevalence and viral load in this fish species compared to Atlantic salmon. Interestingly, brown trout fry that was successfully infected with the virus during the bath challenge showed relatively high viral loads (Ct value < 30) which is comparable to some of the infected Atlantic salmon fry. Similarly, another salmonid virus, ISAV can cause serious disease in Atlantic salmon but not in pacific salmon or brown trout (Nylund and Jakobsen, 1995). Despite achieving high viral load, the ISAV-infected pacific salmon did not develop any clinical sign or mortality. These susceptible species can act as natural reservoirs of virus infection (Rolland and Winton, 2003). Our study also confirms that the disease progression and severity depend on the host species and life stages. The differences in susceptibility and disease development may be attributed to the differences in immune response against the virus infection.
In this study, we investigated three different challenge methods: bath challenge, IP injection and cohabitation challenge. Overall, we found that salmon were infected with PRV-1 by all challenge methods. However, the bath challenge resulted in very low infection success at the parr stage. One possible reason for this result could have been the use of infectious water outside the peak shedding period for the virus. Hence, the amount of infectious PRV-1 in the water used in the bath challenge and/or the time of exposure was too low to establish a successful infection. Since peak virus shedding can vary depending on shedder fish size and the PRV-1 genotype used for infection, cohabitation challenge appears to be a more reliable method for PRV-1 challenge trials as demonstrated by its higher success rate. However, bath infections could perhaps be optimized and improved, as this method has the benefit to precisely know the timing of the infection window, allowing better comparison of infection dynamics between individual fish while still following a natural infection route.
We have observed differences between the fry and parr stages in PRV-1 infection prevalence, highlighting the significance of life stage on susceptibility to infections. There was no mortality in any of the PRV-1 challenged groups during the parr and post-smolt stages for both salmon and brown trout. In the salmon fry cohabitation challenge groups, mortality started on day 14 and continued steadily throughout the experiment, resulting in 18.3% cumulative mortality at the end of the experiment (Figure 11A). The cumulative mortality in the bath challenged group was only 3.4%. There was some unexplained mortality in the control group (approximately 10%) between 3 to 4 wpi. Although mortality was observed in the control groups, none of the sampled fish in these groups were positive for PRV-1. This suggests that the death of the control fish was not due to virus infection, thus excluding any possibility of cross-contamination between treatment groups. The cumulative mortality for trout fry was 14.3%, 9.1%, and 5.0% in the cohabitation, bath, and control groups, respectively (Figure 11B). Survival curves between the different treatment groups were all significantly different from each other, possibly suggesting differences in the cause of death between treatment groups. In addition, growth performance in the fry was significantly lower in the PRV-1 infected group compared to the control group. Based on the growth differences, and mortality data, it appears that the Atlantic salmon and brown trout fry stage is more affected by PRV-1 infection than parr and post-smolt stages To our knowledge, this is the first study describing the PRV-1 infection Atlantic salmon and brown trout fry. PRV-1 has not been shown to cause clinical signs or mortality in laboratory challenge experiments. However, other salmonid viruses such as IPNV (Roberts and Pearson, 2005), and SAV (Herath et al., 2017) causes mortality in Atlantic salmon fry.
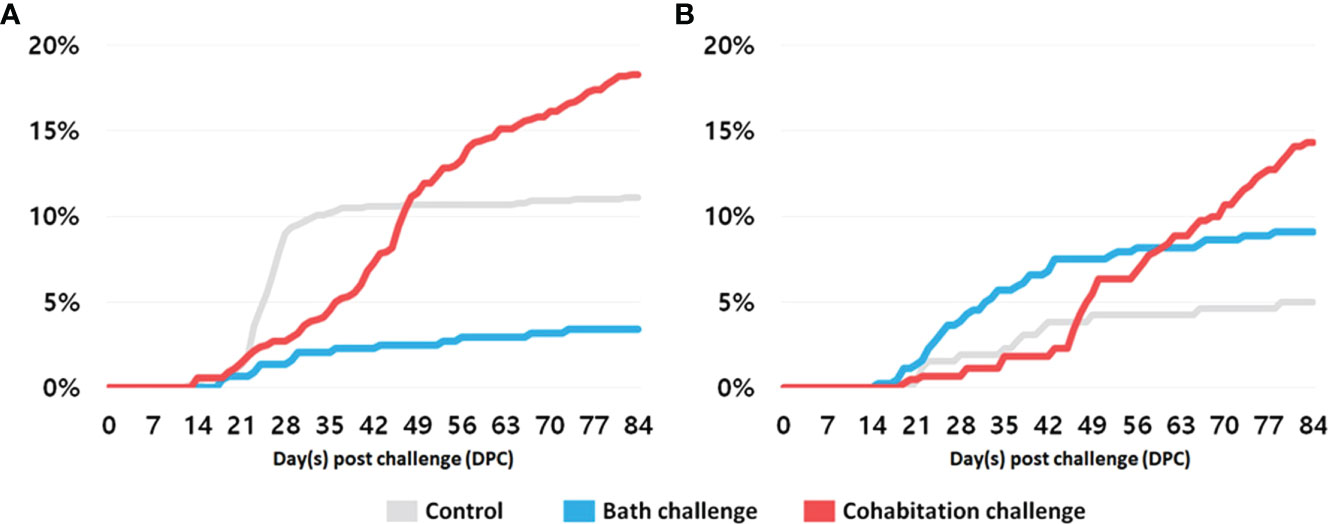
Figure 11 Cumulative mortality in fry (A) Atlantic salmon, and (B) brown trout in accordance with the different methods of PRV-1 infection.
The kinetics of PRV-1 infection was different between each of the developmental stages in Atlantic salmon. The viral load in the post-smolt stage peaked at 8 wpi in the cohabitation challenge and 2 wpi in the IP challenge, but dropped off sharply after that. However, PRV-1 infection levels in the fry and parr stages gradually increased up to 7 – 9 wpi and then maintained a high viral load until at least 12 wpi. In general, the IP challenged fish demonstrated an early peak in viral load followed by histopathological changes, likely due to the delivery of high infectious titers of PRV-1. The time taken to achieve peak in viral load and heart inflammation can be manipulated by adjusting the viral titers in the inoculum as shown in a previous study (Wessel et al., 2017).
Polinski et al. (Polinski et al., 2020) found that PRV-1 infection can be divided into three steps: 1) early entry and dissemination, 2) peak and systemic replication, and 3) long-term persistence stage. These authors found that the persistence stage normally had a lower viral load than the peak stage. This is similar to the PRV-1 kinetics in the salmon post-smolts observed in the present study. In salmon and trout fry and, to some extent, salmon parr stages, the viral load was consistently higher during the experimental period. Future long-term PRV-1 infection studies in the freshwater stage of Atlantic salmon of are of high importance to better understand the infection dynamics, epidemiology, and consequences of infection in farmed and wild fish.
In the present study, we infected brown trout fry, parr and post smolts with PRV-1 through several challenge methods. None of the brown trout groups produced 100% infection prevalence or developed any classical HSMI specific lesions. In previous surveillance studies, PRV-1 was also detected at low levels in wild caught (Ct 25.9 to 39.5) and hatchery-reared (Ct 34.4 to 36.5) brown trout at 3% (4/133) and 1.5% (4/271), respectively (Garseth et al., 2013b; Garseth and Biering, 2018). Low levels of PRV-1 (Ct 34.0 to 36.9) were detected in 1.3% (11/843) of the wild brown trout (Madhun et al., 2016). In the current study, among the different challenge methods and developmental stages tested for brown trout, the cohabitation challenge achieved the highest infection prevalence in fry and post smolt.
Olsen et al. (Olsen et al., 2015) reported an outbreak of PRV in freshwater rainbow trout hatcheries in Norway where 10 - 12,000 trout died every week over a six-month period with clinical signs similar to HSMI. It has been characterized as a new subtype of PRV, named PRV-3, which primarily causes disease in rainbow trout but not in Atlantic salmon (Dhamotharan et al., 2018). In a PRV-3 susceptibility study, 50% infection prevalence was observed among cohabitants of Atlantic salmon, in comparison with 100% of cohabiting rainbow trout (Hauge et al., 2017). PRV-3 is prevalent in wild brown trout (44 of 265 analyzed samples) and at higher viral load (Ct 22.5 to 39.6) (Garseth et al., 2019) which is comparatively higher than PRV-1 in brown trout. All these surveillance studies strongly suggest that brown trout are more susceptible to PRV-3 than PRV-1. Further experimental challenge studies with all subtypes of PRV on brown trout would give more insights into the differences in susceptibility and adaptability.
Transmission of infectious disease between farmed and wild fish is a major concern for wildlife conservation as well as aquaculture (Johansen et al., 2011). The differences in the ecology of wild Atlantic salmon and brown trout mean that the risk of contracting an infection from farmed fish is very different. Wild salmon migrating to spawning or feeding grounds move rapidly through fish-farming areas in fjords and coastal zones and are hence only temporarily exposed to pathogens from infected farming sites. In contrast, anadromous brown trout spend the entire seawater part of their life cycle in fjords and coastal zones, and are theoretically exposed to infectious pathogens from farming sites for a considerably longer period. The results presented here suggest that the youngest stages of brown trout and salmon are the most vulnerable to PRV-1 infection. This is in accordance with other studies showing higher susceptibility and lower tolerances to viruses in the younger life stages (Bergmann et al., 2003). As infected escapees are known to enter rivers there is a risk of environmental impact (Glover et al., 2017). Our results indicate that PRV-1 infection may cause reduced growth as well as direct mortality in fry. However, the frequency and magnitude of such events in a natural ecosystem are not only dependent on susceptibility to disease as described here, but on complicated interactions between the host, the pathogen, and the environment. After migration into sea water, it is quite likely that the post-smolts are exposed to PRV-1. If salmon are infected, morbidity is expected in some individuals, but apparently with little immediate impact on mortality and growth. In contrast, for brown trout post-smolts, which are likely to have a higher risk of exposure, our results indicate that there will be little or no impact. These results support the conclusions of risk assessment studies from Canada and Norway concerning the consequences of PRV-1 infection (Grefsrud Es et al., 2018).
In conclusion, our study has provided insight into the comparative susceptibility of Atlantic salmon and brown trout to PRV-1 at various developmental stages. Our results confirm that PRV-1 is capable of infecting both species throughout their developmental stages. However, brown trout exhibited lower susceptibility to the virus. Nonetheless, once infected, the viral load in some brown trout can reach a relatively high level, particularly during the fry stage. These results highlight the species-specificity and transmission of different PRV subtypes among salmonids. The results presented here further contribute to the fundamental knowledge of PRV-1 infection in salmonids and will help to guide future studies. The observation that trout are less susceptible to PRV-1 infection will have implications for assessing the risk that salmon farming poses to wild stocks of trout.
Data availability statement
The raw data supporting the conclusions of this article will be made available by the authors, without undue reservation.
Ethics statement
The animal study was reviewed and approved by Norwegian animal research authority (FOTS ID: 15111 and 11260).
Author contributions
DK: Experiments, Results analysis, Methodology, Visualization, Writing, Revising, Approving. HR: Results analysis, Methodology, Visualization, Writing. CM: Methodology, Resources, review & editing MP: Methodology, Resources, review & editing. AV: Resources, Writing - Review & Editing. TH: Resources, Writing - Review & Editing. PF: Resources, Writing - Review & Editing. EK: Resources, Writing - Review & Editing. IF: Resources, Writing - Review & Editing. MD: Resources, Writing - Review & Editing. HB: Resources, Writing - Review & Editing. SM: Resources, Writing - Review & Editing. GB: Resources, Writing - Review & Editing. JN: Challenge Experiment, Methodology, Resources. SP: Challenge Experiment, Conceptualization, Methodology, Resources. AM: Challenge Experiment, Conceptualization, Methodology, Resources. SG: Challenge Experiment, Conceptualization, Methodology, Resources. BK: Conceptualization; Project administration, Funding acquisition, Supervision, Writing – review & editing. All authors contributed to the article and approved the submitted version.
Funding
This study was supported by Institute of Marine Research (Bergen, Norway) under the disease transmission project 15821.
Acknowledgments
The authors acknowledge the contributions from IMR, Matre technicians and Bergen fish disease lab personnel.
Conflict of interest
Author AV is currenlty employed by Lerøy Seafood, Ås, Norway.
The remaining authors declare that the research was conducted in the absence of any commercial or financial relationships that could beconstrued as a potential conflict of interest.
Publisher’s note
All claims expressed in this article are solely those of the authors and do not necessarily represent those of their affiliated organizations, or those of the publisher, the editors and the reviewers. Any product that may be evaluated in this article, or claim that may be made by its manufacturer, is not guaranteed or endorsed by the publisher.
Supplementary material
The Supplementary Material for this article can be found online at: https://www.frontiersin.org/articles/10.3389/fmars.2023.1151577/full#supplementary-material
References
Aldrin M., Storvik B., Frigessi A., Viljugrein H., Jansen P. A. (2010). A stochastic model for the assessment of the transmission pathways of heart and skeleton muscle inflammation, pancreas disease and infectious salmon anaemia in marine fish farms in Norway. Prev. Veterinary Med. 93 (1), 51–61. doi: 10.1016/j.prevetmed.2009.09.010
Allen M., Poggiali D., Whitaker K., Marshall T. R., Kievit R. A. (2019). Raincloud plots: a multi-platform tool for robust data visualization. Wellcome Open Res. 4 (63). doi: 10.12688/wellcomeopenres.15191.1
Bergmann S. M., Fichtner D., Skall H. F., Schlotfeldt H. J., Olesen N. J. (2003). Age- and weight-dependent susceptibility of rainbow trout oncorhynchus mykiss to isolates of infectious haematopoietic necrosis virus (IHNV) of varying virulence. Dis. Aquat Organ 55 (3), 205–210. doi: 10.3354/dao055205
Bratland A., Nylund A. (2009). Studies on the possibility of vertical transmission of Norwegian salmonid alphavirus in production of Atlantic salmon in Norway. J. Aquat. Anim. Health 21 (3), 173–178. doi: 10.1577/H08-038.1
Dhamotharan K., Bjorgen H., Malik M. S., Nyman I. B., Markussen T., Dahle M. K., et al. (2020). Dissemination of piscine orthoreovirus-1 (PRV-1) in Atlantic salmon (Salmo salar) during the early and regenerating phases of infection. Pathogens 9 (2). doi: 10.3390/pathogens9020143
Dhamotharan K., Vendramin N., Markussen T., Wessel O., Cuenca A., Nyman I. B., et al. (2018). Molecular and antigenic characterization of piscine orthoreovirus (PRV) from rainbow trout (Oncorhynchus mykiss). Viruses-Basel 10 (4). doi: 10.3390/v10040170
Di Cicco E., Ferguson H. W., Kaukinen K. H., Schulze A. D., Li S. R., Tabata A., et al. (2018). The same strain of piscine orthoreovirus (PRV-1) is involved in the development of different, but related, diseases in Atlantic and pacific salmon in British Columbia. Facets 3, 599–641. doi: 10.1139/facets-2018-0008
Finstad O. W., Dahle M. K., Lindholm T. H., Nyman I. B., Lovoll M., Wallace C., et al. (2014). Piscine orthoreovirus (PRV) infects Atlantic salmon erythrocytes. Veterinary Res. 45. doi: 10.1186/1297-9716-45-35
Finstad O. W., Falk K., Lovoll M., Evensen O., Rimstad E. (2012). Immunohistochemical detection of piscine reovirus (PRV) in hearts of Atlantic salmon coincide with the course of heart and skeletal muscle inflammation (HSMI). Veterinary Res. 43. doi: 10.1186/1297-9716-43-27
Garseth A. H., Biering E. (2018). Little evidence to suggest salmonid freshwater reservoirs of piscine orthoreovirus (PRV). J. Fish Dis. 41 (8), 1313–1315. doi: 10.1111/jfd.12824
Garseth ÅH., Ekrem T., Biering E. (2013a). Phylogenetic evidence of long distance dispersal and transmission of piscine reovirus (PRV) between farmed and wild Atlantic salmon. PloS One 8 (12), e82202. doi: 10.1371/journal.pone.0082202
Garseth A. H., Fritsvold C., Opheim M., Skjerve E., Biering E. (2013b). Piscine reovirus (PRV) in wild Atlantic salmon, salmo salar l., and sea-trout, salmo trutta l., in Norway. J. Fish Dis. 36 (5), 483–493. doi: 10.1111/j.1365-2761.2012.01450.x
Garseth A. H., Moldal T., Gasnes S. K., Hjortaas M. J., Sollien V. P., Gjevre A. G. (2019). Piscine orthoreovirus-3 is prevalent in wild seatrout (Salmo trutta l.) in Norway. J. Fish Dis. 42 (3), 391–396. doi: 10.1111/jfd.12943
Gjessing M. C., Thoen E., Tengs T., Skotheim S. A., Dale O. B. (2017). Salmon gill poxvirus, a recently characterized infectious agent of multifactorial gill disease in freshwater- and seawater-reared Atlantic salmon. J. Fish Dis. 40 (10), 1253–1265. doi: 10.1111/jfd.12608
Glover K. A., Solberg M. F., Mcginnity P., Hindar K., Verspoor E., Coulson M. W., et al. (2017). Half a century of genetic interaction between farmed and wild Atlantic salmon: Status of knowledge and unanswered questions. Fish Fish. 18 (5), 890–927. doi: 10.1111/faf.12214
Grefsrud Es G. K., Grøsvik B., Husa V., Karlsen Ø, Kristiansen T., Kvamme B., et al. (2018). Risikorapport norsk fiskeoppdrett 2018. vol. 182. (Bergen, Norway: Havforskningsinstituttet).
Haatveit H. M., Nyman I. B., Markussen T., Wessel O., Dahle M. K., Rimstad E. (2016). The non-structural protein mu NS of piscine orthoreovirus (PRV) forms viral factory-like structures. Veterinary Res. 47. doi: 10.1186/s13567-015-0302-0
Hauge H., Vendramin N., Taksdal T., Olsen A. B., Wessel O., Mikkelsen S. S., et al. (2017). Infection experiments with novel piscine orthoreovirus from rainbow trout (Oncorhynchus mykiss) in salmonids. PloS One 12 (7). doi: 10.1371/journal.pone.0180293
Herath T. K., Ashby A. J., Jayasuriya N. S., Bron J. E., Taylor J. F., Adams A., et al. (2017). Impact of Salmonid alphavirus infection in diploid and triploid Atlantic salmon (Salmo salar L.) fry. PloS One 12 (9), e0179192. doi: 10.1371/journal.pone.0179192
Hodneland K., Endresen C. (2006). Sensitive and specific detection of salmonid alphavirus using real-time PCR (TaqMan (R)). J. Virol. Methods 131 (2), 184–192. doi: 10.1016/j.jviromet.2005.08.012
Johansen L. H., Jensen I., Mikkelsen H., Bjørn P. A., Jansen P. A., Bergh Ø (2011). Disease interaction and pathogens exchange between wild and farmed fish populations with special reference to Norway. Aquaculture 315 (3), 167–186. doi: 10.1016/j.aquaculture.2011.02.014
Kongtorp R. T., Halse M., Taksdal T., Falk K. (2006). Longitudinal study of a natural outbreak of heart and skeletal muscle inflammation in Atlantic salmon, salmo salar l. J. Fish Dis. 29 (4), 233–244. doi: 10.1111/j.1365-2761.2006.00710.x
Kongtorp R. T., Kjerstad A., Taksdal T., Guttvik A., Falk K. (2004a). Heart and skeletal muscle inflammation in Atlantic salmon, salmo salar l.: a new infectious disease. J. Fish Dis. 27 (6), 351–358. doi: 10.1111/j.1365-2761.2004.00549.x
Kongtorp R. T., Taksdal T., Lyngoy A. (2004b). Pathology of heart and skeletal muscle inflammation (HSMI) in farmed Atlantic salmon salmo salar. Dis. Aquat. Organisms 59 (3), 217–224. doi: 10.3354/dao059217
Kristoffersen A. B., Jensen B. B., Jansen P. A. (2013). Risk mapping of heart and skeletal muscle inflammation in salmon farming. Prev. Veterinary Med. 109 (1-2), 136–143. doi: 10.1016/j.prevetmed.2012.08.012
Kuehn R., Stoeckle B. C., Young M., Popp L., Taeubert J.-E., Pfaffl M. W., et al. (2018). Identification of a piscine reovirus-related pathogen in proliferative darkening syndrome (PDS) infected brown trout (Salmo trutta fario) using a next-generation technology detection pipeline. PloS One 13 (10), e0206164. doi: 10.1371/journal.pone.0206164
Lovoll M., Alarcon M., Jensen B. B., Taksdal T., Kristoffersen A. B., Tengs T. (2012). Quantification of piscine reovirus (PRV) at different stages of Atlantic salmon salmo salar production. Dis. Aquat. Organisms 99 (1), 7–U5. doi: 10.3354/dao02451
Lovoll M., Wiik-Nielsen J., Grove S., Wiik-Nielsen C. R., Kristoffersen A. B., Faller R., et al. (2010). A novel totivirus and piscine reovirus (PRV) in Atlantic salmon (Salmo salar) with cardiomyopathy syndrome (CMS). Virol. J. 7. doi: 10.1186/1743-422X-7-309
Lund M., Dahle M. K., Timmerhaus G., Alarcon M., Powell M., Aspehaug V., et al. (2017). Hypoxia tolerance and responses to hypoxic stress during heart and skeletal muscle inflammation in Atlantic salmon (Salmo salar). PloS One 12 (7). doi: 10.1371/journal.pone.0181109
Madhun A. S., Isachsen C. H., Omdal L. M., Einen A. C. B., Bjorn P. A., Nilsen R., et al. (2016). Occurrence of salmonid alphavirus (SAV) and piscine orthoreovirus (PRV) infections in wild sea trout salmo trutta in Norway. Dis. Aquat. Organisms 120 (2), 109–113. doi: 10.3354/dao03009
Markussen T., Dahle M. K., Tengs T., Lovoll M., Finstad O. W., Wiik-Nielsen C. R., et al. (2013). Sequence analysis of the genome of piscine orthoreovirus (PRV) associated with heart and skeletal muscle inflammation (HSMI) in Atlantic salmon (Salmo salar). PloS One 8 (7). doi: 10.1371/annotation/746a9036-0e54-4a9a-ab16-adb108a3a227
Mccormick S. D., Hansen L. P., Quinn T. P., Saunders R. L. (1998). Movement, migration, and smolting of Atlantic salmon (Salmo salar). Can J Fish Aquat Sci 55 (S1), 77–92. doi: 10.1139/d98-011
Mordecai G. J., Miller K. M., Bass A. L., Bateman A. W., Teffer A. K., Caleta J. M., et al. (2021). Aquaculture mediates global transmission of a viral pathogen to wild salmon. Sci Adv 7 (22), eabe2592. doi: 10.1126/sciadv.abe2592
Mutoloki S., Jossund T. B., Ritchie G., Munang’andu H. M., Evensen O. (2016). Infectious pancreatic necrosis virus causing clinical and subclinical infections in Atlantic salmon have different genetic fingerprints. Front. Microbiol. 7, 10. doi: 10.3389/fmicb.2016.01393
Nevoux M., Finstad B., Davidsen J. G., Finlay R., Josset Q., Poole R., et al. (2019). Environmental influences on life history strategies in partially anadromous brown trout (Salmo trutta, salmonidae). Fish and Fisheries, 20 (6), 1051–1082. doi: 10.1111/faf.12396
Nylund S., Andersen L., Saevareid I., Plarre H., Watanabe K., Arnesen C. E., et al. (2011). Diseases of farmed Atlantic salmon salmo salar associated with infections by the microsporidian paranucleospora theridion. Dis. Aquat Organ 94 (1), 41–57. doi: 10.3354/dao02313
Nylund A., Jakobsen P. (1995). Sea trout as a carrier of infectious salmon anaemia virus. J Fish Biol. 47 (1), 174–176. doi: 10.1111/j.1095-8649.1995.tb01885.x
Olsen A. B., Hjortaas M., Tengs T., Hellberg H., Johansen R. (2015). First description of a new disease in rainbow trout (Oncorhynchus mykiss (Walbaum)) similar to heart and skeletal muscle inflammation (HSMI) and detection of a gene sequence related to piscine orthoreovirus (PRV). PloS One 10 (7). doi: 10.1371/journal.pone.0131638
Olsvik P. A., Lie K. K., Jordal A.-E. O., Nilsen T. O., Hordvik I. (2005). Evaluation of potential reference genes in real-time RT-PCR studies of Atlantic salmon. BMC Mol. Biol. 6 (1), 21. doi: 10.1186/1471-2199-6-21
Polinski M. P., Vendramin N., Cuenca A., Garver K. A. (2020). Piscine orthoreovirus: Biology and distribution in farmed and wild fish. J. Fish Dis. 43 (11), 1331–1352. doi: 10.1111/jfd.13228
Raynard R. S., Murray A. G., Gregory A. (2001). Infectious salmon anaemia virus in wild fish from Scotland. Dis. Aquat. Organisms 46 (2), 93–100. doi: 10.3354/dao046093
Roberts R. J., Pearson M. D. (2005). Infectious pancreatic necrosis in Atlantic salmon, Salmo salar L. J. Fish Dis. 28 (7), 383–390. doi: 10.1111/j.1365-2761.2005.00642.x
Rolland J. B., Winton J. R. (2003). Relative resistance of pacific salmon to infectious salmon anaemia virus. J. Fish Dis. 26 (9), 511–520. doi: 10.1046/j.1365-2761.2003.00473.x
Sommerset I., Bang Jensen B., Bornø B., Haukaas A., Brun E. (2021). The health situation in Norwegian aquaculture 2020. (online version).
Sommerset I., Walde C. S., Bang Jensen B., Bornø B., Haukaas A., Brun E. (2020). The health situation in Norwegian aquaculture 2019. (online version).
Takano T., Nawata A., Sakai T., Matsuyama T., Ito T., Kurita J., et al. (2016). Full-genome sequencing and confirmation of the causative agent of erythrocytic inclusion body syndrome in coho salmon identifies a new type of piscine orthoreovirus. PloS One 11 (10). doi: 10.1371/journal.pone.0165424
Team R. C. (2013). A Language and Environment for Statistical Computing. R Foundation for Statistical Computing, Vienna, Austria.
Vendramin N., Kannimuthu D., Olsen A. B., Cuenca A., Teige L. H., Wessel O., et al. (2019). Piscine orthoreovirus subtype 3 (PRV-3) causes heart inflammation in rainbow trout (Oncorhynchus mykiss). Veterinary Res. 50. doi: 10.1186/s13567-019-0632-4
Warnes G. R., Bolker B., Lumley T., Johnson R. (2015). gmodels: Various R programming tools for model fitting. 2 (2). Available at: https://rdrr.io/cran/gmodels/.
Wessel O., Braaen S., Alarcon M., Haatveit H., Roos N., Markussen T., et al. (2017). Infection with purified piscine orthoreovirus demonstrates a causal relationship with heart and skeletal muscle inflammation in Atlantic salmon. PloS One 12 (8). doi: 10.1371/journal.pone.0183781
Wessel Ø, Hansen E. F., Dahle M. K., Alarcon M., Vatne N. A., Nyman I. B., et al. (2020). Piscine orthoreovirus-1 isolates differ in their ability to induce heart and skeletal muscle inflammation in Atlantic salmon (Salmo salar). Pathogens 9 (12), 1050. doi: 10.3390/pathogens9121050
Wessel O., Olsen C. M., Rimstad E., Dahle M. K. (2015). Piscine orthoreovirus (PRV) replicates in Atlantic salmon (Salmo salar l.) erythrocytes ex vivo. Veterinary Res. 46. doi: 10.1186/s13567-015-0154
Keywords: piscine orthoreovirus (PRV), brown trout, fry, parr, life stages, susceptibility
Citation: Kannimuthu D, Roh H, Morton HC, Peñaranda MMD, Vossgård A, Hansen T, Fjelldal PG, Karlsbakk E, Fiksdal I, Dahle MK, Berg-Rolness H, Mæhle S, Berhe GD, Nordbø J, Patel S, Madhun A, Grove S and Kvamme BO (2023) Experimental transmission of piscine orthoreovirus-1 (PRV-1) in different life stages of Atlantic salmon (Salmo salar) and brown trout (Salmo trutta). Front. Mar. Sci. 10:1151577. doi: 10.3389/fmars.2023.1151577
Received: 26 January 2023; Accepted: 27 March 2023;
Published: 20 April 2023.
Edited by:
Xuan Dong, Yellow Sea Fisheries Research Institute (CAFS), ChinaReviewed by:
Luis Vargas-Chacoff, Austral University of Chile, ChileLiang Qiu, Yellow Sea Fisheries Research Institute (CAFS), China
Copyright © 2023 Kannimuthu, Roh, Morton, Peñaranda, Vossgård, Hansen, Fjelldal, Karlsbakk, Fiksdal, Dahle, Berg-Rolness, Mæhle, Berhe, Nordbø, Patel, Madhun, Grove and Kvamme. This is an open-access article distributed under the terms of the Creative Commons Attribution License (CC BY). The use, distribution or reproduction in other forums is permitted, provided the original author(s) and the copyright owner(s) are credited and that the original publication in this journal is cited, in accordance with accepted academic practice. No use, distribution or reproduction is permitted which does not comply with these terms.
*Correspondence: Dhamotharan Kannimuthu, ZGhhbW90aGFyYW4ua2FubmltdXRodUBoaS5ubw==
†Present addresses: Anne Vossgård, Sonal Patel - Fish Health Research Group, Lerøy Seafood, Ås, Norway
Sonal Patel, Norwegian Veterinary Institute, Bergen, Norway
‡These authors have contributed equally to this work