- 1Animal Production Department, Faculty of Agriculture, Mansoura University, Al-Mansoura, Egypt
- 2Zoology Department, Faculty of Science, Mansoura University, Al-Mansoura, Egypt
- 3Central Laboratory for Aquaculture Research (CLAR), Abbasa, Abo-Hammad, Egypt
- 4Animal and Fish Production Department, College of Agricultural and Food Sciences, King Faisal University, Al-Ahsa, Saudi Arabia
- 5Fish and Animal Production Department, Faculty of Agriculture (Saba Basha), Alexandria University, Alexandria, Egypt
The hybrid red tilapia (♂Oreochromis niloticus × ♀O. mossambicus) is a promising tilapia fish with high growth rate, market acceptability, and the ability to live in a wide range of salinities, but it is sensitive to low water temperature. Therefore, the objective of this experiment was to improve hybrid red tilapia resistance to chronic cold stress by increasing unsaturated fatty acids and evaluating their effects on fish body weight, hepatosomatic index (HSI), body nutrient composition, liver function enzymes, histopathological alterations, and biomarkers of oxidative stress in the gills and liver. For 98 days, fish were fed four diets based on two dietary fat sources: corn oil (a traditional source) and Aquafat-O® (a commercial product rich in high polyunsaturated fatty acids), in different ratios of 3:0 (T0), 2:1 (T1), 1:2 (T2), and 0:3 (T3), respectively. Subsequently, all groups (T0+, T1, T2, and T3) were subjected to water cold stress at 13 °C for 15 days, with half of the control group serving as a negative control (T0-; at a rearing water temperature of 25-26 °C). Chronic old stress caused significant reductions in fish body weight, HSI, aspartate transaminase and alanine transaminase activities, fat and energy contents, and oxidative stress biomarkers in the liver and gills. Red tilapia subjected to low temperatures showed severely altered histopathological conditions in the liver and gills. However, by gradually increasing the Aquafat-O® ratio in the diet, the negative effects of cold stress were mitigated. Thus, it could be concluded that the beneficial use of Aquafat-O® (as a promising fat source) at a percentage of up to 69.60 g kg-1 is necessary to cope with the cold-water stress for sensitive hybrid red tilapia.
Introduction
Fish are cold-blooded creatures with species-specific comfortable temperature ranges for optimal survival and growth rates. In most fish species, low temperatures cause significant physiological, immunological, and metabolic changes accompanied by morphological, behavioral, and histopathological alterations, which are reflected in growth inhibition, increased infection, and even death of fish (Ibarz et al., 2010; Chebaani et al., 2014; Ma et al., 2015; Younis, 2015; Lima de Almeida et al., 2019; Velmurugan et al., 2019; Yilmaz et al., 2021). Many studies have been conducted to reduce the harmful effects of low water temperature on fish, increase their ability to withstand cold stress, and diminish mortality during the winter season. These attempts involved promoting diets that were introduced before and/or during the cold weather conditions, choosing tilapia strains more resistant to low water temperatures, and improving the husbandry systems (Crab et al., 2009; Agouz et al., 2016; El-Bab et al., 2016; Nobrega et al., 2019; Zhou et al., 2019). The importance of nutritional strategies to mitigate the adverse effects of water-cold stress on different fish species was recently investigated (Cheng et al., 2018; Bacchetta et al., 2020; Ale et al., 2021). The pre-cold feeding and reserved fat in fish play imperative roles in facing the cold stress and reducing metabolic changes (Villanueva et al., 2006).
The cold-stressed fish consume a large amount of oxygen to meet the increasing energy demands and metabolic alternations, causing accumulated electrons at certain points of the respiratory chain, which in turn stimulates the production of reactive oxygen species (ROS) and lipid peroxidation (Pavlović et al., 2010; Qi et al., 2013; Cheng et al., 2018). Malek et al. (2004) stated that cold stress induces desaturation of fatty acids (FAs), causing an increase in ROS. Fish developed defense systems to face oxidative stress include antioxidant enzymes, such as superoxide dismutase (SOD), catalase (CAT), and glutathione (GSH), which are the first lines of defense against the production of ROS (Pandey et al., 2003). Halliwell and Gutteridge (1999) indicated that the SOD and CAT enzymes work to break down superoxide anion and hydrogen peroxide, respectively. The GSH enzyme is activated to prevent oxidative damage (Schlenk et al., 2008). In the same context, Bacchetta et al. (2020) recently revealed that the liver plays the main role in preventing the deleterious effects of oxidative stress. A number of studies show histological changes in the gills and liver of fish exposed to low temperatures, highlighting the importance of performing histological studies as indicators of cold stress (Ibarz et al., 2010; Lima de Almeida et al., 2019; Liu et al., 2020; Zhu et al., 2020; Refaey et al., 2022).
FAs are the primary components of cell membrane structure and function (Tocher, 2003). The type of FAs in the cell membranes is affected by temperature of the water. Fish exposed to low-temperature conditions produce more polyunsaturated fatty acids (PUFAs), monounsaturated fatty acids (MUFAs), and a reduction in saturated fatty acids (SFAs) to maintain cell membrane fluidity (Snyder and Hennessey, 2004; Hsieh and Kuo, 2005; Refaey et al., 2022). This helps the membrane function correctly during exposure to cold stress (Kostetsky et al., 2013), which is called homeoviscous (Corrêa et al., 2018). Many studies found that dietary lipid sources improved the tolerance of various fish species to low temperatures (Craig et al., 1995; Kelly and Kohler, 1999; Huang et al., 2022). Consequently, the most important method used to increase the ability of fish to overcome low temperatures is to increase the energy content of the diet by increasing dietary carbohydrates and fats (Zhou et al., 2019). Fish reared in cooler areas had higher levels of PUFAs (Storelli et al., 1998). For tilapia, He et al. (2015) found that fish reared under low-temperature conditions metabolized SFAs and long-chain PUFAs as energy sources, while their requirements for n-3 PUF increased to cope with suboptimal temperature (Huang et al., 2022). Currently, tilapia feed contains some oils as a fat source, such as corn oil, which contains a high percentage of linoleic acid (LA, 18:2 n-6) and a low percentage of linolenic acid (LNA, 18:3n-3), reducing the nutritional value of fish (Tocher et al., 2010; Ng and Romano, 2013). At the same time, oils rich in n-3 PUFAs, such as fish oil and linseed oil, were scarce and at high prices. Therefore, other alternatives are searched for as a balanced source of fats from LA and ALA.
The ability of tilapia fish to tolerate low temperatures varies according to their type. Nile tilapia can survive in temperatures ranging from 10 to 13°C, whereas Mozambican tilapia are more sensitive to cold temperatures (Cnaani et al., 2000). Therefore, the hybrid red tilapia resulting from the mating of both ♂Oreochromis niloticus × ♀ O. mossambicus is characterized by its sensitivity to low temperatures (Cnaani et al., 2000). The hybrid red tilapia characterized by its ability to live in a wide range of salinities, ranging from 1 to 25 ppt, and it’s attractive color and market acceptability (Watanabe et al., 1997). Therefore, in recent years, the importance of red tilapia has increased in Egypt due to the need to support aquaculture in brackish and marine waters. However, red tilapia fish suffer from low temperature sensitivity. Consequently, the present study aimed to improve the resistance of hybrid red tilapia ♂O. niloticus × ♀ O. mossambicus to chronic cold stress by changing the dietary FAs using different fat sources, such as traditional corn oil and Aquafat-O® (AQ-O), a commercial product rich in PUFAs, in different ratios. Furthermore, we studied their effects on fish body weight, hepatosomatic index, liver functions, chemical composition of the fish body, histopathological alterations, biomarkers of oxidative stress of the gills and the liver of hybrid red tilapia exposed to cold water stress at 13°C for 15 days.
Materials and methods
Fish and experimental procedures
Hybrid red tilapia juveniles [n = 216; with an initial body weight of 11.11 ± 1.03 g (mean ± SD)] were acclimated to the experimental conditions and fed with the control diet for two weeks in the Fish Research Laboratory, Animal Production Department, Faculty of Agriculture, Mansoura University, Egypt. In this study, 12 plastic tanks (500 L in volume) were assigned to four experimental treatments (three replicates per treatment). Fish were stocked at 18 fish per tank. The water temperature was set during the feeding period to 25 – 26°C by a thermostat heater. Each tank was provided with enough aeration to maintain water dissolved oxygen (DO) above 5.0 mg L-1, which was monitored two times a week. While water pH value was 8.19 ± 0.2 as measured by pH-meter (Staffordshire ST15 0SA, UK). The fish wastes were removed by siphoning 30% of the water volume three times a week and replaced with dechlorinated tap water.
Experimental diets and feeding regime
Aquafat-O® is a commercial feed supplement (NOREL MISR, Ataka Industrial Zone, Northern Gulf of Suez, Egypt) that is rich in high PUFAs (oleic acid, linoleic acid, and linolenic acid). The FAs analysis of AQ-O was shown in the footer of Table 1, as mentioned previously in our research (Refaey et al., 2018).
Four experimental diets were prepared using local ingredients that were isonitrogenous and isocaloric and contained different proportions of two fat sources, including CO : AQ-O, at 3:0, 2:1, 1:2, and 0:3, respectively, representing four experimental treatments T0, T1, T2, and T3, respectively (Figure 1). The ingredients of the control diet (CD) were thoroughly mixed with 6% corn oil (CO, a common oil), while the other experimental diets contained the appropriate ratio of CO : AQ-O and were pelleted by a manufacturing machine to obtain a size of 1 mm, dried, and stored at -20°C until use. The chemical analysis of experimental diets was carried out according to AOAC (2016) (Table 1). Fish were hand-fed the experimental diets for 98 days at a rate of 4-5% of their live body weight, twice daily, at 10 a.m. and 2 p.m. The body weight of the fish in each tank was measured every two weeks to adjust the amount of feed introduced.
Cold-tolerance test procedure
At the end of the feeding period, all treatments were exposed to chronic cold stress (mean ± SD; 13.0 ± 1.0°C) for 15 days, as the second part of this study. Fish in the control group (n = 48) were split into two groups. The first group (n = 24) was kept at a constant water temperature of 25-26°C by the thermostat-controlled heater as the negative control treatment (T0-). The second group (n = 24) was a positive control treatment (T0+), in which the fish were exposed to cold water (13.3 ± 1.0°C) (Hofer and Watts, 2002). Also, the other groups (T1, T2, and T3) were exposed to the cold stress test for 15 days (Figure 1). The average initial weights of fish in T0-, T0+, T1, T2, and T3 were (mean ± SD) 65.39 ± 5.07 g, 65.17 ± 5.07 g, 67.82 ± 4.94 g, 65.63 ± 5.77 g, and 68.40 ± 3.52 g, respectively. At a rate of three replicates per treatment (eight fish per glass aquarium), fifteen glass aquariums (length: 90 cm × width: 40 cm × height: 30 cm; with a total volume of 108 L) were used. Each aquarium was filled with dechlorinated tap water and supplied with an air stone connected to the electric air compressor.
The water temperature was reduced gradually at a rate of 2-3°C daily for 5 days with ice plastic bags until it reached the required challenge water temperature of 13.0 ± 1.0°C. This cold condition continued for 10 days in glass aquaria insulated with a thermal layer and using ice chilling when required, which is checked by the thermometer and adjusted every two hours (Soltanian et al., 2014). The experiment was carried out during the winter season, and the ambient lab temperature was 16.0 ± 2°C.
Tested measurements
Measurement of fish body weight and hepatosomatic index
Before and after the cold-tolerance test, fish weight (n = 8 in each replicate) was measured to estimate the change in body weight under the cold-stress conditions. The hepatosomatic index (HSI, %) was individually measured according to the following equation:
Collection of blood samples
Blood samples were collected before and after the cold-tolerance test. Briefly, six fish from each treatment were randomly selected and anesthetized with clove oil (50 mg/L water). Blood samples were collected from caudal blood vessels and centrifuged at 3500 ×g for 20 min. to obtain the clear serum. Serum samples were kept at -20°C. Liver enzyme activities were carried out during 20 days of sample collection. After blood collection, the anesthetized fish killed by mean blood vessels and dissected to get the gills and liver samples, which were stored at -80° C for analysis of oxidative stress. Other samples of the gills and the liver tissues were immediately put in 10% formaldehyde for 24 hours as a fixation process for histological examination.
Measurement of serum and hepatic function enzymes
Serum and hepatic aspartate transaminase (AST) and alanine transaminase (ALT) enzyme activities were determined using the commercial kits produced by Diagnostic System Laboratories, Inc., USA, based on the methods of Reitman and Frankel (1957).
The chemical composition of fish body
The proximate chemical composition of the fish’s whole body was determined prior to and after the cold-tolerance test. Fish (n = 3 per treatment) were randomly collected and kept at –20°C for the proximate analysis of the whole body. The approximate analysis of the moisture, fat, protein, and ash contents was done according to AOAC (2016). The energy content (MJ 100 g-1 DM) was calculated at 23.64 and 39.54 MJ g-1 for protein and fat, respectively.
Histological examination of the gills and the liver
The gills and liver (n = 3 per treatment) were fixed in a 10% neutralized formalin solution, washed with tap water, and dehydrated with graduated levels of alcohol (70, 85, 96, and 99%). Samples were purified with xylene and embedded in paraffin wax. The wax blocks were sectioned into six microns. The sections were stained with hematoxylin (H) and eosin (E) and then subjected to a histological examination of the gills and the liver, according to Roberts (2001). The photomicrographs of the gills and the liver were taken by a phase-contrast microscope, the Leica DM 500, with an ICC50W camera at magnifications of 100× (bar = 100 µm).
Regarding to histometric examination of the gill, the thickness of the gill filament, the length and intensity of gill lamellae were measured. On average, 135 (5 gill filaments × 9 examined fields in each slide × 3 slides) and 288 (12 gill lamellae × 8 examined fields in each slide × 3 slides) gill filament thickness and gill lamellae length, respectively, were measured.
Oxidative stress biomarkers
The gills and liver samples (n = 3 per treatment) were carefully homogenized with an ice-cold buffer (consisting of 5 mM potassium phosphate, 0.9% sodium chloride, and 0.1% glucose; pH 7.4) using a glass homogenizer to yield a 10% homogenate. Afterward, the samples were centrifuged at 4000 ×g for 15 min. at 4°C, and the supernatant was collected and stored at –20°C until used. The oxidative stress biomarkers, including total antioxidant capacity (TAC) and lipid peroxidase (LPO), were determined according to Koracevic et al. (2001), while glutathione (GSH) was measured according to Beutler et al. (1963). Meanwhile, the activities of superoxide dismutase (SOD) and catalase (CAT) were analyzed using colorimetric kits (Randox Laboratories Company, UK) according to the methods described by Nishikimi et al. (1972) and Aebi (1984), respectively.
Statistical analysis
All data of fish body weight, HSI, the chemical composition of the fish body, serum and hepatic AST and ALT activities, the gills histometric parameters, and oxidative stress biomarkers in the gills and the liver were statistically tested using SAS® software (Version 9.1.3 for Windows, SAS, 2006). The significant differences among the treatments were examined by ANOVA followed by the Tukey post hoc test. The differences between before and after exposure to cold stress in each treatment were tested using a paired sample t-test. The differences were considered statistically significant at a probability level< 0.05.
Results
Fish body weight and hepatosomatic index
The data in Figure 2A showed that fish body weight tended to improve with dietary AQ-O. Exposure to cold stress reduced fish body weight in T0+ by 22.24% compared to before exposure. Meanwhile, the final weight of AQ-O supplemented treatments decreased by 8.33%, 10.10%, and 5.94% for T1, T2, and T3, respectively. HSI significantly diminished after the hybrid red tilapia were exposed to the cold-tolerance test compared to before the exposure, especially in fish of T0+ and T1 (Figure 2B; p< 0.05). HSI, on the other hand, improved gradually with increasing AQ-O dietary ratio in T3 (p< 0.05).
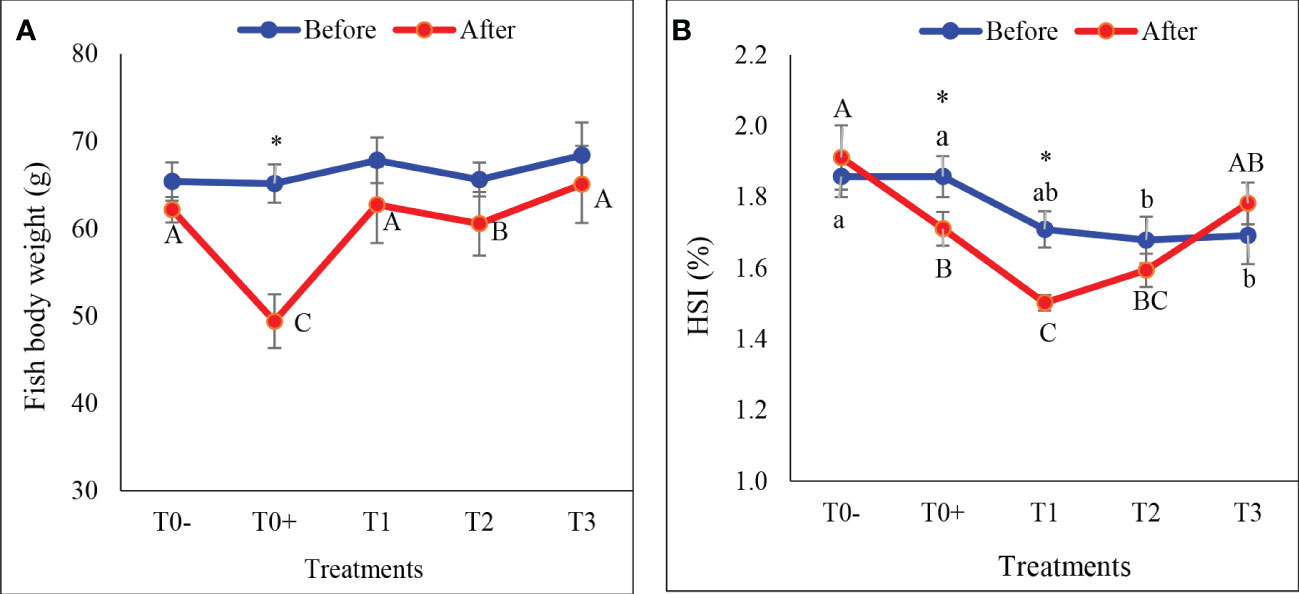
Figure 2 Effect of different ratios of corn oil (CO) and Aquafat-O® (AQ-O) on (A) fish body weight (g), and (B) hepatosomatic index (HSI %) of the hybrid red tilapia fed different ratios of CO: AQ-O and exposed to cold stress. T0- (the negative treatment); T0+ (the positive treatment); T1 (2 CO: 1 AQ-O); T2 (1 CO: 2 AQ-O); T3 (3 CO: 0 AQ-O). Vertical bars indicate the standard errors; means with an asterisk indicate a significant difference between before and after the exposure to cold stress (p< 0.05). Small and capital letters indicate significant differences among treatments before and after exposure to cold stress, respectively.
Serum and liver activities of AST and ALT
Cold stress significantly reduced AST and ALT activities in the serum or liver of cold-stressed red tilapia (Figures 3A–D; p< 0.05). Serum AST and ALT activities decreased significantly in all cold stress treatments (T0+, T1, T2, and T3) compared to T0-, as well as before cold stress (Figures 3A, B; p< 0.05). Under cold stress conditions, the addition of AQ-O reduced the decrease in hepatic AST at rates of 29.67%, 20.24%, 17.82% and 6.81%, and ALT activities at rates of 28.38%, 16.28%, 15.52% and 9.22%, respectively, in treatments T0+, T1, T2 and T3, respectively, compared to before cold stress.
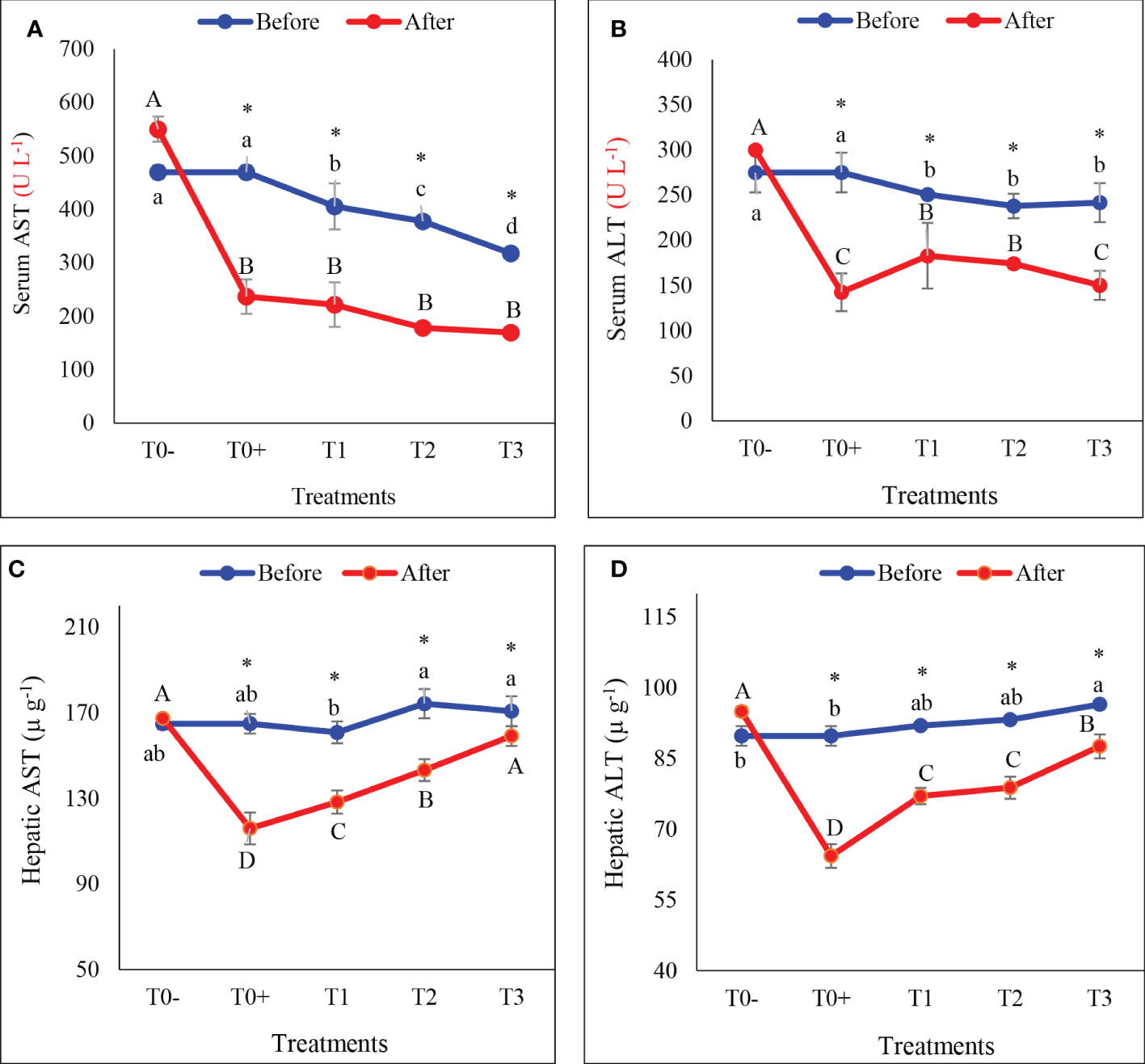
Figure 3 Serum and hepatic activities of aspartate transaminase (AST: A, C, respectively) and alanine transaminase (ALT: B, D, respectively) of hybrid red tilapia fed different ratios of corn oil (CO): Aquafat-O® (AQ-O) and exposed to cold stress. T0- (the negative treatment), T0+ (the positive treatment), T1 (2 CO: 1 AQ-O), T2 (1 CO: 2 AQ-O), T3 (3 CO: 0 AQ-O). Vertical bars indicate the standard errors; means with an asterisk indicate a significant difference between before and after the exposure to cold stress (p< 0.05). Small and capital letters indicate significant differences among treatments before and after the exposure to cold stress, respectively.
Chemical composition of the fish body
The results in Table 2 revealed that cold stress caused a significant increment in moisture and protein contents, and a significant decline in fat and energy contents in the chemical composition of the fish body compared to before exposure to cold stress in all treatments (p< 0.05). For the ash content, T0- and T2 exhibited a significant increase, while T0 + showed a significant reduction in ash after exposure to cold stress (p< 0.05).
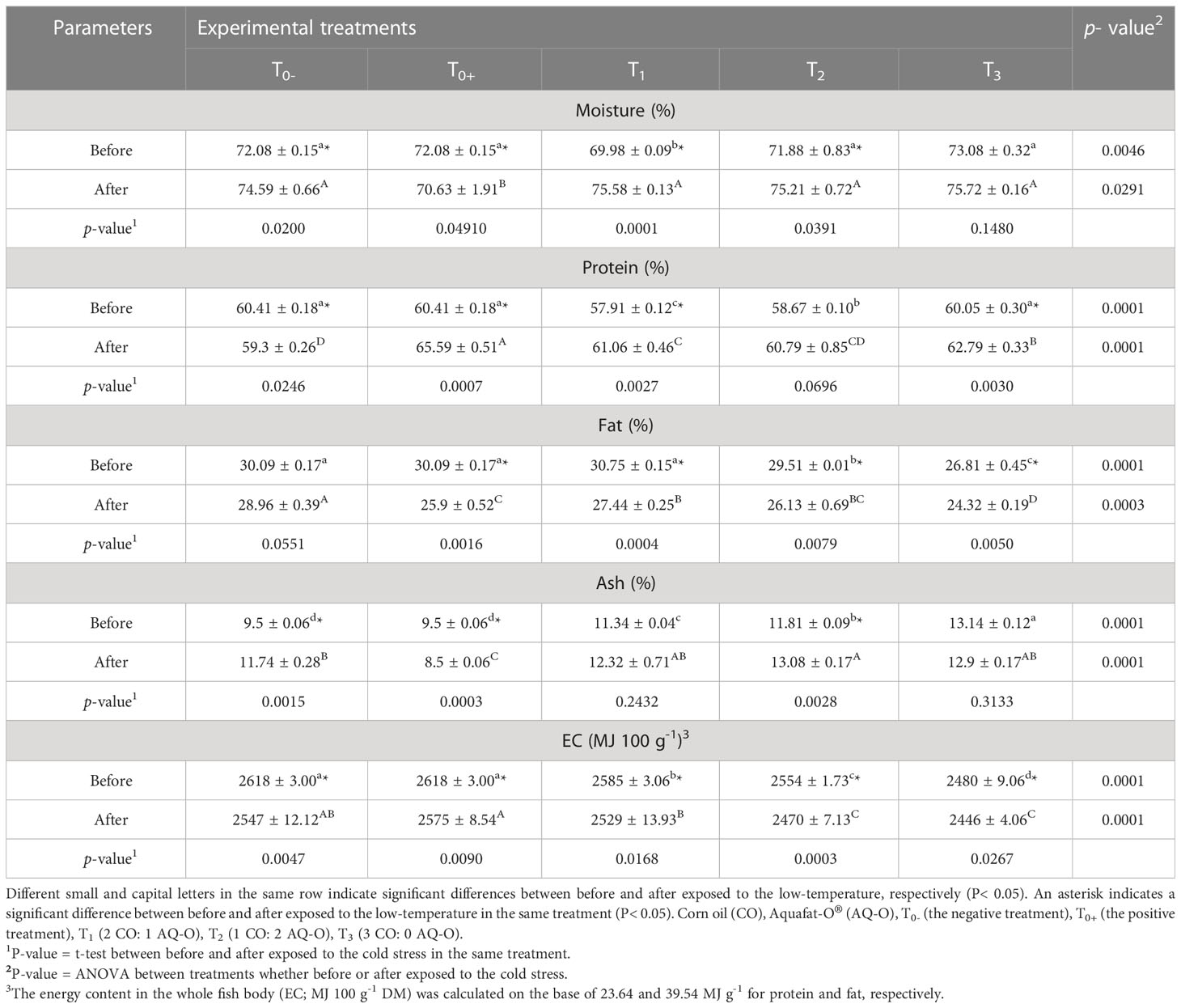
Table 2 Chemical composition of hybrid red tilapia body fed different ratios of corn oil: Aquafat-O® and exposed to chronic cold stress.
Histological alterations of the gills
Figures 4A–E showed the histological alterations of the gills of red tilapia fed different ratios of CO: AQ-O and reared under cold stress conditions. The negative treatment (T0-) revealed normal gill filament and secondary lamellae structure (Figure 4A). Cold stress causes severe alterations in the gills, which can be seriously alleviated by increasing the AQ-O ratio. Histopathological changes involved short secondary lamellae, the tips of the gill filament being thinner, the absence of secondary lamellae, and an increase in cartilage tissue within the gill filament, which are present in treatments T0+ and T1 (Figures 4B, C). However, T2 showed hyperplasia and shortening of the gill lamellae (Figure 4D). An improvement in the structure of the secondary lamellae and a decrease in the thickness of the gill filament were clearly observed in T3 (Figure 4E).
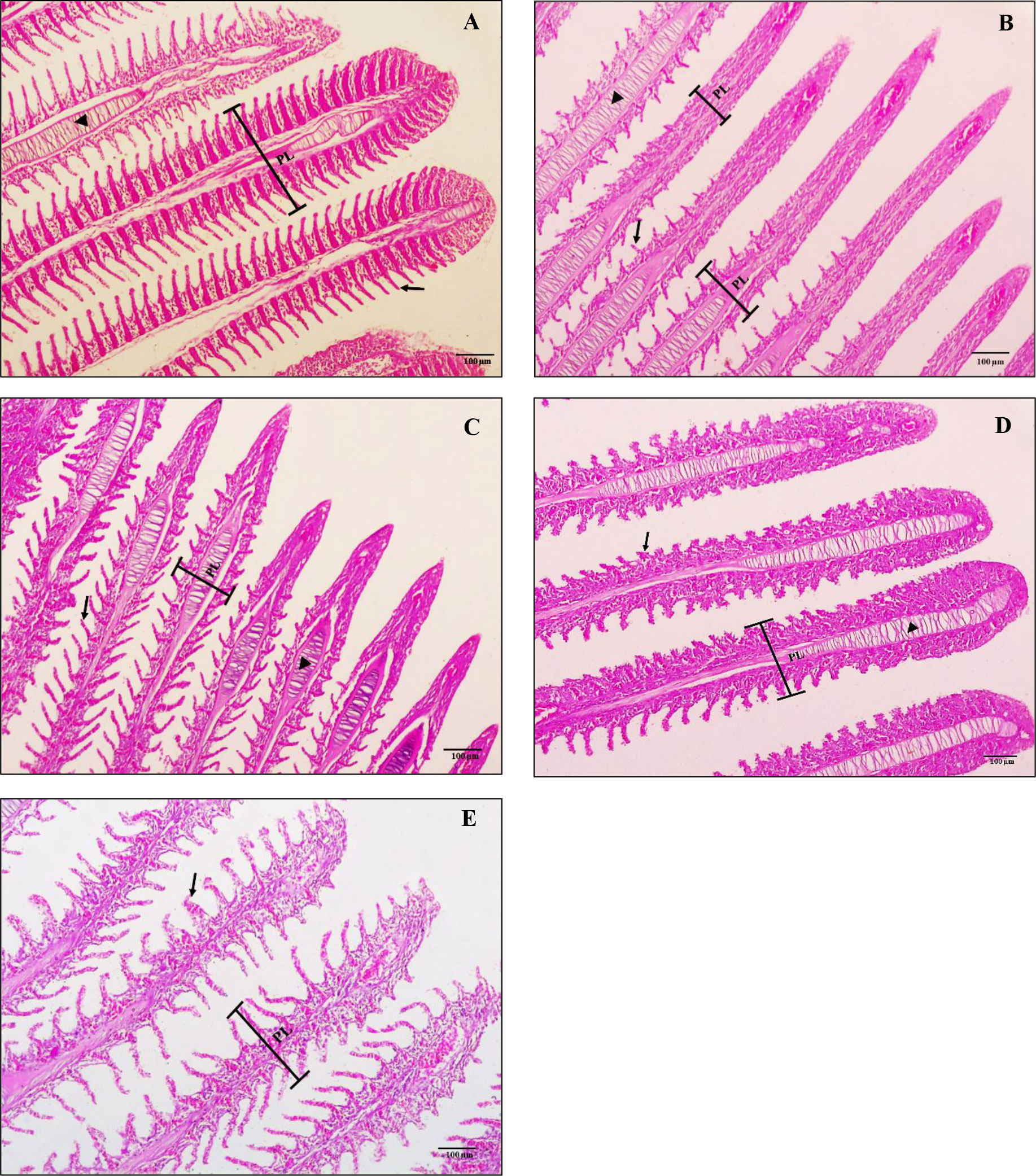
Figure 4 Screening cross-section of the gills of hybrid red tilapia fed different ratios of corn oil (CO): Aquafat-O® (AQ-O) and exposed to cold stress. (A) the negative treatment (T0-), (B) the positive treatment (T0+), (C) T1 (2 CO: 1 AQ-O), (D) T2 (1 CO: 2 AQ-O), and (E) T3 (3 CO: 0 AQ-O). PL: primary lamellae; secondary lamellae (arrows); cartilaginous tissue (arrowhead) (×100; bar = 100µm; H and E stains).
Histometric properties of the gills
There are noticeable variations (p< 0.05) in the gill lamellae length, gill filaments thickness, and intensity of the gill lamellae per 1 mm among red tilapia fed different ratios of CO: AQ-O, and exposed to cold stress, as shown in Table 3. Exposure to cold stress caused a significant decrease in the length of the gill lamellae, the thickness of the gill filaments, and intensity of gill lamellae per 1 mm (p< 0.05). The positive treatment (T0+) recorded the lowest values of gill lamellae length and intensity of gill lamellae per 1 mm, whereas T1 showed the lowest value of gill filaments thickness among all treatments. At the same time, these parameters improved with an increasing AQ-O ratio (p< 0.05).
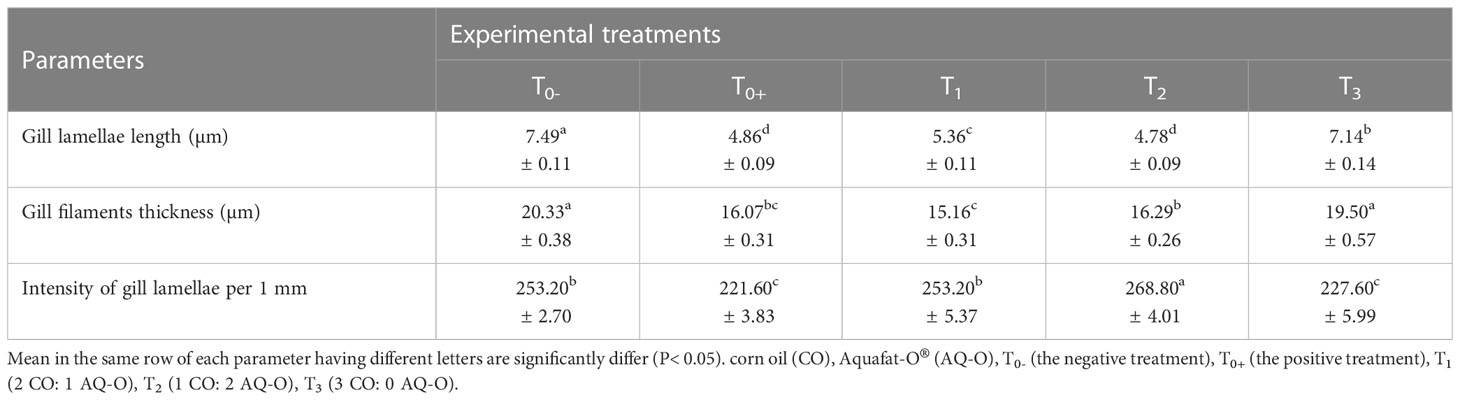
Table 3 Histometric properties of the hybrid red tilapia gills fed different ratios of corn oil: Aquafat-O® and exposed to cold stress.
Histological changes in the liver tissue
Liver histological alterations of red tilapia unexposed or exposed to cold stress and fed different ratios of CO : AQ-O were demonstrated in Figures 5A–E. The fish in T0- showed the normal structure of the hepatocytes arranged around the central vein (CV) (Figure 5A). There were severe alterations in the liver tissues exposed to cold stress, especially in T0+ (Figure 5B), such as thickening and severe elongation of the CV, severe degeneration and necrosis of the hepatocytes, and severe infiltration and hemolysis. With the increasing dietary CO : AQ-O ratio, the severity of the histological changes in the liver of other treatments (T1, T2, and T3) was reduced relative to T0+ (Figures 5C–E).
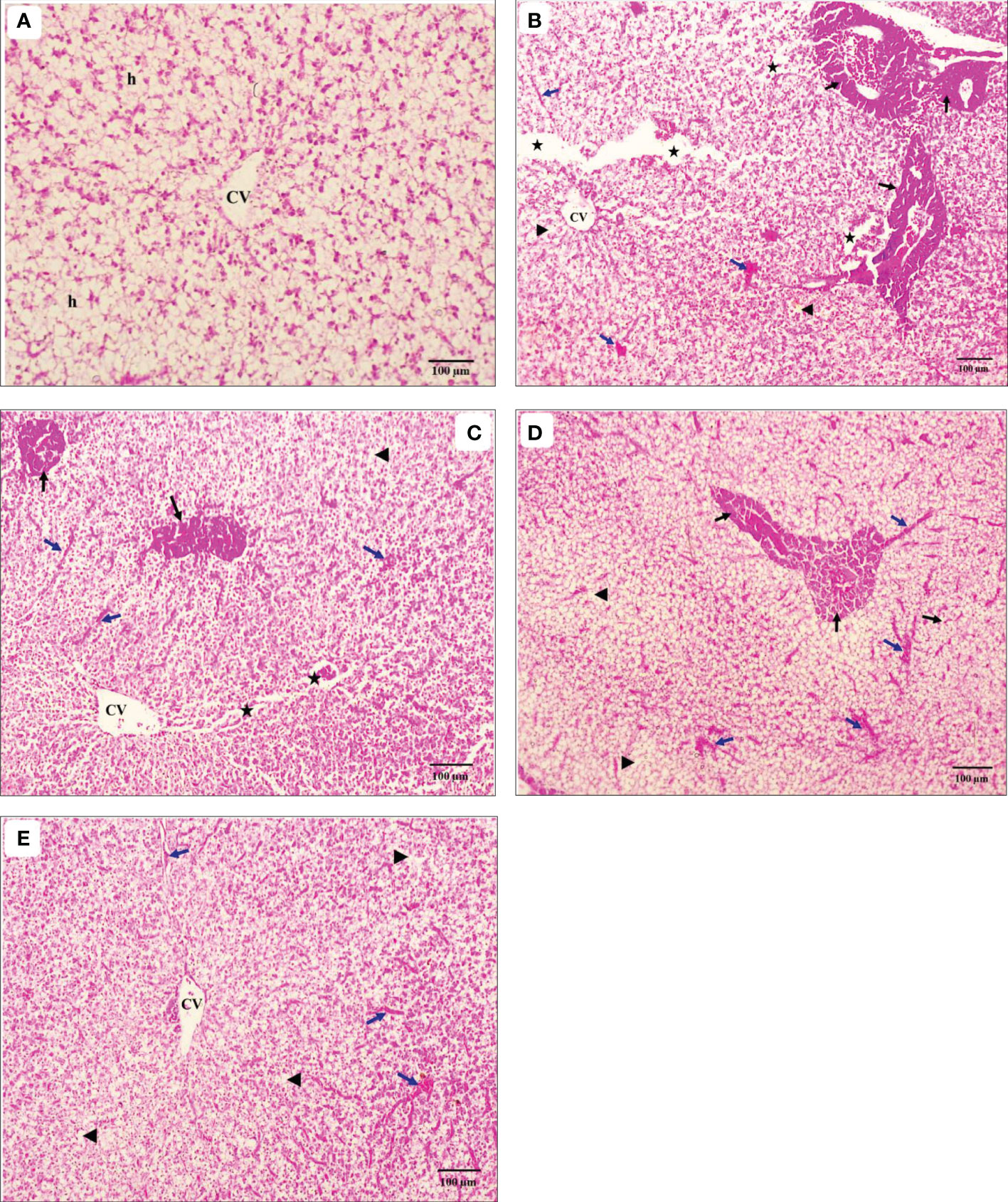
Figure 5 Light micrograph of the liver transverse sections of hybrid red tilapia fed different ratios of corn oil (CO): Aquafat-O® (AQ-O) and exposed to cold stress. (A) the negative treatment (T0-), (B) the positive treatment (T0+), (C) T1 (2 CO: 1 AQ-O), (D) T2 (1 CO: 2 AQ-O), and (E) T3 (3 CO: 0 AQ-O) (×100; bar = 100µm; H & E stains). h: hepatocytes; CV: the central vein (black arrows); necrosis: (arrowheads); degeneration (stars); infiltration and hemolysis (blue arrows) of the hepatocytes.
Oxidative stress biomarkers in the liver
There were significant differences in liver TAC and LPO levels, and the activities of CAT, SOD and GSH before and after the exposure of red tilapia to cold stress (Figures 6A–E; p< 0.05). Compared to before exposure to cold stress, there was a significant decrease in all biomarkers of oxidative stress in all treatments. In addition, increasing AQ-O led to significantly enhanced TAC (18.95%, 13.96%, 0.02%, and -6.92%) and the activities of SOD (18.24%, 9.26%, 9.48%, and 7.64), CAT (30.27%, 19.26%, 18.02%, and 2.77%), and GSH (40.19%, 19.26%, 27.95%, and 18.78%) in T0+, T1, T2, and T3, respectively. However, liver LPO increased significantly in T0+ and T1, then decreased gradually in treatments with T2 and T3 (Figure 6B; p< 0.05).
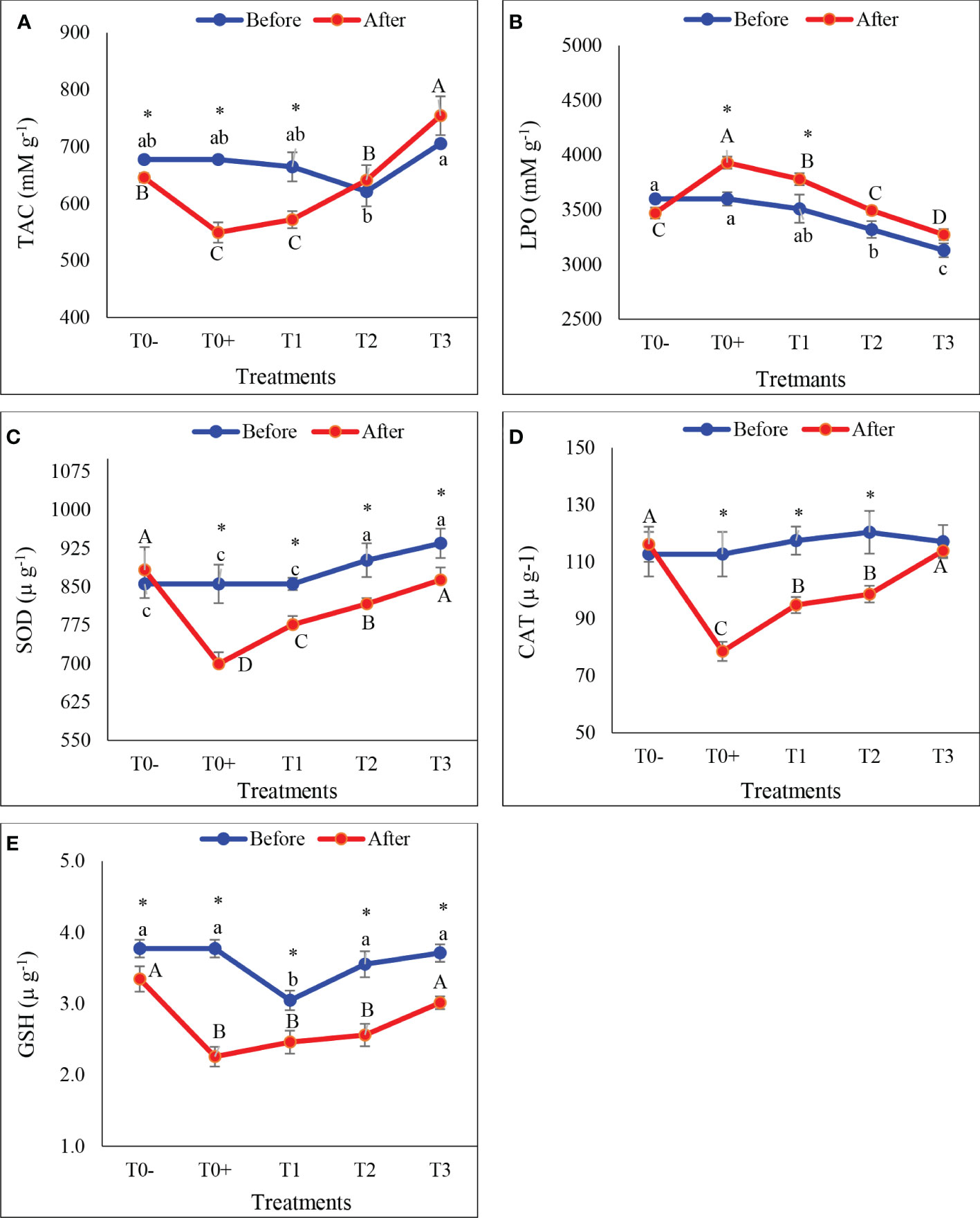
Figure 6 The hepatic total antioxidant capacity (A: TAC), lipid peroxidase (B: LPO), superoxide dismutase (C: SOD), catalase (D: CAT, and glutathione (E: GSH) of red tilapia fed different ratios of corn oil (CO): Aquafat-O® (AQ-O) and exposed to cold stress. T0- (the negative treatment), T0+ (the positive treatment), T1 (2 CO: 1 AQ-O), T2 (1 CO: 2 AQ-O), T3 (3 CO: 0 AQ-O). Vertical bars indicate the standard errors; mean with an asterisk indicate a significant difference between before and after the exposure to cold stress (p< 0.05). Small and capital letters indicate significant differences among treatments before and after the exposure to cold stress, respectively.
Oxidative stress biomarkers in the gills
The effect of different CO: AQ-O ratios on oxidative stress biomarkers in the gill tissues of red tilapia exposed to cold stress are illustrated in Figures7A–E. Cold stress led to a significant decrease in TAC (Figure 7A) and the activities of SOD (Figure 7C), CAT (Figure 7D), and GSH (Figure 7E), and a significant increase in the concentration of LPO (Figure 7B; P< 0.05). The lowest values of TAC and activities of SOD, CAT, and GSH and the highest value of LPO were recorded in T0+ among all treatments in cases after and before exposure to cold stress. TAC levels and SOD, CAT, and GSH activities in the gills were found to be highest in T0- and T3 treatments, among others.
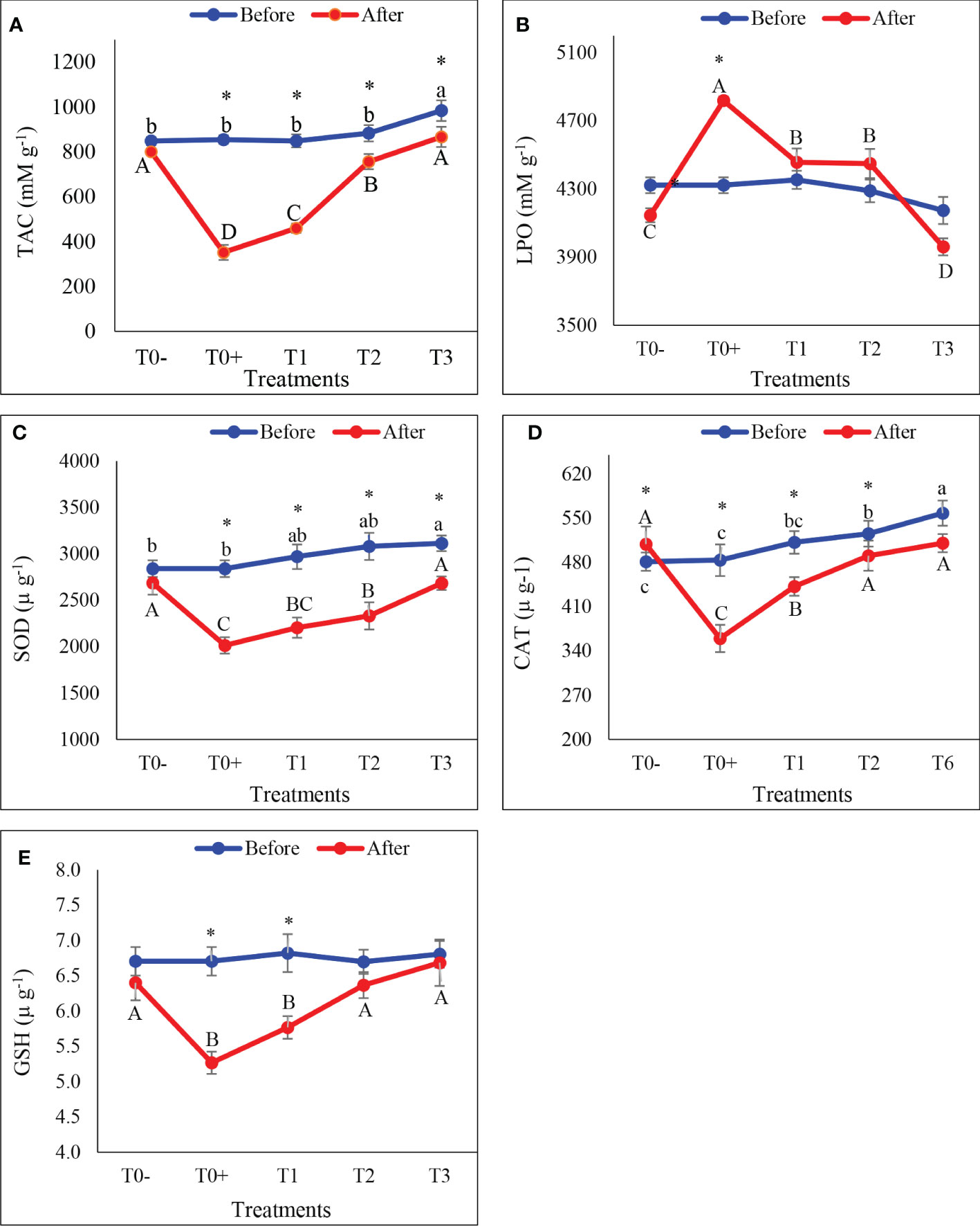
Figure 7 the gills total antioxidant capacity (A: TAC), lipid peroxidase (B: LPO), superoxide dismutase (C: SOD), catalase (D: CAT, and glutathione (E: GSH) of red tilapia fed different ratios of corn oil (CO): Aquafat-O® (AQ-O) and exposed to cold stress. T0- (the negative treatment); T0+ (the positive treatment); T1(2 CO: 1 AQ-O); T2 (1 CO: 2 AQ-O); T3 (3 CO: 0 AQ-O). Vertical bars indicate the standard errors; means with an asterisk indicate a significant difference between before and after the exposure to cold stress (p ≤ 0.05). Small and capital letters indicate significant differences among treatments before and after the exposure to cold stress, respectively.
Discussion
The change in the body weight is one of the necessary indicators to judge the suitability of the environmental conditions surrounding the fish. In the present study, red tilapia exposed to low temperatures (13.0°C) had a significant decline in body weight, particularly in the case of T0+. This decrease is the result of the fish fasting during the cold-stress test and using their stored energy to withstand harsh conditions. This encourages the body to use its stored energy, which is represented by lack of body weight (Wendelaar Bonga, 1997; Ibarz et al., 2010; Zhu et al., 2020). This finding lends support to the fish that have been exposed to cold stress’ impressive reduction in body fat content and liver weight. Tort et al. (2004) also indicated that rearing gilthead sea bream at 13.0°C promotes the use of body reserves in conjunction with changing the rate of metabolism to result in growth arrest. Furthermore, there were no significant differences in fish body weight between treatments containing AQ-O (T1, T2, and T3) and T0-, indicating that red tilapia’s ability to withstand cold stress has improved. This may be attributed to the dietary AQ-O containing high and balanced values of PUFAs, such as LA (18:2n-6) and LNA (18:3n-3), needed to achieve normal growth in fish (Kim et al., 2007). Inversely, He et al. (2017) found that the body weight of Malaysian red tilapia did not significantly change when reared at different low temperatures.
HSI is influenced by water temperature (Ma et al., 2015). The negative effect of cold stress on HSI has been demonstrated in many fish species, such as juvenile Piaractus mesopotamicus (Bacchetta et al., 2020), yellow drum Nibea albiflora (Zhu et al., 2020), and sea bass Dicentrarchus labrax (Katsika et al., 2021). The same phenomenon was observed in this study. On the contrary, Rossi et al. (2017) indicated that the HSI of Hoplosternum littorale increased with decreasing water temperature (10.0°C) for 24 hours (as an acute effect) or for 21 days (as a chronic effect). Under low-temperature conditions, Younis (2015) indicated that O. aureus liver tissue depends on lipids as an energy source. The study showed that the increase in the AQ-O ratios resulted in an improvement in HSI by optimising the use of cold energy and reducing the depletion of the energy stores in the liver. These are in harmony with the current findings regarding liver enzyme activities and the liver’s histological characteristics.
Red tilapia that had been exposed to cold stress settings in the current study showed a clear decrease in the activity of the AST and ALT enzymes in serum or the liver tissue, compared to before exposure to cold stress conditions, which is a sign of liver injury. The liver’s histological characteristics in the current study supported these findings. Changes in ALT and AST activity were used as indicators of liver impairment (Lemaire et al., 1991). The reduced activity of AST and ALT may be due to decreased amino acid metabolism in fish to preserve amino acids under extreme cold stress (Younis, 2015). As in Nile tilapia, O. mossambicus, and their hybrids, both AST and ALT activation decreased after exposure to cold stress (12°C) (Yilmaz et al., 2021). In this regard, Younis (2015) indicated that ALT activity in O. aureus recorded the lowest values in fish acclimated to lower temperatures (18°C). However, this finding is not consistent with those obtained by some studies that have shown a sharp increase in liver enzyme activity in Nile tilapia exposed to cold stress (13.0°C for 24-72 hours) (Liqin et al., 2016; Panase et al., 2018). This variance in the outcomes among studies may be due to the differences in the exposure period to cold-stress conditions.
Lipid metabolism in fish is affected by water temperature (Glencross, 2009), whereas greater metabolic alterations are caused by chronic thermal exposure than by acute exposure (Lermen et al., 2004). He et al. (2015) revealed that cold-stressed GIFT tilapia utilized lipids more than proteins as a source of energy. According to the current results, fish need more energy to deal with cold stress since it causes the body’s fat and energy levels to decrease across all treatments. It was also observed that the rate of loss of body fat decreased with an increased AQ-O ratio, indicating the use of AQ-O as an energy source or improving the fish’s ability to cope with cold stress, which reduces its use of energy. In this respect, Hassan et al. (2013) demonstrated that freshwater fish species lose about 20% of their fat at low temperatures. Additionally, fat contributes to cell membrane synthesis and the maintenance of fluidity and permeability in cells (Weber and Bosworth, 2005), improving the fish’s tolerance to cold stress.
Fish gills have a large external surface area in contact with the external environment, especially water temperature, which makes them sensitive to any changes in the aquatic environment (Koppang et al., 2015), as well as helps in physiological homeostasis under cold stress conditions. Ibarz et al. (2010) and Lima de Almeida et al. (2019) reported that low temperatures have adverse effects on the histological structure of the gills. In this regard, Liu et al. (2020) discovered that tiger barb gills exposed to cold water showed shrinkage, membrane contraction, and even fracture. Cold-stressed red tilapia in T0+ showed sharp changes in the histology and histometric parameters of the gills, with short secondary lamellae length, thinner gill filament tips, and a lack of secondary lamellae. Meanwhile, the addition of AQ-O helps in mitigating the severity of the harmful effects, especially in T3, indicating that adaptation to low temperature occurs. This may be due to high levels of PUFAs that play the main role in maintaining cell membrane fluidity (Cengiz et al., 2012), which is reflected in protecting the gill membrane and adapting to cold stress (Nobrega et al., 2017; Corrêa et al., 2018; Nobrega et al., 2019). In this context, Abdel-Ghany et al. (2019) found that Nile tilapia suffering from cold stress reduces SFAs and increases PUFAs such as n-6 and n-3 to protect cell membranes.
The fish liver is a vital organ involved in several physiological functions, such as body metabolic homeostasis, serum protein synthesis, and detoxification (Bruslé and Anadon, 1996). Recently, Liu et al. (2020) observed that tiger barb fish exposed to cold stress showed severe fatty acid degeneration induced in the hepatocytes. In the current study, cold-stressed red tilapia showed severe changes in liver histopathology, particularly in T0+. However, increasing the AQ-O ratio pointedly reduced the severity of the histopathological changes induced by cold stress. Despite the fish liver being protected from direct exposure to low temperatures, these changes may be due to its major role in metabolism and energy storage (Wolf and Wolfe, 2005), which may help it cope with cold stress. On the other hand, the severity of histological changes in the liver of fish exposed to cold stress in the present study may be due to the sensitivity of red tilapia fish to low temperatures compared to other tilapia species (Cnaani et al., 2000). The observed liver dysfunction in the current study was reflected in low concentrations of liver enzyme activities in serum and liver tissues. Furthermore, under long-term stress conditions, oxidative stress weakens fish resistance and causes cell damage (Dawood et al., 2021). This is confirmed by the current findings, which show severe histological alterations in both the gills and liver tissues of red tilapia suffering from cold stress and are consistent with the results of oxidative stress biomarkers. Moreover, increasing the AQ-O ratio alleviated the severity of liver histological changes and improved the red tilapia’s resistance to cold stress conditions. Similarly to the current findings, Contessi et al. (2006) mentioned several changes in hepato-pancreatic tissue, such as atrophy, degeneration, and granulocyte infiltration. In addition, severe histological alterations in the liver tissue, such as discoloration, degeneration, fat deposition, steatosis, and infiltration of hepatocytes from cold-stressed sea bream have been detected (Gallardo et al., 2003), reflecting the hepatic dysfunctions.
ROS production increased as the water temperature decreased (Cheng et al., 2018). Cold stress stimulated ROS production in numerous species of fish, such as Etroplus suratensis (Joy et al., 2017), adult zebrafish (Wu et al., 2015), and juvenile P. mesopotamicus (Bacchetta et al., 2020), which were exposed to low temperature for 24 hours. Furthermore, in Malaysian red tilapia, the activities of SOD and malondialdehyde (MDA) increased with decreasing water temperature (He et al., 2017) as a defense mechanism against the production of ROS. Cold stress, according to our findings, decreased TAC and CAT, SOD, and GSH activity while increasing LPO concentration in the liver and gill tissues. These adverse effects on oxidative stress biomarkers may be due to the long exposure period to cold stress (15 days). Furthermore, current findings demonstrated that those biomarkers of oxidative stress in the gills of cold-stressed red tilapia were higher than in the liver, which could be due to the gills being exposed directly to low temperatures, leading to an increase in the degree of oxidative stress. Therefore, red tilapia increased antioxidant enzyme activity levels in the gills compared to those in the liver to adapt to low temperatures. Yilmaz et al. (2021) found significant changes in the SOD and CAT activities of Nile tilapia, O. mossambicus, and their hybrids exposed to cold water. Haptic SOD, CAT, and GSH-Px activities, as well as GSH levels, increased in GIFT O. niloticus fish exposed to cold water stress at 13 degrees Celsius (He et al., 2015). Shi et al. (2015) reported that when GIFT O. niloticus was exposed to cold water stress (15 C for 5 days), hepatic SOD activity peaked at the 24th hour and CAT activity did not change. These findings also agree with those obtained by Bouchard and Guderley (2003) for rainbow trout.
At the same time, the increase in the AQ-O ratio showed a gradual improvement in oxidative stress biomarkers and a reduction in LPO concentration, indicating an increase in the immunity of red tilapia to cold stress. This enhancement may be due to the availability of PUFAs that help to repair membrane fluidity and diminish the generation of ROS (Turchini et al., 2009). Phospholipids are the most abundant component of all cell membranes, and variations in the composition of phospholipid FA play an important role in temperature changes. According to Kostetsky et al. (2013), maintaining the liquid-crystal state of the lipid matrix through an increase in the proportions of PUFAs and MUFAs and a decrease in SFAs enables the membrane to continue operating normally. From the previous review, the addition of AQ-O promotes the ability of red tilapia fish to adapt to cold stress by increasing TAC and the activities of CAT, SOD, and GSH.
Conclusion
Cold stress causes obvious reductions in body weight and HSI, as well as clear changes in the proximate body chemical composition in hybrid red tilapia. Chronic cold stress changed liver function enzymes, as well as histopathological and oxidative stress biomarkers in the gills and liver. Meanwhile, a 69.60% dietary Aquafat-O® ratio reduced the severity of negative changes caused by cold stress conditions. Finally, these results help low-temperature-sensitive species, such as hybrid red tilapia, adapt to and resist cold stress conditions, as well as improve their ability to tolerate cold during the overwintering season.
Data availability statement
The raw data supporting the conclusions of this article will be made available by the authors, without undue reservation.
Ethics statement
The animal study was reviewed and approved by Ethics and rules guide for the use of experimental animals in scientific research, Mansoura University, Egypt (MU - ICUC).
Author contributions
MR: conceptualization, methodology, investigation, software, data curation, visualization, writing- original draft preparation. AhM: conceptualization, methodology, supervision, validation, writing- reviewing and editing, project administration. ME-K: investigation, data curation, project administration, formal analysis, resources. OZ: investigation, data curation, validation, formal analysis, software. AbM: software, validation, visualization, funding acquisition, writing- reviewing and editing. All authors contributed to the article and approved the submitted version.
Funding
This work was supported by the Deanship of Scientific Research, Vice Presidency for Graduate Studies and Scientific Research, King Faisal University, Saudi Arabia [GRANT2719].
Acknowledgments
The authors would like to appreciate the continuous help of staff members of the Animal Production Department, Faculty of Agriculture, Mansoura University, and Central Laboratory for Aquaculture Research, Abbasa, Abo-Hammad, Egypt.
Conflict of interest
The authors declare that the research was conducted in the absence of any commercial or financial relationships that could be construed as a potential conflict of interest.
Publisher’s note
All claims expressed in this article are solely those of the authors and do not necessarily represent those of their affiliated organizations, or those of the publisher, the editors and the reviewers. Any product that may be evaluated in this article, or claim that may be made by its manufacturer, is not guaranteed or endorsed by the publisher.
References
Abdel-Ghany H. M., El-Sayed A. M., Ezzat A. A., Essa M. A., Helal A. M. (2019). Dietary lipid sources affect cold tolerance of Nile tilapia (Oreochromis niloticus). J. Therm. Biol. 79, 50–55. doi: 10.1016/j.jtherbio.2018.11.009
Aebi H. (1984). Catalase in vitro. Methods Enzymol. 105, 121–126. doi: 10.1016/S0076-6879(84)05016-3
Agouz H., Soltan M., Esaid A. E., El-Qabatti A. E. (2016). Effect of feed supplementation with l-carnitine on growth and cold tolerance of the Nile tilapia, Oreochromis niloticus. Egypt. J. Aquat. Biol. Fish. 20, 67–75. doi: 10.21608/EJABF.2016.10608
Ale A., Bacchetta C., Rossi A. S., Scarabotti P. A., Cazenave J. (2021). Low temperature stress in a cultured fish (Piaractus mesopotamicus) fed with Pyropia columbina red seaweed-supplemented diet. Fish Physiol. Biochem. 47, 829–839. doi: 10.1007/s10695-021-00944-7
Almoselhy R. I. M., Eid M. M., Abd El-Baset W. S., Aboelhassan A. F. A. (2021). Determination of 3-MCPD in some edible oils using GC-MS/MS. Egypt. J. Chem. 64 (3), 1639–1652. doi: 10.21608/EJCHEM.2021.64084.3373
AOAC (2016). Official methods of analyses. 20th edn (Washington, DC., USA: Association of Official Analytical Chemist).
Bacchetta C., Ale A., Rossi A. S., Karakachoff M., Cazenave J. (2020). Effects of cold stress on juvenile Piaractus mesopotamicus and the mitigation by β-carotene. J. Therm. Biol. 88, 102497. doi: 10.1016/j.jtherbio.2019.102497
Beutler E., Duron O., Kelly B. M. (1963). An improved method for the detection of blood glutathione. J. Lab. Clin. Med. 61, 882–888.
Bouchard P., Guderley H. (2003). Time course of the response of mitochondria from oxidative muscle during thermal acclimation of rainbow trout, Oncorhynchus mykiss. J. Exp. Biol. 206 (19), 3455–3465. doi: 10.1242/jeb.00578
Bruslé J., Anadon G. G. (1996). The structure and function of fish liver. Fish Morphol. 76, 545–551. doi: 10.1201/9780203755990-6
Cengiz E. I., Kan Y., Kizmaz V., Başhan M., Yanar M. (2012). The protective role of vitamin e on the fatty acid composition of phospholipid structure in gill and liver tissues of Oreochromis niloticus exposed to deltamethrin. Ecotoxicol. Environ. Saf. 80, 381–385. doi: 10.1016/j.ecoenv.2012.04.012
Chebaani N., Guardiola F. A., Sihem M., Nabil A., Oumouna M., Meseguer J., et al. (2014). Innate humoral immune parameters in Tilapia zillii under acute stress by low temperature and crowding. Fish Physiol. Biochem. 40, 797–804. doi: 10.1007/s10695-013-9886-3
Cheng C., Guo Z., Wang A. (2018). The protective effects of taurine on oxidative stress, cytoplasmic free-Ca2+ and apoptosis of pufferfish (Takifugu obscurus) under low temperature stress. Fish Shellfish Immunol. 77, 457–464. doi: 10.1016/j.fsi.2018.04.022
Cnaani A., Gall G., Hulata G. (2000). Cold tolerance of tilapia species and hybrids. Aquac. Int. 8, 289–298. doi: 10.1023/A:1009299109614
Contessi B., Volpatti D., Gusmani L., Galeotti M. (2006). Evaluation of immunological parameters in farmed gilthead sea bream, Sparus aurata l., before and during outbreaks of ‘winter syndrome’. J. Fish Dis. 29, 683–690. doi: 10.1111/j.1365-2761.2006.00765.x
Corrêa C. F., Nobrega R. O., Block J. M., Fracalossi D. M. (2018). Mixes of plant oils as fish oil substitutes for Nile tilapia at optimal and cold suboptimal temperature. Aquaculture 497, 82–90. doi: 10.1016/j.aquaculture.2018.07.034
Crab R., Kochva M., Verstraete W., Avnimelech Y. (2009). Bio-flocs technology application in over-wintering of tilapia. Aquac. Eng. 40, 105–112. doi: 10.1016/j.aquaeng.2008.12.004
Craig S. R., Neill W. H., Gatlin D. M. (1995). Effects of dietary lipid and environmental salinity on growth, body composition, and cold tolerance of juvenile red drum (Sciaenops ocellatus). J. Fish. Physiol. Biochem. 14, 49–61. doi: 10.1007/BF00004290
Dawood M. A. O., Noreldin A. E., Sewilam H. (2021) Long term salinity disrupts the hepatic function, intestinal health, and gills antioxidative status in Nile tilapia stressed with hypoxia. Ecotoxicol. Environ. Saf. 220, 112412. doi: 10.1016/j.ecoenv.2021.112412
El-Bab A. F. F., Mostafa M. A., Hassan A. S. (2016). Effect of dietary protein and over-wintering regimes on survival and growth performance of Nile tilapia, Oreochromis niloticus. Glob. Vet. 17 (6), 513–520. doi: 10.5829/idosi.gv.2016.513.520
Gallardo M. A., Sala-Rabanal M., Ibarz A., Padros F., Blasco J., Fernandez-Borras J., et al. (2003). Functional alterations associated with ‘Winter syndrome’ in gilthead sea bream (Sparus aurata). Aquaculture 223, 15–27. doi: 10.1016/S0044-8486(03)00164-9
Glencross B. D. (2009). Exploring the nutritional demand for essential fatty acids byaquaculture species. Rev. Aquac. 1, 71–124. doi: 10.1111/j.1753-5131.2009.01006.x
Halliwell B., Gutteridge J. M. C. (1999). “Free radicals in biology and medicine,” in Free radicals in biology and medicine, 3rd edition. Eds. Halliwell B., Gutteridge J. M. C. (Oxford: Oxford University Press), 1–25.
Hassan B., El-Salhia M., Khalifa A., Assem H., Al Basomy A., El-Sayed M. (2013). Environmental isotonicity improves cold tolerance of Nile tilapia, Oreochromis niloticus, in Egypt. Egypt. J. Aquat. Res. 39, 59–65. doi: 10.1016/j.ejar.2013.03.004
He J., Qiang J., Yang H., Xu P., Zhu Z. X., Yang R. Q. (2015). Changes in the fatty acid composition and regulation of antioxidant enzymes and physiology of juvenile genetically improved farmed tilapia, Oreochromis niloticus (L.), subjected to short-term low temperature stress. J. Therm. Biol. 53, 90–97. doi: 10.1016/j.jtherbio.2015.08.010
He Y.-f., Wang L.-m., Zhu W.-b., Dong Z.-j., Liu N. (2017). Effects of salinity on cold tolerance of Malaysian red tilapia. Aquac. Int. 25, 777–792. doi: 10.1007/s10499-016-0077-y
Hofer SC, Watts SA (2002). Cold tolerance in genetically male tilapia (GMT®), Oreochromis niloticus. World Aquaculture-Baton Rouge 33 (2), 19–22.
Hsieh S. L., Kuo C. M. (2005). Stearoyl-CoA desaturase expression and fatty acid composition between milkfish (Chanos chanos) and grass carp (Ctenopharyngodon idella) during cold acclimation. Comp. Biochem. Physiol. Part B. 141, 95–101. doi: 10.1016/j.cbpc.2005.02.001
Huang X., Chen F., Guan J., Xu C., Li Y., Xie D. (2022). Beneficial effects of re-feeding high α-linolenic acid diets on the muscle quality, cold temperature and disease resistance of tilapia. Fish Shellfish Immunol. 126, 303–310. doi: 10.1016/j.fsi.2022.05.053
Ibarz A., Padrós F., Gallardo M.Á., Fernández-Borràs J., Blasco J., Tort L. (2010). Low-temperature challenges to gilthead sea bream culture: review of cold-induced alterations and ‘Winter syndrome’. Rev. Fish Biol. Fish. 20, 539–556. doi: 10.1007/s11160-010-9159-5
Joy S., Alikunju A. P., Jose J., Sudha H. S. H., Parambath P. M., Puthiyedathu S. T., et al. (2017). Oxidative stress and antioxidant defense responses of Etroplus suratensis to acute temperature fluctuations. J. Therm. Biol. 70, 20–26. doi: 10.1016/j.jtherbio.2017.10.010
Katsika L., Huesca Flores M., Kotzamanis Y., Estevez A., Chatzifotis S. (2021). Understanding the interaction effects between dietary lipid content and rearing temperature on growth performance, feed utilization, and fat deposition of sea bass (Dicentrarchus labrax). Animals 11, 392. doi: 10.3390/ani11020392
Kelly A. M., Kohler C. C. (1999). Cold tolerance and fatty acid composition of striped bass, white bass, and their hybrids. N. Am. J. Aquac. 61, 278–285. doi: 10.1577/1548-8454(1999)061<0278:CTAFAC>2.0.CO;2
Kim K. D., Lee S. M., Park H. G., Bai S., Lee Y. H. (2007). Essentiality of dietary n-3 highly unsaturated fatty acids in juvenile Japanese flounder Paralichthys olivaceus. J. World Aquac. Soc 33, 432–4440. doi: 10.1111/j.1749-7345.2002.tb00022.x
Koppang E. O., Kvellestad A., Fischer U. (2015). “Fish mucosal immunity: gill,” in Mucosal health in aquaculture. Eds. Beck B. H., Peatman E. (Amsterdam, Netherland: Academic Press), 93–133. doi: 10.1016/B978-0-12-417186-2.00005-4
Koracevic D., Koracevic G., Djordjevic V., Andrejevic S., Cosic V. (2001). Method for the measurement of antioxidant activity in human fluids. J. Clin. Pathol. 54, 356–361. doi: 10.1136/jcp.54.5.356
Kostetsky E. Y., Velansky P. V., Sanina N. M. (2013). Phase transitions of phospholipids as a criterion for assessing the capacity for thermal adaptation in fish. Russ. J. Mar. Biol. 39 (2), 214–222. doi: 10.1134/S1063074013030073
Lemaire P., Drai P., Mathieu A., Lemarie S., Carriére S., Guidicelli J., et al. (1991). Changes with different diets in plasma enzymes (GOT, GPT, LDH, ALP) and plasma lipids (cholesterol, triglycerides) of sea–bass (Dicentrarchus labrax). Aquaculture 93, 63–75. doi: 10.1016/0044-8486(91)90205-L
Lermen C. L., Lappe R., Crestani M., Vieira V. P., Gioda C. R., Schetinger M. R. C., et al. (2004). Effect of different temperature regimes on metabolic and blood parameters of silver catfish, Rhamdia quelen. Aquaculture 239, 497–507. doi: 10.1016/j.aquaculture.2004.06.021
Lima de Almeida C. A., Lima de Almeida C. K., Martins E., de F. F., Gomes Â.M., da A. L., et al. (2019). Effect of the dietary linoleic/α-linolenic ratio (n6/n3) on histopathological alterations caused by suboptimal temperature in tilapia (Oreochromis niloticus). J. Therm. Biol. 85, 102386. doi: 10.1016/j.jtherbio.2019.07.028
Liqin J., Keyong J., Mei L., Baojie W., Longjiang H., Mingming Z., et al. (2016). Low temperature stress on the hematological parameters and HSP gene expression in the turbot Scophthalmus maximus. chin. J. Oceanol. Limnol. 34, 430–440. doi: 10.1007/s00343-016-4367-z
Liu L., Zhang R., Wang X., Zhu H., Tian Z. (2020). Transcriptome analysis reveals molecular mechanisms responsive to acute cold stress in the tropical stenothermal fish tiger barb (Puntius tetrazona). BMC Genom. 21, 737. doi: 10.1186/s12864-020-07139-z
Ma X. Y., Qiang J., He J., Gabriel N. N., Xu P. (2015). Changes in the physiological parameters, fatty acid metabolism, and SCD activity and expression in juvenile GIFT tilapia (Oreochromis niloticus) reared at three different temperatures. Fish Physiol. Biochem. 41, 937–950. doi: 10.1007/s10695-015-0059-4
Malek R. L., Sajadi H., Abraham J., Grundy M. A., Gerhard G. S. (2004). The effects of temperature reduction on gene expression and oxidative stress in skeletal muscle from adult zebrafish. Comp. Biochem. Physiol. Part C 138, 363–373. doi: 10.1016/j.cca.2004.08.014
Ng W. K., Romano N. (2013). A review of the nutrition and feeding management of farmed tilapia throughout the culture cycle. Rev. Aquacult. 5, 220–254. doi: 10.1111/raq.12014
Nishikimi M., Rao N. A., Yagi K. (1972). The occurrence of superoxide anion in the reaction of reduced phenazine methosulfate and molecular oxygen. Biochem. Biophys. Res. Commun. 46, 849–854. doi: 10.1016/S0006-291X(72)80218-3
Nobrega R. O., Batista R. O., Corrêa C. F., Mattioni B., Filer K., Pettigrew J. E., et al. (2019). Dietary supplementation of aurantiochytrium sp. meal, a docosahexaenoic-acid source, promotes growth of Nile tilapia at a suboptimal low temperature. Aquaculture 507, 500–509. doi: 10.1016/j.aquaculture.2019.04.030
Nobrega R. O., Corrêa C. F., Mattioni B., Fracalossi D. M. (2017). Dietary α-linolenic for juvenile Nile tilapia at cold suboptimal temperature. Aquaculture 471, 66–71. doi: 10.1016/j.aquaculture.2016.12.026
Panase P., Saenphet S., Saenphet K. (2018). Biochemical and physiological responses of Nile tilapia, Oreochromis niloticus l. @ in subjected to cold shock of water temperature. Aquac. Rep. 11, 17–23. doi: 10.1016/j.aqrep.2018.05.005
Pandey S., Parvez S., Sayeed I., Haque R., Bin-Hafeez B., Raisuddin S. (2003). Biomarkers of oxidative stress: a comparative study of river yamuna fish Wallago attu (Bl. & schn.). Sci. Total Environ. 309, 105–115. doi: 10.1016/S0048-9697(03)00006-8
Pavlović S. Z., Borković Mitić S. S., Radovanović T. B., Perendija B. R., Despotović S. G., Gavrić J. P., et al. (2010). Seasonal variations of the activity of antioxidant defense enzymes in the red mullet (Mullus barbatus l.) from the Adriatic Sea. Mar. Drugs 8, 413–428. doi: 10.3390/md8030413
Qi Z. H., Liu Y. F., Luo S. W., Chen C. X., Liu Y., Wang W. N. (2013). Molecular cloning, characterization and expression analysis of tumor suppressor protein p53 from orange-spotted grouper, Epinephelus coioides in response to temperature stress. Fish Shellfish Immunol. 35, 1466–1476. doi: 10.1016/j.fsi.2013.08.011
Refaey M. M., Mehrim A. I., El-Komy M. M. (2018). Aquafat-o® as a growth and physiological enhancer agent for hybrid red tilapia, Oreochromis niloticus (L.) × Oreochromis mossambicus (Peters). Asian J. Anim. Vet. Adv. 13, 14–24. doi: 10.3923/ajava.2018.14.24
Refaey M. M., Mehrim A. I., Zenhom O. A., Mansour A. T. (2022). Effect of fatty acids manipulation on survival and physiological response of hybrid red tilapia under chronic cold stress. Aquaculture 561, 738663. doi: 10.1016/j.aquaculture.2022.738663
Reitman S., Frankel S. (1957). Colorimetric estimation of serum transaminases. Am. J. Clin. Pathol. 28, 56–63. doi: 10.1093/ajcp/28.1.56
Rossi A., Bacchetta C., Cazenave J. (2017). Effect of thermal stress on metabolic and oxidative stress biomarkers of Hoplosternum littorale (Teleostei, Callichthyidae). Ecol. Indic. 79, 361–370. doi: 10.1016/j.ecolind.2017.04.042
SAS (2006). Statistical Analysis System. SAS Release 9.1.3 for windows (SAS Institute Inc. Cary, NC, USA).
Schlenk D., Handy R., Steinert S., Depledge M. H., Benson W. (2008). “Biomarkers,” in The toxicology of fishes. Eds. Di Giulio R. T., Hinton D. E. (Boca: CRC Press), 683–731 pp.
Shi G. C., Dong X. H., Chen G., Tan B. P., Yang Q. H., Chi S. Y., et al. (2015). Physiological responses and HSP 70 m RNA expression of GIFT strain of Nile tilapia (Oreochromis niloticus) under cold stress. Aquacult. Res. 46 (3), 658–668. doi: 10.1111/are.12212
Snyder R. J., Hennessey T. M. (2004). Cold tolerance and homeoviscous adaptation in freshwater alewives (Alosa pseudoharengus). J. Fish. Physiol. Biochem. 29, 117–126. doi: 10.1023/B:FISH.0000035920.60817.11
Soltanian S., Adloo M. N., Hafeziyeh M., Ghadimi N. (2014). Effect of β-glucan on cold-stress resistance of striped catfish, Pangasianodon hypophthalmus (Sauvage, 1878). Vet. Med. (Praha) 59 (9), 440–446. doi: 10.17221/7684-VETMED
Storelli R., Acierno R., Maffia M. (1998). “Membrane lipid and protein adaptations in Antarctic fish,” in Cold ocean physiology. Eds. Portnerand H. O., Playle R. C. (Cambridge, UK: Cambridge University Press), 166–189.
Tocher D. R. (2003). Metabolism and functions of lipids and fatty acids in teleost fish. Rev. Fish. Sci. Aquac. 11, 107–184. doi: 10.1080/713610925
Tocher D. R., Dabrowski K., Hardy R. (2010). Fatty acid requirements in ontogeny of marine and freshwater fish. Aquacult. Res. 41, 717–732. doi: 10.1111/j.1365-2109.2008.02150.x
Tort L., Rotllant J., Liarte C., Acerete L., Hernandez A., Ceulemans S., et al. (2004). Effects of temperature decrease on feeding rates, immune indicators and histopathological changes of gilthead sea bream, Sparus aurata fed with an experimental diet. Aquaculture 229, 55–65. doi: 10.1016/S0044-8486(03)00403-4
Turchini G. M., Torstensen B. E., Ng W. K. (2009). Fish oil replacement in finfish nutrition. Rev. Aquac. 1, 10–57. doi: 10.1111/j.1753-5131.2008.01001.x
Velmurugan B. K., Chan C. R., Weng C. F. (2019). Innate-immune responses of tilapia (Oreochromis mossambicus) exposure to acute cold stress. J. Cell. Physiol. 234 (9), 16125–16135. doi: 10.1002/jcp.28270
Villanueva M., Ibarz A., Blasco J., Gallardo M. A., Santigosa E., Fenra´ndez-Borras J. (2006). Body composition and metabolism of gilthead sea bream (Sparus aurata): effects of diet and activity (Biarritz, France: ACTA XII international symposium on fish nutrition and feeding).
Watanabe W. O., Olla B. L., Wicklund R. I., Head W. D. (1997). Saltwater culture of the Florida red tilapia and other saline-tolerant tilapias: a review. Tilapia Aquaculture Americas 1, 54–141.
Weber T. E., Bosworth B. G. (2005). Effects of 28-day exposure to cold temperature or feed restriction on growth, body composition, and expression of genes related to muscle growth and metabolism in channel catfish. Aquaculture 246, 483–492. doi: 10.1016/j.aquaculture.2005.02.032
Wendelaar Bonga S. E. (1997). The stress response in fish. Physiol. Rev. 77, 591–625. doi: 10.1152/physrev.1997.77.3.591
Wolf J. C., Wolfe M. J. (2005). A brief overview of nonneoplastic hepatic toxicity in fish. Toxicol. Pathol. 33, 75–85. doi: 10.1080/01926230590890187
Wu S. M., Liu J.-H., Shu L.-H., Chen C. H. (2015). Anti-oxidative responses of zebrafish (Danio rerio) gill, liver and brain tissues upon acute cold shock. Comp. Biochem. Physiol. A 187, 202–213. doi: 10.1016/j.cbpa.2015.05.016
Yilmaz S., Ergün S., Çelik E.Ş., Banni M., Ahmadifar E., Dawood M. A. (2021). The impact of acute cold water stress on blood parameters, mortality rate and stress-related genes in Oreochromis niloticus, Oreochromis mossambicus and their hybrids. J. Therm. Biol. 100, 103049. doi: 10.1016/j.jtherbio.2021.103049
Younis E. M. (2015). Variation in metabolic enzymatic activity in white muscle and liver of blue tilapia, Oreochromis aureus, in response to long-term thermal acclimatization. Chin. J. Oceanol. Limnol. 33, 696–704. doi: 10.1007/s00343-015-4093-y
Zhou T., Gui L., Liu M., Li W., Hu P., Duarte D. F. C., et al. (2019). Transcriptomic responses to low temperature stress in the Nile tilapia, Oreochromis niloticus. Fish Shellfish Immunol. 84, 1145–1156. doi: 10.1016/j.fsi.2018.10.023
Keywords: red tilapia, cold stress, liver integrity, histopathology, oxidative biomarkers
Citation: Refaey MM, Mehrim AI, El-Komy MM, Zenhom OA and Mansour AT (2023) Chronic cold-stress induced histopathological changes, oxidative stress, and alterations in liver functions and nutrient composition of hybrid red tilapia and the potential protection of unsaturated fatty acids. Front. Mar. Sci. 10:1148978. doi: 10.3389/fmars.2023.1148978
Received: 20 January 2023; Accepted: 21 February 2023;
Published: 08 March 2023.
Edited by:
Manuel Gesto, Technical University of Denmark, DenmarkReviewed by:
Sevdan Yilmaz, Çanakkale Onsekiz Mart University, TürkiyeMohamed Hamed, Al Azhar University, Egypt
Copyright © 2023 Refaey, Mehrim, El-Komy, Zenhom and Mansour. This is an open-access article distributed under the terms of the Creative Commons Attribution License (CC BY). The use, distribution or reproduction in other forums is permitted, provided the original author(s) and the copyright owner(s) are credited and that the original publication in this journal is cited, in accordance with accepted academic practice. No use, distribution or reproduction is permitted which does not comply with these terms.
*Correspondence: Mohamed M. Refaey, bV9tb2FhekBtYW5zLmVkdS5lZw==; Abdallah Tageldein Mansour, YW1hbnNvdXJAa2Z1LmVkdS5zYQ==
†ORCID: Mohamed M. Refaey, orcid.org/0000-0001-7824-0184
Ahmed I. Mehrim, orcid.org/0000-0002-2598-3424
Abdallah Tageldein Mansour, orcid.org/0000-0002-5963-5276