- 1Key Laboratory of Ocean and Marginal Sea Geology, South China Sea Institute of Oceanology, Chinese Academy of Sciences, Guangzhou, China
- 2University of Chinese Academy of Sciences, Beijing, China
- 3Key Laboratory of Tropical Marine Bio-resources and Ecology, South China Sea Institute of Oceanology, Chinese Academy of Sciences, Guangzhou, China
The lack of long-term records of coral community composition restricts our understanding of the contemporary ecological states of tropical reefs. Here we integrated paleo-ecological reconstruction, historical mortality evidence, and ecological survey data to determine the temporal variability in reef resilience of the Nansha atolls in the tropical western Pacific. Subfossil coral assemblages extracted from the reef cores exhibited no evidence of community shifts attributable to centennial-scale changes in El Niño variability during the last millennium, suggesting long-term stability in community structure and persistence of reef resilience. By contrast, ecological surveys revealed a major collapse in the reef ecosystem, and high-precision U-series dating of dead Acropora fragments indicated that this collapse occurred in recent decades and was especially relevant to several strong/extreme El Niño episodes. Frequent and intensive El Niño−Southern Oscillation and marine heatwaves have overwhelmed the reefs’ resistive and recovery capacity, thereby impairing reef resilience.
1 Introduction
Coral reef resilience, the capacity to resist stressors or recover from recurrent disturbances, is essential for maintaining ecosystem functions, the delivery of ecological goods and services, and the practice of coral reef restoration (Moberg and Folke, 1999; Hughes et al., 2010; Shaver et al., 2022). However, recurring large-scale coral bleaching and mortality triggered by extremely high sea surface temperature (SST) events (i.e., marine heatwaves, abbreviated as MHWs; Hobday et al., 2016) are at present the greatest threats to global coral reef resilience (Hughes et al., 2018a; Smale et al., 2019; Souter et al., 2021). Concurrent with ongoing climate warming, global MHWs are becoming more intense, frequent, and prolonged (Frölicher et al., 2018; Oliver et al., 2018) and have already occurred throughout all El Niño−Southern Oscillation (ENSO) phases (e.g., Eakin et al., 2019; Mo et al., 2022). Correspondingly, regional- to global-scale bleaching and mortality events are occurring more frequently, increasing the likelihood of an “annual bleaching scenario” in the coming decades (Hughes et al., 2017; Hughes et al., 2018a). The interval between events is therefore becoming insufficient for a full recovery of coral reef ecosystems (Hughes et al., 2018a).
Rapidly increasing MHW-induced bleaching has raised worldwide interest in evaluating the impacts on coral reef resilience and associated ecosystem services at present and in the future (reviewed in Hughes et al., 2010; Roff and Mumby, 2012; Carballo-Bolaños et al., 2020). However, debates on the impacts of regional thermal disturbances, resultant resilience changes, and the future of tropical coral reef systems are still ongoing. The prevailing view is that MHWs erode coral reef resilience by increasing mass coral bleaching and mortality, reducing larval supply and coral recruitment, weakening the reef’s connectivity, and eventually changing coral community composition and ecological function (Hughes et al., 2018b; Hughes et al., 2019; Cheung et al., 2021; Speare et al., 2022). However, several new perspectives from the Lakshadweep archipelago, central Indian Ocean (Yadav et al., 2018), and the Gilbert Islands, central tropical Pacific (Donner and Carilli, 2019; Cannon et al., 2021), have suggested that the reduction in bleaching-sensitive, branching species (e.g., Acropora) is not a signal of lowered resilience. On the contrary, it indicates a shift in the community composition to more heat-tolerant, slow-growing taxa, which is causing the growing resistance of reef systems to recurrent thermal anomalies. Furthermore, evidence from the western (DeCarlo et al., 2019), central (Fox et al., 2021) and eastern (Romero-Torres et al., 2020) tropical Pacific indicated that corals (at least some species) demonstrated more resistance to bleaching and mortality after exposure to repeated thermal stresses, and therefore tropical reefs may have the capability to adapt to near future warming and frequent MHW events.
Almost all knowledge concerning reef thermal resilience is based on observation data from modern coral reefs. Long-term (centennial to millennial timescale) baseline data that document temporal coral community changes forced by changed thermal regimes (e.g., Toth et al., 2012; Toth et al., 2021) are valuable for understanding contemporary reef resilience and vulnerability to climate change and may provide new perspectives for the above debates. However, such long-term records are scarce in the tropical Indo-Pacific region, the location of the world’s most abundant reef-building corals. Here, we present a ~1000-year record of coral community composition from two atolls (Figure 1) in the core area of the Indo-Pacific Warm Pool, where corals are surviving at close to the upper limits of their thermal tolerance and regularly experience heat stresses from El Niño events (Figure 2). A high-resolution paleo-ecological reconstruction based on uranium-thorium (U-Th) dated subfossil branching corals extracted from three reef cores was used to provide baselines and long-term insights into the reef resilience under paleoclimate dynamics. We also assessed the community composition, coral bleaching, and U-Th dated mortality for living and dead assemblages to determine the potential transformations of reef assemblages through statistical comparisons between paleo- and modern records. In this study, branching coral groups (especially the genus Acropora) were given special concern in reef cores and modern surveys, as they are fast-growing but susceptible to heat stress and bleaching and play a significant role in reef ecosystems as key framework builders and ecological service providers.
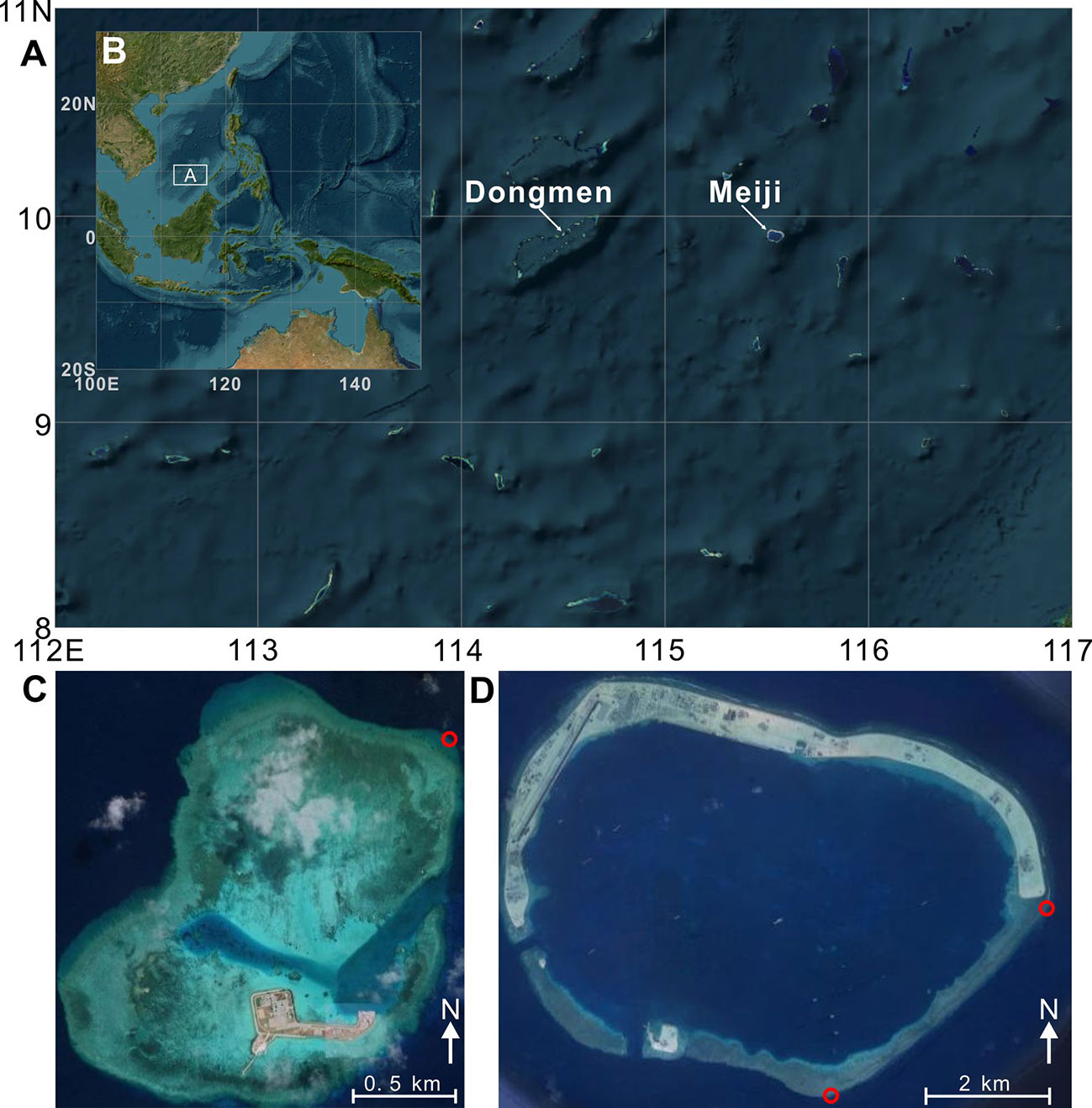
Figure 1 The Nansha Archipelago (A) is in the tropical South China Sea, western Pacific (B). Two atolls, Dongmen (C) and Meiji (D), are located in the north of Nansha region. Red circles indicated the locations of core sampling and ecological surveys. Satellite images were downloaded from the NOAA Marine Trackline Geophysical Data (https://ngdc.noaa.gov/mgg/geodas/trackline.html).
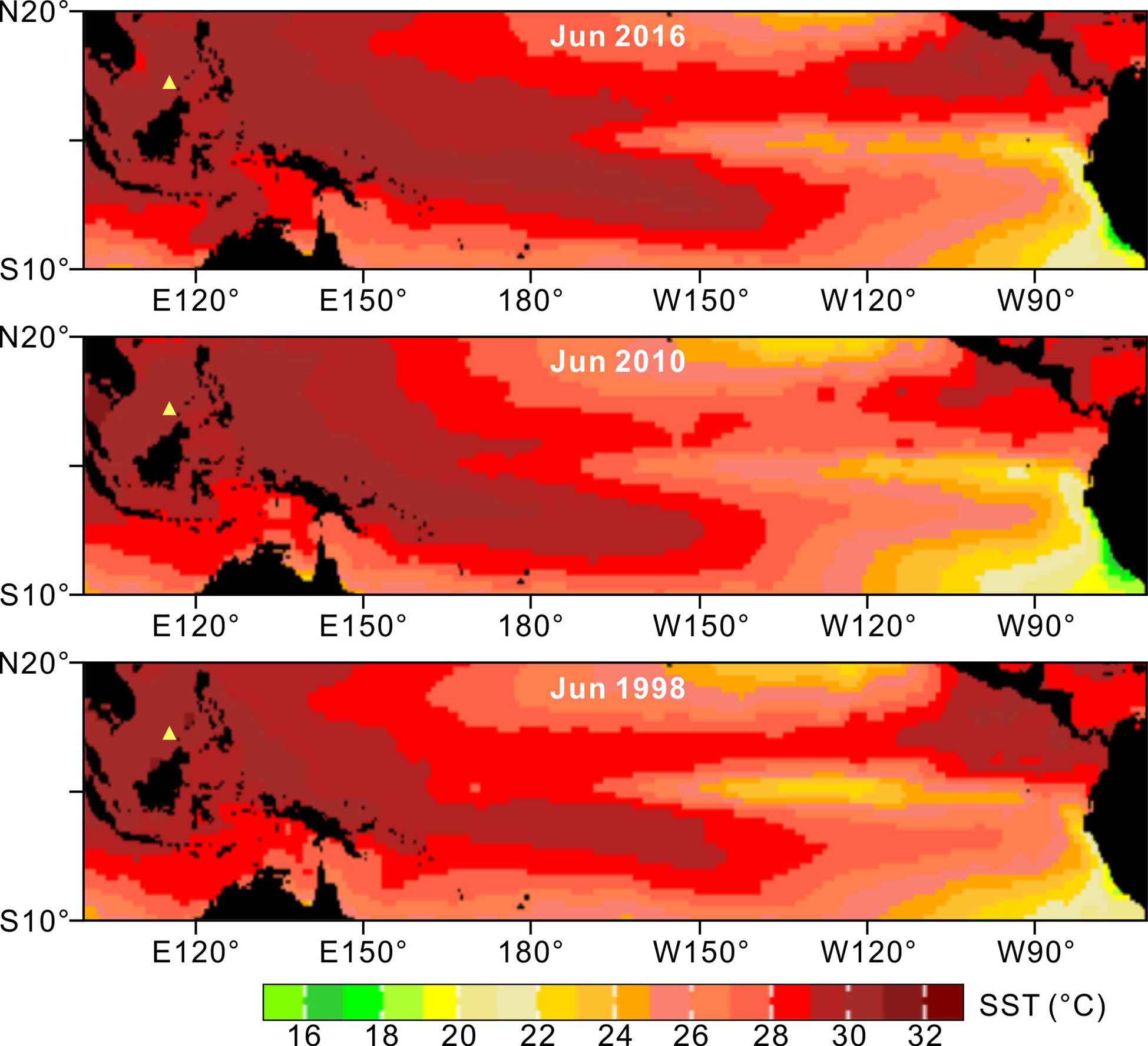
Figure 2 Monthly (June) mean sea surface temperatures (IGOSS nmc Reyn_SmithOIv2 monthly SST; Reynolds et al., 2002) displayed the variability of the warm pool structure associated with three strong/extreme El Niño events. Our study site is indicated with a yellow “Δ” in each image.
2 Materials and methods
2.1 Study Sites
The Nansha Archipelago (Figure 1), situated in the western tropical Pacific, extends southward from ~12°N to ~6°N and consists of over 200 coral atolls, cays, and shoals, and therefore is the largest coral reef system in the South China Sea. The abutting Coral Triangle (http://www.thecoraltriangle.com/) has been widely recognized as marine biodiversity hotspots at the global scale.
Based on the IGOSS dataset (Reynolds et al., 2002) between 1982 and 2020, the monthly mean SST in this area is 28.8°C, ranging from 26.2°C to 31.0°C. Abnormally high SSTs, commonly driven by periodic El Niño events (Figure 2), may severely threaten local reef-building corals (Li et al., 2011). The Nansha area is an excellent laboratory for examining tropical coral reef resilience in response to El Niño and MHW induced-thermal disturbances, because (1) the atolls are located in the core area of the Indo-Pacific Warm Pool, where corals typically live close to the upper limits of their thermal tolerance and are therefore vulnerable to thermal bleaching, (2) this region regularly experiences extreme heat associated with both central Pacific (CP) and eastern Pacific (EP) El Niño events (Figure 2), and (3) the offshore atolls are isolated from terrigenous sediments and pollution, and thus thermal stresses can be considered the primary threat to local reef systems. In 2016 and 2017, reef cores and ecological data were collected from Dongmen (9.91°N, 114.50°E) and Meiji (9.90°N, 115.53°E), two atolls in the center of the Nansha Archipelago (Figure 1).
2.2 Reef core collection and taxonomic analyses
In 2017, three cores (DM-1, collected from Dongmen Atoll; MJ-1 and MJ-2, collected from Meiji Atoll) were collected on the seaward margins (top of the front reef-slopes) of the two atolls (Figure 3), where the reef surfaces are always submerged, even during low tides, except for several large Porites blocks. Reef margins are ideal sites for coring because (1) fossil assemblages in cores can reflect the composition of the original coral assemblage of reef slopes (the main coral growing sites of an atoll) to the greatest extent; (2) reef flats and cays, in contrast, are largely composed of reworked and transported coral materials (e.g., Chen et al., 2019).
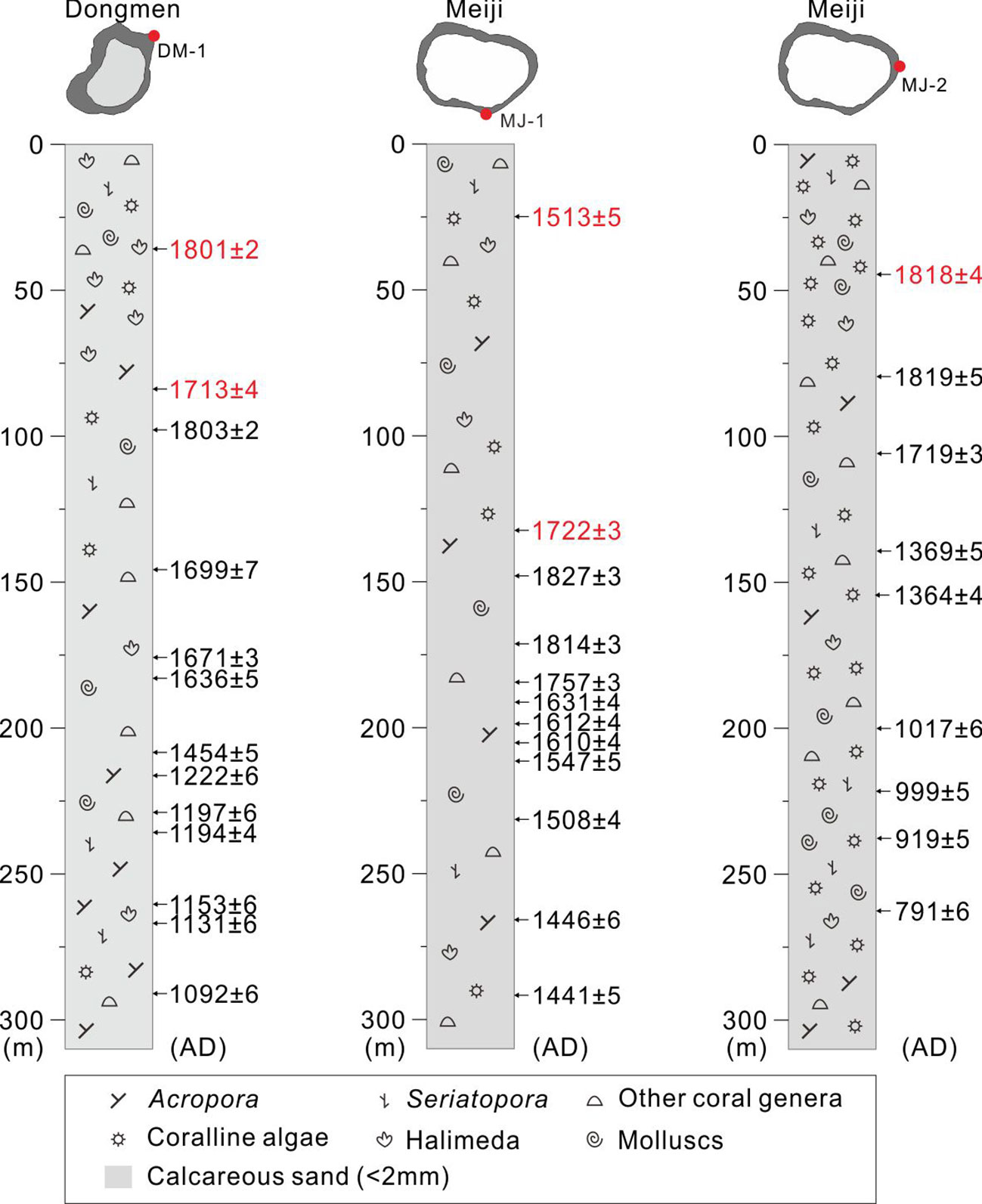
Figure 3 Core logs, bio-sedimentary facies, and U-Th chronology. The abnormal U-Th ages in the upper parts of cores are labelled in red.
To improve the recovery of unconsolidated sediments, cores were recovered by percussion coring using a diver-operated, submersible, hydraulic jackhammer and 76-mm diameter aluminum pipes. During extraction, each top pipe end was sealed by an externally fitted rubber plug, thereby creating a vacuum and preventing loss of material from the buried end. Cores were extracted slowly by the aid of adjustable clamps with handles and inflatable bags with air infilling from self-contained underwater breathing apparatus (SCUBA) regulators. This technique enabled ~3 m of penetration and 100% recovery of each core. A sediment compaction percentage (~ 34%) was calculated for each core to allow conversion to uncompacted depths.
In our lab, the aluminum core pipes were split in half using a circular saw to cut longitudinally. Each core sediment was further sub-sampled at 1-cm intervals. Subsampling interval was occasionally increased (up to 3 cm) due to large coral fragments encountered. Each sediment subsample was desalinized with deionized water, dried in an oven, then divided into > 2-mm and < 2-mm segments in size by sieving. In order to determine downcore changes in sediment composition and log the cores (Figure 3), bio-sedimentary facies for each > 2-mm fraction were identified by using a binocular microscope. In this study, bio-sedimentary facies include corals, coralline algae, molluscs, Halimeda, and others (including foraminifera shells and sea urchin spines). The weights of bio-sedimentary facies were measured using an electronic balance. Among the coral subfossils from the > 2-mm sediment fraction, all branching corals were identified to genus level (e.g., Acropora, Pocillopora, and Seriatopora) according to the morphology and skeletal architecture (colony morphology, corallite arrangement and budding, corallite morphology, and coenosteum and internal corallite structure; Figure 4) with reference to taxonomic features described in Veron (2000); Zou (2001) and Huang et al. (2021). For most non-branching subfossil corals, however, identification was difficult because of insufficient corallites in small fragments. The branching and non-branching coral subfossils were also weighed. Coral abundance was subsequently assessed using the subfossil coral weight divided by the gross weight of the > 2-mm fraction of each sediment subsample. Similarly, the weights of each branching genus, all branching subfossils, and non-branching subfossils were standardized to percent abundance of the total subfossil coral weight. The down-core abundance timeseries (Tables 1 and 2; Figures 5A, B) were calculated as the average from three cores and 50-year intervals based on the interpolated U-Th chronology (see below “2.4 U-Th dating”).
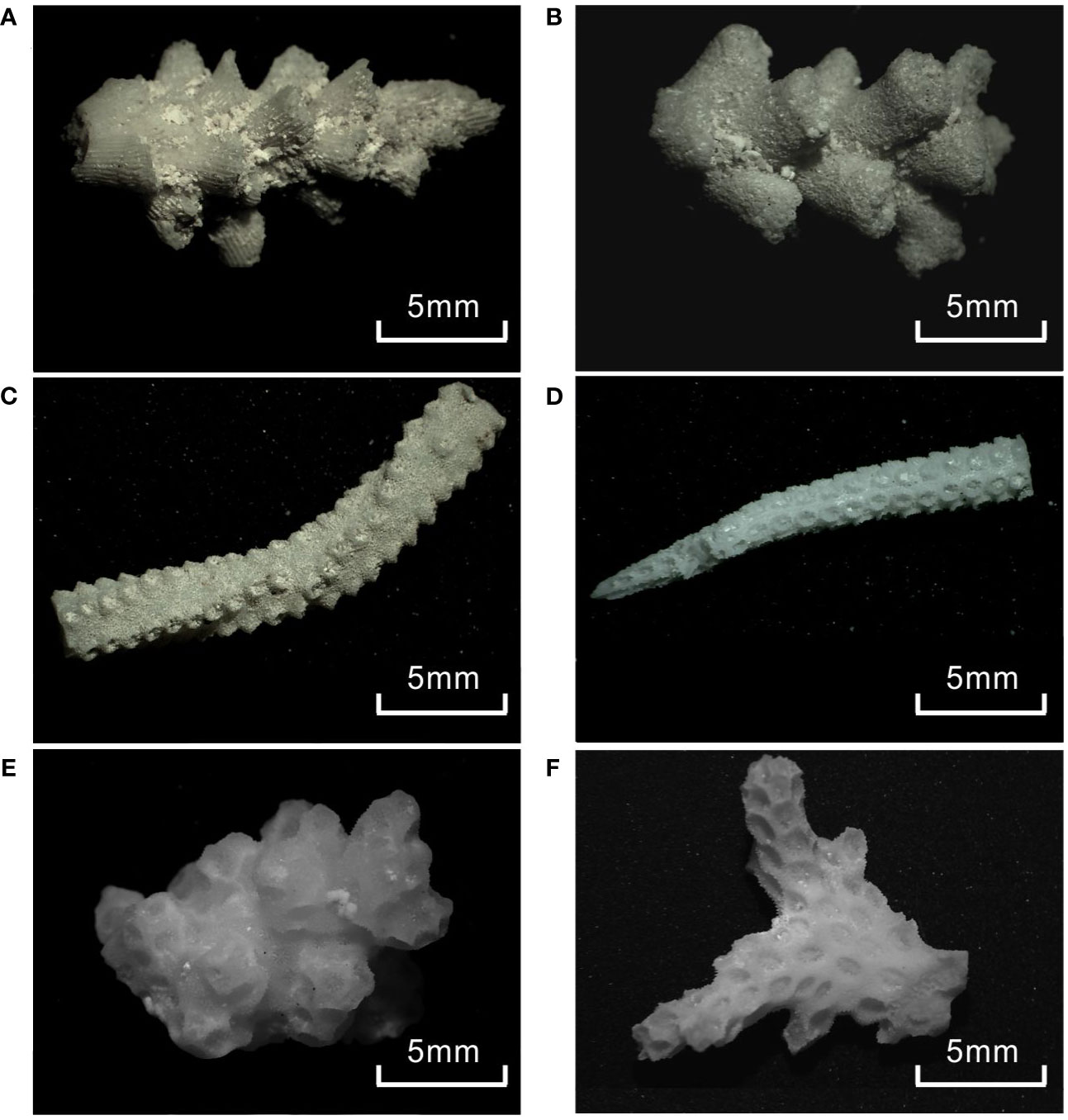
Figure 4 Representative photos of subfossil branching coral samples selected from the reef cores: (A, B) Acropora spp.; (C, D) Seriatopora spp.; (E, F) Pocillopora spp.
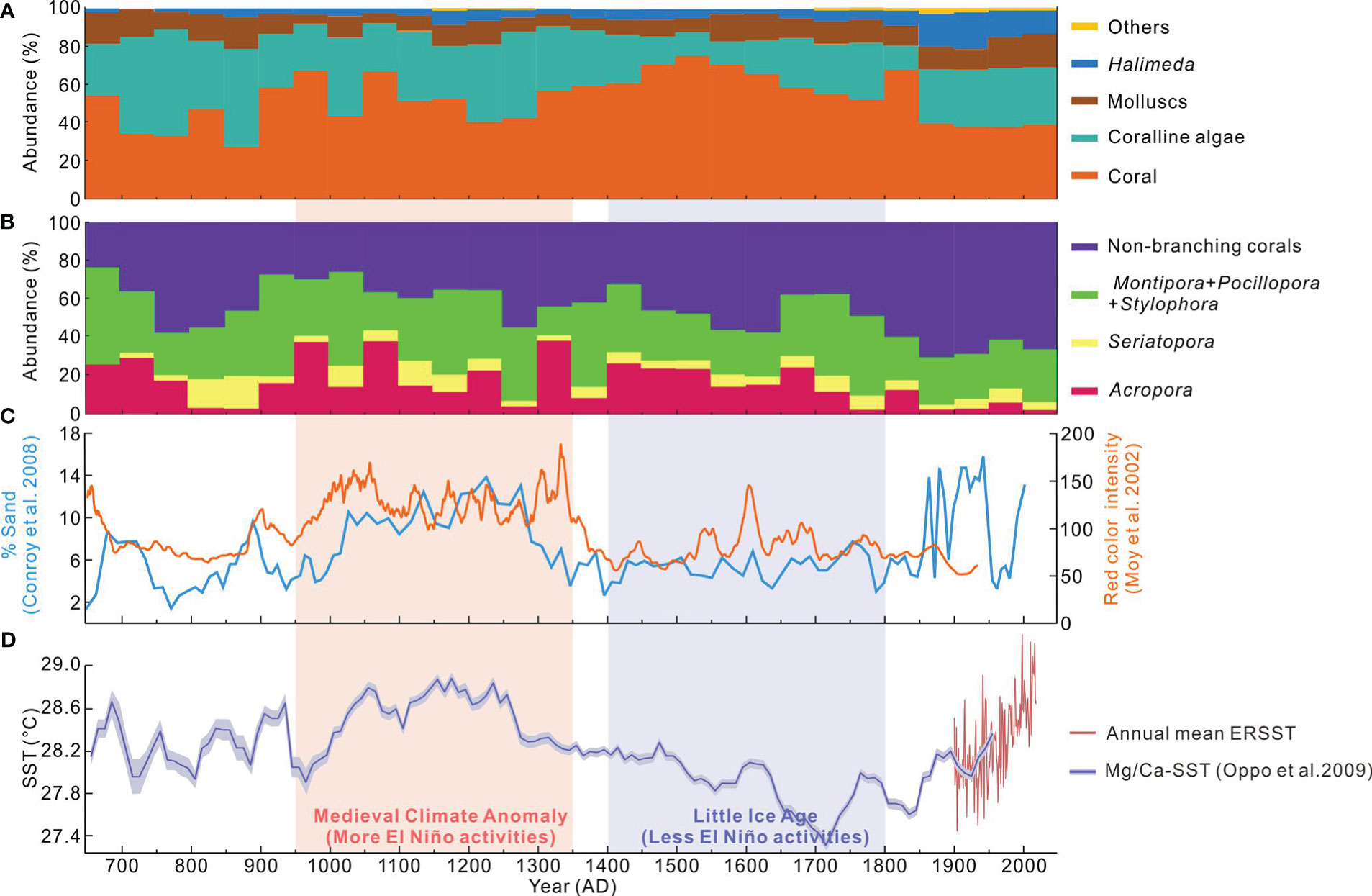
Figure 5 Paleo-ecological and paleo-climatic records: Abundances of (A) each bio-facies and (B) each coral genus, shown as the percent abundance of the total >2 mm sediment fraction and subfossil coral assemblage, respectively, for each 50-year interval (Tables 1 and 2); (C) Millennial-scale El Niño frequency timeseries from 50-year window width running mean of grayscale intensity in sediment cores from Laguna Pallacocha, southern Ecuador (Moy et al., 2002) and the grain size data from El Junco sediment cores, Galápagos (Conroy et al., 2008); and (D) Decadal-resolved SSTs reconstructed by foraminifera Mg/Ca proxy from the Makassar Strait (Oppo et al., 2009) and the annual mean ERSST timeseries.
2.3 Coral assemblage surveys and sampling
Adjacent to each coring site, three 20-m-long transects were placed in parallel at depths of 3 m, 9 m, and 15 m on the fore-reef slopes in 2016 and 2017. Line intercept transect method (English et al., 1997) was used to determine the percentage cover of benthic communities, including reef-building corals (both living and dead assemblages), non-reef-building corals (e.g., Millepora, Heliopora, Gorgonia, and soft corals), and other benthos (e.g., sponges and sea anemones). Living reef-building corals were further identified to genus level (Supplementary Table 1), according to Veron (2000). Along each transect, we also randomly collected dead Acropora coral rubble for U-Th dating to trace historical mortality events.
2.4 U-Th dating
For U-Th dating, 34 subfossil Acropora branches, including 29 well-preserved samples (e.g., Figures 4A, B) and five rubble samples with obvious abrasion were selected from core sediments. Furthermore, 139 surficial dead Acropora samples were selected for dating. For each dead Acropora branch, only the top (< 2 cm) was used for dating to ensure that the skeletogenesis site where sampling took place was within one year of the time of colony death. The surfaces of all selected coral samples were polished to remove surficial calcareous encrustation and bioerosion, and then crushed into ~1-mm-size grains. Approximately 150 mg of grain samples was weighed, H2O2-treated, and then hand-picked under a binocular microscope to remove any grains with discoloration. All U-Th chemistry (e.g., U-Th separation through resin) and analytical procedures were performed under ultra-clean conditions in the Radiogenic Isotope Facility, School of Earth and Environmental Sciences, University of Queensland. The analytical protocol and initial 230Th correction have been described in detail by Clark et al. (2014). U-Th isotopic ratio measurements were performed on a Nu-1 multi-collector inductively coupled plasma mass spectrometer (MC-ICP-MS). The 230Th ages of all samples were calculated using Isoplot program (version 3.75) (Ludwig, 2012). The detailed results of U-Th dating are shown in Supplementary Table 2. With these U-Th data, the chronology of each sediment subsample within reef cores was estimated using linear interpolation between each pair of U-Th ages. The relative probability of age structures of surficial dead corals (Figure 6) was calculated using Isoplot program (Ludwig, 2012).
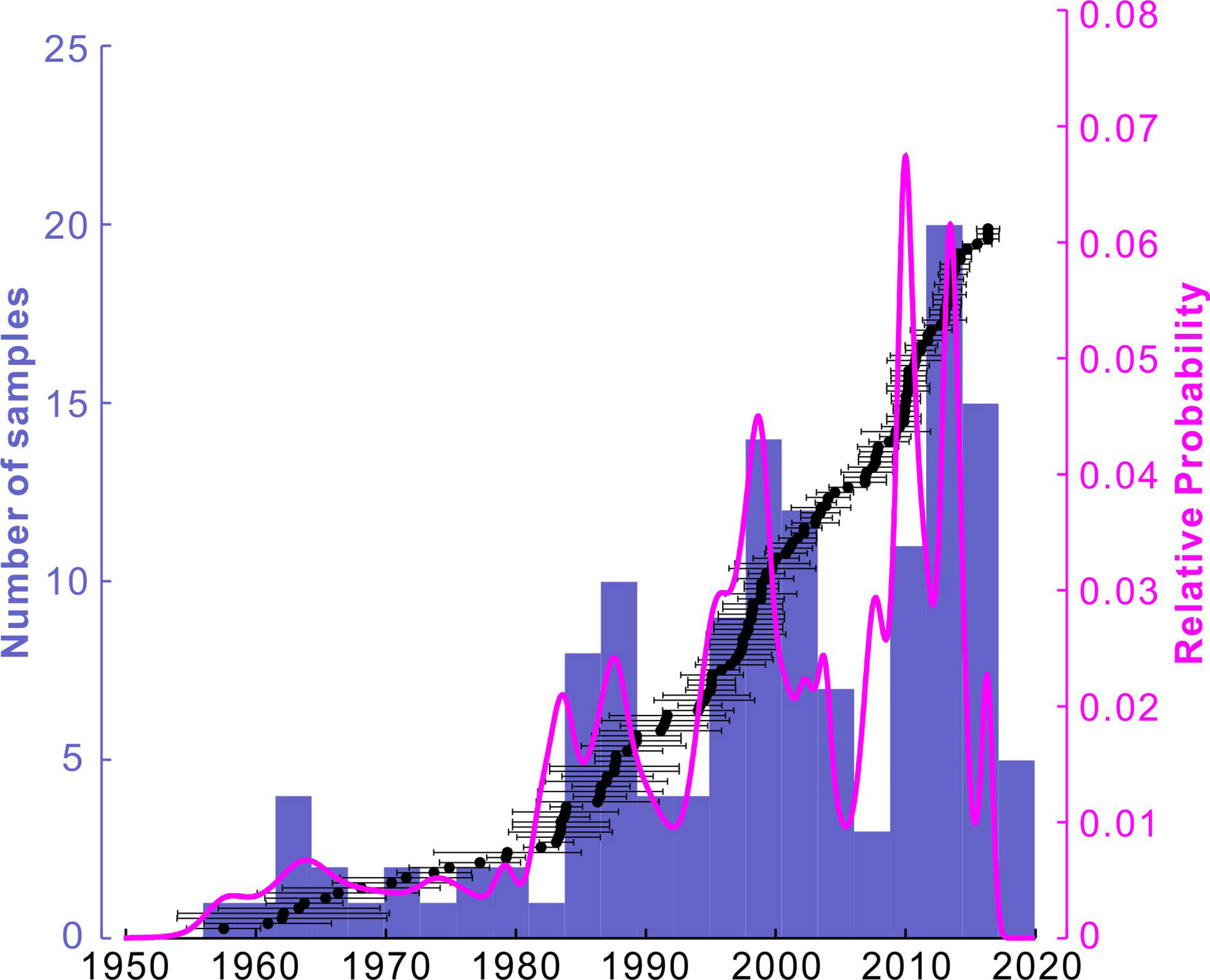
Figure 6 Histogram and relative probability of U-Th ages of surficial dead Acropora corals. Individual U-Th ages ± 2σ errors are shown as black points with error bars. Raw data can be found in Supplementary Table 2.
2.5 Excluding cyclone deposits
The northern South China Sea is among the most active areas of tropical cyclone activity globally (Chen et al., 2019). Although to a lesser extent than the reefs in the northern South China Sea, the southern Nansha atolls are still sporadically exposed to tropical cyclones (Figure 7A) that can transport coarse calcareous sand, coral rubble, and even large coral blocks from windward reef slopes to leeward reef flats and lagoons (Yu et al., 2009). Our cores unavoidably included some cyclone-deposited fragments, although we had avoided drilling at reef flats, sand cays, and lagoons that are normally the sinks for storm deposits. Therefore, this ex-situ coral rubble in reef cores should be excluded from further paleo-ecological reconstruction and statistical analyses, as it has the potential for misinterpretation.
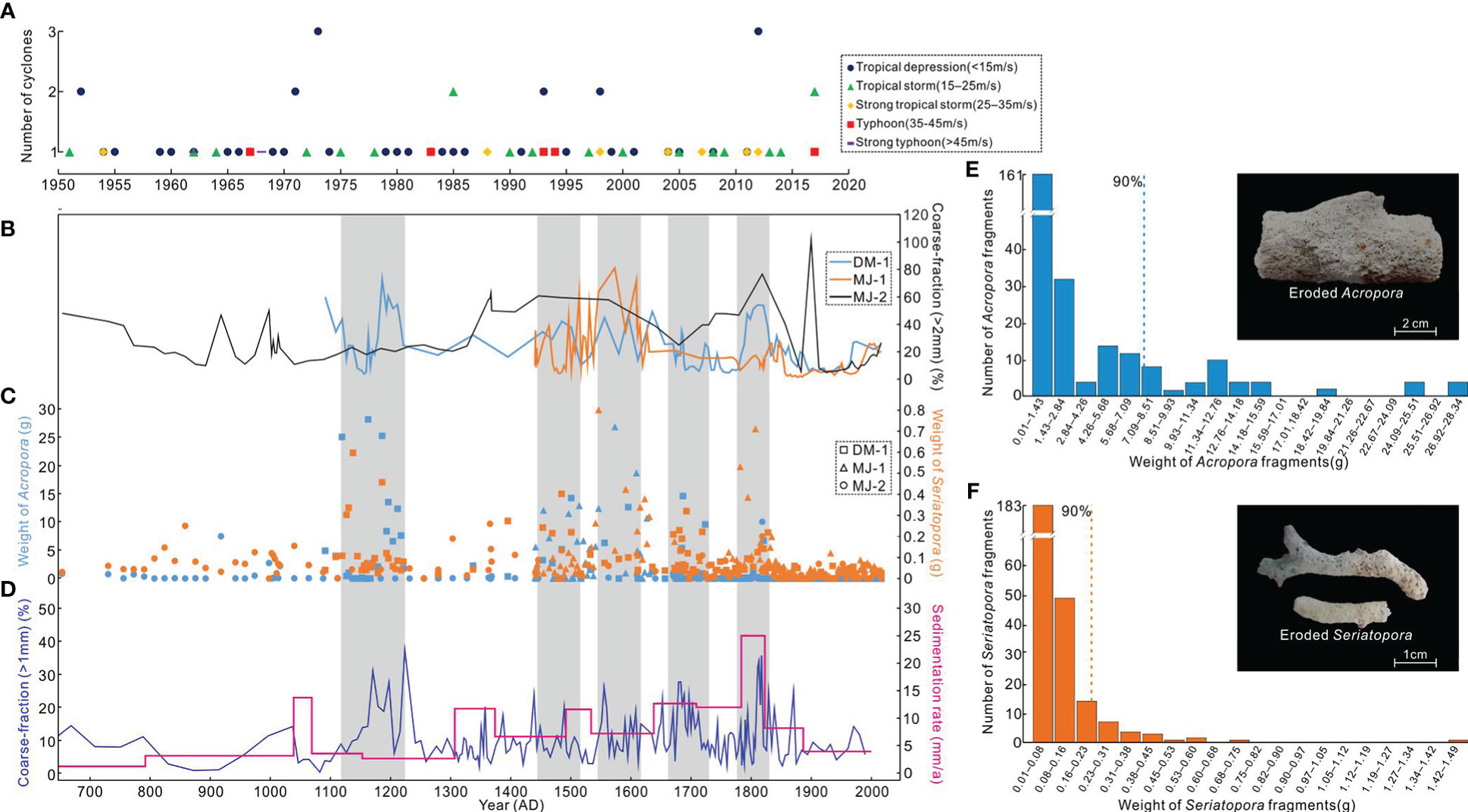
Figure 7 Excluding cyclone-deposited subfossils from the core sediments: (A) Historical tropical cyclones that had the potential to impact our study sites (9−11°N, 114−116°E); (B) Coarse-fraction (> 2 mm) percentages of cores DM-1, MJ-1, and MJ-2. The percentage was calculated as the weight of > 2-mm fraction divided by the weight of each sediment sample; (C) Weight of dominant branching (Acropora and Seriatopora) fragments from the reef cores; (D) Late-Holocene cyclone history of the Nansha (Yu et al., 2009) as revealed by coarse-fraction percentage and sedimentation rate extracted from the Yongshu lagoon (9.60°N, 112.95°E); (E, F) Weight frequency distributions of Acropora and Seriatopora fragments and representative photos of relatively large but heavily eroded coral fragments.
For each core sediment sample, the percentage of the > 2-mm fraction was calculated (Figure 7B), all Acropora and Seriatopora (as dominant genera) fragments were weighed (Figure 7C), and time series were established by linear interpolation based on the U-Th dates. The abnormally high values of > 2-mm fraction percentage and fragment weight (Figures 7B, C) could be linked with relatively large and clearly eroded rubble (Figures 7E, F), and were correlated with historical cyclone activities recorded by lagoon sediment cores (Figure 7D). In total, more than 40 eroded branching fragments were screened out. Although these cyclone-deposited fragments accounted for less than 10% of branching subfossils (Figures 7E, F), they were excluded from the fossil record and further comparative analysis of paleo-reef composition. Based on this conservative approach, we are confident that the records presented in this study provide a reliable history of past coral community composition and changes.
2.6 Statistical analyses
For modern reef ecology, one-way Permutational Multivariate Analysis of Variance (PERMANOVA) was performed to estimate significant differences in living coral cover (1) between the years 2016 and 2017, (2) among the three reef sites (DM-1, MJ-1, and MJ-2; Figure 3), and (3) among the twenty coral genera. The data matrix consisted of years, sites, genera, and coral cover percentages (Supplementary Table 1). Where significant differences were found, Similarity of Percentages Analyses (SIMPER) was used to identify the most important genera contributing to the detected dissimilarity. These statistical analyses were used to test whether coral assemblages varied in response to changes in reef sites and to evaluate whether there were significant shifts in coral composition during recovery periods. Moreover, Principal Components Analysis (PCA) was applied to visualize the differences in coral cover among the twenty coral genera (Figure 8).
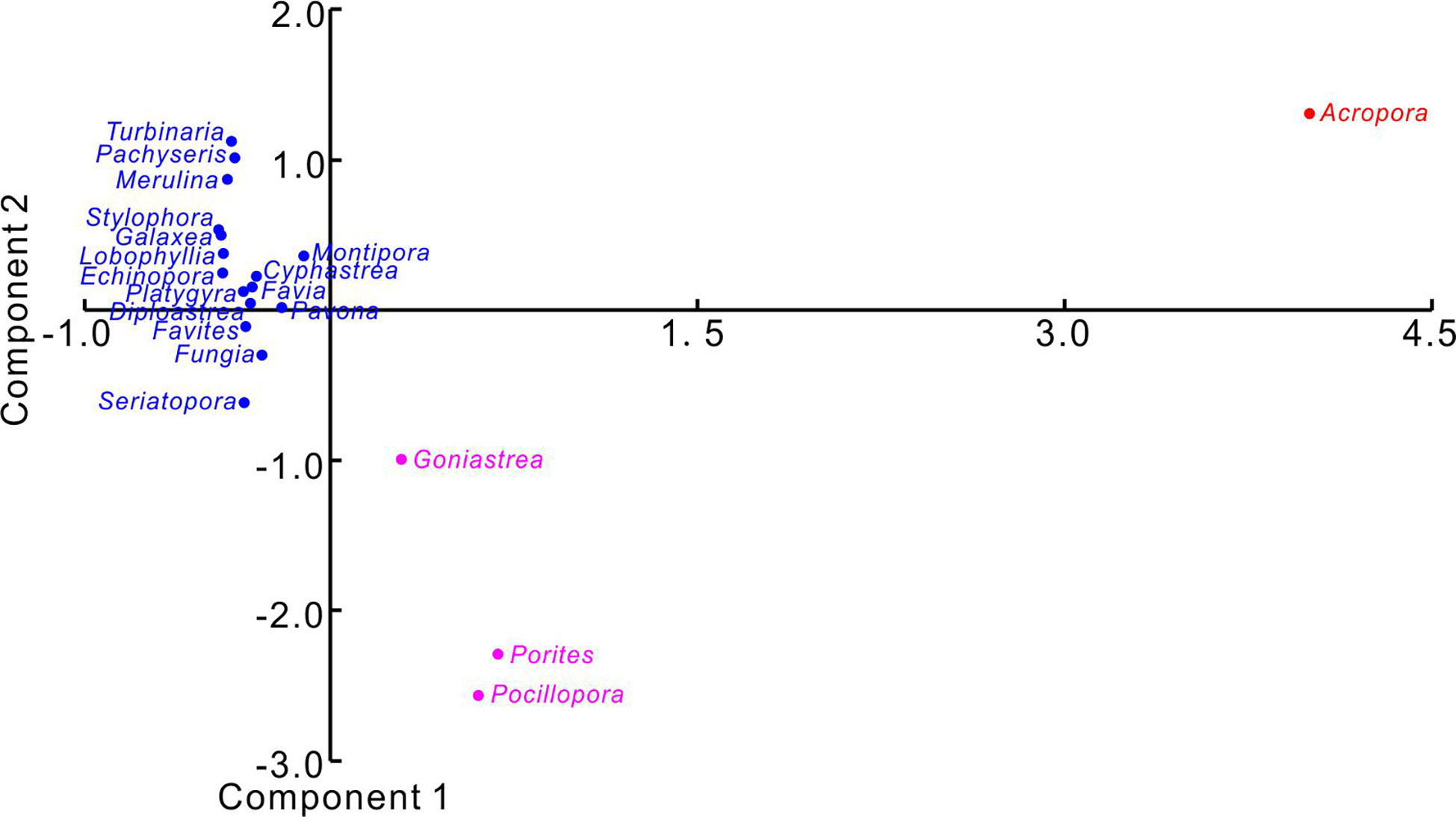
Figure 8 Principal components analysis (PCA) was applied to visualize the differences in coral cover among the twenty coral genera. The data matrix consisted of the coral cover percentages from 2016 and 2017. The raw ecological data can be found in Supplementary Table 1. The branching Acropora population had a relatively high coral cover and significant recovery. Genera Porites, Pocillopora, and Gonistrea also had relatively high cover but no significant recovery. The remaining coral genera (colored as blue) had low coral cover with no significant recovery, or declined further.
For paleo-ecology and paleo-climatology, PERMANOVA was used to evaluate significant differences in (1) paleo-coral community composition based on the abundance of bio-sedimentary facies and subfossil corals (Tables 1 and 2) in the reef cores, (2) 10-year linear interpolated paleo-El Niño records (Moy et al., 2002; Conroy et al., 2008) of two 400-year-intervals (950–1350 AD vs. 1400–1800 AD), and (3) subfossil vs. modern coral assemblages at the genus level. All statistical analyses were performed using PAST (version 4.09) software (Hammer et al., 2001). The Bray–Curtis dissimilarity index was used for PERMANOVA analyses.
2.7 Climate data
The NOAA high-resolution (0.25°×0.25°) daily OISST dataset (v2.1; https://www.ncdc.noaa.gov/oisst) was used to extract long-term (1982–2017) variation in MHW intensity and duration for Dongmen (the nearest pixel centered at 9.875°N, 114.375°E) and Meiji (9.875°N, 115.375°E) atolls (Figures 9B, C). This study focused on summertime (May–September) MHWs, as extremely high SSTs and bleaching events occur in hot summer months. Daily OISST data were analyzed with R (https://www.r-project.org/), using the package heatwaveR (Schlegel and Smit, 2018), according to the definition and statistical methodology developed by Hobday et al. (2016). Furthermore, the 2°×2° (centered at 10°N, 114°E) monthly ERSST anomaly dataset (v5) and the Multivariate ENSO Index (v2) timeseries were downloaded from the NOAA Physical Sciences Laboratory (PSL; https://psl.noaa.gov/) to display the correlations between regional-scale SST variation and local-scale MHW dynamics. Long-term records of tropical cyclones, classified as the maximum wind speed (Figure 7A), were derived from the China Meteorological Administration (http://www.cma.gov.cn).
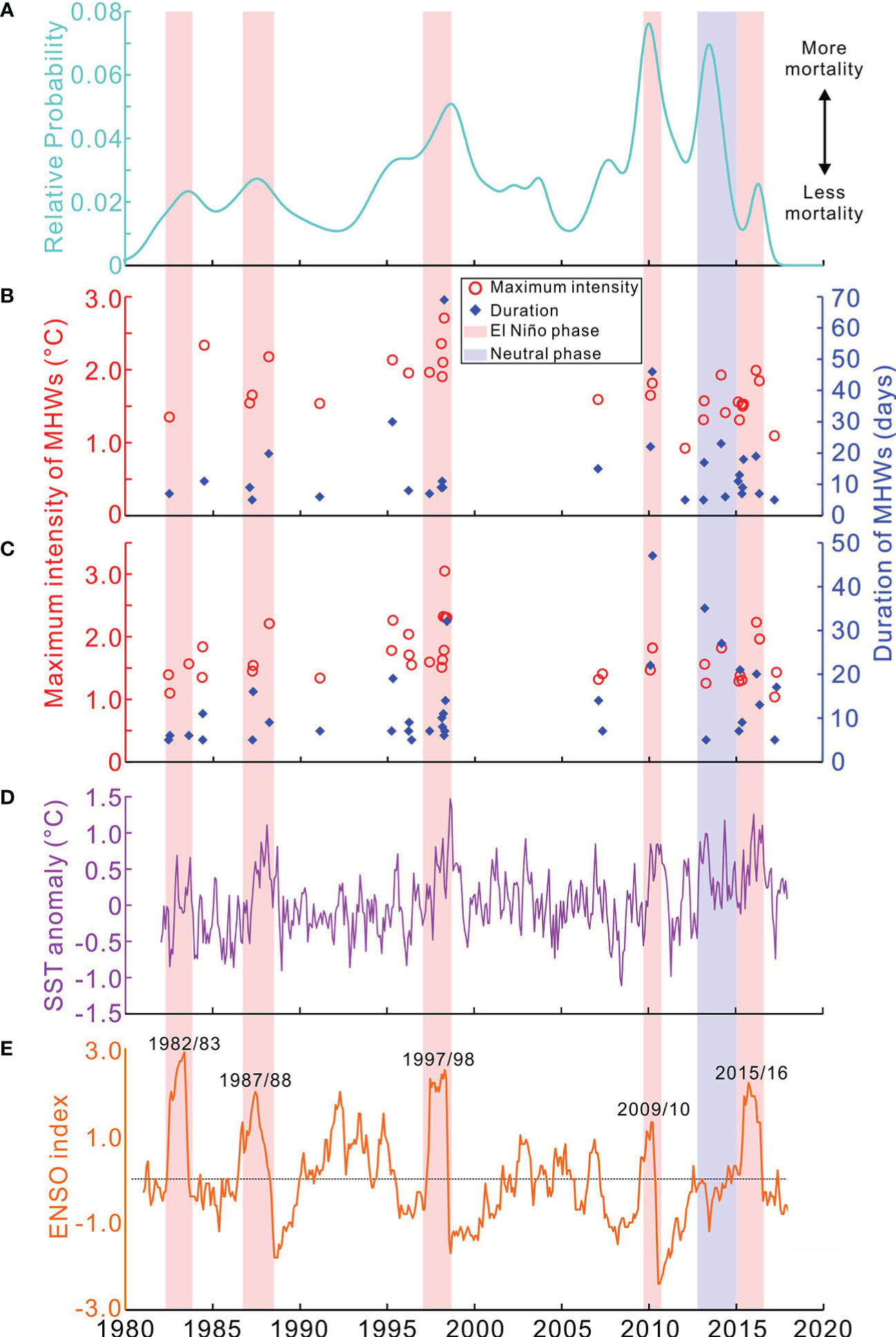
Figure 9 Past coral mortality and thermal disturbances: (A) The relative probability of U-Th dates of dead assemblages; MHWs (represented as maximum intensity) occurred in Meiji (B) and Dongmen (C) extracted from the summertime (May−September) NOAA OISST dataset (0.25×0.25°) with the R package “heatwaveR” (Schlegel and Smit, 2018); (D) monthly SST anomaly extracted from the 2×2° ERSST v5 dataset (centered at 10° N, 114° E); and (E) Multivariate ENSO Index.
3 Results
3.1 Reef core chronology and sedimentology
The chronology of cores DM-1, MJ-1, and MJ-2 was established based on U-Th dating of subfossil Acropora branches (Supplementary Table 2). For each core, most U-Th dates were stratigraphically consistent (Figure 3). But age reversals apparently existed in the upper part of each core. It is notable that the accumulation of the reef core had apparently remained high since the early 1800s. The > 2 mm fraction of sediment in these upper sections was primarily composed of smaller-size coral rubble with abrasion, and their U-Th ages were not in chronological order (Figure 3; Supplementary Table 2). We speculated that the upper parts of each core comprised ex-situ deposits rather than in-situ framework. The reasons probably are that (1) reef accretion had reached the sea level and few in-situ corals had grown, and/or (2) the top parts had been disturbed by island building and dredging (Figures 1C, D). Regardless of the reasons, the top section of each core (approximately since 1800 AD) was excluded in further paleo-ecological reconstruction and statistical analyses. Ultimately, the 29 U-Th ages based on pristine samples revealed that these cores spanned the period 791 ± 6 AD to 1827 ± 3 AD (Figure 3), indicating continual accretion over a period of 1,000 years.
Reef cores are composed of bioclastic gravels (> 2 mm), including coral skeleton fragments, coralline algae, Halimeda, molluscs, and others (e.g., foraminifera shells and sea urchin spines) packed in an unconsolidated sandy matrix of carbonate grains (< 2 mm). The dominant contributors to reef framework development were corals and coralline algae (> 80%; Table 1). Within coral subfossils, taxonomic analysis clearly identified five branching coral genera, Acropora, Montipora, Pocillopora, Seriatopora, and Stylophora, based on their well-preserved skeletons. Acropora and Seriatopora quantitatively dominated throughout the cores (Figure 3). Genus Acropora comprised approximately one-third of the branching subfossils (Table 2). For massive and foliaceous fragments, we identified five genera, Fungia, Galaxea, Goniopora, Pachyseris, and Porites. However, this identification was based on a few relatively large rubble samples with sufficient corallites and distinct microstructures. This number is therefore an underestimate of true genus richness, as most subfossil fragments could not be identified due to poor preservation.
3.2 Living and dead coral assemblages
Based on our line-transect surveys, the substrate of the reef slopes was mostly covered by dead coral rubble (>70%; Figure 10B). Accordingly, the live coral cover was extremely low, ranging from 15.03% at DM-1 in 2016 to 21.77% at MJ-1 in 2017, with an average of 17.12%. Benthic surveys revealed that there were at least 10 families, 20 genera of live corals, with highly variable percentage cover among these coral genera (Supplementary Table 1). There were five genera of branching corals, Acropora, Montipora, Pocillopora, Seriatopora, and Stylophora, consistent with branching subfossils at the genus level. The five branching genera accounted for more than 50% of the living assemblage. Although most colonies were relatively small (Figures 10C−F), Acropora was the most abundant genus, representing 69% of the branching coral assemblage and 41% of the living coral community.
The differences in coral cover between 2016 and 2017 (PERMANOVA, F = 0.14, p = 0.93) and among the three reef sites (PERMANOVA, F = 0.44, p = 0.82) were not significant, suggesting a uniformity of coral community structure associated with different years and habitats. However, significant differences occurred among the 20 genera (PERMANOVA, F = 13.57, p = 0.0001). Four genera, Acropora, Porites, Pocillopora, and Gonistrea, contributed the most to the significance (72%, SIMPER) and were significantly different from other corals (Figure 8).
Dead Acropora skeletons accounted for more than 60% of the substrate (e.g., Figure 10B). Corrected 230Th dates obtained from the 139 dead Acropora samples ranged between 1957.5 ± 2.8 AD and 2016.3 ± 0.8 AD (Figure 6; Supplementary Table 2), indicating a ~60-year history of Acropora mortality. The distribution of U-Th ages revealed that the frequency of mortality events increased dramatically after the 1980s, with 88% of U-Th dates occurring after 1980, and multiple peaks implied repeated episodes of mortality such as in the 1980s, around 1998, and between 2009 and 2014 (Figure 6). The latest mortality episode was constrained to a relatively narrow period but included the two largest peaks at around 2010 and 2013 (Figure 6).
3.3 Subfossil vs. modern coral assemblages
To compare the survey data and the subfossil community data at the genus level, abundances of Acropora, Seriatopora, other branching corals (including Montipora, Pocillopora, and Stylophora), and total branching corals, were standardized to percentage contribution to total living corals and the subfossil coral assemblage, respectively. Statistically significant differences (PERMANOVA, F = 3.974, p = 0.02) existed between the relative abundance of branching corals in assemblages present at the modern reef slopes and the subfossil record preserved in reef cores. The relative abundance of branching corals in the subfossil (1000 years, from 800 AD to 1800 AD) and modern (2016–2017) records was very similar at 57% and 58%, respectively. The most striking differences, however, were in the relative decreases in Seriatopora (7% to 2%), Montipora, Pocillopora, and Stylophora (33% to 16%), and the relative increase in Acropora (17% to 41%) in modern branching assemblages. In other words, the Acropora population comprised 30% of subfossil branching assemblages but comprised more than twice this level (69%) in modern branching communities.
4 Discussion
4.1 Reef resilience persisted for a millennium, but declined contemporarily
Climatic variability, especially frequent thermal anomalies associated with ENSO activities, could be a controlling influence on tropical reef accretion at centennial- to millennial-timescales. For example, stronger and more frequent El Niño events peaked around ~3000 cal yr B.P. and caused a millennial-scale collapse of the reef ecosystem and a resulting hiatus of reef growth in the tropical eastern Pacific (Toth et al., 2012). Similarly, during the last millennium, significantly (PERMANOVA, F = 97.74, p = 0.0001) more El Niño events were revealed between 950 AD and 1350 AD compared to the subsequent 400 years (1400−1800 AD) (Figure 5C). Accordingly, relatively warmer and cooler SSTs during the two 400-yr-intervals in the western tropical Pacific (Figure 5D) generally corresponded to the Medieval Climate Anomaly and the Little Ice Age periods, respectively. A significant difference (PERMANOVA, F = 6.87, p = 0.01) in the abundance of bio-facies was found between the two different El Niño periods (950−1350 AD vs. 1400−1800 AD), primarily due to the changes in coralline algae and coral (83%, SIMPER). The relative abundance of coral (coralline algae) in the Medieval Climate Anomaly decreased ~11% (increased ~14%) on average compared with those in the Little Ice Age (Figure 5A; Table 1). This change could be attributed to the frequent El Niño events (Figure 5C) and thermal stresses (Figure 5D) that caused repeated episodes of coral bleaching and mortality. However, the reef framework accretion was continuous (Figure 3), with no discernible evidence of a hiatus in reef development (e.g., Toth et al., 2012), nor an abrupt transition of the dominant coral genus (e.g., Roff et al., 2013), as described in other reef-core records. Furthermore, no significant differences (PERMANOVA, F = 0.85, p = 0.45) were evident for the subfossil coral community structure (within the branching assemblages and branching vs. non-branching assemblages) between the Medieval Climate Anomaly and Little Ice Age intervals, and the average of each and the total branching coral assemblages were similar (Figure 5B; Table 2), suggesting that the reef system was characterized by a high degree of coral community stability. Such community persistence may be related to long-term resilience of the coral communities to thermal disturbance events.
Before our surveys in 2016 and 2017, the only quantitative records of coral cover at Meiji Atoll could be traced back to the 2007 survey by Li et al. (2011) and Zhao et al. (2013). Although Meiji reef experienced a moderate bleaching event in 2007 (Figure 6, Figure 9A), the ecosystem was generally in a healthy condition with high species diversity (> 90 coral species) and coral cover. For example, the average coral cover on the northern slope (including our sampling site MJ-2) was approximately 50% (Zhao et al., 2013), with a maximum of ~90% (Li et al., 2011; Figure 10A). Branching corals made the greatest contribution (60%) to the coral cover on the reef slope. Among the genera in the branching coral community, the greatest contributor was Acropora, accounting for 21% of the live coral cover. Noticeably, the relative abundances of Acropora and of all branching corals (21% and 60%, respectively) in 2007 were very similar to the corresponding mean values (19.57% and 57.24%, respectively) in the reef cores (Table 2). We therefore speculated that this community structure mode, ~60% of living corals are branching, and one-third of branching corals are Acropora, representing a high degree of coral community stability that can support the persistence and resilience of tropical reef systems.
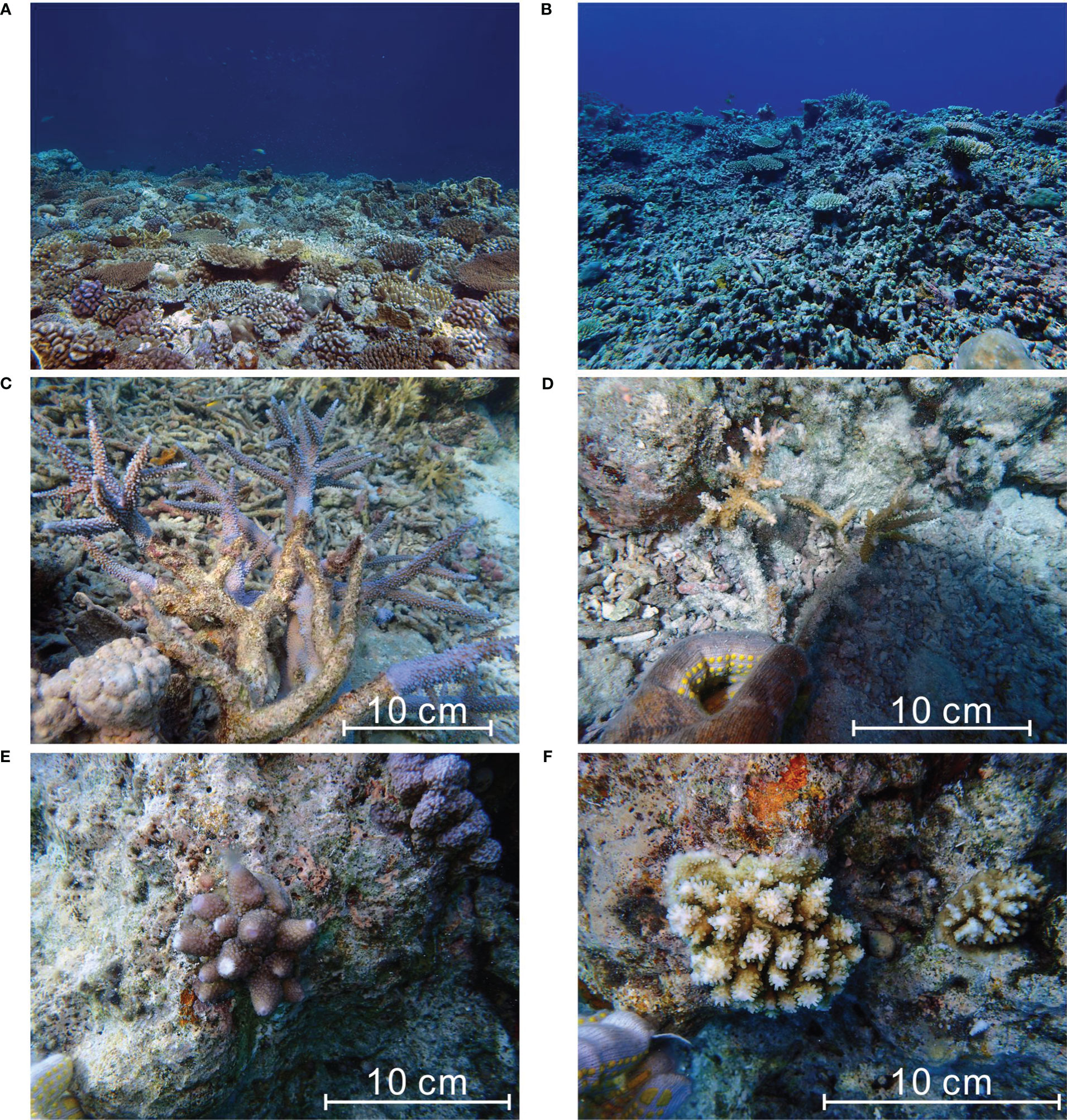
Figure 10 Historical (A; photographed by T. Chen in 2007) vs. modern (B; photographed by T. Chen in 2017) coral communities at the reef slope (MJ-2). Diverse recovery modes of the fast-growing Acropora population, including (C) rapid regeneration of remnant surviving tissue, (D) reproduction by asexual fragmentation, and (E, F) replenishment of juvenile colonies by larvae, were ubiquitous at the sampling sites (photographed by T. Chen). The massive loss of the adult corals, and subsequent shift to the small colonies-dominated or even rubble-dominated states have drastically altered the reef morphology and may further impact the ecological function of coral reefs.
Ten years later in 2017 (when we surveyed the reefs), however, the coral reefs showed a remarkable deterioration, with a relatively low live coral cover and biodiversity (Figure 10B; Supplementary Table 1). The rapid (occurred on a decadal timescale) deterioration of the reef system was undoubtedly attributed to the rate of mortality exceeding the rate of recovery, suggesting a rapid decline in reef resilience. However, this decline did not accompany a shift of coral assemblages toward the weedy and stress-tolerant dominated state, termed the “novel state”, which has been reported from some inshore reefs of western Pacific (e.g., Clark et al., 2017; Chen et al., 2021) and tropical atolls of central Pacific (e.g., Donner and Carilli, 2019; Cannon et al., 2021) from an ecological perspective, or Florida’s reefs (Toth et al., 2019) and Caribbean Panama (O’Dea et al., 2020) from a geologic perspective. In our study, contrarily, the most striking difference between the modern (2016 and 2017) and subfossil reef assemblages was the dramatic increase in the relative abundance of the genus Acropora, likely suggesting another new state of declining reefs, different from the conventional “novel state”, namely stress-tolerant coral-dominated reef state.
4.2 MHWs impaired the reef resilience in recent decades
The CESM1 model simulations implied that modern ENSO amplitude and frequency intensified by an increase in extreme El Niño events eclipsed the paleo-estimates over the Holocene (Lawman et al., 2022). Similarly, oxygen isotopic proxies in tree cellulose from Taiwan (Liu et al., 2017) and fossil corals from Line Islands, central equatorial Pacific (Grothe et al., 2019) implied that ENSO extremes in recent decades were the strongest in centennial- to millennial-scale timeseries. According to modern observations within the past 117 years (Wang et al., 2019), occurrence of extreme events also showed a more frequent pattern, with three out of five extreme El Niño events occurring after 1980 (the 1982–1983, 1997–1998, and 2015–2016 events), along with a trend of El Niño onset regime changing from eastern Pacific to western Pacific since the 1970s. In response to the changing El Niño scenarios, the frequency and intensity of global MHWs and bleaching events have rapidly increased, and consequently the intervals between pairs of severe bleaching events have diminished steadily since the 1980s (Hughes et al., 2018a).
To our knowledge, the 2007 moderate coral bleaching at Meiji Atoll (Li et al., 2011) is to date the only quantitatively recorded and published bleaching event in the Nansha area. In this event, ~27% of corals were bleached, and branching taxa such as Pocillopora and Acropora were the most vulnerable genera. This event correlated well with a moderate-intensity MHW (Figures 9B–D) associated with a weak/moderate El Niño event (Figure 9E). For other years, especially those of strong/extreme El Niño periods (e.g., 1982–1983, 1987–1988, 1997–1998, 2009–2010, and 2015–2016; Figure 9E), recorded basin-wide to global-scale severe bleaching events (Hughes et al., 2018a) provided potential evidence for repeated bleaching and mortality occurring in the Nansha during the past four decades. Here, we use U-Th dating of surficial dead Acropora assemblages to trace historical bleaching events and reef system declines.
Between 1980 and 2010, U-Th dated coral mortality was generally increasing, except for two short-term intervals (~1988–1995 and ~1998–2010) of relatively low coral mortality (Figure 9A). Although the 1982–1983 and 1987–1988 anomalies were identified as strong or extreme events (Figure 9E), they caused relatively short-term MHWs (< 20 days; Figures 9B, C), and likely triggered moderate bleaching and mortality similar to the extent of the 2007 event. In contrast, the U-Th dates showed clear peaks around 1998, 2010, and 2014 (Figure 9A; Supplementary Table 2), suggesting mass losses of branching Acropora (and probably other coral genera) colonies. The 1997–1998 intense coral bleaching, which killed approximately 8% of the world’s coral (Souter et al., 2021), was the first of the three pan-tropical extreme events on record, and the other two were the 2010 and 2015–2016 events (Hughes et al., 2017). Consistent with the extreme El Niño, the intense MHW in the summer of 1998 had the highest maximum SST anomaly (Figures 9B–D) and the longest duration (~70 days). The second was likely the 2010 MHW, with a maximum intensity of 2°C and a duration of ~50 days (Figures 9B, C), that also killed abundant corals (Figure 9A). The relatively low of U-Th dates in the two ~7–12-year periods (before 1998 and 2010, respectively) suggest that there were windows of opportunity for reef systems to recover (indeed, the state of northern Meiji Atoll was still classified as healthy in 2007; Zhao et al., 2013). However, coral communities failed to recover to the “healthy” level due to the following major mortality events, especially after 2010, that have been associated with abrupt heat stress events (Figures 9B–D). The 2013–2014 MHWs and coral mortality were distinct from previous events, because of a neutral phase of ENSO (Figure 9E). This new episode may suggest that coral bleaching is occurring in non-El Niño conditions, because as global warming progresses, summer SSTs (especially in the warm pool) are similar to or even warmer than those during moderate to strong El Niño events only three decades ago (Figure 9D). The 2015–2016 coral bleaching is the latest global-scale severe event and is commonly compared with the 1998 event (e.g., Hughes et al., 2018a; Souter et al., 2021). The intense MHWs (Figures 9B–E), however, did not result in similar or more severe catastrophic mortality. Instead, coral mortality decreased sharply (Figure 9A), because of the low living coral cover associated with the previous thermal disturbances.
Regional trends indicated by our U-Th dates of dead Acropora (Figure 9A) were broadly consistent with the global trend of coral cover and reef decline. A ~10-year window of recovery opportunity led the global average coral cover to recover to the pre-1998 level (33.3%) in 2009, but this was immediately followed by a progressive decrease since 2010, with 14% of corals being lost over the past decade (Souter et al., 2021). This suggests that the Nansha atolls in the Warm Pool are among the globally typical reef systems that are significantly influenced by ENSO variability and climate warming. From regional to global scale, increasing frequency and intensity of MHWs, along with rising summer mean SSTs, have caused thermal disturbances to be characterized as from “acute stresses” in the past to “chronic stresses” contemporarily. As an echo of this, the interval between pairs of recurrent MHWs and bleaching events has diminished from 25 years in the 1980s to only 6 years since 2010 (Hughes et al., 2018a). For some low-latitude regions with naturally higher SSTs such as the Nansha, however, the interval could be shorter than the global average (Figure 9). In a word, recurring coral bleaching events have induced mass coral mortality, precluded coral recovery, and consequently impaired coral reef resilience.
4.3 Tropical atolls still have potential resilience
Branching corals (e.g., acroporid and pocilloporid corals) are normally the most susceptible, whereas massive and encrusting colonies are more robust to bleaching events (e.g., Loya et al., 2001; Li et al., 2011). This is probably the fundamental mechanism underlying the emergence of a “novel reef state” observed in several tropical reef areas (see above). In the Nansha atolls, however, Acropora spp. were dominant across the reef slope, rather than those tolerant coral taxa. The average live coral cover increased from 15.47% in 2016 to 18.77% in 2017, and consequently the dead coral cover decreased from 76.41% to 70.76%, indicating the onset of reef recovery. This recovery was significantly contributed by Acropora colonies (Figure 8; Figures 10C–F; Supplementary Table 1), and the relative abundance of Acropora in live coral communities increased from 35.4% in 2016 to 44.8% in 2017. Based on subsequent reef surveys in 2018–2019 (Tkachenko et al., 2020), juvenile (< 20 cm) Acropora and Pocillopora colonies dominated the survey sites, especially for sites with relatively high coral cover (>30%), suggesting that reef system recovery in the Nansha is ongoing.
Although branching corals, especially Acropora, are susceptible, they are typified by fast growth, structural complexity, and high rates of reproduction, and thus are ecologically classified as “competitive corals” relative to low-growth “weedy” and “stress-tolerant” corals. Despite branching corals being highly susceptible to thermal and hydrodynamic disturbances, their “competitiveness” may enable them to rapidly recolonize reefs after bleaching. For example, evidence from the Great Barrier Reef (Linares et al., 2011; Morais et al., 2021) showed that substantial local recruitment can occur immediately after severe bleaching events due to sufficient larval supply provided by the surviving Acropora population and rapid growth of new colonies (with an average 201% increase in colony size per year). Furthermore, Diaz-Pulido et al. (2009) found an “unusually rapid coral recovery” of branching Acropora corals in the Keppel Islands, Great Barrier Reef (reaching pre-bleaching levels within 12–14 months), due to synergistic effects of factors such as robust tissue regeneration (the ‘‘phoenix effect”) and seasonal dieback in the monospecific seaweed bloom. These recovery modes can also be found in this study (Figures 10C–F).
We suggest that reef systems of tropical atolls like the Nansha retain the potential for resilience and recovery. However, more frequent thermal disturbances and bleaching events in recent decades have resulted in significant shifts of both community and age structures (tending to largely comprise juvenile Acropora colonies) based on historical baselines. Such disproportionate recovery associated with the fecund and fast-growth Acropora relative to corals with weak fertility and low growth will likely not persist if MHWs become more frequent and intense, because the continuous loss of large, reproductive adults (even for Acropora) will slow or prevent reef system recovery on a longer timescale (from years to decades). Increasing recovery time demand but shortening recovery windows presents a paradox for tropical reef systems. The future resilience of tropical atolls (the balance between the coral mortality rate and the coral recovery rate) will depend on the frequency and intensity of El Niño and MHW events in the coming decades.
5 Conclusions
The Nansha atolls developed in the central Warm Pool and periodically exposed to El Niño events create an excellent natural laboratory for characterizing the resilience of low-latitude tropical reefs to thermal regime changes from geological and ecological viewpoints. Our findings are therefore representative of many remote tropical atolls, especially in the Indo-Pacific region. Pre-historical records of quantitative temporal community states based on bio-clastic identification and U-Th dating in the reef cores suggested that the subfossil coral assemblage exhibited no evidence of community shifts attributable to El Niño variability between the Medieval Climate Anomaly and the Little Ice Age, suggesting the long-term persistence of a resilient coral assemblage over these time periods. The more frequent strong/extreme El Niño and MHWs in recent decades have significantly eroded the reef resilience, as characterized by mass loss of corals, coral cover decline, substantially diminished recruitment, and major shifts in coral community assemblages. Future climate change in the coming decades will undoubtedly be critical to determining the trajectory of tropical atolls, be that complete collapse, “novel states” with limited reef functions, or slow but steady recovery.
Data availability statement
The original contributions presented in the study are included in the article/Supplementary Material. Further inquiries can be directed to the corresponding authors.
Author contributions
TZ and TC analyzed the data and produced figures. TC, SLiu and XL coordinated field surveys. TZ wrote the first draft. TC, SLiu and WY acquired funding. TC, TZ, SLiu, SLi and WY revised and edited manuscript. All authors contributed to the article and approved the submitted version.
Funding
This work was supported by the National Science & Technology Fundamental Resources Investigation Program of China (No. 2022FY100600) and the National Science Foundation of China (No. 42076065 and 42176118).
Acknowledgments
We thank Yuexing Feng, Faye Liu, and Ai Nguyen for help with U-Th dating. We also thank Xuefeng Chen and Minhang Hu for help with core sampling during fieldwork.
Conflict of interest
The authors declare that the research was conducted in the absence of any commercial or financial relationships that could be construed as a potential conflict of interest.
Publisher’s note
All claims expressed in this article are solely those of the authors and do not necessarily represent those of their affiliated organizations, or those of the publisher, the editors and the reviewers. Any product that may be evaluated in this article, or claim that may be made by its manufacturer, is not guaranteed or endorsed by the publisher.
Supplementary material
The Supplementary Material for this article can be found online at: https://www.frontiersin.org/articles/10.3389/fmars.2023.1143728/full#supplementary-material
References
Cannon S. E., Aram E., Beiateuea T., Kiareti A., Peter M., Donner S. D. (2021). Coral reefs in the Gilbert islands of Kiribati: Resistance, resilience, and recovery after more than a decade of multiple stressors. PloS One 16 (8), e0255304. doi: 10.1371/journal.pone.0255304
Carballo-Bolaños R., Soto D., Chen C. A. (2020). Thermal stress and resilience of corals in a climate-changing world. J. Mar. Sci. Eng. 8 (1), 15. doi: 10.3390/jmse8010015
Chen T., Li S., Zhao J., Feng Y. (2021). Uranium-thorium dating of coral mortality and community shift in a highly disturbed inshore reef (Weizhou island, northern south China Sea). Sci. Total Environ. 752, 141866. doi: 10.1016/j.scitotenv.2020.141866
Chen T., Roff G., Feng Y., Zhao J. (2019). Tropical sand cays as natural paleocyclone archives. Geophys. Res. Lett. 46 (16), 9796–9803. doi: 10.1029/2019GL084274
Cheung M. W., Hock K., Skirving W., Mumby P. J. (2021). Cumulative bleaching undermines systemic resilience of the great barrier reef. Curr. Biol. 31 (23), 5385–5392. doi: 10.1016/j.cub.2021.09.078
Clark T. R., Roff G., Zhao J., Feng Y., Done T. J., McCook L. J., et al. (2017). U-Th Dating reveals regional-scale decline of branching acropora corals on the great barrier reef over the past century. PNAS. 114 (39), 10350–10355. doi: 10.1073/pnas.1705351114
Clark T. R., Roff G., Zhao J., Feng Y., Done T. J., Pandolfi J. M. (2014). Testing the precision and accuracy of the U-Th chronometer for dating coral mortality events in the last 100 years. Quat. Geochronol. 23, 35–45. doi: 10.1016/j.quageo.2014.05.002
Conroy J. L., Overpeck J. T., Cole J. E., Shanahan T. M., Steinitz-Kannan M. (2008). Holocene Changes in eastern tropical pacific climate inferred from a galápagos lake sediment record. Quat. Sci. Rev. 27 (11-12), 1166–1180. doi: 10.1016/j.quascirev.2008.02.015
DeCarlo T. M., Harrison H. B., Gajdzik L., Alaguarda D., Rodolfo-Metalpa R., D'Olivo J., et al. (2019). Acclimatization of massive reef-building corals to consecutive heatwaves. Proc. R. Soc Ser. B. Biol. Sci. 286 (1898), 20190235. doi: 10.1098/rspb.2019.0235
Diaz-Pulido G., McCook L. J., Dove S., Berkelmans R., Roff G., Kline D. I., et al. (2009). Doom and boom on a resilient reef: Climate change, algal overgrowth and coral recovery. PloS One 4 (4), e5239. doi: 10.1371/journal.pone.0005239
Donner S. D., Carilli J. (2019). Resilience of central pacific reefs subject to frequent heat stress and human disturbance. Sci. Rep. 9, 3484. doi: 10.1038/s41598-019-40150-3
Eakin C. M., Sweatman H., Brainard R. E. (2019). The 2014–2017 global-scale coral bleaching event: insights and impacts. Coral Reefs. 38 (4), 539–545. doi: 10.1007/s00338-019-01844-2
English S., Wilkinson C., Baker V. (1997). Survey manual for tropical marine resources. 2nd Edition (Townsville: Australian Institute of Marine Science).
Fox M. D., Cohen A. L., Rotjan R. D., Mangubhai S., Sandin S. A., Smith J. E., et al. (2021). Increasing coral reef resilience through successive marine heatwaves. Geophys. Res. Lett. 48 (17), e2021GL094128. doi: 10.1029/2021GL094128
Frölicher T. L., Fischer E. M., Gruber N. (2018). Marine heatwaves under global warming. Nature. 560, 360–364. doi: 10.1038/s41586-018-0383-9
Grothe P. R., Cobb K. M., Liguori G., Di Lorenzo E., Capotondi A., Lu Y., et al. (2019). Enhanced El niño–southern oscillation variability in recent decades. Geophys. Res. Lett. 46, e2019GL083906. doi: 10.1029/2019GL083906
Hammer Ø., Harper D. A., Ryan P. D. (2001). PAST: Paleontological statistics software package for education and data analysis. Palaeontol. Electronica. 4 (1), 9.
Hobday A. J., Alexander L. V., Perkins S. E., Smale D. A., Straub S. C., Oliver E. C. J., et al. (2016). A hierarchical approach to defining marine heatwaves. Prog. Oceanogr. 141, 227–238. doi: 10.1016/j.pocean.2015.12.014
Huang H., Jiang L., Yuan T., Liu S. (2021). Reef building scleractinian coral in nansha islands (Beijing: Science Press (in Chinese)).
Hughes T. P., Anderson K. D., Connolly S. R., Heron S. F., Kerry J. T., Lough J. M., et al. (2018a). Spatial and temporal patterns of mass bleaching of corals in the anthropocene. Science. 359 (6371), 80–83. doi: 10.1126/science.aan8048
Hughes T. P., Barnes M. L., Bellwood D. R., Cinner J. E., Cumming G. S., Jackson J. B. C., et al. (2017). Coral reefs in the anthropocene. Nature 546, 82–90. doi: 10.1038/nature22901
Hughes T. P., Graham N. A., Jackson J. B., Mumby P. J., Steneck R. S. (2010). Rising to the challenge of sustaining coral reef resilience. Trends. Ecol. Evol. 25 (11), 633–642. doi: 10.1016/j.tree.2010.07.011
Hughes T. P., Kerry J. T., Baird A. H., Connolly S. R., Chase T. J., Dietzel A., et al. (2019). Global warming impairs stock–recruitment dynamics of corals. Nature 568, 387–390. doi: 10.1038/s41586-019-1081-y
Hughes T. P., Kerry J. T., Baird A. H., Connolly S. R., Dietzel A., Eakin C. M., et al. (2018b). Global warming transforms coral reef assemblages. Nature 556 (7702), 492–496. doi: 10.1038/s41586-018-0041-2
Lawman A. E., Di Nezio P. N., Partin J. W., Dee S. G., Thirumalai K., Quinn T. M. (2022). Unraveling forced responses of extreme El niño variability over the Holocene. Sci. Adv. 8 (9), eabm4313. doi: 10.1126/sciadv.abm4313
Li S., Yu K., Chen T., Shi Q., Zhang H. (2011). Assessment of coral bleaching using symbiotic zooxanthellae density and satellite remote sensing data in the nansha islands, south China Sea. Chin. Sci. Bull. 56 (10), 1031–1037. doi: 10.1007/s11434-011-4390-6
Linares C., Pratchett M. S., Coker D. J. (2011). Recolonisation of acropora hyacinthus following climate-induced coral bleaching on the great barrier reef. Mar. Ecol. Prog. Ser. 438, 97–104. doi: 10.3354/meps09272
Liu Y., Cobb K. M., Song H., Li Q., Li C., Nakatsuka T., et al. (2017). Recent enhancement of central pacific El niño variability relative to last eight centuries. Nat. Commun. 8 (1), 1–8. doi: 10.1038/ncomms15386
Loya Y., Sakai K., Yamazato K., Nakano Y., Sambali H., Van Woesik R. (2001). Coral bleaching: the winners and the losers. Ecol. Lett. 4 (2), 122–131. doi: 10.1046/j.1461-0248.2001.00203.x
Ludwig K. R. (2012). User's manual for isoplot 3.75. special publication no. 5 (Berkeley, California, U.S.A.: Berkeley Geochronology Center U.S.A.).
Mo S., Chen T., Chen Z., Zhang W., Li S. (2022). Marine heatwaves impair the thermal refugia potential of marginal reefs in the northern south China Sea. Sci. Total Environ. 825, 154100. doi: 10.1016/j.scitotenv.2022.154100
Moberg F., Folke C. (1999). Ecological goods and services of coral reef ecosystems. Ecol. Econ. 29 (2), 215–233. doi: 10.1016/S0921-8009(99)00009-9
Morais J., Morais R. A., Tebbett S. B., Pratchett M. S., Bellwood D. R. (2021). Dangerous demographics in post-bleach corals reveal boom-bust versus protracted declines. Sci. Rep. 11 (1), 1–7. doi: 10.1038/s41598-021-98239-7
Moy C. M., Seltzer G. O., Rodbell D. T., Anderson D. M. (2002). Variability of El Niño/Southern oscillation activity at millennial timescales during the Holocene epoch. Nature. 420 (6912), 162–165. doi: 10.1038/nature01194
O’Dea A., Lepore M., Altieri A. H., Chan M., Morales-Saldaña J. M., Muñoz N. H., et al. (2020). Defining variation in pre-human ecosystems can guide conservation: An example from a Caribbean coral reef. Sci. Rep. 10 (1), 1–10. doi: 10.1038/s41598-020-59436-y
Oliver E. C. J., Donat M. G., Burrows M. T., Moore P. J., Smale D. A., Alexander L. V., et al. (2018). Longer and more frequent marine heatwaves over the past century. Nat. Commun. 9 (1), 1–12. doi: 10.1038/s41467-018-03732-9
Oppo D. W., Rosenthal Y., Linsley B. K. (2009). 2,000-year-long temperature and hydrology reconstructions from the indo-pacific warm pool. Nature. 460 (7259), 1113–1116. doi: 10.1038/nature08233
Reynolds R. W., Rayner N. A., Smith T. M., Stokes D. C., Wang W. (2002). An improved in situ and satellite SST analysis for climate. J. Clim. 15 (13), 1609–1625. doi: 10.1175/1520-0442(2002)015<1609:AIISAS>2.0.CO;2
Roff G., Clark T., Reymond C. E., Zhao J., Feng Y., McCook L. J., et al. (2013). Palaeoecological evidence of a historical collapse of corals at pelorus island, inshore great barrier reef, following European settlement. Proc. R. Soc Ser. B Biol. Sci. 280 (1750), 20122100. doi: 10.1098/rspb.2012.2100
Roff G., Mumby P. J. (2012). Global disparity in the resilience of coral reefs. Trends Ecol. Evol. 27 (7), 404–413. doi: 10.1016/j.tree.2012.04.007
Romero-Torres M., Acosta A., Palacio-Castro A. M., Treml E. A., Zapata F. A., Paz-García D. A., et al. (2020). Coral reef resilience to thermal stress in the Eastern tropical pacific. Global Change Biol. 26 (7), 3880–3890. doi: 10.1111/gcb.15126
Schlegel R. W., Smit A. J. (2018). HeatwaveR: A central algorithm for the detection of heatwaves and cold-spells. J. Open Source Software 3 (27), 821. doi: 10.21105/joss.00821
Shaver E. C., McLeod E., Hein M. Y., Palumbi S. R., Quigley. K., Vardi. T., et al. (2022). A roadmap to integrating resilience into the practice of coral reef restoration. Global Change Biol. 28 (16), 4751–4764. doi: 10.1111/gcb.16212
Smale D. A., Wernberg T., Oliver E. C. J., Thomsen M., Harvey B. P., Straub S. C., et al. (2019). Marine heatwaves threaten global biodiversity and the provision of ecosystem services. Nat. Clim. Change. 9 (4), 306–312. doi: 10.1038/s41558-019-0412-1
Souter D., Planes S., Eicquart J., Logan M., Obura D., Staub F. (2021) Status of coral reefs of the world: 2020, executive summary. Available at: https://gcrmn.net/2020-report/.
Speare K. E., Adam T. C., Winslow E. M., Lenihan H. S., Burkepile D. E. (2022). Size-dependent mortality of corals during marine heatwave erodes recovery capacity of a coral reef. Global Change Biol. 28 (4), 1342–1358. doi: 10.1111/gcb.16000
Tkachenko K. S., Hoang D. T., Dang H. N. (2020). Ecological status of coral reefs in the spratly islands, south China Sea (East sea) and its relation to thermal anomalies. Estuar. Coast. Shelf Sci. 238, 106722. doi: 10.1016/j.ecss.2020.106722
Toth L. T., Aronson R. B., Vollmer S. V., Hobbs J. W., Urrego D. H., Cheng H., et al. (2012). ENSO drove 2500-year collapse of eastern pacific coral reefs. Science. 337 (6090), 81–84. doi: 10.1126/science.1221168
Toth L. T., Precht W. F., Modys A. B., Stathakopoulos A., Robbart M. L., Hudson J. H., et al. (2021). Climate and the latitudinal limits of subtropical reef development. Sci. Rep. 11 (1), 1–15. doi: 10.1038/s41598-021-87883-8
Toth L. T., Stathakopoulos A., Kuffner I. B., Ruzicka R. R., Colella M. A., Shinn E. A. (2019). The unprecedented loss of florida's reef-building corals and the emergence of a novel coral-reef assemblage. Ecology. 100 (9), e02781. doi: 10.1002/ecy.2781
Wang B., Luo X., Yang Y., Sun W., Cane M., Cai W., et al. (2019). Historical change of El niño properties sheds light on future changes of extreme El niño. PNAS. 116 (45), 22512–22517. doi: 10.1073/pnas.1911130116
Yadav S., Alcoverro T., Arthur R. (2018). Coral reefs respond to repeated ENSO events with increasing resistance but reduced recovery capacities in the Lakshadweep archipelago. Coral Reefs. 37 (4), 1245–1257. doi: 10.1007/s00338-018-1735-5
Yu K., Zhao J., Shi Q., Meng Q. (2009). Reconstruction of storm/tsunami records over the last 4000 years using transported coral blocks and lagoon sediments in the southern south China Sea. Quat. Int. 195 (1-2), 128–137. doi: 10.1016/j.quaint.2008.05.004
Zhao M., Yu K., Shi Q., Chen T., Zhang H., Chen T. (2013). Coral communities of the remote atoll reefs in the nansha islands, southern south China Sea. Environ. Monit. Assess. 185 (9), 7381–7392. doi: 10.1007/s10661-013-3107-5
Keywords: reef resilience, branching coral, paleo-ecological reconstruction, El Niño, marine heatwaves, tropical atoll
Citation: Zhang T, Chen T, Liu S, Lin X, Li S and Yan W (2023) Coral reef resilience persisted for a millennium but has declined rapidly in recent decades. Front. Mar. Sci. 10:1143728. doi: 10.3389/fmars.2023.1143728
Received: 13 January 2023; Accepted: 10 February 2023;
Published: 23 February 2023.
Edited by:
Wei Jiang, Guangxi University, ChinaReviewed by:
Liang Yi, Tongji University, ChinaShendong Xu, Yantai Institute of Coastal Zone Research (CAS), China
Copyright © 2023 Zhang, Chen, Liu, Lin, Li and Yan. This is an open-access article distributed under the terms of the Creative Commons Attribution License (CC BY). The use, distribution or reproduction in other forums is permitted, provided the original author(s) and the copyright owner(s) are credited and that the original publication in this journal is cited, in accordance with accepted academic practice. No use, distribution or reproduction is permitted which does not comply with these terms.
*Correspondence: Tianran Chen, Y2hlbnRpYW5yYW5Ac2NzaW8uYWMuY24=; Sheng Liu, c2hsaXVAc2NzaW8uYWMuY24=