- 1Fishery College, Zhejiang Ocean University, Zhoushan, China
- 2Laboratory for Marine Fisheries Science and Food Production Processes, Qingdao National Laboratory for Marine Science and Technology, Qingdao, China
Hypoxia and sulfide are inducing potential damage to aquatic organisms. However, the effects of hypoxia and sulfide on their immune systems and molecular mechanisms are not fully understood. In the present study, the clam Tegillarca granosa was exposed to hypoxia alone or in combination with sulfide (0.1, 0.5 mM) to investigate the physiological and transcriptomic responses in haemolymph. The IBR analysis revealed that moderate sulfide stimulated immune responses via increasing the total hemocyte counts, phagocytic activity, antibacterial activity, and antioxidant activity. The transcriptomic analysis revealed many critical signaling pathways (Toll and Imd, FoxO, NLR) and biological processes (antimicrobial/antibacterial peptide, interferon, interleukin, leukocyte, lymphocyte, mitophagy) involved in the immunostimulation. Our results would offer insights into the sulfide-tolerant molecular mechanisms in this species and provide a useful tool for assessing the integrated biological impacts of hypoxia and sulfide on shellfish.
1 Introduction
Since the mid to 20 th century, aquatic hypoxia caused by water eutrophication has expanded more and more quickly in worldwide ocean, especially in gulfs, lakes, estuaries, and aquaculture farming zones (Diaz, 2001; Bocaniov and Scavia, 2016; Saleh et al., 2021; Whitney and Vlahos, 2021). Recently, aquatic hypoxia has become a worldwide concern for environmental issues as it can reduce biodiversity and change community structures in aquatic ecosystem (Gobler and Baumann, 2016; Zhai et al., 2019). It also has direct impacts on the behavior, physiology, and immunity of aquatic animals (Kvamme et al., 2013; Abdel-Tawwab et al., 2019; Zheng et al., 2022). Sulfide, which is produced under anaerobic conditions through decomposition of organic matter and reduction of sulfate, is commonly found in the bottom layer and sediment of aquatic environments (Cutter and Krahforst, 1988; Asaoka et al., 2018). According to previous studies, the content of sulfide could reach to high levels and retained for a long time under hypoxic condition, which means benthic fauna is easily co-exposed to both hypoxia and sulfide conditions (Jørgensen, 1990; Wang and Zhang, 2021). Most commercial marine bivalves are bred in the coastal areas where sulfide and hypoxia frequently happen (Bagarinao and Lantin-Olaguer, 1998; Chang et al., 2013; Kodama et al., 2018; Wang et al., 2021). For instance, the content of sulfide in the sediment of Gamo Lagoon could stay at 6.6 mmol/L during warmer months (Kanaya et al., 2016; Asaoka et al., 2018). Evidences have indicated that high content of sulfide (1 mmol/L) was likely to become lethal level to most aquatic fauna including benthic bivalves, nematodes and polychaete species (Affonso and Rantin, 2005; Hargrave et al., 2008). Due to limited mobility to escape, these species easily suffer from the stressors (Aelion and Warttinger, 2009; Asaoka et al., 2018; Losyuk et al., 2021). Hence, prolonged exposure of hypoxia and sulfide has become a great threat for coastal farming organisms (Christensen et al., 2003; Srithongouthai and Tada, 2017; Meng et al., 2019).
Many studies on marine invertebrates suggested that sulfide exposure could be related to immunosuppression. For instance, 48 h exposure to 0.4-4 mg/L sulfide decreased the total haemocyte count (THC) and phagocytic activity of the haemocytes in the shrimp Macrobrachium nipponense, and higher level of sulfide (1.2-4.4 mg/L) remarkably restrained the total antioxidant capacity (Guan et al., 2011). Concentrations of sulfide higher than 528 μg/L increased the susceptibility of kuruma shrimp Marsupenaeus japonicus against Vibrio alginolyticus infection by a depression in immune responses including THC, phenoloxidase activity, phagocytic activity and bacterial clearance efficiency (Cheng et al., 2007; Xu et al., 2014; Duan et al., 2018; Jiang et al., 2019; Hu et al., 2021). Similar effects were also detected in the shrimp Litopenaeus vannamei and crab Charybdis japonica (Cheng et al., 2007; Xu et al., 2014; Duan et al., 2018; Jiang et al., 2019; Hu et al., 2021). Although many researchers have treated sulfide as an adverse factor for aquatic animals, others found some macrobenthos make use of sulfide. Recently, increasing evidences confirm the essential roles of mitochondrial alternative respiration and sulfide oxidation chains in adaption to sulfide exposure in benthic invertebrates, including polychaetes, crustaceans and bivalves (Grieshaber and V¨olkel, 1998; Hildebrandt and Grieshaber, 2008a; Hildebrandt and Grieshaber, 2008b; Huang et al., 2013; Ren et al., 2015). Studies in the clam Anadara broughtonii revealed that the toxicity of sulfide could be mitigated by its own physiologic functions including energy-balancing in the mitochondrial alternative chains, ROS cleansing, and apoptosis regulating (Wang and Zhang, 2021). Even though it is still not certain how sulfide mediate the oxidative or immune stress in many of these sulfide-tolerant invertebrates, sulfide is widely accepted to be a signaling molecule that exhibits some potentially beneficial therapeutic effects at physiological concentrations, including attenuating oxidative stresses (Kimura and Kimura, 2004; Kimura et al., 2010; Lan et al., 2011; Yang et al., 2011; Sun et al., 2012; Hu et al., 2018; Kimura et al., 2019). Most recently, the important roles of sulfide in inflammation and immunity-related processes have also been noticed. Researchers put forward new therapeutic approaches using H2S donors in inflammation and immune response, regarding H2S as not only a gasotransmitter, but also a key immunomodulatory factor (Yuan et al., 2017; Li et al., 2021). The effects of sulfide on marine invertebrate species have not been adequately investigated, and its impacts on the immunotoxicity of invertebrates are still unknown and need further investigation.
The blood clam Tegillarca granosa, a typical marine bivalve that is of commercial importance and widely distributed in the West Pacific coasts, inhabits flow-retarding mudflat or enclosing mud ponds (Shi et al., 2017; Zha et al., 2019; Zhai et al., 2019; Bao et al., 2021). The muddy feature of the habitat makes this species easy to suffer from hypoxia and sulfide exposure. According to the latest study, the sulfide concentration of the sediment porewater was normally 100-200 μmol/L in Chinese typical habitat of blood clams (Liu et al., 2022). While the concentration could be higher in hot weather. In other words, this species must find ways to live with hypoxia and sulfide. In bivalves, the non-specific immune system plays vital roles in resisting disease and keeping healthy. However, the immune responses can be greatly affected by the environmental factors through either ameliorating or exacerbating the disease (Mishra et al., 2015; Sui et al., 2016; Zanuzzo et al., 2020; Pozzi et al., 2022). Hence, it is meaningful to uncover how the immune responses to hypoxia and sulfide happen in this species. Like the other invertebrates that are lack of specific immune system, T. granosa depends on the haemocyte phagocytosis and various active factors released from haemolymph to mediate non-specific immune responses to virus, bacteria, and pollutants (Su et al., 2018). The latest study on the clams showed that high sulfide concentration (1 mM, 7 days) induced hemocyte toxicity and microbiota dysbiosis, as indicated by THC, cell viability, ROS levels, phagocytic activities, and microbial community structure (Liu et al., 2022). In the studies on other aquatic invertebrates, the humoral factors including lysozyme (LZM), peroxidase (POD), acid phosphatase (ACP), alkaline phosphatase (AKP), superoxide dismutase (SOD), catalase (CAT) are commonly used indicators of the immunocompetence (Li et al., 2009; Xia and Wu, 2018; Bao et al., 2020). These antioxidant, phagocytosis and antibacterial defense systems coordinate the immune responses to biotic and abiotic factors (Jiang et al., 2017; Han et al., 2021b; Zhang et al., 2021). Evidences in some aquatic invertebrates also indicated that immune responses including phagocytosis of haemocytes could be regulated by several crosslinked molecular pathways (Huang et al., 2019; Nie et al., 2020). For instances, activation of mammalian NFκB signaling pathway not only enhance immune responses, but also counteract with haemocytes apoptosis signaling in haemocytes by activating B-cell lymphoma-2 (Bcl-2), which inhibits the activation of apoptosis executor, the caspases (Vallabhapurapu and Karin, 2009). The study on the clam A. broughtonii found that exposure to sulfide significantly enriched genes related to apoptosis, TNF and NFκB signaling pathways which could mediate the inflammation and immune defense, and two-sided effects of sulfide on mitochondrial apoptotic cascade were also detected (Wang et al., 2019; Wang et al., 2021). These cases provide references for the studies of immune responses to hypoxia and sulfide in the clam T. granosa.
Therefore, to better understand the immunotoxic effects of sulfide and hypoxia on T. granosa, we evaluated the haemocyte immune function using the cellular and humoral indicators. We also investigated the underlying molecular mechanisms using transcriptomic analysis.
2 Materials and methods
2.1 Animal collection and acclimation conditions
Adult 2-year old clams (shell length of 31.13 ± 0.37 mm, mean ± SE) were obtained from Xiangshan, China (29°24’ N and 121°25’ E) in April 2021. The individuals were acclimated in circulating seawater systems, which were filled with filtered natural seawater (temperature 19.93 ± 0.22 °C, salinity 25 ± 0.1 ‰, dissolved oxygen 9.05 ± 0.07 mg/L, PH = 8.1). During the acclimation period, the clams were fed twice a day with diatom Cylindrotheca fusiformis at a rate of 5% of the tissue dry weight until one day before the experiment. The clams were not fed during the experiment, and three hundred individuals were randomly and evenly assigned to twelve experimental buckets (25 individuals in each experimental bucket). Each bucket was sealable and filled with five liters seawater that has been disinfected and filtered through 0.45 μm filter membrane.
2.2 Experimental design
The clams were exposed to hypoxia alone or sulfide in combination for 96 h. According to the results of pre-experiment and studies on the clams, 0.1mM and 0.5mM was defined as the mild and moderate level of sulfide, respectively (Liu et al., 2022). Four experimental groups were designed in triplicate, including (1) control group (DO = 9 mg/L, C); (2) hypoxia group (DO ≤ 0.5 mg/L, Q); (3) hypoxia and mild-sulfide group (DO ≤ 0.5 mg/L, 0.1 mM sulfide, QD); (4) hypoxia and moderate-sulfide group (DO ≤ 0.5 mg/L, 0.5 mM sulfide, QG). The hypoxic seawater was prepared by bubbling with pure nitrogen until DO ≤ 0.5 mg/L in a storage tank which was connected to the experimental buckets. The concentration of sulfide was acquired by adding the sulfide stock solution (0.1 M, pH = 8.0 ± 0.1), which was prepared with Na2S·9H2O in oxygen-free water and were determined by the methylene blue method (Reese et al., 2011). Based on our preliminary experiment on the clam, to maintain target DO and sulfide levels (100% ± 10% of target concentration), and water quality (nitrite< 0.01 mg/L, ammonia nitrogen< 0.2 mg/L) at the given loading density with clams, the experimental water was completely renewed every 4–6 h.
2.3 Analyses of THC and phagocytic activity
After 96 h of exposure, three individual clams from each replicate bucket were randomly selected to collect the haemolymph. The samples were immediately used for measurements of THC and phagocytic activity by using the method described by Tang et al. (Tang et al., 2020). Briefly, a volume of 100 µL haemolymph extracted individually from the cavity using a 1 milliliter syringe. The extract that was used for the THC was diluted with 100 µL 2.5% glutaraldehyde and 800 µL of phosphate buffered saline (PBS, pH at 7.4) in a 1.5 mL centrifuge tube. The THC was estimated with a hemocytometer under an microscope at magnification of 400 ×. The extract that was used for phagocytic analysis was mixed with 100 µL Alsever’s solution. The mixtures was added yeast suspensions (5.80 ± 0.03 × 107/mL) at a haemocyte: yeast ratio of 10: 1 and followed by an incubation at 25°C for 30 min. After fixation with 100 µL 2.5% glutaraldehyde, blood smears were made and stained with Wright’s Gimesa stain, and the phagocytic rate and phagocytic index of haemocytes were determined at magnification of 400 × under a microscope. To ensure data accuracy of experiments, more than 200 haemocytes were calculated for each sample. The phagocytic rate refers to the number of 200 haemocytes participating in phagocytosis divided by the total number of haemocytes in the visual field. The phagocytic index refers to the total number of phagocytosed yeast divided by the total number of haemocytes participating in phagocytosis in the visual field.
2.4 Measurement of immune-related enzyme activities
The haemolymph samples that were extracted from nine individuals in each group were centrifuged at 800 g for 10 min. The supernatant was collected to determine the activities of immune-related enzymes including SOD, CAT, ACP, AKP, POD, LZM. The supernatant was either immediately used for measurements or snap-frozen in liquid nitrogen and stored at -80 °C for later usage. According to the methods described and tested by Bao et al. (Bao et al., 2020), Nan Xiang et al. (Xiang et al., 2017) and Cui et al. (Cui et al., 2020), we use spectrophotometric assays (Jiancheng Bioengineering Institute, Nanjing, China) with a microplate reader (SPARK, Groding, Austria) to determine the activities or contents. SOD, CAT and POD were expressed as U/mL, ACP and AKP activities were expressed as U/L, LZM contents were expressed as μg/mL, respectively.
2.5 Integrated biological response index analysis on the biomarkers
The integrated biomarker response (IBR) index analysis was performed for all immune-related biomarkers measured, and the IBR was calculated according to the method described by Beliaeff and Burgeot (Beliaeff and Burgeot, 2002). The IBR index was computed as follows: (1) Calculation of the mean value (X) for per biomarker at each treatment and sampling time, as well as the total mean value (m) and standard deviation (s) for each biomarker. (2) Standardization of the response data for per biomarker: Y = (X - m)/s, where Y is the standardized value of the biomarker. (3) Use standardized value, the Zi was defined as +Yi or -Yi if a biomarker in the case of activation or inhibition, and the absolute minimum value (|Z min|) of per biomarker at all sampling times was obtained. (4) The score Si for per biomarker was calculated as Si = Y + |Z min|, where Si can be shown by the length of the radiation line in the star plots. (5) The corresponding IBR value was calculated according to the following formula:
where n represented the number of biomarkers.
2.6 RNA extraction and samples preparation
The haemolymph from three individuals of C (here in after referred to as N), Q, QG groups were sampled after 24 h challenge, respectively. The total RNA was extracted following the manufacturer’s instructions (Invitrogen) and treated with DNase (Promega RQ1 DNase I, Madison, WI, USA) to remove genomic DNA contamination. RNA degradation and contamination were monitored on 1% agarose gels. RNA concentration and quality were determined using the Agilent Bioanalyzer 2100 system (Agilent Technologies, Palo Alto, CA, USA).
2.7 Library construction and Illumina sequencing
Nine cDNA libraries were constructed from T. granosa in the sulfide challenged group QG (QG1, QG2 and QG3), hypoxia challenged group Q (Q1, Q2 and Q3), and normoxia control group N (N1, N2 and N3) for RNA-seq analysis. The RNA-seq libraries were performed at Biomarker Technologies Co., Ltd. (Beijing, China) following the manufacturer’s recommendations. The mRNA was isolated by Oligo (dT) and fragmented by NEBNext First Strand Synthesis Reaction Buffer. The fragments were amplified for the synthesis of first- and second-strand cDNA using random hexamers. The cDNA libraries were built after the PCR products being purified using the AMPure XP system and were then assessed on the Agilent Bioanalyzer 2100 system. At last, the libraries were sequenced on an Illumina Hiseq 2500 platform.
2.8 Assembling and functional annotation
Clean reads were obtained by removing reads containing adapters, ploy-N and low-quality reads from raw data. The remaining high quality clean reads were assembled using Trinity for transcriptome assembly without reference genome (Grabherr et al., 2011). The longest transcript of each single gene was selected as a unigene. For annotation analysis, unigenes were BLASTX-searched against eight databases, including the NCBI non-redundant protein sequence (Nr), Protein family (Pfam), Clusters of Orthologous Groups (KOG/COG), Gene Ontology (GO), Kyoto Encyclopedia of Genes and Genomes (KEGG) Orthology (KO), Evolutionary Genealogy of Genes: Non-supervised Orthologous Groups (eggNOG), TrEMBL, and the Swiss-prot database, using a cut-off E-value of 10−5.
2.9 Differential expression and enrichment analyses
Differentially expressed genes (DEGs) were measured by counting tags from hypoxia/sulfide treated samples against the control, which were normalized using the RNA Sequence Expected Maximization (RSEM) method (Li and Dewey, 2011). Unigenes with adjusted P-value (Padj)< 0.05 and |log2 (fold change)| > 0 were set as the DEGs. The annotated DEGs were further assigned to GO, KEGG enrichment analysis, and the annotated unigenes were performed gene set enrichment analysis (GSEA) based on the GO and KEGG database. GSEA ranks genes based on the degree of differential expression between two groups, followed by testing whether the gene set is significantly enriched at the top or bottom of the ranking list. Rather than focusing on individual genes, this analysis evaluates the expression of the whole transcriptome, which enables the inclusion and analysis of genes with more subtle changes (Aravind Subramanian et al., 2005).
2.10 Validation of DEGs by quantitative real-time PCR
Six DEGs were randomly selected for the validation of DGE data by quantitative real-time PCR (qRT-PCR) in the QG vs. C groups. The 18s rRNA of T. granosa (GenBank accession no. JN974506.1) was used as the internal control (Han et al., 2021a). Each sample with a total mixture of 20 μl was reacted by the following program: denaturation at 95°C for 5 min, followed by 40 cycles of amplification (95°C for 10 s, 60°C for 20 s for annealing, and 72°C for 30 s for extension). The primer sequences of the tested genes used in the qRT-PCR study were designed by Primer Premier 5 software (Table 1). The expression level of different genes was analyzed using the 2−ΔΔCT method (Pfaffl, 2001).
2.11 Statistical analysis
Indicators of the humoral factors were analysed using one-way ANOVA followed by Tukey’s post-hoc tests. For all analyses, Levene’s tests and Shapiro-Wilk’s tests were used to verify the homogeneity of variances and normality, respectively. The statistical analyses were carried out using Origin-Pro 8.0 software package, and P value< 0.05 was described as significant difference.
3 Results
3.1 Effects of hypoxia and sulfide on the THC and phagocytic activity of haemocytes
THC levels in all treatment groups showed similar temporal trends. In Q, QD, and QG group, THC reached to the maximum level during 12 h - 24 h and was significantly higher than initial level (P< 0.05). Since then, THC gradually dropped to the minimum level at 96 h and was significantly lower than the control level (P< 0.05) (Figure 1A).
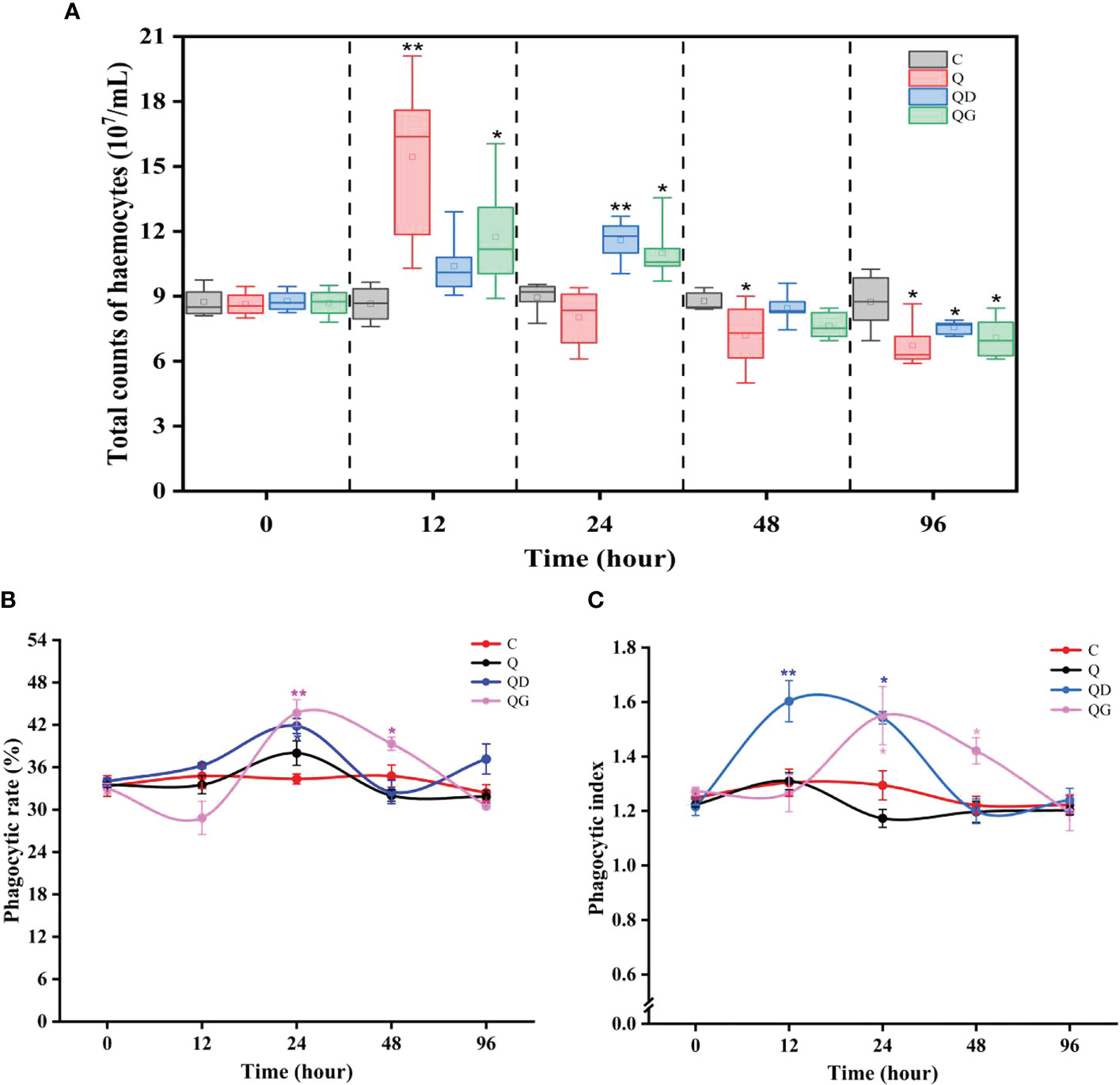
Figure 1 Total count of haemocytes (A), phagocytic rate of haemocytes (B), and phagocytic index of haemocytes (C). C, Q, QD, and QG stand for the control group, hypoxia group, hypoxia and mild-sulfide group, hypoxia and high-sulfide group, respectively. The asterisk * and ** indicate significant difference (P< 0.05) and highly significant difference (P< 0.01) compare to the control.
In the C and Q groups, the phagocytic rate and phagocytic index showed no significant difference. However, the phagocytic rate and phagocytic index in the QD group significantly increased during 12 h - 24 h. The phagocytic rate and phagocytic index in the QG group significantly increased during 24 h - 48 h (P< 0.05) (Figures 1B, C).
3.2 Effects of hypoxia and sulfide on the activities of immune-related enzymes
SOD activities in the C and Q groups remained stable in 96 h (28.66 ± 0.31). SOD in the QD group gradually increased since 24 h and was significantly higher than the Q and QG levels at 96 h (P< 0.05). SOD in the QG group only increased at 24 h and was significantly higher than that in Q group (P< 0.05) (Figure 2A).
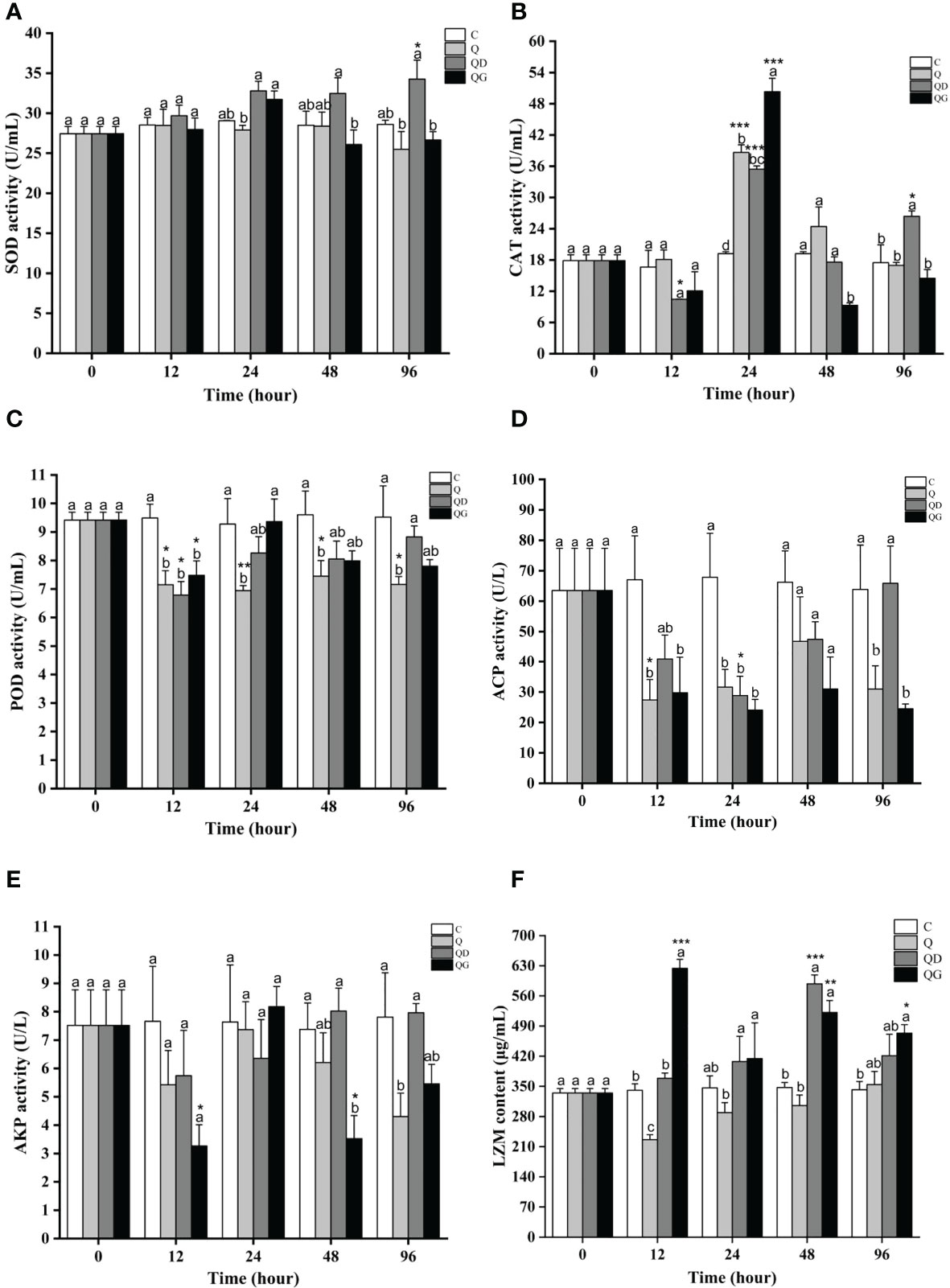
Figure 2 The activities of immune-related enzymes after 96 h of exposure to hypoxia alone or sulfide in combination (C, Q, QD, and QG stand for the control group, hypoxia group, hypoxia and mild-sulfide group, hypoxia and high-sulfide group, respectively). Different letters indicate values that are significantly different among the treatments within each time point, and the asterisk *, ** and *** indicate significant difference (P< 0.05) and highly significant difference (P< 0.01, P< 0.001) under the same treatment conditions. (A): SOD activity; (B): CAT activity; (C): POD activity; (D): ACP activity; (E): AKP activity; (F): LZM content.
CAT activities in all treatment groups reached to their maximum levels at 24 h and have significant differences with the initial levels (P< 0.05). Besides, CAT in the QD group also significantly increased at 96 h (P< 0.05). At 48 h, CAT in the QG group was significantly lower than that in the other groups (Figure 2B).
POD activities were significantly inhibited in all treatment groups at 12 h (P< 0.05). Since then, POD in the Q group was stable at 7.19 ± 0.11, and that in the QD and QG groups gradually increased to initial levels. At 96 h, POD in the QD group was significantly higher than that in the Q group (P< 0.05) (Figure 2C).
ACP activity in the Q group was significantly inhibited at 12 h, 24 h, 48 h (P< 0.05), while was recovered at 96 h. ACP in the QD group gradually decreased to the minimum level (0.45 folds, P< 0.05) at 24 h and was recovered to initial level since then. ACP in the QG group was significantly inhibited since 12 h (P< 0.05) and remained at relatively low level (0.37 folds - 0.48 folds) (Figure 2D).
AKP activity in the Q group was relatively stable in 48 h (5.42 - 7.66 U/L), but significantly dropped to 0.55 folds lower than the initial level at 96 h (P< 0.05). AKP in the QD group was relatively stable (5.74 - 8.02 U/L) while that in the QG group significantly dropped at 12 h (0.42 folds) and 48 h (0.45 folds) (P< 0.05) (Figure 2E).
LZM activity in the Q group had a significant decrease at 12 h (0.67 folds, P< 0.05). In the QD group, LZM significantly increased since 48 h (1.25 - 1.76 folds, P< 0.05). In the QG group, LZM increased significantly at 12 h (1.86 folds) and 48 h - 96 h (1.42 - 1.56 folds) (P< 0.05) (Figure 2F). At 24 h, LZM in the QD and QG groups were significantly higher than that in the Q group (P< 0.05).
3.3 Integration of biomarker responses in immune-related biomarkers
By comparing the coverage areas of the star plots, the IBR indexes of Q and QG groups (the control value was standardized to 0) fluctuated with increasing time (Figure 3). To further investigate the integrated responses, the biomarkers were assembled into 3 categories, including cellular immune indexes (THC, PR, PI), antioxidant indexes (SOD, CAT, POD), and antibacterial indexes (ACP, AKP, LZM). The IBR values for cellular immune, antioxidant, and antibacterial responses in the Q group stay low for 96 h. While in the QD and QG groups, the IBR values for the cellular immune indexes were remarkably high at 24 h (QD=2.13, QG=2.45). In the QG group, antibacterial response was inhibited at 12 h and the antioxidant response was also decreased at 48 - 96 h. At 96 h, the cellular immune responses, antibacterial responses, and antioxidant responses in the QD group were much higher than those in other groups.
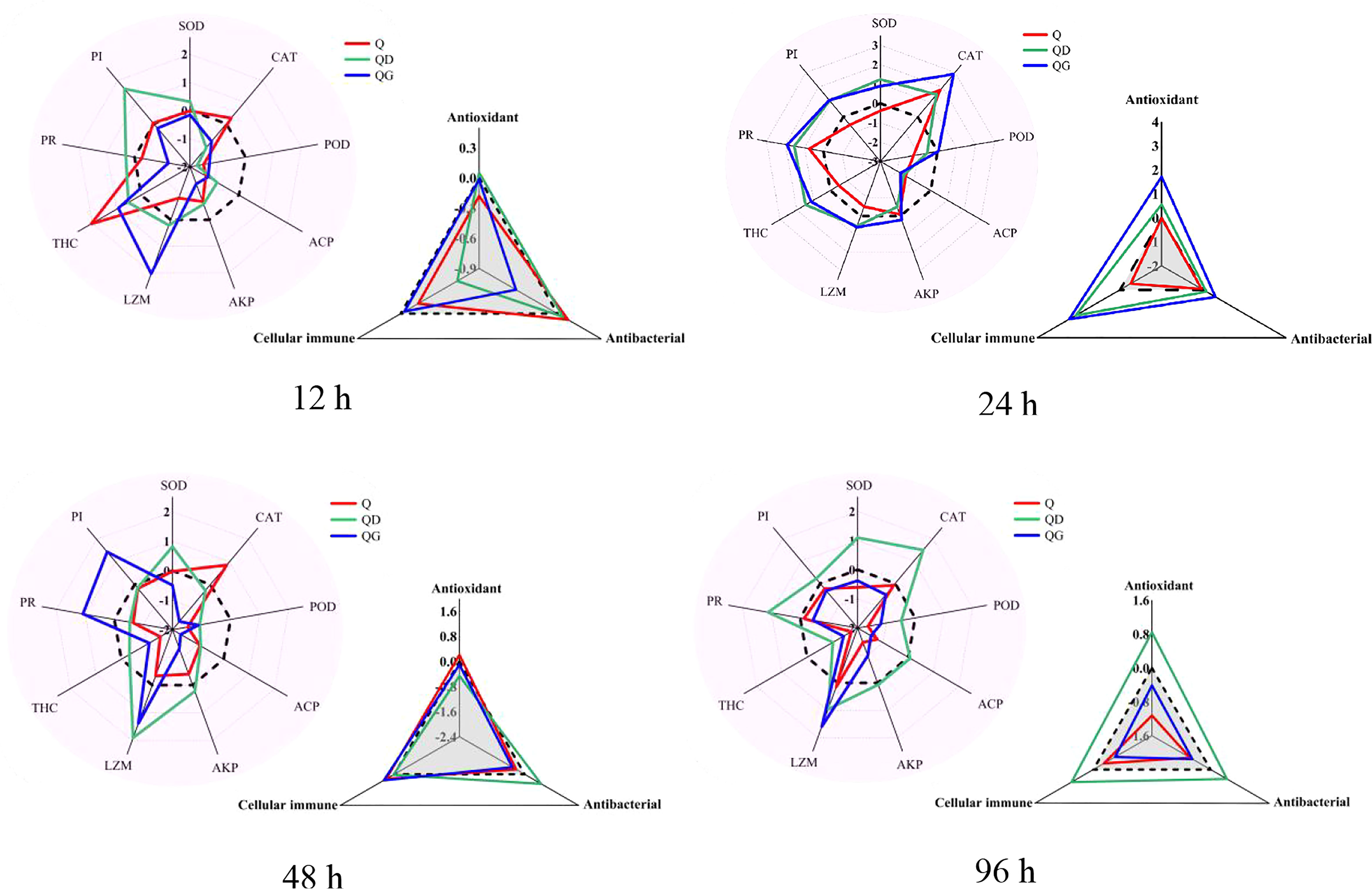
Figure 3 Star plots and the integrated biomarker response (IBR) of immune-related biomarkers in the adult Tegillarca granosa exposed to hypoxia and sulfide. Zero, Q, QD, QG, stand for the control level, hypoxia group, hypoxia and mild-sulfide group, hypoxia and high-sulfide group; THC, Total count of haemocytes; PR, phagocytic rate; PI, phagocytic index; SOD, superoxide dismutases; CAT, catalase; POD, peroxidase; ACP, acid phosphatase; AKP, alkaline phosphatase; LZM, lysozyme.
3.4 Sequencing assessment, gene identification, and annotation of DEGs
To better understand the underlying molecular mechanism of the immunostimulation that was induced by moderate sulfide (0.5 mM, 24 h), a transcriptomic analysis was carried out. The Illumina sequencing yielded more than 60 million reads from the control, hypoxia and moderate-sulfide groups (Table S1). A total of 57,583 unigenes were obtained with an average length of 1417 bp (N50 length: 2351 bp). The statistics of the unigenes annotated in public databases were shown in Table 2. In the distribution of annotated unigenes in Nr, 22.60% unigenes have similarities with Pecten maximus, followed by Mizuhopecten yessoensis (21.04%), Mytilus coruscus (14.92%), Crassostrea gigas (14.66%) (Figure 4A). A total of 536 DEGs were detected from all the stressed groups. Among them, 102 DEGs were detected between N vs. Q groups, 423 DEGs were detected between N vs. QG groups, 123 DEGs were detected between Q vs. QG groups, and only 7 DEGs were commonly annotated in the stressed groups (Figure 4B). The heatmap and hierarchical clustering of the DEGs revealed differences between the control, hypoxia, and sulfide groups (Figure 4C).
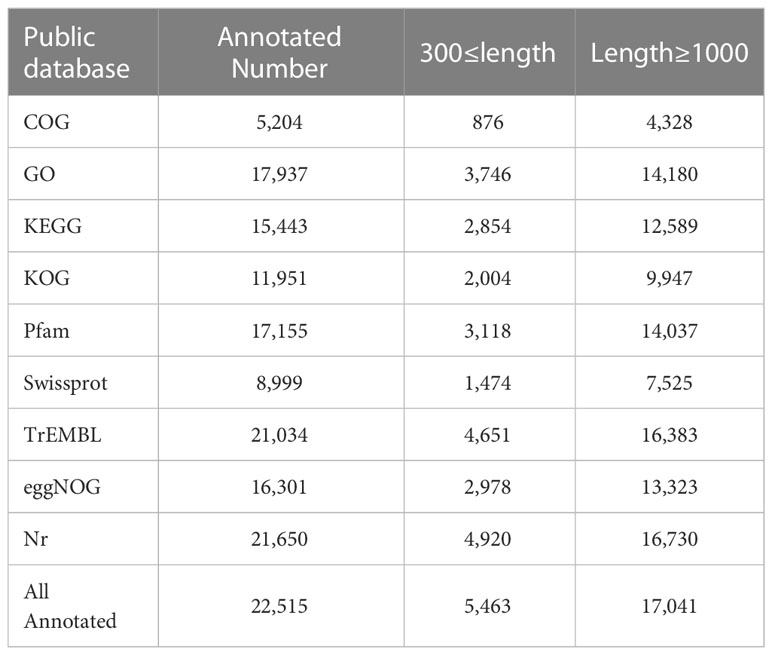
Table 2 Summary statistics of functional annotation of Tegillarca granosa unigenes in public databases.
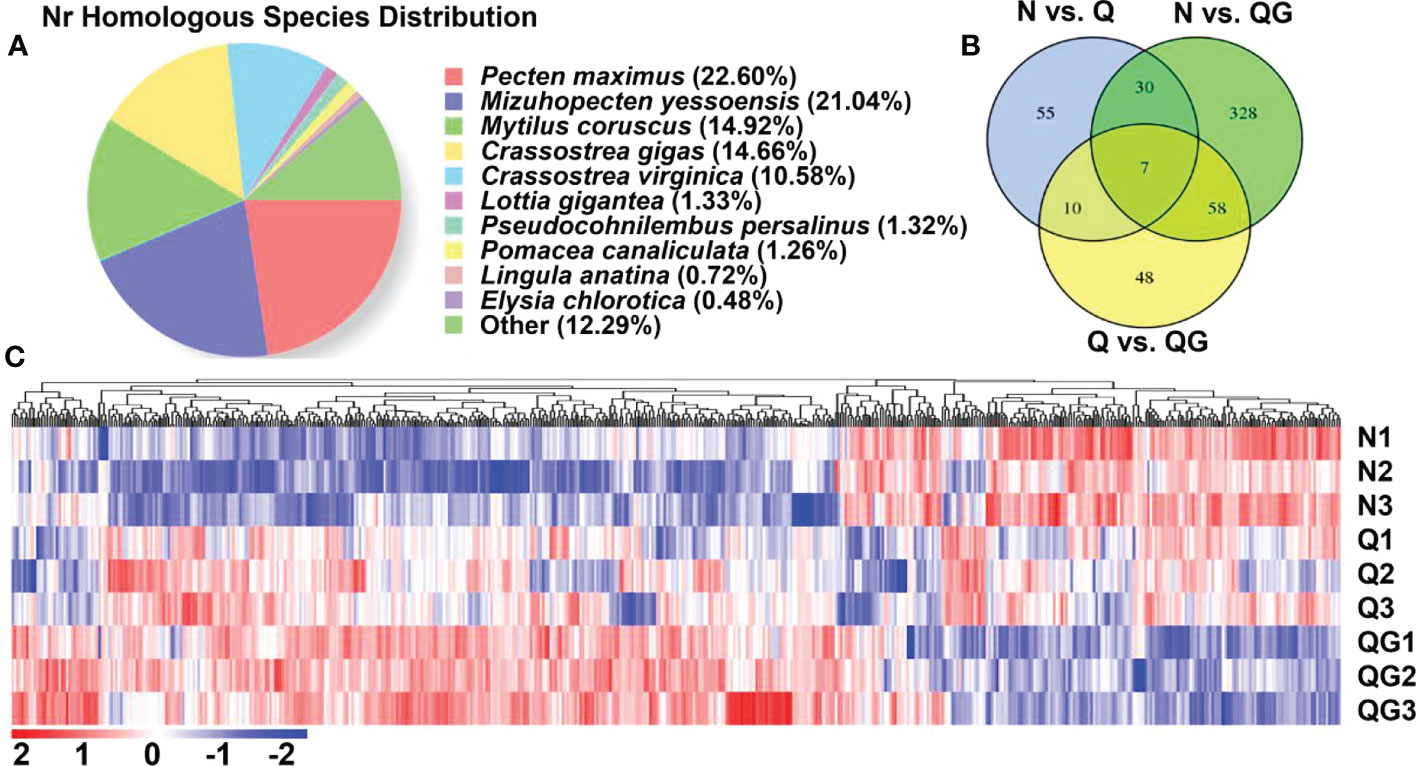
Figure 4 Nr homologous species distribution of the annotated unigenes (A); Venn diagram of the differentially expressed unigenes (DEGs) (B); Hierarchical cluster of the DEGs (C).
3.5 Enrichment analysis of DEGs
Many genes related to immuno-regulation and apoptotic process were detected in the DEGs (Additional file 1). In N vs. Q groups, we found immuno-related gene tollo, which plays key role in Toll and Imd signaling pathway, was significantly up-regulated. While the immuno-related genes nlrp3, hmcn1 were significantly down-regulated. We also noticed that calcium-binding related gene eip63F-1 was significantly inhibited. In N vs. QG groups, many immuno-related genes, including cd22, tollo, foxo, gimap4, sting, ileu, birc2/3, gadd45a, src, sqstml, trim56, dhx15, lbp, irf2, and klhl20, were significantly up-regulated. Besides, genes involved in MAPK signaling pathway (dusp1/10, gadd45a, jun, aifm3) and calcium signaling pathway (cckar, octb2) were significantly up-regulated. In Q vs. QG groups, genes involved in immunity (cd22, tlr13, irf1/2, birc2/3, sqstml), MAPK signaling pathway (dusp1, gadd45a, aifm3) and calcium signaling pathway (cckar) were significantly up-regulated.
GO classification was performed to identify the cellular, biological and molecular processes associated with hypoxia and sulfide exposure in T. granosa. DEGs of three comparisons (N vs. Q; N vs. QG; and Q vs. QG) were assigned to three GO categories (biological process, BP; cellular components, CC; molecular functions, MF) and 60 GO terms (Figure S1). In N vs. QG and Q vs. QG groups, cellular process, integral component of membrane and protein binding significantly enriched in the biological process, cellular components, molecular functions, respectively.
KEGG pathway analysis was performed to identify the broad functional categories of DEGs. Several signaling pathways were enriched in glutathione metabolism, arachidonic acid metabolism, FoxO, MAPK, NOD-like receptor (NLR), and Toll and Imd (Figure S2). These pathways were related to processes of metabolism, apoptosis, and immunity.
We used GSEA analysis to dig the responsive immuno-related pathways based on the KEGG and GO annotation on the gene set of unigenes. In the GO database, the gene set was significantly enriched (|NES| > 1, Pvalue< 0.05, qvalue< 0.25) in many immuno-related “biological process” (Additional file 2). In N vs. Q group, a total of 10 immuno-related terms, involving in autophagy, macrophage activation, and toll-like receptor signaling pathway (Toll), were identified in 78 positively and significantly enriched terms (NES > 1, Pvalue< 0.05, qvalue< 0.25). Moreover, the cellular response to virus (GO:0098586) was identified as negatively and significantly enriched terms (NES< -1, Pvalue< 0.05, qvalue< 0.25). In N vs. QG group, over a third (125/353) of positively and significantly enriched terms had clear connection with immune responses, involving the responses of antimicrobial/antibacterial peptide, interferon, interleukin, leukocyte, lymphocyte, and Toll. Moreover, only two immuno-related terms were identified in the negatively and significantly enriched terms. In Q vs. QG group, over a forth (119/440) of positively and significantly enriched terms involved in the similar immune responses to those in N vs. QG group, and only six immuno-related terms were identified in the negatively and significantly enriched terms. In the N vs. QG and Q vs. QG groups, many terms related to MAPK, TNF, and Apoptotic signaling pathways were also positively and significantly enriched. The top 10 most enriched immuno-related “biological process” were shown in the Figure 5.
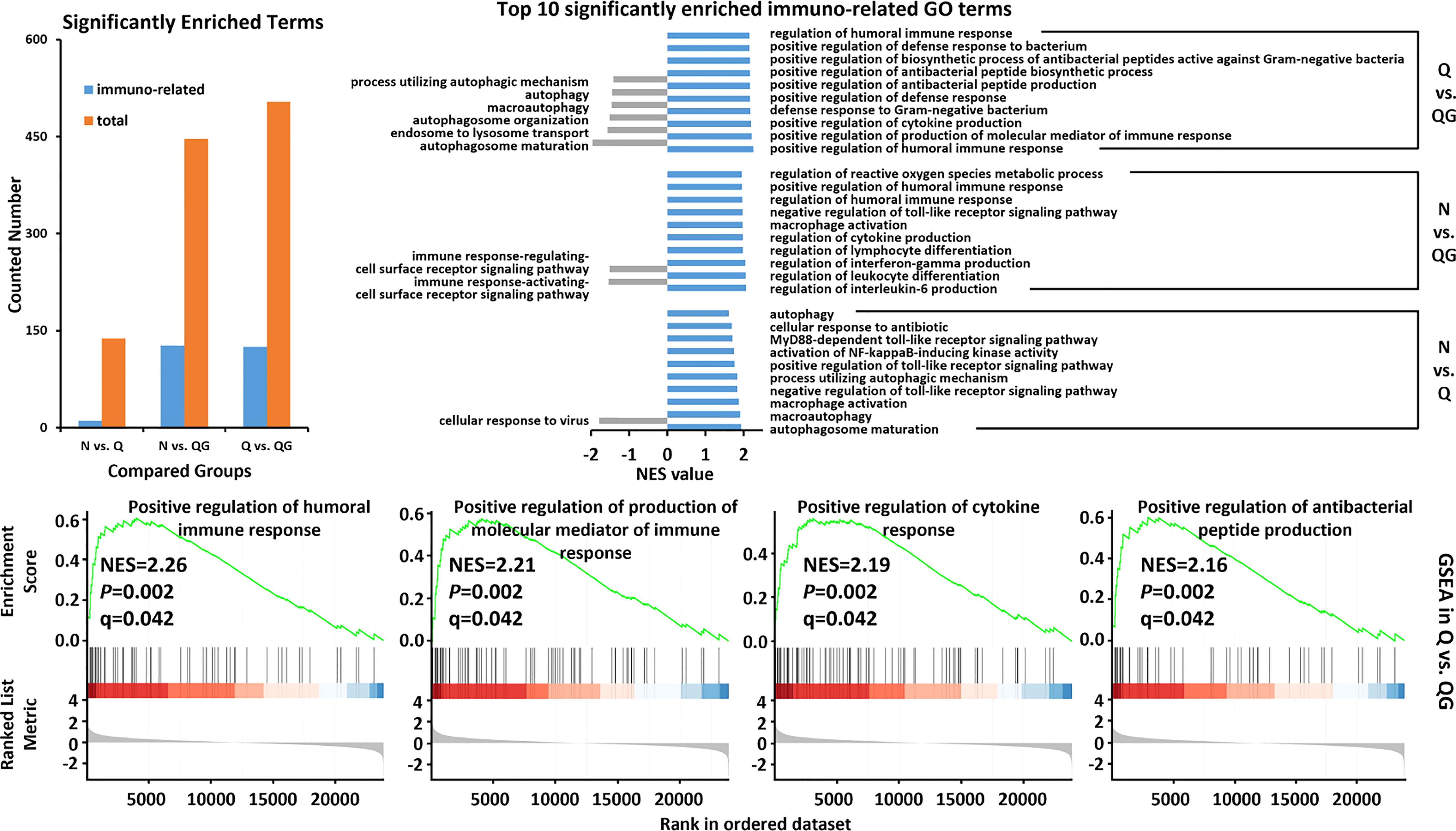
Figure 5 The critical GO pathways associated with immune response to hypoxia and sulfide in haemolymph of Tegillarca granosa were explored by gene set enrichment analysis.
In the KEGG database, the gene set was significantly enriched in FoxO signaling pathway (FoxO, ko04068) in all compared groups (Figure 6). In Q vs. QG groups, the gene set was also enriched in NOD-like receptor signaling pathway (NOD, ko04621) and Apoptosis (ko04210). The FoxO signaling pathway was predicted to be the main immuno-related pathway in the clam in response to hypoxia and sulfide.
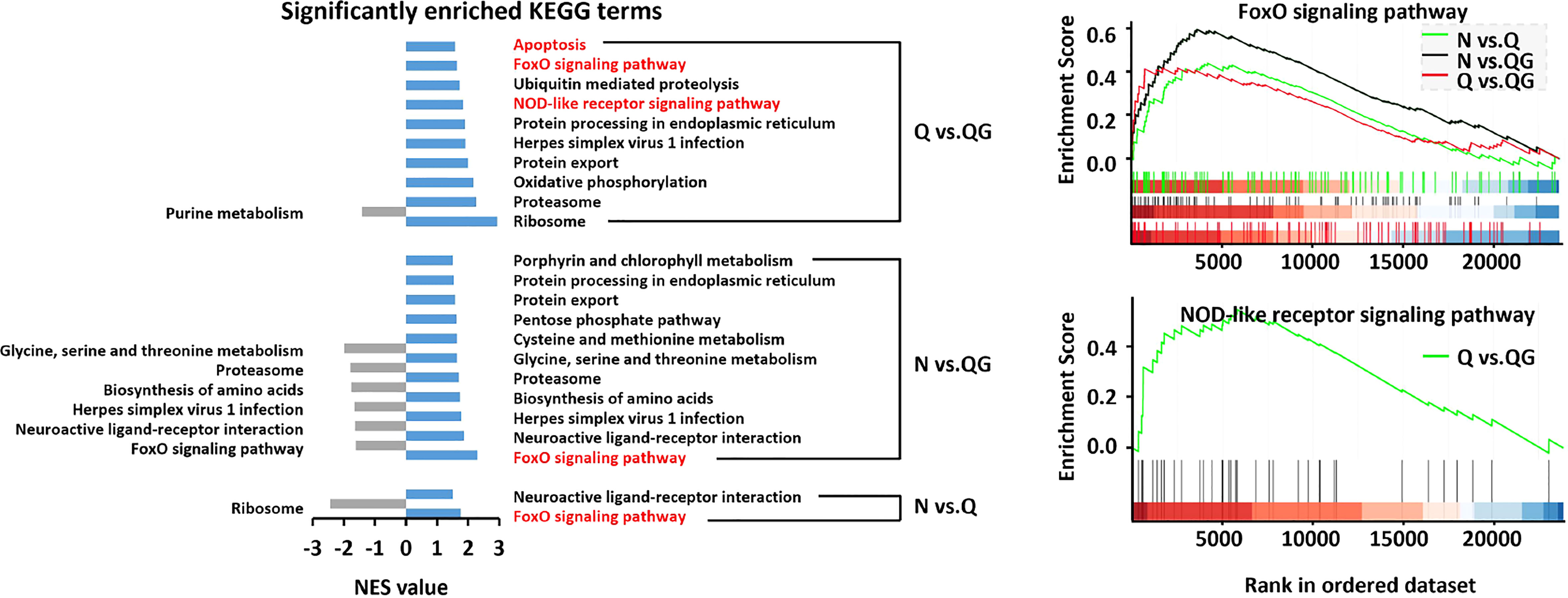
Figure 6 The critical KEGG pathways associated with immune response to hypoxia and sulfide in haemolymph of Tegillarca granosa were explored by gene set enrichment analysis.
4 Discussion
4.1 Cellular and humoral immune responses to hypoxia and sulfide
In marine invertebrates, cellular and humoral immune systems play crucial roles in antimicrobial defense and immuno-regulation. The THC and phagocytosis of haemocytes are the commonly used indicators of cellular immune responses in invertebrates (Loker et al., 2004; Allam and Raftos, 2015). Previous studies on many marine invertebrates, including the bivalves Perna perna, M. coruscus, Perna viridis (Wang et al., 2014; Sui et al., 2016; Nogueira et al., 2017; Shen et al., 2019) and the crustaceans Eriocheir sinensis, Carcinus aestuarii, Nephrops norvegicus, Macrobrachium rosenbergii (Cheng et al., 2002; Hernroth et al., 2015; Qin et al., 2016; Qyli et al., 2020), showed that exposure to prolonged hypoxia could decrease THC and phagocytic activities. The results indicated that the cellular immune responses were intervened by hypoxia exposure. In the present study on T. granosa, THC might be rapidly stimulated by hypoxia but the stimulation did not last more than 24 h. Interestingly, hypoxia with additional sulfide significantly induced both the THC and phagocytic levels around 24 h, which indicated that the cellular immune response of T. granosa was stimulated by certain concentration of sulfide. This finding was different from previous reports in various marine species (Cheng et al., 2007; Sun et al., 2014; Xu et al., 2014).
Humoral factors such as agglutinins (e.g., lectins), lysosomal enzymes (e.g., acid phosphatase, lysozyme), and various antimicrobial peptides (Canesi et al., 2002) are considered as important indicators to investigate the humoral immunity of invertebrates. Prolonged hypoxia causes significant decrease of antioxidant and other humoral immune responses has been demonstrated in many invertebrates including the bivalves Arctica islandica, P. perna, M. coruscus and Hyriopsis cumingii (Philipp et al., 2012; Hu et al., 2015; Sui et al., 2016; Nogueira et al., 2017; Li et al., 2022b), as well as the crabs M. japonicus, Callinectes sapidus, and Scylla paramamosain (Tanner et al., 2006; Cheng et al., 2020; Wang et al., 2022). In this study, hypoxia significantly increased CAT at 24 h. However, POD and ACP in hypoxia group significantly decreased in 12-96 h. Besides, AKP and LZM in hypoxia group also decreased to a certain extent. The results indicated that hypoxia significantly enhanced the CAT activity at 24 h, but the overall humoral immunity might be suppressed within 96 h. After adding sulfide, SOD (24-96 h), CAT (24 h, 96 h), POD (48 h, 96 h), ACP (96 h), AKP (96 h), and LZM (12-96 h) significantly increased compared to the hypoxia group. The results indicated that sulfide could stimulate the humoral immunity to some extent under hypoxic conditions. However, after adding sulfide, only SOD (24-96 h), CAT (24 h, 96 h), and LZM (12 h, 48 h, 96 h) significantly increased compared to the normoxia control group, CAT (48 h), POD (12 h), ACP (12-96 h), and AKP (48 h) were still significantly lower than the normoxia control group. This indicated that sulfide have certain limitations and specificity in stimulating the humoral immunity under hypoxic conditions.
We inferred that moderate exogenous sulfide attenuated the immunosuppression caused by hypoxia through both cellular and humoral immune responses. The attenuation through cellular way happened around 24 h while the humoral way took effects mainly at 48 - 96 h.
4.2 Integration of biomarkers using IBR
To support our infers, we induce IBR method. The IBR index proposed by Beliaeff and Burgeot (2002) (Benoit Beliaeff and Burgeot, 2002) novelly integrated biomarkers of CAT, GST and AchE in Mytilus edulis and showed diverse stress levels along the coasts of Baltic Sea. Recently, the IBR has been addressed as an integrative method for the assessment of environmental stress, as well as for the measurement of the sensitivity of organisms after exposed to toxicants (Broeg and Lehtonen, 2006; Pytharopoulou et al., 2008; Raftopoulou and Dimitriadis, 2010; Ji et al., 2018; Chang et al., 2020). By using IBR method, we found that CAT and LZM were the most sensitive antioxidant and antibacterial indexes in the haemolymph of T. granosa in response to hypoxia and sulfide. The IBR analysis also demonstrated that moderate sulfide had a remarkable effect in attenuating the immunosuppression caused by hypoxia exposure through multiple ways, including stimulating the cellular immune response at around 24 h, and stimulating the antibacterial and antioxidant responses by low level sulfide at around 96 h. Although no similar studies of sulfide have been reported in invertebrates, H2S has been regarded as not only a gasotransmitter, but also a key factor in immunity-related processes in clinical medicine (Yuan et al., 2017; Li et al., 2021).
Even though IBR discriminates the stress types or levels, it provides a simple method for the quantitative evaluation of the combination effects of hypoxia and sulfide in this study, and it can be recommended for future quantified risk assessment of hypoxia and sulfide on aquatic organisms.
4.3 Transcriptomic analysis revealed immunomodulatory effects of sulfide
Studies had confirmed that the clams had extremely tolerance to hypoxia and sulfide with different adaptive mechanisms (Joyner-Matos et al., 2006; Kodama et al., 2018; Wang et al., 2019; Wang and Zhang, 2021; Wang et al., 2021). In the present study, our findings supplemented the immune response mechanisms to hypoxia and sulfide in the clam T. granosa. During the hypoxia, the clam downregulated DEGs involved in NLR signaling pathway (nlrp3) and activated autophagy (mTOR), which might alleviate cell damages and inflammation (Cosin-Roger et al., 2017). Hypoxic challenge also inhibited DEGs involved in calcium-binding signaling pathways (eip63F-1), while hypoxic challenge with additional sulfide significantly induced both NLR and calcium-binding signaling pathways. The result suggested the potential immunostimulation effects of sulfide, as it has been reported that NLR and calcium-binding signaling pathway play important roles in recognizing pathogen-associated molecular patterns (PAMPs) and regulation immune responses (Wen et al., 2013; Horng, 2014; Zhao and Zhao, 2020).
After treated with exogenous sulfide, the clam positively enriched many biological processes that were involved in antimicrobial/antibacterial peptide, interferon, interleukin, leukocyte and lymphocyte, according to the GSEA analysis. Those factors play significant roles in the defense against pathogens infection and elimination of bacterial action. For instance, antimicrobial peptides (AMPs) are important component of the innate immune system and executive antibacterial activity in mollusks. AMP that was isolated from M. coruscus displayed strong antimicrobial activity against gram-positive bacteria (Liao et al., 2013; Oh et al., 2020). Interferon is a cytokine mainly produced by lymphocytes, involving the activation of macrophages and control of cell proliferation and apoptosis (Schoenbom and Wilson, 2007; Pereiro et al., 2019). The activation of those biological processes explained the stimulation of THC, phagocytic activity, and LZM after the 24 h exposure to sulfide.
We also identified many other stimulated immuno-related signaling pathways and genes such as Toll and Imd (tollo), FoxO (foxo, gadd45a, klhl20, egfr), TLR (tlr13), NF-κB (vps9d1), TNF (c1ql3), MAPK (dusp1/10, jun), apoptosis (aifm3, birc2/3). Toll and Imd signaling pathway have been paid great attention in immunomodulatory (Tanji and Ip, 2005; Rances et al., 2013). These pathways are critical to the expression of antimicrobial peptides, which fight against fungi, Gram-negative bacterial and Gram-positive bacterial (Tanji and Ip, 2005; Tanji et al., 2007). In the mud crab S. paramamosain and the Pacific white shrimp L. vannamei, Toll and Imd signaling pathways are activated in innate immune responses to white spot syndrome virus (WSSV) and sulfide exposure (Li and Xiang, 2013; Duan et al., 2017; Chen and Wang, 2019; Li et al., 2022a). FoxO signaling pathway is involved in mitochondria-dependent processes of apoptosis by triggering the expression of TNF apoptosis ligand and Bcl-XL from Bcl-2 family members (Farhan et al., 2017). In aquatic animals, such as grass carp (Ctenopharyngodon idellus), Asian clam (Corbicula fluminea), and sea cucumber (Apostichopus japonicus), genetic and transcriptomic analysis revealed that activation of FoxO could regulate antioxidant and apoptosis pathways (Wang et al., 2015; Jin et al., 2017; Zhang et al., 2019). Toll-like receptors (TLRs) signaling pathway also plays an important role in innate immunity in invertebrates, and the activation of TLR can activate multiple inflammatory pathways (Brown et al., 2011). In the present study, a variety of genes related to TLRs and NF-κB, TNF and MAPK signaling pathway was significantly upregulated by exposing to sulfide, which indicated that TLRs signaling pathway activated the downstream MAPK, NF-κB, TNF signaling pathways (Figure 7). The present results were consistent with the transcriptomic profile of the clam A. broughtonii that was exposed to hypoxia and sulfide for 24 h (Wang et al., 2019), in which MAPK and TNF signaling pathways were significantly enriched, and some genes related to TLRs and NF-κB signaling pathways were also upregulated. In the clam, the active apoptosis signaling pathway indicated that sulfide might regulate cell apoptosis through TLR, MAPK, NF-κB, and TNF, as the connection of apoptosis and TLR, MAPK, NF-κB, TNF has been described in many invertebrates (Cao et al., 2018; Wang et al., 2020). Wang et al. (2021) also suggested the possible ways of MAPK that regulated sulfide-induced apoptosis in the clam A. broughtonii.
5 Conclusion
In conclusion, our study found that hypoxia alone suppressed the overall immune response, while additional sulfide attenuated the immunosuppression through multiple cellular and humoral ways. Besides, Toll, NOD, FoxO, MAPK, TNF, and Apoptosis signaling pathways might play critical roles in the sulfide-mediated immune responses. Our study would provide useful tools for assessing the integrated biological and transcriptomic impacts of hypoxia and sulfide on T. granosa and offer deep insight into the sulfide-tolerant mechanisms in shellfish.
Data availability statement
The datasets presented in this study can be found in online repositories. The name of the repository and accession number can be found below: NCBI; PRJNA931778. https://www.ncbi.nlm.nih.gov/sra/PRJNA931778.
Author contributions
ZY: conceptualization, experimental design, carry out experiments, writing-original draft. YW: Verfication of the results and revision of the manuscript. PJ: visualization, data curation. FX: investigation, methodology, software. YX: software, data curation. XT: data curation, validation. XZ: funding acquisition, writing-review and editing. All authors contributed to the article and approved the submitted version.
Funding
The research was financial supported by the Key Research and Development Program of Zhejiang Province (2021C02047), the Basic Public Welfare Research Project of Zhejiang Province (LQ22C190004), and Innovation Plan of Science and Technology for College Students of Zhejiang Province (2021R411011).
Conflict of interest
The authors declare that the research was conducted in the absence of any commercial or financial relationships that could be construed as a potential conflict of interest.
Publisher’s note
All claims expressed in this article are solely those of the authors and do not necessarily represent those of their affiliated organizations, or those of the publisher, the editors and the reviewers. Any product that may be evaluated in this article, or claim that may be made by its manufacturer, is not guaranteed or endorsed by the publisher.
Supplementary material
The Supplementary Material for this article can be found online at: https://www.frontiersin.org/articles/10.3389/fmars.2023.1139973/full#supplementary-material
References
Abdel-Tawwab M., Monier M. N., Hoseinifar S. H., Faggio C. (2019). Fish response to hypoxia stress: growth, physiological, and immunological biomarkers. Fish Physiol. Biochem. 45, 997–1013. doi: 10.1007/s10695-019-00614-9
Aelion C. M., Warttinger U. (2009). Low sulfide concentrations affect nitrate transformations in freshwater and saline coastal retention pond sediments. Soil Biol. Biochem. 41, 735–741. doi: 10.1016/j.soilbio.2009.01.015
Affonso E. G., Rantin F. T. (2005). Respiratory responses of the air-breathing fish Hoplosternum littorale to hypoxia and hydrogen sulfide. Comp. Biochem. Physiol. Part C: Toxicol. Pharmacol. 141, 275–280. doi: 10.1016/j.cca.2005.07.003
Allam B., Raftos D. (2015). Immune responses to infectious diseases in bivalves. J. Invertebrate Pathol. 131, 121–136. doi: 10.1016/j.jip.2015.05.005
Asaoka S., Umehara A., Otani S., Fujii N., Okuda T., Nakai S., et al. (2018). Spatial distribution of hydrogen sulfide and sulfur species in coastal marine sediments Hiroshima bay, Japan. Mar. pollut. Bull. 133, 891–899. doi: 10.1016/j.marpolbul.2018.06.042
Bagarinao T., Lantin-Olaguer I. (1998). The sulfide tolerance of milkfish and tilapia in relation to fish kills in farms and natural waters in the Philippines. Hydrobiologia 382, 137–150. doi: 10.1023/A:1003420312764
Bao J., Li X., Xing Y., Feng C., Jiang H. (2020). Effects of hypoxia on immune responses and carbohydrate metabolism in the Chinese mitten crab, eriocheir sinensis. Aquaculture Res. 51, 2735–2744. doi: 10.1111/are.14612
Bao Y., Zeng Q., Wang J., Zhang Z., Zhang Y., Wang S., et al. (2021). Genomic insights into the origin and evolution of molluscan red-bloodedness in the blood clam tegillarca granosa. Mol. Biol. Evol. 38, 2351–2365. doi: 10.1093/molbev/msab030
Beliaeff B., Burgeot T. (2002). Integrated biomarker response: a useful tool for ecological risk assessment. Environ. Toxicol. Chem. 21, 1316–1322. doi: 10.1002/etc.5620210629
Bocaniov S. A., Scavia D. (2016). Temporal and spatial dynamics of large lake hypoxia: integrating statistical and three-dimensional dynamic models to enhance lake management criteria. Water Resour. Res. 52, 4247–4263. doi: 10.1002/2015WR018170
Broeg K., Lehtonen K. K. (2006). Indices for the assessment of environmental pollution of the Baltic Sea coasts: integrated assessment of a multi-biomarker approach. Mar. pollut. Bull. 53, 508–522. doi: 10.1016/j.marpolbul.2006.02.004
Brown J., Wang H., Hajishengallis G. N., Martin M. (2011). TLR-signaling networks: an integration of adaptor molecules, kinases, and cross-talk. J. Dental Res. 90, 417–427. doi: 10.1177/0022034510381264
Canesi L., Gallo G., Gavioli M., Pruzzo C. (2002). Bacteria-hemocyte interactions and phagocytosis in marine bivalves. Microscopy Res. Technique 57, 469–476. doi: 10.1002/jemt.10100
Cao R., Liu Y., Wang Q., Zhang Q., Yang D., Liu H., et al. (2018). The impact of ocean acidification and cadmium on the immune responses of pacific oyster, crassostrea gigas. Fish Shellfish Immunol. 81, 456–462. doi: 10.1016/j.fsi.2018.07.055
Chang B. D., Page F. H., Losier R. J. (2013). Variables affecting sediment sulfide concentrations in regulatory monitoring at salmon farms in the bay of fundy, Canada. Aquaculture Environ. Interact. 4, 67–79. doi: 10.3354/aei00074
Chang T., Wei B., Wang Q., He Y., Wang C. (2020). Toxicity assessment of municipal sewage treatment plant effluent by an integrated biomarker response in the liver of crucian carp (Carassius auratus). Environ. Sci. pollut. Res. 27, 7280–7288. doi: 10.1007/s11356-019-07463-2
Chen F., Wang K. (2019). Characterization of the innate immunity in the mud crab Scylla paramamosain. Fish Shellfish Immunol. 93, 436–448. doi: 10.1016/j.fsi.2019.07.076
Cheng S. Y., Hsu S. W., Chen J. C. (2007). Effect of sulfide on the immune response and susceptibility to Vibrio alginolyticus in the kuruma shrimp marsupenaeus japonicus. Fish Shellfish Immunol. 22, 16–26. doi: 10.1016/j.fsi.2006.03.008
Cheng W., Liu C. H., Hsu J. P., Chen J. C. (2002). Effect of hypoxia on the immune response of giant freshwater prawn Macrobrachium rosenbergii and its susceptibility to pathogen enterococcus. Fish Shellfish Immunol. 13, 351–365. doi: 10.1006/fsim.2001.0411
Cheng C. H., Ma H. L., Deng Y. Q., Feng J., Jie Y. K., Guo Z. X. (2020). Immune and physiological responses of mud crab (Scylla paramamosain) under air exposure. Comp. Biochem. Physiol. Part C: Toxicol. Pharmacol. 233:108767. doi: 10.1016/j.cbpc.2020.108767
Christensen P. B., Glud R. N., Dalsgaard T., Gillespie P. (2003). Impacts of longline mussel farming on oxygen and nitrogen dynamics and biological communities of coastal sediments. Aquaculture 218, 567–588. doi: 10.1016/S0044-8486(02)00587-2
Cosin-Roger J., Simmen S., Melhem H., Atrott K., Frey-Wagner I., Hausmann M., et al. (2017). Hypoxia ameliorates intestinal inflammation through NLRP3/mTOR downregulation and autophagy activation. Nat. Commun. 8, 98. doi: 10.1038/s41467-017-00213-3
Cui W., Cao L., Liu J., Ren Z., Zhao B., Dou S. (2020). Effects of seawater acidification and cadmium on the antioxidant defense of flounder Paralichthys olivaceus larvae. Sci. Total Environ. 718, 137234. doi: 10.1016/j.scitotenv.2020.137234
Cutter G. A., Krahforst C. F. (1988). Sulfide in surface waters of the western Atlantic ocean. Geophysical Res. Lett. 15, 1393–1396. doi: 10.1029/GL015i012p01393
Diaz R. J. (2001). Overview of hypoxia around the world. J. Environ. Qual. 30, 275–281. doi: 10.2134/jeq2001.302275x
Duan Y., Dong H., Wang Y., Li H., Liu Q., Zhang Y., et al. (2017). Intestine oxidative stress and immune response to sulfide stress in pacific white shrimp litopenaeus vannamei. Fish Shellfish Immunol. 63, 201–207. doi: 10.1016/j.fsi.2017.02.013
Duan Y., Wang Y., Dong H., Li H., Liu Q., Zhang J., et al. (2018). Physiological and immune response in the gills of Litopenaeus vannamei exposed to acute sulfide stress. Fish Shellfish Immunol. 81, 161–167. doi: 10.1016/j.fsi.2018.07.018
Farhan M., Wang H., Gaur U., Little P. J., Xu J., Zheng W. (2017). FOXO signaling pathways as therapeutic targets in cancer. Int. J. Biol. Sci. 13, 815–827. doi: 10.7150/ijbs.20052
Gobler C. J., Baumann H. (2016). Hypoxia and acidification in ocean ecosystems: coupled dynamics and effects on marine life. Biol. Lett. 12, 20150976. doi: 10.1098/rsbl.2015.0976
Grabherr M. G., Haas B. J., Yassour M., Levin J. Z., Thompson D. A., Amit I., et al. (2011). Full-length transcriptome assembly from RNA-seq data without a reference genome. Nat. Biotechnol. 29, 644–652. doi: 10.1038/nbt.1883
Grieshaber M. K., V¨olkel S. (1998). Animal adaptations for tolerance and exploitation of poisonous sulfide. Annu. Rev. Of Physiol. 60, 33–53. doi: 10.1146/annurev.physiol.60.1.33
Guan Y. Q., Pei S. R., Li Z. J. (2011). Effects of acute sulfide stress on immune responses and antioxidant system of macrobrachium nipponense. J. Hydroecology 32, 89–94. doi: 10.15928/j.1674-3075.2011.06.022
Han Y., Tang Y., Sun S., Kim T., Ju K., Ri S., et al. (2021a). Modulatory function of calmodulin on phagocytosis and potential regulation mechanisms in the blood clam tegillarca granosa. Dev. Comp. Immunol. 116, 103910. doi: 10.1016/j.dci.2020.103910
Han Y., Zhou W., Tang Y., Shi W., Shao Y., Ren P., et al. (2021b). Microplastics aggravate the bioaccumulation of three veterinary antibiotics in the thick shell mussel mytilus coruscus and induce synergistic immunotoxic effects. Sci. Total Environ. 770, 145273. doi: 10.1016/j.scitotenv.2021.145273
Hargrave B. T., Holmer M., Newcombe C. P. (2008). Towards a classification of organic enrichment in marine sediments based on biogeochemical indicators. Mar. pollut. Bull. 56, 810–824. doi: 10.1016/j.marpolbul.2008.02.006
Hernroth B., Krang A. S., Baden S. (2015). Bacteriostatic suppression in Norway lobster (Nephrops norvegicus) exposed to manganese or hypoxia under pressure of ocean acidification. Aquat. Toxicol. 159, 217–224. doi: 10.1016/j.aquatox.2014.11.025
Hildebrandt T. M., Grieshaber M. K. (2008a). Redox regulation of mitochondrial sulfide oxidation in the lugworm, arenicola marina. J. Exp. Biol. 211, 2617–2623. doi: 10.1242/jeb.019729
Hildebrandt T. M., Grieshaber M. K. (2008b). Three enzymatic activities catalyze the oxidation of sulfide to thiosulfate in mammalian and invertebrate mitochondria. FEBS J. 275, 3352–3361. doi: 10.1111/j.1742-4658.2008.06482.x
Horng T. (2014). Calcium signaling and mitochondrial destabilization in the triggering of the NLRP3 inflammasome. Trends In Immunol. 35, 253–261. doi: 10.1016/j.it.2014.02.007
Hu X., Chi Q., Liu Z., Tao D., Wang Y., Cong Y., et al. (2021). Transcriptome analysis reveals that hydrogen sulfide exposure suppresses cell proliferation and induces apoptosis through ciR-PTPN23/miR-15a/E2F3 signaling in broiler thymus. Environ. pollut. 284, 117466. doi: 10.1016/j.envpol.2021.117466
Hu X., Chi Q., Wang D., Chi X., Teng X., Li S. (2018). Hydrogen sulfide inhalation-induced immune damage is involved in oxidative stress, inflammation, apoptosis and the Th1/Th2 imbalance in broiler bursa of fabricius. Ecotoxicology Environ. Saf. 164, 201–209. doi: 10.1016/j.ecoenv.2018.08.029
Hu M., Wu F., Yuan M., Li Q., Gu Y., Wang Y., et al. (2015). Antioxidant responses of triangle sail mussel Hyriopsis cumingii exposed to harmful algae microcystis aeruginosa and hypoxia. Chemosphere 139, 541–549. doi: 10.1016/j.chemosphere.2015.07.074
Huang B., Tang X., Zhang L., Li L., Wang W., Liu M., et al. (2019). IKKepsilon-like plays an important role in the innate immune signaling of the pacific oyster (Crassostrea gigas). Fish Shellfish Immunol. 93, 551–558. doi: 10.1016/j.fsi.2019.07.074
Huang J., Zhang L., Li J., Shi X., Zhang Z. (2013). Proposed function of alternative oxidase in mitochondrial sulphide oxidation detoxification in the echiuran worm, urechis unicinctus. J. Mar. Biol. Assoc. United Kingdom 93, 2145–2154. doi: 10.1017/S0025315413000696
Jørgensen B. B. (1990). A thiosulfate shunt in the sulfir cycle of marine sediments. Science 249, 152–154. doi: 10.1126/science.249.4965.152
Ji Y., Wu P., Zhang J., Zhang J., Zhou Y., Peng Y., et al. (2018). Heavy metal accumulation, risk assessment and integrated biomarker responses of local vegetables: a case study along the le'an river. Chemosphere 199, 361–371. doi: 10.1016/j.chemosphere.2018.02.045
Jiang L., Feng J., Ying R., Yin F., Pei S., Lu J., et al. (2019). Individual and combined effects of ammonia-n and sulfide on the immune function and intestinal microbiota of pacific white shrimp litopenaeus vannamei. Fish Shellfish Immunol. 92, 230–240. doi: 10.1016/j.fsi.2019.06.020
Jiang J., Zhou Z., Dong Y., Gao S., Sun H., Chen Z., et al. (2017). Comparative analysis of immunocompetence between females and males in the sea cucumber apostichopus japonicus. Fish Shellfish Immunol. 63, 438–443. doi: 10.1016/j.fsi.2017.02.038
Jin J., Wang Y., Wu Z., Hergazy A., Lan J., Zhao L., et al. (2017). Transcriptomic analysis of liver from grass carp (Ctenopharyngodon idellus) exposed to high environmental ammonia reveals the activation of antioxidant and apoptosis pathways. Fish Shellfish Immunol. 63, 444–451. doi: 10.1016/j.fsi.2017.02.037
Joyner-Matos J., Downs C. A., Julian D. (2006). Increased expression of stress proteins in the surf clam Donax variabilis following hydrogen sulfide exposure. Comp. Biochem. Physiol. Part A Mol. Integr. Physiol. 145, 245–257. doi: 10.1016/j.cbpa.2006.06.033
Kanaya G., Uehara T., Kikuchi E. (2016). Effects of sedimentary sulfide on community structure, population dynamics, and colonization depth of macrozoobenthos in organic-rich estuarine sediments. Mar. pollut. Bull. 109, 393–401. doi: 10.1016/j.marpolbul.2016.05.043
Kimura Y., Goto Y.-I., Kimura H. (2010). Hydrogen sulfide increases glutathione production and suppresses oxidative stress in mitochondria. Antioxidants Redox Signaling 12, 1–13. doi: 10.1089/ars.2008.2282
Kimura Y., Kimura H. (2004). Hydrogen sulfide protects neurons from oxidative stress. FASEB J. 18, 1165–1167. doi: 10.1096/fj.04-1815fje
Kimura Y., Shibuya N., Kimura H. (2019). Sulfite protects neurons from oxidative stress. Br. J. Pharmacol. 176, 571–582. doi: 10.1111/bph.14373
Kodama K., Waku M., Sone R., Miyawaki D., Ishida T., Akatsuka T., et al. (2018). Ontogenetic and temperature-dependent changes in tolerance to hypoxia and hydrogen sulfide during the early life stages of the Manila clam ruditapes philippinarum. Mar. Environ. Res. 137, 177–187. doi: 10.1016/j.marenvres.2017.12.019
Kvamme B. O., Gadan K., Finne-Fridell F., Niklasson L., Sundh H., Sundell K., et al. (2013). Modulation of innate immune responses in Atlantic Salmon by chronic hypoxia-induced stress. Fish Shellfish Immunol. 34, 55–65. doi: 10.1016/j.fsi.2012.10.006
Lan A., Liao X., Mo L., Yang C., Yang Z., Wang X., et al. (2011). Hydrogen sulfide protects against chemical hypoxia-induced injury by inhibiting ROS-activated ERK1/2 and p38MAPK signaling pathways in PC12 cells. PloS One 6, e25921. doi: 10.1371/journal.pone.0025921
Li B., Dewey C. N. (2011). RSEM: accurate transcript quantification from RNA-seq data with or without a reference genome. BMC Bioinf. 12, 323. doi: 10.1186/1471-2105-12-323
Li M., Mao J., Zhu Y. (2021). New therapeutic approaches using hydrogen sulfide donors in inflammation and immune response. Antioxidants Redox Signaling 35, 341–356. doi: 10.1089/ars.2020.8249
Li J., Sun X., Zheng F., Sun H. (2009). Histochemical localization and characterization of AKP, ACP, NSE, and POD from cultured apostichopus japonicus. Chin. J. Oceanology Limnology 27, 550–554. doi: 10.1007/s00343-009-9125-z
Li F., Xiang J. (2013). Signaling pathways regulating innate immune responses in shrimp. Fish Shellfish Immunol. 34, 973–980. doi: 10.1016/j.fsi.2012.08.023
Li B. W., Xu W. B., Dong W. R., Zhang Y. M., Cheng Y. X., Chen D. Y., et al. (2022a). Identification and function analysis of two fibroblast growth factor receptor (FGFR) from Scylla Paramamosain: the evidence of FGFR involved in innate immunity in crustacean. Fish Shellfish Immunol. 131, 602–611. doi: 10.1016/j.fsi.2022.08.075
Li Q., Zhang F., Sun S. (2022b). The survival and responses of blue mussel mytilus edulis to 16-day sustained hypoxia stress. Mar. Environ. Res. 176, 105601. doi: 10.1016/j.marenvres.2022.105601
Liao Z., Wang X. C., Liu H. H., Fan M. H., Sun J. J., Shen W. (2013). Molecular characterization of a novel antimicrobial peptide from mytilus coruscus. Fish Shellfish Immunol. 34, 610–616. doi: 10.1016/j.fsi.2012.11.030
Liu H., Zha S., Yang Z., Zhang W., Lin Z., Wang S., et al. (2022). Acute sulfide exposure induces hemocyte toxicity and microbiota dysbiosis in blood clam tegillarca granosa. Aquat. Toxicol. 249, 106224. doi: 10.1016/j.aquatox.2022.106224
Loker E. S., Adema C. M., Zhang S. M., Kepler T. B. (2004). Invertebrate immune systems–not homogeneous, not simple, not well understood. Immunol. Rev. 198, 10–24. doi: 10.1111/j.0105-2896.2004.0117.x
Losyuk G. N., Kokryatskaya N. M., Krasnova E. D. (2021). Hydrogen sulfide contamination of coastal lakes at different stages of isolation from the white Sea. Oceanology 61, 351–361. doi: 10.1134/S0001437021020120
Meng T., Zhu M. X., Ma W. W., Gan Z. X. (2019). Sulfur, iron, and phosphorus geochemistry in an intertidal mudflat impacted by shellfish aquaculture. Environ. Sci. pollut. Res. Int. 26, 6460–6471. doi: 10.1007/s11356-018-04114-w
Mishra K. P., Ganju L., Singh S. B. (2015). Hypoxia modulates innate immune factors: a review. Int. Immunopharmacol. 28, 425–428. doi: 10.1016/j.intimp.2015.07.008
Nie H., Wang H., Jiang K., Yan X. (2020). Transcriptome analysis reveals differential immune related genes expression in Ruditapes philippinarum under hypoxia stress: potential HIF and NF-κB crosstalk in immune responses in clam. BMC Genomics 21, 21:318. doi: 10.1186/s12864-020-6734-6
Nogueira L., Mello D. F., Trevisan R., Garcia D., da Silva Acosta D., Dafre A. L., et al. (2017). Hypoxia effects on oxidative stress and immunocompetence biomarkers in the mussel Perna perna (Mytilidae, bivalvia). Mar. Environ. Res. 126, 109–115. doi: 10.1016/j.marenvres.2017.02.009
Oh R., Lee M. J., Kim Y. O., Nam B. H., Kong H. J., Kim J. W., et al. (2020). Myticusin-beta, antimicrobial peptide from the marine bivalve, mytilus coruscus. Fish Shellfish Immunol. 99, 342–352. doi: 10.1016/j.fsi.2020.02.020
Pereiro P., Figueras A., Novoa B. (2019). Insights into teleost interferon-gamma biology: an update. Fish Shellfish Immunol. 90, 150–164. doi: 10.1016/j.fsi.2019.04.002
Pfaffl M. W. (2001). A new mathematical model for relative quantification in real-time RT-PCR. Nucleic Acids Res. 29, e45. doi: 10.1093/nar/29.9.e45
Philipp E. E., Wessels W., Gruber H., Strahl J., Wagner A. E., Ernst I. M., et al. (2012). Gene expression and physiological changes of different populations of the long-lived bivalve Arctica islandica under low oxygen conditions. PloS One 7, e44621. doi: 10.1371/journal.pone.0044621
Pozzi G., Gobbi G., Masselli E., Carubbi C., Presta V., Ambrosini L., et al. (2022). Buffering adaptive immunity by hydrogen sulfide. Cells 11, 1–16. doi: 10.3390/cells11030325
Pytharopoulou S., Sazakli E., Grintzalis K., Georgiou C. D., Leotsinidis M., Kalpaxis D. L. (2008). Translational responses of mytilus galloprovincialis to environmental pollution: integrating the responses to oxidative stress and other biomarker responses into a general stress index. Aquat Toxicol. 89, 18–27. doi: 10.1016/j.aquatox.2008.05.013
Qin F., Shi M., Yuan H., Yuan L., Lu W., Zhang J., et al. (2016). Dietary nano-selenium relieves hypoxia stress and, improves immunity and disease resistance in the Chinese mitten crab (Eriocheir sinensis). Fish Shellfish Immunol. 54, 481–488. doi: 10.1016/j.fsi.2016.04.131
Qyli M., Aliko V., Faggio C. (2020). Physiological and biochemical responses of Mediterranean green crab, Carcinus aestuarii, to different environmental stressors: evaluation of hemocyte toxicity and its possible effects on immune response. Comp. Biochem. Physiology Part C 231, 108739. doi: 10.1016/j.cbpc.2020.108739
Raftopoulou E. K., Dimitriadis V. K. (2010). Assessment of the health status of mussels Mytilus galloprovincialis along thermaikos gulf (Northern greece): an integrative biomarker approach using ecosystem health indices. Ecotoxicology Environ. Saf. 73, 1580–1587. doi: 10.1016/j.ecoenv.2010.06.027
Rances E., Johnson T. K., Popovici J., Iturbe-Ormaetxe I., Zakir T., Warr C. G., et al. (2013). The toll and imd pathways are not required for wolbachia-mediated dengue virus interference. J. Virol. 87, 11945–11949. doi: 10.1128/JVI.01522-13
Reese B. K., Finneran D. W., Mills H. J., Zhu M. X., Morse J. W. (2011). Examination and refinement of the determination of aqueous hydrogen sulfide by the methylene blue method. Aquat. geochemistry 17, 567–582. doi: 10.1007/s10498-011-9128-1
Ren Z., Zhang L., Liu X., Liu J., Zhang Z. (2015). Response of alternative oxidase in the mid-gut and hindgut of urechis unicinctus after sulfide stress. Periodical Ocean Univ. China 45, 66–71. doi: 10.16441/j.cnki.hdxb.20130400
Saleh A., Abtahi B., Mirzaei N., Chen C.-T. A., Ershadifar H., Ghaemi M., et al. (2021). Hypoxia in the persian gulf and the strait of hormuz. Mar. pollut. Bull. 167, 112354. doi: 10.1016/j.marpolbul.2021.112354
Schoenbom J. R., Wilson C. B. (2007). Regulation of interferong during innate and adaptive immune responses. Adv. Immunol. 96, 41–101. doi: 10.1016/S0065-2776(07)96002-2
Shen Y., Huang Z., Liu G., Ke C., You W. (2019). Hemolymph and transcriptome analysis to understand innate immune responses to hypoxia in pacific abalone. Comp. Biochem. Physiol. Part D Genomics Proteomics 30, 102–112. doi: 10.1016/j.cbd.2019.02.001
Shi W., Han Y., Guo C., Zhao X., Liu S., Su W., et al. (2017). Immunotoxicity of nanoparticle nTiO2 to a commercial marine bivalve species, tegillarca granosa. Fish Shellfish Immunol. 66, 300–306. doi: 10.1016/j.fsi.2017.05.036
Srithongouthai S., Tada K. (2017). Impacts of organic waste from a yellowtail cage farm on surface sediment and bottom water in shido bay (the seto inland Sea, Japan). Aquaculture 471, 140–145. doi: 10.1016/j.aquaculture.2017.01.021
Su W., Rong J., Zha S., Yan M., Fang J., Liu G. (2018). Ocean acidification affects the cytoskeleton, lysozymes, and nitric oxide of hemocytes: a possible explanation for the hampered phagocytosis in blood clams, tegillarca granosa. Front. Physiol. 9, 619. doi: 10.3389/fphys.2018.00619
Subramanian A., Tamayo P., Mootha V. K., Mukherjee S., Ebert B. L., Gillette M. A., et al. (2005). Gene set enrichment analysis: a knowledge-based approach for interpreting genome-wide expression profiles. Proc. Natl. Acad. Sci. United States America 102, 15545–15550. doi: 10.1073/pnas.0506580102
Sui Y., Kong H., Shang Y., Huang X., Wu F., Hu M., et al. (2016). Effects of short-term hypoxia and seawater acidification on hemocyte responses of the mussel mytilus coruscus. Mar. pollut. Bull. 108, 46–52. doi: 10.1016/j.marpolbul.2016.05.001
Sun W. H., Liu F., Chen Y., Zhu Y. C. (2012). Hydrogen sulfide decreases the levels of ROS by inhibiting mitochondrial complex IV and increasing SOD activities in cardiomyocytes under ischemia/reperfusion. Biochem. Biophys. Res. Commun. 421, 164–169. doi: 10.1016/j.bbrc.2012.03.121
Sun Z., Wang L., Zhang T., Zhou Z., Jiang Q., Yi Q., et al. (2014). The immunomodulation of inducible hydrogen sulfide in pacific oyster crassostrea gigas. Dev. Comp. Immunol. 46, 530–536. doi: 10.1016/j.dci.2014.03.011
Tang Y., Zhou W., Sun S., Du X., Han Y., Shi W., et al. (2020). Immunotoxicity and neurotoxicity of bisphenol a and microplastics alone or in combination to a bivalve species, tegillarca granosa. Environ. pollut. 265, 115115. doi: 10.1016/j.envpol.2020.115115
Tanji T., Hu X., Weber A. N., Ip Y. T. (2007). Toll and IMD pathways synergistically activate an innate immune response in drosophila melanogaster. Mol. Cell. Biol. 27, 4578–4588. doi: 10.1128/MCB.01814-06
Tanji T., Ip Y. T. (2005). Regulators of the toll and imd pathways in the drosophila innate immune response. Trends Immunol. 26, 193–198. doi: 10.1016/j.it.2005.02.006
Tanner C. A., Burnett L. E., Burnett K. G. (2006). The effects of hypoxia and pH on phenoloxidase activity in the Atlantic blue crab, callinectes sapidus. Comp. Biochem. Physiol. Part A Mol. Integr. Physiol. 144, 218–223. doi: 10.1016/j.cbpa.2006.02.042
Vallabhapurapu S., Karin M. (2009). Regulation and function of NF-kappaB transcription factors in the immune system. Annu. Rev. Immunol. 27, 693–733. doi: 10.1146/annurev.immunol.021908.132641
Wang Y., Hu M., Li Q., Li J., Lin D., Lu W. (2014). Immune toxicity of TiO(2) under hypoxia in the green-lipped mussel perna viridis based on flow cytometric analysis of hemocyte parameters. Sci. Total Environ. 470-471, 791–799. doi: 10.1016/j.scitotenv.2013.09.060
Wang P., Liu H., Zhao S., Yu S., Xie S., Hua S., et al. (2022). Hypoxia stress affects the physiological responses, apoptosis and innate immunity of kuruma shrimp, marsupenaeus japonicus. Fish Shellfish Immunol. 122, 206–214. doi: 10.1016/j.fsi.2022.02.016
Wang W., Wang L., Liu Z., Song X., Yi Q., Yang C., et al. (2020). The involvement of TLR signaling and anti-bacterial effectors in enhanced immune protection of oysters after Vibrio splendidus pre-exposure. Dev. Comp. Immunol. 103, 103498. doi: 10.1016/j.dci.2019.103498
Wang Y., Zhang X. (2021). The critical roles of mitochondrial alternative chains in juvenile ark shells (Anadara broughtonii) exposed to acute hypoxia with or without sulfide. Aquat. Toxicol. 241, 105996. doi: 10.1016/j.aquatox.2021.105996
Wang H., Zhang W., Li C., Lv Z., Jin C. (2015). Identification and characterization of a novel foxo transcription factors in apostichopus japonicus. Fish Shellfish Immunol. 44, 164–171. doi: 10.1016/j.fsi.2015.02.006
Wang Y., Zheng Y., Dong J., Zhang X. (2021). Two-sided effects of prolonged hypoxia and sulfide exposure on juvenile ark shells (Anadara broughtonii). Mar. Environ. Res. 169, 105326. doi: 10.1016/j.marenvres.2021.105326
Wang Y., Zhou S., Liu T., Chen M., Li W., Zhang X. (2019). The transcriptomic responses of the ark shell, Anadara broughtonii, to sulfide and hypoxia exposure. Mol. Biol. Rep. 46, 4245–4257. doi: 10.1007/s11033-019-04879-4
Wen H., Miao E. A., Ting J. P. (2013). Mechanisms of NOD-like receptor-associated inflammasome activation. Immunity 39, 432–441. doi: 10.1016/j.immuni.2013.08.037
Whitney M. M., Vlahos P. (2021). Reducing hypoxia in an urban estuary despite climate warming. Environ. Sci. Technol. 55, 941–951. doi: 10.1021/acs.est.0c03964
Xia Z., Wu S. (2018). Effects of glutathione on the survival, growth performance and non-specific immunity of white shrimps (Litopenaeus vannamei). Fish Shellfish Immunol. 73, 141–144. doi: 10.1016/j.fsi.2017.12.015
Xiang N., Zhao C., Diao X., Han Q., Zhou H. (2017). Dynamic responses of antioxidant enzymes in pearl oyster Pinctada martensii exposed to di(2-ethylhexyl) phthalate (DEHP). Environ. Toxicol. Pharmacol. 54, 184–190. doi: 10.1016/j.etap.2017.07.009
Xu X. H., Zhang Y. Q., Yan B. L., Xu J. T., Tang Y., Du D. D. (2014). Immunological and histological responses to sulfide in the crab charybdis japonica. Aquat. Toxicol. 150, 144–150. doi: 10.1016/j.aquatox.2014.03.006
Yang C., Yang Z., Zhang M., Dong Q., Wang X., Lan A., et al. (2011). Hydrogen sulfide protects against chemical hypoxia-induced cytotoxicity and inflammation in HaCaT cells through inhibition of ROS/NF-kappaB/COX-2 pathway. PloS One 6, e21971. doi: 10.1371/journal.pone.0021971
Yuan S., Shen X., Kevil C. G. (2017). Beyond a gasotransmitter: hydrogen sulfide and polysulfide in cardiovascular health and immune response. Antioxidants Redox Signaling 27, 634–653. doi: 10.1089/ars.2017.7096
Zanuzzo F. S., Beemelmanns A., Hall J. R., Rise M. L., Gamperl A. K. (2020). The innate immune response of Atlantic Salmon (Salmo salar) is not negatively affected by high temperature and moderate hypoxia. Front. Immunol. 11, 1009. doi: 10.3389/fimmu.2020.01009
Zha S., Rong J., Guan X., Tang Y., Han Y., Liu G. (2019). Immunotoxicity of four nanoparticles to a marine bivalve species, tegillarca granosa. J. Hazardous Materials 377, 237–248. doi: 10.1016/j.jhazmat.2019.05.071
Zhai W. D., Zhao H. D., Su J. L., Liu P. F., Li Y. W., Zheng N. (2019). Emergence of summertime hypoxia and concurrent carbonate mineral suppression in the central bohai Sea, China. J. Geophysical Research: Biogeosciences 124. doi: 10.1029/2019JG005120
Zhang T., Qu Y., Zhang Q., Tang J., Cao R., Dong Z., et al. (2021). Risks to the stability of coral reefs in the south China Sea: an integrated biomarker approach to assess the physiological responses of trochus niloticus to ocean acidification and warming. Sci. Total Environ. 782. doi: 10.1016/j.scitotenv.2021.146876
Zhang T., Yan Z., Zheng X., Fan J., Wang S., Wei Y., et al. (2019). Transcriptome analysis of response mechanism to ammonia stress in Asian clam (Corbicula fluminea). Aquat. Toxicol. 214, 105235. doi: 10.1016/j.aquatox.2019.105235
Zhao C., Zhao W. (2020). NLRP3 inflammasome-a key player in antiviral responses. Front. In Immunol. 11, 211. doi: 10.3389/fimmu.2020.00211
Keywords: hypoxia, sulfide, Tegillarca granosa, haemolymph, Immunomodulatory
Citation: Yang Z, Wang Y, Jiang P, Xia F, Xu Y, Tian X and Zhang X (2023) Physiological and transcriptomic analyses reveal critical immune responses to hypoxia and sulfide in the haemolymph of clam Tegillarca granosa. Front. Mar. Sci. 10:1139973. doi: 10.3389/fmars.2023.1139973
Received: 08 January 2023; Accepted: 13 April 2023;
Published: 28 April 2023.
Edited by:
Bin Xia, Qingdao Agricultural University, ChinaReviewed by:
Maocang Yan, Zhejiang Mariculture Research Institute, ChinaZhiguo Dong, Jiangsu Ocean Universiity, China
Copyright © 2023 Yang, Wang, Jiang, Xia, Xu, Tian and Zhang. This is an open-access article distributed under the terms of the Creative Commons Attribution License (CC BY). The use, distribution or reproduction in other forums is permitted, provided the original author(s) and the copyright owner(s) are credited and that the original publication in this journal is cited, in accordance with accepted academic practice. No use, distribution or reproduction is permitted which does not comply with these terms.
*Correspondence: Xiumei Zhang, zhangxiumei@zjou.edu.cn
†These authors have contributed equally to this work