- 1MARE - Marine and Environmental Sciences Centre/ARNET - Aquatic Research Network, ISPA, Instituto Universitário, Lisbon, Portugal
- 2IUCN Species Survival Commission - Seahorse, Pipefish and Seadragon Specialist Group, c/o Project Seahorse, Fisheries Centre, The University of British Columbia, Vancouver, BC, Canada
Temperature is a determinant cue for several behavioral, physiological, and metabolic processes in fish, which occur within a range set to optimize species fitness. Understanding how ocean warming will impact species, at individual and population levels, is, therefore, of utmost relevance for management and conservation purposes. This knowledge assumes particular relevance when it comes to species with unique life history traits that experience multiple threats, such as seahorses. This study aimed to assess the effects of warming on growth, feed intake, and behavioral patterns of the long-snouted seahorse, Hippocampus guttulatus. Fish were collected, before the breeding season, in the Sado estuary, Portugal, and subsequently exposed for an 8-week period to three different temperatures: 17°C, 20°C, and 24°C. Three times a week, behavioral observations were performed. Feed intake was measured every day and individuals were weighed once a week. Results indicate differences in behavioral responses of seahorses exposed to increasingly warming conditions. Under extreme temperature conditions (24°C), fish were more active, and fed more, but this increment of energy through feeding did not translate into increased growth in weight. Altogether, these results indicate that Sado’s population of H. guttulatus may become under thermal stress when exposed, for a long term, to warming conditions that are expected to occur by the end of the century.
1 Introduction
Anthropogenic influence on the production and release of greenhouse gases into the atmosphere has rapidly increased over the last century (Rahman, 2013; Fawzy et al., 2020). Since the mid of the 20th century, the ocean absorbed more than 90% of the excess heat in the atmosphere (Jewett and Romanou, 2017) and more than 25% of the atmospheric CO2 (Sabine et al., 2004). According to the Intergovernmental Panel on Climate Change (IPCC) models, the temperature is projected to increase 1.4 to 4.4°C (more conservative and more extreme scenarios, respectively) (IPCC, 2021), until the end of the century. Ocean warming is expected to affect all levels of the structure and function of marine ecosystems and organisms (Parmesan, 1996; McCarthy et al., 2001; Walther et al., 2002. For ectotherms, such as fish, the temperature is a determinant factor for several behavioral (Wong and Candolin, 2015), physiological (Pörtner and Farrell, 2008), and metabolic processes (Clarke and Fraser, 2004). Warming has been shown to lead to increased metabolic rates (Roessig et al., 2004), interfere with growth (Boltaña et al., 2017), reproduction (Lopes et al., 2020; Servili et al., 2020), digestion processes and feeding rates (Rangel and Johnson, 2018; Pilakouta et al., 2020). It has also the potential to induce behavioral deviations, such as changes in swimming performance (Simon et al., 2017), predator-prey interactions (Domenici et al., 2019), and social interactions (Colchen et al., 2017).
In addition to ocean warming and other climate change-related stressors (e.g. acidification, hypoxia) (Sampaio et al., 2021), marine species are also being impacted by other human-related pressures, such as overexploitation of resources (Jackson et al., 2001), poaching (Correia, 2022), habitat degradation and fragmentation (Airoldi et al., 2008) and pollution (Shahidul Islam and Tanaka, 2004). When combined, these pressures are particularly severe for species with unique life history traits, such as seahorses. Seahorses (Hippocampus spp.) are benthic species, with low mobility, small home ranges, low fecundity, sparse distribution, mate fidelity, and lengthy parental care (Foster and Vincent, 2004). These traits, combined with illegal trade for Traditional Chinese Medicine and unsustainable aquarium display (Foster and Vincent, 2004), and habitat loss (Harasti, 2016; Correia, 2022), render them extremely vulnerable to population declines and local extinctions (Foster and Vincent, 2004; Chong et al., 2010; Macusi et al., 2011; Vincent et al., 2011). Hence, for better control over seahorse trade, all species in the genus have been included in Appendix II of the Convention on International Trade in Endangered Species of Wild Fauna and Flora (CITES Convention) (Foster and Vincent, 2005), and in the International Union for Conservation of Nature (IUCN) Red List of Threatened Species (IUCN, 2020). More recently, IUCN World Conservation Congress approved a resolution (WCC-2020-Res-095) that urges IUCN members to take action toward ensuring the status assessment of all syngnathids (seahorses, pipefish, and seadragons), and their inclusion in national/regional Red Lists, while promoting the protection and restoration of their habitats.
Experimental Studies on the effects of global warming on the behavior and physiology of seahorses are still scarce and limited to just a few species. The temperate long-snouted seahorse, Hippocampus guttulatus, distributes across most of Europe and North Africa, including the Atlantic Ocean, Mediterranean Sea, and Black Sea (Woodall et al., 2015; Lourie et al., 2016). Concurrent with this wide geographic range, the species can also experience a wide range of temperatures throughout the year (Planas et al., 2012), which will vary depending on the latitude. Temperatures in the South of the Iberian Peninsula can range from 10 to 28 °C (Ria Formosa lagoon, Curtis and Vincent, 2006), which clearly differs from temperatures experienced in Northern France or the British Isles, where temperatures can range from 4 to 20°C (Garrick-Maidment et al., 2014). To date, two studies looked into the effects of prolonged exposure (1 month) to increasingly warmer water conditions (18°C – 26°C – 30°C) on H. guttulatus (Aurélio et al., 2013; Faleiro et al., 2015). Authors report an increase in ventilation and metabolic rates of adult seahorses with warming, but fail to detect any further physiological and behavioral impairments, which led the authors to conclude that adults of the species are thermally resilient to heat stress. A similar study on a congener sub-tropical species, H. erectus, which occurs from the Gulf of Mexico to Nova Scotia in water temperatures ranging from 5 to 28°C (Teixeira and Musick, 2001; Lourie et al., 2016), exposed fish for 1 month to increasingly warmer water conditions (22°C – 26°C – 30°C) (Qin et al., 2018). Authors found evidence of persistently higher basal metabolic rate at 30°C, higher stress response on gene expression, and higher mortality rates. Further, a transcriptomic analysis suggests an arrest of muscle development processes, activation of heat shock proteins, and a switch to anaerobic metabolism from the first moments of exposure to high temperatures (30 or 33 °C) (del Vecchio et al., 2022).
The extensive latitudinal range these species inhabit might contribute to differences in thermal tolerance across populations (Helmuth et al., 2002; Pereira et al., 2017), with population-specific thermal limits being set by physiological limitations in aerobic performance (Eliason et al., 2011). Therefore, when assessing the potential impact of climate change it is important to use scenarios that reflect temperatures within and outside a population’s natural variability, and not only scenarios that reflect the variability across the species geographical distribution.
‘The present study aimed to assess the effects of chronic exposure to increasing temperature scenarios on growth, feed intake, and behavioral patterns of adults of H. guttulatus. Individuals were collected in the Sado estuary (Portugal), where temperature can annually range from average 15°C to 19°C, but minimum and maximum temperatures range from 13.8°C (January) and 20.5°C (September) (European Centre for Medium-Range Weather Forecasts, ECMWF, 2022) (Table S1). Fish were exposed for a 2-month period to three different temperature scenarios: ambient temperature (17°C); high temperature (20°C); and an extreme temperature scenario (24°C), which is likely to occur by the end of the century (IPCC, 2021). Furthermore, as the study took place during the species’ breeding season, we provide a descriptive discussion of temperature effects on reproduction based on anecdotal observations.
2 Materials and methods
2.1 Study species
Two species of seahorses inhabit the Portuguese coastal areas, H. guttulatus (Pollom, 2017), and Hippocampus hippocampus (Pollom, 2016). Both species can be found in inshore waters from the coastal environment and are associated with habitats of different complexity (Correia et al., 2015; Correia et al., 2018; Woodall et al., 2018). National legislation has recently included syngnathids under Decree-Law n°38/2021, which approves the legal regime applicable to the protection and conservation of wild flora and fauna and natural habitats of the species listed in the Berne and Bonn Conventions (https://dre.pt/dre/detalhe/decreto-lei/38-2021-164258742). The most extensively studied population in Portugal is the Ria Formosa lagoon’s population, where several studies have taken place to assess population status, identify major threats and apply mitigation measures to support its conservation (Curtis and Vincent, 2006; Caldwell and Vincent, 2012; Correia et al., 2013; Correia et al., 2015; Correia et al., 2018; Correia, 2022). However, there are newly recorded seahorse populations, such as in the Sado and Tagus estuaries which are understudied and in need of attention (unpublished data). The Sado Estuary is the second-largest estuary in Portugal and one of the most important wetlands in the national territory, integrating an important natural reserve. This region has the largest distribution of seagrass species in Portugal (Cunha and Serrão, 2011), only surpassed by the Ria Formosa lagoon, and supports species with both economic and conservation value, including seahorses.
2.2 Fish collection and experimental setup
Adult males and females (n=22 individuals; 11 males and 11 females) of the long-snouted seahorse were individually collected by scuba diving in the seagrass meadows of Tróia, Portugal (38.462311, -8.856218), on April 8th, 2022 (Figure 1). Permit to collect fish from the wild was granted by the National Institute of Nature Conservation and Forests (ICNF, permits 288 – 290/2022/CAPT). Individuals were immediately placed in large containers filled with seawater from the collection site, with aeration, and controlled temperature, and transported to ISPA fish facilities.
At arrival, seahorses were placed in six 80-L aquaria (40x40x60 cm), at a density of 2 males and 2 females per aquarium (except 1 tank, with only 1 male and 1 female). Soon after being in these conditions, males and females paired and formed couples based on body size. All aquaria were equipped with a protein skimmer (TMC Reef-Skim 200), biological and mechanical filtration (internal filter, Max 104 F), and maintained under temperature and salinity conditions matching field site (average 15°C and 35 PSU), and a photoperiod of 14L: 10D. To provide environmental enrichment and holdfast, plastic chains were added to each aquarium (Correia et al., 2013). Seahorses were left under these conditions for 15 days, to acclimate to laboratory conditions. Fish were fed once per day, ad libitum, with frozen wild-caught mysis (Mesopodopsis slabberi). Temperature and salinity were daily measured, in the morning. Ammonia, nitrites and nitrates, were monitored frequently, and kept below critical levels (0.1, 0.3, and 10.0 mg L-1, respectively). Aquaria were cleaned daily, in the morning, before feeding. Subsequently, aquaria were randomly assigned to three temperature treatments: ambient temperature (17°C), matching average temperature at the beginning of the species breeding season; high temperature (20°C), matching temperatures within the species range of variability which they can experience in their natural habitat for a short period; and extreme temperature (24°C), reflecting a scenario that falls outside the natural thermal variability of this population and are likely to occur by the end of the century. Sea surface temperatures, represented as monthly averages from daily means, were extracted from remotely sensed climate models (European Centre for Medium-Range Weather Forecasts, ECMWF, 2022) from spatial points (geo-referenced occurrence data) representing the habitat where the species was captured (Table S1). Temperatures in all treatments increased gradually, using heaters, by about 1°C per day to avoid stress and heat shock associated with rapid temperature changes. Aquaria from the extreme treatment (24°C) were the first to start increasing temperature, followed by aquaria from the high treatment (20°C), 4 days later, and finally, aquaria from the ambient treatment (17°C) had their temperature increased 7 days after the first aquaria. This ensured that all treatments reached the experimental treatment temperature (considered day 0 of exposure) at the same time. At this stage, each individual was measured for height (Lourie, 2003) and weight (wet weight) (Table S2). Within sex, initial weight (W) and height (H) did not differ across treatments (Wmales: F = 0.176, p = 0.277; Hmales: F = 0.933, p = 0.432; Wfemales: F = 0.677, p = 0.535; Hfemales: F = 0.402, p = 0.682), with males average weight and height of 11.99 ± 1.62 g, and 13.79 ± 0.75 cm, respectively, and females average weight and height of 10.70 ± 1.40 g, and 13.71 ± 0.70 cm, respectively. Each treatment had two replicate aquaria, each with 2 couples (i.e., 4 couples for treatment). Aquaria were placed side by side, divided by a white opaque plastic sheet to avoid intraspecific interactions between tanks (Papoutsoglou et al., 2000; Tamazouzt et al., 2000). Ambient temperature treatment had only 3 couples (one aquarium had only 1 couple), since it was only possible to collect 11 females and 11 males. As this is a species with national conservation status and protected by law, it was decided to keep only three couples under control conditions.
Exposure to temperature treatments lasted 2 months. At the end of the experimental period, temperatures from high and extreme treatments were gradually decreased till the matching temperature from ambient treatment, which matched the temperature at the collection site at that time of the year (i.e. July). Seahorses were then released back to the same area where were previously collected, as per requirement from the permit issuer.
2.3 Behavior
Behavioral observations were performed three times a week, based on an ethogram adapted from Faleiro et al. (Faleiro et al., 2008; Faleiro et al., 2015) (Table 1). Focal observations were made in the morning (between 07:00 and 10:00 hours), before feeding, to avoid post-prandial bias. Observations were made by a motionless observer standing in front of the aquarium. Each focal observation lasted 3 minutes, and sea horses were observed one at a time in each aquarium. In every observation day, the order of the seahorses observed changed, to add randomization to the process and avoid an effect of the order of observation. As individuals from each aquarium had a set of distinctive traits (body size and spot patterns), we were able to keep the ID of each individual throughout the exposure period (Correia et al., 2014) and thus avoiding invasive individual identification methods such as VIE tagging (Curtis, 2006).
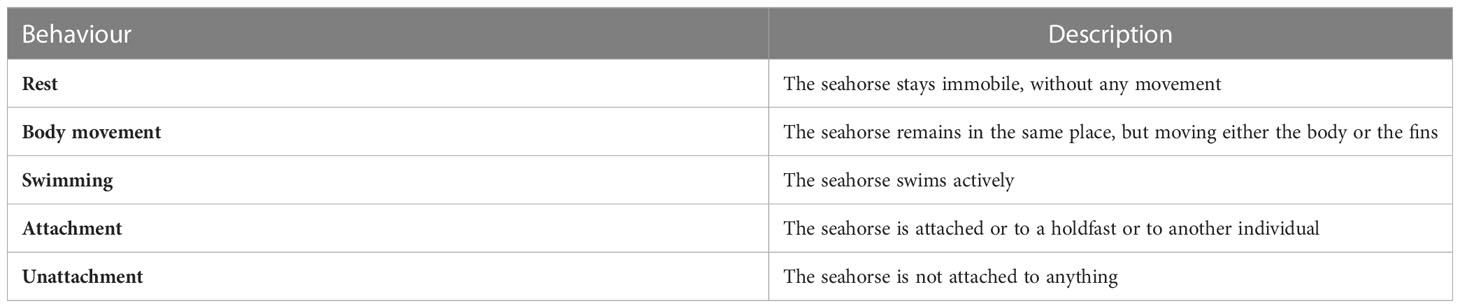
Table 1 Ethogram of Hippocampus guttulatus describing activity patterns and holdfast attachment (adapted from Faleiro et al., 2008).
2.4 Feed intake
To allow ad libitum conditions for every fish, fish under treatments A and B were daily fed 5% of body weight (BW) and fish from treatment C were daily fed 7% of BW (Palma et al., 2011) . To maintain the proper feed rations, the daily wet weight of each ration was altered in accordance with the average wet weight growth for each treatment (Palma et al., 2008). The values were initially adjusted based on uneaten food to avoid degradation of water quality. Food was weighed and thawed before feeding seahorses, daily. After 24h, uneaten food items were siphoned out of the tank and collected in a hand-held net with fine mesh, over a paper to dry. Then, every piece of uneaten food was sorted using a tweezer and placed in a plastic petri dish for weighing. Indistinct matter was considered as debri and part of faeces and discarded from the weighing. Feed intake (FI) was calculated using the formula:
where FS is the amount of food supplied (g) and FU is the amount of uneaten food (g). Feed intake was measured daily, except for weekends.
2.5 Weight
Individuals were weighed (g) on a weekly basis to determine the amount of food (5 and 7% of body weight) to provide throughout the exposure period. The procedure involved placing each individual in a shallow tray with seawater and placed on a scale, and was performed as quickly as possible. Manipulation of individuals might induce stress and alter behavioral responses. Although we did not measure stress responses to manipulation, we did not observe signs of disturbance attributable to handling stress. Fish resumed their normal rest state as soon as they were placed back in the aquaria, and were seen feeding when food was supplied. Therefore, we consider that fish recovered from handling stress in just a few hours, and this standardized procedure across treatments did not alter behavioral observations throughout the study period.
Data on the initial and final weight were used to calculate mean weight gain (Palma et al., 2011):
where Wf is the final seahorse wet weight and Wi is the initial wet weight.
2.6 Data analysis
Linear mixed-effects models (“lmerTest” package in R) were used to access the effect of temperature treatments throughout time in the different seahorse behaviours: rest, swimming, body movement, attach and unattached, and also feed intake. Treatment and Day of Observation (Dobs) were used as fixed effects and Animal ID (Individual) and Holding Aquarium as random effects, for all behaviours, except feed intake. For feed intake only Holding Aquarium was considered as random effect. Day of Observation (Dobs) considered the first day of observation as day 0. A generalized linear model was used to determine the effect of the treatments in the weight gain of the seahorses. Assumptions of homoscedasticity and normality of the residuals were examined by visual inspection of residual fitted plots.The statistical analysis was performed with R statistics, Rstudio (Version 4.2.1). Statistical significance was considered when p<0.05.
3 Results
3.1 Temperature effects on behaviour
Long-snouted seahorses spent significantly less time resting when exposed to extreme (24°C) temperature, and spent significantly more time swimming when compared with ambient temperature (17°C), where individuals spent more time motionless (Figures 2, 3; Table 2, Table S3). Dobs had also a significant effect on rest and swimming behaviors, but no interation between Dobs and Temperature was detected (Table 2).
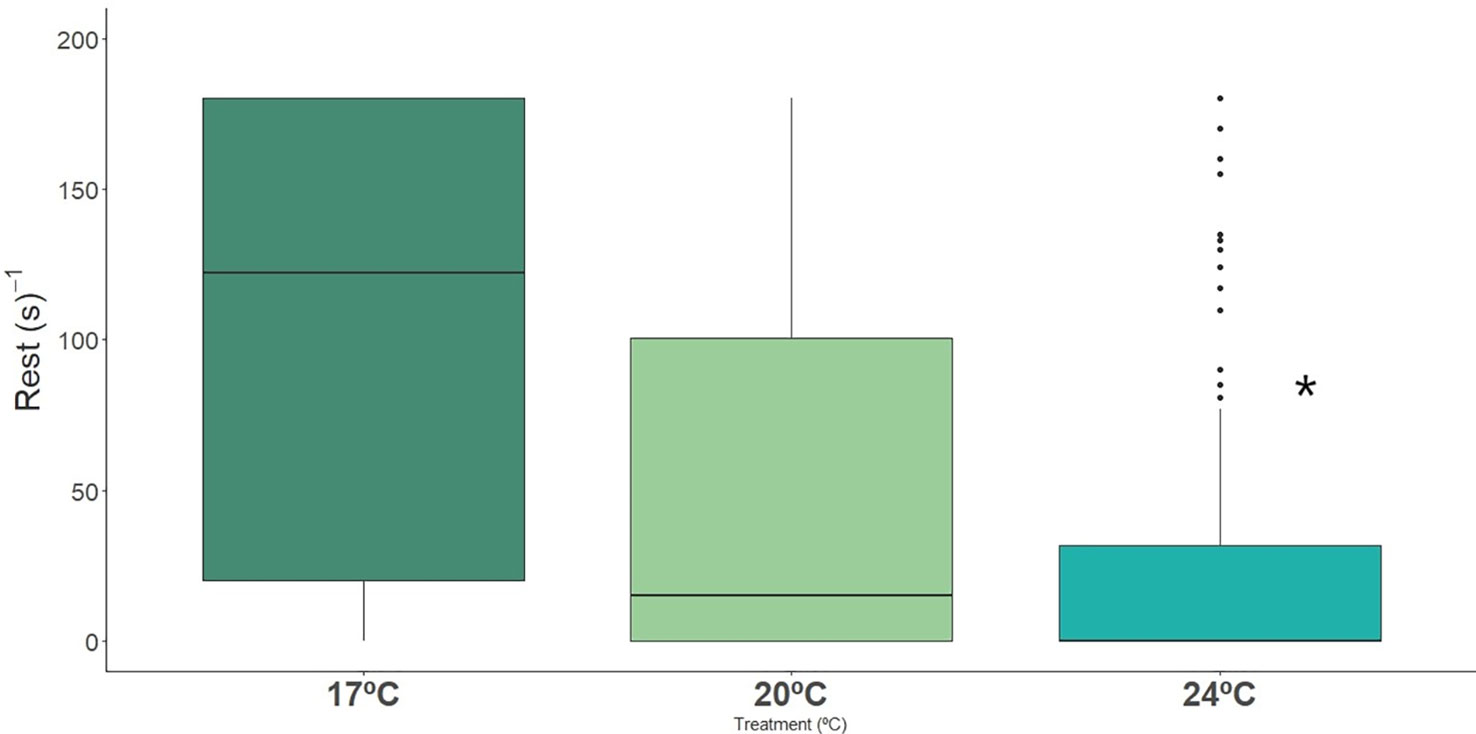
Figure 2 Time spent in stationary behavior (s-1) by long-snouted seahorse exposed to different temperature treatments (17°C, 20°C and 24°C). Bold * represent significant differences from control treatment.
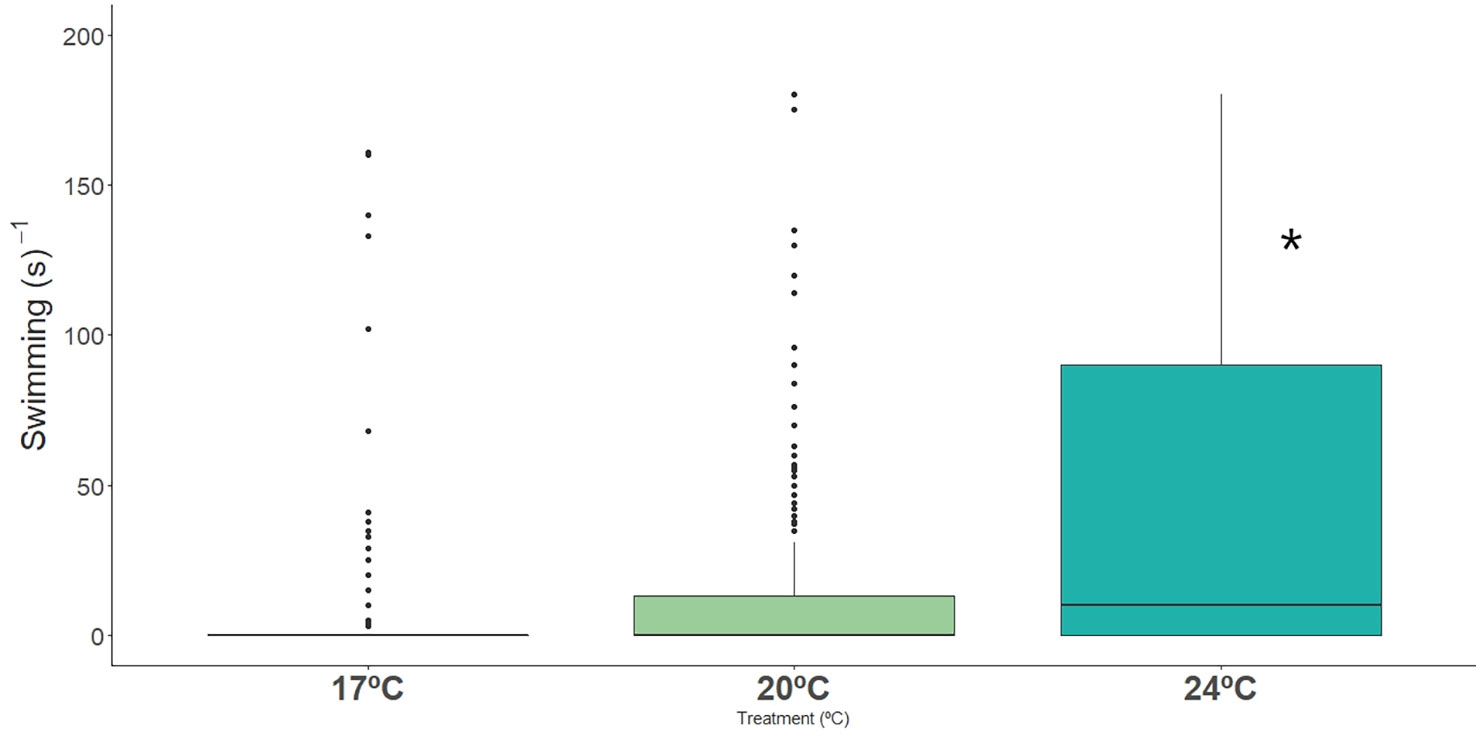
Figure 3 Time spent swimming (s-1) by long-snouted seahorse exposed to different temperature treatments (17°C, 20°C and 24°C). Bold * represent significant differences from control treatment.
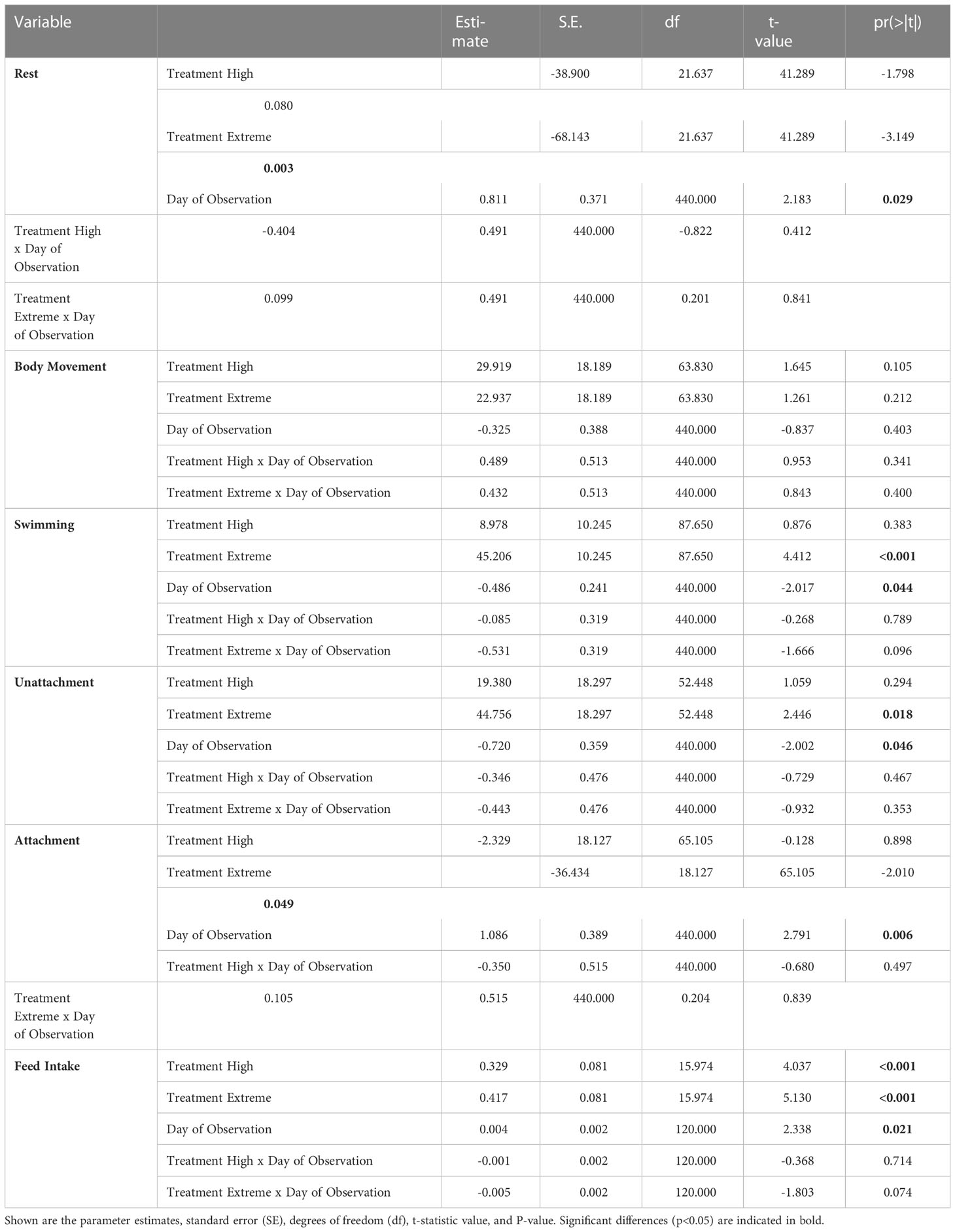
Table 2 Statistical results of the Linear mixed effects models on time (s) seahorse spent in rest, body movement, swimming, attached and unattached, and feed intake of long-snouted seahorse exposed to three temperature treatments – control (17°C), high (20°C), and extreme (24°C).
Under extreme temperature (24 °C) fish were also seen spending less time attached compared to fish from ambient temperature, and Dobs had also a significant effect (Figure 4, Table 2, Table S3).
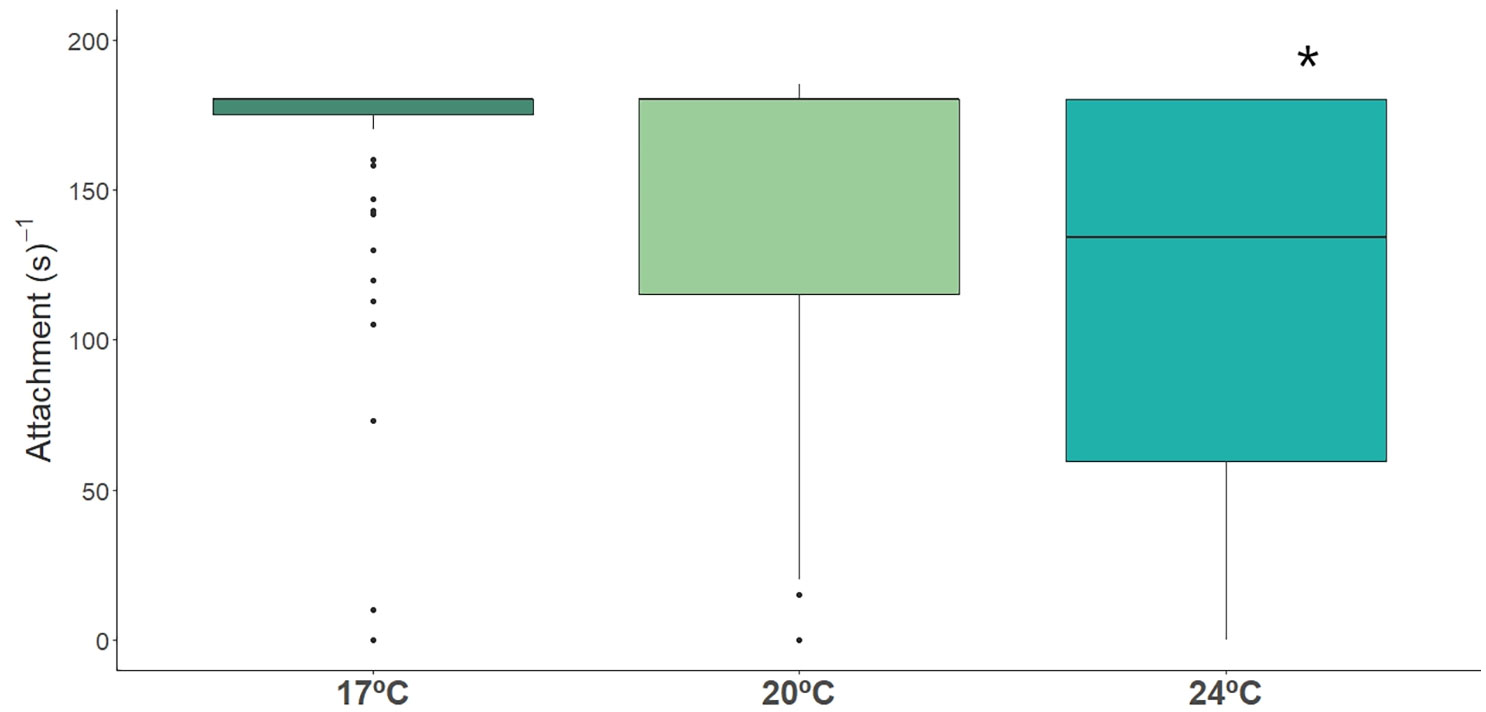
Figure 4 Time spent attached (s-1) by long-snouted seahorse exposed to different temperature treatments (17°C, 20°C and 24°C). Bold * represent significant differences from control treatment.
Time spent stationary but moving either the body or the fins (body movement) did not differ across treatments, or Dobs (Table 2, Table S3).
3.2 Temperature effects on feed intake
Fish under warming conditions (20 and 24°C) showed significantly higher feed intake when compared to fish under ambient temperature (17°C) (Figure 5, Table 2, Table S3). Dobs had also a significant effect on feed intake, but no interation between Dobs and Temperature was detected (Table 3).
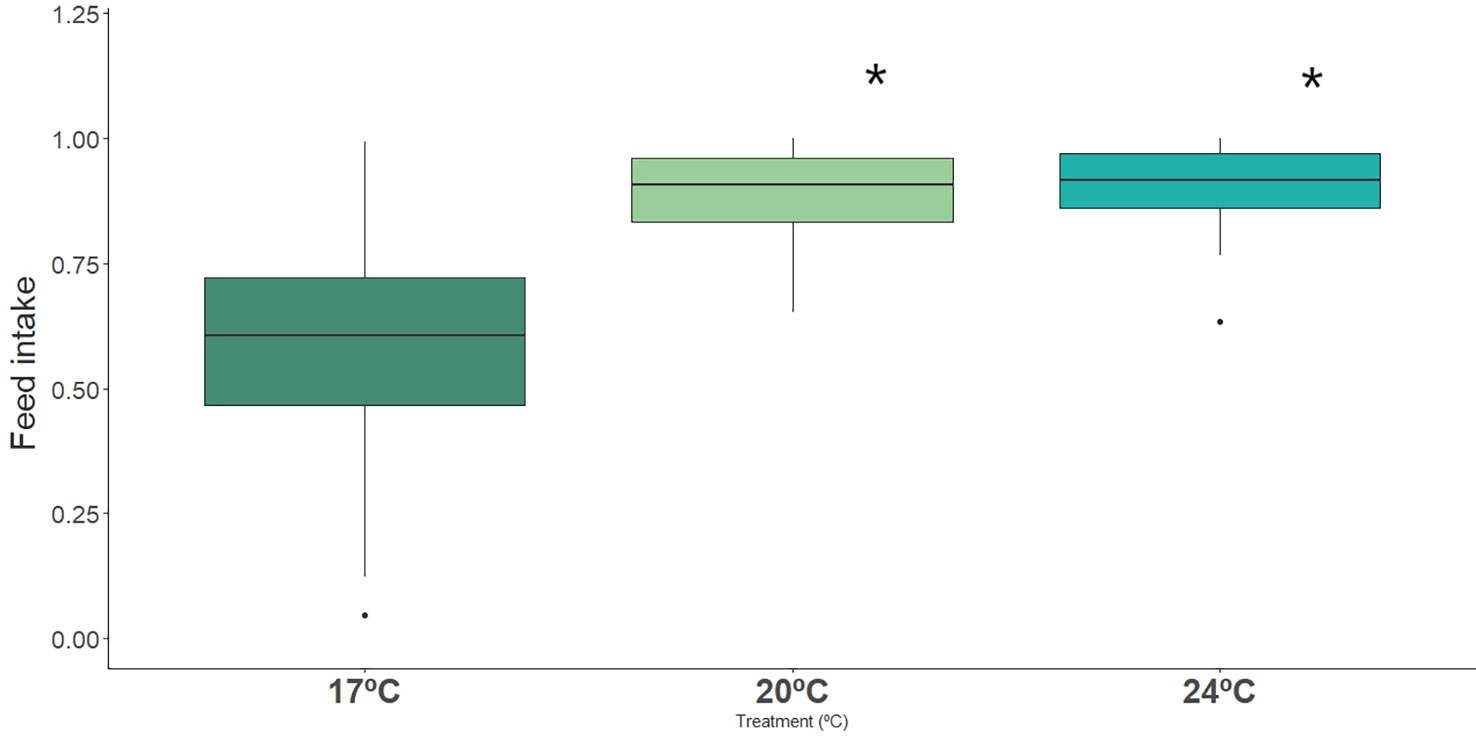
Figure 5 Impact of ocean warming on the feed intake of long-snouted seahorse exposed to different temperature treatments (17°C, 20°C and 24°C). Bold * represent significant differences from control treatment.

Table 3 Statistical results of the Generalized Linear on weight gain (%) of long-snouted seahorse exposed to three temperature treatments – control (17°C), high (20°C), and extreme (24°C).
3.3 Temperature effects on weight gain
Weight gain, at the end of the exposure period, did not differ across treatments (Figure 6, Table 3, Table S2).
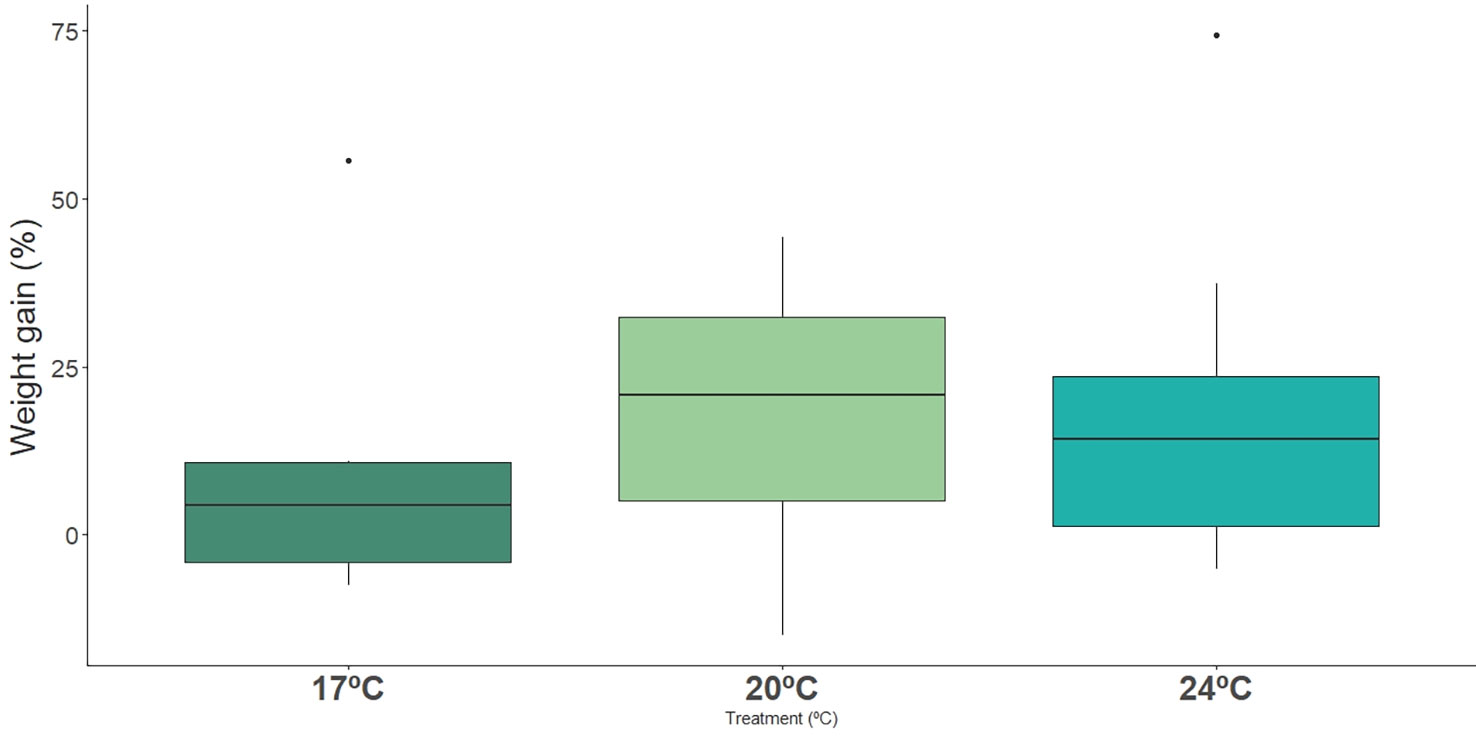
Figure 6 Impact of ocean warming on weight gain (%) of long-snouted seahorse exposed to different temperature treatments (17°C, 20°C and 24°C).
4 Discussion
Results from the present study reflect differences in behavioral responses in seahorses exposed to different elevated temperatures for a long-term period (2 months). Fish were significantly more active under extreme temperature conditions (24°C) (less time resting, more time swimming and unattached), and were also seen feeding more under both high and extreme temperatures (20°C and 24°C). Higher food ingestion did not translate into an increased body condition, though, as the weight gain of the fish did not differ across treatments. Temperature can impact differently fish locomotion and activity. While some studies suggest that individual activity increases with temperature (Ojanguren and Brañta, 2000; Biro et al., 2010), others conclude that swimming ability decreases under warming conditions (Johansen and Jones, 2011). The elevated temperature was shown to increase swimming activity in Atlantic cod, Gadus morhua (Claireaux et al., 2000), but impact negatively swimming activity in juvenile brown trout, Salmo trutta (Ojanguren and Brañta, 2000). In the current study, we found a significant temperature effect on swimming activity, with fish under extreme temperature treatment (24°C) being more active (more time spent swimming) and spending less time resting. Similarly, a study on the activity of adult lined seahorses showed that when they were exposed to higher temperatures close to the species’ thermal limit (30°C), they increased their swimming as opposed to seahorses maintained at lower temperatures (22 and 26°C) (Qin et al., 2018). Interestingly, two other studies conducted on the long-snouted seahorse, from the same collection site, exposed to much more extreme temperature conditions (26°C, 28°C, 30°C), did not find an effect of temperature on frequency of swimming or periods of inactivity when compared to fish from control temperatures (18°C) (Aurélio et al., 2013; Faleiro et al., 2015). Some studies in other fish species have demonstrated that swimming activity decreases at low temperatures, increase to a peak and then decrease as the temperature approaches the upper thermal limit (Myrick and Cech, 2000; Ojanguren and Brañta, 2000). This could explain the results from those authors’ studies – the extreme temperatures the seahorses were exposed to might already be close to their thermal limit. Another possible explanation for the observed differences might relate to methodological aspects. In the current study, we run behavioral observations three times a week, for 2 months, and observations were performed early in the morning before feeding. Aurélio et al. (2013) and Faleiro et al. (2015) had shorter periods of behavioral observations and run observations 30 min after feeding. This might have influenced the time spent swimming/inactive as fish might still be under post-prandial effects.
In response to a warmer environment, fish will increase their energetic demands and consequently will need to ingest more food (Sswat et al., 2018) , to maintain core activities. As expected, in our study, fish under the extreme temperature treatment (24°C) significantly ingested more food than fish under ambient and high-temperature treatments (17°C and 20°C). Contrasting results were reported by Aurélio et al. (2013) and Faleiro et al. (2015), with no changes in feed intake rates of H. guttulatus under extreme water temperatures (26°C, 28°C, 30°C). Also, Qin et al. (2018) fail to detect an influence of temperature on the feeding frequency of the H. erectus after 1 week at 30°C, which is close to the species’ thermal limit. However, in these 3 studies, authors observed an increase in metabolic rates and ventilation rates (which we did not measure in the current study), supporting an increased metabolic activity under higher temperatures. The increase in metabolic rates but the absence of a temperature effect on feeding rates might indicate that the species was already outside their optimum temperature limit, as feed intake might decrease if the temperature surpasses the species’ optimum limits (Volkoff and Rønnestad, 2020). Therefore, individuals might have adopted a saving-energy strategy by not spending energy on feeding but making digestion more efficient.
Temperature increases within an appropriate range usually promote fish growth (Green and Fisher, 2004), and therefore we were expecting to have fish under the better condition at higher temperatures. In our study, fish did not show any significant change in their body weight among the different temperatures they were exposed to. Similarly, Qin et al. (2018) did not find differences in weight or body height of adult H. erectus exposed to different temperature scenarios (22, 26, and 30 °C) for a 4-week period. However, the seahorse hepatosomatic index (HSI) was lower under 30 °C, while metabolic rates were higher at this temperature. Authors argue that thermal stress inhibited hepatic energy reserves and leads to lower HSI by increasing energy consumption. In our study the lack of temperature-induces effects in fish growth was possible due to fish being more active under extreme temperatures (24°C), thus spending more energy. Nonetheless, we can also not rule out the possibility that seahorses were already under thermal stress at these extreme temperatures. Mascaró et al. (Mascaró et al., 2016, Mascaró et al., 2019), for example, report that chronic exposure (1 month) to the extreme temperature of 30°C significantly reduced juvenile H. erectus growth. Using transcriptome data, del Vecchio et al. (2022) provide evidence of repression of the MEF2A gene within the first 0.5 h of exposure of the H. erectus to target temperatures (30 and 33 °C). This gene is implicated in the regulation of growth and differentiation of muscle fibers, and its repression might justify changes in growth under temperatures close to the s’ecies’ thermal limits. Despite the relevance of this study, the rate of thermal increase untill reaching the target temperatures (abrupt: < 5 min; gradual, 1-1.5 °C every 3 h) and the fact that the authors only collected data after 0.5 h of exposure prevents us from making further conclusions on the effects of prolonged warming on growth.
A word of caution is needed when interpreting results of the current study, as it took place during the breeding season of the species, and reproduction changes activity rate (which likely justifies the observed significant effect of Day of Observation) and physiological condition (weight gain) of individuals. Therefore, its influence on the response variables under measure is difficult to control. Nevertheless, courtship behaviors and daily greetings (Vincent, 1995) were regularly and evenly recorded across the three temperature treatments during the 8-week exposure period (19 courtship events under ambient temperature; 21 events under high temperature; 21 events under extreme temperatures). Moreover, all males across treatments were seen to become pregnant at a certain point of the experimental period, even though the number of successful pregnancies differed across treatments. Therefore, the observed changes at the behavioral level and feeding rate are likely to truly reflect a temperature effect, rather than just an influence of reproductive activity. A total of 7 successful breeding events were registered, being 4 events (in 3 out of the 4 males) in couples from the extreme temperature (24°C), 2 events (in 2 out of the 4 males) in couples from the high temperature (20°C), and 1 event (in 1 out of the 3 males) in ambient temperature (17°C). As reproduction is considered to be sensitive to thermal stress (Pörtner and Farrell, 2008) our results, then, might indicate that the range of tested temperatures was not enough to negatively impact reproduction, and it could even suggest that breeding is stimulated at higher temperatures. Qin et al. (2018) found no significant negative effects on gonad development or reproductive endocrine regulation genes in response to chronic thermal stress (1 month, at 30 °C) in the H. erectus. The authors argue that the species’ reproductive behavior is adapted to higher-temperature conditions, as H. erectus seasonally migrate into warm waters for breeding and return to offshore areas when the water cools (Boehm et al., 2015). Nevertheless, in the present study, and despite the suggestion of increased reproductive activity under high and extreme temperature conditions (20 and 24°C), episodes of unsuccessful egg transfer from the female to the male were recorded only under these temperature conditions and were frequently observed in couples where males were not responsive to females approach and courtship. Moreover, abortion was also registered at high temperatures (20°C), with the male releasing embryos of several developmental stages. Altogether, these observations might suggest that there is a high but unsuccessful investment in reproduction under warming conditions. Consequences at the offspring level were not possible to infer, as we were unable to maintain the newborns. Future studies should take a closer look at the impacts of high temperatures on the reproductive success of these species.
Overall, and despite the limited number of individuals available to run this experimental study, our results points to a negative consequence of ocean warming on behavior and feeding rate of adult H. guttulatus. To truly understand if these individuals were already under thermal stress at the extreme temperature of 24°C, additional physiological and biochemical endpoints would be needed. Determination of metabolic rates, such as minimum and maximum metabolic rate, is a highly useful physiological tool that allows a better understanding of fish metabolic performance under different environmental conditions, and, as it involves minimum manipulation of the individual, there is a low risk of death. The set of physiological responses to warming is the reflection of a prompt cellular stress reaction triggered to maintain the organism’s homeostasis, and it involves biochemical adjustments of metabolic pathways and the regulatory activity of the antioxidant system (Sopinka et al., 2016). These indicators provide highly relevant information for evaluating stress response levels, but the downside is that they require the euthanasia of individuals, for tissue and blood sampling. To avoid invasive and lethal practices with a species that holds a special conservation status, we decided not to evaluate these physiological responses.
Seahorses are a great model to study the effects of climate change on the fitness and behavior of sedentary and low dispersal species, acting as an indicator of ecosystem health. These species can be used as flagship species for conservation issues and can be found worldwide in some of the most vulnerable marine ecosystems in shallow waters (Vincent et al., 2011). The population of the H. guttulatus from the Sado estuary is subject to intense pressure from other anthropogenic activities, such as overexploitation, pollution, and habitat destruction. All these pressures reduce the likelihood of these species to acclimatize and hinder the opportunity to persist in a changing environment. If protective measures are not taken, seahorse populations might suffer a sharp decline in numbers, and in long term affect this population’s fitness and survival.
Data availability statement
The raw data supporting the conclusions of this article will be made available by the authors, without undue reservation.
Ethics statement
This study was carried out under the approval of Direção-Geral de Alimentação e Veterinária (DGAV, Portuguese Authority for Animal Health, permit 0421/000/000/2020) and according to the ISPA University’s animal ethics guidelines.
Author contributions
AC: methodology, data collection, data analysis, writing- original draft preparation, writing - review and editing; MC: conceptualization, methodology, writing- original draft preparation, writing - review and editing; GS: methodology, writing - review and editing; AL: data analysis, writing - review and editing; AF: funding acquisition, project administration, conceptualization, methodology, writing- original draft preparation. All authors contributed to the article and approved the submitted version.
Funding
This study had the support of FCT through UIDB/MAR/04292/2020, UIDP/MAR/04292/2020 awarded to MARE, and through the project LA/P/0069/2020 granted to the Associate Laboratory ARNET. This work was further supported by the Projects NextGen (PTDC/CTA-AMB/31532/2017), and SARDITEMP (PTDC/BIA-BMA/32209/2017), co-financed by FCT and European Union through FEDER (European Regional Development Fund), and CavAlmar, funded by Câmara Municipal Almada. The study also benefited from a PADI Foundation grant attributed to AF.
Acknowledgments
The authors would like to thank António Roleira for the valuable assistance throughout the experimental work; Friederike Peiffer, Mariana Coxey, Mário Rolim, Noelia Rios, and Sílvia Tavares for the support on the fieldwork; and Roland Pfeiffer for statistical advice.
Conflict of interest
The authors declare that the research was conducted in the absence of any commercial or financial relationships that could be construed as a potential conflict of interest.
Publisher’s note
All claims expressed in this article are solely those of the authors and do not necessarily represent those of their affiliated organizations, or those of the publisher, the editors and the reviewers. Any product that may be evaluated in this article, or claim that may be made by its manufacturer, is not guaranteed or endorsed by the publisher.
Supplementary material
The Supplementary Material for this article can be found online at: https://www.frontiersin.org/articles/10.3389/fmars.2023.1136748/full#supplementary-material
References
Airoldi L., Balata D., Beck M. W. (2008). The Gray zone: Relationships between habitat loss and marine diversity and their applications in conservation. J. Exp. Mar. Biol. Ecol. 366 (1–2), 8–15. doi: 10.1016/j.jembe.2008.07.034
Aurélio M., Faleiro F., Lopes V. M., Pires V., Lopes A. R., Pimentel M. S., et al. (2013). Physiological and behavioral responses of temperate seahorses (Hippocampus guttulatus) to environmental warming. Mar. Biol. 160 (10), 2663–2670. doi: 10.1007/s00227-013-2259-8
Biro P. A., Beckmann C., Stamps J. A. (2010). Small within-day increases in temperature affects boldness and alters personality in coral reef fish. Proc. R. Soc. B: Biol. Sci. 277 (1678), 71–77. doi: 10.1098/rspb.2009.1346
Boehm J. T., Waldman J., Robinson J. D., Hickerson M. J. (2015). Population genomics reveals seahorses (Hippocampus erectus) of the Western mid-Atlantic coast to be residents rather than vagrants. PloS One 10 (1), e0116219-. doi: 10.1371/journal.pone.0116219
Boltaña S., Sanhueza N., Aguilar A., Gallardo-Escarate C., Arriagada G., Valdes J. A., et al. (2017). Influences of thermal environment on fish growth. Ecol. Evol. 7 (17), 6814–6825. doi: 10.1002/ece3.3239
Caldwell I. R., Vincent A. C. J. (2012). Revisiting two sympatric European seahorse species: apparent decline in the absence of exploitation. Aquat. Conserv.: Mar. Freshw. Ecosyst. 22 (4), 427–435. doi: 10.1002/aqc.2238
Chong V. C., Lee P. K. Y., Lau C. M. (2010). Diversity, extinction risk and conservation of Malaysian fishes. J. Fish Biol. 76 (9), 2009–2066. doi: 10.1111/j.1095-8649.2010.02685.x
Claireaux G., Webber D. M., Lagardère J.-P., Kerr S. R. (2000). Influence of water temperature and oxygenation on the aerobic metabolic scope of Atlantic cod (Gadus morhua). J. Sea Res. 44 (3–4), 257–265. doi: 10.1016/S1385-1101(00)00053-8
Clarke A., Fraser K. P. P. (2004). Why does metabolism scale with temperature? Functional Ecology 18 (2), 243–251. doi: 10.1111/j.0269-8463.2004.00841.x
Colchen T., Teletchea F., Fontaine P., Pasquet A. (2017). Temperature modifies activity, inter-individual relationships and group structure in a fish. Curr. Zool. 63 (2), 175–183. doi: 10.1093/cz/zow048
Correia M. (2022). Monitoring of seahorse populations, in the ria Formosa lagoon (Portugal), reveals steep fluctuations: Potential causes and future mitigations. Proc. Zool. Soc. 75 (2), 190–199. doi: 10.1007/s12595-021-00394-2
Correia M., Caldwell I. R., Koldewey H. J., Andrade J. P., Palma J. (2015). Seahorse (Hippocampinae) population fluctuations in the ria Formosa lagoon, south Portugal. J. Fish Biol. 87 (3), 679–690. doi: 10.1111/jfb.12748
Correia M., Koldewey H. J., Andrade J. P., Esteves E., Palma J. (2018). Identifying key environmental variables of two seahorse species (Hippocampus guttulatus and hippocampus hippocampus) in the ria Formosa lagoon, south Portugal. Environ. Biol. Fish. 101 (9), 1357–1367. doi: 10.1007/s10641-018-0782-7
Correia M., Palma J., Koldewey H., Andrade J. P. (2013). Can artificial holdfast units work as a habitat restoration tool for long-snouted seahorse (Hippocampus guttulatus cuvier)? J. Exp. Mar. Biol. Ecol. 448, 258–264. doi: 10.1016/j.jembe.2013.08.001
Correia M., Palma J., Koldewey H., Andrade J. P. (2014). The use of a non-invasive tool for capture–recapture studies on a seahorse hippocampus guttulatus population. J. Fish Biol. 84 (4), 872–884. doi: 10.1111/jfb.12304
Cunha A. H., Serrão E. A. (2011). Tools for seagrass conservation and management in Portugal. Ecologia 3, 23–36.
Curtis J. M. R. (2006). Visible implant elastomer color determination, tag visibility, and tag loss: Potential sources of error for mark–recapture studies. North Am. J. Fish. Manage. 26 (2), 327–337. doi: 10.1577/M05-099.1
Curtis J. M. R., Vincent A. C. J. (2006). Life history of an unusual marine fish: survival, growth and movement patterns of hippocampus guttulatus cuvier 1829. J. Fish Biol. 68 (3), 707–733. doi: 10.1111/j.0022-1112.2006.00952.x
del Vecchio G., Galindo-Sánchez C. E., Tripp-Valdez M. A., López-Landavery E. A., Rosas C., Mascaró M. (2022). Transcriptomic response in thermally challenged seahorses hippocampus erectus: The effect of magnitude and rate of temperature change. Comp. Biochem. Physiol. Part B: Biochem. Mol. Biol. 262, 110771. doi: 10.1016/j.cbpb.2022.110771
Domenici P., Allan B. J. M., Lefrançois C., McCormick M. I. (2019). The effect of climate change on the escape kinematics and performance of fishes: implications for future predator–prey interactions. Conserv. Physiol. 7 (1), coz078. doi: 10.1093/conphys/coz078
ECMWF. (2022). Available at: https://www.ecmwf.int/
Eliason E. J., Clark T. D., Hague M. J., Hanson L. M., Gallagher Z. S., Jeffries K. M., et al. (2011). Differences in thermal tolerance among sockeye salmon populations. Science 332 (6025), 109–112. doi: 10.1126/science.1199158
Faleiro F., Baptista M., Santos C., Aurélio M. L., Pimentel M., Pegado M. R., et al. (2015). Seahorses under a changing ocean: the impact of warming and acidification on the behaviour and physiology of a poor-swimming bony-armoured fish. Conserv. Physiol. 3 (1), cov009. doi: 10.1093/conphys/cov009
Faleiro F., Narciso L., Vicente L. (2008). Seahorse behaviour and aquaculture: How to improve hippocampus guttulatus husbandry and reproduction? Aquaculture 282 (1), 33–40. doi: 10.1016/j.aquaculture.2008.05.038
Fawzy S., Osman A., Doran J. W., Rooney D. (2020). Strategies for mitigation of climate change: A review. Environ. Chem.Lett. 18 (6), 2069–2094. doi: 10.1007/s10311-020-01059-w
Foster S. J., Vincent A. C. J. (2004). Life history and ecology of seahorses: implications for conservation and management. J. Fish Biol. 65 (1), 1–61. doi: 10.1111/j.0022-1112.2004.00429.x
Foster S. J., Vincent A. C. J. (2005). Enhancing sustainability of the international trade in seahorses with a single minimum size limit. Conserv. Biol. 19 (4), 1044–1050. doi: 10.1111/j.1523-1739.2004.00157.x-i1
Garrick-Maidment N., Durant E., Newman J. (2014). Year 5 report on the Seahorse Tagging Project at South Beach, Studland Bay in Dorset. The Seahorse Trust. Published online. http://www.theseahorsetrust.org.
Green B. S., Fisher R. (2004). Temperature influences swimming speed, growth and larval duration in coral reef fish larvae. J. Exp. Mar. Biol. Ecol. 299 (1), 115–132. doi: 10.1016/j.jembe.2003.09.001
Harasti D. (2016). Declining seahorse populations linked to loss of essential marine habitats. Mar. Ecol. Prog. Ser. 546, 173–181. doi: 10.3354/meps11619
Helmuth B., Harley C. D. G., Halpin P. A., O’Donnell M. P., Hofmann G. E., Blanchette C. A. (2002). Climate change and latitudinal patterns of intertidal thermal stress. Science 298(5595), 1015–1017. doi: 10.1126/science.1076814
IPCC (2021). “Climate change 2021 the physical science basis summary for policymakers working group I contribution to the sixth assessment report of the intergovernmental panel on climate change,” in Climate change 2021: The physical science basis.
IUCN (2020) The IUCN red list of threatened species version 2020–1. Available at: http://www.iucnredlist.org.
Jackson J. B., Kirby M. X., Berger W. H., Bjorndal K., Botsford L. W., Bourque B. J., et al. (2001). Historical overfishing and the recent collapse of coastal ecosystems. Science 293, 629–637. doi: 10.1126/science.1059199
Jewett L., Romanou A. (2017). Ocean acidification and other ocean changes. In: Climate Science Special Report: Fourth National Climate Assessment, Volume I [Wuebbles D. J., Fahey D. W., Hibbard K. A., Dokken D. J., Stewart B.C., Maycock T. K., et al (eds.)]. U.S. Global Change Research Program, Washington, DC, USA, pp. 364–392. doi: 10.7930/J0QV3JQB
Johansen J. L., Jones G. P. (2011). Increasing ocean temperature reduces the metabolic performance and swimming ability of coral reef damselfishes. Global Change Biol. 17 (9), 2971–2979. doi: 10.1111/j.1365-2486.2011.02436.x
Lopes A. F., Faria A. M., Dupont S. (2020). Elevated temperature, but not decreased pH, impairs reproduction in a temperate fish. Sci. Rep. 10 (1). doi: 10.1038/s41598-020-77906-1
Lourie S. (2004). A Guide to the Identification of Seahorses. Project Seahorse and TRAFFIC North America. (Washington D.C.: University of British Columbia and World Wildlife Fund). Available at: https://projectseahorse.org/wp-content/uploads/2021/06/Seahorse_ID_Guide_2004.pdf
Lourie S. A., Pollom R. A., Foster S. J. (2016). A global revision of the seahorses hippocampus rafinesque 1810 (Actinopterygii: Syngnathiformes): taxonomy and biogeography with recommendations for further research. Zootaxa 4146 (1), 1–66. doi: 10.11646/zootaxa.4146.1.1
Macusi E. D., Katikiro R. E., Deepananda K. A., Jimenez L. A., Conte A. R., Fadli N. (2011). Human induced degradation of coastal resources in Asia Pacific and implications on management and food security. J. Nat. Studies 9(10), 13–28.
Mascaró M., Amaral-Ruiz M., Huipe-Zamora I., Martínez-Moreno G., Simões N., Rosas C. (2016). Thermal tolerance and phenotypic plasticity in juvenile hippocampus erectus perry 1810: Effect of acute and chronic exposure to contrasting temperatures. J. Exp. Mar. Biol. Ecol. 483, 112–119. doi: 10.1016/j.jembe.2016.07.005
Mascaró M., Horta J. L., Diaz F., Paschke K., Rosas C., Simões N. (2019). Effect of a gradually increasing temperature on the behavioural and physiological response of juvenile hippocampus erectus: Thermal preference, tolerance, energy balance and growth. J. Thermal Biol. 85, 102406.
McCarthy J. J., Canziani O. F., Leary N. A., Dokken D. J., White K. S. (Eds.) (2001). Climate change 2001: impacts, adaptation, and vulnerability: contribution of Working Group II to the third assessment report of the Intergovernmental Panel on Climate Change. (Vol. 2). Cambridge: Cambridge University Press.
Myrick C. A., Cech J. J. (2000). Swimming Performances of Four California Stream Fishes: Temperature Effects. Environmental Biology of Fishes 58(3), 289–295. doi: 10.1023/a:1007649931414
Ojanguren A. F., Brañta F. (2000). Thermal dependence of swimming endurance in juvenile brown trout. J. Fish Biol. 56 (6), 1342–1347. doi: 10.1111/j.1095-8649.2000.tb02147.x
Palma J., Bureau D. P., Andrade J. P. (2011). Effect of different artemia enrichments and feeding protocol for rearing juvenile long snout seahorse, hippocampus guttulatus. Aquaculture 318 (3), 439–443. doi: 10.1016/j.aquaculture.2011.05.035
Palma J., Stockdale J., Correia M., Andrade J. P. (2008). Growth and survival of adult long snout seahorse (Hippocampus guttulatus) using frozen diets. Aquaculture 278 (1), 55–59. doi: 10.1016/j.aquaculture.2008.03.019
Papoutsoglou S. E., Mylonakis G., Miliou H., Karakatsouli N. P., Chadio S. (2000). Effects of background color on growth performances and physiological responses of scaled carp (Cyprinus carpio l.) reared in a closed circulated system. Aquacult. Eng. 22 (4), 309–318. doi: 10.1016/S0144-8609(00)00056-X
Pereira R. J., Sasaki M. C., Burton R. S. (2017). Adaptation to a latitudinal thermal gradient within a widespread copepod species: the contributions of genetic divergence and phenotypic plasticity. Proc. R. Soc London Ser.B 284, 20170236. doi: 10.1098/rspb.2017.0236
Pilakouta N., Killen S. S., Kristjánsson B. K., Skúlason S., Lindström J., Metcalfe N. B., et al. (2020). Multigenerational exposure to elevated temperatures leads to a reduction in standard metabolic rate in the wild. Funct. Ecol. 34 (6), 1205–1214. doi: 10.1111/1365-2435.13538
Planas M., Blanco A., Chamorro A., Valladares S., Pintado J. (2012). Temperature-induced changes of growth and survival in the early development of the seahorse hippocampus guttulatus. J. Exp. Mar. Biol. Ecol. 438, 154–162. doi: 10.1016/j.jembe.2012.10.003
Pollom R. (2017). Hippocampus guttulatus. The IUCN Red List of Threatened Species 2017: e.T41006A67617766. doi: 10.2305/IUCN.UK.2017-3.RLTS.T41006A67617766.en
Pörtner H. O., Farrell A. P. (2008). Physiology and climate change. Science 322 (5902), 690–692. doi: 10.1126/science.1163156
Qin G., Johnson C., Zhang Y., Zhang H., Yin J., Miller G., et al. (2018). Temperature-induced physiological stress and reproductive characteristics of the migratory seahorse hippocampus erectus during a thermal stress simulation. Biol. Open 7 (6), bio032888. doi: 10.1242/bio.032888
Rahman M. I. (2013). Climate change: a theoretical review. Interdiscip. Description Complex Syst. 11 (1), 1–13. doi: 10.7906/indecs.11.1.1
Rangel R. E., Johnson D. W. (2018). Metabolic responses to temperature in a sedentary reef fish, the bluebanded goby (Lythrypnus dalli, Gilbert). J. Exp. Mar. Biol. Ecol. 501, 83–89. doi: 10.1016/j.jembe.2018.01.011
Roessig J. M., Woodley C. M., Cech J. J., Hansen L. J. (2004). Effects of global climate change on marine and estuarine fishes and fisheries. Rev. Fish Biol. Fish. 14 (2), 251–275. doi: 10.1007/s11160-004-6749-0
Sabine C. L., Feely R. A., Gruber N., Key R. M., Lee K., Bullister J. L., et al. (2004). The Oceanic Sink for Anthropogenic CO 2. Science 305 (5682), 367–371. doi: 10.1126/science.1097403
Sampaio E., Santos C., Rosa I. C., Ferreira V., Pörtner H.-O., Duarte C. M., et al. (2021). Impacts of hypoxic events surpass those of future ocean warming and acidification. Nat. Ecol. Evol. 5 (3), 311–321. doi: 10.1038/s41559-020-01370-3
Servili A., Canario A. V. M., Mouchel O., Muñoz-Cueto J. A. (2020). Climate change impacts on fish reproduction are mediated at multiple levels of the brain-pituitary-gonad axis. Gen. Comp. Endocrinol. 291, 113439. doi: 10.1016/j.ygcen.2020.113439
Shahidul Islam Md., Tanaka M. (2004). Impacts of pollution on coastal and marine ecosystems including coastal and marine fisheries and approach for management: a review and synthesis. Mar. pollut. Bull. 48 (7), 624–649. doi: 10.1016/j.marpolbul.2003.12.004
Simon Y., Levavi-Sivan B., Cahaner A., Hulata G., Antler A., Rozenfeld L., et al. (2017). A behavioural sensor for fish stress. Aquacult. Eng. 77, 107–111. doi: 10.1016/j.aquaeng.2017.04.001
Sopinka N. M., Donaldson M. R., O’Connor C. M., Suski C. D., Cooke S. J. (2016). Stress indicators in fish. In Fish physiology (Elsevier Inc) 35, 405–462. doi: 10.1016/B978-0-12-802728-8.00011-4
Sswat M., Stiasny M. H., Taucher J., Algueró-Muñiz M., Bach L. T., Jutfelt F., et al. (2018). Food web changes under ocean acidification promote herring larvae survival /631/158/2165 /631/158/2446 article. Nat. Ecol. Evol. 2 (5), 836–840. doi: 10.1038/s41559-018-0514-6
Tamazouzt L., Chatain B., Fontaine P. (2000). Tank wall colour and light level affect growth and survival of Eurasian perch larvae (Perca fluviatilis l.). Aquaculture 182 (1), 85–90. doi: 10.1016/S0044-8486(99)00244-6
Teixeira R., Musick J. A. (2001). Reproduction and food habits of the lined seahorse, Hippocampus erectus (Teleostei: Syngnathidae) of Chesapeake Bay, Virginia. Revista Brasileira De Biologia 61 (1), 79–90. doi: 10.1590/s0034-71082001000100011
Vincent A. C. J. (1995). A role for daily greetings in maintaining seahorse pair bonds. Anim. Behav. 49, 258–260. doi: 10.1016/0003-3472(95)80178-2
Vincent A. C. J., Foster S. J., Koldewey H. J. (2011). Conservation and management of seahorses and other syngnathidae. J. Fish Biol. 78 (6), 1681–1724. doi: 10.1111/j.1095-8649.2011.03003.x
Volkoff H., Rønnestad I. (2020). Effects of temperature on feeding and digestive processes in fish. Temperature 7 (4), 307–320. doi: 10.1080/23328940.2020.1765950
Walther G.-R., Post E., Convey P., Menzel A., Parmesan C., Beebee T. J. C., et al. (2002). Ecological responses to recent climate change. Nature 416 (6879), 389–395. doi: 10.1038/416389a
Wong B. B. M., Candolin U. (2015). Behavioral responses to changing environments. Behavioral Ecology 26 (3), 665–673. doi: 10.1093/beheco/aru183
Woodall L. (2017). Hippocampus hippocampus. The IUCN Red List of Threatened Species 2017: e.T10069A67618259. doi: 10.2305/IUCN.UK.2017-3.RLTS.T10069A67618259.en
Woodall L. C., Koldewey H. J., Boehm J. T., Shaw P. E. (2015). Past and present drivers of population structure in a small coastal fish, the European long snouted seahorse Hippocampus guttulatus. Conservation Genetics 16 (5), 1139–1153. doi: 10.1007/s10592-015-0728-y
Woodall L. C., Otero-Ferrer F., Correia M., Curtis J. M. R., Garrick-Maidment N., Shaw P. W., et al. (2018). A synthesis of European seahorse taxonomy, population structure, and habitat use as a basis for assessment, monitoring and conservation. Mar. Biol. 165 (1), 1–19. doi: 10.1007/s00227-017-3274-y
Keywords: activity, feed intake, climate change, syngnathidae, body condition
Citation: Costa AB, Correia M, Silva G, Lopes AF and Faria AM (2023) Performance of the long-snouted seahorse, Hippocampus guttulatus, under warming conditions. Front. Mar. Sci. 10:1136748. doi: 10.3389/fmars.2023.1136748
Received: 03 January 2023; Accepted: 08 March 2023;
Published: 22 March 2023.
Edited by:
Miquel Planas, Institute of Marine Research (CSIC), SpainReviewed by:
Carlos Rosas, National Autonomous University of Mexico, MexicoMaite Mascaro, National Autonomous University of Mexico, Mexico
Copyright © 2023 Costa, Correia, Silva, Lopes and Faria. This is an open-access article distributed under the terms of the Creative Commons Attribution License (CC BY). The use, distribution or reproduction in other forums is permitted, provided the original author(s) and the copyright owner(s) are credited and that the original publication in this journal is cited, in accordance with accepted academic practice. No use, distribution or reproduction is permitted which does not comply with these terms.
*Correspondence: Ana Margarida Faria, YWZhcmlhQGlzcGEucHQ=