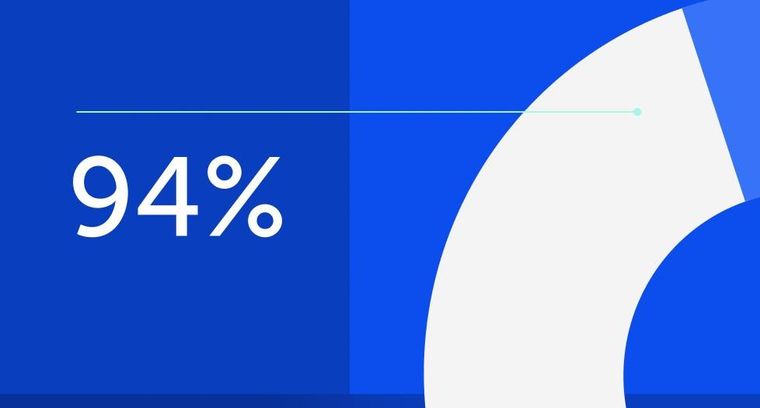
94% of researchers rate our articles as excellent or good
Learn more about the work of our research integrity team to safeguard the quality of each article we publish.
Find out more
ORIGINAL RESEARCH article
Front. Mar. Sci., 19 April 2023
Sec. Marine Fisheries, Aquaculture and Living Resources
Volume 10 - 2023 | https://doi.org/10.3389/fmars.2023.1135417
This article is part of the Research TopicIntegration of Sustainability, Preservation of Biodiversity and Conservation Goals in AquacultureView all 8 articles
Successful bottom planting of indigenous macroalgae Saccharina latissima aimed at coastal restoration purposes require the mass production in controlled conditions of strongly fixed, healthy sporophytes followed by optimal transfer techniques in order to ensure viability and vigor of the young seedlings about to be directly introduced in the coastal environment. Early development of S. latissima submitted to different combinations of substrate type (natural vs artificial brick-shaped substrate), gametophyte spraying method (water-based vs binder-based) and water velocity (0.1 vs 0.2 m s-1) was evaluated during a growth trial that lasted 42 days. Overall, all experimental groups (8 in triplicate) reached the targeted length of 15 mm between 35-42 days post-seeding. No strong indications that the proposed 2×2×2 factorial design generated long lasting effects on growth and development indicators were observed (thallus length, SGR and % coverage). The observation of no persistent difference in the growth response of S. latissima under all experimental conditions, demonstrates that it is well suited for mass production of seedlings. Our results and evidenced-based practices led us to conclude that the use of an artificial substrate in combination with a binder-based gametophyte pulverization and the application of a velocity 0.2 m s-1 during early-growth could be adopted in a standardized protocol. We argue that 1) artificial substrates (uniform shape, stackable and rough surface) will most likely allow better use of a vessel’s open deck space and adherence of the developing holdfast; 2) the use of a binder may slow down the dehydration of the propagules and promote adhesiveness to the substrate during rearing, handling and transfer operations and under varying flow rates or wave actions respectively and 3) highest velocity should promote the selection of propagules with strongest attachment and thus possibly limit post-transfer dislodgement. We suggest further studies should 1) focus on identifying optimal gametophyte concentration at the spraying step, in order to reduce production costs and maximise productivity of seedling operations and 2) include biomass determination (g of tissue per cm2) in combination to the semi-quantitative density evaluation (% coverage) based on image-analysis, in order to improve our global assessment of growth.
The coasts of the St-Lawrence estuary, the Magdalen Islands and the Baie-des-Chaleurs region in Quebec, Canada, are among the areas of the world most affected by coastal erosion due to climate change (Slangen et al., 2014). Rise in sea level, more frequent storms, and decrease or absence of ice cover during the winter season leaves these coasts increasingly vulnerable to wave and tidal actions. Coastal erosion is already well under way and many fragile sandy environments are at risk of being severely eroded (Lajoie et al., 2007; Bernatchez et al., 2008; Raby et al., 2008; Bernatchez and Quintin, 2016). To protect coastal communities and infrastructures, several engineering solutions have been applied around the world. In Québec (Canada), depending on the location, recent restoration and erosion control efforts have mainly focused on high investment techniques like retaining wall, riprap, beach lowering, groyne, beach fill with dune reconstruction and revegetation (Aubé, 2020). However, engineering solutions are increasingly perceived as being ecologically and economically unsustainable due to the inescapable infrastructure maintenance needs over time (Hinkel et al., 2014). More natural integrated and holistic approaches inspired by conservation aquaculture techniques (eco-engineering) with proven efficacy to create or restore disrupted natural habitat are warranted, in place or in complement to artificial structures (Bouma et al., 2014; Narayan et al., 2016; Morris et al., 2017). Benefits of nature-based solutions are that these systems can adapt to changes in climate and self-repair after major storm events (Gittman et al., 2014). In return, additional ecological benefits in the selected habitats include biodiversity enhancement (Temmerman et al., 2013).
Kelp forests can play a vital role by mitigating wave’s intensity before they reach the coastline and by helping trap sediment on the beach (Hynes et al., 2021). As discussed in Christie et al. (2003), kelp forest not only mitigates the effect of climate change, but grows into a rich and productive ecosystem that promotes coastal biodiversity. Accordingly, kelp forests are sometimes called blue green infrastructure (Deely and Hynes, 2020), and their ecological services have been estimated to millions of dollars for each kilometer of coastline (Eger et al., 2022). A downward trend in the number of kelp forests in some regions of the world has already occurred (Hynes et al., 2021). In Canada, British-Columbia and on a lesser scale Nova Scotia and Québec, kelp restoration projects, in response to the foreseen impacts of climate changes and observed general declines, were evaluated (Merzouk and Johnson, 2011; Tamigneaux and Johnson, 2016; Burek et al., 2018; Eger et al., 2022). In Québec (Canada), algal assemblage of the subtidal zone is dominated by Laminaria species (Alaria, Saccharina and Agarum species) and contrary to many similar coastal ecosystems, spatial expansion is largely dictated by ice scouring and uncontrolled grazing by the sea urchin (Merzouk and Johnson, 2011; Tamigneaux and Johnson, 2016). The Gulf of St-Lawrence is a semi-enclosed sea, covering an area of 226 000 km2 also identified as a biogeographical boundary between subarctic and boreal waters and inclined to be the stage of large-scale environmental changes brought about by climate changes that will be affecting distribution of kelp beds significantly (Merzouk and Johnson, 2011).
Different techniques for seeding of kelps in the wild have been developed and used for afforestation or restoration of kelp forests ecosystems (Eger et al., 2022). Bottom planting techniques include seeding kelp populations by settling mesh bags filled with fertile kelp blades on the seafloor (see review by Westermeier et al., 2014) or adding artificial reefs by introducing different new material to the seabed, more recently material that is similar to concrete (Bishop et al., 2017). Transplanting of adult or juvenile kelps (Falace et al., 2006; Fredriksen et al., 2020) are also shown to be applicable and one of the most successful seaweed restoration programs was initiated by the experimental transplanting of adult crayweed (Phyllospora comosa), a brown macroalgae, in the Sydney Harbour area in Australia (Campbell et al., 2014). Formerly extinct from the area, these efforts gave rise to a complete recovery of the local population within a single generation. However, transplanting adult kelps to the seafloor remains financially limiting for large-scale projects (Eger et al., 2022). More recently, green gravel technique was used along the southern coast of Norway to restore kelp forest with confirmed success. Spores of S. latissima were fixed on small rocks (⌀ 2-5 cm) and cultivated in tanks until they reached 2-3 cm of length. Rocks were then simply dropped into the sea from the surface to 3-7 m depths allowing the kelp to develop over 9 months with a high survival rate and evidence that the gravel remained where delivered (Fredriksen et al., 2020). Green gravel (a bottom planting approach) as a potential vector of dispersal for kelp restoration, was experimentally evaluated by Alsuwaiyan et al. (2022).
Our study was part of a large-scale nature-based solution program to counter coastal erosion and contribute to the restoration of natural kelp populations in various coastal regions of Québec, Canada. The project, supported by the Coastal Restoration Fund (Fisheries and Oceans, Canada), proposed the implementation of an experimental kelp seeding program in the natural environment as a coastal restoration strategy, based on the cultured production and introduction of seedlings of indigenous kelp species fixed on brick-sized rocky substrates in preselected areas at sea. The proposed approach also provides an ecosystemic enhancement measure to increase the representativeness of marine exhibits on display in large aquariums (Le François et al., 2015; Tremblay-Gratton et al., 2018) mainly by the creation of microhabitats and display of natural behaviors such as feeding, reproduction and hiding.
Based on different attributes, considered indigenous kelp species for our trials were: Saccharina latissima (Laminariaceae), Saccorhiza dermatodea, Agarum clathratum (Phaeophyceae) and Alaria esculenta (Alariaceae). This study focused on the production of the sugar kelp, Saccharina latissimi based on: 1) the availability of well-developed and simple culture protocols (Tamigneaux et al., 2014; Kerrison et al., 2020; Solvang et al., 2021); 2) their predominance in the areas of Québec targeted for coastal restoration efforts (Tamigneaux and Johnson, 2016); 3) high growth rate (Reid et al., 2013); 4) well developed holdfast securing anchoring (Kerrison et al., 2019); 5) strong potential as a candidate species for aquaculture diversification in the North Atlantic (Tamigneaux and Johnson, 2016; Forbord et al., 2018; Visch et al., 2020) and 6) provision of substantial ecosystem services (Hasselström et al., 2018; Grebe et al., 2019; Boderskov et al., 2021; Picard et al., 2022).
Sugar kelp is generally found on rocky substrates and distributed intermittently on the North East coast and West coast of the Atlantic Ocean as well as in the northern Pacific Ocean at average depths between 8-30 meters (Brijesh and Troy, 2015). This seaweed has a heteromorphic life cycle that alternates between a microscopic gametophyte phase and a macroscopic sporophyte phase. The sporophyte releases spores that grow into male and female gametophytes that in turn will produce gametes. After fertilization, the resulting embryonic sporophyte will settle on a substrate and grow into a sporophyte (Pearson et al., 2019). S. latissima grows best at 10-15°C and salinities ranging from 23 and up to 31 PSU (Werner et al., 2003 in Bruton et al., 2009; Peteiro and Freire, 2013).
Water velocity is known to influence and promote growth and biomass yield of Laminaria by reducing the size of the diffusion boundary layer at the blade surface thus facilitating the molecular diffusion exchange process and nutrient uptake (Hurd, 2000; Hepburn et al., 2007). In a field-based study Peteiro and Freire (2013) demonstrated that a water velocity up to 0.3 m s-1 resulted in a higher biomass yield (16 kg per meter of ropes) of S. latissima 2 mm seedlings, compared to a less exposed natural site with less than ≤ 0.1 m s-1 (12 kg per meter of ropes). In a laboratory-based study, Peteiro et al. (2019) designed an experiment to find the optimal water velocity for growth of Undaria pinnatifida on strings, from 2 to 8.5 mm seedlings, and found that optimal water velocity lied between 0.158-0.171 m s-1 beyond which growth rate declined. Based on this, we hypothesized that water velocity could also affect the early growth of the seedlings.
Sugar kelp seeding methods were first developed to meet the requirements and constraints of the aquaculture sector. Spores or gametophytes are usually seeded on ropes and then cultured in a controlled environment weeks before deployment at sea or, more recently, directly seeded with a binder just before deployment based on the premise that the high viscosity of the product would prevent the sporophytes from being washed away before the holdfast would attach to gametophyte-seeded twine or ropes (Forbord et al., 2020; Fredriksen et al., 2020; Visch et al., 2023) and that it could potentially protect the gametophytes from dehydration during transfer operations (Lemoine and Lemaire, 2019). This method could potentially decrease the time and cost associated with culture of sporophytes in controlled environments. To our knowledge, the efficiency of binders to promote attachment of gametophytes to rocky substrates has not yet been tested in the sugar kelp production cycle. Binder use was however studied for seeding of juvenile sporophytes of S. latissima into textile and twine substrates (≤ 1mm sporophytes: Kerrison et al., 2018; 2020) with contrasting results. Furthermore, in comparison to a natural rocky substrate, an artificial substrate that is evenly shaped and stackable should reduce storage footprint on small size workboats in comparison to natural shaped substrate. Surface roughness can be modified on the former to provide a better surface/texture for gametophyte fixation and for the development of the holdfast and the persistence of young seedlings in real-life conditions (Kerrison et al., 2019). To our knowledge, this is the first study to evaluate brick-shaped artificial substrate for kelp restoration and erosion defense purposes.
The objective of our study was to evaluate the effect and interactions of gametophyte spraying method (with and without binder), flow velocity (moderate = 0.1 m s-1 and strong = 0.2 m s-1) and substrate (natural and artificial) on the early growth of S. latissima sporophytes (target length of 15 mm) under predetermined conditions of light intensity, spectral composition, photoperiod, temperature, pH, salinity and nutrients. Our results should contribute to the development of optimized production and transfer protocols aimed primarily at large-scale natural population restoration activities at sea that could improve defense against erosion locally.
Mature individuals of S. latissima with visible sori were collected by divers at 5-10 meters depths in the fall 2017 on the coastline of Grande-Rivière, QC, Canada (Pointe Verte, Anse du Loup 48°23’26”N 64°29’20”W). Gametophytes were obtained according to a production protocol aimed at providing seedlings for commercial production of kelp at sea (Tamigneaux et al., 2013). More precisely, the sori tissues of the kelps were cut and brushed with brown paper to remove epiphytes. The sori samples were then disinfected in three successive baths of autoclaved seawater at 30 mg L-1 sodium hypochlorite for 2 minutes, 1 mg L-1 germanium oxide (GeO2) for 2 minutes and lastly 1 mg L-1 GeO for another 2 minutes. Following disinfection, the sori were brushed again with brown paper to remove the excess water and placed between two layers of laboratory brown paper towel in the dark at 10°C for 17 hours. Then they were rehydrated in filtered autoclaved seawater with mechanical agitation to induce spore release and observed with an optical microscope in search of mobile spores (method inspired by Pang and Lüning, 2004). After filtering the liquid twice at 250 µm, once at 100 µm and twice at 60 µm to remove debris and colloids that were released with the spores, the filtrate containing the spores was poured in 2 L round bottom flasks containing 1.5 L autoclaved seawater with 2 ml L-1 Miquel A, 1 ml L-1 Miquel B, 0.2 ml L-1 Fritz Aquatics Part A and B each (F/2 Algae Food, Fritz Aquatics, TX, USA) and 1 mg L-1 of GeO2. Gametophyte production was induced by controlling temperature and light regime. The gametophyte solutions were kept at 10 °C under constant red fluorescent light (20 µmol m-2 s -1) to maintain them in an immature vegetative state until they were sprayed on experimental substrates. When the first germination tubes were visible, aeration (filtered at 0.45 µm) was added to the culture flasks using small aquarium air pumps (Marina). The nutrient solution (Fritz Aquatics Part A and B at 0.2 ml L-1 each) was replenished every two weeks and GeO2 (1 mg L-1) was added only when diatoms were observed.
Artificial substrates produced under the supervision of CERMIN (Centre de recherche sur les milieux insulaires et maritimes) were made of dredged-sand and cement in a brick-shape of approximately 25 x 16 x 7 cm with a textured surface of 400 cm2. In addition, three 0.5 cm groves were shaped on the exposed top surface of each brick to add relief/irregularities to the otherwise flat and rough surface generated by the fabrication process (equal depth and distance in-between). Natural substrates were natural rocks of variable dimensions and surfaces (181-431 cm2) with a smooth surface. All substrates were cleaned with an ecological neutral quaternary ammonium disinfectant cleaner (EKO-QUAT, DIN: 02423391, Lalema inc., Montreal, QC, Canada) then rinsed with fresh water at least three times and kept at 10°C for 3 days preceding gametophyte spraying operations. Experimental tanks were also cleaned using the same product, then rinsed at least three times with fresh water. A 48-hour soaking period with fresh water and the addition of bleach (NaClO) (6%) was made in order to clean the filtration system pipes (biofilters, UV filters, coolers). The tanks were rinsed with plenty of water 24 hours before the beginning of the trials. Artificial seawater (Crystal Sea Bioassay formula, Marine Enterprise inc., Baltimore, MD, USA) was produced to reach 27.5 PSU that resulted in the pH range of pH 8.0.
Ten days before spraying gametophyte cultures on the substrates, they were fragmented with a mixer at maximum speed for one minute to help trigger gametogenesis, increase the quantity of oocytes and prevent the filaments from blocking the sprayer. In between uses the mixer was washed with soap and disinfected with chlorine (200 ppm for one hour), rinsed and dried. The detachable metallic pieces were autoclaved (121°C for 30 min). Once fragmented, the gametophyte cultures were placed in a culture chamber at 10°C, illuminated under white light: 40 μmol photons m-2 s-1, 10:14 (L:D) for 10 days. Gametophyte cultures were then filtered with a nylon mesh filter screen (Nitex)(Dynamic Aqua Supply Ltd., Canada, Product no. NTX60) (60 µm × 60µm pore size) and the underside of the filter was patted with a paper towel to remove excess water before weighing.
One natural seawater solution and one natural seawater solution with the addition of binder AlgaeBinder (AtSeaNova inc., Ronse, Belgiuum) was prepared. The binder solution consisted of 2.5g of binder powder mixed with a cement mixer in 500 ml of seawater. In both solutions, gametophytes with visible oocytes were added at a concentration of 5.6 ×10-3 g·cm-2 equivalent to 2 g in total for artificial substrates and 1 to 2 g for natural substrates depending on their total surface (Lemoine and Lemaire, 2019). Gametophytes were sprayed evenly on substrates June 5, 2018, the first day of the trial at the laboratory of the Biodôme de Montréal (Montreal, QC, Canada) (Figure 1). A pressurized spray bottle system was used and the substrates were kept at 10°C for 10 minutes to allow them to dry before their direct transfer into the tanks.
Figure 1 Natural rock sprayed with water (A) and with binder (B), artificial rock sprayed with water (C) and with binder (D) prior to trials.
Six polyethylene tanks (135 L) were operated as independent closed systems, each equipped with canister filters (Fluval 306) and a UV unit (Turbo-Twist. 6x, 18 Watt) connected to a water chiller (1/2 HP). The experiment was conducted following a split-split plot design with water velocity (0.1 and 0.2 m s-1) as main plot (A), substrate (natural and artificial) as subplot (B) and fixation method for gametophyte (seawater with and without the binder/binder) as sub-subplot C for a total of 8 treatments. Each tank featured one water velocity in which substrate and fixation methods combinations were randomly distributed. The experiment was conducted in triplicates (see Figure 2 experimental design). Once a week, one third of the water of each tank was renewed with fresh artificial seawater (as previously detailed), followed by an addition of nutrient solution in each tank with 0.2 ml L-1 Fritz Aquatics Part A and B. The addition of 1 ml of a stock solution of GeO2 at 1 g L-1 was done at the beginning of the experiment to reduce the risk of diatom growth (Shea and Chopin, 2007; Steinhagen et al., 2022). The experiment was carried out for 42 days post gametophyte seeding. A slight and short-lived variability of unknown cause affecting the culture parameters (temperature, pH and salinity) was observed among tanks between days 35-42. As it remained in the optimal threshold developmental stages of the species (pH 7.6 - 8; Redmond, 2013; 26-29 ppm, 8-15°C; Monteiro et al., 2021) data was treated and presented as previously described.
Figure 2 Experimental design during the trials where (A) is either natural (A1) or artificial (A2) substrate; (B) is moderate 0.1 m s-1 (B1) or elevated 0.2 m s-1 (B2) water velocity and (C) is a spraying technique with (C1) or without (C2) binder. Both levels of each factor are distributed randomly amongst the 6 tanks and are represented in each block, n = 3.
Each tank had two circulation pumps (Turbelle Nanostream 6095, Tunze Aquarientechnik GMBH, Penzberg, Germany) installed at mid-height in opposite inner corners of the tank which maintained a continuous and circular flow. Pumps were started on day 2 of the experiment. For moderate and strong water velocity treatments, pumps were maintained at a horizontal water velocity of 0.05 and 0.1 m s-1 until day 4 and then adjusted to 0.1 and 0.2 m-1 respectively. Horizontal water velocity was measured each day, 1 cm above the center of each substrate, with an electromagnetic propeller anemometer (MiniWater20) combined with a MC20 velocity sensor (22 mm internal diameter and 0.03-10 m s-1 sensitivity range) (Schiltknecht, Switzerland).
Photoperiod was 12:12 (L:D) supplied with LED lamps (Hydra 26 HD Series, Aqua Illumination inc., Bethlehem, PA, USA), suspended above each tank. Photosynthetically active radiation (PAR) light intensity was measured at the center of the bottom of the tanks with a light meter and an underwater quantum sensor (LI-250A and LI-192SA, LI-COR inc. NE, USA) and adjusted once a week with increasing PAR irradiance mean value of 60, 100, 150, 175 μmol photons m−2 s−1 at week 1, 2, 3, 4 and 200 μmol photons m−2 s−1 at week 5 and 6 respectively. Light spectrum included royal blue, blue, green and white light (Table 1).
Table 1 Percentage of each light spectrum (%) that was programmed for the LED lamps and total PAR irradiance (μmol photons m−2 s−1) that was recorded at the bottom of the tanks.
Experimental temperature until day 42, i.e 10°C (9.9 ± 0.4°C) was monitored four times per day with a mercury thermometer. The pH was automatically monitored and adjusted every 10 minutes with an aquarium controller (Profilux Aquatic Bus 4, GHL, Germany) and the mean value was 8.01 ± 0.1 until day 42. This module was connected to six pH electrodes (Aquatronica, ACQ310N-PH), whose base soaked into the water of each tank. An acid solution, H2SO4 [0.1 mol L-1], or a base solution, NaOH [0.2 mol L-1], was injected as needed via a peristaltic pump in each tank. No CO2 injection was performed during the trials. Salinity during the trials was maintained between 26 and 28 PSU and had a mean value of 27.29 ± 0.24 ppm.
Weekly percentage of coverage (also referred to density) and thallus lengths were analysed at a two-day interval. For density analysis, pictures of each substrate were taken on days (D) 16, 23 and 37 at a fixed distance with an immersible GoPro camera (Hero 4, San Mateo, CA, USA). The images were processed and analyzed with the ImageJ software (2013 version). Pixel threshold range was manually set to segment grayscale (8-bit type) images into entities of interest. The background was removed from the image to preserve the substrate area for analysis using the Elliptical Selection plugin. Then, minimum size and maximum pixel area were set to automatically calculate the percentage of seedling coverage on each segmented image with the Analyze Particles plugin.
For analysis of thallus lengths, seedlings from three different areas of each substrate were randomly sampled with a transfer pipette weekly starting from day 14 as the sporophytes stage was initiated. Thirty fronds randomly chosen were measured for each substrate, each week (D14, D21, D28, D35 and D42). Thallus were measured the same day of collection or kept in 50 ml Eppendorfs in a thermostatic chamber at 10°C away from light, to be measured the next day. Length measurements were made using a stereomicroscope (MEIJI Techno, Japan) at ×64 magnification, paired with a camera (Moticam 5+, 5.0 MP and Motic Image Plus software).
Specific growth rate (SGR) was calculated at different periods during the growth trials using the following formula, from Kim et al. (2007) and Yong et al. (2013).
Where L0 and Lt (µm) are the length at initial and final days during a culture period (t = days), respectively.
All statistical analyses were performed with R (version 3.5.2, CRAN, Vienna Austria) statistical software. Data are expressed as means ± standard deviations (SD). Normal distribution and homoscedasticity of density and seedling length were visually assessed and verified with a Shapiro Wilk’s test, and data on density at day 37 were transformed with the box cox function (lambda = 0.8) of the MASS package (Venables and Ripley, 2002). Transformed data were analyzed but original data are presented. The effect of the interactions between independent variables on seedling length and density was analyzed in a mixed model with random block factor (n = 3) and fixed factors substrate (A), water velocity (B), and fixation method (C) at each day as per Gomez and Gomez (1984) with the aov function of the dplyr package (Wickham et al., 2022). Tukey’s Honest Significance Test (HSD) was used as a post hoc multiple comparison test and degrees of freedom were adjusted with the Kenward-Roger method.
On D16, the statistical model detected a significant interaction between water velocity and the fixation method (p = 0.0490). Although the post hoc test then revealed no significant interaction between those factors, combinations of the use of a binder and higher velocity, and water fixation method with moderate water velocity yielded respectively 1.5-fold (49.7 ± 26.1%, p > 0.05), and 1.9-fold (63.4 ± 18.1%, p = 0.069) greater densities compared to the use of a binder in a moderate water velocity (32.9 ± 22.2%) (Figure 3A). At D23, there was a significant interaction between all three factors on thallus density (p = 0.0103). When artificial substrate was used and water velocity was moderate, the water fixation method (A2B1C1) yielded a significantly higher mean density than with the addition of a binder (A2B1C2) (74.00 ± 16.42% vs. 39.65 ± 20.87% respectively, p = 0.0334) (Figure 3B). These treatments also have the highest and the smallest density values respectively (Figure 4). A significant effect of substrate was observed at D37 (p = 0.0085), but post hoc tests revealed no significant differences between groups and mean density was 95.17 ± 8.44% (Figure 3C) on the final day of density evaluation.
Figure 3 Seedling density (%) of S. latissima (means ± SD) on day 16 (A), 23 (B) and 37 (C) cultured on different substrate (A1: Natural; A2: Artificial), water velocity (B1: 0.1 m s-1; B2: 0.2 m s-1) and spraying technique (C1: water; C2: water+binder); bars with different letters are significantly different (p< 0.05), n = 3.
Figure 4 Combination of factors on day 23 yielding highest density value (74.00 ± 16.42%, artificial substrate, moderate velocity, water spray, or A2B1C1) (A) and smallest density value (39.65 ± 20.87%, artificial substrate, moderate velocity, binder spray, or A2B1C2) (B).
On D14, a significant interaction of water velocity and fixation method on thallus length was observed (p = 0.042), as well as substrate and spraying technique (p< 0.001). Specifically, water velocity did not affect thallus length when combined with the use of a binder (mean of 127.81 ± 28.67 µm), but did when combined with water spray and yielded significantly higher thallus lengths with elevated water velocity (B2C1, 163.02 ± 54.85 µm, p = 0.007) compared to medium water velocity (B1C1, 145.80 ± 41.55 µm) (Figure 5A). Water spray yielded a greater seedling length than binder spray when combined with natural substrate (154.9 ± 47.9 vs. 132.8 ± 55.4 µm respectively, p<0.001) and artificial substrates (153.9 ± 56.9 vs. 124.3 ± 47.1 µm respectively, p< 0.001) (Figure 5B).
Figure 5 Seedlings length (µm) of S. latissima (means ± SD) on day 14 according to the combination of spraying technique (water vs. binder) with water velocity (0.1 m s-1 vs. 0.2 m s-1) (A), and with substrate (natural vs artificial) (B); bars with different letters are significantly different (p< 0.05), n = 6.
At D21, there was a significant interaction between all three factors on thallus length (p< 0.001). Stronger velocity in general tends to result in longer thallus, as the mean thallus length was between 825.77 and 947.63 µm with strong velocity and between 743.18 and 821.81 µm with medium velocity. However, statistical analysis is more nuanced as the tendency is only accurate for a few combinations of treatments. For example, the combination of artificial substrate\binder yielded longer thallus when exposed to high water velocity (946.24 ± 279.04 µm) than when exposed to medium water velocity (743.18 ± 232.35 µm, p< 0.001). The combination of natural substrate\water spray also gave significantly higher thallus length when combined with strong water velocity (947.63 ± 309.85 µm; highest length value) then with medium water velocity (746.81 ± 201.26 µm, p = 0.001) (Table 2).
Table 2 Seedlings length (µm) of S. latissima (means ± SD) according to substrate (Natural or Artificial, A), water velocity (0.1 m s-1 or 0.2 m s-1, B), and spraying technique (water vs. binder, C) at day 14, 21, 28 and 35 of the experiment.
At D28, a significant interaction of water velocity and substrate on the thallus length was observed (p = 0.017). Water velocity had a significant effect on the length of the thallus cultured on artificial substrate where they were significantly longer under strong velocity (2437.85 ± 577.36 µm, p< 0.001) then under moderate velocity (2193.67 ± 446.56 µm) (p = 0.0004) (Figure 6). Also, the combination of artificial substrate with moderate velocity (2193.67 ± 446.56µm) yielded significantly smaller thallus length compared with natural substrate and higher velocity (2365.24 ± 559.22µm) at day 28 (p = 0.023). All three factors did not have a significant impact on the thallus length at D35. The average thallus length was 4821.65 ± 1472.53 µm (p > 0.05).
Figure 6 Seedlings length (µm) of S. latissima (means ± SD) on day 28 according to the substrate (natural vs. artificial) and water velocity (0.1 m s-1 vs. 0.2 m s-1); bars with different letters are significantly different (p< 0.05), n = 6.
On the final day (D42), two pairwise interactions between water velocity and substrate (p< 0.001), and water velocity and fixation method (p = 0.020) displayed significant effects on thallus length. Post hoc analysis revealed that significantly longer fronds were obtained on natural substrate with moderate water velocity (A1B1, 18 938.89 ± 6 179.31µm) compared to elevated water velocity (A1B2, 16 355.56 ± 5 506.24µm) (p< 0.0001), and when a binder was used with moderate (B1C2, 18 166.67 ± 5 674.54µm) compared to elevated (B2C2, 16 000 ± 5 289.49µm) water velocity (p = 0.001). The correlation between densities and frond lengths within each group was evaluated but remained insignificant (p > 0.05).
Specific growth rates (SGR) in terms of daily percentage growth of fronds were first evaluated every 7 days after day 14 (D14-21, D21-28, D28-35, D35-42). Main factors seemed to only affect growth during the first (D14-21) and last (D35-42)7 days where interactions of all three parameters (p=0.013) and of water velocity and substrate (p = 0.028) were detected at both periods respectively. For D14-21, post hoc tests failed to identify significant differences between groups. For final SGR values (D35-42), moderate water velocity and natural substrate yielded significantly greater growth (A1B1, average, 21.1 ± 0.03% day-1) compared to groups reared on artificial substrate in both elevated (A2B2, average, 17.0 ± 0.02% day-1, p = 0.003) and moderate (A2B1, average, 16.9 ± 0.02% day-1, p=0.003) water velocities (Figure 7). Average SGR D14-21, D-21-28, D28-35 and D35-42 were 25.27 ± 2.84, 14.99 ± 3.34, 10.32 ± 2.31, and 18.04 ± 2.66% day-1. To further evaluate the effect of the factors on frond growth, SGR were calculated for the period where growth appeared more pronounced; D14-35. During the D14-35 period, fixation method had a significant effect (p = 0.007) on the growth of S. latissima and specific growth rates were significantly higher with the binder (C2, 17.46 ± 0.91% per day) than without (C1, 16.30 ± 0.96% per day) (Figure 8). Growth appeared somewhat steady throughout the experiment as SGR measured at both periods displayed similar values (D14-D35: 16.9 ± 0.01, days 35-42: 17.9 ± 0.03). No effect of all factors was detected on overall growth measured between D14 and D42.
Figure 7 Specific growth rate (SGR, % day-1) of S. latissima between days 35 and 42 according to the interaction between substrate (artificial or natural) and water velocities (0.1 or 0.2 m s-1); bars with different letters are significantly different (p< 0.05), n = 6.
Figure 8 Specific growth rate (SGR, % day-1) of S. latissima between days 14 and 35 according to the fixation method; bars with different letters are significantly different (p< 0.05), n = 3.
The addition of the binder at the gametophyte stage promoted in our study proved efficient to support the attachment of the propagules when at their most vulnerable stage. The binder product was first added to the gametophyte solution to secure the rapid transfer at sea and the development of the holdfast in order to prevent dislodgement following transfer operations. Results showed that early-on, at day 14, the use of the binder resulted in a 1.9-fold smaller seedling density (or % coverage) on both substrates. The binder may have prevented an extension of the seedlings’ coverage on the substrate because they were more strongly fixed to their original point. Later on, the elevated water velocity promoted a 1.5-fold higher density coverage than the moderate velocity treatment. Overall, the presence of the binder did not induce significant differences in density, but its use is still recommended given our needs of fast deployment at sea and the possible prevention, according to Lemoine and Lemaire (2019) of gametophyte desiccation between the time gametophytes are sprayed on the substrates and the time the substrates are effectively launched at sea, which could take several hours. To optimize efforts related to the transfer of young seedlings from hatchery to sea and ultimately to reduce time and cost in the hatchery, many studies tested the use of a binder treatment (Kerrison et al., 2018; Kerrison et al., 2020; Solvang et al., 2021; Visch et al., 2023). Many authors including Forbord et al. (2020), strongly advocate the virtues of using a binder, arguing that it is widely used by commercial farms and in research projects and that it is 100× more space-efficient during the laboratory phase of the production cycle as it promotes the strength and resilience of the attachment to the substrate and rapid deployment at sea (Xu et al., 2009; Forbord et al., 2018; Kerrison et al., 2018); rendering the period of pre-growth of seedling unnecessary. On the other hand, the efficiency and utility of the binder to specifically promote biomass increases appears to be inconclusive based on recent studies. For example, Kerrison et al. (2018) and Kerrison et al. (2020) achieved opposite results with S. latissima (sporophytes in suspension with or without binder) with no observable biomass differences. Visch et al. (2023), in a controlled laboratory study, did not observe any effect on seeding of kelp (S. latissima) propagules of a binder (agar and κ-carrageenan) nor an effect of flow velocity (0, 5 and 15 cm s-1) for the binder treatments.
Substrate roughness and texture may also facilitate physical entanglement and attachment of meiospores, gametophytes or small sporophytes (65-1000 µm) and thus reduce their loss when transfer at sea (Conitz et al., 2013; Kerrison et al., 2016; Kerrison et al., 2018; Kerrison et al., 2020). Natural rocks used in this study had irregularities but had overall a smoother surface than the artificial rocks that were more concrete-like and that were shaped with three groves to add more relief. Reliefs add surface area compared to the natural rocks, to offer a variety of options to facilitate holdfast adhesion (Fletcher and Callow, 1992). However, this difference in roughness did not seem to have had an impact on density throughout the experiment because the densities observed throughout the experiment were in general similar between the natural and artificial rocks. We also promote the use of the artificial substrate based on another criterion, the fact that their rectangular shape allows them to be more easily stackable during transport between the hatchery and the drop-off location at sea. We further argue that the absence of differences in sporophyte density observed between treatments in the later stages, could very well be linked to the use of gametophyte seeding concentration in excess. This assumption is based on Lemoine and Lemaire (2019) who used in their trials 0.5 g, 1.0 and 2 g gametophytes per liter of seeding solutions, and observed similar development at 0.5 g/L after 15 and 29 days of growth.
By analysing seedling’s length throughout the experiment, we can see that the combination of factors had an effect on total length in one way or another. On day 14 (first day of length data), the presence of the binder might have had an inhibitory effect on the beginning of the growth of the seedlings on both substrates because they were smaller compared to seedlings that had not been in contact with a binder. When they exposed small sporophytes of 1-2 mm to binder, Kerrison et al. (2018) reported that the binder did not have an impact on sporophyte growth, but in our case, it was the gametophyte that contained oocytes that were exposed to it. The addition of a high viscosity component could have restricted or slowed down the emergence of the sporophytes. The seedlings that were not in contact with a binder exhibited a higher length when exposed to elevated water velocity, indicating that even at this early stage, high current was probably favoring transport of nutrients across the diffusion boundary layer. On days 21 and 28, all sporophytes benefited significantly from the elevated water velocity, regardless of the spraying method. This also suggests that binder did not influence the growth of sporophytes from about 700 µm (minimum average length on day 21). The effect of water velocity in our study is consistent with other work that suggested that increasing water velocity increases nutrient uptake and thus growth rate (Parker, 1981; Larned and Atkinson, 1997; Kregting et al., 2008). Nanba et al. (2011) and Peteiro et al. (2016) showed that sporophytes of the kelp U. pinnatifida and S. latissima had a higher biomass yield when cultivated in coastal areas with moderate to high water velocities than under low water velocity conditions. Peteiro et al. (2019) reported that the growth rate of seedlings of Undaria pinnatifida on strings in indoor tanks increased with increasing water velocity until it reached a point beyond which it started to decline. In our experiment we did not test enough different water velocities to find the optimal for S. latissima, but elevated velocity of 0.2 m s-1 still promoted longer seedlings, and this value is even higher than the optimal water velocity for growth of U. pinnatifida (0.158-0.171 m s-1).
On day 35, there were no more differences in length between treatments (average value of 4821.65 ± 1472.53 µm) and on day 37, there were no more differences in densities between treatments (mean value of 95.17 ± 8.44%). At this point, the density has reached a point where the sporophytes are in complete competition for vital space, light and nutrients. To better evaluate the impact of these factors on the time frame of the experiment, we may benefit from a smaller gametophyte density on the substrates right from the beginning. Various densities may be achieved by manipulating the concentration of gametophytes or spores suspended in the media for fixation as suggested in a study by Reed (1990). Finding the optimal gametophyte density that allows the better growth rate until the target length of 15 mm, under our environmental conditions, would also favor the production of healthy sporophytes while reducing the time and cost related to their production in laboratory condition.
Complementary to density or % coverage measurement evaluated by image analysis, biomass assessment would be relevant to include because it would provide a better understanding of the effect of the treatments on growth not only spatial distribution. Peteiro et al. (2019) calculate biomass in terms of dry biomass. Overall, the use of the imageJ software for density analysis yielded data with a satisfactory degree of precision for the preliminary evaluation of the relationship between the chosen factors and the density of S. latissima during early developmental stages. The main advantage of using the point counting method is that it is systematically done, eliminating possible manual biases, and is therefore more accurate. However, at some point of the growth trial, this technique shows less discriminatory power between treatment (when all the area is covered by the developing sporophytes). Biomass evaluation under our conditions, could provide better assessment since growth/development could also be expressed in mass through frond thickness and shape differences.
Production of small sporophytes of S. latissima for purposes of coastal restoration and erosion defense goals was overall a success. Some indications that substrate, binder and velocity treatment interactions could affect thallus length were observed but no overall effect on SGR could be detected. All combinations of factors resulted in the initiation of sporophyte growth on day 14 after gametophyte spraying (24 days after switching to white light), which was consistent with the observations of other authors (Flavin et al., 2013; Fredriksen et al., 2020). In the present study, sporophytes had an average length of 4.8 ± 1.5 mm on day 35 and on day 42, all treatments indicated that fronds reached the length target of 15 mm, the average length was 16.99 ± 5.2 mm. Other authors on S. latissima indicated a length of 2-4 mm after 35 days (Peteiro et al., 2014) or 40 days (Flavin et al., 2013) prior to launching at sea.
The raw data supporting the conclusions of this article will be made available by the authors, without undue reservation.
NLF: Writing of proposal, administration, supervision, analyses, scientific writing, corresponding author AT-G: scientific writing CD-J: statistical analyses, scientific writing J-CB: analysis M-PP: experiments, preliminary data analysis JP: experiments, preliminary data analysis, FP-L: Image analysis IG-L: scientific writing (mat & met). All authors contributed to the article and approved the submitted version.
We would like to thank the Coastal Restoration Fund Program which is part of the National Ocean Protection Plan of the Department of Fisheries and Oceans Canada, for its financial support (Report No. 033119).
The authors also acknowledge the contribution of Nicolas Lemaire, Grégoire Cholat-Namy and Mathilde Lemoine at Merinov. We also express our gratitude to the Société des Amis du Biodôme de Montréal (SABM) for administrative and financial support.
The authors declare that the research was conducted in the absence of any commercial or financial relationships that could be construed as a potential conflict of interest.
All claims expressed in this article are solely those of the authors and do not necessarily represent those of their affiliated organizations, or those of the publisher, the editors and the reviewers. Any product that may be evaluated in this article, or claim that may be made by its manufacturer, is not guaranteed or endorsed by the publisher.
Alsuwaiyan N. A., Filbee-Dexter K., Vranken S., Burkholz C., Cambridge M., Coleman M. A., et al. (2022). Green gravel as a vector of dispersal for kelp restoration. Front. Mar. Sci. 9. doi: 10.3389/fmars.2022.910417
Aubé S. (2020). Solutions pour diminuer l’érosion des berges: Analyse des pratiques dans les régions du bas-Saint-Laurent et de la gaspésie (Université de Montréal), 17. Terminal project/Bachelor in Urban Planning. Available at: https://effa.umontreal.ca/2020/projet/urbanisme/Solutions+pour+diminuer+l%26rsquo%3B%26eacute%3Brosion+des+berges+%3A+analyse+des+pratiques+dans+les+r%26eacute%3Bgions+du+Bas-Saint-Laurent+et+de+la+Gasp%26eacute%3Bsie.
Bernatchez P., Fraser C., Friesinger S., Jolivet Y., Dugas S., Drejza S., et al. (2008). Sensibilité des côtes et vulnérabilité des communautés du golfe du saint-Laurent aux impacts des changements climatiques (Université du Québec à Rimouski), 256. Research report submitted to the Consortium OURANOS and to FACC.
Bernatchez P., Quintin C. (2016). Potentiel de migration des écosystèmes côtiers meubles québécois de l’estuaire et du golfe du saint-Laurent dans le contexte de la hausse appréhendée du niveau de la mer. Nat. Can. 140, 91–104. doi: 10.7202/1036507ar
Bishop M. J., Mayer-Pinto M., Airoldi L., Firth L. B., Morris R. L., Loke L. H. L., et al. (2017). Effects of ocean sprawl on ecological connectivity: impacts and solutions. J. Exp. Mar. Biol. Ecol. 492, 7–30. doi: 10.1016/j.jembe.2017.01.021
Boderskov T., Nielsen M. M., Rasmussen M. B., Sokvbjerg Balsby T. J., Macleod A., Holdt S. L., et al. (2021). Effects of seeding method, timing and site selection on the production and quality of sugar kelp, Saccharina latissima: a Danish case study. Alg. Res. 53, 102160. doi: 10.1016/j.algal.2020.102160
Bouma T. J., Van Belzen J., Balke T., Zhu Z., Airoldi L., Blight A. J., et al. (2014). Identifying knowledge gaps hampering application of intertidal habitats in coastal protection: opportunities and steps to take. Coast. Eng. 87, 147–157. doi: 10.1016/j.coastaleng.2013.11.014
Brijesh K. T., Troy D. J. (2015). Seaweed sustainability: Food and non-food applications. 1st Edition (USA: Academic Press).
Bruton T., Lyons H., Lerat Y., Stanley M., Rasmussen M. B. (2009). A review of the potential of marine algae as a source of biofuel in Ireland (Dublin: Sustainable energy Ireland (SEI), 88.
Burek K. E., O’Brien J. M., Scheibling R. E. (2018). Wasted effort: recruitment and persistence of kelp on algal turf. Mar. Ecol. Prog. Ser. 600, 3–19. doi: 10.3354/meps12677
Campbell A. H., Marzinelli E. M., Vergés A., Coleman M. A., Steinberg P. D. (2014). Towards restoration of missing underwater forests. PloS One 9, e84106. doi: 10.1371/journal.pone.0084106
Christie H., Jørgensen N. M., Norderhaug K. M., Waage-Nielsen E. (2003). Species distribution and habitat exploitation of fauna associated with kelp (Laminaria hyperborea) along the Norwegian coast. J. Mar. Biol. Assoc. U.K. 83, 687–699. doi: 10.1017/S0025315403007653h
Conitz J. M., Fagen R., Stekoll M. S. (2013). Effects of density and substrate type on recruitment and growth of Pyropia torta (Rhodophyta) gametophytes. Bot. Mar. 56, 525–533. doi: 10.1515/bot-2013-0067
Deely J., Hynes S. (2020). Blue-green or grey, how much is the public willing to pay? Landsc. Urban. Plan. 203, 103909. doi: 10.1016/j.landurbplan.2020.103909
Eger A. M., Marzinelli E. M., Christie H., Fagerli C. W., Fujita D., Gonzalez A. P., et al. (2022). Global kelp forest restoration: past lessons, present status, and future directions. Biol. Rev. 97, 1449–1475. doi: 10.1111/brv.12850
Falace A., Zanelli E., Bressan G. (2006). Algal transplantation as a potential tool for artificial reef management and environmental mitigation. Bull. Mar. Sci. 78, 161–166.
Flavin K., Flavin N., Flahive B. (2013). Kelp farming Manual: A guide to the processes, techniques, and equipment for farming kelp in new England waters (Portland: Saco, ME, Ocean Approved LLC).
Fletcher R. L., Callow M. E. (1992). The settlement, attachment and establishment of marine algal spores. Br. Phycol. J. 27, 309–329. doi: 10.1080/00071619200650281
Forbord S., Steinhovden K. G., Rød K. K., Handå A., Skjerno J. (2018). Cultivation protocol for saccharina latissima in protocols for macroalgae research. 1st ed. Eds. Charrier B. T. W., Reddy C. (Boca Raton, FL: CRC Press), 37–59.
Forbord S., Steinhovden K. B., Solvang T., Handå A., Skjermo J. (2020). Effect of seeding methods and hatchery periods on sea cultivation of Saccharina latissima (Phaeophyceae): a Norwegian case study. J. Appl. Phycol. 32, 2201–2212. doi: 10.1007/s10811-019-01936-0
Fredriksen S., Filbee-Dexter K., Norderhaug K. M., Steen H., Bodvin T., Coleman M. A., et al. (2020). Green gravel: a novel restoration tool to combat kelp forest decline. Sci. Rep. 10, 3983. doi: 10.1038/s41598-020-60553-x
Gittman R. K., Popowich A. M., Bruno J. F., Peterson C. H. (2014). Marshes with and without silt protect estuarine shorelines from erosion better than bulkheads during a category 1 hurricane. Ocean Coast. Manage. 102, 94–102. doi: 10.1016/j.ocecoaman.2014.09.016
Gomez K. A., Gomez A. A. (1984). Statistical procedures for agricultural research. (New York: John Wiley and Sons), 704.
Grebe G. S., Byron C. J., St. Gelais A., Kotowicz D. M., Olson T. K. (2019). An ecosystem approach to kelp aquaculture in the americas and Europe. Aquac. Rep. 15, 100215. doi: 10.1016/j.aqrep.2019.100215
Hasselström L., Visch W., Gröndahl F., Nylund G. M., Pavia H. (2018). The impact of seaweed cultivation on ecosystem services - a case study from the west coast of Sweden. Mar. pollut. Bull. 133, 53–54. doi: 10.1016/j.marpolbul.2018.05.005
Hepburn C. D., Holborow J. D., Wing S. R., Frew R. D., Hurd C. L. (2007). Exposure to waves enhances the growth rate and nitrogen status of the giant kelp Macrocystis pyrifera. Mar. Ecol. Prog. Ser. 339, 99–108. doi: 10.3354/meps339099
Hinkel J., Lincke D., Vafeidis A. T., Perrette M., Nicholls R. J., Tol R. S. J., et al. (2014). Coastal flood damage and adaptation costs under 21st century sea-level rise. P. Natl. A. Sci. 111, 3292–3297. doi: 10.1073/pnas.1222469111
Hurd C. L. (2000). Water motion, marine macroalgal physiology and production. J. Phycol. 36, 453–472. doi: 10.1046/j.1529-8817.2000.99139.x
Hynes S., Chen W., Vondolia K., Armstrong C., O’Connor E. (2021). Valuing the ecosystem service benefits from kelp forest restoration: A choice experiment from Norway. Ecol. Econ. 179, 106833. doi: 10.1016/j.ecolecon.2020.106833
Kerrison P. D., Innes M., Macleod A., McCormick E., Elbourne P. D., Stanley M. S., et al. (2020). Comparing the effectiveness of twine- and binder-seeding in the laminariales species Alaria esculenta and Saccharina latissima. J. Appl. Phycol. 32, 2173–2181. doi: 10.1007/s10811-020-02069-5
Kerrison P. D., Stanley M. S., De Smet D., Buyle G., Hughes A. D. (2019). Holding (not so) fast: surface chemistry constrains kelp adhesion. Eur. J. Phycol. 54, 291–299. doi: 10.1080/09670262.2018.1547924
Kerrison P. D., Stanley M. S., Hughes A. D. (2018). Textile substrate seeding of Saccharina latissima sporophytes using a binder: An effective method for the aquaculture of kelp. Algal Res. 33, 352–357. doi: 10.1016/j.algal.2018.06.005
Kerrison P. D., Stanley M. S., Kelly M., MacLeod A., Black K. D., Hughes A. D. (2016). Optimising the settlement and hatchery culture of Saccharina latissima (Phaeophyta) by manipulation of growth medium and substrate surface condition. J. Appl. Phycol. 28, 1181–1191. doi: 10.1007/s10811-015-0621-6
Kim J. K., Kraemer G. P., Neefus C. D., Chung I. K., Yarish C. (2007). Effects of temperature and ammonium on growth, pigment production and nitrogen uptake by four species of porphyra (Bangiales, rhodophyta) native to the new England coast. J. Appl. Phycol. 19, 431–440. doi: 10.1007/s10811-006-9150-7
Kregting L. T., Hurd C. L., Pilditch C. A., Stevens C. L. (2008). The relative importance of water motion on nitrogen uptake by the subtidal macroalga Adamsiella chauvinii (Rhodophyta) in winter and summer. J. Phycol. 44, 320–330. doi: 10.1016/j.jembe.2016.02.006
Lajoie M., Baillargeon S., Boyer-Villemaire U., Crousset Y. (2007). L’érosion des berges au québec maritime (Comité ZIP Côte-Nord du Golfe). Information document.
Larned S. T., Atkinson M. J. (1997). Effects of water velocity on NH4 and PO4 uptake and nutrient-limited growth in the macroalga Dictyosphaeria cavernosa. Mar. Ecol. Prog. Ser. 157, 295–302. doi: 10.3354/meps157295
Le François N. R., Picq S., Savoie A., Boussin J.-C., Wong E., Misserey L., et al. (2015). Coupling salinity reduction to aquatic animal well-being and ecosystem representativeness at the biodôme de montréal. J. Zoo. Aquar. Res. 3, 70–76. doi: 10.19227/jzar.v3i2.96
Lemoine M., Lemaire M. (2019), 1. Optimisation des cultures et de la stratégie d’ensemencement des algues sur les récifs. Rapport DFO-MPO, No. 19-14.
Merzouk A., Johnson L. E. (2011). Kelp distribution in the northwest Atlantic ocean under a changing climate. J. Exp. Mar. Biol. Ecol. 400, 90–98. doi: 10.1016/j.jembe.2011.02.020
Monteiro C., Li H., Diehl N., Collén J., Heinrich S., Bischof K., et al. (2021). Modulation of physiological performance by temperature and salinity in the sugar kelp Saccharina latissima. Phycol. Res. 69, 48–57. doi: 10.1111/pre.12443
Morris R. L., Konlecher T. M., Ghisalberti M., Swearer S. E. (2017). From grey to green: efficacy of eco-engineering solutions for nature-based coastal defense. Glob. Change. Biol. 24, 1827–1842. doi: 10.1111/gcb.14063
Nanba N., Fujiwara T., Kuwano K., Ishikawa Y., Ogawa H., Kado R. (2011). Effect of water flow velocity on growth and morphology of cultured Undaria pinnatifida sporophytes (Laminariales, phaeophyceae) in okirai bay on the sanriku coast, northeast Japan. J. Appl. Phycol. 23, 1023–1030. doi: 10.1007/s10811-010-9635-2
Narayan S., Beck M. W., Reguero B. G., Losada I. J., van Wesenbeeck B., Pontee N., et al. (2016). The effectiveness costs and coastal protection benefits of natural and nature-based defences. PloS One 11, e0154735. doi: 10.1371/journal.pone.0154735
Pang S., Lüning K. (2004). Tank cultivation of the red alga Palmaria palmata: Effects of intermittent light on growth rate, yield and growth kinetics. J. Appl. Phycol. 16, 93–99. doi: 10.1023/b:japh.0000044779.30182.d8
Parker H. S. (1981). Influence of relative water motion on the growth, ammonium uptake and carbon and nitrogen composition of Ulva lactuca (Chlorophyta). Mar. Biol. 63, 309–318. doi: 10.1007/bf00396001
Pearson G. A., Martins N., Madeira P., Serrão E. A., Bartsch I. (2019). Sex-dependent and -independent transcriptional changes during haploid phase gametogenesis in the sugar kelp Saccharina latissima. PloS One 14, e0219723. doi: 10.1371/journal.pone.0219723
Peteiro C., Bidegain G., Sánchez N. (2019). Experimental evaluation of the effect of water velocity on the development of string-attached seedlings (Laminariales) with implications for hatchery and nursery production. Algal Res. 44, 101678. doi: 10.1016/j.algal.2019.101678
Peteiro C., Freire O. (2013). Biomass yield and morphological features of the seaweed Saccharina latissima cultivated at two different sites in a coastal bay in the Atlantic coast of Spain. J. Appl. Phycol. 25, 205–2013. doi: 10.1007/s10811-012-9854-9
Peteiro C., Sánchez N., Dueñas-Liaño C., Martinez B. (2014). Open-sea cultivation by transplanting young fronds of the kelp Saccharina latissima. J. Appl. Phycol. 26, 519–528. doi: 10.1007/s10811-013-0096-2
Peteiro C., Sánchez N., Martínez B. (2016). Mariculture of the Asian kelp Undaria pinnatifida and the native kelp Saccharina lattisima along the Atlantic coast of southern Europe: an overview. Algal. Res. 15, 9–23. doi: 10.1016/j.algal.2016.01.012
Picard M. M. M., Johnson L. E., Ferrario F., Garrido I., Archambault P., Carrières J., et al. (2022). Drivers of kelp distribution in the gulf of st. Lawrence: insights from a transplant experiment. Mar. Biol. 169, 50. doi: 10.1007/s00227-022-04031-0
Raby D., Pinna S., Joubert J-É., Ouellet M., Brière M.-C. (2008). Plan de protection et de mise en valeur de la barre de sandy beach (Gaspé, Québec). Report of the Comité de concertation de la Baie-de-Gaspé, 145 p.
Redmond S. (2013). Effects of increasing temperature and ocean acidification on the microstages of two populations of saccharina latissima in the Northwest Atlantic (Storrs (SL: University of Connecticut). dissertation/master’s thesis.
Reed D. C. (1990). The effects of variable settlement and early competition on patterns of kelp recruitment. Ecology 71, 776–787. doi: 10.2307/1940329
Reid G. K., Chopin T., Robinson S. M. C., Azevedo P., Quinton M., Belyea E. (2013). Weight ratios of the kelps, Alaria esculenta and Saccharina latissima, required to sequester dissolved inorganic nutrients and supply oxygen for Atlantic salmon, Salmo salar, in integrated multi-trophic aquaculture systems. Aquaculture 408, 34–46. doi: 10.1016/j.aquaculture.2013.05.004
Shea R., Chopin T. (2007). Effects of germanium dioxide, an inhibitor of diatom growth, on the microscopic laboratory cultivation stage of the kelp, Laminaria saccharina. J. Appl. Phycol. 19, 27–32. doi: 10.1007/s10811-006-9107-x
Slangen A. B. A., Wal Van De R. S. W., Wada Y., Vermeersen L. L. A. (2014). Comparing tide gauge observations to regional patterns of sea-level change, (1961-2003). Earth. Syst. Dyn. Discuss. 5, 169–201. doi: 10.5194/esd-5-243-2014
Solvang T., Bale E. S., Broch O. J., Handå A., Alver M. O. (2021). Automation concepts for industrial-scale production of seaweed. Front. Mar. Sci. 8. doi: 10.3389/fmars.2021.613093
Steinhagen S., Enge S., Cervin G., Larsson K., Edlund U., Schmidt A. E. M., et al. (2022). Harvest time can affect the optimal yield and quality of sea lettuce (Ulva fenestrata) in a sustainable sea-based cultivation. Front. Mar. Sci. 9. doi: 10.3389/fmars.2022.816890
Tamigneaux É., Johnson L. E. (2016). Les Macroalgues du saint-Laurent: une composante essentielle d’un écosystème marin unique et une ressource naturelle précieuse dans un contexte de changement global. Nat. Can. 140, 62–73. doi: 10.7202/1036505ar
Tamigneaux É., Licois A., Bourdages D., Leblanc M.-J. (2013). Protocoles pour la culture de la laminaire à long stipe (Saccharina longicruris) et de la laminaire sucrée (Saccharina latissima) dans le contexte du Québec, 1st ed. Guide No. 13-01, Merinov, Gaspé 38 p.
Tamigneaux É., Pedneault E., Gendron L. (2014). Comparaison des rendements de l’algue brune saccharina longicruris cultivée en milieu ouvert en gaspésie et en lagune aux Îles-de-la-Madeleine: Final report. Research and development report Guide No. 14-04, Merinov, Gaspé 34 p.
Temmerman S., Meire P., Bouma T. J., Herman P. M. J., Ysebaert T., De Vriend H. J. (2013). Ecosystem-based coastal defence in the face of global change. Nature 504, 79–83. doi: 10.1038/nature12859
Tremblay-Gratton A., Boussin J.-C., Tamigneaux É., Vandenberg G. W., Le François N. R. (2018). Bioremediation efficiency of Palmaria palmata and Ulva lactuca for use in a fully recirculated cold-seawater naturalistic exhibit: effect of temperature and high NO3 and PO4 concentrations. J. Appl. Phycol. 30, 1295–1304. doi: 10.1007/s10811-017-1333-x
Venables W. N., Ripley B. D. (2002). Modern applied statistics with s. 4th ed. (New York: Springer).
Visch W., Kononets M., Hall P. O. J., Nylund G. M., Pavia H. (2020). Environmental impact of kelp (Saccharina latissima) aquaculture. Mar. pollut. Bull. 155, 110962. doi: 10.1016/j.marpolbul.2020.110962
Visch W., Larsson A. I., Aberg P., Toth G. B. (2023). Adherence of kelp (Saccharina latissima) gametophytes on ropes with different binder treatments and flow regimes. J. Appl. Phycol. 35, 195–200. doi: 10.1007/s10811-022-02860-6
Werner A., Clarke D., Kraan S. (2003). Strategic review and the feasibility of seaweed aquaculture in Ireland (Galway: Marine Institute).
Westermeier R., Murúa P., Patiño D. J., Muñoz L., Atero C., Müller D. G. (2014). Repopulation techniques for Macrocystis integrifolia (Phaeophyceae: Laminariales) in atacama, Chile. J. Appl. Phycol. 26, 511–518. doi: 10.1007/s10811-013-0069-5
Wickham H., François R., Henry L., Müller K. (2022) Dplyr: A grammar of data manipulation. Available at: https://dplyr.tidyverse.orghttps://github.com/tidyverse/dplyr (Accessed November 2nd, 2022).
Xu B., Zhang Q. S., Qu S. C., Cong Y. Z., Tang X. X. (2009). Introduction of a seedling production method using vegetative gametophytes to the commercial farming of laminaria in China. J. Appl. Phycol. 21, 171–178. doi: 10.1007/s10811-008-9347-z
Keywords: bottom planting, kelp, sporophyte early-growth, seedling production, coastal restoration
Citation: Le François NR, Tremblay-Gratton A, Drouin-Johnson C, Prégent J, Presne-Poissant M-P, Boussin J-C, Piché-Lebel F and Gendron-Lemieux I (2023) Nature-based coastal restoration: Development of an early-rearing production protocol of sugar kelp (Saccharina latissima Linnaeus) for bottom planting activities in the Gulf of St-Lawrence (Québec, Canada). Front. Mar. Sci. 10:1135417. doi: 10.3389/fmars.2023.1135417
Received: 31 December 2022; Accepted: 27 March 2023;
Published: 19 April 2023.
Edited by:
Albertus J. Smit, University of the Western Cape, South AfricaReviewed by:
Antoine De Ramon N’Yeurt, University of the South Pacific, FijiCopyright © 2023 Le François, Tremblay-Gratton, Drouin-Johnson, Prégent, Presne-Poissant, Boussin, Piché-Lebel and Gendron-Lemieux. This is an open-access article distributed under the terms of the Creative Commons Attribution License (CC BY). The use, distribution or reproduction in other forums is permitted, provided the original author(s) and the copyright owner(s) are credited and that the original publication in this journal is cited, in accordance with accepted academic practice. No use, distribution or reproduction is permitted which does not comply with these terms.
*Correspondence: Nathalie R. Le François, TmF0aGFsaWVSb3NlLkxlRnJhbmNvaXNAbW9udHJlYWwuY2E=
Disclaimer: All claims expressed in this article are solely those of the authors and do not necessarily represent those of their affiliated organizations, or those of the publisher, the editors and the reviewers. Any product that may be evaluated in this article or claim that may be made by its manufacturer is not guaranteed or endorsed by the publisher.
Research integrity at Frontiers
Learn more about the work of our research integrity team to safeguard the quality of each article we publish.