- 1Fisheries and Aquatic Sciences Program, School of Forest Fisheries and Geomatic Sciences, Institute of Food and Agriculture Sciences, University of Florida, Gainesville, FL, United States
- 2Cooperative Institute of Marine and Atmospheric Sciences, Rosenstiel School of Marine Atmospheric and Earth Science, University of Miami, Miami, FL, United States
- 3Southeast Fisheries Science Center, National Marine Fisheries Service, National Oceanic and Atmospheric Administration, Miami, FL, United States
- 4Office of National Marine Sanctuaries, National Oceanic and Atmospheric Administration, Silver Spring, MD, United States
- 5Nature Coast Biological Station, Institute of Food and Agriculture Sciences, University of Florida, Cedar Key, FL, United States
A deepwater (>40 m) fishery for invasive lionfish (Pterois volitans/miles) offers a potential means to control invasive lionfish densities and mitigate their impacts on reefs too deep for SCUBA removals. Trapping could provide a scalable solution—if an effective fishing gear with minimal environmental impacts could be permitted and adopted by fishers. We tested the efficacy of wooden slat lobster traps, wire sea bass traps, and experimental non-containment Gittings traps. One hundred deployments of each trap type were made at 120 mesophotic (38–78 m deep) natural reef sites in the northeastern Gulf of Mexico (29.6–30.1°N, 86.1–87.6°W). Reef sites were surveyed with remotely operated vehicles (ROV) before and after trap deployments, and remote time-lapse video cameras were affixed above 86 traps to sample in situ recruitment to the traps. The video data showed that lionfish were attracted to the vicinity of the three trap types at similar rates, but that lionfish rarely entered the lobster or sea bass traps. The high bycatch rates of sea bass traps suggested their use is likely unsuitable for targeting lionfish. Lobster traps had lower rates of bycatch, but their relatively high ratio of bycatch-to-lionfish catches suggests that modifications will be needed to make them more efficient. The Gittings traps had the highest lionfish catch rates and lowest bycatches of native fishes, but operational issues were also identified. They failed to open on 20% of deployments and one entangled a green sea turtle (Chelonia mydas). Even with the best-performing trap design, the average catch rate of lionfish was less than one lionfish per trap. A potential explanation could be the low biomass of lionfish observed during the ROV surveys, which averaged 0.3 kg lionfish per site. The time-lapse video data suggested that lionfish recruitment to Gittings traps could increase with higher densities of lionfish on the nearby reefs, if traps were retrieved after approximately two days of deployment, and if traps were retrieved during dawn or dusk. Further research, development, and testing is needed for lionfish traps, and critical bio- and techno-economic assessments appear warranted to evaluate the feasibility of a deepwater lionfish fishery.
1 Introduction
Controlling invasive lionfish (Pterois volitans/miles complex) populations is a major objective for marine conservation and fishery managers in the western Atlantic Ocean (Morris et al., 2012; Hixon et al., 2016; Sutherland et al., 2017) and the Mediterranean Sea (Kletou et al., 2016; Savva et al., 2020; Ulman et al., 2020). To date, removal efforts have primarily utilized spearfishing by SCUBA divers (Morris et al., 2012; Clements et al., 2021), which can efficiently remove lionfish (Usseglio et al., 2017; Harris et al., 2019; Ulman et al., 2022) and reduce local lionfish densities regions (Green et al., 2014; Harms-Tuohy et al., 2018; Harris et al., 2019). Market demand for invasive lionfish has also increased (Blakeway et al., 2019; Blakeway et al., 2021; Bogdanoff et al., 2020), and commercial fisheries have developed for lionfish in some areas (Chapman et al., 2016; Harris et al., 2020a; Ulman et al., 2022).
A key issue for lionfish removal efforts and lionfish fisheries is that spearfishing is limited to the reefs accessible to SCUBA diving and thus in depths generally less than 40 m. Lionfish have been observed as deep as 300 m (Gress et al., 2017), and, throughout the invaded Western Atlantic, lionfish densities on mesophotic reefs range are higher than on shallower reefs nearby (Table 1). High densities of lionfish have correlated with community shifts on tropical (Lesser and Slattery, 2011) and semi-tropical (Lewis et al., 2020) mesophotic reefs. Relatively little is known about the impacts of lionfish on reefs deeper than 90 m (Andradi-Brown, 2019), but ecosystem models suggest that community impacts by lionfish are likely driven or exacerbated by lionfish predation on and competition with native species (Arias-González et al., 2011; Chagaris et al., 2017; Chagaris et al., 2020). Deepwater lionfish populations also may serve as a refuge for larger, highly fecund females that are a source of larvae to shallower reefs (Andradi-Brown et al., 2017a; Andradi-Brown et al., 2017b; Andradi-Brown, 2019).
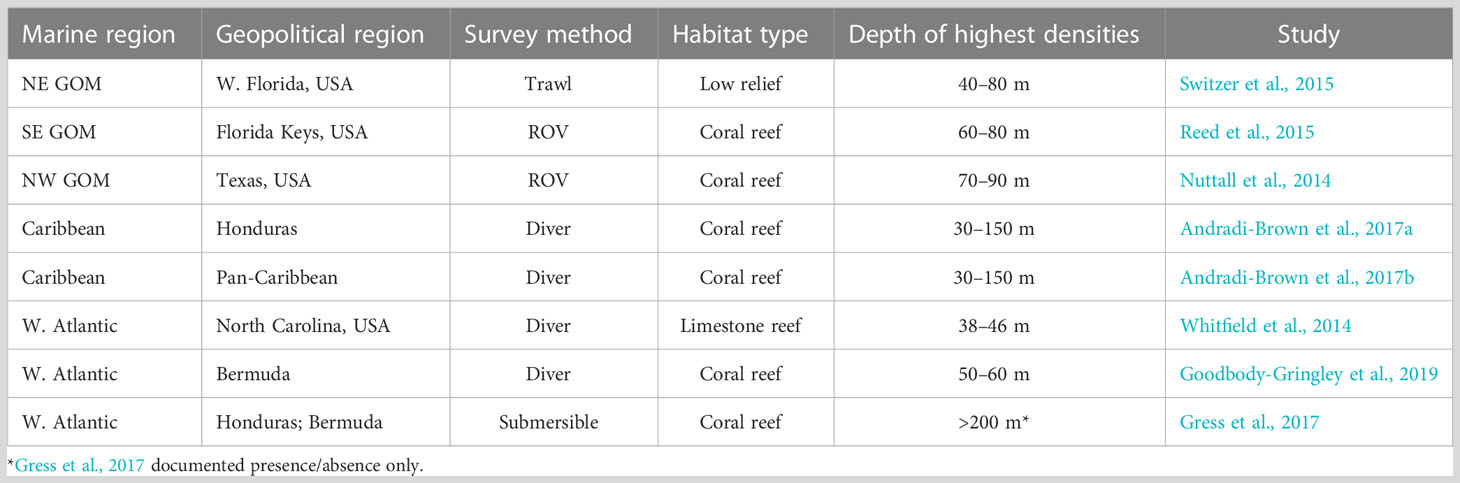
Table 1 Western Atlantic invasive lionfish with population density surveys conducted at different depths found that lionfish densities are highest >30 m compared to concurrent surveys at shallower reefs.
Although lionfish are incidentally captured from mesophotic depths with hook-and-line (Morris et al., 2012) and trawls (Switzer et al., 2015; Campbell et al., 2021), their catch rates are likely too low to control population densities (Arias-González et al., 2011). These deepwater lionfish populations could potentially be controlled via fishery removals if a suitable harvest gear could be developed, tested, and permitted. A new harvest stream could also stabilize commercial lionfish supply chains, which have had limited market development (Chapman et al., 2016; Blakeway et al., 2019). Management agencies in the United States have thus designed regulatory pathways to encourage lionfish trap research, development, and testing (RD&T) for harvest gears that show potential for encouraging lionfish fisheries and controlling their ecological and economic impacts (Johnston et al., 2015; Gittings et al., 2017; U.S. National Marine Fisheries Service, 2018).
We examined three trap designs for catching lionfish (Figure 1). These included i) wooden slat traps used to target spiny lobsters in the Florida Keys (henceforth “lobster traps”), ii) wire mesh traps used to target black sea bass, Centropristis striata, in the US Atlantic (henceforth “sea bass traps”), and iii) experimental Gittings non-containment lionfish traps (henceforth, “Gittings traps”). Lobster traps were selected for testing because lionfish are routinely captured as bycatch within the Florida Keys spiny lobster (Panulirus argus) trap fishery (Akins et al., 2012). Between 2010 and 2018, over 31,000 kg of lionfish bycatch was harvested by commercial trappers (Florida Fish and Wildlife Conservation Commission, 2019). Sea bass traps were selected for testing because they target marine piscivorous fish with a similar size, diet, and trophic position as lionfish. They are also the only marine fish trap permitted for use in continental US federal waters. The Gittings non-containment lionfish trap is an experimental, semi-actively fished trap designed to attract lionfish to a vertical lattice structure that extends upward from two semicircular trap wings that lay flat on the bottom. The baitless, non-containment design of the Gittings trap, which requires active closing of the hinged trap wings during retrieval, was intended to minimize bycatch and ghost-fishing in the event that the trap is lost (Gittings, 2016; Gittings et al., 2017; Harris et al., 2020b).
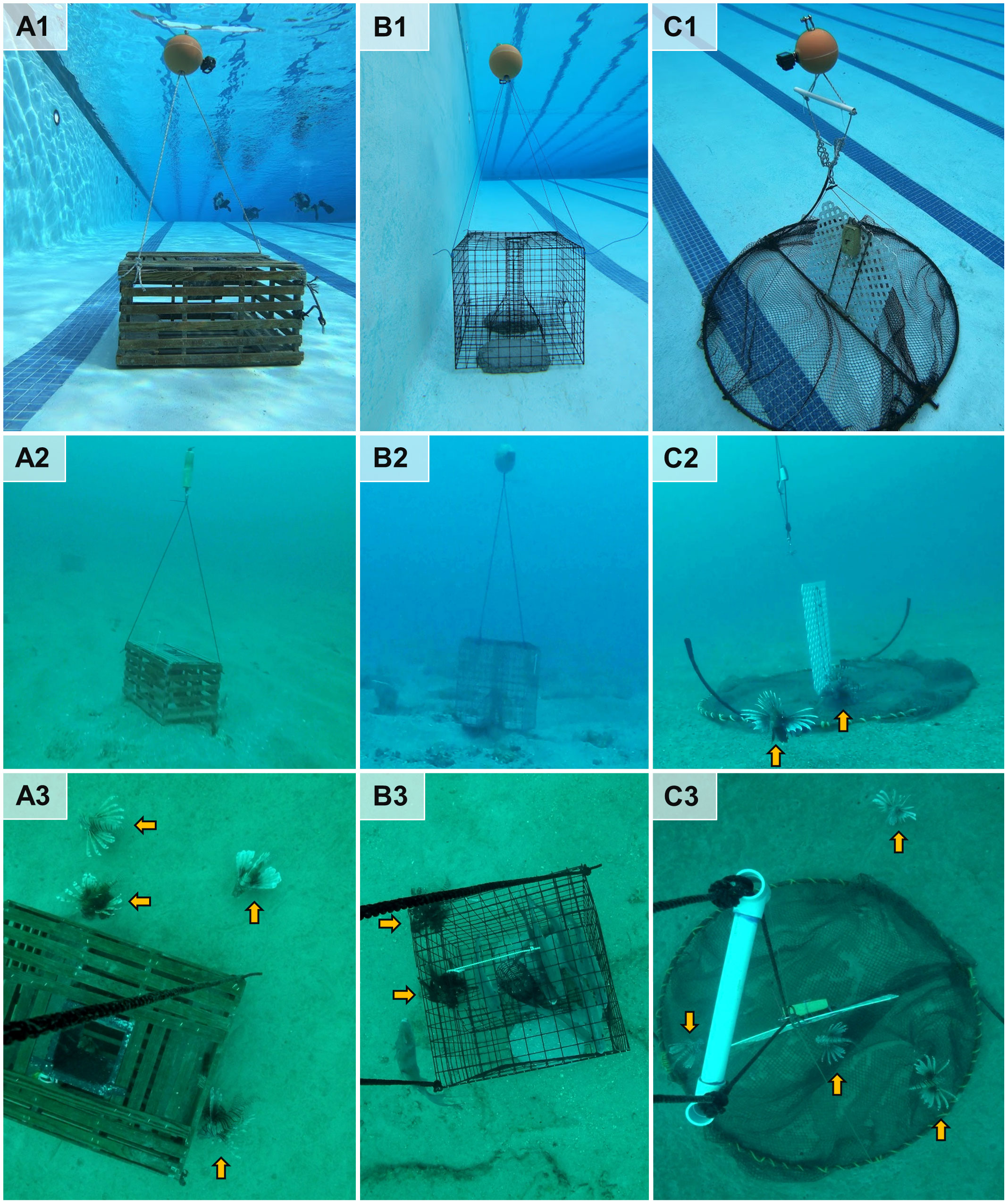
Figure 1 Experimental traps tested for catching invasive lionfish. Trap designs included (A) wooden slat traps used in the Florida spiny lobster fishery, (B) wire mesh traps used in the South Atlantic sea bass fishery, and (C) Gittings non-containment lionfish traps. Traps were gear tested in the University of Florida pool (first row) before the study’s experimental deployments near northeastern Gulf of Mexico mesophotic reefs (second row; images from a remotely operated vehicle). A subset of traps were monitored with remote time-lapse cameras from a subsurface buoy above the trap (third row). Photos by the authors.
Our objectives were to assess the gear performance and catch efficiencies for the three trap designs when deployed near deepwater reefs (approximately 40–80 m) that would be inaccessible to conventional SCUBA diving. Gear performance for the trap designs was evaluated by measuring the frequency of successful deployments, lionfish catches (in number and biomass), and their bycatch of native species. We examined trap efficacy by testing the differences in catch per unit effort (CPUE, per trap) of lionfish versus native fishes for each trap type and the differences between in situ trap recruitment (i.e., number of lionfish inside the footprint of the Gittings trap or inside the container of the lobster and sea bass traps) monitored with remote time-lapse cameras. We discuss our results in the context of developing a deepwater trap fishery for lionfish and provide suggestions for future research on lionfish traps and novel harvest technologies.
2 Materials and methods
2.1 Traps
Lobster traps used in this study were the permitted design under the US code of federal regulations 50 CFR 622.405 for commercial spiny lobster harvest. Their dimensions were 0.6 × 0.9 × 0.6 m (W × L × H), and they were constructed from wooden slats. A single plastic funnel, which allows lobster and fish to enter the trap, was located at the top center of each trap (Figure 1A). Sea bass traps also followed a federally permitted design (50 CFR 648.144) and had dimensions of 0.6 m × 0.9 m × 0.6 m (Figure 1B). The lobster and sea bass traps were both weighted with approximately 20 kg of concrete to ensure rapid sinking. Both lobster and sea bass traps were baited with 15 Atlantic mackerel (Scomber scombrus) per trap following Collins (1990). The mackerel were placed in a mesh bag (for lobster traps) or wire chamber (for sea bass traps) affixed inside each trap. A subsurface syntactic foam buoy was necessary for trap recovery and for affixing the remote time-lapse camera units centered 2 m above each trap. The buoy was attached by threading a nylon line through the buoy’s center and tying the ends to two opposing corners at the top of each trap.
Gittings traps (Figure 1C) were constructed of nylon mesh netting (#420 green knotless, 22 mm diameter mesh) around two semicircular hinging rebar frames as described by Harris et al. (2020b). These traps were designed to descend vertically and then spring open along a central axle hinge when the trap contacted the seafloor. An example video of a Gittings trap opening during deployment is provided at https://youtu.be/XlyNuLxEqgQ. A 71 cm × 75 cm piece of positively buoyant vinyl lattice (Core Molding Technologies) with 2.5 cm openings was attached along the midline. This “white fencing” provides vertical relief to act as a fish aggregating device for attracting lionfish within the footprint of the trap. No bait was used in the Gittings traps. Upon trap retrieval, constant tension applied to the buoy line from the surface causes the semicircular frame to hinge vertically and enclose fish within the trap’s footprint in the mesh netting.
Three modifications were made to the Gittings trap design to address issues identified by Harris et al. (2020b). First, the mesh area of the net was increased from 3 to 4 m2 to allow the net to billow during closure in an attempt to minimize the number of escaping lionfish. The trap circumference remained unchanged. Second, a larger subsurface float (20 cm diameter trawl buoy, 2.5 kg buoyancy, Seattle Marine and Fishing Supply) was used to increase drag and keep the trap oriented vertically during descent. The goal was to increase deployment success by helping the trap to land upright and facilitate opening. Third, the lines just below the subsurface buoy were separated by a 40 cm long, 2.5 cm diameter PVC bar approximately 50 cm from the subsurface float to allow the ROV to attach a surface line for retrieval (shown in Figure 1C).
2.2 Study location and design
Our experimental trap deployments were conducted near northeastern Gulf of Mexico (nGOM) mesophotic natural reefs in depths of 38–78 m. Traps were deployed within four distinct reef areas: the Trysler Grounds, Yellow Gravel, Pensacola Edge, and the Desoto Canyon Rim (Figure 2). The former three are located in the west subregion and the latter is in the east subregion. Ten deployment trips were equally divided between the two subregions (n = 5 each) with 87.2 degrees longitude demarcating east vs. west subregions.
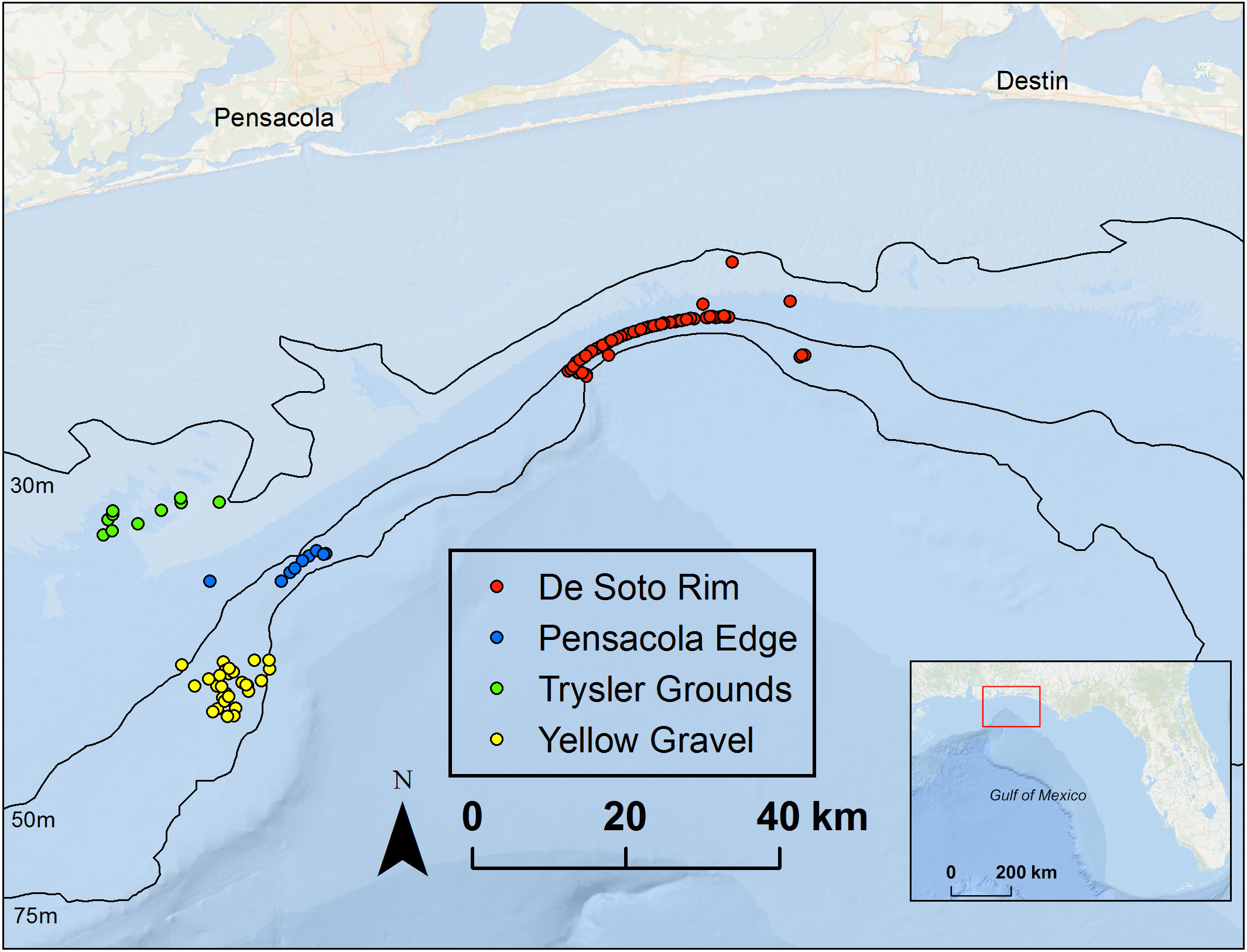
Figure 2 Experimental lionfish trap deployment locations near mesophotic reef sites in the northeastern Gulf of Mexico deployed at depths of 38–78 m.
Traps were deployed at 12 reef sites during each trip. Reef sites for trap deployment were selected based on real-time acoustic sonar collected from echo sounders on board the chartered fishing vessels. Site characteristics were ground-truthed and categorized from video collected with the ROV. Each trap deployment site was ≥0.5 km from adjacent deployment sites within the same reef area, which exceeds the typical lionfish home range estimates (Bacheler et al., 2015; McCallister et al., 2018; Dahl and Patterson III, 2020). The trap “configuration” consisted of 1, 2, 3, or 4 traps of a single type deployed at a given site, with each trap type and configuration combination deployed once among the 12 sites per trip (Figure 3). The trap type and deployment combination was randomly treated per reef site.
Mesophotic reef sites comprised of expansive limestone hardbottom habitat, including rocky ledges ranging from 0.5–6.0 m in relief and surrounded by sand. Various ahermatypic corals (Orders Alcyonacea and Antipatharia), sponges, and dense fouling communities contributed to the structural complexity that provided refugia for diverse fish and invertebrate communities (Etnoyer et al., 2016; Garner et al., 2019). Lionfish were first reported in this region in 2010 (Dahl and Patterson III, 2014; Switzer et al., 2015). Within several years, their populations rapidly expanded throughout the northern Gulf of Mexico, with many reefs having the highest densities reported in the invaded range (Dahl et al., 2019; Campbell et al., 2021; Blakeway et al., 2022).
2.3 Data collection
Video surveys were conducted at each site immediately before trap deployment (pre) and again immediately before trap retrieval (post) to estimate pre- and post-deployment lionfish densities and fish community structure. Video transects were conducted using a VideoRay Pro4 mini ROV (Patterson et al., 2014; Harris et al., 2019). The ROV was flown 1–2 m above the seafloor, depending on visibility, with a GoPro Hero5 camera (2.7k resolution and 120 fps) mounted to the front of the ROV and angled downward at 45°. Lighting was provided by the ROV’s twin 20-watt halogen lights. Four orthogonal 25-m transects were flown away from a central point demarcated with a 5.5 kg downrigger weight attached to the ROV tether such that 500–1,000 m2 of the seafloor was surveyed at each site depending on ROV height off the bottom. Video files from the ROV transects were analyzed on a high-definition monitor in the laboratory. Each fish was identified to the lowest taxonomic level possible and enumerated. Total taxa-specific counts at a given site were divided by the estimated area surveyed to estimate fish density. A 7.5-cm red laser scaler integrated into the ROV system enabled fish length to be estimated if both lasers struck an individual at an angle of incidence <20° from perpendicular. Bias correction for laser-scaled fish followed the method of Garner et al. (2021).
Traps were deployed from the surface and allowed to freefall to the bottom. After the deployment period, traps were retrieved following the method of Tarnecki and Patterson (2020). Deployment success (% open) for Gittings traps was observed during the post ROV survey. To retrieve the traps, a detachable surface line affixed to the ROV was hooked to the trap’s buoy line, either just below the PVC bar for the Gittings traps or just below the subsurface float on the lobster and sea bass traps. The ROV was then flown in reverse until the mooring hook detached from the mount, and its spring-loaded latch closed. This connection technique is demonstrated at https://doi.org/10.1371/journal.pone.0235321.g003 (Tarnecki and Patterson (2020)). Once the surface line is connected to the trap, traps were raised to the surface at a constant speed of approximately 1 m/s with an electric winch (Arctic 900-watt vertical capstan, 50-amp draw) attached to a 2 m tall davit. For the Gittings traps, this line retrieval by the winch closed the trap before raising it to the surface. Upon retrieval of each trap, all captured fishes were euthanized, placed in labeled mesh bags, and put on ice for storage and transport back to the University of Florida’s Fisheries Ecology Laboratory. Here they were identified to species, weighed (kg), and measured to the nearest mm.
Recruitment of fishes to traps was monitored with remote time-lapse camera units attached to one trap at each trap deployment site. Each camera unit included a GoPro Hero4 camera paired with a CamDo Blink time-lapse controller housed within a GolemGear deep water housing. Cameras were deployed on subsurface buoys 2 m above the traps and programmed to record 10 seconds of video every hour during daylight hours. For each time-lapse video, the maximum number of individuals of each species (or higher-order taxa) observed in any one video frame was counted as the “minimum count”, which is the minimum number of a given taxon present in a still frame of the video. Fish counts were categorized as “inside” (i.e., recruited inside the lobster and sea bass traps or within the 2-m diameter footprint area of the Gittings traps) or “outside” of a trap (i.e., observed within the video frame but not inside the trap). Video counts were taken from one diel period per day (i.e., dawn, midday, and dusk for each day), resulting in 1714 video samples. Dawn videos occurred within two hours of sunrise and were selected as the first video of a given day where there was enough light for the video reader to identify fishes. Similarly, dusk videos were those occurring within two hours of sunset and were selected as the last video of the day with enough light to identify fishes. Midday videos were taken at noon.
2.4 Animal collection and gear use authorization
Methods for data collection were reviewed and approved by the University of Florida’s Institutional Animal Care and Use Committee (UF IACUC Protocols #201810225 and #201810394) in accordance with relevant guidelines and regulations by American Veterinary Medicine Association. Euthanasia of fishes was performed via pithing the braincase 3–10 mm posterior of the center of the eyes with a commercial rabbit pither (F. Dick Prod. No. 9 0232 000). Authorization for experimental trap deployments in US federal waters and the collection of fishes for research purposes was granted by a Letter of Acknowledgment from the NOAA Fisheries Southeast Regional Office following the definitions and guidance at 50 CFR 600.10.
2.5 Data analysis
We categorized the trap catches and time-lapse video fish counts as lionfish, fishery species (i.e., federally managed species), or non-fishery species (i.e., all other fishes). A full species list with category designations is provided in the Supplementary Table S1. Differences in pre versus post trap deployment mean biomass densities (fish per 100 m2 as averaged between pre and post deployment ROV surveys) for lionfish, red snapper, gray triggerfish, lane snapper, red porgy, and scamp were assessed with paired t-tests.
A generalized linear modeling (GLM) framework was used to test whether the deployment or reef site factors affected catches. Models were computed separately for each fishery category: i.e., lionfish catches, fishery species catches, and non-fishery species catches (Eq. 1). Given the number of zeros in our catches, a zero-inflated approach was used whereby a logistic regression (i.e., the binomial component) estimated the probability of zero catch and a separate regression model (i.e., the positive component) estimated the relationship between the non-zero catch per unit effort (CPUE in g) and the predictors. Quantile-quantile plots indicated non-zero data were overdispersed for the three response categories and best fit by a negative binomial distribution; thus, zero-inflated negative binomial (ZINB) models were used. Specifically, the ZINB positive component (Eq. 1A, log-linked negative binomial GLM) assessed the effects of trap type (lobster, Gittings, sea bass), trap configuration (1, 2, 3, or 4 traps), soak time of traps at the given site (numeric days), lionfish density at the site (per 100 m2) , site depth (m), site relief (maximum reef height in m estimated from ROV surveys), and region (east or west). The ZINB binomial component (Eq. 1B, logit-linked logistic GLM) assessed whether the deployment factors of trap type and trap configuration affected the probability of zero catches.
A similar zero-inflated GLM approach was applied to the time-lapse video data to assess the significant predictors for lionfish recruitment, i.e., the number of lionfish inside the footprint of the Gittings trap or inside the container of the lobster and sea bass traps. Similar to the catch data, the QQ plots indicated that the data were zero-inflated and overdispersed, thus ZINB models were used. The ZINB positive component (Eq. 2A, log-linked negative binomial GLM) assessed whether trap type, lionfish density, and diel period (dawn, midday, or dusk) had significant effects. The effect of these factors specific to each experimental trap type was examined by testing the interaction of trap type × diel period and the interaction of trap type × lionfish density. The ZINB binomial component (Eq. 2B, logit-linked logistic GLM) assessed whether trap type or lionfish density affected the probability of zero lionfish counts. To account for repeated measures from videos taken during a given deployment at a reef site, reef site was included as a random effect (random intercept) in the models and assumed to be normally distributed with a mean of zero and variance σ2.
Laplace approximation was used to estimate likelihood and test statistics based on GLM fitting and inference protocols (Bolker et al., 2009, Crawley, 2015). Effect sizes were calculated by exponentiating model coefficients, and confidence intervals were estimated from the unconditional standard error to account for model uncertainty (Burnham, 2002; Grueber et al., 2011). Plots and models were built in R (version 4.1.1). The ZINB models were developed with the pscl (Jackman, 2020) and glmmTMB (Brooks et al., 2017) packages. Data manipulation and plotting used the ggplot (Wickham, 2016) and dplyr (Wickham, 2021) packages. Our data, code, and analyses are available at https://github.com/holdenharris-NOAA/Lionfish-Trap-Testing.git and included in the supplementary materials.
3 Results
3.1 Trap deployments and operations
Data collection was conducted from February to June 2019. Three hundred traps (n = 100 each of lobster, sea bass, and Gittings traps) were deployed and retrieved during ten trap deployment field trips (n = 5 trips per region) at a total of 120 reef sites (n = 60 sites per region). The mesophotic reef sites ranged in depth from 38 to 78 m, and their maximum estimated relief ranged from 0.2 to 6.0 m. The average soak time for traps was 8.35 days and ranged from 5 to 15 days.
Trap deployments targeted sand bottom near limestone reefs to avoid damage and entanglement in reef structure and maximize the opening success of Gittings traps. However, traps occasionally landed on primary or proximal reefs. Among trap deployments monitored with camera units, 23% of lobster, 23% of sea bass, and 16% of Gittings traps landed on primary or proximal reef structures (Figures 4A–C). Of the 100 Gittings trap deployments, 80 deployed successfully and 20 failed to open. Traps that failed to open often appeared lodged in a crevice or ledge, or were entangled on reef structures. Some Gittings traps failed to open on sand (Figure 4D), which appeared to occur when the mesh net entangled on the metal frame.
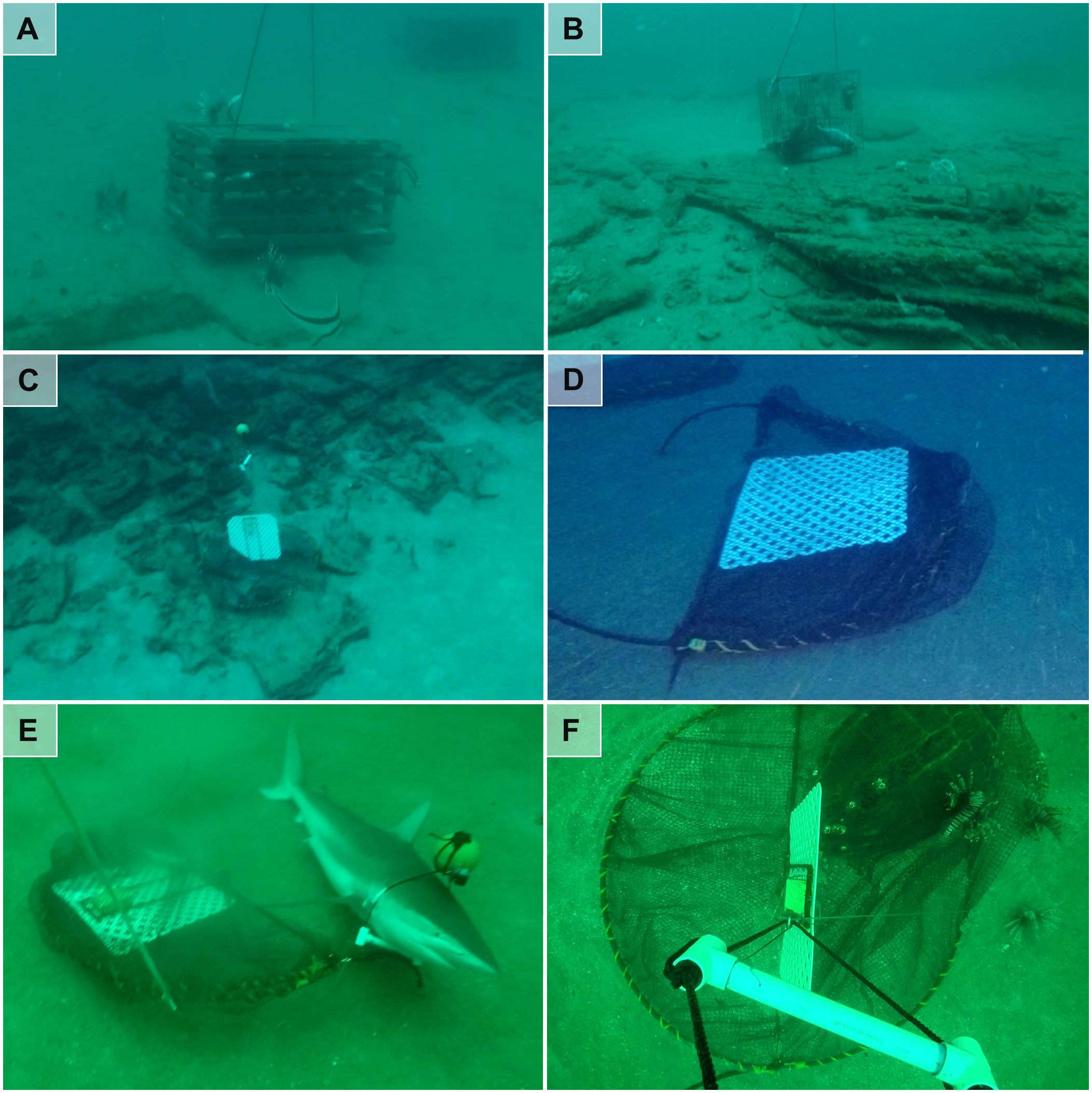
Figure 4 Operational performance issues observed while testing experimental lionfish traps near northeastern Gulf of Mexico mesophotic reefs. Photos show unintended placement on natural reef structure by (A) lobster, (B) sea bass, and (C) Gittings traps, as well as (D) a Gittings trap that failed to open upon contact with the bottom, (E) an unexpected megafauna entanglement of a dusky shark (Carcharhinus obscurus) in the buoy line, and (F) a green sea turtle (Chelonia mydas) that crawled under a Gittings trap and became entangled in the trap’s netting. Photos by the authors.
The experimental trap testing resulted in the unexpected entanglement of two non-fish megafauna species by Gittings traps. First, a dusky shark (Carcharhinus obscurus) attempted to swim through the triangular section of the buoy line formed by the PVC bar and the float of a Gittings trap (Figure 3E). The girth of the approximately 2.5-m shark was too large to pass through the opening, and the shark dragged the trap about 50 m from its original GPS coordinates. We observed the shark ventilating its gills and moving while entangled in the buoy line on the seafloor. The live shark freed itself during trap retrieval and was not viewed lying on the bottom during subsequent trap retrievals at the same site, although its ultimate fate is unknown. The second megafauna bycatch event involved a green sea turtle (Chelonia mydas) that became trapped in the mesh of a Gittings trap and drowned (Figure 4F). Video from the remote time-lapse camera on the trap indicates the turtle crawled underneath the frame during the second night of deployment and could not escape. The mortality was reported at the time of occurrence to the NOAA Fisheries Southeast Regional Office.
3.2 Fish densities pre versus post trap deployment
In total, 49,489 individual fish were observed among the pre (n = 120) and post (n = 120) deployment ROV video samples. Ninety-four different reef fish taxa were represented with 86% identified to species (n = 81). The most abundant species identified among ROV surveys were vermilion snapper (Rhomboplites aurorubens), round scad (Decapterus punctatus), tomtate (Haemulon aurolineatum), yellowtail reef fish (Chromis enchrysura), short bigeye (Pristigenys alta), red snapper (Lutjanus campechanus), and mackerel scad (D. macarellus). The total lionfish biomass estimated among all the ROV surveys was approximately 70 kg, with a mean ( ± SD) of 0.29 kg ( ± 0.35) per site. Fish density was typically lower in the post deployment video samples compared to the pre deployment video for lionfish (Figure 5A) and the predominant bycatch fishes of red snapper, gray triggerfish, lane snapper, red porgy, and scamp (Figures 5B–F); however, none of these differences were significant with all p-values from the paired t-tests >0.05.
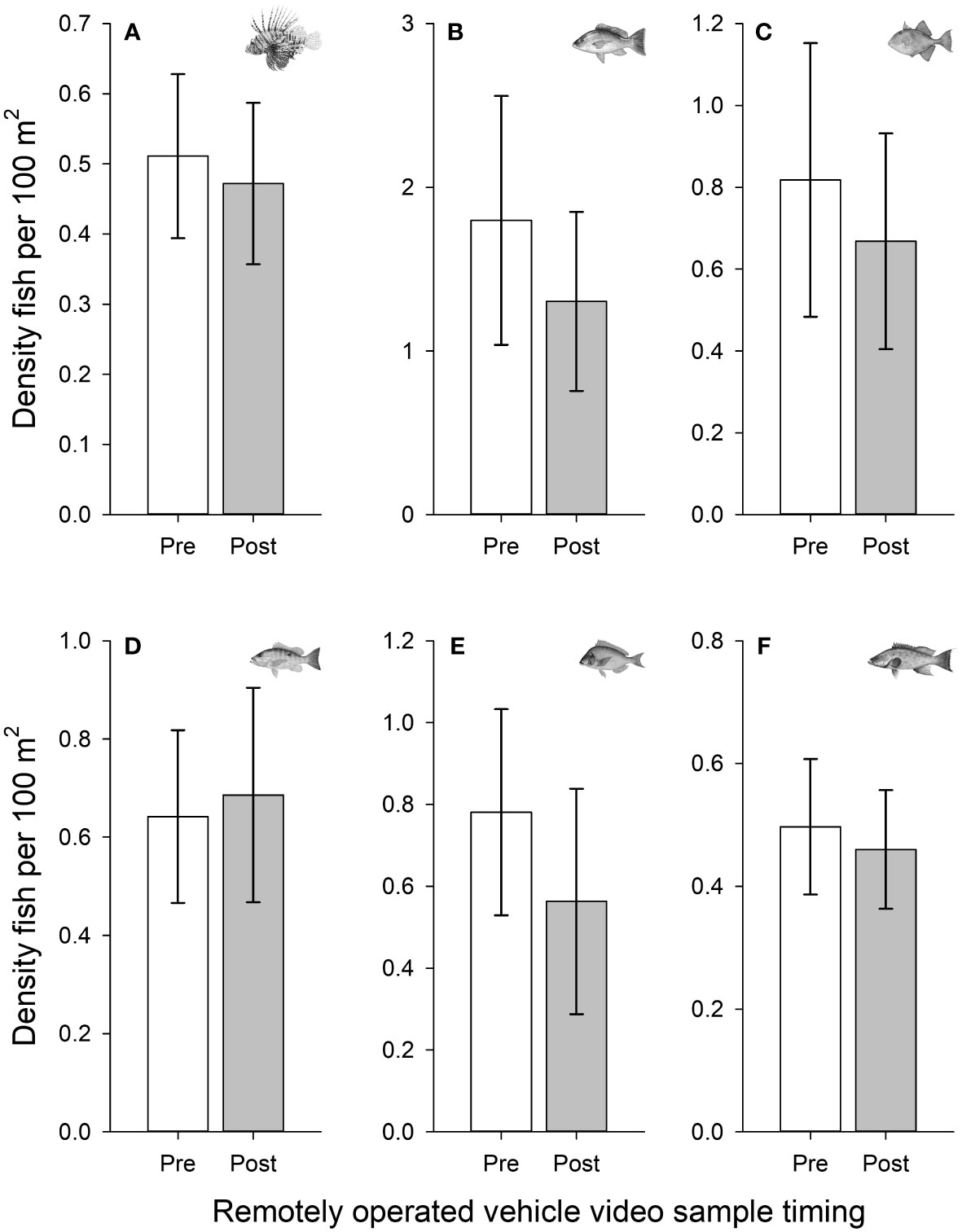
Figure 5 Fish densities pre-deployment (pre) and before retrieval (post) of experimental lionfish traps. Mean (± 95% CI) densities are shown for (A) lionfish, (B) red snapper, (C) gray triggerfish, (D) lane snapper, (E) red porgy, and (F) scamp (note scale differences among panels). Densities were estimated via remotely operated vehicle video surveys conducted at 120 trap deployment sites on northeastern Gulf of Mexico mesophotic reefs.
3.3 Trap catches and CPUE
In total, 660 fish were captured from the 120 reef sites. Catches included 39 invasive lionfish, 538 individuals in the native fishery group (among 14 species), and 83 individuals in the native non-fishery group (among 20 species). By mass, a total of 9.8 kg of lionfish, 46.3 kg of non-fishery species, and 429.3 kg of fishery species were captured among all traps.
The Gittings traps had the highest lionfish catches and lowest native species catches (Figure 6A) with a total of 24 lionfish (4.4 kg), five non-fishery individuals (0.8 kg total), and zero fishery species. The total biomass of lionfish captured in Gittings traps was over 5.5 times the total biomass of native species that they captured. In comparison, lobster traps caught 8 lionfish (3.0 kg) and sea bass traps caught 7 lionfish (2.4 kg), and their catches were dominated by native species bycatch (Figures 6B, C). Lobster traps captured 70 native fish (both fishery and non-fishery species) with a total biomass of 99.4 kg, which was 33 times higher than the total lionfish biomass captured by lobster traps. The bycatch from sea bass traps was higher still: 542 native fish were captured with a total biomass of 363.5 kg, which is 151 times the biomass of lionfish they captured. Most of the bycatch in sea bass traps were fishery species (Figure 6C), including (in order of abundance) red snapper, lane snapper (L. synagris), gray triggerfish (Balistes capriscus), red porgy (Pagrus pagrus), and scamp (Mycteroperca phenax). Total catches by trap type are provide in Supplementary Table S2.
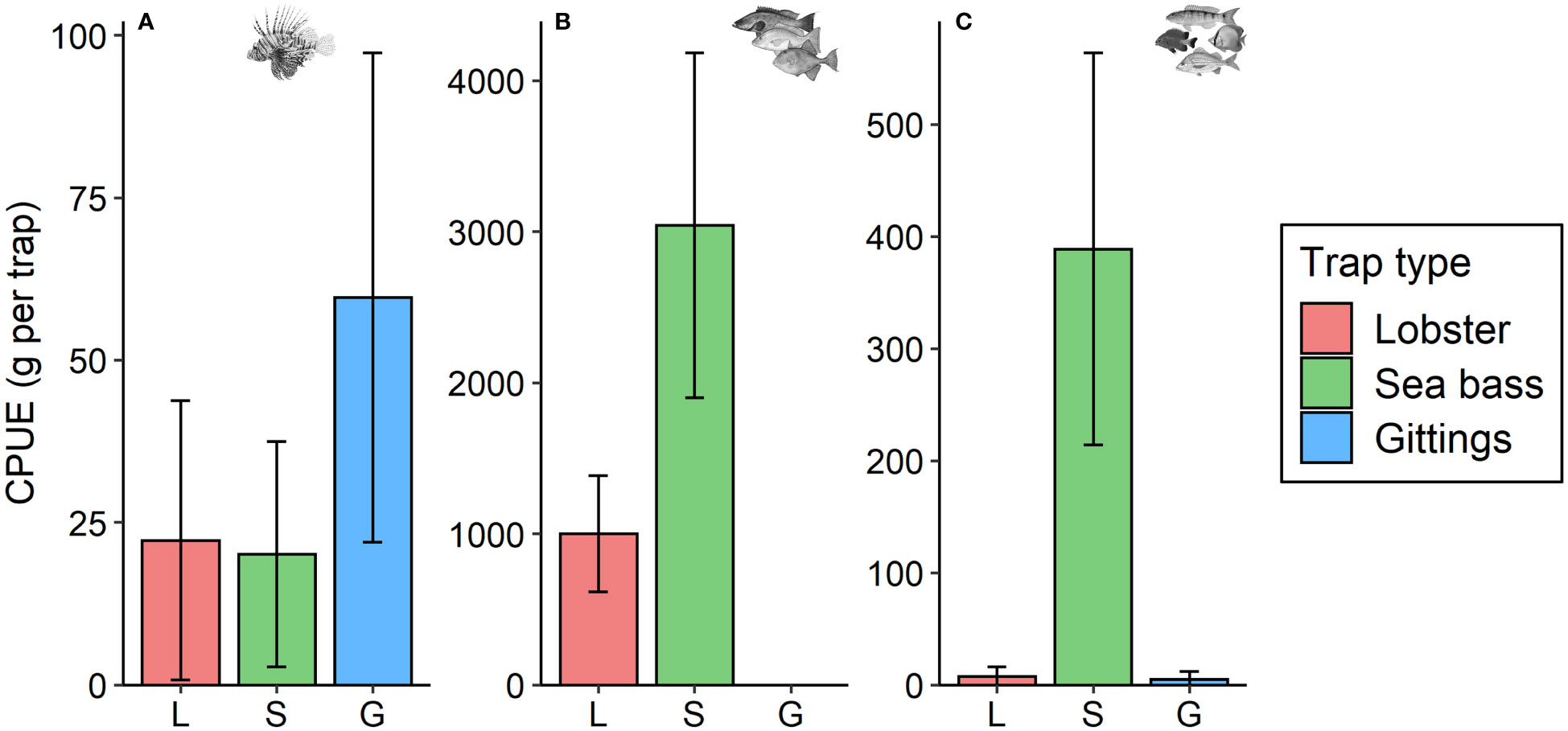
Figure 6 Catch per unit effort (CPUE) by trap type. Mean (± 95% CI) CPUE per trap is shown for (A) lionfish, (B) native fishery species, and (C) native non-fishery species captured with each of three experimental trap types (lobster, sea bass, or Gittings traps) deployed near mesophotic reefs in the northeastern Gulf of Mexico. Note scale differences among panels.
Results from the ZINB catch models (Table 2, analysis deviance table provided in Table S3) primarily showed how trap type and configuration (i.e., the number of traps of a given type at the site) affected the probability of catch (the binomial ZINB component) and the CPUE biomass (the positive ZINB component) for lionfish, fisheries species, and non-fisheries species. No significant relationships were identified between soak time, lionfish density, reef relief, or region and any of the response variables. The only reef site factor found to affect catch rates was a negative relationship (3% per m) between depth and fisheries species CPUE. The likely driver for this is the high catches of gray triggerfish and red snapper caught in sea bass traps deployed at the shallower reef sites (< 50 m), particularly in the Trysler Grounds.
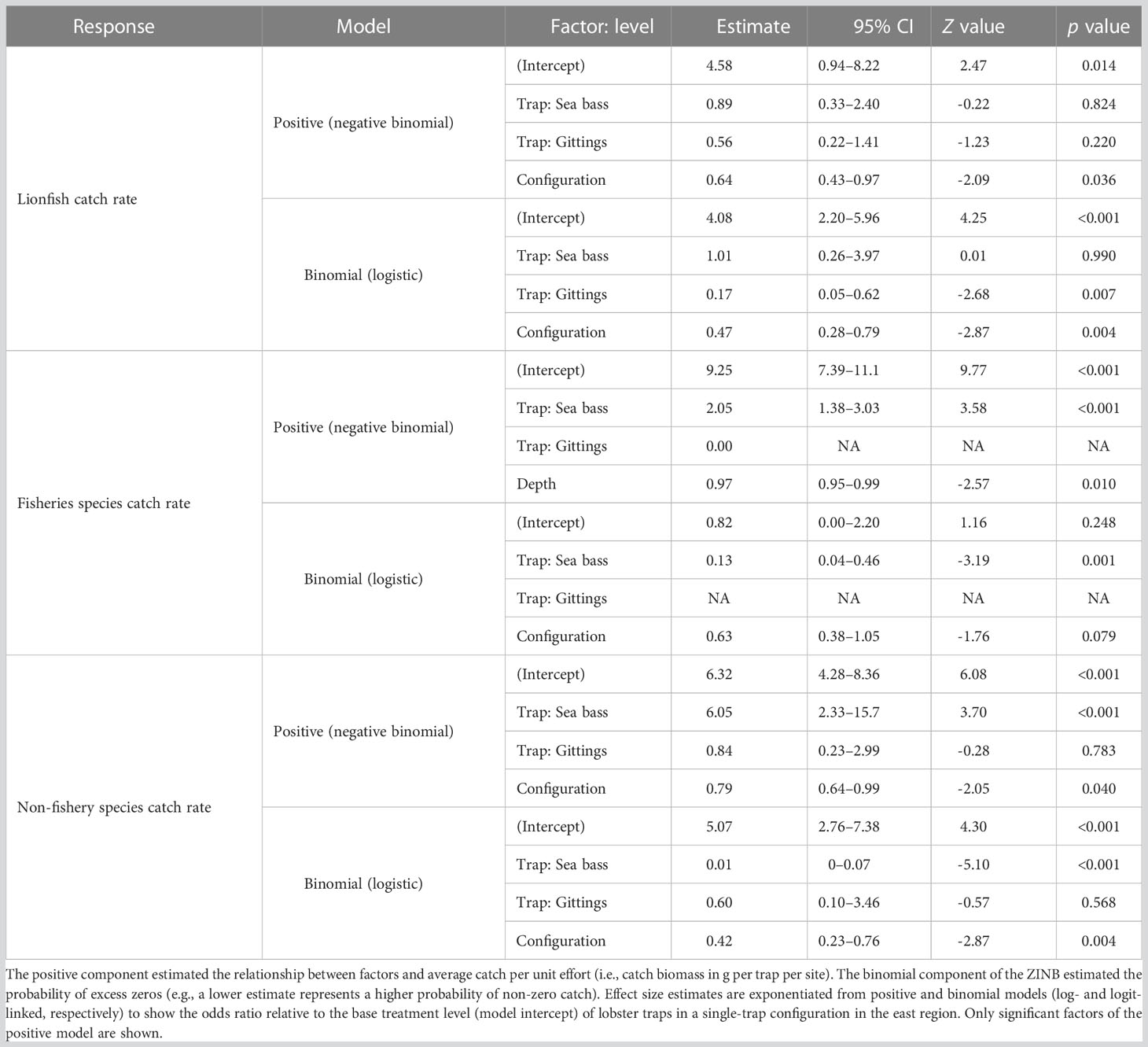
Table 2 Zero-inflated negative binomial (ZINB) model results assessing the effects of trap deployment and reef site factors on catches of lionfish, fisheries species, and non-fisheries species.
Our analysis indicated that trap configuration had a similar effect size in the binomial component of 42%–63% for all three responses (Table 2). In other words, for lionfish, fishery species, and non-fishery species catches, each additional trap placed decreased the chance of non-zero catch by approximately 50%. However, the effect configuration in the positive component of the ZINB models were different between the three response groups. Adding more traps to configuration decreased the CPUE for lionfish and non-fishery species, while CPUE per trap for fisheries catches did not decrease with additional traps placed at a site. For lionfish, this indicates that placing more traps at a site decreases the probability of a non-zero catch (in other words, increases the probability of cathing a lionfish at the site) but also decreases the average CPUE per trap when more traps are plaed at the site.
Trap type was not significant in the positive component of the lionfish catch ZINB model, but had a significant effect in the ZINB binomial component. The biomass of lionfish caught per trap was not different among the three trap types, but Gittings traps demonstrated a significantly higher chance of a non-zero catch of lionfish with the Gittings traps having an approximately 83% lower probability of a zero catch than the lobster or sea bass traps. As would be suggested by their high overall catch rates of sea bass traps, these had very low and near-zero probabilities of having zero catches of fisheries and non-fisheries species, respectively, and had 2–6× higher biomass CPUE.
3.4 Trap recruitment to traps monitored by remote time-lapse cameras
In situ lionfish recruitment to traps was estimated from 1714 video samples from remote time-lapse cameras affixed above 86 traps. Lionfish were observed in or near a trap in 545 (26.1%) video samples. Lionfish were more likely to be observed outside versus inside traps regardless of trap type, but this inside:outside ratio varied among the three trap types (Figure 7A). Gittings traps had the highest inside:outside ratio of 1:4 lionfish inside versus outside, compared to 1:32 for lobster traps and 1:86 for sea bass traps. The recruitment of native species inside the footprint of Gittings traps was similar to the recruitment of native species near the lobster and sea bass traps (Figures 7B, C). The fact that only five native species individuals were caught by Gittings traps indicates that these fishes were likely able to escape while the trap was closing.
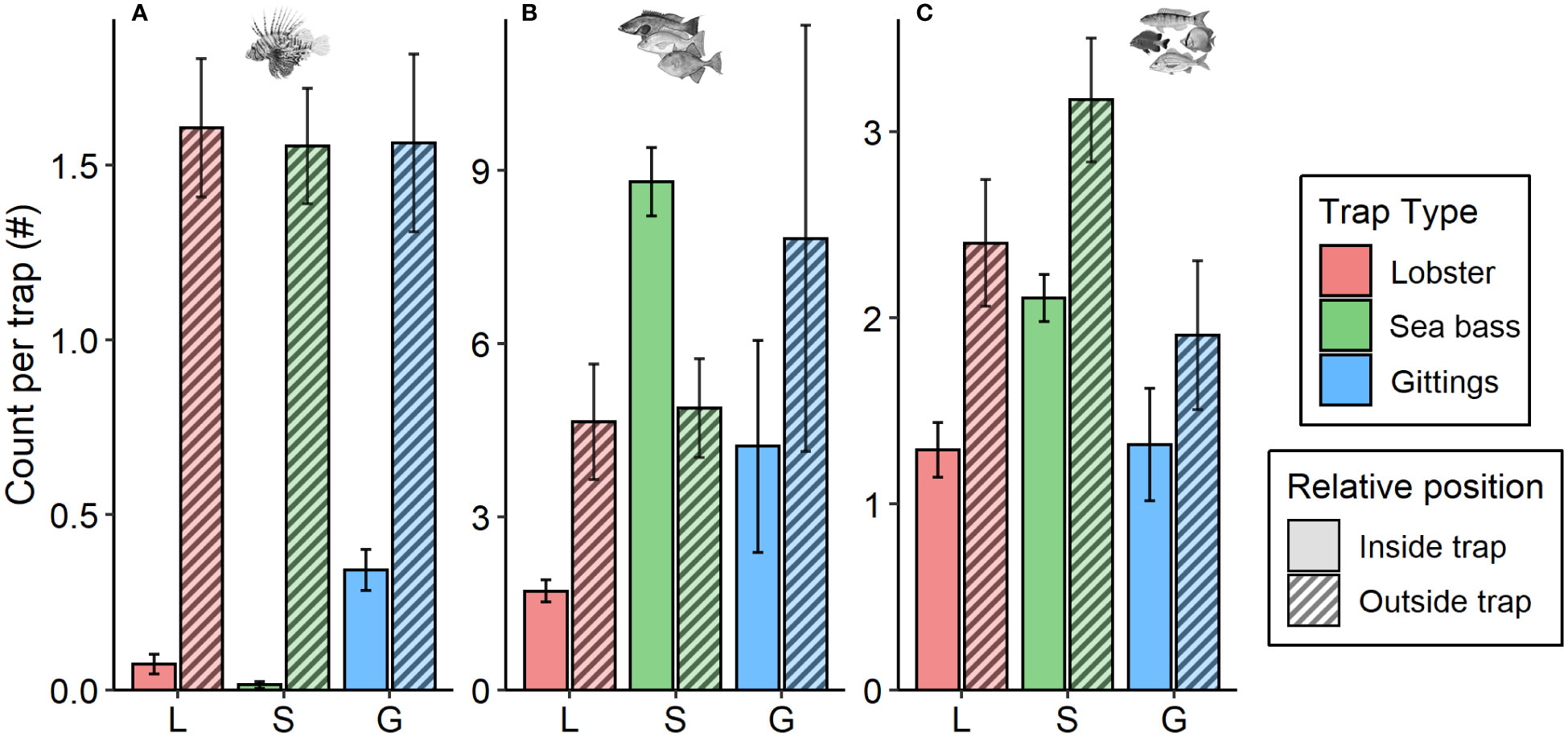
Figure 7 In situ recruitment to traps monitored with remote time-lapse videos. Mean (± 95% CI) fish count per trap is shown for (A) lionfish, (B) native fishery species, and (C) native non-fishery species (note scale differences). Fishes were categorized as inside the trap (i.e., inside the lobster or sea bass trap container or within the frame of the Gittings trap) or outside the trap (i.e., visible in the video but not within a lobster or sea bass trap container nor within the footprint of the Gittings trap frame).
The time-lapse video data indicated several trends with respect to trap type, soak time, lionfish density, and diel period. Lionfish recruitment over time was non-linear and showed a relative peak after two days (Figure 8A). Higher ambient lionfish density was related to higher lionfish recruitment to Gittings traps, but had no significant effect on recruitment of lionfish to the lobster or sea bass traps (Figure 8B; Table 3, analysis deviance table provided in Table S4). Diel period also had a significant effect on recruitment to the Gittings traps (Figure 8C); compared to the midday period, mean lionfish recruitment to Gittings traps was 53–78% higher at dawn or dusk (Figure 8C; Table 3).
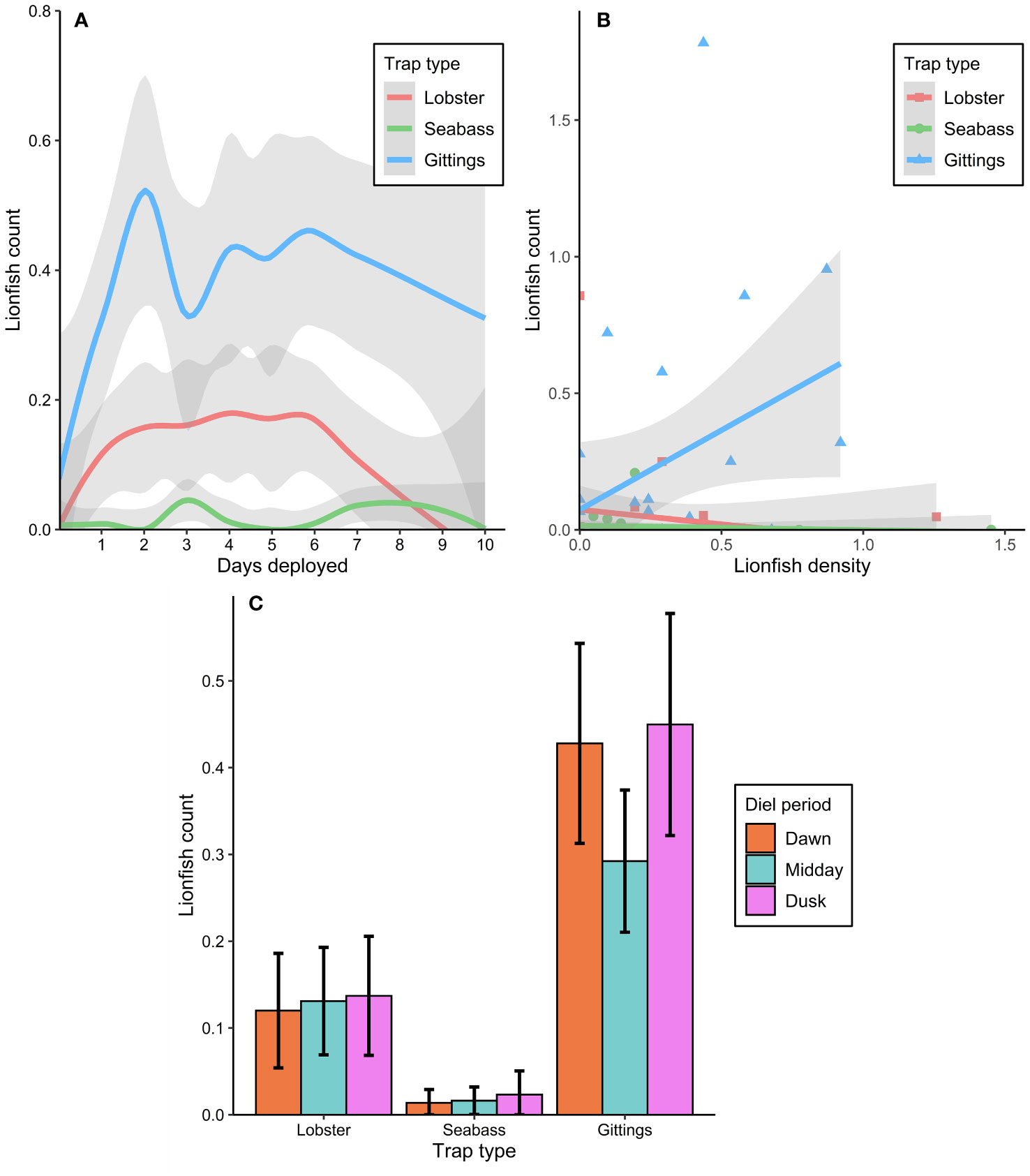
Figure 8 Effects of trap type, soak time, lionfish density, and diel period on lionfish recruitment to traps (i.e., number of lionfish inside the footprint of the Gittings trap or inside the container of the lobster and sea bass traps) as measured by remote time-lapse video cameras. (A) Non-parametric locally estimated scatterplot smoothing curves (± 95% CI) for mean lionfish recruitment plotted against soak time and by trap type. (B) Mean (± 95% CI) lionfish recruitment plotted against a site’s lionfish density as estimated by remotely operated vehicle surveys, with regression lines for each trap type. (C) Bar plot of mean (± 95% CI) lionfish recruitment by trap type during dawn (within 2 h of sunrise), midday (noon), or dusk (within 2 h of sunset) diel periods.
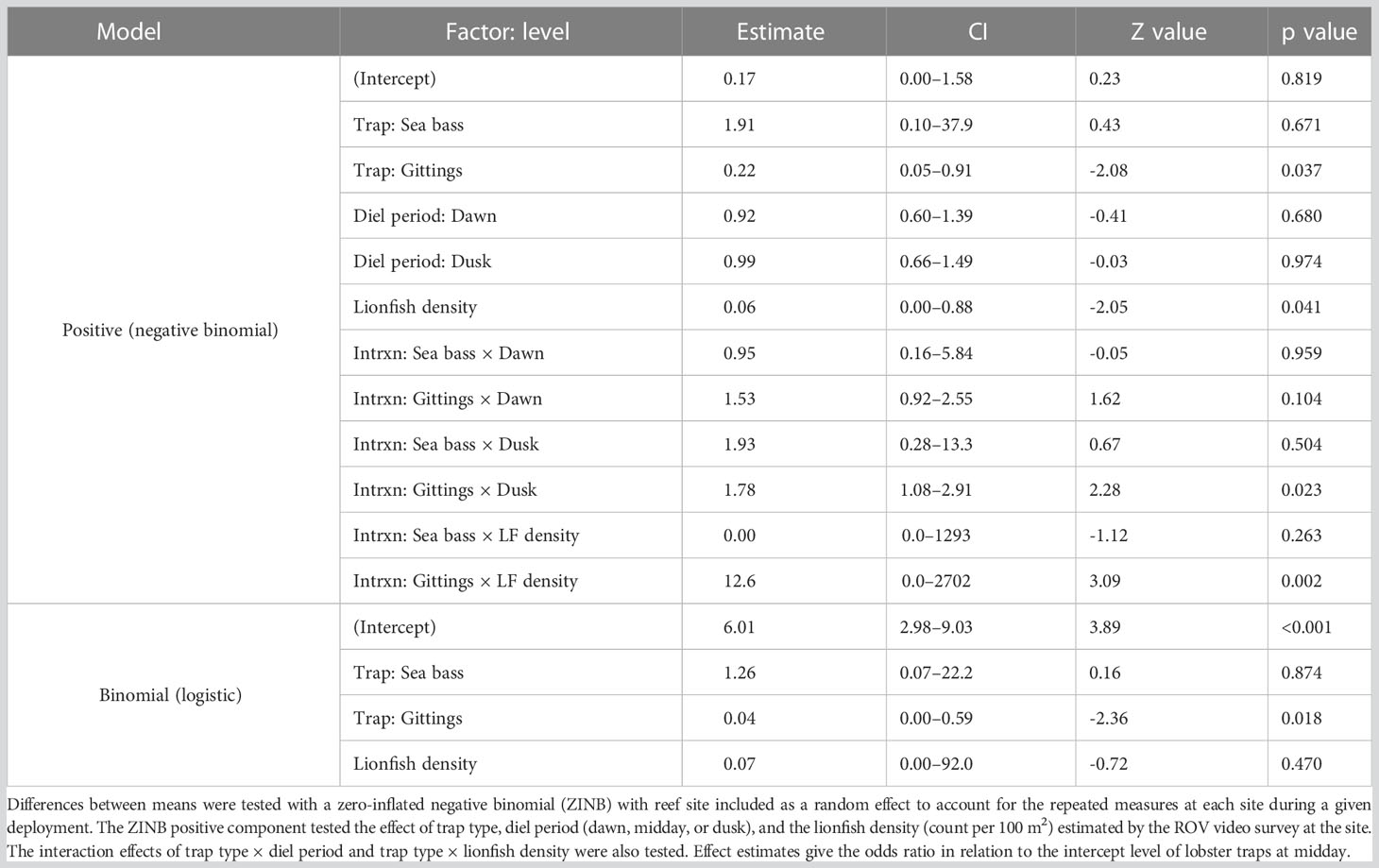
Table 3 Lionfish recruitment (in situ observation of fish within the trap structure during deployment) model outputs estimated from time-lapse video fish counts.
4 Discussion
This study expands on previous experimental tests of lionfish traps in the nGOM (Johnston et al., 2015; Gittings, 2016; Harris et al., 2020b) by considerably increasing sampling effort, testing multiple trap types, and testing near deepwater natural reefs (approximately 40–80 m). Three hundred trap deployments were conducted where a potential commercial fishery could target lionfish inaccessible to conventional SCUBA diving. Overall, Gittings traps were relatively efficient at harvesting lionfish in terms of catch-to-bycatch ratios (5:1 kg lionfish: native species), while lobster (1:33 kg lionfish: native species) and sea bass traps (1:151 kg lionfish: native species) biomass catch ratios were grossly inefficient. However, further RD&T will be needed for lionfish traps before a design could serve as a fishing gear that could allow a viable commercial fishery. Gittings traps demonstrated several critical operational issues, including entangled megafauna, as discussed below. Despite having the highest lionfish catches among the three trap types tested, they also had low lionfish CPUE.
The low catch rates may be explained by the low densities of lionfish we observed in the ROV surveys, which averaged less than 0.3 kg of lionfish per site. For comparison, both lionfish catches and lionfish densities were approximately 10 times higher for Gittings traps tested near nGOM artificial reefs in 2017 (Harris et al., 2020b). This study was also conducted less than two years after high numbers of lionfish in the region presented ulcerative skin lesions (Harris et al., 2018; Cody et al., 2023) and less than a year after lionfish densities on nGOM natural reefs declined by approximately 75% (Harris et al., 2020a). Two results from our experiment suggest lionfish catches could increase if lionfish densities rebound in the region or if traps were tested in areas of higher lionfish densities. First, we observed a positive relationship between lionfish density and their recruitment to the Gittings traps. Second, we found that deploying more traps of the same type at a given site concurrently increased the probability of catching a lionfish at the site and also decreased the average biomass per trap from the site. In contrast, deploying more seabass or lobster traps at a site did not decrease the biomass catch per trap of fishery species. This could indicated that most of the available lionfish are being caught (i.e., catch saturation), while, in comparison, a relatively small proportion of fishery species biomass were being caught per trap. Still, it remains unclear if traps deployed near higher lionfish densities could allow for a profitable commercial fishery, and is the subject of further discussion below.
4.1 Research, development, and testing recommendations for lionfish traps
The high bycatch rates of fishery species by sea bass traps suggest their use is likely untenable for a fishery that primarily targets lionfish, at least how they were deployed in this study. The use of organic baits appeared to attract native piscivorous and protected fishery species (predominantly snappers, groupers, and triggerfish) rather than lionfish. The reasoning for our decision to use organic baits for the lobster and sea bass traps was because we tried to replicate the current commercial use of these traps; however, we suggest that future testing of similar traps be performed without bait.
For the lobster traps, a modified wire spiny lobster trap may present an alternative gear to the wooden slat lobster traps that we tested. Preliminary experimental tests with lobster traps have demonstrated that lionfish catches may be increased and bycatches decreased by using a modified funnel design with a larger opening and adding escape gaps (Pitt and Trott, 2013; Pitt and Trott, 2015; Hutchinson et al., 2019). Using live lionfish as bait in wire lobster traps also increased lionfish catches and lowered bycatch (Hutchinson et al., 2019), although it is unknown whether such live bait could be scaled for commercial use.
The potential for Gittings traps to entangle marine turtles could jeopardize their ability to receive legal permitting and social licensing (Demuijnck and Fasterling, 2016). Six of the seven species of Chelonioidea marine turtles are found within the western Atlantic range of lionfish (Braun-McNeill and Joanne Epperly, 2002), all of which are listed as vulnerable, endangered, or critically endangered by the International Union for Conservation of Nature (Mast and Casale, 2020) and protected under the Convention on International Trade in Endangered Species. Two design modifications that could prevent turtle entanglement include i) incorporating rigid plastic mesh panels into the netting or ii) attaching several bars across each jaw. These could prevent turtles from moving upward into the netting from below and allow a turtle that crawls under a trap to escape out the side of the trap. We expect that a repeat of the dusky shark entanglement in a trap used for commercial purposes is unlikely. The shark passed through the opening made in the subsurface line for the ROV to attach a mooring hook; a commercial fishery would likely retrieve the trap using a surface line rather than an ROV and thus obviate the need for the triangular section. Nevertheless, consideration may be needed to reduce the potential for marine mammal entanglement with the surface line, as fishing gear entanglements are a primary cause of unnatural mortality in whales (van der Hoop et al., 2013; Hamilton and Baker, 2019; Brown and Niedzwecki, 2020).
Other operational aspects may also need improvement or testing for the Gittings traps. The deployment success of Gittings traps was 80%, which represents a 12% improvement over that reported for Gittings traps tested in Harris et al. (2020b). We infer that this improvement may be from using a larger subsurface buoy to create more drag and keep the trap vertical during descent. Still, a 20% failure to open rate would likely be too inefficient for commercial use. We found that 16% of Gittings traps landed on reef structures. This was a little less than that of lobster traps (23%), which are actively deployed by commercial fishers near coral reefs. We also observed that traps were unmoved between deployments. Commercial fishing operations would likely use surface buoys to locate and retrieve traps. We did not employ surface buoys and their use may require additional testing. Experimental tests of wind-driven movement of lobster traps indicate that movement is much higher for buoyed-versus-unbuoyed lobster traps and that trap movement decreases for traps deployed at deeper depths (Lewis et al., 2009). Deploying traps connected in series via a trawl line would require fewer surface buoys than single traps and may be less prone to wind- or wave-driven movement, particularly with weighted marker buoys.
Analysis of the time-lapse video data provides insights into ways to potentially increase lionfish catch rates. Lionfish were frequently observed in the vicinity of all three trap types and at similar rates, but lionfish were more likely to recruit inside the footprint of the Gittings traps than to enter the lobster or sea bass traps. Lobster traps attracted the highest number of lionfish nearby, but most did not enter the trap. If the use of modified funnels for lobster traps increased this inside:outside ratio, they could show potential to be viable lionfish trapping gear. Even for the Gittings traps, which had the highest inside:outside ratio, approximately 4 times more lionfish were observed outside of Gittings traps than within the trap footprint. Future trap testing could identify ways to attract lionfish closer to the trap center of a Gittings trap. To our knowledge, different fish aggregating structure designs, trap sizes, lights, or sounds to better attract lionfish have not been tested. Lionfish escapement during Gittings trap retrieval (i.e., their active escapement during the trap’s closing or ascent) may also have been a continuing issue (Harris et al., 2020b). This escapement could be reduced with harness modifications (e.g., a latch that engages after trap closure) or by using a hydraulic trap puller to close the trap faster, and keep it closed more securely, than the electric winch used in this study.
Time-lapse camera data also indicated that lionfish recruitment to traps was rapid and that the optimal soak time for traps was approximately two days. Moreover, we found that recruitment to traps was also higher during the crepuscular periods, suggesting that catch rates may be increased by retrieving traps at dawn or dusk. It is unknown whether lionfish are attracted to the traps for shelter or foraging, as lionfish exhibit activity patterns that may correspond to foraging on different prey sources during different diel periods (Dahl et al., 2016; Dahl and Patterson III, 2020). Interestingly, acoustic tagging data on lionfish movement indicates that their foraging is higher during the midday periods for lionfish on nGOM artificial reefs (Dahl and Patterson III, 2020) but higher during crepuscular periods for lionfish on coral reefs (Green et al., 2011; Green et al., 2021; McCallister et al., 2018).
4.2 Considerations for developing a deepwater lionfish trap fishery
A novel lionfish harvesting gear will likely need to integrate into the capacity of a current fishery in order for a lionfish trap fishery to be feasible. For example, a lionfish trap could be effective if it were adopted by the Florida Keys lobster trap fishery, which has a fleet of approximately 650 commercial vessels and about four months of seasonal closures each year (Buesa, 2018). The use of a modified lobster trap may thus be the most easily adopted lionfish trap. Commercial fishers themselves also represent a valuable resource for co-managing fishery resources (Johannes et al., 2000; Hind, 2015) and co-developing new fishing technologies or gear modifications (Kirk et al., 2020; Tookes et al., 2022). Some Florida Keys lobster trappers sell the lionfish bycaught from their traps (Akins et al., 2012), and, in our experience, lobster fishers are interested in further assisting research efforts to develop lionfish trap designs. Continued work should leverage their expertise to co-design innovative fishing gear, field test their use, assess risks, and integrate them into commercial fishing operations.
Despite the challenges identified in this study for developing a deepwater harvest gear for lionfish, traps may still present a viable means to remove deepwater lionfish. Widespread interest in developing deepwater harvest technologies for lionfish has motivated research on lionfish traps in the western Atlantic (Pitt and Trott, 2013; Pitt and Trott, 2015; Hutchinson et al., 2019) and nGOM (Gittings, 2016; Harris et al., 2020b). Testing lionfish traps have also been recommended for the Mediterranean invasion (Kleitou et al., 2021a; Kleitou et al., 2021b; Ulman et al., 2022). We encourage open communication to facilitate efficient research and design iteration. In addition to lionfish traps, robotics technologies have been proposed to control and commercially exploit deepwater lionfish populations. Several business ventures have stated plans for developing weaponized ROVs and autonomous vehicles for commercial lionfish harvest, e.g., RSE (robotsise.org), Atlantic Lionshare Ltd. (atlanticlionshare.com), and Lionfish International LLC (sec.report/CIK/0001708706). Such approaches will necessitate advanced technologies and require high fixed and operating capital, thus catch rates and efficiencies would need to be high to enable cost-effective harvest.
Even for the best-performing trap design, the low lionfish catch rates in this study suggest it is unclear whether a deepwater lionfish fishery could be economically sustainable. Techno-economic and bioeconomic analyses of lionfish population dynamics, fishing costs, and market prices could help estimate what levels of lionfish densities, gear catchability, and market scenarios might be needed to support lionfish commercial fisheries (Harris et al., 2023). Linking these to ecosystem or bioenergetic models could help determine the potential benefits to biodiversity and native reef fish fisheries from commercial lionfish removals (Green et al., 2014; Chagaris et al., 2017; Bogdanoff et al., 2020; Chagaris et al., 2020). If catch rates are too low to support a food-based fishery, then public subsidies or value-added products—e.g., leather from lionfish skin or jewelry from lionfish fins (Galloway and Porter, 2019; Ulman et al., 2022)—could help increase the ex-vessel price of lionfish to incentivize harvest efforts. Collectively, these assessments could help set the catchability targets for traps and quantify metrics for the benefits, costs, risks, uncertainties, and timeframes for testing lionfish harvest technologies.
4.3 Conclusions and research directions
Our experimental testing indicated that the Gittings traps had the highest lionfish CPUE and lowest native fish CPUE of the three trap types tested. Time-lapse video monitoring showed that lionfish were attracted to the vicinity of the three trap types, but that lionfish rarely entered the lobster or sea bass traps. For the Gittings traps, further RD&T is needed to i) increase lionfish recruitment into the trap footprint (e.g., with lights, sounds, scents, or lionfish decoys.), ii) decrease lionfish escapement while the trap closes, iii) ensure that marine megafauna are not entangled, iv) improve deployment opening success, and v) test their use with surface buoys. The positive relationshiop we found between ambient lionfish densities and their recruitment to Gittings traps indicates that using these traps could be more viable in areas of higher lionfish densities. Lobster traps demonstrated more promise than the sea bass traps, whose high bycatch rates suggest they would be untenable for directed lionfish harvest. Future work on modified lobster traps could test wire traps rather than the wooden slat traps used in our study. Potential modifications for lobster traps to catch more lionfish could include i) modified openings to encourage lionfish to enter the trap, ii) escape gaps for other fishes, and iii) alternative baits. These modified lobster traps may also pose a lower risk of snagging hardbottom than Gittings traps, and may be more readily adopted by commercial lobster trappers.
The low catch rates observed by lionfish traps may be explained in part by the low lionfish densities observed by the ROV surveys. Nevertheless, it is presently unclear what catch rates are needed for a deepwater trap fishery to be profitable. Critical techno-economic and bioeconomic assessments appear warranted to consider the range of scenarios for lionfish densities, gear catchability, market prices, and operating costs that could support the development of deepwater lionfish fisheries. These values could then serve as targets for future experimental testing efforts. Ultimately, a deepwater lionfish fishery will likely need to integrate into already established fisheries such as trap fisheries for lobster or other fishes, and we recommend that research efforts cooperate with commercial fishers in all stages of the RD&T process.
Data availability statement
The original contributions presented in the study are included in the article/Supplementary Material. Data and code is also available at https://github.com/holdenharris-NOAA/Lionfish-Trap-Testing. Further inquiries can be directed to the corresponding author.
Ethics statement
The animal study was reviewed and approved by the University of Florida’s Institutional Animal Care and Use Committee (UF IACUC Protocols #201810225 and #201810394) in accordance with relevant guidelines and regulations by American Veterinary Medicine Association.
Author contributions
HH and WP conceived and designed this study with input from co-authors. Trap designs were developed by HH, SRG, and WP. Data was collected by HH, SBG, and JT. HH analyzed the data with assistance and review by SBG, DC, and WP. The first draft of the manuscript was developed by HH with subsequent input, review, and final approval from all co-authors. All authors contributed to the article and approved the submitted version.
Funding
Data collection was supported by the Florida Fish and Wildlife Conservation Commission (Grant No. FWC-13416-M3403) to WP. Support for HH was provided in part by the National Science Foundation Graduate Research Fellowship Program (Grant Nos. DGE-1315138 and DGE-182473). Opinions, findings, or conclusions expressed in this document do not necessarily reflect the views of our supporting organizations.
Acknowledgments
We thank Alexander Fogg (Okaloosa County Board of Commissioners) for logistical support and for developing the map figure. Cooperating fishing captains, Captain Johnny Greene (Intimidator Charters) and Captain Josh Livingston (Dreadknot Charters) and their crews provided invaluable local ecological knowledge and support on the water. Captain Ritchie Stigletz generously loaned the lobster traps for this study. Field and laboratory work was assisted by UF personnel Jordan Bajema, Jessica Van Vaerenbergh, and Miaya Glabach.
Conflict of interest
The authors declare that the research was conducted in the absence of any commercial or financial relationships that could be construed as a potential conflict of interest.
Publisher’s note
All claims expressed in this article are solely those of the authors and do not necessarily represent those of their affiliated organizations, or those of the publisher, the editors and the reviewers. Any product that may be evaluated in this article, or claim that may be made by its manufacturer, is not guaranteed or endorsed by the publisher.
Supplementary material
The Supplementary Material for this article can be found online at: https://www.frontiersin.org/articles/10.3389/fmars.2023.1121642/full#supplementary-material
References
Akins J. L., Lazarre D., Die D., Morris J. A. (2012). Lionfish bycatch in the Florida lobster fishery: first evidence of occurrence and impacts. Proc. 65th Gulf Caribbean Fisheries Institute (Santa Marta, Colombia), 65–65.
Andradi-Brown D. A. (2019). “Invasive lionfish (Pterois volitans and P. miles): Distribution, impact, and management,”. Mesophotic Coral Ecosyst., 931–941. doi: 10.1007/978-3-319-92735-0_48
Andradi-Brown D. A., Grey R., Hendrix A., Hitchner D., Hunt C. L., Gress E., et al. (2017a). Depth-dependent effects of culling–do mesophotic lionfish populations undermine current management? R Soc. Open Sci. 4, 170027. doi: 10.1098/rsos.170027
Andradi-Brown D. A., Vermeij M. J. A., Slattery M., Lesser M., Bejarano I., Appeldoorn R., et al. (2017b). Large-Scale invasion of western Atlantic mesophotic reefs by lionfish potentially undermines culling-based management. Biol. Invasions 19, 939–954. doi: 10.1007/s10530-016-1358-0
Arias-González J. E., González-Gándara C., Luis Cabrera J., Christensen V. (2011). Predicted impact of the invasive lionfish Pterois volitans on the food web of a Caribbean coral reef. Environ. Res. 111, 917–925. doi: 10.1016/j.envres.2011.07.008
Bacheler N. M., Whitfield P. E., Muñoz R. C., Harrison B. B., Harms C. A., Buckel C. A. (2015). Movement of invasive adult lionfish pterois volitans using telemetry: Importance of controls to estimate and explain variable detection probabilities. Mar. Ecol. Prog. Ser. 527, 205–220. doi: 10.3354/meps11241
Blakeway R. D., Fogg A. Q., Johnston M. A., Rooker J. R., Jones G. A. (2022). Key life history attributes and removal efforts of invasive lionfish (Pterois volitans) in the flower garden banks national marine sanctuary, northwestern gulf of Mexico. Front. Mar. Sci. 9. doi: 10.3389/FMARS.2022.774407/BIBTEX
Blakeway R. D., Jones G. A., Boekhoudt B. (2019). Controlling lionfishes (Pterois spp.) with consumption: survey data from Aruba demonstrate acceptance of non-native lionfishes on the menu and in seafood markets. Fish Manag Ecol., fme.12404. doi: 10.1111/fme.12404
Blakeway R. D., Ross A. D., Jones G. A. (2021). Insights from a survey of Texas gulf coast residents on the social factors contributing to willingness to consume and purchase lionfish. Sustainability 2021 Vol. 13 Page 9621 13, 9621. doi: 10.3390/SU13179621
Bogdanoff A. K., Shertzer K. W., Layman C. A., Chapman J. K., Fruitema M. L., Solomon J., et al. (2020). Optimum lionfish yield: a non-traditional management concept for invasive lionfish (Pterois spp.) fisheries. Biol. Invasions 23, 795–810. doi: 10.1007/s10530-020-02398-z
Bolker B. M., Brooks M. E., Clark C. J., Geange S. W., Poulsen J. R., Stevens M. H. H., et al. (2009). Generalized linear mixed models: a practical guide for ecology and evolution. Trends Ecol. Evol. 24, 127–135. doi: 10.1016/J.TREE.2008.10.008
Braun-McNeill, Joanne Epperly S. P. (2002). Temporal distribution of sea turtles in the western north Atlantic and the U.S. gulf of Mexico from marine recreational fishery statistics survey. Mar. Fisheries Rev. 64, 50–56.
Brooks M. E., Kristensen K., Van Benthem K. J., Magnusson A., Berg C. W., Nielsen A., et al. (2017). glmmTMB balances speed and flexibility among packages for zero-inflated generalized linear mixed modeling. R J. 9 (2), 378–400. doi: 10.32614/RJ-2017-066
Brown A. H., Niedzwecki J. M. (2020). Assessing the risk of whale entanglement with fishing gear debris. Mar. pollut. Bull. 161, 111720. doi: 10.1016/j.marpolbul.2020.111720
Buesa R. J. (2018). Spiny lobsters fisheries in the Western Central Atlantic. Report to the Gulf of Mexico Fisheries Management Council Special Spiny Lobster Scientific and Statistical Committee.
Burnham K. P. (2002). Model selection and multi-model inference: a practical information-theoretic approach. 2nd ed (New York: Springer). doi: 10.1007/b97636
Campbell M. D., Pollack A. G., Thompson K., Switzer T., Driggers W. B., Hoffmayer E. R., et al. (2021). Rapid spatial expansion and population increase of invasive lionfish (Pterois spp.) observed on natural habitats in the northern gulf of Mexico. Biol. Invasions, 1–13. doi: 10.1007/S10530-021-02625-1
Chagaris D. D., Binion-Rock S., Bogdanoff A., Dahl K., Granneman J., Harris H. E., et al. (2017). An ecosystem-based approach to evaluating impacts and management of invasive lionfish. Fisheries (Bethesda) 42, 421–431. doi: 10.1080/03632415.2017.1340273
Chagaris D. D., Patterson W. F. III, Allen M. S. (2020). Relative effects of multiple stressors on reef food webs in the northern gulf of Mexico revealed via ecosystem modeling. Front. Mar. Sci. 7. doi: 10.3389/FMARS.2020.00513
Chapman J. K., Anderson L. G., Gough C. L. A., Harris A. R. (2016). Working up an appetite for lionfish: a market-based approach to manage the invasion of Pterois volitans in Belize. Mar. Policy 73, 256–262. doi: 10.1016/J.MARPOL.2016.07.023
Clements K. R., Karp P., Harris H. E., Ali F., Candelmo A., Rodríguez S. J., et al. (2021). The role of citizen science in the research and management of invasive lionfish across the Western Atlantic. Diversity 13, 673. doi: 10.3390/D13120673
Cody T. T., Kiryu Y., Bakenhaster M. D., Subramaniam K., Tabuchi M, Ahasan M. S., et al. (2023). Cutaneous ulcerative lesions of unknown etiology affecting lionfish Pterois spp. in the gulf of Mexico. J. Aquat. Anim. Health 35 (1), 20–23. doi: 10.1002/aah.10174
Collins M. R. (1990). A comparison of three fish trap designs. Fish Res. 9, 325–332. doi: 10.1016/0165-7836(90)90051-V
Crawley M. J. (2015). “Multiple regression,” in statistics: An introduction using r Vol. 193–211 (West Sussex, UK: John Wiley & Sons, Ltd).
Dahl K., Edwards M., Patterson W. F. III (2019). Density-dependent condition and growth of invasive lionfish in the northern gulf of Mexico. Mar. Ecol. Prog. Ser. 623, 145–159. doi: 10.3354/meps13028
Dahl K. A., Patterson W. F. III (2014). Habitat-specific density and diet of rapidly expanding invasive red lionfish, pterois volitans, populations in the northern gulf of Mexico. PloS One 9, e105852. doi: 10.1371/journal.pone.0105852
Dahl K. A., Patterson W. F. III (2020). Movement, home range, and depredation of invasive lionfish revealed by fine-scale acoustic telemetry in the northern gulf of Mexico. Mar. Biol. 167, 1–22. doi: 10.1007/s00227-020-03728-4
Dahl K. A., Patterson W. F. III, Snyder R. A. (2016). Experimental assessment of lionfish removals to mitigate reef fish community shifts on northern gulf of Mexico artificial reefs. Mar. Ecol. Prog. Ser. 558, 207–221. doi: 10.3354/meps11898
Demuijnck G., Fasterling B. (2016). The social license to operate. J. Business Ethics 136, 675–685. doi: 10.1007/s10551-015-2976-7
Etnoyer P. J., Wickes L. N., Silva M., Dubick J. D., Balthis L., Salgado E., et al. (2016). Decline in condition of gorgonian octocorals on mesophotic reefs in the northern gulf of Mexico: before and after the deepwater horizon oil spill. Coral Reefs 35, 77–90. doi: 10.1007/S00338-015-1363-2/FIGURES/7
Florida Fish and Wildlife Conservation Commission (2019). 2010–2018 commercial fishery landings data through batch 1402 (St. Petersburg, FL, USA).
Galloway K. A., Porter M. E. (2019). Mechanical properties of the venomous spines of pterois volitans and morphology among lionfish species. J. Exp. Biol. 222. doi: 10.1242/JEB.197905/259532/AM/MECHANICAL-PROPERTIES-OF-THE-VENOMOUS-SPINES-OF
Garner S. B., Olsen A. M., Caillouet R., Campbell M. D., Patterson W. F. III (2021). Estimating reef fish size distributions with a mini remotely operated vehicle-integrated stereo camera system. PLoS One 16 (3), e0247985. doi: 10.1371/journal.pone.0247985
Garner S. B., Boswell K. M., Lewis J. P., Tarnecki J. H., Patterson W. F. (2019). Effect of reef morphology and depth on fish community and trophic structure in the northcentral gulf of Mexico. Estuar. Coast. Shelf Sci. 230, 106423. doi: 10.1016/J.ECSS.2019.106423
Gittings S. R. (2016). Encouraging results in tests of new lionfish trap design. in. 69th Gulf Caribbean Fisheries Institute, 206–214.
Gittings S. R., Fogg A. Q., Frank S., Hart J. v, Clark A., Clark B., et al. (2017). Going deep for lionfish: designs for two new traps for capturing lionfish in deep water. Silver Spring. doi: 10.13140/RG.2.2.22898.50886
Goodbody-Gringley G., Eddy C., Pitt J. M., Chequer A. D., Smith S. R. (2019). Ecological drivers of invasive lionfish (Pterois volitans and Pterois miles) distribution across mesophotic reefs in Bermuda. Front. Mar. Sci. 6. doi: 10.3389/fmars.2019.00258
Green S. J., Akins J. L., Cote I. M. (2011). Foraging behaviour and prey consumption in the indo-pacific lionfish on Bahamian coral reefs. Mar. Ecol. Prog. Ser. 433, 159–167. doi: 10.3354/meps09208
Green S. J., Dulvy N. K., Brooks A. M. L., Akins J. L., Cooper A. B., Miller S., et al. (2014). Linking removal targets to the ecological effects of invaders: a predictive model and field test. Ecol. Appl. 24, 1311–1322. doi: 10.1890/13-0979.1
Green S. J., Matley J. K., Smith D. E., II B. C., Akins J. L., Nemeth R. S., et al. (2021). Broad-scale acoustic telemetry reveals long-distance movements and large home ranges for invasive lionfish on Atlantic coral reefs. Mar. Ecol. Prog. Ser. 673, 117–134. doi: 10.3354/MEPS13818
Gress E., Andradi-Brown D. A., Woodall L., Schofield P. J., Stanley K., Rogers A. D. (2017). Lionfish (Pterois spp.) invade the upper-bathyal zone in the western Atlantic. PeerJ 5, e3683. doi: 10.7717/peerj.3683
Grueber C. E., Nakagawa S., Laws R. J., Jamieson I. G. (2011). Multimodel inference in ecology and evolution: challenges and solutions. J. Evol. Biol. 24, 699–711. doi: 10.1111/j.1420-9101.2010.02210.x
Hamilton S., Baker G. B. (2019). Technical mitigation to reduce marine mammal bycatch and entanglement in commercial fishing gear: lessons learnt and future directions. Rev. Fish Biol. Fish 29, 223–247. doi: 10.1007/s11160-019-09550-6
Harms-Tuohy C., Appeldoorn R., Craig M. (2018). The effectiveness of small-scale lionfish removals as a management strategy: effort, impacts and the response of native prey and piscivores. Manage. Biol. Invasions 9, 149–162. doi: 10.3391/mbi.2018.9.2.08
Harris H. E., Fogg A. Q., Allen M. S., Ahrens R. N. M., Patterson W. F. III (2020a). Precipitous declines in northern gulf of Mexico invasive lionfish populations following the emergence of an ulcerative skin disease. Sci. Rep. 10, 1–17. doi: 10.1038/s41598-020-58886-8
Harris H. E., Fogg A. Q., Gittings S. R., Ahrens R. N. M., Allen M. S., Patterson W. F. III (2020b). Testing the efficacy of lionfish traps in the northern gulf of Mexico. PloS One 15, e0230985. doi: 10.1371/journal.pone.0230985
Harris H. E., Fogg A. Q., Yanong R. P. E., Frasca S., Cody T., Waltzek T. B., et al. (2018). First report of an emerging ulcerative skin disease in invasive lionfish. UF/IFAS Extension Electronic Data Inf. Source FA209, 1–7. doi: 10.32473/edis-fa209-2018
Harris H. E., Patterson W. F. III, Ahrens R. N. M., Allen M. S. (2019). Detection and removal efficiency of invasive lionfish in the northern gulf of Mexico. Fish Res. 213, 22–32. doi: 10.1016/j.fishres.2019.01.002
Harris H. E., Patterson W. F. III, Ahrens R. N., Allen M. S., Chagaris D. D., Larkin S. L. (2023). The bioeconomic paradox of market-based invasive species harvest: a case study of the commercial lionfish fishery. Biol. Invasions. 1–18. doi: 10.1007/s10530-023-02998-5
Hind E. J. (2015). A review of the past, the present, and the future of fishers’ knowledge research: a challenge to established fisheries science. ICES J. Mar. Sci. 72, 341–358. doi: 10.1093/ICESJMS/FSU169
Hixon M. A., Green S. J., Albins M. A., Akins J. L., Morris J. A. (2016). Lionfish: a major marine invasion. Mar. Ecol. Prog. Ser. 558, 161–165. doi: 10.3354/meps11909
Hutchinson E., Sweetman C., Butler J. (2019). Development of a trap to catch the invasive lionfish. Proc. 72nd Gulf Caribbean Fisheries Institute (Punta Cana, Dominican Republic).
Jackman S. (2020). Pscl: Classes and methods for r (Sydney, New South Wales, Australia). Available at: https://github.com/atahk/pscl/.
Johannes R. E., Freeman M. M. R., Hamilton R. J. (2000). Ignore fishers’ knowledge and miss the boat. Fish Fisheries 1, 257–271. doi: 10.1111/J.1467-2979.2000.00019.X
Johnston M. A., Gittings S. R., Morris J. A. (2015) NOAA National marine sanctuaries lionfish response plan, (2015-2018): responding, controlling, and adapting to an active marine invasion. Available at: https://repository.library.noaa.gov/view/noaa/13954 (Accessed 8, 2018).
Kirk N., Kannemeyer R., Greenaway A., MacDonald E., Stronge D. (2020). Understanding attitudes on new technologies to manage invasive species. Pacific Conserv. Biol. 26, 35. doi: 10.1071/PC18080
Kleitou P., Crocetta F., Giakoumi S., Giovos I., Hall-Spencer J. M., Kalogirou S., et al. (2021a). Fishery reforms for the management of non-indigenous species. J. Environ. Manage 280, 111690. doi: 10.1016/J.JENVMAN.2020.111690
Kleitou P., Hall-Spencer J. M., Savva I., Kletou D., Hadjistylli M., Azzurro E., et al. (2021b). The case of lionfish (Pterois miles) in the Mediterranean Sea demonstrates limitations in EU legislation to address marine biological invasions. J. Mar. Sci. Eng. 2021 Vol. 9 Page 325 9, 325. doi: 10.3390/JMSE9030325
Kletou D., Hall-Spencer J. M., Kleitou P. (2016). A lionfish (Pterois miles) invasion has begun in the Mediterranean Sea. Mar. Biodivers Rec 9, 46. doi: 10.1186/s41200-016-0065-y
Lesser M. P., Slattery M. (2011). Phase shift to algal dominated communities at mesophotic depths associated with lionfish (Pterois volitans) invasion on a Bahamian coral reef. Biol. Invasions 13, 1855–1868. doi: 10.1007/s10530-011-0005-z
Lewis C. F., Slade S. L., Maxwell K. E., Matthews T. R. (2009). Lobster trap impact on coral reefs: effects of wind-driven trap movement. New Z. J. Mar. Freshw. Res., 271–282. doi: 10.1080/00288330909510000
Lewis J. P., Tarnecki J. H., Garner S. B., Chagaris D. D., Patterson W. F. (2020). Changes in reef fish community structure following the deepwater horizon oil spill. Sci. Rep. 10, 5621, 1–13. doi: 10.1038/s41598-020-62574-y
Mast R. B., Casale P. (2020). IUCN marine turtle specialist group. Available at: https://www.iucnredlist.org/ Accessed November 15, 2020.
McCallister M., Renchen J., Binder B., Acosta A. (2018). Diel activity patterns and movement of invasive lionfish (Pterois volitans/P. miles) in the Florida keys identified using acoustic telemetry. Gulf Caribb Res. 27–40. doi: 10.18785/gcr.2901.13
Morris J. A., Akins J. L., Green S. J., Buddo D. S. A., Lozano R. G. (2012). Invasive Lionfish: A Guide to Control and Management. Marathon, FL, USA: Gulf and Caribbean Fisheries Institute.
Nuttall M., Johnston M. A., Eckert R. J., Embesi J. A., Hickerson E. L., Schmahl G. P. (2014). Lionfish (Pterois volitans [Linnaeus 1758] and P. miles [Bennett 1828]) records within mesophotic depth ranges on natural banks in the northwestern gulf of Mexico. Bioinvasions Rec 3, 111–115. doi: 10.3391/bir.2014.3.2.09
Pitt J. M., Trott T. M. (2013). Efforts to develop a lionfish-specific trap for use in Bermuda waters. Proc. 66th Gulf Caribbean Fisheries Institute (TX), 187–190.
Pitt J. M., Trott T. M. (2015). A lionfish trap for use in Bermuda, with potential applications elsewhere. 2–3. doi: 10.13140/RG.2.2.22051.55844
Reed J., Farrington S., Harter S., Moe H., Hanisak D., David A. (2015). Characterization of the mesophotic benthic habitat and fish assemblages from ROV dives on pulley ridge and tortugas during 2014 R/V Walton smith cruise. Available at: https://repository.library.noaa.gov/view/noaa/17810 [Accessed November 21, 2018].
Savva I., Chartosia N., Antoniou C., Kleitou P., Georgiou A., Stern N., et al. (2020). They are here to stay: the biology and ecology of lionfish (Pterois miles) in the Mediterranean Sea. J. Fish Biol. 97, 148–162. doi: 10.1111/jfb.14340
Sutherland W. J., Barnard P., Broad S., Clout M., Connor B., Côté I. M., et al. (2017). A 2017 horizon scan of emerging issues for global conservation and biological diversity. Trends Ecol. Evol. 32, 31–40. doi: 10.1016/j.tree.2016.11.005
Switzer T. S., Tremain D. M., Keenan S. F., Stafford C. J., Parks S. L., McMichael R. H. (2015). Temporal and spatial dynamics of the lionfish invasion in the eastern gulf of Mexico: perspectives from a broadscale trawl survey. Mar. Coast. Fisheries 7, 1–8. doi: 10.1080/19425120.2014.987888
Tarnecki J. H., Patterson W. F. (2020). A mini ROV-based method for recovering marine instruments at depth. PloS One 15, e0235321. doi: 10.1371/journal.pone.0235321
Tookes J. S., Yandle T., Fluech B. (2022). The role of fisher engagement in the acceptance of turtle excluder devices in georgia’s shrimping industry. ICES J. Mar. Sci. doi: 10.1093/ICESJMS/FSAC062
Ulman A., Ali F. Z., Harris H. E., Adel M., al Mabruk S. A. A., Bariche M., et al. (2022). Lessons from the Western Atlantic lionfish invasion to inform management in the Mediterranean. Front. Mar. Sci. 9:865162, 538–550. doi: 10.3389/FMARS.2022.865162
Ulman A., Tunçer S., Kizilkaya I. T., Zilifli A., Alford P., Giovos I. (2020). The lionfish expansion in the Aegean Sea in Turkey: A looming potential ecological disaster. Reg. Stud. Mar. Sci. 36, 101271. doi: 10.1016/j.rsma.2020.101271
U. S. National Marine Fisheries Service (2018). Testing traps to target lionfish in the gulf of Mexico and south Atlantic, including within the Florida keys national marine sanctuary: final programmatic environmental assessment (FL, USA: St. Petersburg).
Usseglio P., Selwyn J. D., Downey-Wall A. M., Hogan J. D. (2017). Effectiveness of removals of the invasive lionfish: how many dives are needed to deplete a reef? PeerJ 5, e3043. doi: 10.7717/peerj.3043
van der Hoop J. M., Moore M. J., Barco S. G., Cole T. V. N., Daoust P. Y., Henry A. G., et al. (2013). Assessment of management to mitigate anthropogenic effects on Large whales. Conserv. Biol. 27, 121–133. doi: 10.1111/j.1523-1739.2012.01934.x
Whitfield P. E., Muñoz R. C., Buckel C. A., Degan B. P., Freshwater D. W., Hare J. A. (2014). Native fish community structure and indo-pacific lionfish pterois volitans densities along a depth-temperature gradient in onslow bay, north Carolina, USA. Mar. Ecol. Prog. Ser. 509, 241–254. doi: 10.3354/meps10882
Keywords: deepwater lionfish, gear testing, fishing innovation, Pterois volitans, ocean solutions, research development and testing, Gulf of Mexico, mesophotic reefs
Citation: Harris HE, Garner SB, Tarnecki JH, Gittings SR, Chagaris DD and Patterson WF III (2023) Three trap designs evaluated for a deepwater lionfish fishery. Front. Mar. Sci. 10:1121642. doi: 10.3389/fmars.2023.1121642
Received: 12 December 2022; Accepted: 22 March 2023;
Published: 18 April 2023.
Edited by:
Alessandro Lucchetti, National Research Council (CNR), ItalyReviewed by:
Teresa Romeo, Anton Dohrn Zoological Station Naples, ItalyGiacomo Milisenda, Stazione Zoologica Anton Dohrn di Napoli, Italy
Copyright © 2023 Harris, Garner, Tarnecki, Gittings, Chagaris and Patterson. This is an open-access article distributed under the terms of the Creative Commons Attribution License (CC BY). The use, distribution or reproduction in other forums is permitted, provided the original author(s) and the copyright owner(s) are credited and that the original publication in this journal is cited, in accordance with accepted academic practice. No use, distribution or reproduction is permitted which does not comply with these terms.
*Correspondence: Holden E. Harris, aG9sZGVuLmhhcnJpc0Bub2FhLmdvdg==; aG9sZGVuaGFycmlzQHVmbC5lZHU=
†Senior author