- 1Rosenstiel School of Marine, Atmospheric, and Earth Science, Department of Marine Biology and Ecology, University of Miami, Miami, FL, United States
- 2School of Basic and Marine Sciences, The University of Jordan, Aqaba, Jordan
Introduction: Climate change, and the increase in sea surface temperature, is exacerbating ocean deoxygenation because of the inherent property of seawater to sequester less dissolved gas, such as oxygen, at warmer temperatures. While most coral reef studies focus on the effects of thermal stress and ocean acidification, few studies acknowledge the threat of hypoxia. Hypoxia is traditionally defined as 6.3 kPa (2 mg L-1 O2), however, a universal hypoxia threshold is not useful given the vast range of responses among marine organisms. The range of metabolic responses and tolerances to hypoxia are unknown for Caribbean coral species and their algal symbionts.
Objective: Here, we quantified the spectrum of acute hypoxia tolerances and the range of metabolic responses of six ecologically and structurally important Caribbean coral species (Acropora cervicornis, Siderastrea radians, Siderastrea siderea, Porites astreoides, Porites porites, and Orbicella faveolata) and their algal symbionts (Symbiodinium, Breviolum, and Durusdinium spp.).
Methods: A total of 24 coral fragments (4 individuals per species) were exposed to 10 distinct oxygen concentrations ranging from normoxia (20.38 kPa) to severe hypoxia (3.3 kPa). We used intermittent flow respirometry to measure coral host respiration in the dark and algal symbiont photosynthesis in the light at each oxygen level. We determined a line of best fit for the metabolic rate vs. PO2 data and calculated the critical oxygen partial pressure (PO2 crit), a method that has not been tested on symbiotic species.
Results: Coral species and their algal symbionts measured here displayed a wide range of hypoxia tolerances. For the coral hosts, PO2 crit values differed roughly two-fold ranging from 5.74 kPa to 16.93 kPa, and for the algal symbionts, PO2 crit values differed roughly three-fold ranging from 3.9 kPa to 11.3 kPa.
Discussion: These results should be regarded as a first step to characterizing the metabolic response and acute tolerance of multiple coral hosts and algal symbionts to a wide range of oxygen concentrations. Given that some PO2 crit values were above the generally accepted hypoxia threshold, these results have implications for the community composition of reefs under a rapidly changing climate and can guide purposeful reef restoration.
1 Introduction
Global ocean deoxygenation and local hypoxia events have been recognized as a major threat to the health of coral reef ecosystems (Steckbauer et al., 2020). Indeed, ocean deoxygenation likely poses a greater and more immediate threat than warming and acidification to the survival of coral reefs (Hughes et al., 2020). Coastal hypoxia events leading to mass mortality have been well documented in recent years in locations such as Panama (Altieri et al., 2017), Flower Garden Banks in the Gulf of Mexico (Johnston et al., 2019; Kealoha et al., 2020), and Biscayne Bay in South Florida. These mass mortality events have been linked to low dissolved oxygen levels that are often the result of warming, local eutrophication, bacterial/algal decomposition, stratification, or a combination of these factors (Altieri et al., 2020).
Hypoxia has been defined as the dissolved oxygen concentration at which an organism experiences lethal or sublethal impacts, with a generally accepted threshold of ~2 mg L-1 O2 (6.3 kPa) (Renaud, 1983). However, it is important to note that ‘hypoxia’ is a subjective term that fails to accurately represent a universal threshold of lethal or sublethal responses among marine organisms. Indeed, organisms of different taxa (Mangum and Van Winkle, 1973; Vaquer-Sunyer and Duarte, 2011; Gilmore et al., 2019) and even different species within the same taxa (Johnson et al., 2021; Alva García et al., 2022) have been shown to have vastly different responses to the same level of oxygen limitation. In fact, field surveys performed after hypoxic events reveal differential survivorship among coral species and changes in community structure (Altieri et al., 2017). As such, a universal threshold for hypoxia is not useful. Given the projected increase in frequency and severity of local hypoxic or anoxic events due to climate change (Altieri and Gedan, 2015; Pezner et al., 2023), it will become critical to understand the relative tolerance to hypoxia of multiple reef-building coral species.
The term ‘tolerance’ is also a subjective, and time-dependent term that frequently fails to accurately describe the full scope of an organism’s response to low oxygen conditions. Much like the term ‘degree-heating-weeks’ used to describe thermal stress, hypoxia tolerance is a time-dependent metric that is reliant on long-term energy stores (Sokolova et al., 2012) and the ability of an organism to efficiently regulate its metabolism. A recent study by Hughes et al., 2022 revealed that at least 17 coral species from the Great Barrier Reef can regulate their oxygen consumption, however, it is unknown if Caribbean coral species maintain the same ability. The extent of oxygen regulation among different coral species is not well understood, and rates of gross photosynthesis under acute hypoxia exposure have not been previously explored.
Calculating the critical oxygen partial pressure (hereby referred to as PO2 crit) is one method of determining hypoxia tolerance among marine organisms (Seibel, 2011), with a lower PO2 crit indicating a greater tolerance for low oxygen (Rogers et al., 2016). PO2 crit is defined as the oxygen concentration at which an organism’s metabolic rate becomes oxygen-limited (Seibel et al., 2021). In other words, PO2 crit is defined as the oxygen concentration at which an organism switches from maintaining its metabolic function (regulating) to adjusting its metabolic rate to conform with oxygen availability (conforming) (Mueller and Seymour, 2011). Despite literature debates regarding the usefulness of PO2 crit (Wood, 2018), we argue that providing the full spectrum of metabolic responses, in addition to the PO2 crit values, contributes valuable baseline knowledge regarding the acute hypoxia tolerance among coral species (Regan et al., 2019). Additionally, using a standardized respirometry methodology will allow for easy comparison among myriad marine species including the scleractinian coral species studied here (Killen et al., 2021).
The growth, survival, and metabolism of reef-building corals are reliant on their symbiotic algal partners of the family Symbiodiniacae. It is well established that certain species of algal symbiont can confer tolerance to a particular stressor (Cunning and Baker, 2020). For example, coral colonies hosting Durusdinium symbionts are better able to resist bleaching when compared to colonies hosting Cladocopium or Breviolum (Cunning et al., 2015a; Cunning et al., 2015b). While not much is known regarding the response of the algal symbiont to hypoxia, a study by Deleja et al. (2022) revealed a significant reduction in photochemical activity of the algal symbiont caused by the impairment of the electron transport chain under diel hypoxia. Given the capacity of algal symbionts to adapt to certain stressors (Howells et al., 2016), it’s not unreasonable to assume that some species of Symbiodiniacae are better equipped to handle oxidative stress than others.
Here, we investigated the potential hypoxia tolerance of six common Caribbean coral species (Acropora cervicornis, Siderastrea radians, Siderastrea siderea, Porites astreoides, Porites porites, and Orbicella faveolata) by measuring the metabolic response (dark respiration and net photosynthesis) of each species during exposure to a range of oxygen concentrations (20.38 – 3.3 kPa). We propose an experimental framework with which to determine acute hypoxia tolerance of reef-building coral species which includes (1) standardized dark respirometry and net photosynthesis measurements and (2) calculation of PO2 crit via a hyperbolic tangent function that uses the ‘broken stick’ methodology for both respiration and photosynthesis data. Through this framework, coral species can be identified as relatively hypoxia ‘tolerant’ or ‘sensitive’.
2 Materials and methods
2.1 Coral husbandry and acclimation
A total of six Caribbean, scleractinian coral species were utilized: Acropora cervicornis, Siderastrea siderea, Siderastrea radians, Porites asteroides, Orbicella faveolata, and Porites porites. A. cervicornis fragments were collected from the Rescue a Reef Coral Nursery in Miami Florida (25.676267, -80.109067), S. siderea and both Porites species colonies were scavenged from the Port of Miami (25.7761, -80.1601), and O. faveolata and S. radians fragments were collected by the Reef Renewal Nursery in Tavernier Florida (25.980650, -80.438833). Coral fragments of the same species were collected from the same colony (same genotype) to reduce any genotypic differences in the hypoxia response among coral individuals. Given that different genotypes of the same species often respond differently to stressors, the likelihood of any genotypic effects was reduced by selecting individuals of the same genotype. In this way, the hypoxia tolerance among coral species (not among coral genotypes of the same species) could be assessed. By necessity, the corals in this study came from different environments. Characterizing the pre-study oxygen environments of these corals was beyond the scope of this study, however, all corals were held under constant normoxic conditions in the lab for four months before this study was conducted to minimize pre-study effects. All corals were immediately fragmented upon arrival to the laboratory and reglued to numbered tags using CorAffix Pro (mounding species) or All Game Epoxy (A. cervicornis). A total of four coral fragments per species were acclimated in an indoor flow-through aquarium at 28°C, a salinity value of 34.7, and normoxia (6.5 mg L-1 O2). Seawater from Biscayne Bay was passed through a sand filter and then a secondary 10μm bag filter before reaching the aquaria. Before experimentation began, corals were monitored for lesion recovery following fragmentation for a minimum of three weeks. Corals received lighting from Cool-White fluorescent lights (209 μmol m-2 s-2) on a 12:12 photoperiod and were fed Ziegler’s Larval Diet AP100 twice weekly. Coral tanks were cleaned thoroughly once per week and individual coral fragments were cleaned once per week to reduce algal growth.
2.2 Acute hypoxia exposure
Ten distinct oxygen concentrations were identified as treatments ranging from normoxia (20.38 kPa) to severe hypoxia (3.3 kPa) (Figure 1, Table 1). Four coral fragments of six coral species (24 total individuals) were pre-conditioned to each oxygen treatment for one hour before metabolic measurements began and were only exposed to one oxygen treatment per day. The total time of exposure to hypoxic conditions before returning to normoxia was 180 minutes. Corals were allowed to recover for a minimum of 24 hours between treatments, and exposure to each oxygen treatment was selected at random to reduce any negative effects from pre-exposure to hypoxia. All treatments were performed between 5/9/22 and 6/15/22. Oxygen concentrations were achieved by bubbling either air or nitrogen gas directly into the treatment tank and were continuously monitored with a Vernier Dissolved Oxygen Optical probe in combination with a Vernier LabQuest3 Interface. The LabQuest3 Interface was programmed to open a pinch valve connected to a nitrogen cylinder, allowing nitrogen to flow freely into the treatment tank only when the measured oxygen concentration was higher than the set point. Conversely, if oxygen concentration dipped below the set point, a second pinch-valve opened to allow air to flow into the tank subsequently raising the oxygen to the target level, and replenishing any CO2 lost due to the nitrogen bubbling. Seawater pH was not measured in this experiment even though nitrogen bubbling can change the pH of the surrounding seawater (Klein et al., 2017). However, given the very short exposure to hypoxia (three hours maximum) along with the replenishment of CO2 from the periodic bubbling of air, changes in pH will not have a significant effect on the results of this study. A follow-up study was performed to determine if pH changes significantly as oxygen concentration drops (Figure 2). Over three hours, pH only rose by 0.022, an insignificant change that is not expected to have significant effects on coral health and function.
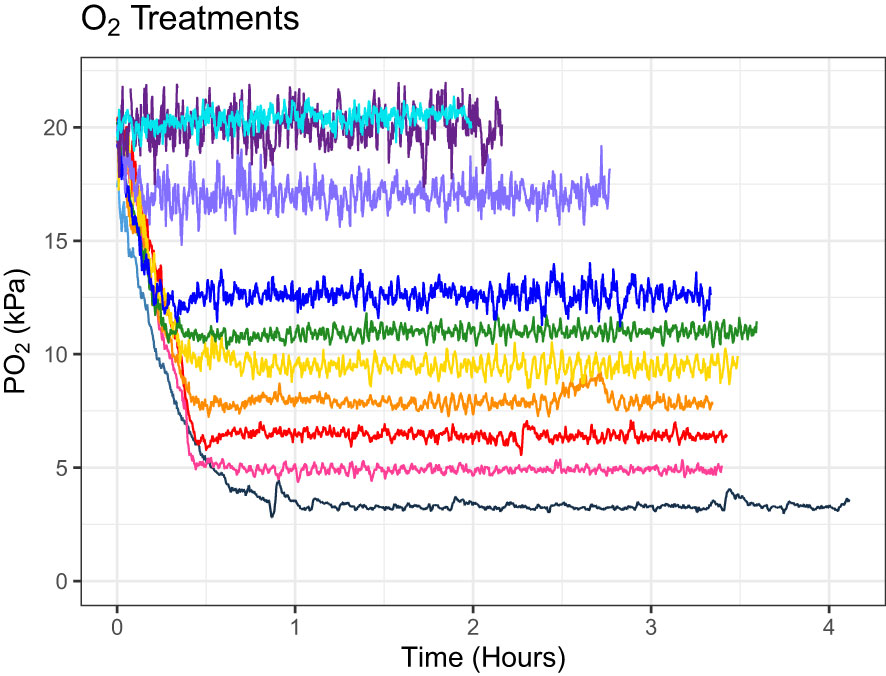
Figure 1 Raw oxygen concentration values (PO2 kPa) represented over time (hours) during each acute hypoxia exposure. Each line represents one oxygen treatment. Corals were only placed in the treatment tank once the set point oxygen concentration was reached. The time to reach the set point ranged from 0.069 to 1.031 hours.
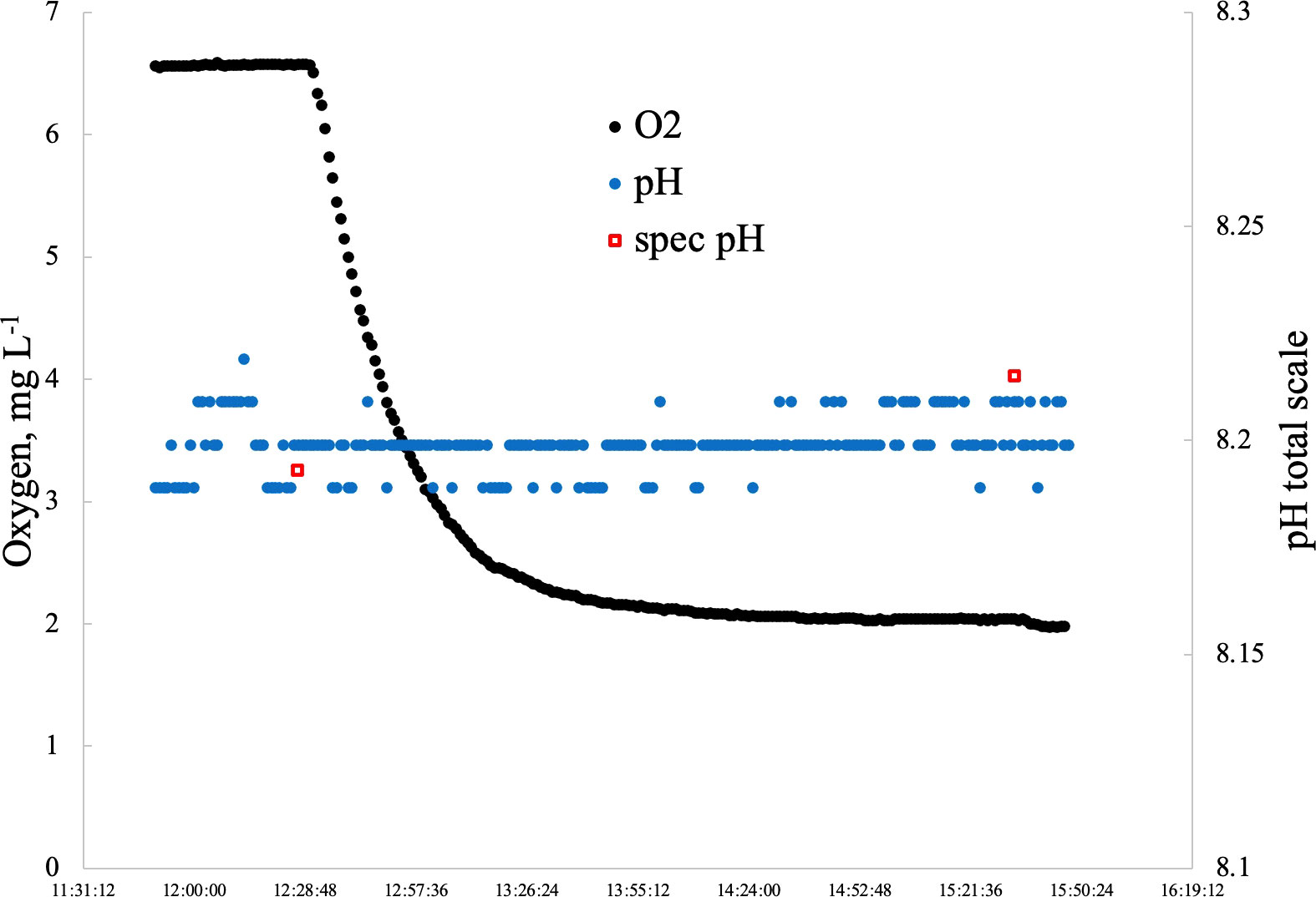
Figure 2 Results of follow-up study to determine if pH changed significantly with deoxygenation over a three hour period.
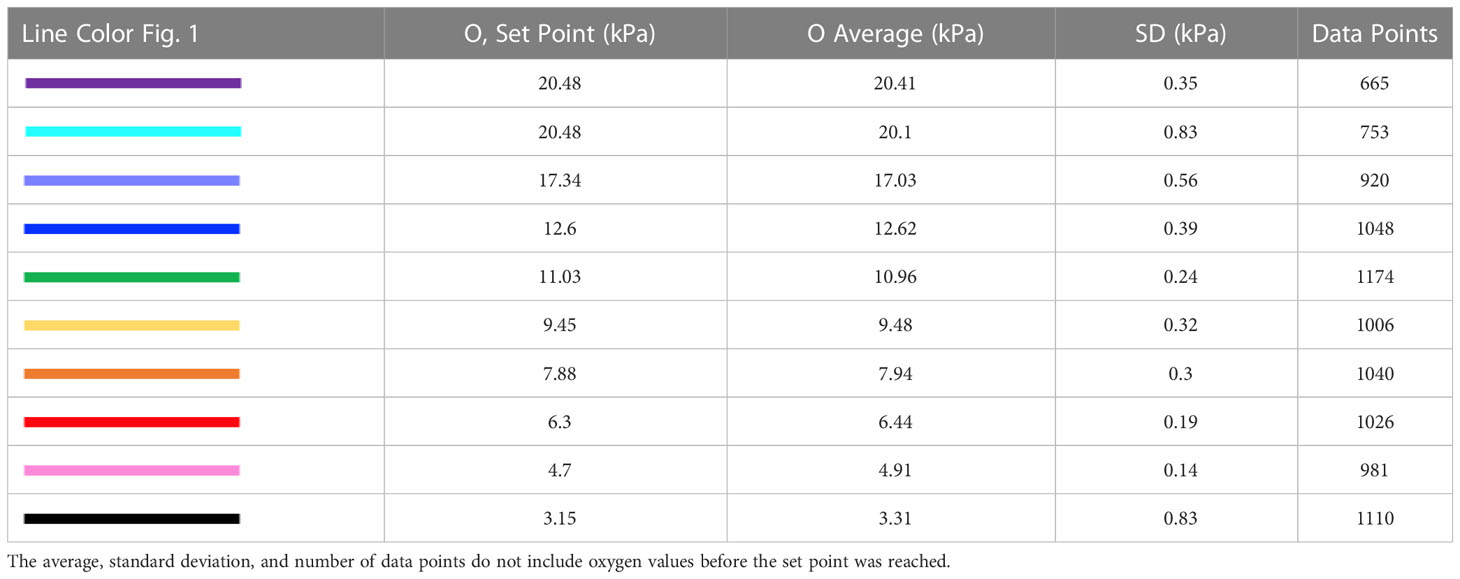
Table 1 Legend for Figure 1 including line color identification, oxygen set point in kPa, average oxygen concentration in kPa, standard deviation of oxygen values in kPa, and the number of data points recorded for each treatment.
Foil-laminated bubble wrap was positioned on the surface of the water in the treatment tank to reduce the diffusion of oxygen into the seawater. An area was cut out of the bubble wrap so that light exposure to the experimental corals was not obstructed. Photosynthetic active radiation (PAR) was measured both with (205 μmol m-2 s-1) and without bubble wrap (209 μmol m-2 s-1) revealing that light intensity was only reduced by 4 μmol m-2 s-1 during hypoxia exposure treatments.
2.3 Photosynthesis and respiration by intermittent flow respirometry
Rates of dark respiration (DR) and net photosynthesis (NP) were measured by intermittent flow respirometry following methods from Killen et al., 2021. All measurements were collected during daylight between 12pm and 3pm and were performed in a 200-liter tank which served as a water bath maintained at 28°C. Four coral fragments per species (n=4 per species, n=24 total) were measured individually such that each respirometry chamber contained only one coral at a time. Corals fasted for a minimum of 36 hours before metabolic measurements were collected to ensure that the standard metabolic rate (SMR) is measured.
Glass respirometry chambers had a volume of 254 mL with a 0.5-inch-thick clear acrylic lid fitted with an O-ring for a gas-tight seal. Chambers were outfitted with two 3/16” ports and vinyl tubing for connection to a small submersible pump used for circulating water within the chamber and flushing between measurements. Oxygen concentration inside the chamber was measured every five seconds using a fluorescence sensor spot affixed to the internal wall of the chamber. Optical fibers outside the jar connect this sensor to a Presens Oxy-4 SMA meter and the phase of the fluorescent signal from the sensor spot is converted to an oxygen concentration value in μmol O2 L-1. Sensor spots were calibrated before each treatment was performed. Each coral was positioned inside the chamber for approximately ten minutes to measure respiration in the dark or net photosynthesis in the light. Chambers were flushed for a minimum of five minutes between coral replicates or until the seawater inside the chamber returned to the target oxygen concentration. Corals were allowed to acclimate inside the chamber for two minutes before data collection began. Rates of respiration in the dark and net photosynthesis in the light were determined by calculating the slope of ΔO2/Δt (μmol L-1 h-1). All rates were corrected for background microbial respiration by subtracting the average oxygen consumption in an empty chamber (0.7 μmol L-1 h-1). The rate of dark respiration or net photosynthesis was computed using the following formula:
where ‘RateO2’ is the background corrected rate of oxygen consumption (DR) or production (NP), ‘Volcham’ is the volume of water in the chamber including the pump and tubing, minus the volume displaced by the coral, and ‘SA’ is the surface area of each coral in cm2. The average displacement of water by corals within the chamber was calculated to be roughly 1% of the total chamber volume, including the volume of the tubing. Gross photosynthesis was computed as NP+|DR| assuming that the rate of respiration in the light was the same as in the dark.
2.4 Calculation of PO2 crit
For each species, a hyperbolic tangent function of the form
was fit to the MO2 vs. pO2 data using the nls command in R. MO2 values used in this function represent the average respiration or photosynthesis rate per species per oxygen treatment. The curve fitting procedure returns best-fit values for MRmax and PO2 crit and their standard errors. This form of rigorous curve fitting is commonly referred to as the ‘broken stick method’ such that two linear regressions are created: (1) through the oxygen-limited portion and (2) through the oxygen-independent portion (MRmax) of the MO2 vs. pO2 graph. The intersection of these two lines is taken as PO2 crit (Yeager and Ultsch, 1989; Seibel et al., 2021). PO2 crit values were calculated for both the coral host (dark respiration) and the algal symbiont (gross photosynthesis).
2.5 Surface area determination
The surface area for each A. cervicornis coral fragment was calculated using the “wax dipping” method (Stimson and Kinzie, 1991). Following blasting, the coral skeletons were thoroughly dried. Wax dipping was performed using paraffin wax heated in a large coffee tin on a hot plate to a temperature of 82 C. Each coral or calibration object (wooden dowels of various diameters and lengths) was weighed prior to dipping to an accuracy of 0.001 g and then dipped for 2 seconds before removing and shaking to dislodge any excess wax. Dipped corals or calibration objects were allowed to cool for 15 min before being reweighed. Dimensions of each calibration dowel were measured with venier calipers and the surface area in cm2 was computed using the equation:
Where SA is the surface area in cm2, D is the diameter and L is the length both in cm. The calibration objects were selected so that their surface areas would span the range of the corals being analyzed. A standard curve was prepared relating the wax weight of the dipped calibration objects to their measured surface area. The best fit relationship to the pooled data (n=123 observations) is:
where SA is the surface of the coral or calibration object in cm2 and Wax wt is the weight of the wax on the dipped object in g. The R2 was 0.96 and the standard error of the regression was 2.8 cm2 meaning that surface areas of objects can be determined with a typical uncertainty of ± 2.8 cm2.
The surface area of each mounding coral species (O. faveolata, P. astreoides, P. porites, S. radians, and S. siderea) was calculated using aerial photographs imported into Coral Point Count with Excel extensions (Kohler and Gill, 2006). Briefly, photographs were scaled using a calibration object (coral tag, 2.8 cm), and the Area/Length Analysis Tool was used to trace the area of live coral tissue.
2.6 Quantitative PCR: identifying Symbiodiniaceae to genus level
Small, minimally invasive tissue biopsies were taken from four replicates per species and preserved in 500 uL of 1% SDS in DNAB. Genomic DNA was extracted from these archives using a modified organic extraction method (Rowan and Powers, 1991; Baker and Cunning, 2016). Assays for Cladocopium and Durusdinium followed reactions described in Cunning and Baker, 2013 (Cunning and Baker, 2013), and assays for Breviolum were multiplexed as described in Cunning et al. (2015a). Assays for Symbiodinium followed methods outlined in Winter, 2017 (Winter, 2017). The QuantStudio 3 Real-Time PCR instrument (Applied Biosystems, USA) was used to target the actin loci for each genus, and the StepOneR software package was used to quantify relative symbiont abundance, however each species was dominated (>99%) by the genus indicated in this study.
2.7 Statistics
A series of linear regressions were fit to the MO2 vs. pO2 data for both coral host respiration and algal symbiont photosynthesis, where oxygen consumption (respiration) or production (photosynthesis) was the dependent variable and partial pressure of oxygen was the independent variable. Linear regressions were fit for each species (Figures 3, 4; n=4 per species). Slopes were analyzed to determine if they were significantly different from zero, which would indicate a significant change in metabolism as a function of changing oxygen partial pressure. Data are reported as the mean ± one standard error. All analyses were performed using R Statistical Software (v4.1.2; R Core Team, 2021).
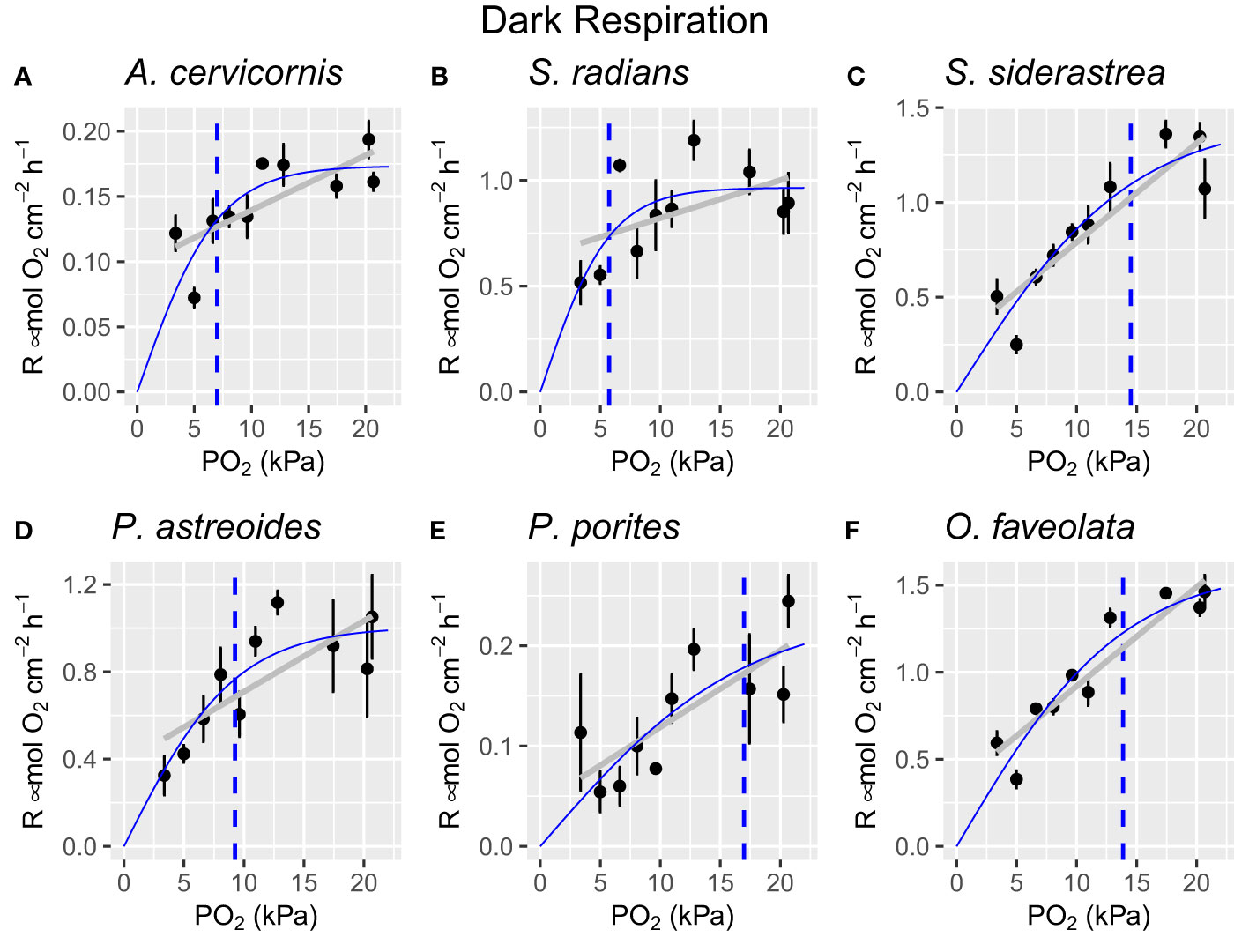
Figure 3 Respiration values for all species at each oxygen treatment level indicating PO2 crit (dotted blue line), the hyperbolic tangent line of best fit (solid blue line), and linear regression line (grey line). Each dot represents the average respiration rate of all individuals per species (n=4 per species) and error bars represent ± one standard error. (A) Acropora cervicornis, (B) Sidereastrea radians, (C) Siderastrea siderea, (D) Porites astreoides, (E) Porites porites, (F) Orbicella faveolata..
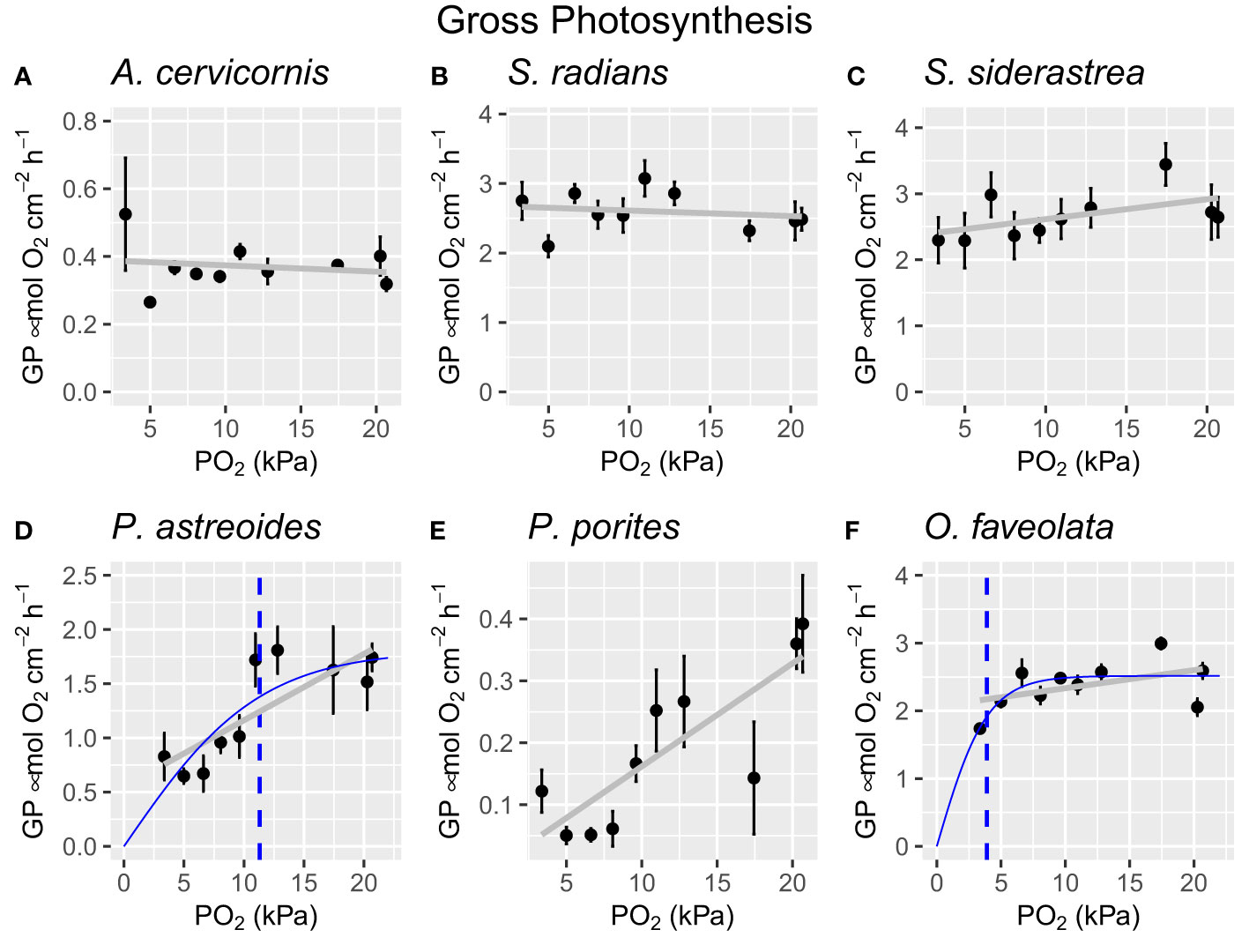
Figure 4 Gross photosynthesis values for all species at each oxygen level indicating PO2 crit (dotted blue line), the hyperbolic tangent line of best fit (solid blue line), and the linear regression line (grey line). Each dot represents the average gross photosynthetic rate of all individuals per species (n=4 per species) and error bars represent ± one standard error. (A) Acropora cervicornis, (B) Sidereastrea radians, (C) Siderastrea siderea, (D) Porites astreoides, (E) Porites porites, (F) Orbicella faveolata.
3 Results
3.1 Dark respiration
All species exhibited reduced respiratory capacity as ambient oxygen concentration decreased (Figure 3, Supplementary Material), however, the severity of reduced respiration differed between each species. A linear regression analysis revealed that oxygen treatment did have a statistically significant effect on respiration rate in A. cervicornis (R2 = [0.39], F(1, 38) = 26.39, p = [8.7e-06]), S. radians (R2 = [0.12], F(1, 38) = 6.435, p = [0.0154]), S. siderastrea (R2 = [0.67], F(1, 38) = 81.04, p = [5.83e-11]), P. astreoides (R2 = [0.29], F(1, 38) = 17.13, p = [0.00019]), P. porites (R2 = [0.30], F(1, 38) = 17.53, p = [0.000162]), and O. faveolata (R2 = [0.8082], F(1, 38) = 165.4, p = [2.04e-16]). All species exhibited at least a 60% reduction in respiration, while two species experienced up to 83% reduction (S. siderea and P. porites). PO2 crit was identified to be 7 ± 1 kPa (A. cervicornis), 5.8 ± 1.19 kPa (S. radians), 14.5 ± 2.5 kPa (S. siderea), 9.3 ± 2.1 (P. astreoides), 16.9 ± 7.6 (P. porites), and 13.9 ± 1.5 kPa (O. faveolata) (Figure 3- dotted line). Maximum rates of respiration ranged from 0.2 μmol O2 cm-2 h-1 (A. cervicornis) to 1.5 μmol O2 cm-2 h-1 (S. siderea and O. faveolata).
3.2 Gross photosynthesis
Only three species (P. astreoides, P. porites, and O. faveolata) experienced reduced gross photosynthesis under acute hypoxia exposure (Figure 4, Supplementary Material). A linear regression analysis revealed that oxygen treatment did have a statistically significant effect on gross photosynthesis in P. astreoides (R2 = [0.36], F(1, 38) = 23.23, p = [2.33e-5]), P. porites (R2 = [0.40], F(1, 38) = 27.49, p = [6.2e-6]), and O. faveolata (R2 = [0.14], F(1, 38) = 7.499, p = [0.00934]). Reductions in gross photosynthesis ranged from 42% (O. faveolata) to 88% (P. porites). All other species did not experience a significant reduction in gross photosynthesis. Maximum rates of gross photosynthesis ranged from 0.55 μmol O2 cm-2 h-1 (A. cervicornis) to 3.5 μmol O2 cm-2 h-1 (S. siderea). PO2 crit was calculated to be 3.9 ± 0.48 kPa (O. faveolata), and 11.3 ± 4.22 kPa (P. astreoides). PO2 crit could not be calculated for P. porites given its apparent oxy-conformation throughout the duration of this experiment.
3.3 Symbiont identity
All corals were dominated by (>99%) Symbiodinium symbionts (A. cervicornis, P. astreoides, and P. porites), Brevioloum symbionts (S. radians and O. faveolata), or Durusdinium symbionts (S. siderea) with each coral species associating with only one species of algal symbiont (Table 2).
4 Discussion
4.1 Metabolic suppression
As expected, all species experienced significant metabolic suppression under hypoxia (Figure 3). This result supports findings of other studies in which the respiratory capacity of corals is reduced under decreasing oxygen conditions (Seibel, 2011; Gravinese et al., 2022). However, the severity and extent of metabolic suppression differed between coral species. Some species appear to possess some capacity for oxy-regulation above PO2 crit (A. cervicornis and S. radians), while others experienced a steep drop off in oxygen consumption under hypoxia (Porites spp. and O. faveolata).
4.2 PO2 crit: dark respiration
Based on respiration PO2 crit values alone, the coral species tested here can be ranked from most to least hypoxia tolerant as follows: (1) S. radians, (2) A. cervicornis, (3) P. astreoides, (4) O. faveolata, (5) S. siderea, and (6) P. porites. PO2 crit was highly variable among the different coral species ranging from 5.74 kPa (S. radians) to 16.93 kPa (P. porites), representing a roughly three-fold difference. These results indicate that S. radians is the most tolerant to acute hypoxia stress, given its low PO2 crit, while P. porites is the least tolerant based on its high PO2 crit. Additionally, these results suggest that neither source location nor phylogeny dictate a coral’s hypoxia tolerance or sensitivity. Source location and phylogeny were not statistically tested, however, based on the ranking of PO2 crit values alone, there is no trend based on source location or coral genus.
Interestingly, A. cervicornis had a higher PO2 crit than O. faveolata. This contrasts with previous studies which identify O. faveolata as a relatively hypoxia-tolerant species (Johnson et al., 2021) at least when compared to A. cervicornis. Indeed, our results show that O. faveolata may experience metabolic suppression before A. cervicornis does, however, given a longer time scale of exposure to hypoxia, we may expect to see mortality in A. cervicornis before O. faveolata based on the findings of Johnson et al., 2021. Given this contrast in results, it is possible that a higher PO2 crit may highlight a coral’s ability to adjust its metabolism to lower oxygen levels and withstand oxygen limitation longer than a coral that must expend energy to maintain a normal metabolic rate at lower oxygen levels. In other words, the cost of maintaining normal metabolic function may be too high to support tissue integrity or other vital life processes, which may be why A. cervicornis had a lower PO2 crit that O. faveolata in this study, but succumbed to mortality quickly in Johnson et al., 2021. On the other hand, varying taxa composition of symbiotic algae and bacteria in O. faveolata correspond with varying bleaching susceptibility (Parker et al., 2020). It is unknown if symbiont genus played a role in hypoxia sensitivity in the Johnson et al. study as it was not identified.
A PO2 crit for P. porites could not be calculated due to its apparent lack of oxy-regulation (Figure 3E). This was a surprising result given that all other species tested here exhibited some level of oxy-regulation, although the extent of this regulation differed between species. While most coral species on the Great Barrier Reef are regarded as oxy-regulators (Hughes et al., 2022), our results show that not all Caribbean coral species possess the same ability to oxy-regulate and that P. porites in particular may be an oxy-conforming species.
Most species in this study (A. cervicornis, P. astreoides, O. faveolata, P. porites, and S. siderea) had PO2 crits higher than the generally accepted hypoxia threshold of 6.3 kPa (2 mg L-1 O2), suggesting that these species may experience metabolic suppression and other sublethal effects even before a hypoxia event is recognized (Hughes et al., 2022). These results also show that a universal hypoxia tolerance threshold for marine species is not useful given the roughly three-fold difference in PO2 crit values among scleractinian species.
There are very few studies that assess the hypoxia tolerance or sensitivity of reef-building corals, however, Altieri et al. (2017) showed that Agaraicia lamarcki colonies succumbed to mortality during a hypoxia event in Panama, while Stephanocoenia intersepta species colonies persisted (Altieri et al., 2017). This may suggest that flat growth forms such as S. intersepta and S. radians are better adapted to oxygen limitation due to their proximity to the benthos. Symbiont genus could not be identified in Altieri et al. (2017) which may have played a role in the response to hypoxia.
4.3 PO2 crit: gross photosynthesis
Half of the species tested here experienced significantly reduced photosynthesis under hypoxia (Figure 4), which supports previous literature that identified significant decreases in photochemical efficiency under hypoxia (Ulstrup et al., 2005; Alva García et al., 2022). This result is likely due to the build-up of free radicals, such as reactive oxygen species (ROS), which are generated during periods of stress. ROS cause damage to not only the coral host tissue, but to the delicate membranes and photosynthetic machinery of the algal symbiont leaving them functionally impaired (Gardner et al., 2017; Deleja et al., 2022). The severity of damage will depend on the intensity of the stressor and the identity of the algal symbiont.
Not surprisingly, both Porites species were dominated by symbionts of the same genus (Symbiodinium), however, symbionts were not identified to the species level. The symbiont genus here does not explain the differences in PO2 crit. The flat growth form of P. astreoides may have conferred some level of hypoxia tolerance when compared to the erect growth form, P. porites, given that hypoxia often arises from the benthos which may have preadapted P. astreoides to hypoxia (Ferguson et al., 2013).
While Durusdinium colonies are known to be more thermotolerant than others (Cunning and Baker, 2020), our results reveal that a thermotolerant symbiont does not confer tolerance to hypoxia given the relatively high PO2 crit measured for S. siderea. This is not surprising given that tradeoffs are known to exist in the delicate partnership between coral host and algal symbiont such that colonies hosting Durusdinium maintain a higher thermotolerance, but a slower growth rate than their counterparts in symbiosis with a different algal symbiont (Cunning et al., 2015a).
4.4 Summary
The results presented here have major implications for the future of coral reefs under climate-change-driven hypoxia. Hypoxia is already pervasive on many coral reefs; presently, 84% of reefs experience weak to moderate hypoxia and 13% of reefs experience severe hypoxia, and these values are projected to increase under climate change scenario SSP5-8.5 (Pezner et al., 2023). Indeed, an increase in intensity, duration, and severity of hypoxia on reefs worldwide will continue to cause mass mortality on coral reefs. However, not all coral species will respond the same way to deoxygenation. Our results suggest that some species can easily withstand acute hypoxia exposure (A. cervicornis and S. radians) while others may experience serious sublethal or lethal effects if hypoxia persists (Porites spp.). Differential survivability among coral species may lead to changes in community composition such that corals are naturally selected for their hypoxia tolerance (Altieri et al., 2017). Additionally, deoxygenation has been proven to lower the thermal threshold of bleaching in Acropora species (Alderdice et al., 2022) suggesting that bleaching events, and their subsequent mortality, will be exacerbated by hypoxia. It’s also possible that coral may acclimate to lower oxygen conditions (Hebbeln et al., 2020), however, this is highly dependent on food availability and ambient flow rate, and the longevity of deoxygenation acclimation is not well understood.
This study serves as a first step in characterizing the acute hypoxia tolerance among Caribbean scleractinian coral species using a metabolic framework. Given the variability among algal symbiont responses, future studies would benefit from assessing the photochemical efficiency, chlorophyll concentration, and symbiont density and species under hypoxia. Given the range of responses among coral hosts, future studies should aim to quantify the total antioxidant capacity, identify molecular changes in genes related to stress, and explore physiological changes such as total lipid content and protein concentration. Additionally, this study only assesses the short-term hypoxia tolerance, and future studies should aim to quantify the long-term effects of hypoxia exposure in multiple coral species, especially species not utilized in this study. Future studies should build upon this study by incorporating multiple methods that will provide new insights into the coral host’s molecular and physiological response and the algal symbiont photosynthetic capacity.
Data availability statement
The original contributions presented in the study are included in the article/Supplementary Material. Further inquiries can be directed to the corresponding author.
Author contributions
EP designed the experiment, collected data, analyzed data, and prepared the manuscript with contributions from CL. CL critically reviewed the manuscript, assisted with data analysis and visualization, and assisted in preparing the manuscript. FA-H assisted with data collection and critically reviewed the manuscript. All individuals have agreed to be listed as authors and approve the submitted form of the manuscript. All authors contributed to the article.
Funding
EP received financial support from the University of Miami’s Rosenstiel School of Marine, Atmospheric, and Earth Science Dean’s Office for publication of this article. This research has been performed during the sabbatical leave of FA-H from the University of Jordan, Aqaba for the academic year 2022. We also gratefully acknowledge the Marine ScienceGraduate Student Organization’s Student Travel Fund for theirconference travel funding.
Acknowledgments
The authors thank A. Wen of the Coral Reef Futures lab for collecting tissue samples and performing qPCR to determine the algal symbiont genus. We thank Z. Zagon, R. Rodriguez, C. Baum and S. Perez for their assistance with data collection during metabolic measurements. We thank H. Babbitz and K. Haider for their help with coral husbandry and constant moral support. We thank K. Nedimyer and D. Lirman for providing the coral subjects used in this study. Finally, the authors thank D. Murphy for his expert scientific writing suggestions.
Conflict of interest
The authors declare that the research was conducted in the absence of any commercial or financial relationships that could be construed as a potential conflict of interest.
Publisher’s note
All claims expressed in this article are solely those of the authors and do not necessarily represent those of their affiliated organizations, or those of the publisher, the editors and the reviewers. Any product that may be evaluated in this article, or claim that may be made by its manufacturer, is not guaranteed or endorsed by the publisher.
Supplementary material
The Supplementary Material for this article can be found online at: https://www.frontiersin.org/articles/10.3389/fmars.2023.1120262/full#supplementary-material
References
Alderdice R., Perna G., Cárdenas A., Hume B. C. C., Wolf M, Kühl M, et al. (2022). Deoxygenation lowers thermal threshold of coral bleaching. Sci Rep 12, 18273. doi: 10.1038/s41598-022-22604-3
Altieri A. H., Harrison S.B., Seemann J., Collin R., Diaz R. J., Knowlton N.. (2017). Tropical dead zones and mass mortalities on coral reefs. Proc. Natl. Acad. Sci. United States America 114 (14), 3660–3665. doi: 10.1073/pnas.1621517114
Altieri A. H., Johnson M. D., Swaminathan S. D., Nelson H. R., Gedan K. B. (2020). Resilience of tropical ecosystems to ocean deoxygenation. Trends Ecol. Evol. xx (xx), 1–12. doi: 10.1016/j.tree.2020.11.003
Altieri A. H., Gedan K. B. (2015). ‘Climate change and dead zones’. Global Change Biol. 21, 1395–1406. doi: 10.1111/gcb.12754
Alva García J. V., Klein S. G., Alamoudi T., Arossa S., Parry A. J., Steckbauer A., et al. (2022). Thresholds of hypoxia of two red Sea coral species (Porites sp. and galaxea fascicularis). Front. Mar. Sci. 9 (October). doi: 10.3389/fmars.2022.945293
Baker A., Cunning R. (2016). Bulk gDNA extraction from coral samples. Evolutionary Appl. 1991), 1–6. doi: 10.17504/protocols.io.dyq7vv
Cunning R., Baker A. C. (2013). Excess algal symbionts increase the susceptibility of reef corals to bleaching. Nat. Climate Change 3 (3), 259–262. doi: 10.1038/nclimate1711
Cunning R., Baker A. C. (2020). Thermotolerant coral symbionts modulate heat stress-responsive genes in their hosts. Mol. Ecol. 29 (15), 2940–2950. doi: 10.1111/mec.15526
Cunning R., Gillette P., Capo T., Galvez K., Baker A. C. (2015a). Growth tradeoffs associated with thermotolerant symbionts in the coral pocillopora damicornis are lost in warmer oceans. Coral Reefs 34 (1), 155–160. doi: 10.1007/s00338-014-1216-4
Cunning R., Silverstein R. N., Baker A. C. (2015b). Investigating the causes and consequences of symbiont shuffling in a multi-partner reef coral symbiosis under environmental change. Proc. R. Soc. B: Biol. Sci. 282 (1809), 1–8. doi: 10.1098/rspb.2014.1725
Deleja M., Paula J. R., Repolho T., Franzitta M., Baptista M., Lopes V., et al. (2022). Effects of hypoxia on coral photobiology and oxidative stress. Biology 11 (7), 1–17. doi: 10.3390/biology11071068
Ferguson N., White C. R., Marshall D. J. (2013). Competition in benthic marine invertebrates : the unrecognized role of exploitative competition for oxygen. Ecology 94 (1), 126–135. doi: 10.1890/12-0795.1
Gardner S. G., Raina J.-B., Ralph P. J., Petrou K. (2017). Reactive oxygen species (ROS) and dimethylated sulphur compounds in coral explants under acute thermal stress. J. Exp. Biol. 220 (10), 1787–1791. doi: 10.1242/jeb.153049
Gilmore K. L., Doubleday Z. A., Gillanders B. M. (2019). Prolonged exposure to low oxygen improves hypoxia tolerance in a freshwater fish. Conserv. Physiol. 7 (1), 1–10. doi: 10.1093/conphys/coz058
Gravinese P. M., Douwes A., Eaton K. R., Muller E. M. (2022). Ephemeral hypoxia reduces oxygen consumption in the Caribbean coral orbicella faveolata. Coral Reefs 41 (1), 13–18. doi: 10.1007/s00338-021-02197-5
Hebbeln D., Wienberg C., Dullo W.-C., Freiwald A., Mienis F., Orejas C., et al. (2020). Cold-water coral reefs thriving under hypoxia. Coral Reefs 39 (4), 853–859. doi: 10.1007/s00338-020-01934-6
Howells E. J., Abrego D., Meyer E., Kirk N. L., Burt J. A. (2016). Host adaptation and unexpected symbiont partners enable reef-building corals to tolerate extreme temperatures. Global Change Biol. 22 (8), 2702–2714. doi: 10.1111/gcb.13250
Hughes D. J., Alexander J., Cobbs G., Kühl M., Cooney C., Pernice M., et al. (2020). Coral reef survival under accelerating ocean deoxygenation. Nat. Climate Change 10 (4), 296–307. doi: 10.1038/s41558-020-0737-9
Hughes D. J., Alexander J., Cobbs G., Kühl M., Cooney C., Pernice M.. (2022). Widespread oxyregulation in tropical corals under hypoxia. Mar. pollut. Bulletin 179 (October 2021), 113722. doi: 10.1016/j.marpolbul.2022.113722
Johnson M. D., Swaminathan S. D., Nixon E. N., Paul V. J., Altieri A. H. (2021). Differential susceptibility of reef − building corals to deoxygenation reveals remarkable hypoxia tolerance. Sci. Rep. 11, 1–12. doi: 10.1038/s41598-021-01078-9
Johnston M. A., Nuttall M. F., Eckert R. J., Blakeway R. D., Sterne T. K., Hickerson E. L. (2019). Localized coral reef mortality event at East flower garden bank, gulf of Mexico. Bull. Mar. Sci. 95 (2), 239–250. doi: 10.5343/bms.2018.0057
Kealoha A. K., Doyle S. M., Shamberger K. E. F., Sylvan J. B., Hetland R. D., DiMarco S. F., et al. (2020). Localized hypoxia may have caused coral reef mortality at the flower garden banks. Coral Reefs 39 (1), 119–132. doi: 10.1007/s00338-019-01883-9
Killen S. S., Christensen E. A. F., Cortese D., Závorka L., Norin T., Cotgrove L., et al. (2021). Guidelines for reporting methods to estimate metabolic rates by aquatic intermittent-flow respirometry. J. Exp. Biol. 224 (18), 1–13. doi: 10.1242/jeb.242522
Klein S. G., Pitt K. A., Nitschke M. R., Goyen S., Welsh D. T., Suggett D. J., et al. (2017). Symbiodinium mitigate the combined effects of hypoxia and acidification on a noncalcifying cnidarian. Global Change Biol. 23 (9), 3690–3703. doi: 10.1111/gcb.13718
Kohler K. E., Gill S. M. (2006). Coral point count with excel extensions (CPCe): a visual basic program for the determination of coral and substrate coverage using random point count methodology. Comput. Geosci. 32 (9), 1259–1269. doi: 10.1016/j.cageo.2005.11.009
Mangum C., Van Winkle W. (1973). Responses of aquatic invertebrates to declining oxygen conditions. Am. Zool. 13 (2), 529–541. doi: 10.1093/icb/13.2.529
Mueller C. A., Seymour R. S. (2011). The regulation index: a new method for assessing the relationship between oxygen consumption and environmental oxygen. Physiol. Biochem. Zool. 84 (5), 522–532. doi: 10.1086/661953
Parker K. E., Ward J. O., Eggleston E. M., Fedorov E., Parkinson J. E, Dahlgren C. P., et al. (2020). Characterization of a thermally tolerant orbicella faveolata reef in abaco, the Bahamas. Coral Reefs 39 (3), 675–685. doi: 10.1007/s00338-020-01948-0
Pezner A. K., Courtney T.A., Barkley H. C., Chou W.-C., Chu H.-C., Clements S. M., et al. (2023). Increasing hypoxia on global coral reefs under ocean warming. Nat. Climate Change 13, 403–409. doi: 10.1038/s41558-023-01619-2
R Core Team. (2021). R: A language and environment for statistical computing. Vienna, Austria: R Foundation for Statistical Computing. https://www.R-project.org/.
Regan M. D., Mandic M., Dhillon R. S., Lau G. Y., Farrell A. P., Schulte P. M., et al. (2019). Don’t throw the fish out with the respirometry water. J. Exp. Biol. 222 (6), 9–10. doi: 10.1242/jeb.200253
Renaud M. L. (1983). Hypoxia in Louisiana coastal waters during 1983: implications for fisheries. Fishery Bull. 84, 19–26.
Rogers N. J., Urbina M. A., Reardon E. E., McKenzie D. J., Wilson R. W. (2016). A new analysis of hypoxia tolerance in fishes using a database of critical oxygen level (Pcrit). Conserv. Physiol. 4 (1), 1–19. doi: 10.1093/conphys/cow012
Rowan R., Powers D. (1991). Molecular genetic identification of symbiotic dinoflagellates (zooxanthellae). Mar. Ecol. Prog. Ser. 71, 65–73. doi: 10.3354/meps071065
Seibel B. A. (2011). Critical oxygen levels and metabolic suppression in oceanic oxygen minimum zones. J. Exp. Biol. 214 (2), 326–336. doi: 10.1242/jeb.049171
Seibel B. A., Andres A., Birk M. A., Burns A. L., Shaw C. T., Timpe A. W., et al. (2021). Oxygen supply capacity breathes new life into critical oxygen partial pressure (Pcrit). J. Exp. Biol. 224 (8), 1–12. doi: 10.1242/JEB.242210
Sokolova I. M., Frederich M., Bagwe R., Lannig G., Sukhotin A. A. (2012). Energy homeostasis as an integrative tool for assessing limits of environmental stress tolerance in aquatic invertebrates. Mar. Environ. Res. 79, 1–15. doi: 10.1016/j.marenvres.2012.04.003
Steckbauer A., Klein S. G., Duarte C. M. (2020). Additive impacts of deoxygenation and acidification threaten marine biota. Global Change Biol. 26 (10), 5602–5612. doi: 10.1111/gcb.15252
Stimson J., Kinzie R. A. (1991). The temporal pattern and rate of release of zooxanthellae from the reef coral pocillopora damicornis (Linnaeus) under nitrogen-enrichment and control conditions. J. Exp. Mar. Biol. Ecol. 153 (1), 63–74. doi: 10.1016/S0022-0981(05)80006-1
Ulstrup K. E., Hill R., Ralph P. J. (2005). Photosynthetic impact of hypoxia on in hospite zooxanthellae in the scleractinian coral pocillopora damicornis. Mar. Ecol. Prog. Ser. 286, 125–132. doi: 10.3354/meps286125
Vaquer-Sunyer R., Duarte C. M. (2011). Temperature effects on oxygen thresholds for hypoxia in marine benthic organisms. Global Change Biol. 17 (5), 1788–1797. doi: 10.1111/j.1365-2486.2010.02343.x
Winter R. N. (2017). Environmental controls on the reassembly of symbiodinium communities in reef corals following Perturbation : implications for reef futures under climate change (Miami, FL: University of Miami's Rosenstiel School of Marine, Atmospheric, and Earth Science).
Wood C. M. (2018). The fallacy of the pcrit - are there more useful alternatives? J. Exp. Biol. 221 (22), 1–9. doi: 10.1242/jeb.163717
Keywords: hypoxia, PO2 crit, tolerance, coral reefs, ocean deoxygenation, photosynthesis, respiration
Citation: Pontes E, Langdon C and Al-Horani FA (2023) Caribbean scleractinian corals exhibit highly variable tolerances to acute hypoxia. Front. Mar. Sci. 10:1120262. doi: 10.3389/fmars.2023.1120262
Received: 09 December 2022; Accepted: 02 May 2023;
Published: 17 May 2023.
Edited by:
Maggie D. Johnson, King Abdullah University of Science and Technology, Saudi ArabiaReviewed by:
Rachel Alderdice, University of Technology Sydney, AustraliaSilvia Arossa, King Abdullah University of Science and Technology, Saudi Arabia
Copyright © 2023 Pontes, Langdon and Al-Horani. This is an open-access article distributed under the terms of the Creative Commons Attribution License (CC BY). The use, distribution or reproduction in other forums is permitted, provided the original author(s) and the copyright owner(s) are credited and that the original publication in this journal is cited, in accordance with accepted academic practice. No use, distribution or reproduction is permitted which does not comply with these terms.
*Correspondence: Emma Pontes, ZS5wb250ZXMxQHVtaWFtaS5lZHU=