- 1Key Laboratory of Marine Biotechnology of Zhejiang Province, School of Marine Sciences, Ningbo University, Ningbo, China
- 2Ningbo Institute of Oceanography, Ningbo, China
- 3College of Food and Pharmaceutical Sciences, Ningbo University, Ningbo, China
Nitrogen is one of the main pollution sources in aquaculture system. Microalgae are considered as one of the ideal bio-absorbents used in wastewater purification, due to their nitrogen removal capacity and more importantly nutritional value. Nannochloropsis oceanica, Cyclotella atomus, and Conticribra weissflogii are famous as diet microalgae. However, estimation of nitrogen removal capability and concomitant nutritional properties of the three species have been rarely reported, which was performed in this study. N. oceanica, C. atomus, and C. weissflogii were cultivated with two initial nitrate-N concentration, noted as NC (13.85 mg·L–1) and NW (5 mg·L–1) groups, respectively. All the three microalgal strains in NC group showed higher maximum cell density, specific growth rate, and biomass concentration, maximal quantum yield of PSII (Fv/Fm), total Chlorophyll and carotenoids contents than that in NW group. These results confirmed the importance of nitrogen for microalgal biomass generation and photosynthetic performance. From Fv/Fm, N. oceanica has better adaptability towards nitrogen depletion compared to other two selected strains. The three microalgae exhibited significantly stronger nitrate-N absorption efficiencies in NC group at the same timepoint, compared to NW. Analyzing the average amount of nitrate-N absorbed by each cell daily, C. weissflogii gained the largest value, followed successively by C. atomus and N. oceanica, likely due to species specificity. Moreover, three strains removed 90% of nitrate-N within five days and 99% of that within seven days, showing splendid nitrogen removal potentials. These results confirmed the feasibility of removing nitrogen from wastewater with the selected three microalgae. Nutritional properties of microalgal biomass were also investigated. For the three species, lower nitrate-N was beneficial for the production of soluble sugar, total lipid, and saturated fatty acid, while higher nitrate-N led to more soluble protein and polyunsaturated fatty acid. In summary, N. oceanica, C. atomus, and C. weissflogii all showed strong nitrogen removal capacity, whose growth characteristics and nutritional properties varied with nitrogen concentration. In practical application for assimilating nitrogen, these findings could provide some references for the selection of suitable microalgae species in order to satisfy different nutritional requirements of various aquatic animals.
Introduction
Recently, Nature published a review characterizing the rapid development in global aquaculture in the past two decades and highlighting the integration of aquaculture in the global food system (Naylor et al., 2021). It was expected that aquaculture would contribute 52% of the world total fishery production in 2025 (Ramli et al., 2020). Inevitably, the fast expansion of largescale intensive culture activities has brought about adverse effects, especially pollutions on the aquaculture ecosystem itself and the ambient environment (Liu and Su, 2017). According to statistics, aquaculture pollution represented above 20% of the total nutrient input into freshwater in some provinces of China (Naylor et al., 2021). It was reported by Ramli et al. (2020) that over 50% of feed nitrogen turned into waste in the aquaculture system, instead of being assimilated by cultured organisms (Ramli et al., 2020). And, the extensive use of antibiotics and chemicals further aggravated the excessive accumulation of nitrogenous waste in aquaculture ecosystems, which could not be ignored (Luo et al., 2018). It has been generally accepted that nitrogen is one of the main pollution sources (Yuan et al., 2021).
Improving the ecological status of aquaculture system has become a growing concern worldwide, in particular with reducing nitrogen in wastewater effluent to diminish the occurrence of eutrophication (Mohsenpour et al., 2021). To achieve the nitrogen concentration in wastewater effluent that is in compliance with the environmental quality standards for surface water of China (GB 3838-2002), various measures have been exerted in the intensive aquaculture practices. Among them, bioremediation is considered as an innovative and optimistic technology owing to its cost-effectiveness and environmental compatibility, with a diverse range of microorganisms, including fungi, bacteria, and microalgae (Bilal and Iqbal, 2020).
Unicellular microalgae, the microscopic eukaryotic organisms with short life cycles, are capable of generating biomasses via photosynthesis (Dai et al., 2022). For one thing, as a robust source of pigments, proteins, essential fatty acids, etc., microalgae have been regarded as a promising feed additive in aquaculture to boost the growth, immunity, and meat coloration/quality of aquatic animals (Ye et al., 2022). In many independent studies, certain microalgae belonging to genera Nitzschia, Chaetoceros, Nannochloropsis, Pavlova, Chlorella, Isochrysis, Cyclotella, Thalassiosira, Porphyridium, and Phaeodactylum are famous for great potentials for use in aquaculture (Pahl et al., 2010; Haas et al., 2016; Ding et al., 2021; Ye et al., 2022). For another thing, microalgae have received much interest in implementing them in wastewater treatment, profiting from their ability to utilize nutrition sources in wastewater (Saeed et al., 2022). Extensive studies have evaluated many microalgae species in treating a range wastewater types, including municipal, brewery, agricultural, and industrial effluents (Choi, 2016; Prandini et al., 2016). Thereof, Scendesmus sp., Chlorella sp., Spirulina sp., and Chaetoceros muelleriare were widely used in the treatment of aquaculture wastewater, with varying nitrogen absorption efficiencies (Godoy et al., 2012; Ansari et al., 2017; Ahmad et al., 2020). In brief, due to the potential for nitrogen uptake and more importantly the nutritional value of algal biomass generated, microalgae have been referred as one of the ideal bio-absorbents used in wastewater purification (Mohsenpour et al., 2021). However, most of these attempts were performed independent of one another under different conditions and did not focus on the nutritional properties of microalgae. Thus, to effectively couple nitrogen removal with nutritional value, selecting the proper microalgae species is the key in this field.
Nannochloropsis sp., Cyclotella sp., and Conticribra weissflogii (previously known as Thalassiosira weissflogii) are well-known as feed ingredients or live diets for aquaculture. It was reported that a 50 % fish oil replacement by Nannochloropsis sp. meal had positive effects on the growth performance and nutrient utilization of juvenile sea bass (Haas et al., 2016). Moreover, the disease resistance of gilthead seabream (Sparus aurata) was enhanced with Nannochloropsis sp. as functional ingredient in low fishmeal diets (Jorge et al., 2019). Several studies showed that C. weissflogii contributed to the improvement of the growth performance of shrimps (Litopenaeus vannamei), emphasizing the survival rate of juveniles (Godoy et al., 2012). Similar study was also conducted with Cyclotella sp. to investigate its nutritional value as an alternative feed for aquaculture (Pahl et al., 2010). Compared with their feed potential, there are very few reports on the assessment of nitrogen removal capacity for aquaculture wastewater treatment with these three algae, especially Cyclotella sp. and C. weissflogii. Only a few attempts have been made to improve the water quality of aquaculture system by aid of Nannochloropsis sp. (Ding et al., 2021), while ignoring analyses on their nutritional properties.
Over the years, our team has been committed to separation of various diet microalgae and exploration of their nutritional properties (Yang et al., 2015; Liao et al., 2017). In our previous work, Nannochloropsis oceanica and two diatom strains (Cyclotella atomus and C. weissflogii) were screened and characterized from the rearing water of the shellfish hatchery (27°19′27″N, 120°13′0.16″E) in Fuding city, Fujian province, China. Here, the comprehensive analyses on growth performances, absorption efficiencies of nitrate-N (), and nutritional properties were conducted in N. oceanica, C. atomus, and C. weissflogii.
Materials and methods
Microalgae strain and preculture condition
N. oceanica (NMBluh014), C. atomus (NMBguh026), and C. weissflogii (NMBguh021) were provided by the Microalgae Collection Center of Ningbo University (China) and maintained in the NMB3# medium (pH 7.9, salinity 24) (Cao et al., 2019). The microalgal cultures were cultivated at 25°C and under 60 μmol photons·m–2·s–1, following a light regime of 12 h/12 h light/darkness.
Experimental design
According to the environmental quality standards for surface water of China (GB 3838-2002), the limit of nitrogen concentration in the aquaculture area is 1 mg·L–1. Based on the previous investigation on water quality in several intensive rearing ponds, the nitrogen concentration basically exceeds the limit and the main form of nitrogen is nitrate-N (Ding et al., 2021; Huang et al., 2022). In this context, this study aimed to evaluate the nitrogen absorption capacity and nutritional properties of N. oceanica, C. atomus, and C. weissflogii with nitrate-N as the only nitrogen source, with two initial concentrations. And, both of them were above the limit of 1 mg·L–1.
Reaching the post-exponential phase, the three microalgal cells were respectively collected by centrifuging and then washed three times with sterilized seawater. Collected cells were divided into two parts. One part continued to be incubated in NMB3# medium with nitrate-N of 13.85 mg·L–1, designated as NC group. The other part was incubated in the adjusted NMB3# medium with nitrate-N of 5 mg·L–1, designated as NW group. And other components were consistent with NMB3#. The initial cell density of each group was adjusted to one third of that at post-exponential phase. Cultures were all filled with ultraviolet sterilized air with the flow rate of 0.5 L·min–1 and maintained at 25 °C and under 60 μmol photons·m–2·s–1. Growth performance, total Chlorophyll content, carotenoids (Car) content, maximal quantum yield of PSII (Fv/Fm), and nitrate-N concentration were monitored on a daily basis. Until day 8, microalgal cells were harvested and stored at -80 °C for analyses of dry biomass and nutritional properties (soluble sugar, soluble protein, total lipid, and fatty acid). The experiment was performed in three biological replicates and three technical replicates for each sample.
Microalgal growth performance
To plot the growth curve, cell density was monitored by cell counting with an optical microscope. At the end of cultivation, samples (30 mL each) were harvested and lyophilized to measure the dry biomass concentration. The specific growth rate (μ) was calculated as follows.
where Nt, and N1 represented the maximum and initial density, respectively. Δt was the cultivation period (d).
Chlorophyll, carotenoids, and Fv/Fm
The contents of total Chlorophyll and Car were determined using a modified method described by Li et al. (2019). For spectrophotometric measurement of the above pigments, cells from 1.5 mL sample were centrifuged every day, resuspended in 80% acetone, and vortexed three times. The extract was recentrifuged at 14,000 g for 5 min and the supernatant was kept overnight in the dark. And then, the absorbance of the supernatant was respectively read at 450, 645, and 663 nm, in a 96-well plate reader. Total chlorophyll and Car contents were calculated using the equations of Li et al. (2019) and Singh et al. (2022). Fv/Fm was measured with a PSI fluorometer (AquaPen-C, Photon System Instruments, Czech Republic) as previous description of Li et al. (2019). All the samples were analyzed in triplicate.
Nitrate-N absorption efficiency and removal rate
To determine the nitrate-N concentration, 10 mL of algal culture was filtered with a polycarbonate membrane (0.22 μm pore-sized) every day. The filtered water was further analyzed using an automated spectrophotometer (Smart-Chem 450 Discrete Analyzer, Westco Scientific Instruments, Brookfield, USA). Referring to the description of Xu et al. (2012) and Ali et al. (2022), the nitrate-N absorption efficiency (pg·cell–1·d–1) was defined as the average amount (pg) of nitrate-N absorbed by each cell daily in this study. Nitrate-N removal rate was calculated with the equation given by Messer et al. (2022). The equations were listed as:
where C1 and Ct represented the nitrate-N concentration (mg·L–1) at day 1 and day t, respectively; Num was the initial cell density (cells·mL–1); Δt was the cultivation period (d). All the samples were analyzed in triplicate.
Soluble sugar, soluble protein, total lipid, and fatty acid
The content of soluble sugar was measured with anthracene ketone sulphuric acid colorimetric method (Tian et al., 2015). The soluble protein was quantified by Bradford Coomassie Brilliant Blue G-250 method (Bradford, 1976). The lyophilized algal powder of 10 mg was used to determine the total lipid content with the gravimetric method (Bligh and Dyer, 1959). And, chloroform and methanol were used as solvents for lipid extraction. Fatty acid extraction was conducted with KOH-CH3OH and HCl-CH3OH, following the protocol reported by Zhang et al. (2021). Fatty acid was determined with gas chromatography–mass spectrometry (GC–MS) (7890B/7000C, Agilent Technologies, USA) and further identified based on the NIST14 commercial mass spectral database. The GC–MS analysis was performed with the method of Zhang et al. (2020). All the samples were analyzed in triplicate.
Statistical analysis
Statistical analysis was performed using one-way analysis of variance (ANOVA) with SPSS 20. The difference was analyzed with a Tukey’s post hoc test and considered statistically significant when P< 0.05. All the data were displayed as mean ± SD (standard deviation) (n =3).
Results
Growth performances of three microalgae
The growth curves of N. oceanica, C. atomus, and C. weissflogii with different initial nitrate-N concentrations (NC and NW groups) were depicted in Figure 1. In the first five days, all three microalgae experienced a period of rapid proliferation, and then the trend tended to flatten out. Reasonably, the maximum cell density, specific growth rate, and biomass concentration in NC group were all significantly higher than that in NW group (p< 0.05), applicable for all three microalgae. At day 8, the biomass concentration of N. oceanica, C. atomus, and C. weissflogii in NC group was 1.59, 1.29, and 1.25 times that in NW group, respectively. These finding showed that the initial nitrate-N concentration had a substantial effect on microalgal growth performance.
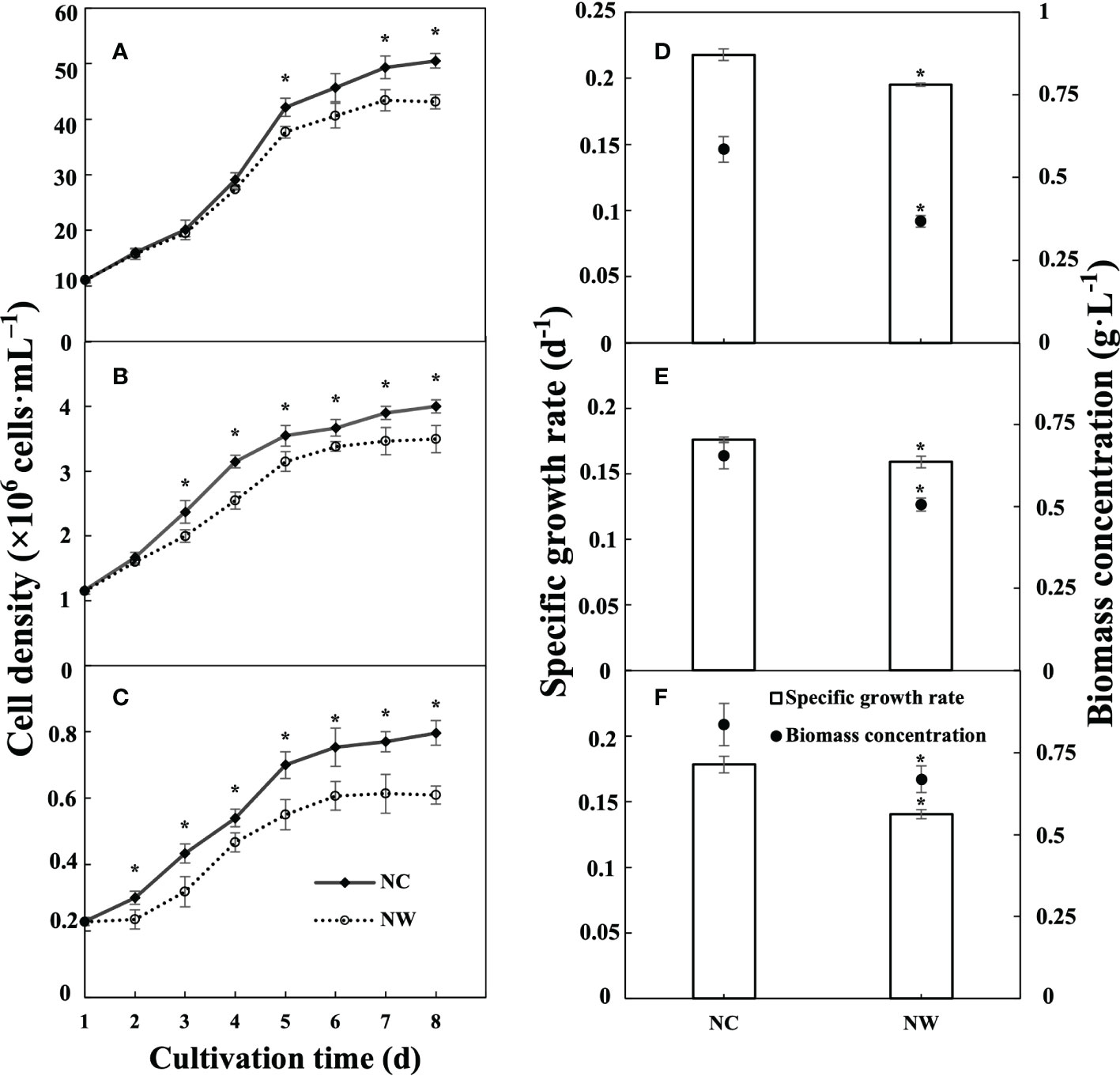
Figure 1 Growth performances of N. oceanica (A, D), C. atomus (B, E), and C. weissflogii (C, F). NC, nitrate-N concentration of 13.85 mg·L–1. NW, nitrate-N concentration of 5 mg·L–1. The one-way ANOVA analysis and the Tukey’s post hoc test were executed to estimate the differences. “*” indicates a significant difference (P< 0.05) between NC and NW at the same time point. Data are displayed as the mean ± SD (standard deviation) (n = 3).
Fv/Fm and pigment content
As a whole, the Fv/Fm values of N. oceanica, C. atomus, and C. weissflogii increased first and then decreased gradually with the process of culture (Table 1). Before day 3, there were almost no significant differences (p > 0.05) between two groups of all three microalgae. From day 4, higher values were observed in NC groups, compared to NW. At the end of cultivation, Fv/Fm values in NC groups were between 0.74 and 0.78, while that in NW groups were between 0.57 and 0.73.
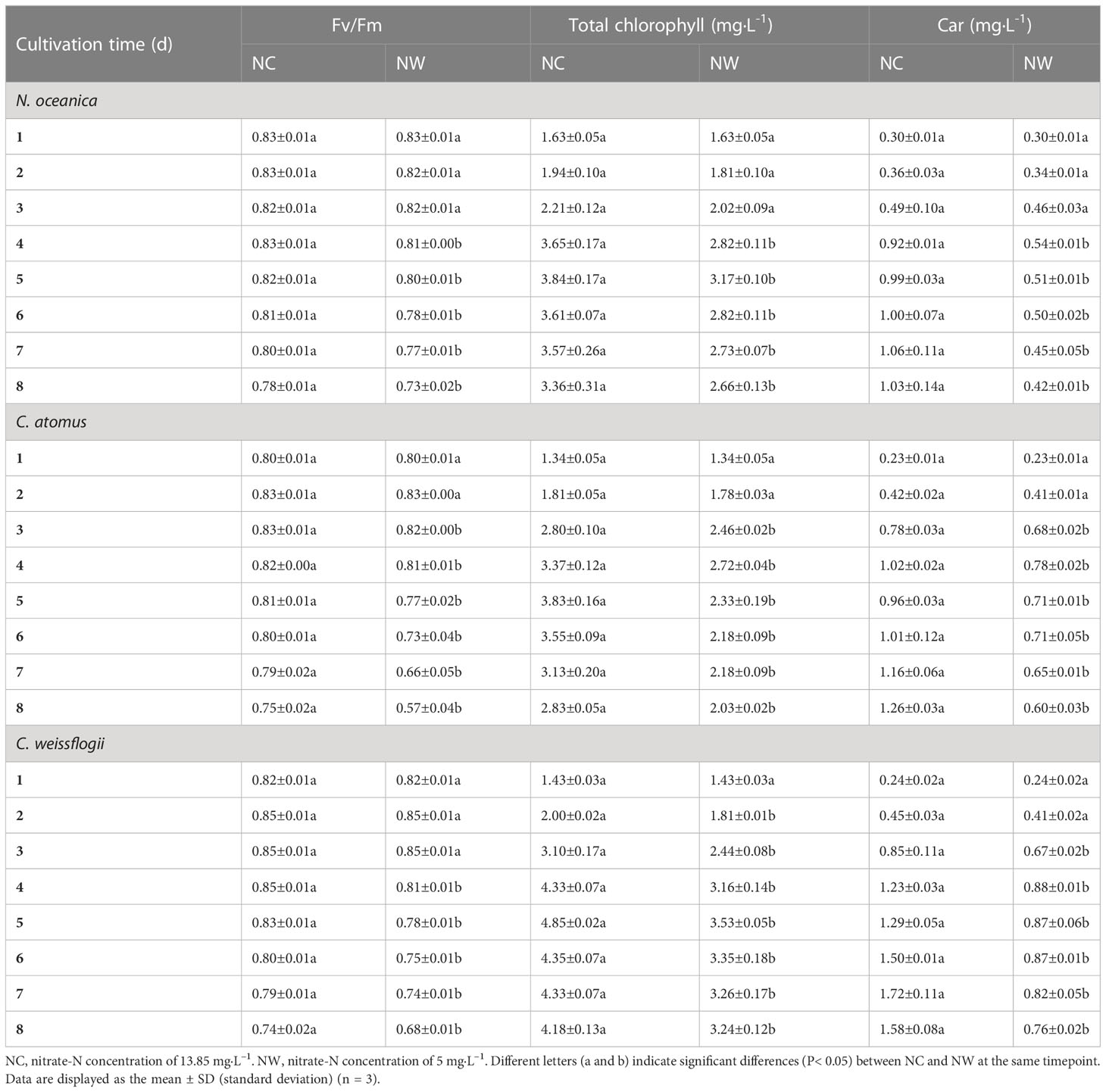
Table 1 The maximal quantum yield of PSII (Fv/Fm) and contents of total Chlorophyll and carotenoids (Car) in N. oceanica, C. atomus, and C. weissflogii in two groups.
Data on the total chlorophyll were shown in Table 1. Before day 5, total chlorophyll contents of N. oceanica, C. atomus, and C. weissflogii steadily increased both in NC and NW groups, which slightly decreased from day 6 to day 8. What is more, for the three microalgae, the total chlorophyll content in NW group saw a significant decline compared to NC (p< 0.05). These results were aligned with the growth curves of three microalgae described above. As for Car content (Table 1), the general trend was similar to total chlorophyll for all the three microalgae. However, the Car peaks of NC and NW groups occurred at different timepoints. Specifically, the highest Car content occurred at day 7, 8, and 7 in NC group for N. oceanica, C. atomus, and C. weissflogii, respectively, whereas the highest values were observed at day 4 in NW groups for all of them.
Nitrate-N utilization by microalgae
In this study, nitrate-N was served as the only nitrogen source for N. oceanica, C. atomus, and C. weissflogii, with two initial concentrations. The concentration of residual nitrate-N in cultural water was monitored on a daily basis and hence the nitrate-N absorption efficiency and nitrate-N removal rate were calculated.
Overall, the absorption efficiencies of three microalgae decreased consistently over time in all groups (Figure 2). From Figure 2A, the highest absorption efficiencies by N. oceanica were observed at day 2, i.e., 0.40 (NC) and 0.36 pg·cell–1·d–1 (NW). For C. atomus, the absorption efficiencies were between 4.61 and 1.91 pg·cell–1·d–1 in NC group and between 4.23 and 0.98 pg·cell–1·d–1 in NW group (Figure 2B). Compared to N. oceanica and C. atomus, the highest absorption efficiencies were obtained with C. weissflogii at day 2, reaching 19.02 (NC) and 16.28 pg·cell–1·d–1 (NW), respectively (Figure 2C). The absorption efficiencies in NC groups by all the three microalgae were significantly higher than NW group (p< 0.05) at the same timepoint. These results revealed that the absorption efficiency was significantly affected by the initial N concentration.
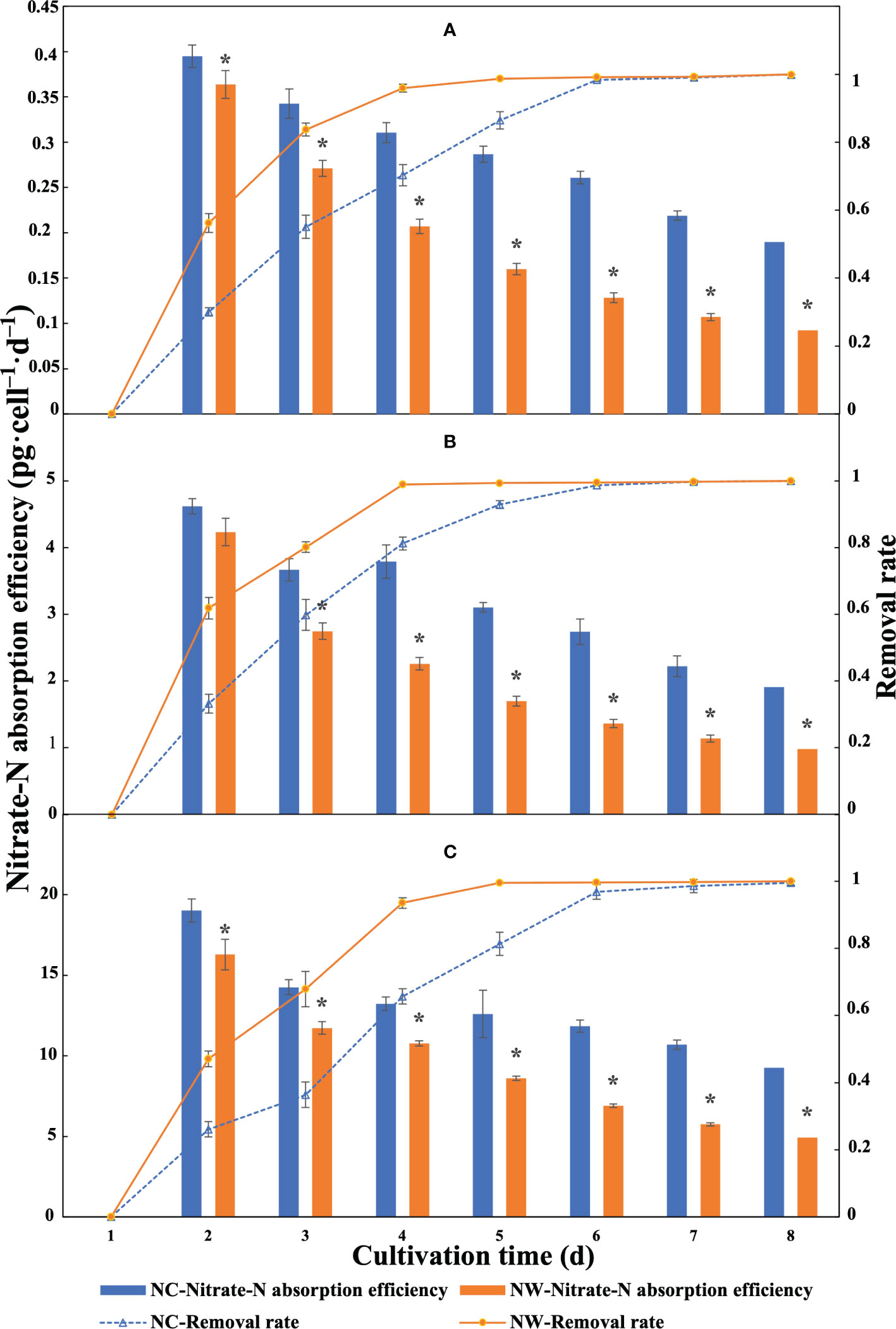
Figure 2 Nitrate-N absorption efficiency and nitrate-N removal rate of N. oceanica (A), C. atomus (B), and C. weissflogii (C). NC, nitrate-N concentration of 13.85 mg·L–1. NW, nitrate-N concentration of 5 mg·L–1. The one-way ANOVA analysis and the Tukey’s post hoc test were executed to estimate the differences. “*” above the column indicates a significant difference (P< 0.05) between NC and NW at the same timepoint. Data are displayed as the mean ± SD (standard deviation) (n = 3).
By monitoring the residual nitrate-N in cultural water every day, it was found that nitrate-N was exhausted at day 8. As shown in Figure 2, the removal rates of N. oceanica, C. atomus, and C. weissflogii all exceeded 90% at day 4 and 99% at day 5 in NW group (Figure 2). Moreover, despite with higher initial N concentration in NC groups, they could also remove 90%/99% of nitrate-N within five/seven days (Figure 2), showing excellent nitrate-N removal capacities.
Analyses on nutritional properties
At the end of cultivation, microalgal cells were respectively harvested for the assessment of nutritional composition. As displayed in Figure 3A, the soluble sugar and total lipid of N. oceanica in NW group were obviously higher (56.8% and 28.8%) than that in NC group (p< 0.05), while the soluble protein decreased by 37.3% (p< 0.05). Eleven fatty acids were identified in N. oceanica and listed in Table 2. C16:0, C16:1, C18:1 (trans-9), and C20:5 were the predominant types. C16:0 and C16:1 increased by 8.2% and 8.1% (P< 0.05) in NW group compared to NC, further leading to the significant increases in SFA and MUFA (P< 0.05). Conversely, C18:2, C20:4 (ARA), and C20:5 declined by 21.0%, 24.7%, and 30.6% (P< 0.05) in NW group compared to NC. As a result, PUFA decreased dramatically (27.4%, P< 0.05) in NW group.
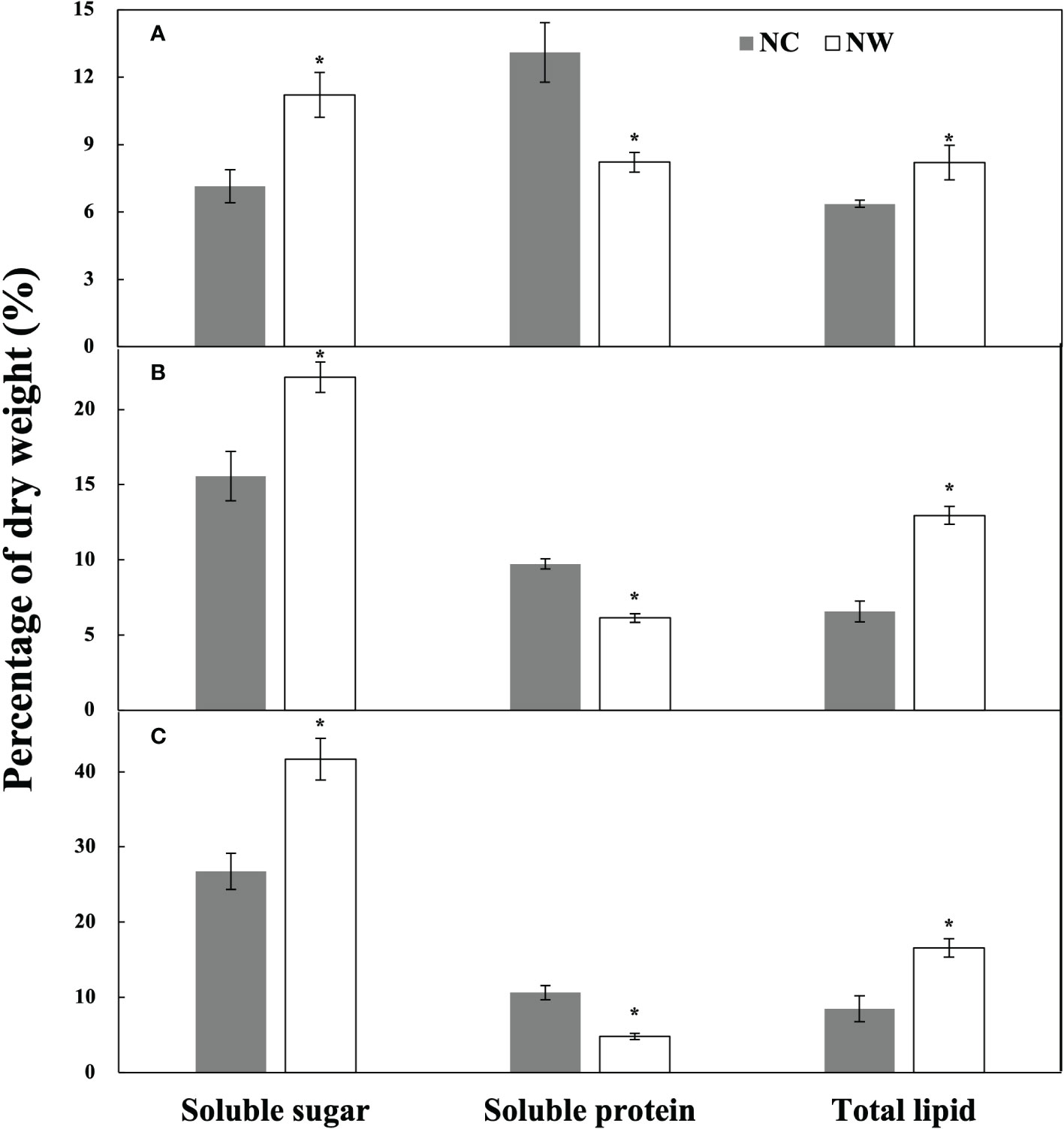
Figure 3 Contents of soluble sugar, soluble protein, and total lipid of N. oceanica (A), C. atomus (B), and C. weissflogii (C). NC, nitrate-N concentration of 13.85 mg·L–1. NW, nitrate-N concentration of 5 mg·L–1. The one-way ANOVA analysis and the Tukey’s post hoc test were executed to estimate the differences. “*” above the column indicates a significant difference (P< 0.05) between NC and NW. Data are displayed as the mean ± SD (standard deviation) (n = 3).
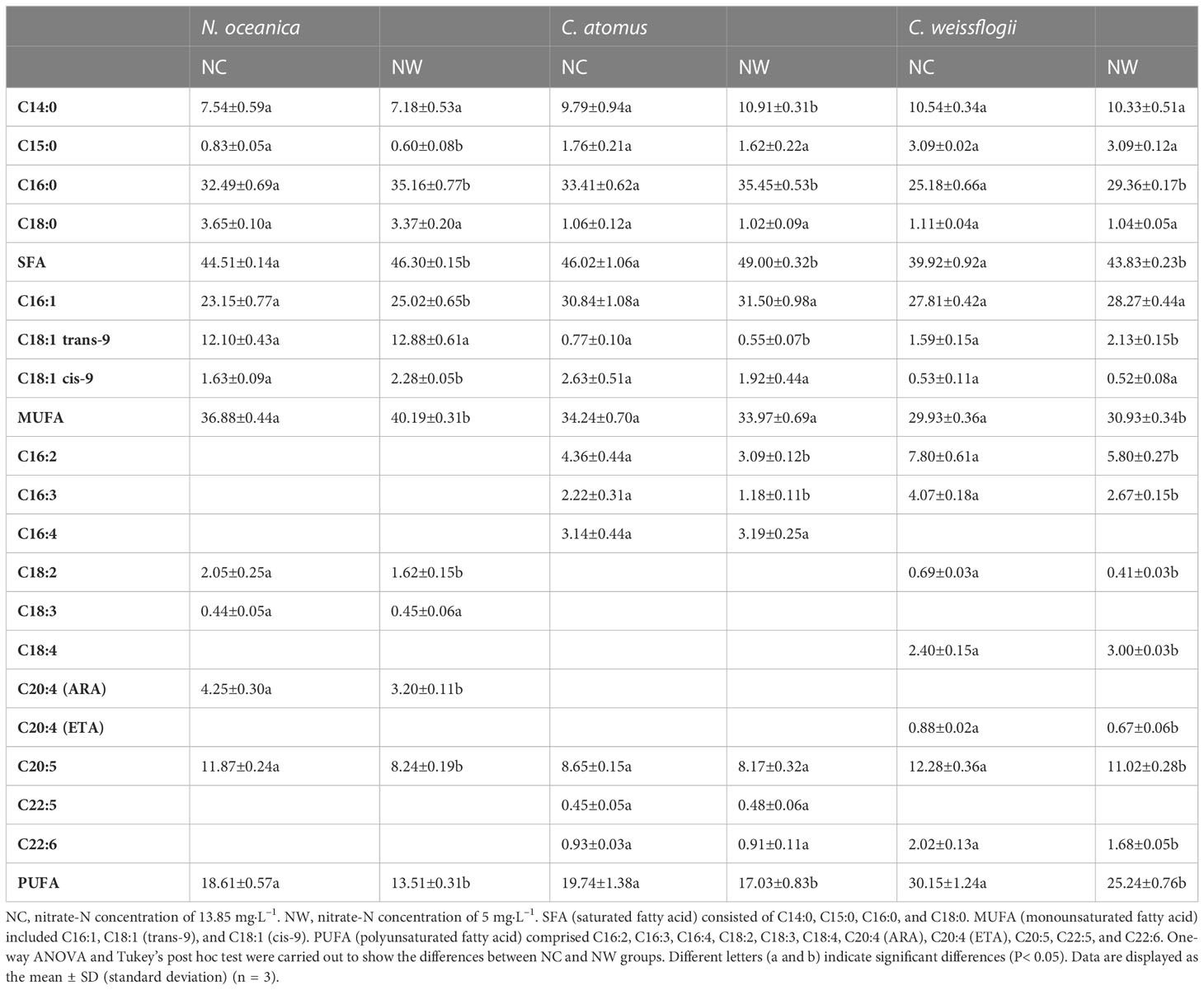
Table 2 The fatty acid composition (% of total fatty acids) of N. oceanica, C. atomus, and C. weissflogii under different nitrate-N concentrations.
As for C. atomus (Figure 3B), compared to NC, the soluble sugar and total lipid increased by 42.3% and 97.5%, while the soluble protein decreased by 36.9% (p< 0.05) in NW. Compared to N. oceanica, thirteen fatty acids were detected in C. atomus (Table 2). C14:0, C16:0, C16:1, and C20:5 were the predominant ones. SFA obviously increased in NW group compared to NC (P< 0.05), mainly attributing to the increasements of C14:0 (11.4%, P< 0.05) and C16:0 (6.1%, P< 0.05). Different from N. oceanica and C. weissflogii, no significant difference was observed in MUFA between NC and NW groups (P > 0.05). PUFA decreased significantly in NW group (13.7%, P< 0.05) by reason of the substantial reductions of C16:2 (29.1%, P< 0.05) and C16:3 (46.8%, P< 0.05).
Similar to N. oceanica and C. atomus, C. weissflogii accumulated more soluble sugar and total lipid but less soluble protein in NW group, in comparison to NC (p< 0.05) (Figure 3C). In particular, C. weissflogii seemed to produce more soluble sugars than N. oceanica and C. atomus, reaching 41.7% dry cell weight in NW group. Here, fourteen fatty acids were detected in C. weissflogii (Table 2). C14:0, C16:0, C16:1, and C20:5 accounted for the largest proportion, just like C. atomus. SFA (9.8%, P< 0.05) and MUFA (3.3%, P< 0.05) enjoyed the mark increases in NW groups compared to NC, caused by the increases of 16:0 (16.6%, P< 0.05) and C18:1 (trans-9) (33.9%, P< 0.05). What is more, higher PUFA was observed in NC group (P< 0.05).
Discussion
The demand for innovative wastewater treatment techniques has been growing in many nations, in particular with nitrogen removal. Microalgal cultivation using aquaculture wastewater is a promising technology for integrated nitrogen removal and subsequent biomass accumulation. Apart from improving water quality, microalgae have demonstrated to be an excellent living diet, which is the principal advantage of incorporating them into wastewater treatment (Mohsenpour et al., 2021). However, several practical challenges still hinder the industrial application of microalgae in this field, which need to be addressed (Mohsenpour et al., 2021). One such challenge is the availability of superior microalgal strains with optimal nitrogen removal capacity and nutritional properties. Many species of microalgae, e.g., N. oceanica, C. atomus, and C. weissflogii, have been applied in aquaculture as live diets. However, estimation of nitrogen removal capability and concomitant nutritional properties of the three species have been rarely reported. It is necessary to comprehensively analyze their growth performances, nitrogen removal capacities, and accumulations of sugar, protein, lipid, and fatty acid, which have been carried out in this study.
As referred above, nitrogen is one of the main pollution sources of aquaculture wastewater. The inorganic nitrogen forms in aquaculture wastewater comprise of nitrate, nitrite, nitric acid, ammonium, etc. (Wuang et al., 2016). Nitrate-N is the most oxidized and the most thermodynamically stable form. It is hence more common to find nitrate-N in aquaculture environments (Goncalves et al., 2017). Nitrate-N is also the main concern of this study and chosen as the nitrogen source with two concentrations, both of which exceeded the limit of the environmental quality standards for surface water of China (GB 3838-2002). For the selected three strains, there were significant differences observed in the maximum cell density, specific growth rate, biomass concentration, Fv/Fm, total chlorophyll and Car content between two groups. These results indicated nitrate-N concentration had a substantial effect on microalgal growth and photosynthetic performance. This has been a broad consensus proved in many microalgae (Ma et al., 2016). For instance, it was observed that Chlorella zofingiensis showed rapid growth with sufficient nitrogen, whereas growth inhibition under nitrogen limitation condition (Zhu et al., 2014). Additionally, the nitrogen removal rates were in line with the growth curves of the three microalgal strains in this study (Figures 1, 2), clearly reflecting the importance of nitrogen for microalgal biomass generation and confirming the feasibility of removing nitrogen from wastewater with them.
Fv/Fm is a sensitive indicator of plant photosynthetic performance (Jägerbrand and Kudo, 2016). Generally, lower values of Fv/Fm may at least partly reflect stress, photoinhibition, or downregulation of photosynthesis (Jägerbrand and Kudo, 2016). Fv/Fm has been used as an indicator of environmental stress in algae, including nutrient depletion. For example, studies in Scenedesmus obliquus, Chlorella sorokiniana, and Ankistrodesmus falcatus demonstrated that Fv/Fm values obviously decreased with nutrient depletion (Ansari et al., 2017). Another study also indicated that the negative effect of ultraviolet radiation on Isochrysis galbana was partly supported by the decline of Fv/Fm (Cao et al., 2019). In the present study, photosynthetic efficiencies of the three strains were monitored in terms of Fv/Fm. The decline of Fv/Fm values was indicative of nitrogen consumption in both groups, which was further confirmed by analyses of nitrate-N utilization (Figure 2). It is worth mentioning that Fv/Fm of N. oceanica still maintained at a high level (0.78 and 0.73), even though there was almost no residual nitrate-N in cultural water at the end of culture process (Figure 2). However, Fv/Fm values of C. atomus and C. weissflogii decrease by a much wider margin, compared to that of the first day. It can be speculated that N. oceanica has better adaptability towards nitrogen depletion compared to other two selected strains.
In the current study, N. oceanica, C. atomus, and C. weissflogii removed 90% of nitrate-N within five days and 99% of that within seven days, showing splendid nitrogen removal potentials. Nevertheless, the nitrogen absorption efficiencies were quite different from one another. Analyzing the average amount of nitrate-N absorbed by each cell daily, C. weissflogii gained the largest value, followed successively by C. atomus and N. oceanica. This was likely due to species specificity. Similarly in a comparative study by Ansari et al. (2017), A. falcatus showed stronger nitrate-N absorption capacity than S. obliquus and C. sorokiniana. An additional aspect was that the absorption efficiency in NC group was significantly higher than that in NW group at the same timepoint, applicable for all three microalgae. We speculated that it was mainly caused by the discrepancy in nitrogen concentration. In two independent studies of Wang et al. (2010) and Cabanelas et al. (2013), Chlorella sp. both showed an improved nitrogen absorption efficiency when treating wastewater with higher nitrogen concentration, similar to the results observed in this study.
Indeed, apart from microalgal species and nitrogen concentration, there are many other factors influencing nitrogen absorption efficiency. One of them is the preference of nitrogen species. The assimilation of nitrate-N requires previous reduction into ammonium, in a two-step process catalyzed by nitrate reductase and nitrite reductase, while ammonium assimilation does not (Goncalves et al., 2017). Therefore, most algae prefer ammonium as the nitrogen source rather than nitrate-N, as less energy is needed (Ramli et al., 2020). Nannochloropsis oculata, Stigeoclonium nanum, and Chlorella vulgaris fall into this category (Ansari et al., 2017; Ramli et al., 2017). There are, of course, some exceptions. For example, diatoms prefer nitrate-N over ammonium (Ramli et al., 2020). Other factors, such as light intensity and temperature, are also deemed closely correlated with nitrogen uptake by microalgae (Goncalves et al., 2017). Considering that nitrate-N is the main nitrogen form in aquaculture wastewater, this study was conducted with nitrate-N as the only nitrogen source under the same light intensity and temperature. Further investigation is needed to ascertain the impacts of other factors.
Nitrogen, one of fundamental macronutrients, is required for the accumulation of nutritional components (Zarrinmehr et al., 2020). It was reported that many microalgal species could transform protein to lipid or sugar as energy reserve component when encountering nitrogen-depletion (Huo et al., 2011). For example, studies in N. oceanica, I. galbana, and Scenedesmus sp. have demonstrated that nitrogen depletion triggered more lipids (Pancha et al., 2014; Jia et al., 2015; Zarrinmehr et al., 2020). Nitrogen starvation was thus proposed to be an effective technique for lipid production in microalgae (Yaakob et al., 2021). On the other hand, limitation of nitrogen availability also seemed to be the most promising way of accumulating sugars in microalgae (Chen et al., 2013). Zarrinmehr et al. (2020) found that the sugar content in I. galbana was enhanced by 47% under nitrogen-deficient condition. Cultivating Spirulina sp. in nitrogen-reduction medium caused the dramatical elevation in sugars (Braga et al., 2018). Additionally, there are many reports showing that nitrogen bears directly on the fatty acid profile. The common view is that low nitrogen leads to more saturated fatty acid and monounsaturated fatty acid, while high nitrogen is more conducive to the synthesis of polyunsaturated fatty acid (Zhang et al., 2021). Our results further confirmed this view. Another notable aspect was C20:5, one famous polyunsaturated fatty acid (Zhang et al., 2021). The content of C20:5 changed drastically with nitrogen concentration, especially in N. oceanica and C. weissflogii.
Over the past decades, abundant studies have clearly shown that the nutritional requirements of aquatic animals depend on different species (fish, shrimp, shellfish, etc.) and different ages (e.g., larva or juvenile) (Ayisi et al., 2017; <xr rid="r106">Li et al., 2021</xr>). The appropriate supply of nutrition is beneficial for the growth, development, and production performance of aquatic animals. According to literatures, the concentration of nitrogen in aquaculture wastewater ranges from 2-110 mg·L−1 (Ansari et al., 2017). Since the nutritional properties of microalgae varies with nitrogen concentration, the selection of suitable microalgae species should be taken into consideration in practical application for assimilating nitrogen and simultaneously producing biomass as diet for various aquatic animals. Nitrogen concentration is one of key factors in the coupling system of wastewater treatment and biomass production of microalgae. The present study was designed to estimate the growth performances, absorption efficiencies of nitrate-N, and biochemical compositions of three diet microalgae with synthetic medium. However, Microalgal treatment of real wastewater exhibited many complexities, mainly resulting from the increased physiological stresses caused by variable nutrient levels as well as high concentrations of organics in real wastewater. In the follow-up studies, the effects of other nutrient (e.g., phosphorus) on the potentials of diet microalgae for wastewater treatment will be investigated. Furthermore, cultivation with real wastewater without any additional nutrients will be carried out.
Conclusion
In this study, N. oceanica, C. atomus, and C. weissflogii were cultivated with two initial nitrate-N concentration, designated as NC (13.85 mg·L–1) and NW (5 mg·L–1) groups, respectively. Two nitrate-N concentrations both exceeded the limit of the environmental quality standards for surface water of China (GB 3838-2002). For the selected three strains, significant differences were observed in the maximum cell density, specific growth rate, biomass concentration, Fv/Fm, total chlorophyll and Car content between two groups. Judging from Fv/Fm, N. oceanica has better adaptability towards nitrogen depletion compared to other two selected strains. At the end of cultivation, the biomass concentration of N. oceanica, C. atomus, and C. weissflogii in NC group was 1.59, 1.29, and 1.25 times that in NW group, respectively. In addition, the three strains all showed excellent nitrogen removal potentials. The nitrate-N absorption efficiencies in NC group were all significantly higher than that in NW group at the same timepoint. From the average amount of nitrate-N absorbed by each cell daily, C. weissflogii gained the largest value, followed successively by C. atomus and N. oceanica. Further analyses on nutritional properties demonstrated lower nitrate-N was conductive to the synthesis of soluble sugar, total lipid, and saturated fatty acid, while higher nitrate-N resulted in more soluble protein and polyunsaturated fatty acid. To sum up, N. oceanica, C. atomus, and C. weissflogii all possessed strong nitrogen removal capacity. And their growth performance and nutritional properties were closely related with nitrogen concentration.
Data availability statement
The raw data supporting the conclusions of this article will be made available by the authors, without undue reservation.
Author contributions
LZ and JX designed the experiment. LZ analyzed the data and drafted the manuscript. JH and JX revised the paper. SM and YZ measured the microalgal growth and nitrogen removal capacity. YW contributed to the analyses of nutritional properties. All authors contributed to the article and approved the submitted version.
Funding
This work was supported by the Zhejiang Provincial Natural Science Foundation of China (grant number LY22C190001); the National Key Research and Development Program of China (grant number 2019YFD0900400); the Natural Science Foundation of Ningbo Government (grant number 2021J114); the Ningbo Science and Technology Research Projects, China (grant number 2019B10006); the Zhejiang Major Science Project, China (grant number 2019C02057); Ningbo Public Welfare Science and Technology Program (2022S161); the earmarked fund for CARS-49 and was partly sponsored by K. C. Wong Magna Fund in Ningbo University.
Conflict of interest
The authors declare that the research was conducted in the absence of any commercial or financial relationships that could be construed as a potential conflict of interest.
Publisher’s note
All claims expressed in this article are solely those of the authors and do not necessarily represent those of their affiliated organizations, or those of the publisher, the editors and the reviewers. Any product that may be evaluated in this article, or claim that may be made by its manufacturer, is not guaranteed or endorsed by the publisher.
References
Ahmad M. T., Shariff M., Yusoff F. M., Goh Y. M., Banerjee S. (2020). Applications of microalga Chlorella vulgaris in aquaculture. Rev. Aquacult. 12, 328–346. doi: 10.1111/raq.12320
Ali M. A., Ghazy A. I., Alotaibi K. D., Ibrahim O. M., Al-Doss A. A. (2022). Nitrogen efficiency indexes association with nitrogen recovery, utilization, and use efficiency in spring barley at various nitrogen application rates. Agron. J. 114, 2290–2309. doi: 10.1002/agj2.21128
Ansari F. A., Singh P., Guldhe A., Bux F. (2017). Microalgal cultivation using aquaculture wastewater: Integrated biomass generation and nutrient remediation. Algal. Res. 21, 169–177. doi: 10.1016/j.algal.2016.11.015
Ayisi C. L., Hua X., Apraku A., Afriyie G., Kyei B. A. (2017). Recent studies toward the development of practical diets for shrimp and their nutritional requirements. HAYATI J. Biosci. 24, 109–117. doi: 10.1016/j.hjb.2017.09.004
Bilal M., Iqbal H. M. (2020). Microbial bioremediation as a robust process to mitigate pollutants of environmental concern. Case Stud. Chem. Environ. Eng. 2, 100011. doi: 10.1016/j.cscee.2020.100011
Bligh E. G., Dyer W. J. (1959). A rapid method of total lipid extraction and purification. Can. J. Biochem. Physiol. 37, 911–917. doi: 10.1139/o59-099
Bradford M. M. (1976). A rapid and sensitive method for the quantitation of microgram quantities of protein utilizing the principle of protein-dye binding. Anal. Biochem. 72, 248–254. doi: 10.1006/abio.1976.9999
Braga V., Mastrantonio D.J.d. S., Costa J. A. V., de Morais M. G. (2018). Cultivation strategy to stimulate high carbohydrate content in Spirulina biomass. Bioresour. Technol. 269, 221–226. doi: 10.1016/j.biortech.2018.08.105
Cabanelas I. T. D., Ruiz J., Arbib Z., Chinalia F. A., Garrido-Pérez C., Rogalla F., et al. (2013). Comparing the use of different domestic wastewaters for coupling microalgal production and nutrient removal. Bioresour. Technol. 131, 429–436. doi: 10.1016/j.biortech.2012.12.152
Cao J., Kong Z., Ye M., Zhang Y., Xu J., Zhou C., et al. (2019). Metabolomic and transcriptomic analyses reveal the effects of ultraviolet radiation deprivation on Isochrysis galbana at high temperature. Algal. Res. 38, 101424. doi: 10.1016/j.algal.2019.101424
Chen C. Y., Zhao X. Q., Yen H. W., Ho S. H., Cheng C. L., Lee D. J., et al. (2013). Microalgae-based carbohydrates for biofuel production. Biochem. Eng. J. 78, 1–10. doi: 10.1016/j.bej.2013.03.006
Choi H. (2016). Parametric study of brewery wastewater effluent treatment using Chlorella vulgaris microalgae. Environ. Eng. Res. 21, 401–408. doi: 10.4491/eer.2016.024
Dai N., Wang Q., Xu B., Chen H. (2022). Remarkable natural biological resource of algae for medical applications. Front. Mar. Sci. 9. doi: 10.3389/fmars.2022.912924
Ding Y., Chen N., Ke J., Qi Z., Chen W., Sun S., et al. (2021). Response of the rearing water bacterial community to the beneficial microalga Nannochloropsis oculata cocultured with pacific white shrimp (Litopenaeus vannamei). Aquaculture 542, 736895. doi: 10.1016/j.aquaculture.2021.736895
Godoy L. C., Odebrecht C., Ballester E., Martins T. G., Wasielesky W. (2012). Effect of diatom supplementation during the nursery rearing of Litopenaeus vannamei (Boone 1931) in a heterotrophic culture system. Aquacult. Int. 20, 559–569. doi: 10.1007/s10499-011-9485-1
Goncalves A. L., Pires J. C. M., Simoes M. (2017). A review on the use of microalgal consortia for wastewater treatment. Algal. Res. 24, 403–415. doi: 10.1016/j.algal.2016.11.008
Haas S., Bauer J. L., Adakli A., Meyer S., Schulz C. (2016). Marine microalgae Pavlova viridis and Nannochloropsis sp. as n-3 PUFA source in diets for juvenile European Sea bass (Dicentrarchus labrax l.). J. Appl. Phycol. 28, 1011–1021. doi: 10.1007/s10811-015-0622-5
Huang C., Luo Y., Zeng G., Zhang P., Peng R., Jiang X., et al. (2022). Effect of adding microalgae to whiteleg shrimp culture on water quality, shrimp development and yield. Aquacult. Rep. 22, 100916. doi: 10.1016/j.aqrep.2021.100916
Huo Y. X., Cho K. M., Rivera J. G. L., Monte E., Shen C. R., Yan Y. J., et al. (2011). Conversion of proteins into biofuels by engineering nitrogen flux. Nat. Biotechnol. 29, 346–351. doi: 10.1038/nbt.1789
Jägerbrand A. K., Kudo G. (2016). Short-term responses in maximum quantum yield of PSII (Fv/Fm) to ex situ temperature treatment of populations of bryophytes originating from different sites in Hokkaido, northern Japan. Plants (Basel) 5, 22. doi: 10.3390/plants5020022
Jia J., Han D., Gerken H. G., Li Y., Sommerfeld M., Hu Q., et al. (2015). Molecular mechanisms for photosynthetic carbon partitioning into storage neutral lipids in Nannochloropsis oceanica under nitrogen-depletion conditions. Algal. Res. 7, 66–77. doi: 10.1016/j.algal.2014.11.005
Jorge S. S., Enes P., Serra C. R., Castro C., Iglesias P., Teles A. O., et al. (2019). Short-term supplementation of gilthead seabream (Sparus aurata) diets with Nannochloropsis gaditana modulates intestinal microbiota without affecting intestinal morphology and function. Aquacult. Nutr. 25, 1388–1398. doi: 10.1111/anu.12959
Liao K., Chen W., Zhang R., Zhou H., Xu J., Zhou C., et al. (2017). qPCR analysis of bivalve larvae feeding preferences when grazing on mixed microalgal diets. PloS One 12, e0180730. doi: 10.1371/journal.pone.0180730
Liu H., Su J. (2017). Vulnerability of china’s nearshore ecosystems under intensive mariculture development. Environ. Sci. pollut. Res. 24, 8957–8966. doi: 10.1007/s11356-015-5239-3
Li Y., Zheng M., Lin J., Zhou S., Sun T., Xu N. (2019). Darkness and low nighttime temperature modulate the growth and photosynthetic performance of Ulva prolifera under lower salinity. Mar. pollut. Bull. 146, 85–91. doi: 10.1016/j.marpolbul.2019.05.058
Li X., Zheng S., Wu G. (2021). Nutrition and functions of amino acids in fish. Adv. Exp. Med. Biol. 1285, 133–168. doi: 10.1007/978-3-030-54462-1_8
Luo Z., Hu S., Chen D. (2018). The trends of aquacultural nitrogen budget and its environmental implications in China. Sci. Rep. 8, 10877. doi: 10.1038/s41598-018-29214-y
Ma X., Liu J., Liu B., Chen T., Yang B., Chen F. (2016). Physiological and biochemical changes reveal stress-associated photosynthetic carbon partitioning into triacylglycerol in the oleaginous marine alga Nannochloropsis oculata. Algal. Res. 16, 28–35. doi: 10.1016/j.algal.2016.03.005
Messer T. L., Miller D. N., Little H., Oathout K. (2022). Nitrate-n removal rate variabilities in floating treatment wetland mesocosms with diverse planting and carbon amendment designs. Ecol. Eng. 174, 106444. doi: 10.1016/j.ecoleng.2021.106444
Mohsenpour S. F., Hennige S., Willoughby N., Adeloye A., Gutierrez T. (2021). Integrating micro-algae into wastewater treatment: A review. Sci. Total. Environ. 752, 142168. doi: 10.1016/j.scitotenv.2020.142168
Naylor R. L., Hardy R. W., Buschmann A. H., Bush S. R., Cao L., Klinger D. H., et al. (2021). A 20-year retrospective review of global aquaculture. Nature 591, 551–563. doi: 10.1038/s41586-021-03308-6
Pahl S. L., Lewis D. M., Chen F., King K. D. (2010). Growth dynamics and the proximate biochemical composition and fatty acid profile of the heterotrophically grown diatom. Cyclotella Cryptica. J. Appl. Phycol. 22, 165–171. doi: 10.1007/s10811-009-9436-7
Pancha I., Chokshi K., George B., Ghosh T., Paliwal C., Maurya R., et al. (2014). Nitrogen stress triggered biochemical and morphological changes in the microalgae scenedesmus sp. CCNM 1077. Bioresour. Technol. 156, 146–154. doi: 10.1016/j.biortech.2014.01.025
Prandini J. M., da Silva M. L. B., Mezzari M. P., Pirolli M., Michelon W., Soares H. M. (2016). Enhancement of nutrient removal from swine wastewater digestate coupled to biogas purification by microalgae Scenedesmus spp. Bioresour. Technol. 202, 67–75. doi: 10.1016/j.biortech.2015.11.082
Ramli N. M., Verdegem M. C. J., Yusoff F. M., Zulkifely M. K., Verreth J. A. J. (2017). Removal of ammonium and nitrate in recirculating aquaculture systems by the epiphyte Stigeoclonium nanum immobilized in alginate beads. Aquac. Environ. Interact. 9, 213–222. doi: 10.3354/aei00225
Ramli N. M., Verreth J. A. J., Yusoff F. M., Nurulhuda K., Nagao N., Verdegem M. C. J. (2020). Integration of algae to improve nitrogenous waste management in recirculating aquaculture systems: A review. Front. Bioeng. Biotech. 8, 1004. doi: 10.3389/fbioe.2020.01004
Saeed M. U., Hussain N., Sumrin A., Shahbaz A., Noor S., Bilal M., et al. (2022). Microbial bioremediation strategies with wastewater treatment potentialities - a review. Sci. Total. Environ. 818, 151754. doi: 10.1016/j.scitotenv.2021.151754
Singh V., Mandhania S., Pal A., Kaur T., Banakar P., Sankaranarayanan K., et al. (2022). Morpho-physiological and biochemical responses of cotton (Gossypium hirsutum l.) genotypes upon sucking insect-pest infestations. Physiol. Mol. Biol. Plants 28, 2023–2039. doi: 10.1007/s12298-022-01253-w
Tian X., He M., Wang Z., Zhang J., Song Y., He Z., et al. (2015). Application of nitric oxide and calcium nitrate enhances tolerance of wheat seedlings to salt stress. Plant Growth Regul. 77, 343–356. doi: 10.1007/s10725-015-0069-3
Wang L., Min M., Li Y., Chen P., Chen Y., Liu Y., et al. (2010). Cultivation of green algae chlorella sp. in different wastewaters from municipal wastewater treatment plant. Appl. Biochem. Biotechnol. 162, 1174–1186. doi: 10.1007/s12010-009-8866-7
Wuang S. C., Khin M. C., Chua P. Q. D., Luo Y. D. (2016). Use of Spirulina biomass produced from treatment of aquaculture wastewater as agricultural fertilizers. Algal. Res. 15, 59–64. doi: 10.1016/j.algal.2016.02.009
Xu G., Fan X., Miller A. J. (2012). Plant nitrogen assimilation and use efficiency. Annu. Rev. Plant Biol. 63, 153–182. doi: 10.1146/annurev-arplant-042811-105532
Yaakob M. A., Mohamed R. M. S. R., Al-Gheethi A., Aswathnarayana Gokare R., Ambati R. R. (2021). Influence of nitrogen and phosphorus on microalgal growth, biomass, lipid, and fatty acid production: An overview. Cells 10, 393. doi: 10.3390/cells10020393
Yang F., Chen S., Miao Z., Sheng Z., Xu J., Wan J., et al. (2015). The effect of several microalgae isolated from East China Sea on growth and survival rate of postset juveniles of razor clam, Sinonovacula constricta (Lamarck 1818). Aquacult. Nutr. 22, 846–856. doi: 10.1111/anu.12310
Ye B., Gu Z., Zhang X., Yang Y., Wang A., Liu C. (2022). Comparative effects of microalgal species on growth, feeding, and metabolism of pearl oysters, Pinctada fucata martensii and Pinctada maxima. Front. Mar. Sci. 9. doi: 10.3389/fmars.2022.895386
Yuan B., Yue F., Wang X., Xu H. (2021). The impact of pollution on China marine fishery culture: An econometric analysis of heterogeneous growth. Front. Mar. Sci. 8. doi: 10.3389/fmars.2021.760539
Zarrinmehr M. J., Farhadian O., Heyrati F. P., Keramat J., Koutra E., Kornaros M., et al. (2020). Effect of nitrogen concentration on the growth rate and biochemical composition of the microalga, Isochrysis galbana. Egypt. J. Aquat. Res. 46, 153–158. doi: 10.1016/j.ejar.2019.11.003
Zhang L., Yang S., Xu J., Liu T., Yang D., Wu Z., et al. (2020). Application of exogenous salicylic acid on improving high temperature resistance of Nannochloropsis oceanica. Aquacult. Int. 28, 2235–2246. doi: 10.1007/s10499-020-00592-3
Zhang L., Ye S., Chen W., Han J., Tian J., Zhang Y., et al. (2021). Screening the rate-limiting genes in the ω6 polyunsaturated fatty acid biosynthesis pathway in Nannochloropsis oceanica. Algal. Res. 57, 102342. doi: 10.1016/j.algal.2021.102342
Keywords: aquaculture, nitrate-N, microalgae, diet, growth, nitrogen removal capacity, nutritional property
Citation: Zhang L, Han J, Ma S, Zhang Y, Wang Y and Xu J (2023) Comprehensive evaluation of growth characteristics, nitrogen removal capacity, and nutritional properties of three diet microalgae. Front. Mar. Sci. 10:1117043. doi: 10.3389/fmars.2023.1117043
Received: 06 December 2022; Accepted: 27 January 2023;
Published: 03 February 2023.
Edited by:
Zhangying Ye, Zhejiang University, ChinaReviewed by:
Zengling Ma, Wenzhou University, ChinaTonmoy Ghosh, Indian Institute of Technology Indore, India
Copyright © 2023 Zhang, Han, Ma, Zhang, Wang and Xu. This is an open-access article distributed under the terms of the Creative Commons Attribution License (CC BY). The use, distribution or reproduction in other forums is permitted, provided the original author(s) and the copyright owner(s) are credited and that the original publication in this journal is cited, in accordance with accepted academic practice. No use, distribution or reproduction is permitted which does not comply with these terms.
*Correspondence: Jilin Xu, eHVqaWxpbkBuYnUuZWR1LmNu