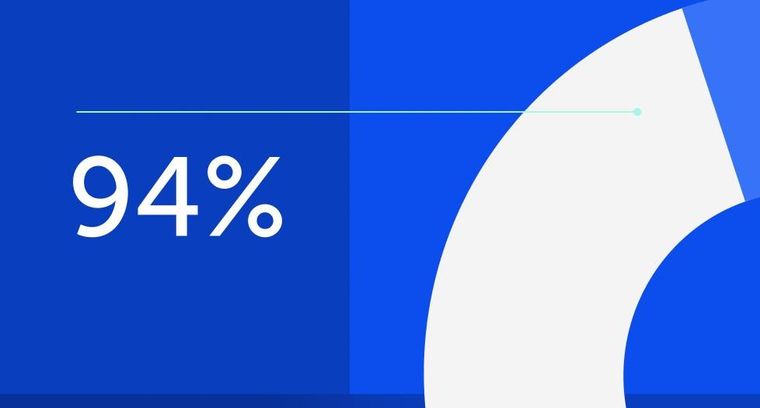
94% of researchers rate our articles as excellent or good
Learn more about the work of our research integrity team to safeguard the quality of each article we publish.
Find out more
ORIGINAL RESEARCH article
Front. Mar. Sci., 27 February 2023
Sec. Aquatic Physiology
Volume 10 - 2023 | https://doi.org/10.3389/fmars.2023.1114120
Water temperature plays a crucial role in the growth, survival, and reproduction of fish species, as they make up the majority of aquatic fauna. In this study, the effects of low temperature were studied on the functional state of juvenile golden pompano (Trachinotus ovatus) under low-temperature stress. The study was conducted at 28°C in the control group and 18°C in the cold group for 14 d to determine the intestinal tissue, digestive and antioxidant enzyme activities, and metabolites of juvenile fish. The results showed that: (1) the swelling degree of the muscle layer deepened and was congested with a longer low-temperature stress period. The folds were sparse, from slight swelling to shedding and deformation. The intestinal mucosa was necrotic and had vacuoles, and the number gradually increased. Serious erosion of the villi occurred. (2) The specific activities of digestive enzymes showed a downward trend. (3) The intestinal superoxide dismutase (SOD) activity, malondialdehyde (MDA) content, and glutathione peroxidase (GSH-Px) activity showed an upward trend. The intestinal catalase (CAT) activity showed a downward trend. (4) Compared with the control group, there were 28 metabolites in the cold group showing significant differences, among which Z, 11Z, 14Z-eicosatrienoic acid, stearic acid, and adrenic acid showed an upward trend. In contrast, spermidine and uracil showed a downward trend. Among the enriched metabolic pathways, the main differential pathways were unsaturated fatty acid biosynthesis, fatty acid biosynthesis, linoleic acid metabolism, pyrimidine metabolism, and β-alanine metabolism. According to metabolomic analysis, under low-temperature stress, the fish body improved the synthesis of unsaturated fatty acids and saturated fatty acids to adapt to a low-temperature environment and consumed spermidine to improve its immune ability to clear the peroxide generated by the synthesis of unsaturated fatty acids in the body so that the cells were protected from oxidative damage. After 14 days, low-temperature stress affected metabolites and enzyme activity indices in juvenile golden pompano. Low-temperature stress causes changes in intestinal antioxidants and digestive enzymes and damage intestinal tissues. As a result of this exploration of how low temperatures affect the juvenile golden pompano, the foundation is laid for future studies, such as the molecular mechanisms of low-temperature adaptation in fish species.
In the context of global climate change and differences in seasonal temperatures, the impact of temperature changes on biological systems is a relevant topic. Due to the increased climate variability, extreme temperature events, such as cold snaps, occur more often and intensely (IPCC (Intergovernmental Panel on Climate Change), 2012; Tingley and Huybers, 2013; Abram et al., 2017). An example of these extreme temperature events was the cold weather in the Gulf of Mexico in 2010, causing the air and water temperatures to drop 12°C and 6°C, respectively, over two weeks and leading to widespread fish mortality (Pirhalla et al., 2015). Water temperature is an important factor affecting fish culture, which affects fish’s growth, development, fish’s ability to obtain food and digestion, nutrient absorption, and energy storage, and physiological activities (Bowden, 2008; Abram et al., 2017; Hassenrück et al., 2020; Volkoff and Rønnestad, 2020; Cai R-J et al., 2021). Temperature effects on fish are complex because they vary in habitats, feeding habits, anatomical features, and physiological characteristics (Volkoff and Rønnestad, 2020). When the external water temperature drops, a series of physiological stress reactions occur in fish itself, disturbing its normal physiological activities and causing its death (Hassenrück et al., 2020). Low-temperature stress is an important means to study the cold tolerance mechanism of fish (Wang et al., 2022). The study of low-temperature stress includes cold acclimation and the sudden drop in water. Cold acclimation refers to the slow decrease of water temperature, which causes a compensatory mechanism inside the fish to maintain the steady state of the internal environment (Islam et al., 2021; Schleger et al., 2021; Wang et al., 2022). The sudden drop in water temperature will break this stable situation and cause the fish to respond to stress (Goos and Consten, 2002). In general, voluntary food consumption is impacted by temperature depending on the species, but when temperatures are moderate, voluntary consumption increases, and when temperatures are outside the fish’s optimal temperature range, voluntary food consumption decreases (Ahmad et al., 2014; Sharma et al., 2017; Nadermann et al., 2019; Nguyen et al., 2019; Volkoff and Rønnestad, 2020). According to available data, fish lose their appetite, cease ingesting food, and eventually stop eating at temperatures well below their max critical temperature (Shafland and Pestrak, 1982; Volkoff and Rønnestad, 2020).
The intestine is a key and the largest immune organ in the body for lipid absorption, and intestinal enzymes play a key role in the digestion and absorption of nutrients after ingesting (Bates et al., 2007; Llewellyn et al., 2014; Sæle et al., 2018) and producing anticarcinogenic and anti-inflammatory substances (Hamer et al., 2009). It was the first barrier to the body’s defense system and played an important role in maintaining the body’s normal nutrition, metabolism, and immune defense (Lall, 2020). As an important mucosal system of fish, the intestinal mucosa is an effective barrier to maintain intestinal health due to its integrity of morphology, structure, and function (Lin et al., 2020). A variety of beneficial functions are associated with the fish gut, including mediating and stimulating optimal gastrointestinal growth (Bates et al., 2007; Hassenrück et al., 2020), producing and supplying vitamins to the organism (Tsuchiya et al., 2008; Roeselers et al., 2011), and providing the organism with additional enzymes that improve nutrient uptake (Llewellyn et al., 2014). Intestinal microvilli, mucosal fold height, villus width, and muscularis thickness are important indicators for evaluating intestinal function (Di Maiuta et al., 2013; Hassenrück et al., 2020; Cheng et al., 2022). Stress leads to a significant decrease in the number of goblet cells and an increase in the number of vacuoles in the intestinal tissue of hybrid yellow catfish (Pelteobagrus vachelli ♂ × Pelteobagrus fulvidraco ♀). With the prolonged stress time, the intestinal villi were disorderly arranged and erosive (Cheng et al., 2022).
Despite the widespread acknowledgment of the importance of fish gut (Egerton et al., 2018; Butt and Volkoff, 2019) and extensive research on their use in aquaculture (Llewellyn et al., 2014; Perry et al., 2020; Yukgehnaish et al., 2020), a substantial amount of information is still missing about tropical aquaculture species, such as golden pompano (Trachinotus ovatus), and how low thermal stress affects gut interactions with the host. Climate change projections include rapid water temperature changes that continue to be a great concern. Golden pompano raised outdoors in aquaculture will have to cope with water temperature-changing conditions in the future. At present, metabolomic methods are used to study the temperature stress of fish, such as sea bass (Dicentrarchus labrax) (Zhang et al., 2021), Nile tilapia (Oreochromis niloticus) (Hu et al., 2020), and flounder (Paralichthys olivaceus) (Liu et al., 2022).
The golden pompano, belonging to Perciformes, and Carangidae, is mainly distributed in the warm tropical waters of the Pacific, Indian, and Atlantic Oceans. With delicate meat, high nutritional value, short breeding cycle, and the use of compound feed throughout the whole process, the golden pompano has become one of the important mariculture fish in the coastal areas of South China, and the production scale is increasing (Sun et al., 2019). The effects of climate change on oceans, rivers, and lakes are significant. Understanding how fishes cope with low temperatures is crucial as well as how environmental fluctuations affect fish biology. It is very important to implement the necessary conservation measures for aquatic organisms. There is a lack enough information on how fishes react to low temperatures biologically. Temperature changes significantly affect fish physiological parameters. Fishes must modify their endocrine processes, metabolic rates, and immune and antioxidant systems to adapt to environmental temperature changes. In the course of the production of golden pompano, due to seasonal changes, climate changes, and cold waves, the water temperature has rhythmic or sudden temperature changes, which causes a certain degree of low-temperature stress. We previously found that when the water temperature dropped to 18°C, the golden pompano showed stress responses, such as low feeding capacity and reduced swimming activity. The mechanism of how the fish body adapts to the low-temperature environment through self-regulation is further explored (Wegner et al., 2015; Soyano and Mushirobira, 2018). The research on the intestinal response of golden pompano to low-temperature stress needs to be further explored. In this study, the structure, physiology, and metabolite changes of the intestine in response to low-temperature stress were studied to understand the mechanism of intestinal adaptation to low temperature and provide a scientific basis for the healthy breeding and cold tolerance mechanism of golden pompano.
The fish were derived from juveniles bred by the fish seed engineering and breeding team of the Fisheries College of Guangdong Ocean University, Zhanjiang, China, in the biological research base of Donghai Island. A total of 300 healthy individuals were randomly selected and transported to the breeding base of Guangdong Evergreen Feed Industry Co., Ltd., Zhanjiang, China, with a special fry transport vehicle (Wang W-Z et al., 2021). The breeding facility was an indoor 300 L breeding tank and a 24 h continuous aerated micro-flow aquaculture system. The special compound feed was fed once a day (Guangdong Evergreen Feed Industry Co., Ltd., China). Feed nutrient composition was as follows: crude protein content (≥39%), crude fat content (≥2.5%), crude fiber content- (≤8.0%), and crude ash content (≤10%). The feces and the remaining feed were cleaned up 1 h after feeding. The experiment started after two weeks of temporary rearing.
The aquaculture water was natural seawater after sedimentation and sand filtration, and the water quality conformed to the national fishery water quality standard (GB 11607-89) (national fishery water quality standard (GB 11607-89)). During the holding period, dissolved oxygen (DO) in the water (≥5 mg/L), pH (7.8-8.0), salinity (28.8 ± 0.5 ppt), and total ammonia nitrogen (0.10 ± 0.03 mg/L) were maintained. At 8:00 and 17:00 every day, these parameters were measured. The water temperature was 28 ± 0.5°C. The daily water exchange rate was 1/2, and the temperature difference before and after water exchange was not greater than 0.5°C.
After the temporary rearing, the juvenile golden pompano with good health and vitality, consistent specifications, an initial body weight of 336.88 ± 20.85 g, and a body length of 25.35 ± 1.56 cm were selected for the experiment. After 24 h of fasting, the fish were randomly divided into low-temperature stress and control groups. Each treatment group was set with 3 replicates each of 30 fish. The water temperature of the low-temperature stress group was 18°C, and the water temperature of the control group was 28°C. The cooling mode was slightly modified according to the method described by Cai R et al. (2021). The specific operation was as follows: the water in the control group was used as the initial water temperature, the ice cubes were put into the plastic bag and sealed, and then put them into the water for cooling. The water temperature was controlled by the temperature-control heating rod, and the water temperature was reduced to 18 ± 0.5°C at 0.5°C/h speed. Then, the low-temperature treatment group was maintained until the end of the experiment. In the control group, a temperature-controlled heating rod was used to maintain the water temperature at 28 ± 0.5°C. During the experiment, the microflow water was turned off. The gas was aerated continuously, and 50% of the isothermal fresh seawater was replaced daily.
The control group was kept fed at 8:00 and 17:00 every day, and the low-temperature stress group was fed a small amount of food every day due to the low appetite of the juveniles. The DO in the water body was maintained above 5 mg/L, and ammonia nitrogen (≤0.02 mg/L), pH (7.8-8.0), and salinity (28-30) were also maintained. The water temperature was measured every 30 min, and the temperature difference before and after the water change was not greater than 0.5°C. According to the pre-experiment, the experimental period was set to 14 d. At the sampling time points of 1 d, 4 d, 7 d and 14 d, ten fish were randomly selected from both control and low-temperature treatment groups. After rapid anesthesia with eugenol (200 mg/L), weighing was performed, and ten fish from each group were then placed on ice for dissection. For the effect of hypothermia on intestinal structure, the changes of intestinal villi, folds, intestinal mucosa, and intestinal tissue vacuoles were observed in the hypothermia group and the control group on the 1st, 4th, 7th and 14th day by tissue sectioning, and evaluated the effect of hypothermia on intestinal structure using the midgut. The intestines were taken out and cut into small pieces, rinsed in 0.9% normal saline, and a part of the intestine was fixed in 4% paraformaldehyde (Shanghai yuanye Bio-Technology Co., Ltd., China) for tissue sectioning. The other part of the intestine was stored in a 2 mL cryopreservation tube to liquid nitrogen and transferred at -80°C for subsequent analysis. Five biological replicates of midgut samples were used in the control group and the hypothermia group for metabolomic analysis. Three biological treatments midgut samples were used for histological analysis and specific digestive enzyme activity analysis.
Following the method normally used in our laboratory as stated by Fu et al., 2022b, the intestinal tissue structure was observed. Briefly, the fixed intestinal tissues were first dehydrated with a series of concentrations of ethanol in a gradient manner. Secondly, the tissues were made transparent using xylene. Thirdly, the paraffin-impregnated tissues were put in an embedding machine and then made into sections of 4 μm with a microtome. The paraformaldehyde-fixed intestine was taken, rinsed with water, dehydrated with 70%-95% ethanol, and transparented with xylene. After paraffin embedding, the intestine was sectioned with Leica and a microtome with a thickness of 5-6 μm. Finally, the sections were stained via hematoxylin and eosin (H&E) and sealed with the neutral resin. The sections were observed using the ortho-fluorescence microscope (Nikon ECLIPSE Ni-E, Japan) to measure and record the fold height, fold width, and muscular thickness of the PI, MI, and DI in hybrid grouper.
Following the methods normally used in our laboratory (Laboratory of Fish Seed Engineering and Healthy Farming) (Huang et al., 2020; Zhang et al., 2021; Amenyogbe et al., 2022a; Fu et al., 2022a; Amenyogbe et al., 2022b; Fu et al., 2022b; Amenyogbe et al., 2022c), the activities of total protein, α - amylase (AMS), lipase (LPS), trypsin (TPS), catalase (CAT), glutathione peroxidase (GSH-Px), superoxide dismutase (SOD) and the content of Malondialdehyde (MDA) in intestinal tissues of the juvenile were determined by test kits (Nanjing Jiancheng Technology Co., Ltd., China). The cryopreserved intestinal tissue was thawed and weighed accurately. Nine times of normal saline was added according to the weight (g) ratio: Volume (ML) = 1:9. Three frozen intestinal tissues samples were put together and homogenized in an ice water bath for 3-5 min and centrifuged at 4°C and 2500 r/min for 10 min. According to the manufacturer’s instructions, the supernatant was used to determine the total protein content, AMS, LPS, TPS, CAT, GSH-Px, SOD, and MDA. The total protein concentration was determined by total protein quantitative assay kit (Nanjing Jiancheng Technology Co., Ltd.), of which coomassie brilliant blue was main chemical agent. Amylase adopts the starch-iodine colorimetric method, which is defined as the reaction of each milligram of protein in the tissue with the substrate for 30 min at 37°C, and the hydrolysis of 10 mg starch is defined as 1 unit of enzyme activity. Lipase adopts a colorimetric method, which is defined as the reaction of each gram of tissue protein with the substrate in this reaction system for 1 min at 37°C, and each consumption of 1 μmol of the substrate is an enzyme activity unit. Trypsin adopts the ultraviolet colorimetric method, which is defined as a unit of enzyme activity when the trypsin contained in each milligram of protein changes the absorbance by 0.003 per minute under the reaction of pH 8.0 and 37°C (Yang et al., 2021).
The thiobarbituric acid (TBA) method was used for MDA. The reaction system produces a red product, and its absorbance was measured at 532 nm wavelength to calculate the content of MDA. CAT was determined by the ammonium molybdate method (Amenyogbe et al., 2022c; Fu et al., 2022a). The amount of hydrogen peroxide (H2O2) decomposed by 1 umol per milligram of tissue protein per second was defined as one activity unit. GSH-Px was determined by colorimetry. The effect of nonenzymatic reaction was deducted per milligram of protein per minute, and the GSH concentration in the reaction system was reduced by 1 umol/L as an enzyme activity unit. The hydroxylamine method was used for SOD (Amenyogbe et al., 2022c; Fu et al., 2022a). The enzyme amount corresponded to 1 mg tissue protein when the sod inhibition rate was 50% in 1 mL reaction solution was defined as the SOD activity unit. The corresponding operation steps were described in the manufacturer’s instructions.
The metabolite extraction and detection analysis were done following the method described by Yang et al. (2022) with modifications. In the control and low-temperature treatment groups (cold group), the intestinal tissues of 6 fish were randomly selected for metabonomic sequencing. We weighed 100 ± 2 mg of sample into a 2 mL EP tube, added 1 mL of tissue extract (V methanol: V chloroform = 3:1), ground it twice in a tissue grinder (50 Hz, 60 s), gave an ultrasonic wave at room temperature for 30 min, and placed it on ice for 30 min, and then centrifuged at 12000 rpm and 4°C for 10 min. We took 650 μL of the supernatant, transferred it to a 2 mL centrifuge tube and concentrated in a vacuum concentrator until it was completely dry. Subsequently, 200 μL of 50% acetonitrile solution (Shanghai yuanye Bio-Technology Co., Ltd., China) was added to configure 2-chlorophenylalanine solution (Shanghai yuanye Bio-Technology Co., Ltd., China) (4 ppm) for reconstitution, filter, and take the filtrate for liquid chromatography–mass spectrometry (LC-MS) detection.
Chromatographic analysis was performed with the Thermo Vanquish instrument with ACQUITY UPLC® HSS T31.8 μm (2.1×150 mm) chromatographic column. The autosampler performed a 2μL gradient elution. Temperature (8°C), flow rate (0.25 mL/min), and column temperature (40°C) were maintained. The mobile phase was positive ions (0.1% formic acid water (B1)-0.1% formic acid acetonitrile (A1)) and negative ions (5 mM ammonium formate water (B3)-acetonitrile (A3)). The gradient elution program was as follows: 0~1 min, 2% A1/A3; 1~9 min, 2%~50% A1/A3; 9~12 min, 50%~98% A1/A3; 12~13.5 min, 98% A1/A3; 13.5~14 min, 98%~2% A1/A3; 14~20 min, 2% A1-positive mode (14~17 min, 2%A3-negative mode).
Mass spectrometry was conducted using a Thermo Q Exactive Plus instrument with an electrospray ion source (ESI) and positive and negative ion ionization modes. The positive ion spray voltage was 3.50 kV; negative ion spray voltage was 2.50 kV; sheath gas was 30 arb; auxiliary gas was 10 arb. The capillary temperature was 325°C; full scan was provided with a resolution of 70 000, scan range (81~1000). Higher-energy collision-induced dissociation (HCD) was used for secondary cracking with a collision voltage of 30 eV. Dynamic exclusion was used to remove unnecessary MS/MS information. In addition, 20 μL of each sample to be tested was mixed into a QC sample to evaluate the stability of the LC-MS during the entire data collection process.
Following the method described by Yang et al. (2022), the original data obtained were converted into mzXML format by Proteowizard software (v3.0.8789), and R’s XCMS package (Smith et al., 2006) was used for peak identification, peaks filtration, and peaks alignment. The main parameters included bw=5, ppm=15, peakwidth=c(5,30), mzwid=0.015, mzdiff=0.01, and method=centWave. Furthermore, a data matrix of information, such as mass-to-charge ratio (m/z), retention time, and intensity, was obtained. The data of precursor molecules obtained in positive and negative ion modes were imported into Excel. Then, the data was compared after batch normalization. Finally, the metabolites were identified based on precise molecular weight (molecular weight error <20 ppm), and metabolites were annotated for Metlin (http://metlin.scripps.edu), MoNA (https://mona.fiehnlab.ucdavis.edu//) and self-built data based on MS/MS fragmentation mode.
We used the R package gmodels to perform principal component analysis (PCA) analysis on the data and also used the R language ropls package to perform supervised, orthogonal partial least squares discriminant analysis (OPLS-DA). Seven cross-validations were used to evaluate the robustness and predictive ability of the model to verify its reliability of the model (Hao et al., 2019). The variable important in the projection (VIP) value of OPLS-DA and the P-value of the t-test of univariate statistical analysis were combined to screen the significantly different metabolites between different comparison groups. The threshold value was VIP≥1, and the t-test (P<0.05). Finally, the differential metabolites were compared to the Kyoto Encyclopedia of Genes and Genomes (KEGG) database for pathway enrichment analysis (Liu et al., 2020).
Graphpad prism 5 software was used to process and map the data. A paired t-test was used to analyze the difference between the control and hypothermia groups through one-way analysis of variance (ANOVA). Statistical analysis were all carried out via SPSS software (version 22.0, SPSS Inc., USA) based on Windows. Prior to ANOVA analysis, the data were tested for normal distribution, equal variances between treatments, and homogeneity. Percentage data were angularly transformed before being statistically evaluated. The difference was significant if the P value was less than 0.05 and extremely significant if the P value was less than 0.01.
The effect of low-temperature stress on the intestinal morphological structure of juvenile golden pompano is shown in Figure 1. The intestinal tissue of the control group had a normal morphological structure; with single-layer columnar epithelial cells arranged orderly, compact and slender lamina propria, and full goblet cells (Figure 1). Microvilli were arranged in order, evenly, and closely distributed, with a clear structure and clear and complete microvilli base bottom. The internal structure of the cells was normal without any damage (Figure 1A). At different times, low-temperature stress showed different degrees of damage to the intestinal tissue of juveniles. After 1 d of hypothermia stress, the muscle layer appeared damaged and swollen, and the folds were high but slightly swollen (Figure 1B). After 4 d of low-temperature stress, the folds were shed and deformed, and the intestinal mucosa was necrotic and had vacuolar structures (Figure 1C). After 7 d of low-temperature stress, the number of vacuoles in the intestinal tissue increased, the villous erosion was severe, and the muscle layer was severely damaged (Figure 1D). After 14 d of low-temperature stress, the intestinal villi were eroded, the muscle layer was swollen, and hyperemia and damage were severe. The folds were sparse, deformed, and severely damaged (Figure 1E).
Figure 1 Effects of low-temperature stress on intestinal tissue of juvenile golden pompano. (A) was the control group, (B) was the low-temperature stress for 1 d, (C) was the low-temperature stress for 4 d, (D) was the low-temperature stress for 7 d, and (E) was the low-temperature stress for 14 d. The magnification scale is x20.
As shown in Figure 2, the specific enzyme activities of golden pompano under low-temperature stress in this study showed an overall downward trend. Compared with the control group, the lipase activity at 1 d was significantly decreased (P<0.05), and at 4 d, 7 d, and 14 d, it was significantly lower than that in the control group (P<0.01). The lipase, amylase, and trypsin activities were significantly lower than the control group at 4 d (P <0.05) and extremely significantly lower than the control group at 7 d and 14 d (P <0.01).
Figure 2 Effects of low-temperature stress on intestinal lipase activity. (A),amylase activity (B) and trypsin activity (C) of juvenile golden pompano. ** indicate significant difference at (P <0.01), * indicate significant difference at P < 0.05.
The effect of low-temperature stress on the intestinal antioxidant enzymes of the golden pompano was shown in Figure 3. As shown in Figure 3, the extension of low-temperature stress, the SOD activity of the experimental group was significantly higher than that of the control group, and there was no significant difference between the experimental and control groups for 1 d (P<0.05). The CAT activity showed a decreasing trend. There was a significant difference between the control group and 1 d and 4 d (P < 0.05), and there was a significant difference between 7 d and 14 d (P< 0.01). MDA content and GSH-Px activity were increased, and there was a significant difference between them in 7 d and the control group (P < 0.05). There was a significant difference between them on 14 d (P < 0.01).
Figure 3 Effects of low-temperature stress on superoxide dismutase activity. (A), catalase activity (B), glutathione peroxidase activity (C), and malondialdehyde content (D) in the intestinal of juvenile golden pompano. ** indicate significant difference at (P <0.01), * indicate significant difference at P < 0.05.
By LC-MS mass spectrometry detection, a total of 742 species were obtained in positive ion mode, and 454 species were obtained in negative ion mode. During the detection process, QC samples were used for quality control to ensure the quality of the data. After performing the PCA analysis using the R language gmodels (v2.18.1), the PCA model was obtained after repeated verifications (Figure 4). As shown in Figure 4, under the positive and negative ion modes, the distribution of QC samples was relatively dense, and the system also had high stability, indicating that the data were good.
Figure 4 The PCA score plot of QC samples. (A) Positive ion mode. (B) Negative ion mode. A green spot in the PCA model represents a Cold sample, and A blue spot in the PCA model represents a Control. The red spot was a QC sample as a mixture of all samples.
The cross-validation of the OPLS-DA model showed that R2X was 0.496, R2Y was 0.829, and Q2Y was 0.328 (POS) (Figure 5A); R2X was 0.65, R2Y was 0.848, and Q2Y was 0.472 (NEG) (Figure 5B), and Q2 was greater than 0.5, indicating that the prediction ability of the model was good. The OPLS-DA model was established by a permutation test, and the order of the categorical variable Y was randomly changed to obtain the R2 and Q2 of the random model. All Q2 points were lower than the original black Q2 points from left to right (Figures 5C, D).
Figure 5 OPLS-DA model score diagram and OPLS-DA permutation test model of juvenile Trachinotus ovatus under low-temperature stress intestinal metabolites. (A) Positive ion mode, A blue spot in the OPLS-DA modes score diagram a Cold sample, and A red spot in the OPLS-DA modes score diagram a Control. (B) Negative ion mode. (C) Positive ion mode OPLS-DA permutation test model. (D) Negative ion mode.
The screening of differential metabolites was carried out based on the criteria of VIP of the mode (P <0.05) in the OPLS-DA model. The results of drawing volcano plots are shown in Figure 6. In positive ion mode, 3 differential metabolites were significantly up-regulated, and 14 differential metabolites were significantly down-regulated. In negative ion mode, 7 differential metabolites were significantly up-regulated, and 4 differential metabolites were significantly down-regulated. In analysis and identification, the main differential metabolites are shown in Table 1. The types of lipid metabolites in the table were relatively rich, and the difference was obvious, which indicates that lipid metabolism is the key factor for golden pompano to cope with low-temperature stress.
Figure 6 Volcano plot of juvenile Trachinotus ovatus under low-temperature intestinal metabolites. (A) Positive ion mode, A red spot represents a predominance of down-regulation metabolite. A blue spot represents a predominance of up-regulation metabolite. (B) Negative ion mode.
Table 1 Differential metabolites in the intestine of juvenile Trachinotus ovatus under low-temperature stress.
The significant metabolites were enriched by KEGG, and the metabolic pathways were analyzed. A total of 172 metabolic pathways were enriched. A total of 15 pathways with the most significant differences were selected for classification. The top 15 with the largest differences were firstly enriched and classified into 5 A-class pathways of metabolism, organic systems, cellular processes, gene information processes, and human diseases. As shown in Table 2, there were significant differences in the biosynthesis of unsaturated fatty acids, pyrimidine metabolism, β-alanine metabolism, fatty acid biosynthesis, and linoleic acid metabolism (P<0.05). Lipid metabolism was the most important metabolic pathway of low-temperature stress on the juvenile.
The results of the significantly differential metabolite analysis of the metabolic pathways with significant differences are shown in Table 3. Compared with the control group, adrenic acid, 8Z, 11Z, 14Z-Eicosatrienoic acid, and stearic acid synthesizing unsaturated fatty acids were up-regulated under low-temperature stress. Stearic acid in fatty acid biosynthesis and 8Z, 11Z, and 14Z-eicosatrienoic acids in linoleic acid metabolism were also up-regulated. Compared with the control group, uridine and uracil were down-regulated in pyrimidine metabolism, while cytidine-5’-monophosphate was up-regulated. In β-alanine metabolism, both uracil and spermidine were down-regulated.
Table 3 Pathways involved by differential metabolites of juvenile Trachinotus ovatus under low-temperature stress.
Stress damages the intestinal epithelial cells of rainbow trout (Oncorhynchus mykiss), increases epithelial permeability, and damages gastrointestinal barrier function (Olsen et al., 2005). In the study, there was no significant change in the intestinal structure of juvenile T. ovatus under low-temperature stress for 1 d. With the extension of low-temperature stress time, the number of vacuoles in intestinal tissue increased at 7 d, the villus erosion was serious, and the muscle layer was seriously damaged. At 14 d, the intestinal villi were eroded, and the muscle layer was swollen and congested. There was significant damage. The folds were sparse, deformed, and seriously damaged. Low-temperature stress might change the stability of the intestinal mucus layer of juvenile T. ovatus, destroying the mucosal mechanical barrier of intestinal tissue (Xing et al., 2019). The results of this study were similar to those of hybrid yellow catfish (P. fulvidraco♀×P. vachelli) (Cheng et al., 2022) and rainbow trout (Olsen et al., 2005). Therefore, the results of this study showed that the intestinal morphology and structure of juvenile T. ovatus were damaged by low-temperature stress, resulting in the decline of intestinal digestion.
Digestive physiology studies of fish can help us better understand the way in which they adapt to dietary changes since digestion provides nutrients that are required for a variety of biological functions (Solovyev et al., 2018). Digestive enzymes present in various regions of the digestive tract play a key role in food utilization (Solovyev et al., 2018). Poikilothermic animals, including fish, are strongly influenced by temperature, which is one of the most critical environmental factors (Solovyev et al., 2021). Physiological responses to temperature changes have been examined from a variety of perspectives (Yúfera et al., 2019; Volkoff and Rønnestad, 2020). Particularly relevant is how ingested nutrients are digested before their incorporation into growing tissues. In spite of a large research effort, the effect of temperature on fish digestion is far from being well understood. Temperature can affect different digestive functions, including feed capture and nutrient absorption, in different ways affecting the growth and development speed of the fish. Digestive enzyme activity was an important indicator for identifying fish’s healthy growth (Amin et al., 2016; Yúfera et al., 2019). Lower temperatures result in fish losing appetite and slowing growth (Jobling, 1994; Koskela et al., 1997). Each species of fish has its suitable temperature range, and temperature changes resulting in slowing down or even stopping growth (Das et al., 2021). The temperature adaptation of enzymes is genetically determined and may involve phenotypic changes. These adaptations include differences in structure, an affinity for substrates, and reactions in the rate at which enzymatic activity is produced, but with optimal efficiency at different temperatures (Gelman et al., 2008). With the prolongation of low-temperature time, the activity of digestive enzymes in juvenile golden pompano showed a significant downward trend in this study (P<0.05). The metabolic rate of the digestive system was also weakened, and the basal secretion of digestive enzymes was reduced. When the low temperature exceeds its tolerance, the secretion of digestive enzymes may be greatly reduced due to the impact of the entire metabolic process, leading to a rapid decline in enzyme activity. However, a fish’s digestive enzymes are also influenced by the habitat and climate in which it resides. Genetic factors determine how enzymes adapt to temperature, which can involve phenotypic changes. Isozymes catalyze the same reaction but at different temperatures with optimal efficiency due to differences in structure, affinity to substrates, and activation energy (Gelman et al., 2008; Volkoff and Rønnestad, 2020). At low and moderate temperatures, digestive enzymes from fish that are adapted to cold environments, such as the Atlantic cod (Gadus morhua) (Stefansson et al., 2017) and Antarctic icefish (Pleuragramma antarcticum) (Krogdahl et al., 2011), have higher catalytic efficiency than enzymes from mammals or fish that are adapted to warmer environments. In this study, the food intake of juvenile golden pompano decreased with the decrease in ambient temperature, and the secretion activity of fish digestive enzymes was related to the degree of satiety (Hasanpour et al., 2021). The effects of water temperature on fish digestive enzyme activities are species-specific. Two methods have traditionally been used to assess digestive enzyme activity. A study conducted with enzyme extracts for in vitro enzyme characterization shows, on the one hand, that activity increases as temperature increases, usually up to values that exceed those associated with the enzyme’s natural environment and beyond lethal levels (Fernández et al., 2001; Solovyev et al., 2018). Several other studies have also investigated the digestive enzyme activity of live fish at various temperatures (Bowyer et al., 2013; Hani et al., 2018; Mazumder et al., 2018; Miegel et al., 2010; Sharma et al., 2017). For example, in some subtropical/tropical species: 18–28°C in catla (Catla catla) (Sharma et al., 2017), 10–35°C in walking catfish (Clarias batrachus) (Ahmad et al., 2014), 4–20°C in three spine stickleback (Gasterosteus aculeatus) (Hani et al., 2018), and 8–24°C in seabass (Dicentrarchus labrax) (Pereira et al., 2018) .The studies, however, report contradictory responses among the different investigated species. The efficiency of digestion depends on the relation between the activity of the enzymes and the transit time of the gut, which is not a simple fact but rather a long process that happens every day over a long period of time (Solovyev et al., 2018). Digestion may also be affected by other species-specific digestive characteristics. Despite a large amount of research, how temperature affects fish digestion still needs to be further clarified. Temperature changes may affect digestive function differently, from feed capture to nutrient absorption. Our results indicate that changes in water temperature alter digestive enzyme activities hence the nutrients absorption was affected.
In healthy organisms, free radical production and elimination are in dynamic balance. However, in adversity, stress will trigger reactions from mitochondria, microsomes, and the cytoplasm’s enzyme systems and non-enzyme systems, resulting in excess reactive oxygen species (ROS) and oxygen free radicals, breaking the reactive oxygen metabolism balance (Ren et al., 2021; Wade et al., 2017). Cellular damage caused by oxidative stress can be determined by the level of lipid peroxidation in cells and tissues (Mensah et al., 2012). Enzymatic and nonenzymatic antioxidants scavenge ROS and free radicals as part of the primary defense response against oxidative stress and cell damage (El-Gendy et al., 2010). Glutathione S-transferase (GST), glutathione reductase (GR), catalase (CAT), glutathione peroxidase (GPx), and SOD, along with reduced glutathione (GSH), are the main antioxidative enzymes that detoxify ROS in all organisms (Lesser, 2006; Zheng et al., 2019; Ren et al., 2021). The antioxidant capacity of aquatic organisms has a high correlation with water temperature (Gieseg et al., 2000; Ren et al., 2021). Therefore, low-temperature stress greatly impacted the antioxidant defense system of fish, which may affect the enzyme activities and content related to SOD, CAT, GSH-Px, and MDA. These indicators were used to evaluate the antioxidant capacity of fish. SOD activity reflected the ability of the body to scavenge oxygen free radicals, while CAT was the main enzyme that decomposes H2O2 into H2O in the body (Hoseinifar et al., 2020). GSH-Px could reduce lipid peroxides to alcohols, reducing the chance of lipid oxidation and the damage of MDA to cells (Henry et al., 2018). SOD, CAT, and GSH-Px cooperated to complete the antioxidant process. MDA was the final product of lipid oxidation, and the level of MDA in the body showed the degree of lipid oxidation damage by ROS and free radicals (Hoseinifar et al., 2020).
A small amount of ROS is necessary for the normal physiological activities of the organism (Zhang et al., 2007). When the organism produces a large amount of ROS under environmental stress, which exceeds the elimination ability of the organism, the functions of DNA, proteins, and lipids will be damaged (Morel and Barouki, 1999). The antioxidant system of fish was susceptible to temperature. For example, long-term temperature stress in largemouth bass (Micropterus salmoides) could cause stress damage and reduce immune levels (Lu et al., 2020). In low-temperature stress, cobia (Rachycentron canadum) juveniles have reduced antioxidant capacity and accumulated a large amount of MDA (Cai R-J et al., 2021). The activities of antioxidant enzymes in the liver of juvenile orange-spotted spinefoot (Siganus guttatus) were significantly decreased after low-temperature stress (14°C) (Song et al., 2015). In the previous study, low-temperature stress led to increased MDA concentration in fish, which caused damage to fish (Pan et al., 2016).
In this study, the intestinal SOD activity increased significantly after 4 d of low-temperature stress, indicating that after 4 d of low-temperature stress, the body was in a state of oxidative stress, and the intestinal tissue was damaged. The antioxidant defense system was activated to eliminate excessive ROS. After 14 d of low-temperature stress, the SOD and GSH-Px activities of intestinal tissues showed an upward trend. There was a significant difference between them and the control group (P < 0.01), which indicated that low-temperature stress caused excessive production of ROS in golden pompano and the increase of ROS in body tissues can induce the increase of antioxidant enzyme activity (Li et al., 2003). The activity of CAT showed a significant downward trend, which was similar to the study of cobia (Rachycentron canadum) (Wang et al., 2020). The intestinal tissue produced excessive ROS during this period, which inhibited the activity of CAT. MDA is the final product of lipid peroxidation in tissues and has strong cytotoxicity (Peng et al., 2009). In this study, the content of MDA increased significantly with the prolongation of low-temperature stress, which indicated that low-temperature stress had an impact on the antioxidant capacity of golden pompano. The antioxidant capacity of the intestine decreased under low-temperature stress and was vulnerable to free radicals, which indicated that the antioxidant defense ability of the juvenile golden pompano under 14 d low-temperature stress was damaged, the body could not remove excess ROS in time, and the increase of ROS concentration in the body deepened the degree of lipid peroxidation.
Aquatic organisms’ respiration and energy metabolism can be directly affected by temperature. As a result, the production of neurohormones and digestion enzymes decreases, and enzyme activity and metabolic modes relevant to energy metabolism are altered (Ren et al., 2021; Anestis et al., 2008). In this study, the expression levels of 8Z, 11Z, and 14Z-eicosatrienoic acids were significantly up-regulated under low-temperature stress, which was presumed to be the protective mechanism of the golden pompano against low-temperature stress. The synthesis of unsaturated fatty acids was to stabilize the fluidity of cell membranes. Although this method can improve cell membranes’ fluidity, it also makes the body more prone to oxidative stress. In the n-3 and n-6 oxidation pathways, two kinds of peroxides, malondialdehyde and nonene, will be produced, which will further aggravate the lipid peroxidation of the body and eventually cause lipid damage.
In normal organisms, the content of free arachidonic acid was very low, but when the cell membrane was stimulated, it would be released from the phospholipid pool of the cell membrane, and arachidonic acid was produced by the metabolism of the organism (Rivero-Ramírez et al., 2020). Arachidonic acid is related to stress immunity (Qi et al., 2022). As a result of low-temperature stress, a large amount of linoleic acid was decomposed into 8Z, 11Z, and 14Z -eicosatrienoic acid in the linoleic acid metabolic pathway (Ko00591). The 8Z, 11Z, and 14Z -eicosatrienoic acid was further synthesized into arachidonic acid, which improved one’s immunity. Prostaglandins synthesized from arachidonic acid as a substrate have the effect of vasoconstriction and dilation. When the expression levels of PGB1 and PGB3 were decreased, the blood vessels were dilated, and when the expressions of PGB2 and PGB4 were decreased, the blood vessels were constricted (Zhou et al., 2021). In this study, when the metabolic pathway of arachidonic acid was analyzed, the expression of PGB2 was reduced, but the exact reason and its implications are not immediately known, which needs further studies. The immune level of fish under low-temperature stress will be sharply reduced and slowly recovered, even higher than the original level (Kammer et al., 2011). This phenomenon has not been found in this study, which may be related to the difference in experimental settings and fish species.
As a natural substance in cells, pyrimidines have biological activities, such as antibacterial, antiviral, and antibiotics, in addition to being a constituent of DNA and RNA (Kumar et al., 2019). In this study, the expression of uracil and uridine was downregulated, which may be used for RNA synthesis. In the analysis of differential metabolites, cytidine-5’-monophosphate of the same pathway showed an upward trend, and purine as a complement to pyrimidine did not change significantly. Under low-temperature stress, the protein synthesis of golden pompano larvae may be reduced. In the pyrimidine metabolic pathway (ko00240), the reduced uridine and uracil may be used for synthesis with other substances, such as β- Synthesis of alanine. In the present study, the levels of uracil and spermidine in the β-alanine metabolic pathway (ko00410) were significantly down-regulated under low-temperature stress, and these two substances play an important role in the pathway as β-alanine. The synthetic substrate of pyrimidine mainly came from the decomposition of pyrimidine, but the content of β-alanine as the product had no obvious change. The study showed that β-alanine had the effects of antioxidants, neurotransmitters, and enhancement of exercise capacity (Wang L et al., 2021), which indicated that there was no obvious change in the movement ability of juvenile golden pompano when faced with low-temperature stress. While synthesizing unsaturated fatty acids to increase cell membrane fluidity, peroxides are produced to cause damage to cells. Therefore, β-alanine, which is synthesized from uracil and spermidine, was used more in antioxidant aspects to protect the organism from oxidative damage. Synthetic β-alanine also acted as a neurotransmitter to deactivate the lipid synthesis pathway due to enhanced expression of the pathway for fatty acid biosynthesis. Spermidine is a natural polyamine that can prevent cell damage by inducing immune cells to participate in stabilizing the internal environment of cells, ensuring the stability of DNA, and has many biological processes such as antioxidant and anti-inflammatory (Madeo et al., 2020). In the study, spermidine levels were significantly lower. On the one hand, it may be used to synthesize β-alanine. On the other hand, it was used to scavenge peroxides generated due to the synthesis of unsaturated fatty acids to protect the body from oxidative damage.
Fish secretory tissues immediately change their function and biochemical properties when their body temperature changes. The reaction of the intestinal tract of juvenile golden pompano to sudden changes in the environment’s temperature was studied in the current study. In this study a low-temperature stress model for juvenile golden pompano was developed. The current results showed that low temperature could cause changes in antioxidant and digestive enzyme activities in golden pompano, and that low temperature causes damage to intestinal tissue, and its function could be disordered. Findings like these reveal how low-temperature stress affects fish in a specific way, which may help gain a deeper understanding of those effects and find ways to help fish cope with them in the future.
The raw data supporting the conclusions of this article will be made available by the authors, without undue reservation.
The studies involving animals were reviewed and approved by the Guangdong Ocean University Research Council’s guidelines for the care and use of laboratory animals (approval number: GDOU-LAE-2022-011).
R-XL and EA were responsible for project administration, data collection, formal analysis, processing, and writing of the original draft and reviewing, and editing. YL and J-HJ were involved in data curation. R-TX provided experimental guidance. J-SH revised the experimental design, supervised the experiment and reviewed the article, and provided funding. All authors contributed to the article and approved the submitted version.
This study was supported by grants from the Zhanjiang Marine youth talent innovation project in 2021 (2021E05026); Key scientific research platforms and projects of ordinary universities in Guangdong Province in 2022 (2022KCXTD013); Southern Marine Science and Engineering Guangdong Laboratory (Zhanjiang) (ZJW-2019-06).
All funders of this study are dully acknowledged.
Author R-TX was employed by Guangdong Evergreen Feed Industry Co.Ltd, Zhanjiang, 524003, China.
The remaining authors declare that the research was conducted in the absence of any commercial or financial relationships that could be construed as a potential conflict of interest.
All claims expressed in this article are solely those of the authors and do not necessarily represent those of their affiliated organizations, or those of the publisher, the editors and the reviewers. Any product that may be evaluated in this article, or claim that may be made by its manufacturer, is not guaranteed or endorsed by the publisher.
Abram Q., Dixon B., Katzenback B. (2017). Impacts of low temperature on the teleost immune system. Biology 6 (4), 39. doi: 10.3390/biology6040039
Ahmad T., Singh S. P., Khangembam B. K., Sharma J. G., Chakrabarti R. (2014). Food consumption and digestive enzyme activity of Clarias batrachus exposed to various temperatures. Aquacult Nutr. 20 (3), 265–272. doi: 10.1111/anu.12072
Amenyogbe E., Luo J., Fu W. J., Abarike E. D., Wang Z.-L., Huang J.-S., et al. (2022c). Effects of autochthonous strains mixture on gut microbiota and metabolic profile in cobia (Rachycentron canadum). Sci. Rep. 12, 17410. doi: 10.1038/s41598-022-19663-x
Amenyogbe E., Yang E.-J., Xie R.-T., Huang J.-S., Chen G. (2022a). Influences of indigenous isolates Pantoea agglomerans RCS2 on growth, proximate analysis, haematological parameters, digestive enzyme activities, serum biochemical parameters, antioxidants activities, intestinal morphology, disease resistance, and molecular immune response in juvenile's cobia fish (Rachycentron canadum). Aquaculture 737942, 0044–8486. doi: 10.1016/j.aquaculture.2022.737942
Amenyogbe E., Zhang J.-D., Huang J.-S., Chen G. (2022b). The efficiency of indigenous isolates Bacillus sp. RCS1 and Bacillus cereus RCS3 on growth performance, blood biochemical indices and resistance against Vibrio harveyi in cobia fish (Rachycentron canadum) juveniles. Aquacult Rep. 25, 2352–5134. doi: 10.1016/j.aqrep.2022.101241
Amin M. N., Carter C. G., Katersky Barnes R. S., Adams L. R. (2016). Protein and energy nutrition of brook trout (Salvelinus fontinalis) at optimal and elevated temperatures. Aquacult Nutr. 22 (3), 527–540. doi: 10.1111/anu.12274
Anestis A., Pörtner H. O., Lazou A., Michaelidis B. (2008). Metabolic and molecular stress responses of sublittoral bearded horse mussel modiolus barbatus to warming sea water: implications for vertical zonation. J. Exp. Biol. 211, 2889–2898. doi: 10.1242/jeb.016782
Bates J. M., Akerlund J., Mittge E., Guillemin K. (2007). Intestinal alkaline phosphatase detoxifies lipopolysaccharide and prevents inflammation in zebrafish in response to the gut microbiota. Cell Host Microbe 2, 371–382. doi: 10.1016/j.chom.2007.10.010
Bowden T. J. (2008). Modulation of the immune system of fish by their environment. Fish Shellfish Immunol. 25, 373–383. doi: 10.1016/j.fsi.2008.03.017
Bowyer J. N., Booth M. A., Qin J. G., D’Antignana T., Thomson M. J. S., Stone D. A. J. (2013). Temperature and dissolved oxygen influence growth and digestive enzyme activities of yellowtail kingfishSeriola lalandi(Valencienne). Aquaculture Res. 45 (12), 2010–2020. doi: 10.1111/are.12146
Butt R. L., Volkoff H. (2019). Gut microbiota and energy homeostasis in fish. front. Endocrinol. 10. doi: 10.3389/fendo.2019.00009
Cai R.-J., Zhang J., Huang J.-S., Chen G., Zhang J.-D., Pan C.-H., et al. (2021). Effects of low temperature stress on physiology and biochemistry of lipid metabolism of juvenile cobia, Rachycentron canadum. J. Guangdong Ocean Univ. 41(3), 123–130. doi: 10.3969/j.issn.1673-9159.2021.03.016
Cai R., Zhang J., Huang J.-S., Shi G., Pan C., Xie R.-T., et al. (2021). Effects of low temperature stress on the expression of genes related to lipid metabolism of juvenile cobia, Rachycentron canadum. Haiyang Xuebao 43 (11), 116(11)n. doi: 10.12284/hyxb2021122
Cheng J.-H., Li Y., Shen M.-H., Yang Z.-R., Zhang W.-J., Zhang K., et al. (2022). Effects of hypoxia stress and recovery on intestinal tissue of hybrid yellow catfish “Huangyou-1” (Pelteobagrus vachelli eltePelteobagrus fulvidraco el [J/OL]. Acta Hydrobiologica Sin. 46(11), 1598–1608. doi: 10.7541/2023.2021.0342
Das S. K., Xiang T. W., Noor N. M., De M., Mazumder S. K., Goutham-Bharathi M. P. (2021). Temperature physiology in grouper (Epinephelinae: Serranidae) aquaculture: A brief review. Aquacult Rep. 20, 100682. doi: 10.1016/j.aqrep.2021.100682
Di Maiuta N., Schwarzentruber P., Schenker M., Schoelkopf J. (2013). Microbial population dynamics in the faeces of wood-eating loricariid catfishes. Lett. Appl. Microbiol. 56, 401–407. doi: 10.1111/lam.12061
Egerton S., Culloty S., Whooley J., Stanton C., Ross R. P. (2018). The gut microbiota of marine fish. Front. Microbiol. 9. doi: 10.3389/fmicb.2018.00873
El-Gendy K. S., Aly N. M., Mahmoud F. H., Kenawy A., El-Sebae A. K. H. (2010). The role of vitamin c as antioxidant in protection of oxidative stress induced by imidacloprid. Food Chem. Toxicol. 48, 215–221. doi: 10.1016/j.fct.2009.10.003
Fernández I., Moyano F. J., Dıaz M., Martınez T. (2001). Characterization of a-amylase activity in five species of Mediterranean sparid fishes (Sparidae, teleostei). J. Exp. Mar. Biol. Ecol. 262, 1–12.
Fu W., Amenyogbe E., Luo J., Yang E., Huang J.-S., Chen Y., et al. (2022b). Influences of ferulic acid on intestinal digestive and antioxidant enzymes, immune, antioxidant gene and tight junction protein expression and microbiota in hybrid grouper (Epinephelus fuscoguttatus♀× Epinephelus polyphekadion♂). Aquacult Rep. 27, 101348, 2352–5134. doi: 10.1016/j.aqrep.2022.101348
Fu W., Amenyogbe E., Yang E., Luo J., Huang J.-S., Xie R.-T., et al. (2022a). Effects of dietary supplementation of ferulic acid on growth performance, antioxidant ability, non-specific immunity, hepatic morphology and genes expression related to growth and immunity in juvenile hybrid grouper (Epinephelus fuscoguttatus♀ × epinephelus polyphekadion♂). Aquaculture 552, 737988, 0044–8486. doi: 10.1016/j.aquaculture.2022.737988
Gelman A., Kuz’mina V., Drabkin V., Glatman L., Cyrino J., Bureau D., et al. (2008). Temperature adaptation of digestive enzymes in fish. In: Cyrino JEP, Bureau DP, Kapoor BG 1st Edition, Feeding and digestive functions in fished. Science Publishers, Enfield NH, pp. 155–225. eBook ISBN9780429061578. doi: 10.1201/b10749
Gieseg S. P., Cuddihy S., Hill J. V., Davison W. (2000). A comparison of plasma vitamin c and e levels in two Antarctic and two temperate water fish species. Comp. Biochem. Physiol. Part B: Biochem. Mol. Biol. 125 (3), 371–378. doi: 10.1016/s0305-0491(99)00186-8
Goos H. T., Consten D. (2002). Stress adaptation, cortisol and pubertal development in the male common carp, cyprinus carpio. Mol. Cell. Endocrinol. 197 (1-2), 105–116. doi: 10.1016/S0303-7207(02)00284-8
Hamer H. M., Jonkers D. M. A. E., Bast A., Vanhoutvin S. A. L. W., Fischer M. A. J. G., Kodde A., et al. (2009). Butyrate modulates oxidative stress in the colonic mucosa of healthy humans. Clin. Nutr. 28, 88–93. doi: 10.1016/j.clnu.2008.11.002
Hani Y. M. I., Marchand A., Turies C., Kerambrun E., Palluel O., Bado-Nilles A., et al. (2018). Digestive enzymes and gut morphometric parameters of threespine stickleback (Gasterosteus aculeatus): Influence of body size and temperature. PloS One 13 (4), e0194932. doi: 10.1371/journal.pone.0194932
Hao R., Du X., Yang C., Deng Y., Zheng Z., Wang Q. (2019). Integrated application of transcriptomics and metabolomics provides insights into unsynchronized growth in pearl oyster pinctada fucata martensii. Sci. Total Environ. 666, 46–56. doi: 10.1016/j.scitotenv.2019.02.221
Hasanpour S., Oujifard A., Torfi Mozanzadeh M., Safari O. (2021). Compensatory growth, antioxidant capacity and digestive enzyme activities of sobaity (Sparidentex hasta) and yellowfin seabreams (Acanthopagrus latus) subjected to ration restriction. Aquacult Nutr. 27 (6), 2448–2458. doi: 10.1111/anu.13376
Hassenrück C., Reinwald H., Kunzmann A., Tiedemann I., Gärdes A. (2020). Effects of thermal stress on the gut microbiome of juvenile milkfish (Chanos chanos). Microorganisms 9 (1), 5. doi: 10.3390/microorganisms9010005
Henry M. A., Gai F., Enes P., Peréz-Jiménez A., Gasco L. (2018). Effect of partial dietary replacement of fishmeal by yellow mealworm (Tenebrio molitor) larvae meal on the innate immune response and intestinal antioxidant enzymes of rainbow trout (Oncorhynchus mykiss). Fish Shellfish Immunol. 83, 308–313. doi: 10.1016/j.fsi.2018.09.040
Hoseinifar S. H., Yousefi S., Van Doan H., Ashouri G., Gioacchini G., Maradonna F., et al. (2020). ). oxidative stress and antioxidant defense in fish: The implications of probiotic, prebiotic, and synbiotics. Rev. Fisheries Sci. Aquacult 1–20, 2330–8249. doi: 10.1080/23308249.2020.1795616
Hu W.-T., Bai J.-Y., Fei X., Hu Y.-N., Wu M.-X., Zhang Y.-Z., et al. (2020). Metabolomic responses of Nile tilapia (Oreochromis niloticus) maintained under different temperatures and challenged with streptococcus agalactiae. Aquaculture 524, 735209. doi: 10.1016/j.aquaculture.2020.735209
Huang J.-S., Amenyogbe E., Chen G., Wang W.-Z. (2020). Biochemical composition and activities of digestive and antioxidant enzymes during the egg and yolk-sac larval development of the cobia (Rachycentron canadum). Aquacult Res. 00, 1–14. doi: 10.1111/are.15017
IPCC (Intergovernmental Panel on Climate Change) (2012). Managing the risks of extreme events and disasters to advance climate change adaptation (Cambridge, UK: Cambridge University Press).
Islam M. J., Kunzmann A., Slater M. J. (2021). Extreme winter cold-induced osmoregulatory, metabolic, and physiological responses in European seabass (Dicentrarchus labrax) acclimatized at different salinities. Science of The Total Environment 771, 145202. doi: 10.1016/j.scitotenv.2021.145202
Kammer A. R., Orczewska J. I., O'brien K. M. (2011). Oxidative stress is transient and tissue specific during cold acclimation of threespine stickleback. J. Exp. Biol. 214 (8), 1248–1256. doi: 10.1242/jeb.053207
Koskela J., Pirhonen J., Jobling M. (1997). Effect of low temperature on feed intake, growth rate and body composition of juvenile Baltic salmon. Aquacult Int. 5, 479–488. doi: 10.1023/A:1018397014684
Krogdahl Å., Sundby A., Bakke A. M. (2011). “Gut secretion and digestion,” in Encyclopedia of fish physiology. Ed. Farrell A. P. (San Diego: Academic Press), 1301–1310.
Kumar S., Deep A., Narasimhan B. (2019). A review on synthesis, anticancer and antiviral potentials of pyrimidine derivatives. Curr. Bioactive Compounds 15 (3), 289–303. doi: 10.2174/1573407214666180124160405
Lall S. J. P. (2020). Intestinal alkaline phosphatase in the gastrointestinal tract of fish: biology, ontogeny, and environmental and nutritional modulation. Rev. Aquacult 12 (2), 555–581. doi: 10.1111/raq.12340
Lesser M. P. (2006). Oxidative stress in marine environments: biochemistry and physiological ecology. Annu. Rev. Physiol. 68, 253–278. doi: 10.1146/annurev.physiol.68.040104.110001
Li X.-Y., Liu Y.-D., Song L.-I. R., Qiao Z.-I. G. (2003). Response of antioxidant systems in the hepatocytes of common carp (Cyprinus carpio l) to the toxicity of microcystin-LR. Acta Hydrobiologica Sin. 27 (5), 472–475. doi: 10.1016/S0041-0101(03)00104-1
Lin S.-M., Zhou X.-M., Zhou Y.-L., Kuang W.-M., Chen Y.-J., Luo L., et al. (2020). Intestinal morphology, immunity and microbiota response to dietary fibers in largemouth bass, Micropterus salmoide. Fish Shellfish Immunol. 103, 135–142. doi: 10.1016/j.fsi.2020.04.070
Liu F., Li S., Yu Y., Sun M., Xiang J., Li F. (2020). Effects of ammonia stress on the hemocytes of the pacific white shrimp Litopenaeus vannamei. Chemosphere 239, 124759. doi: 10.1016/j.chemosphere.2019.124759
Liu G., Wang H., Lv Z., Tang X., Yu M. (2022). A comprehensive metabolomic and lipidomic analysis reveals the effect of temperature on flounder (Paralichthys olivaceus). J. Thermal Biol. 104, 103203, 0306–4565. doi: 10.1016/j.jtherbio.2022.103203
Llewellyn M. S., Boutin S., Hoseinifar S. H., Derome N. (2014). Teleost microbiomes: The state of the art in their characterization, manipulation and importance in aquaculture and fisheries. Front. Microbiol. 5. doi: 10.3389/fmicb.2014.00207
Lu J., Zhnag J.-J., Wang P.-P., Zhou G.-Q., Qing H. (2020). Effect of acute temperature stress on survival rate and biochemical indices in the liver of micropterus salmoides”Youlu no. 3”. Freshw. Fisheries 50 (2), 87–93. doi: 10.13721/j.cnki.dsyy.2020.02.013
Madeo F., Hofer S. J., Pendl T., Bauer M. A., Eisenberg T., Carmona-Gutierrez D., et al. (2020). Nutritional aspects of spermidine. Annu. Rev. Nutr. 40 (1), 135–159. doi: 10.1146/annurev-nutr-120419-015419
Mazumder S. K., Das S. K., Rahim S. M., Ghaffar M. A. (2018). Temperature and diet effect on the pepsin enzyme activities, digestive somatic index and relative gut length of malabar blood snapper ( lutjanus malabaricus Bloch & schneide). Aquaculture Rep. 9, 1–9. doi: 10.1016/j.aqrep.2017.11.003
Mensah P. K., Palmer C. G., Muller W. J. (2012). Lipid peroxidation in the freshwater shrimp caridina nilotica as a biomarker of roundup r herbicide pollution of freshwater systems in south Africa. Water Sci. Technol. 65, 1660–1666. doi: 10.2166/wst.2012.060
Miegel R. P., Pain S. J., van Wettere W. H. E. J., Howarth G. S., Stone D. A. J. (2010). Effect of water temperature on gut transit time, digestive enzyme activity and nutrient digestibility in yellowtail kingfish (Seriola lalandi). Aquaculture 308 (3-4), 145–151. doi: 10.1016/j.aquaculture.2010.07.036
Morel Y., Barouki R. (1999). Repression of gene expression by oxidative stress. Biochem. J. 342 (3), 481–496. doi: 10.1042/bj3420481
Nadermann N., Seward R. K., Volkoff H. (2019). Effects of potential climate change -induced environmental modifications on food intake and the expression of appetite regulators in goldfish. Comp. Biochem. Physiol. A Mol. Integr. Physiol. 235, 138–147. doi: 10.1016/j.cbpa.2019.06.001
Nguyen M. V., Espe M., Conceição L. E. C., Le H. M., Yúfera M., Engrola S. A. D., et al. (2019). The role of dietary methionine concentrations on growth, metabolism and n-retention in cobia (Rachycentron canadum) at elevated water temperatures. Aquac Nutr. 25 (2), 495–507. doi: 10.1111/anu.12875
Olsen R. E., Sundell K., Mayhew T. M., Myklebust R., Ringø E. (2005). Acute stress alters intestinal function of rainbow trout, Oncorhynchus mykiss (Walbaum). Aquaculture 250 (1-2), 480–495. doi: 10.1016/j.aquaculture.2005.03.014
Pan G., Liu B., Zhou W. (2016). The effects of cold stress on the antioxidant defense and immune parameters of juvenile Epinephelus moara. J. Shanghai Ocean Univ. 25 (1), 78–85. doi: 1674-5566(2016)01-0078-08
Peng S., Chen L., Qin J. G., Hou J., Yu N., Long Z., et al. (2009). Effects of dietary vitamin e supplementation on growth performance, lipid peroxidation and tissue fatty acid composition of black sea bream (Acanthopagrus schlegeli) fed oxidized fish oil. Aquacult Nutr. 15 (3), 329–337. doi: 10.1111/j.1365-2095.2009.00657.x
Pereira L. F., Peixoto M. J., Carvalho P., Sansuwan K., Santos G. A., Gonçalves J. F. M., et al. (2018). Cross-effects of dietary probiotic supplementation and rearing temperature on growth performance, digestive enzyme activities, cumulative mortality and innate immune response in seabass (Dicentrarchus labrax ). Aquacult Nutr. 24 (1), 453–460. doi: 10.1111/anu.12578
Perry W. B., Lindsay E., Payne C. J., Brodie C., Kazlauskaite R. (2020). The role of the gut microbiome in sustainable teleost aquaculture. Proc. R. Soc B Biol. Sci. 287, 20200184. doi: 10.1098/rspb.2020.0184
Pirhalla D. E., Sheridan S. C., Ransibrahmanakul V., Lee C. C. (2015). Assessing cold-snap and mortality events in south Florida coastal ecosystems: Development of a biological cold stress index using satellite SST and weather pattern forcing. Estuar. Coast. 38, 2310–2322. doi: 10.1007/s12237-014-9918-y
Qi H., Liu Y., Jian F., Xing X., Wang J., Li C. (2022). Effects of dietary arachidonic acid (ARA) on immunity, growth and fatty acids of apostichopus japonicus. Fish Shellfish Immunol. 127(901–909). doi: 10.1016/j.fsi.2022.07.037
Ren X., Wang Q., Shao H., Xu Y., Liu P., Li J. (2021). Effects of low temperature on shrimp and crab physiology, behavior, and growth: A review. Front. Mar. Sci. 8. doi: 10.3389/fmars.2021.746177
Rivero-Ramírez F., Torrecillas S., Betancor M. B., Izquierdo M. S., Caballero M. J., Montero D. (2020). Effects of dietary arachidonic acid in European sea bass (Dicentrarchus labrax) distal intestine lipid classes and gut health. Fish Physiol. Biochem. 46 (2), 681–697. doi: 10.1007/s10695-019-00744-0
Roeselers G., Mittge E. K., Stephens W. Z., Parichy D. M., Cavanaugh C. M., Guillemin K., et al. (2011). Evidence for a core gut microbiota in the zebrafish. ISME J. 5, 1595–1608. doi: 10.1038/ismej.2011.38
Sæle Ø., Rød K. E. L., Vanessa H. Q., Shengrong L., Farber S. (2018). A novel system to quantify intestinal lipid digestion and transport. Biochim. Biophys. Acta (BBA)-Molecular Cell Biol. Lipids 1863 (9), 948–957. doi: 10.1016/j.bbalip.2018.05.006
Schleger I. C., Pereira D. M. C., Resende A. C., Romão S., Herrerias T., Neundorf A. K. A., et al. (2021). Cold and warm waters: energy metabolism and antioxidant defenses of the freshwater fish astyanax lacustris (Characiformes: Characidae) under thermal stress. Journal of Comparative Physiology B. 192, 77–94. doi: 10.1007/s00360-021-01409-2
Shafland P. L., Pestrak J. M. (1982). Lower lethal temperatures for fourteen non-native fishes in Florida. Environ. Biol. Fish 7, 149–156. doi: 10.1007/BF00001785
Sharma J., Singh S. P., Chakrabarti R. (2017). Effect of temperature on digestive physiology, immune-modulatory parameters, and expression level of hsp and LDH genes in Catla catla (Hamilton 1822). Aquaculture 479, 134–141. doi: 10.1016/j.aquaculture.2017.05.031
Smith C. A., Want E. J., O’Maille G., Abagyan R., Siuzdak G. (2006). XCMS: processing mass spectrometry data for metabolite profiling using nonlinear peak alignment, matching, and identification. Anal. Chem. 78 (3), 779–787. doi: 10.1021/ac05143
Solovyev M. M., Izvekova G. I., Kashinskaya E. N., Gisbert E. (2018). Dependence of pH values in the digestive tract of freshwater fishes on some abiotic and biotic factors. Hydrobiologia 807 (1), 67–85. doi: 10.1007/s10750-017-3383-0
Solovyev M. M., Kashinskaya E. N., Rogozhin E. A., Moyano F. J. (2021). Seasonal changes in kinetic parameters of trypsin in gastric and agastric fish. Fish Physiol. Biochem. 47 (2), 381–391. doi: 10.1007/s10695-020-00919-0
Song Z.-M., Liu J.-Y., Zhuang P., Wang Y., Zhang L.-Z., Hu Y., et al. (2015). Influence of low-temperature stress on the antioxidant enzymes activities and malondialdehyde contents in liver of juvenile Siganus guttatas. Mar. Fisheries 37 (2), 142–150. doi: 10.13233/j.cnki.mar.fish.2015.02.007
Soyano K., Mushirobira Y. (2018). The mechanism of low-temperature tolerance in fish. Adv. Exp. Med. Biol. 1081, 149–164. doi: 10.1007/978-981-13-1244-1_9
Stefansson B., Sandholt G. B., Gudmundsdottir Á. (2017). Elucidation of different cold-adapted Atlantic cod (Gadus morhua) trypsin X isoenzymes. Biochim. Biophys. Acta 1865 (1), 11–19. doi: 10.1016/j.bbapap.2016.10.005
Sun X., Huang X., Huang Z., Cao X., Zhou T., Lin H., et al. (2019). Diet feeding, oxygen consumption rhythm and gastrointestinal evacuation time of Trachinotus ovatus. South China Fisheries Sci. 15 (5), 77–83. doi: 10.12131/20190072
Tingley M. P., Huybers P. (2013). Recent temperature extremes at high northern latitudes unprecedented in the past 600 years. Nature 496, 201–205. doi: 10.1038/nature11969
Tsuchiya C., Sakata T., Sugita H. (2008). Novel ecological niche of Cetobacterium somerae, an anaerobic bacterium in the intestinal tracts of freshwater fish. Lett. Appl. Microbiol. 46, 43–48. doi: 10.1111/j.1472-765X.2007.02258.x
Volkoff H., Rønnestad I. (2020). Effects of temperature on feeding and digestive processes in fish. Temperature (Austin) 18;7 (4), 307–320. doi: 10.1080/23328940.2020.1765950
Wade N. M., Gabaudan J., Glencross B. D. (2017). A review of carotenoid utilisation and function in crustacean aquaculture. Rev. Aquacult. 9, 141–156. doi: 10.1111/raq.12109
Wang W.-Z., Huang J.-S., Zhang J.-D., Wang Z.-L., Li H.-J., Amenyogbe E., et al. (2021). Effects of hypoxia stress on the intestinal microflora of juvenile of cobia (Rachycentron canadum). Aquaculture 536, 736419, 0044–8486. doi: 10.1016/j.aquaculture.2021.736419
Wang L., Mao Y., Wang Z., Ma H., Chen T. (2021). Advances in biotechnological production of β-alanine. World J. Microbiol. Biotechnol. 37 (5), 79. doi: 10.1007/s11274-021-03042-1
Wang H., Wang Y., Niu M., Hu L., Chen L. (2022). Cold acclimation for enhancing the cold tolerance of zebrafish cells. Front. Physiol. 12. doi: 10.3389/fphys.2021.813451
Wang W.-Z., Zeng Z.-Q., Huang J.-S., Guo Z.-X., Li H.-J., Chen G. (2020). Effects of hypoxia stress on antioxidation, immunity and energy metabolism of juvenile cobia, Rachycentron canadum. J. Guangdong Ocean Univ. 40 (5), 12–18. doi: 10.3969/j.issn.1673-9159.2020.05.002
Wegner N. C., Snodgrass O. E., Dewar H., Hyde J. R. (2015). Animal physiology. whole-body endothermy in a mesopelagic fish, the opah, Lampris guttatus. Sci. 348(6236), 786–789. doi: 10.1126/science.aaa8902
Xing X., Song R.-X., Wang L., Niu C.-J., Zhang Z.-B. (2019). The different response of the intestinal mucosal histological features in different sections of juvenile Chinese soft-shelled turtle under acute cold stress. Acta Hydrobiologica Sin. 43 (1), 102–108. doi: 10.7541/2019.013
Yang E.-J., Amenyogbe E., Zhang J.-D., Wang W.-Z., Huang J.-S., Chen G. (2022). Integrated transcriptomics and metabolomics analysis of the intestine of cobia (Rachycentron canadum) under hypoxia stress. Aquacult Rep. 25, 101261, ISSN 2352–5134. doi: 10.1016/j.aqrep.2022.101261
Yang E.-J., Zhang J.-D., Yang L.-T., Amenyogbe E., Wang W.-Z., Huang J.-S., et al. (2021). Effects of hypoxia stress on digestive enzyme activities, intestinal structure and the expression of tight junction proteins coding genes in juvenile cobia (Rachycentron canadum). Aquacult Res. 00, 1–12. doi: 10.1111/are.15438
Yúfera M., Nguyen M. V., Navarro-Guillén C., Moyano F. J., Jordal A.-E. O., Espe M., et al. (2019). Effect of increased rearing temperature on digestive function in cobia early juvenile. Comp. Biochem. Physiol. Part A: Mol. Integr. Physiol. 230, 71–80. doi: 10.1016/j.cbpa.2019.01.007
Yukgehnaish K., Kumar P., Sivachandran P., Marimuthu K., Arshad A., Paray B. A., et al. (2020). Gut microbiota metagenomics in aquaculture: Factors influencing gut microbiome and its physiological role in fish. Rev. Aquac. 12, 1903–1927. doi: 10.1111/raq.12416
Zhang K.-F., Zhang Z.-I. P., Chen Y., Lin P., Wang Y.-I. L. (2007). Antioxidant defense system in animals. Chin. J. Zool 42 (2), 153–160. doi: 10.13859/j.cjz.2007.02.035
Zhang Z., Zhou C., Fan K., Zhang L., Liu Y., Liu P. (2021). Metabolomics analysis of the effects of temperature on the growth and development of juvenile European seabass (Dicentrarchus labrax). Sci. Total Environ. 769, 145155. doi: 10.1016/j.scitotenv.2021.145155
Zheng J. B., Cao J. W., Mao Y., Su Y. Q., Wang J. (2019). Effects of thermal stress on oxidative stress and antioxidant response, heat shock proteins expression profiles and histological changes in marsupenaeus japonicus. Ecol. Indic. 101, 780–791. doi: 10.1016/j.ecolind.2018.11.044
Keywords: Trachinotus ovatus, low temperature, intestinal enzymes, intestinal histology, metabolomic analysis
Citation: Li R-x, Amenyogbe E, Lu Y, Jin J-h, Xie R-t and Huang J-s (2023) Effects of low-temperature stress on intestinal structure, enzyme activities and metabolomic analysis of juvenile golden pompano (Trachinotus ovatus). Front. Mar. Sci. 10:1114120. doi: 10.3389/fmars.2023.1114120
Received: 02 December 2022; Accepted: 08 February 2023;
Published: 27 February 2023.
Edited by:
Samad Rahimnejad, University of Murcia, SpainReviewed by:
Mikhail Solovyev, Institute of Systematics and Ecology of Animals (RAS), RussiaCopyright © 2023 Li, Amenyogbe, Lu, Jin, Xie and Huang. This is an open-access article distributed under the terms of the Creative Commons Attribution License (CC BY). The use, distribution or reproduction in other forums is permitted, provided the original author(s) and the copyright owner(s) are credited and that the original publication in this journal is cited, in accordance with accepted academic practice. No use, distribution or reproduction is permitted which does not comply with these terms.
*Correspondence: Jian-sheng Huang, aHVhbmdqc0BnZG91LmVkdS5jbg==
†These authors have contributed equally to this work
Disclaimer: All claims expressed in this article are solely those of the authors and do not necessarily represent those of their affiliated organizations, or those of the publisher, the editors and the reviewers. Any product that may be evaluated in this article or claim that may be made by its manufacturer is not guaranteed or endorsed by the publisher.
Research integrity at Frontiers
Learn more about the work of our research integrity team to safeguard the quality of each article we publish.