- 1Institut des Sciences de la Mer, Université du Québec à Rimouski, Rimouski, QC, Canada
- 2TBM environnement et SOMME, Brest, France
- 3CNRS, LEMAR, UMR 6539, Institut Universitaire Européen de la Mer, Plouzané, France
- 4Department of Biology, University of Victoria, Victoria, BC, Canada
- 5BOREA, UMR-MNHN, CNRS, UPMC, IRD, UCN, UA, Muséum National d’Histoire Naturelle, Paris, France
Anthropogenic noise is a pervasive feature of the coastal ocean soundscape and is intensifying as vessel traffic activity increases. Low-frequency sounds from wave action on coastal reefs or anthropogenic noise have been shown to initiate larval settlement of marine invertebrates and accelerate metamorphosis to juvenile stages. These results suggest that some planktonic species can perceive and be impacted by anthropogenic sound. Hence, we tested the hypothesis that vessel noise has an impact on the feeding behavior of blue mussel (Mytilus edulis) veligers and of the copepod Eurytemora herdmani as well as on the growth of the rotifer Brachionus plicatilis. The results show that microalgae and feeding behavior of early life stages of mussels and copepods are not influenced by the presence of vessel noise. The growth of the rotifers was similar between the two sound treatments, but rotifers’ egg production in the absence of vessel noise was higher and eggs were also larger. Our results suggest that the effects of noise on plankton are complex; much more work is needed to unravel these often subtle effects.
1 Introduction
Acoustics are an emerging field of research in coastal ecology. Scientists use underwater acoustic technologies not only to determine the sound composition of the aquatic environment (the “soundscape”), but also to study wildlife responses to natural and anthropogenic sounds (Rountree et al., 2006; Gannon, 2008; Jolivet et al., 2016). There has been an expansion in using ocean environments by humans over the last 50 years (Simard et al., 2016), and low-frequency noise has increased by 32-fold and is now dominated by anthropogenic noise, particularly in coastal environments (McDonald et al., 2008). Studies on the impact of anthropogenic noise on marine life have mostly focussed on marine mammals and fishes (Popper, 2003; Barlow and Gisiner, 2006; Popper and Hawkins, 2016), but few data are available for zooplankton species (Day et al., 2016; McCauley et al., 2017; Fields et al., 2019). The importance of zooplankton in marine food webs is well known (Sameoto et al., 1994). They sustain major fisheries and aquaculture industries, and any factor modifying their diversity or productivity can have important environmental impacts. Noisy environments may also affect the behavior of invertebrates (Olivier et al., 2023; Solé et al., 2023), for example mussel larvae settle more rapidly and at a higher rate when they are exposed to vessel noise leading to smaller settlers (Wilkens et al., 2012; Jolivet et al., 2016). Other invertebrate larvae also change their behavior when exposed to vessel noise including the ascidian, Ciona intestinalis, which shows more intensive settling in the presence of vessel noise (McDonald et al., 2014). However, little information is available on the effect of vessel noise on feeding, growth and survival of zooplankton.
In this study, we tested the hypothesis that the level of vessel noise measured in coastal environments by Jolivet et al. (2016) negatively impacts feeding behavior, growth and egg production of different zooplankton species. We used different biological models, such as larvae of the blue mussel (Mytilys edulis), rotifers and copepods to get a better understanding of the impact of vessel noise on organisms with different life cycles and the presence of feeding appendages for copepods. Mussel larvae feed with a ciliate velum and copepods with feeding appendages (Koehl and Strickier, 1981) and we suggest that cilia from the velum could be perturbed by water vibration or particle motion generated by vessel noise, decreasing feeding success. The blue mussel, a major aquaculture species around the world, has been mainly grown in protected nearshore areas, like bays and estuaries (Camacho et al., 1991; Drapeau et al., 2006) corresponding to environments that are exposed to important levels of vessel noise. Pelagic stages of the blue mussel include the D-stage veliger up to the pediveliger stage, representing the competent stage to explore the substrate, settle and metamorphose into juveniles. In contrast to mussels, copepods spend their entire life cycle in the water column. They are a major zooplankton component and present in all oceans. Eurytemora herdmani, is a neritic species and dominates the coastal and estuarine zooplanktonic community (Runge and Simard, 1990). As their feeding behavior is mainly influenced by abiotic factors (Escribano and McLaren, 1992), it could also be affected by vessel noise. Modification of feeding behavior could negatively impact growth and reproduction of copepods as well as the other species that are dependent on them. The non-crustacean zooplankton, Brachionus plicatilis is a rotifer, which is easy to rear in large quantities and to harvest. It is the most commonly used species for live feed in aquaculture hatcheries all over the world. B. plicatilis can reproduce by parthenogenesis (Gilbert, 1977), so the number of individuals in a population can double in 24 h (Hirayama and Kusano, 1972). When conditions are suboptimal, rotifers may use sexual reproduction (Gilbert, 1977) and population density may decrease. Its small size (less than 400 µm) and its cruising swimming behavior in the water column makes it a suitable first live prey for first feeding stages of fish larvae.
In this study, our objectives were to determine the impact of vessel noise on: i) the feeding behavior of blue mussels (D-larvae and veligers) and copepods (E. herdmani) and ii) the growth and egg production of rotifers (B. plicatilis) under optimal and suboptimal physiological conditions obtained by different feeding treatments. Clearance rates were used to measure feeding behavior while counts and size measurements were used to quantify rotifer growth and egg production. No information is available on potential perception of noise in zooplankton species. However, generally these species have ciliated mechanosensory cells, in their statocyst or corona depending on species, suggesting potential perception and negative impacts of anthropogenic sounds considered now as emergent pollutants. We then hypothesized that vessel noise would modify reproductive behavior and number/size of eggs.
2 Materials and methods
2.1 Underwater sound
As described in Jolivet et al. (2016), the vessel noise emitted in the experimental tanks was originally recorded at a mussel aquaculture site at St. Peter’s Bay on Prince Edward Island (Canada, 46° 25.963 N; 62° 39.914 W). A hydrophone (High Tech, Inc., Mississippi, USA, HTI-99-HF: sensitivity −169.7 dB re 1 V/μ Pa; frequency range 2 Hz to 125 kHz flat response) connected to an underwater acoustic recorder (RTSYS-Marine Technologies, France, EA-SDA14, 156 kHz, 24-bit resolution) was placed 25 cm from the bottom, near the anchor of the mussel line. The boat (11 meters long, D & H Boatbuilding hull with diesel motors, Cummins 300 hp C series) passed three times above the recording hydrophone during calm natural conditions characterized by a wave height of 0.2 m and wind speed of 3.8 m s−1 (http://climat.meteo.gc.ca/). Source sound levels were determined with MATLAB (The MathWorks, Inc.) to obtain a 30 s sequence corresponding to vessel noise at maximum sound intensity which was looped during experiments.
2.2 Organism maintenance
All experiments were carried out at the UQAR-ISMER wet laboratory facilities (Rimouski, Qc, Canada). Mussels, Mytilus edulis, from St. Peter’s Bays, Prince Edward Island (Canada) were spawned and reared according to Rayssac et al. (2010). Briefly, spawning was induced by thermal shock and the larvae were reared in three 60 L conical tanks. Water was changed every 2-3 days before the addition of a food mixture of Diacronema lutheri, Tetraselmis suecica and Chaetoceros gracilis at 30 000 cells ml-1. When larvae were competent to settle (development of eyespot and foot), they were transferred to three downweller systems to facilitate metamorphosis. For experiments, we used D-larvae (7-day post-fertilization, 120.5 ± 0.2 µm) and veligers (16-day post-fertilization, 150.5 ± 0.38 µm).
Adult copepods (E. herdmani) without sex differentiation were sampled in the St. Lawrence Estuary (Rimouski: 48° 28’ 51.0”N 68° 31’ 03.4”W) on October 24 and November 8, 2017. Zooplankton was obtained by 100 m horizontal tows from a pier repeated 6 times using a ring plankton net of 0.5 m diameter and 250 μm mesh size. Samples were preserved in a cooler with air bubbling for transport to the wet laboratory within one hour. Zooplankton was maintained in 40 L tanks at 15°C with air bubbling and fed Tetraselmis suecica (a green alga) at a concentration of 30 000 cells ml-1 until the start of the experiments. The mean prosomen length of the copepods in the experiments was 689 ± 5.19 µm.
Rotifers (B. plicatilis) were reared in an 18 L tank using filtered (0.2 µm) seawater in a greenhouse under natural photoperiod conditions and at temperatures >20°C following methodology described in Martinez-Silva et al. (2018). Each morning, the number of individuals in rearing tanks was estimated to adjust food concentration according to culture density. Rotifers were fed three times a day. Two batches of rotifers were reared to obtain rotifers with two physiological conditions. One was fed with the commercial formulation SELCO® (Sparkle, INVE Aquaculture Ltd., Thailand), corresponding to the optimal conditions, and the second with the microalgae concentrate REED (1:1:1 Nannochloropsis occulata: Isochrysis galbana: Diacronema lutherii, Instant algae, REED Mariculture, CA, USA), corresponding to the suboptimal conditions. Rotifers of 159 ± 2.3 µm were used for experiments. Lipid analysis was used to obtain the physiological conditions of those rotifers fed with different foods, SELCO® or REED, as lipids represent their main energetic reserves (Seychelles et al., 2009).
2.3 Experimental design
All experiments were conducted in a similar system described in Jolivet et al. (2016) consisting of an isolated quiet room with four 40 L tanks, each one containing 30 L of water and two multiwell plates (6 x 20 mL) placed on a platform 18.5 cm from the tank bottom to keep plates’ rims 1 cm above the surface (Figure 1). Each tank was placed individually on 13 cm of isolating foam (Foamular C-300, Owens Corning, Toledo, OH, USA). Tanks were used to emit underwater sound and to maintain constant temperature (19 ± 2°C) monitored with HOBOware (Hobo Pendant Temperature/Light 64K Data logger UA-002-64, Onset, Bourne, MA, USA). Low intensity lights (133.18 ± 24.02 lux) were aligned and adjusted above each tank with a photometer (Q201 Quantum PAR Radiometer, Irradian Limited, East Lothian, Scotland) with a natural light period (12:12 h). Each tank corresponded to an acoustic treatment (two sound treatments tanks and two control tanks). Each experiment was replicated twice for each species on different rearing batches. Filtered (until 0.2 µm mesh) and UV treated sea water (23.7 PSU to 27.6 PSU between experiments) was used in the experimental chambers and organisms were fed with microalgae culture at a final concentration of 30 000 cell ml-1 per chamber. The microalgae, D. lutheri was used in the experiments with the mussels (D-larvae and veligers) and the rotifers, whereas Tetraselmis suecica in the experiments with the copepods. Microalgae species were selected for their optimal retention efficiency. Motile flagellate species were selected to decrease sedimentation potential during the 24 h experiment and preliminary tests on two plates (12 chambers) by phytoplankton species (T. sueccica and D. lutheri) showed less than 10% of sedimentation for both species. Sedimentation was estimated by cell concentration measured on the 5 ml surface seawater in each 6 chambers. Initial and final microalgae concentrations were measured using a coulter particle analyzer (Multisizer 4e, Beckman Coulter, Indianapolis, IN, USA).
For each vessel noise tank, underwater loud speakers (AQUA 30, 8 Ohms, 80–20,000 Hz, DNH, Sharon Hill, PA, USA) were placed in the middle of two sound treatment tanks and were connected to an amplifier (Brio-R, Rega, UK) and a computer that continuously replayed vessel noise using VLC software. Consequently, each multiwell plate was located 10 cm from the centre of the source. The sound under experimental conditions was calibrated to replicate as best as possible the shape of the in situ spectrum of vessel noise with a digital recorder (Song Meter SM4 Acoustic Recorder, Wildlife acoustics, Maynard, MA, USA) connected to a hydrophone (SM3/SM4, Wildlife Acoustics) recording frequencies from 2 Hz to 48 kHz with a sensitivity of -165 dB re 1 V/uPa. To realize calibration, the two multiwell plates were replaced by 250-ml jar on the platform, as the hydrophone was too large for the 20-ml well, and sound level analysed using MATLAB (The MathWorks, Inc.). Sound measurements can’t be made directly within one cell, but we reasonably expect similar sound transmission between the underwater speaker and the jar as fluid characteristics are similar on both sides of plexiglass walls of either jar or wells. Thus, two measures were obtained per tank, two tanks per treatment for a total of 4 measures per treatment. The results allowed us to adjust the sound level in the tank by changing the gain from the amplifier and the sound level in the VLC software to match the sound conditions measured in the field. As noted by Jolivet et al. (2016) with the use of the same system, the multiple reflections off the glass sides of the tanks produced relatively homogeneous sound conditions (SEM: ± 1.5 dB) over the jars. Recordings in the control tanks were also made to validate the presence of “silent” conditions where sound without vessel noise was played.
2.4 Feeding experiments
For the experiments with the copepods, the organisms were individually selected and three of them were placed per chamber, each one containing 5 ml of filtered sea water. For the experiments with the mussel D-larvae or veligers, a prior count of larval concentration in the tank was obtained to use around 7.5 mussels ml-1 per chamber. When all the chambers were filled with organisms, food (30 000 cell ml-1 of microalgae), and the last 5 ml of filtered seawater were added and animals were exposed to sound treatments. In each tank, one plate of 6 X 10 ml chambers with the organisms and one control plate (6 chambers) with only the microalgae were used (Figure 1). Individuals in one chamber being independent from other chambers, each chamber was considered as a replicate. Thus, for each treatment, the n= 12 (6 chamber X 2 tanks). Control plates with only microalgae were used to estimate if vessel noise impacted survival of microalgae. After 24 hours, 50 µl of Lugol fixative was added to each chamber to fix the microalgae and the organisms. The remaining liquid was then passed through a 20 µm filter to remove experimental organisms (mussels, copepods, and rotifers) and then microalgae concentration was measured using a coulter counter. Organisms were counted and identification of sex, stage, species, and length of the copepods was done using an Olympus SZ61 binocular microscope (4.5-20X; model SZ2-ST; Olympus Corporation, Tokyo, Japan). Mussel larvae were measured with an Olympus BX41 microscope (100X). Pictures were taken using an Evolution VF colour camera and the software Image-Pro Express 5.1.0.12 (Media Cybernetics, Inc., USA).
The clearance rate (CR) was calculated using a modified formula described in Comeau et al. (2008):
where C1 is the microalgae concentration (cells ml-1) in the control chamber after 24h; C2 is the microalgae concentration in each chamber at T0; C3is the microalgae concentration in the experimental chamber after 24h; V is the volume (ml) of filtered sea water in chambers; T is the duration (days) of the experiment; and N the number of organisms per chamber.
2.5 Growth experiments
To estimate growth, twenty small rotifers were selected and placed in a cell with 5 ml of filtered sea water, and as already described, microalgae and the last 5 ml of filtered sea water were added when all chambers had been filled with rotifers. In each tank, two plates of 6 X 10 ml chambers were used (one with microalgae and organisms, and one control with only microalgae). After 24 hours, each cell received 50 µl of lugol and the number of rotifers was counted. Total numbers in each cell and plate were pooled together to obtain the total per aquarium (two tanks replicates per sound treatment). The body length of each individual was measured with a microscope (Olympus BX41) as described above and the number of eggs attached to each individual also counted.
2.6 Lipids analysis
Two samples of 20 000 rotifers were collected from each replicate rearing tank and rinsed with filtered sea water (0.2 µm) with a 50 µm net. The samples were filtered onto precombusted (450°C) 25 mm GF/C filters. One filter was stored in 1 ml chloroform in amber glass vials with Teflon-lined caps at -80°C until lipid analyses, and the other was rinsed with ammonium formate (3%) and used for dry weight determination (70°C for 24 h). Lipids were extracted in dichloromethane–methanol using the modified Folch procedure (Folch et al., 1957) described in Parrish (1987). Fatty acid methyl esters (FAME) were prepared by transesterification as described in Lepage and Roy (1984) and eluted on an activated silica gel with hexane and diethyl ether to eliminate the free sterols. Fatty acids were analysed using a multichannel Trace GC ultra (Thermo Scientific) gas chromatograph equipped with a Triplus autosampler, a PTV injector, and a ITQ900 (Thermo Scientific) mass detector, and analyzed with Xcalibur v.2.1 software (ThermoScientific, Mississauga, ON, CA). FAMEs were identified with known standards (Supelco 37 Component FAME Mix and menhaden oil; Supleco Inc., Belfonte, PA, USA) after manual verification of the fatty acids integration.
2.7 Data analysis
We used Rstudio v.1.1.368 for data analysis. The levels of sound emitted in tanks in the presence or absence of vessel noise were compared using t-tests for each of the three frequency groups (100-10 000, 100-1 000 and 1 000-10 000 Hz). All analyses on feeding and growth experiments were done using linear mixed-effect models (lmer in R). For feeding, clearance rate in each species was compared with sound (presence or absence of vessel noise) as a fixed factor, and batch (two batches for each experiment) and tanks (two tanks per sound treatment) as random factors. Effects of sound exposure on microalgae used as food were tested on all experiments combined together. We used a linear mixed-effect model (lmer in R) with sound effect (presence or absence of vessel noise) as a fixed factor, and experiments (6 experiments represented by two batches of copepods, D-larvae and veliger larvae) and tanks (two tanks per sound treatment) as random factors. For growth experiments on rotifers, t-tests were used for each rotifer experiment (fed with SELCO or REED) to compare sizes of rotifers exposed or not to vessel noise. Similar analyses were used for egg production by rotifers. Total fatty acid content in rotifers fed with SELCO and REED were compared with Student t-tests.
Homoscedasticity and normality were tested using Levene and Kolmogorov-Smirnov tests respectively. When necessary, data were transformed using logarithm functions. PRIMER software (version 7.0.13) was used to perform multivariate PERMANOVA analyses to compare fatty acid composition of each rotifer feeding treatment (REED and SELCO) based on Euclidean dissimilarities following validation of the assumptions of homoscedasticity using PERMDISP tests. The SIMPER procedure was performed to identify FA explaining the most important dissimilarity between treatments.
3 Results
The results observed for each frequency group indicates that the sound level was relatively homogeneous through all the experiments for each sound treatment (Table 1, Figure 2). Sound levels in the tank in the presence of vessel noise corresponded to the in situ source signal for the three different frequency groups. For the two other tanks – treatments without sound emission – sound levels differed sharply from the two tanks exposed to vessel noise. The differences were significant for the three frequency groups (all comparisons: t = 0, p< 0.001).
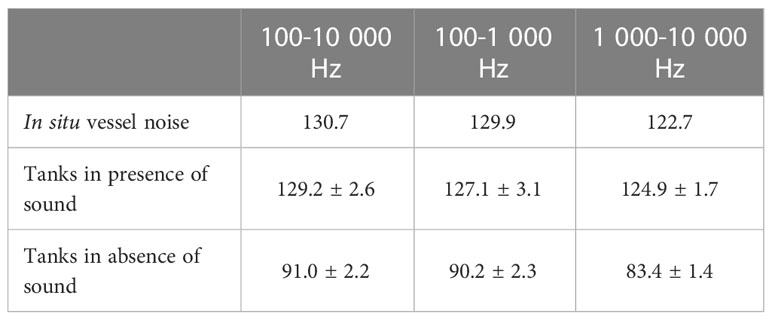
Table 1 Sound levels (dB re 1 µPa) measured in situ and in the experimental tanks: two tanks in presence of vessels sound and two tanks in absence of vessels sound.
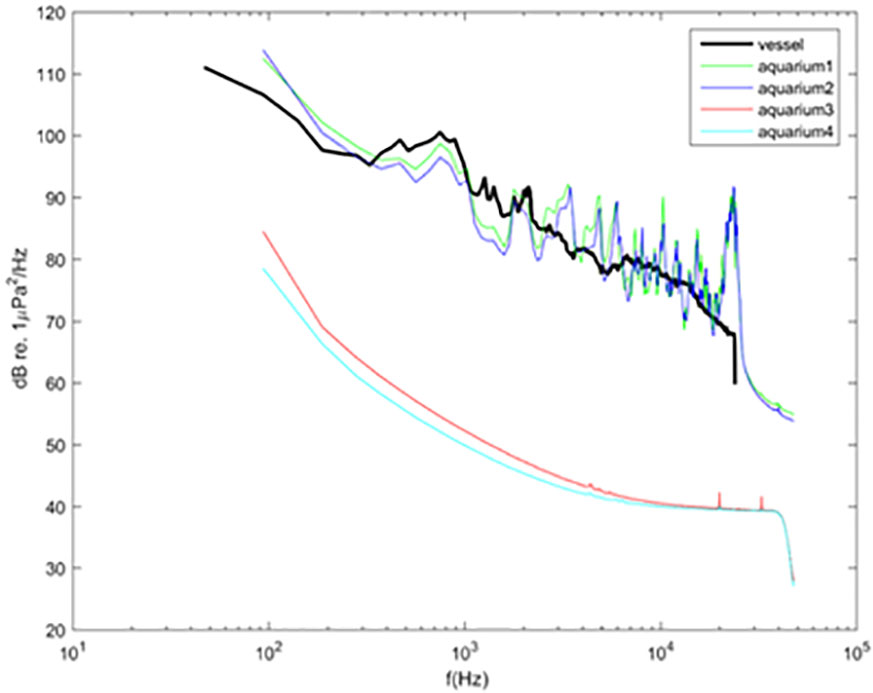
Figure 2 Mean sound spectra (dB re 1 μPa 2 Hz −1) for the different experiments. Bold black line represents the vessel noise recorded in situ and the other lines of the spectra of sounds recorded in the four tanks used. Green and blue lines are from tanks 1 and 2 for the sound treatment and red and light-blue lines are from tanks 3 and 4 for the silent treatment.
3.1 Phytoplankton
Microalgae concentration at the end of the control experiments (without zooplankton species) was not modified by vessel noise (df = 1 and 11, F = 0.15, p = 0.74) and there was no tank effect (df = 1, X2 = 2.67, p = 0.1). However, we observed a difference in the initial concentration between experiments (df = 1, X2 = 80.64, p< 0.001) related to the estimation of microalgae concentration at the beginning of each experiment. Copepods were fed initially with an average of 23 338 ± 76 cell ml-1, mussel D-larvae with 24 156 ± 127 cell ml-1 and mussel veligers with 21 830 ± 115 cell ml-1.
3.2 Mussel larvae
The clearance rates of the one-week-old D-larvae were similar for individuals exposed or not to vessel noise (df = 1 and 11, F = 0.02, p = 0.90) with no aquarium effect (df = 1, X2 = 3.34, p = 0.07) and no batch effects (df = 1, X2 = 0.0, p = 1.0) (Figure 3). The two-week-old veliger mussels also showed clearance rates independent of the presence or absence of the vessel noise (df = 1 and 11, F = 2.08, p = 0.16), no tank (df = 1, X2 = 0.0, p = 1.0) or batch (df = 1, X2 = 0.0, p = 1.0) effects (Figure 3).
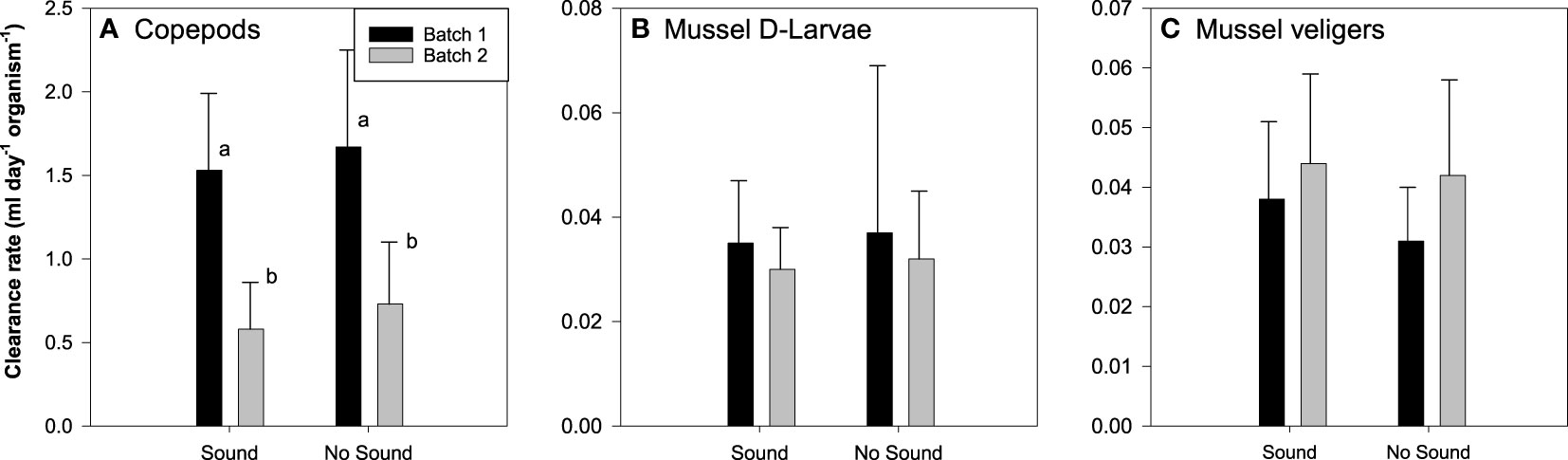
Figure 3 Mean clearance rates (ml day-1 organism-1) ± standard deviation of zooplanktonic species [(A) Copepods, (B) Mussel D-larvae, (C) Mussel veligers] submitted or not to vessels noise exposure. Different letters indicate a significant difference. N = 12.
3.3 Copepods
Vessel noise showed no impact on feeding behavior of the copepod E. herdmani (df 1 and 11, F = 0.119, p = 0.74) and no tank effect was noted (df = 1, X2 = 2.84-14, p = 1). Each of the two batches of copepods (each one with 12 replication levels) showed different clearance rates (df = 1, X2 = 0.33, p< 0.001), but each batch showed no impact of vessel noise on their respective feeding behavior (df = 1 and 11, F = 1.15, p = 0.29 and df = 1 and 11, F = 0.28, p = 0.65) (Figure 3).
3.4 Rotifers
The sum of total fatty acid concentrations of rotifers fed the SELCO formulation (347 ± 41 µg·mg-1) was higher (t = 10.883, p< 0.0001) than those of rotifers fed the REED microalgae concentrate (76 ± 6 µg·mg-1) (Table 2). Their fatty acids composition was also significantly different (df=1 and 9, pseudo-F = 15.48, p = 0.007). The SIMPER analysis showed that 16:0 and 18:0 saturated fatty acids explained over 43.8% of the differences in fatty acids composition of rotifers fed with REED and SELCO. Rotifers fed the REED microalgae concentrate showed higher levels of saturated fatty acids. Rotifers fed SELCO formulation accumulated 2 to 3 times more essential polyunsaturated fatty acids (20:5n3, 22:6n3 and 20:4n6) than those fed microalgae.
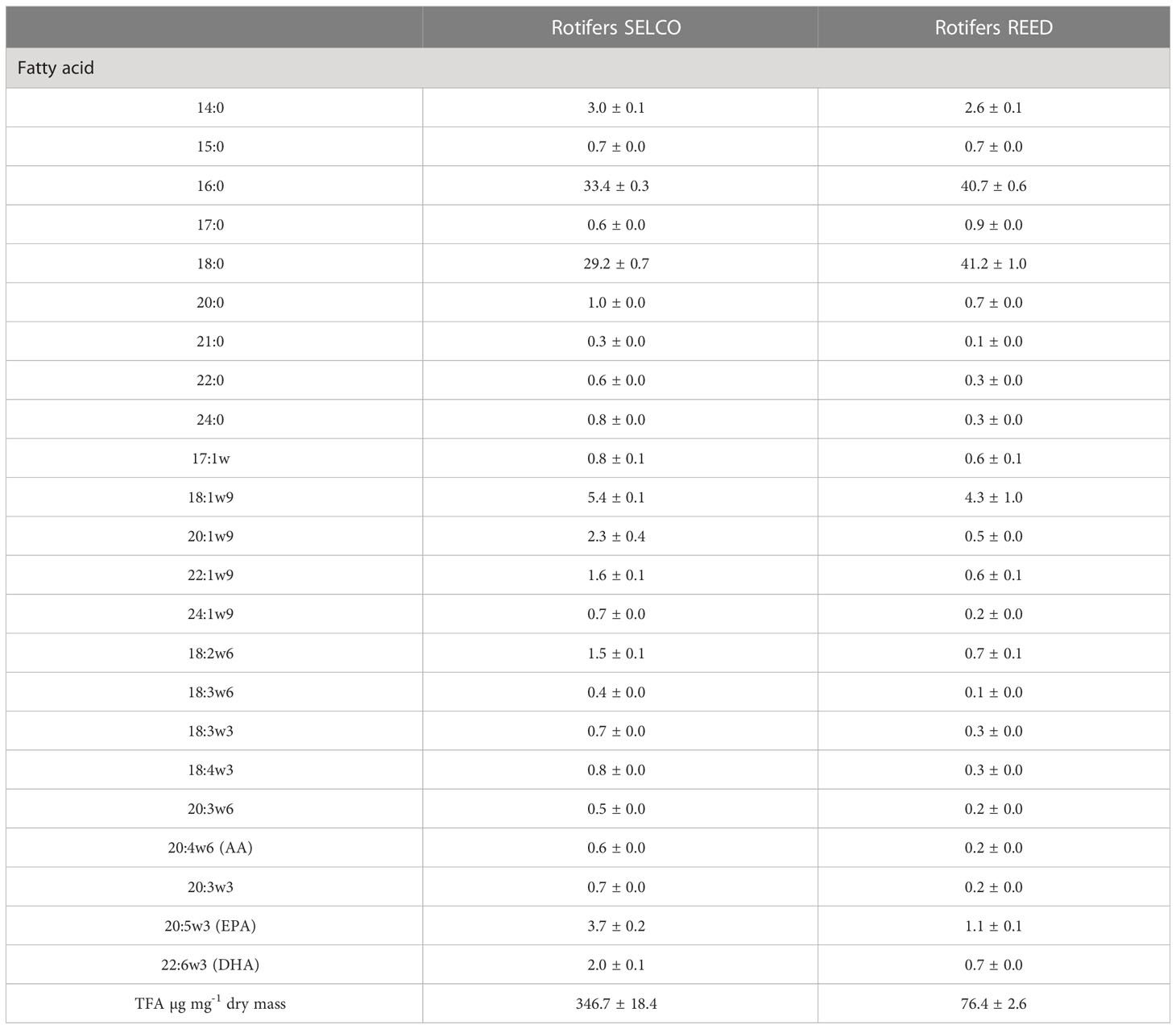
Table 2 Fatty acid composition and total fatty acid concentration of rotifers fed SELCO formulation or REED microalgae concentrate.
For experiments using rotifers fed with REED, no impact of vessel noise was observed on total length (n = 718, t = 1.72, p = 0.09) with a mean ( ± SD) of 164.6 ± 18.5 µm (presence and absence of vessel noise treatments together). Similarly, for rotifers fed SELCO, there was no effect of vessel sound (n = 793, t = 1.654, p = 0.10). However those fed SELCO were slightly longer (9%, but not significantly) than the REED fed rotifers (n = 718, t = 0.27, p = 0.63), resulting in a mean rotifer length of 178.2 ± 14.5 µm. Females with one egg occurred in experiments with the SELCO feeding regime but, females with 2 eggs were not observed in any of the experiments. Vessel noise had a significant effect on the egg production and the egg size of SELCO fed rotifers. In the control treatment (absence of vessel noise), 44% more eggs were produced (U = 0.011, p = 0.029) and eggs were slightly larger (t = 2.154, p = 0.034) compared to the sound treatment (Figure 4).
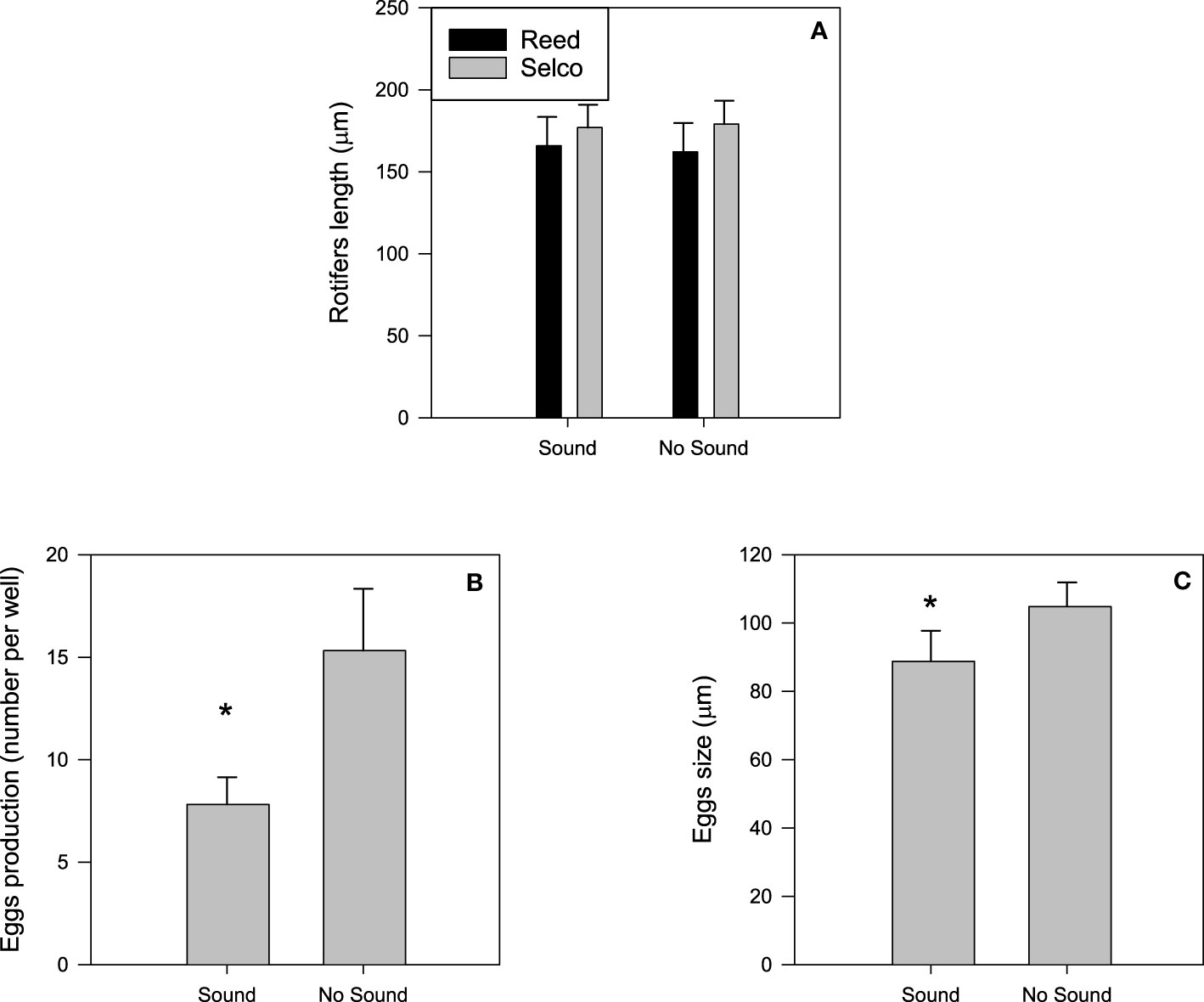
Figure 4 Impact of vessels noise exposure on length of rotifers (A) fed on two feeding diets (Reed and Selco) and on eggs productivity (B) and their length (C) produced by rotifers feed on Selco. No egg production was observed for rotifers feed on Reed. Asterix indicates a significant difference. Mean ± standard deviation, N= 12.
4 Discussion
Our results do not support the hypothesis that vessel noise negatively affects feeding behavior and growth of different zooplankton species. However, vessel noise negatively impacted rotifers egg production in those fed with higher fatty acid content. Our study represents a rare example of experimental studies focusing on the impact of anthropogenic noise on planktonic marine life (Chauvaud et al., 2018). We obtained data in small tanks characterized by the presence of reverberation, absent in field conditions. However, we replicated the sound level measured in the field and the sound spectrum exposure was similar to the natural exposure of zooplankton. In the present experiment the water volume was small, so that particle motion could not be measured. In the absence of technology to measure particle motion in small volumes of 200 ml, as used in the present study, only the pressure component of sound has been measured.
4.1 Phytoplankton
Microalgae concentrations from the control cells (no consumer/predator) at the beginning and at the end of each experiment showed no differences in presence or absence of vessel noise. Thus, vessel noise did not stimulate microalgae culture growth or cell death. In our experiments, the light level was too limiting to stimulate important culture growth. Concentrations were therefore stable during the 24h experiment and microalgae were still in suspension and available for zooplankton feeding.
4.2 Feeding behavior
We still do not completely understand how small invertebrates like mussel larvae and copepods detect marine sounds. However, McCauley et al. (2017), observed that low-frequency acoustic air gun impulse used at high level during seismic surveys decreased zooplankton abundance, by a level over two-fold. Copepods showed higher mortality within 10m distance of to an air gun impulse but no effect further form the sound source (Fields et al., 2019). Whereas, Jolivet et al. (2016) showed a positive impact of vessel noise on the settlement of mussel larvae which strongly suggests that mussel larvae might be able to sense the water vibration or the particle motion generated by vessel noise, similar to adults sensing substrate-borne vibration in the range of 5 Hz to 400 Hz (Roberts et al., 2015; Olivier et al., 2023). This perception may be due to the presence of a pair of statocysts at the base of the foot, as observed in pediveliger of different bivalve species (Cragg and Nott, 1977; Bellolio et al., 1993). Statocysts are formed by invagination of the foot epithelium forming a spherical sac connected to the mantle cavity by a cylindrical ciliated canal and are used to orientate crawling. This ability to perceive noise and use it as a cue may also explain why we did not see any effect of noise on feeding behavior. As suggested by Jolivet et al. (2016), the natural habitat of the blue mussel is the near shore which is characterized by wave crashes on rocks producing a large range of underwater sound, including the range of intensity and frequency produced by the vessel noise used in our study. Thus, if we consider that vessel noise mimics natural noise present in the near shore, it was not surprising that mussel feeding behavior was not affected by it. Thus, our results suggest that mussels exposed to vessel noise maintain their ability to gain the energy needed for their future settlement and metamorphosis. The absence of an impact of vessel noise on the clearance rates of mussels was observed on two ontogenetic larval stages.
When comparing the veliger clearance rates with literature values for larvae of similar size (156 µm mussel larvae, Sprung, 1984), our results show lower values despite similar food concentration and temperature conditions. Sprung (1984) used a food concentration of Isochrysis galbana of 20,000 cells ml-1 and obtained a clearance rate of 0.1056 ml day-1 larva-1 with a decrease to 0.0504 ml day-1 larva-1 when algal concentration was 40,000 cells ml-1. The microalgae size of D. lutheri (4–6µm), used here, was slightly larger than the size of I. galbana (4.5 µm) used in Sprung’s (1984) experiment. Since retention efficiency of mussel larvae is maximal for phytoplankton of 3.5 µm in diameter, the size of D. lutheri could explain the lower clearance rate we observed. Food availability can also affect the filtration rate of bivalves (Hawkins et al., 1998), but in our experimental conditions, no food limitation was observed at the level of 30,000 cells ml-1.
We also found no impact of vessel noise on the clearance rate of the copepod Eurytemora herdmani. Previous studies have found that the clearance rate of different species of copepods is dependent on algal concentration and can be adjusted until a maximum rate is reached (Conover, 1956; Mullin, 1963). Tackx et al. (2003) obtained clearance rates of E. affinis ranging from 0.24 ml to 0.36 ml day-1 copepod-1, similar to clearance rates in our experiment. There is few information on the effects of noise on copepods as emphasized in comprehensive reviews, such as those by Popper and Hawkins (2016); Chauvaud et al. (2018) and more recently Bonnel et al. (2022) and Solé et al. (2023). Copepods can perceive underwater sound at the adult (Yen et al., 1992) or copepodite stage (Solé et al., 2021b) through mechanoreceptors (sensory setae) found on the first antenna (Weatherby and Lenz, 2000). Yen et al. (1992) showed that the effective range of stimulation was 40-1000 Hz and that spikes could be triggered with displacement velocities as small as 10 nm that fits within the range of particle motion associated to underwater sounds. The response to these mechanical stimuli were variable among the 15 copepod species tested (Yen et al., 1992). The absence of a response in feeding rate when exposed to vessel noise is thus surprising but might be related to the small model species Eurytemora herdmani tested in the present study. Indeed, McCauley et al. (2017) and Fields et al. (2019) assessed via in situ sampling and experiments, respectively, the seismic air gun impacts on copepods. Results were contradictory as low mortality was observed after seismic surveys exposure for Calanus finmarchicus (Fields et al., 2019) whereas major impacts were shown on diverse zooplankton assemblage including copepods (McCauley et al., 2017). Solé et al. (submitted) suggest that such opposite results can be explained by the size of the plankton species as the less impacted C. finmarchicus has a much larger size than the small copepod species that were mostly affected by the seismic air gun impulses in the study of McCauley et al. (2017). Solé et al. (2023) suggests that the impact of noise on marine organisms might be species-specific.
Our study used different invertebrate organisms that each feed with morphologically different apparatus. Mussel larvae feed with a velum and copepods with feeding appendages (Koehl and Strickier, 1981). In spite of those differences in the feeding appendages, we did not find an impact of vessels noise on any of these organisms.
4.3 Growth and egg production
The higher total fatty acid concentration and higher content in essential polyunsaturated fatty acids in the rotifers fed the SELCO formulation explains at least partially their better growth and egg production compared to those fed REED (Srivastava et al., 2006). The REED fed rotifers accumulated high levels of saturated fatty acids (Lubzens et al., 1985). SELCO is a commercial formulation specifically designed for the production and rearing of rotifers. Rotifers fed with SELCO contained sufficient essential fatty acids (EPA: 20:5n-3, DHA: 22:6n-3 and AA: 20:4n6) to stimulate high levels of growth and reproduction (Fernandez-Reiriz et al., 1993; Dhert et al., 2001). For example, EPA is known to be a fatty acid that is essentially required to sustain growth and reproduction of different invertebrates (Ravet et al., 2003; Guo et al., 2016) such as Daphnia (Müller-Navarra et al., 2000; Gladyshev et al., 2008), or purple sea urchin (Sanna et al., 2017), insects (Stanley-Samuelson, 1994a), and other invertebrates (Stanley-Samuelson, 1994b). The rotifer B. plicatilis is one of the very few organisms able to biosynthesize PUFA in conditions of food deficiency (Lubzens et al., 1985; Bell and Tocher, 2009). However, the rate of this biosynthesis is low and food deficiency in essential fatty acids does not support high levels of growth and reproduction (Lubzens et al., 1985). Thus, due to the use of REED and SELCO in different rotifer batches, it was possible to obtain rotifers with different physiological conditions. The poor condition of rotifers fed REED did not allow us to detect an impact of vessel noise. In the absence of vessel noise, rotifers fed REED did not produce eggs suggesting that their condition was not good enough to invest energy in their reproduction. However, we observed an impact of vessel noise in rotifers fed SELCO. Since the rotifers in the absence of sound and fed with SELCO produced eggs, their physiological condition was able to sustain energy investment in reproduction. When exposed to sound, rotifers were probably more stressed, leading to a decrease in their energy investment in egg production which resulted in low numbers of smaller eggs. No information is available on organs in rotifers that would allow them to perceive underwater sound. However, the ciliated mechanosensory cells in their corona could be involved, as suggested in cnidarian medusae by Solé et al. (2016). The corona is a ciliated crown of the apical region of the body helping to acquire food and is used for locomotion.
5 Conclusion
No impact of vessels noise was observed on the feeding behavior of the mussel larvae or the copepods. Our study only found an impact of vessel noise on the egg production of rotifers. This information is important for the understanding of the effect of anthropogenic noise on marine life, as zooplanktonic species are at the basis of the marine food web. Thus, this study contributes to fill the gaps in knowledge on the impacts of anthropogenic noise on zooplankton for which little is known.
Data availability statement
The raw data supporting the conclusions of this article will be made available by the authors, without undue reservation.
Author contributions
AA carried out the experiments, performed statistical analysis and has written the manuscript. RT supervised the entire study, established the methodology and provided technical support. FO and LC, provided the underwater acoustic recorder, acoustic support and participated in data analyses. AJ performed the acoustic analysis. CA, GW, and FJ participated in data analyses. All authors contributed to the article and approved the submitted version.
Funding
This work was supported by the Fonds de Recherche du Québec - Nature et Technologies (FRQNT; team project 2016-PR-190063) and Ressources Aquatiques Québec Research Network (FQRNT, strategic network program #2014-RS-171172).
Acknowledgments
The authors wish to thank Nathalie Gauthier for logistical support during the experiment, Jean-Bruno Nadalini and Mathieu Babin for assistance in lipid analyses. The study is a contribution to the international laboratory BeBEST.
Conflict of interest
The authors declare that the research was conducted in the absence of any commercial or financial relationships that could be construed as a potential conflict of interest.
Publisher’s note
All claims expressed in this article are solely those of the authors and do not necessarily represent those of their affiliated organizations, or those of the publisher, the editors and the reviewers. Any product that may be evaluated in this article, or claim that may be made by its manufacturer, is not guaranteed or endorsed by the publisher.
References
Barlow J., Gisiner R. (2006). Mitigating, monitoring and assessing the effects of anthropogenic sound on beaked whales. J. Cetacean Res. Manage. 7, 239–249. doi: 10.47536/jcrm.v7i3.734
Bell M. V., Tocher D. R. (2009). “Biosynthesis of polyunsaturated fatty acids in aquatic ecosystems: general pathways and new directions,” in Lipids in aquatic ecosystems. Eds. Bell M. V., Tocher D. R. (New York, NY: Springer), 211–236. doi: 10.1007/978-0-387-89366-2
Bellolio G., Lohrmann K., Dupre E. (1993). Larval morphology of the scallop Argopecten purpuratus as revealed by scanning electronmicroscopy. Veliger 36, 332–342.
Bonnel J., Chauvaud S., Chauvaud L., Mars J., Mathias D., Olivier F. (2022). “Effets des sons anthropiques sur la faune marine,” in Cas des projets éoliens offshore (Vewrsaille: Editions Quae), 167 p. doi: 10.35690/978-2-7592-3545-2
Camacho A. P., Gonzalez R., Fuentes J. (1991). Mussel culture in Galicia (NW Spain). Aquaculture 94, 263–278. doi: 10.1016/0044-8486(91)90122-N
Chauvaud S., Chauvaud L., Jolivet A. (2018). Impacts des sons anthropiques sur la faune marine (Versailles: Éditions Quae), 112p.
Comeau L. A., Pernet F., Tremblay R., Bates S. S., LeBlanc A. (2008). Comparison of eastern oyster (Crassostrea virginica) and blue mussel (Mytilus edulis) filtration rates at low temperatures. Can. Tech. Rep. ofFisheries Aquat. Sci. 2810, 1–17.
Conover R. J. (1956). Biology of Acartia clausi and A. tonsa. Bull. Bingham Oceanography Collection 15, 156–233.
Cragg S. M., Nott J. A. (1977). The ultrastructure of the statocysts in the pediveliger larvae of Pecten maximus (L.) (Bivalvia). J. Exp. Mar. Biol. Ecol. 27, 23–36. doi: 10.1016/0022-0981(77)90051-X
Day R. D., McCauley R. D., Fitzgibbon Q. P., Semmens J. M. (2016). Seismic air gun exposure during early-stage embryonic development does not negatively affect spiny lobster Jasus edwardsii larvae (Decapoda:Palinuridae). Sci. Rep. 6, 22723. doi: 10.1038/srep22723
Dhert P., Rombaut G., Suantika G., Sorgeloos P. (2001). Advancement of rotifer culture and manipulation techniques in Europe. Aquaculture 200, 129–146. doi: 10.1016/S0044-8486(01)00697-4
Drapeau A., Comeau L. A., Landry T., Stryhn H., Davidson J. (2006). Association between longline design and mussel productivity in prince Edward island, Canada. Aquaculture 261, 879–889. doi: 10.1016/j.aquaculture.2006.07.045
Escribano R., McLaren L. A. (1992). Influence of food and temperature on lengths and weights of marine copepods. J. Exp. Mar. Biol. Ecol. 159, 77–88. doi: 10.1016/0022-0981(92)90259-D
Fernandez-Reiriz M. J., Labarta U., Ferreiro M. J. (1993). Effects of commercial enrichment diets on the nutritional-value of the rotifer (Brachionus plicatilis). Aquaculture 112, 195–206. doi: 10.1016/0044-8486(93)90445-5
Fields D. M., Handegard N. O., Dalen J., Eichner C., Malde K., Karlsen Ø., et al. (2019). Airgun blasts used in marine seismic surveys have limited effects on mortality, and no sublethal effects on behaviour or gene expression, in the copepod Calanus finmarchicus. ICES J. Mar. Sci. 76, 2033–2044. doi: 10.1093/icesjms/fsz126
Folch J., Lees M., Sloane Stanley G. (1957). A simple method for the isolation and purification of total lipids from animal tissues. J. Biol. Chem. 226, 497–509. doi: 10.1016/S0021-9258(18)64849-5
Gannon D. P. (2008). Passive acoustic techniques in fisheries science: a review and prospectus. Trans. Am. Fisheries Soc. 137, 638–656. doi: 10.1577/T04-142.1
Gilbert J. (1977). Mictic-female production in monogonont rotifers. Archiv für Hydrobiologie 8, 142–155.
Gladyshev M. I., Sushchik N. N., Dubovskaya O. P., Makhutova O. N., Kalachova G. S. (2008). Growth rate of daphnia feeding on seston in a Siberian reservoir: the role of essential fatty acid. Aquat. Ecol. 42, 617–627. doi: 10.1007/s10452-007-9146-7
Guo F., Kainz M. J., Sheldon F., Bunn S. E. (2016). The importance of high-quality algal food sources in stream food webs - current status and future perspectives. Freshw. Biol. 61, 815–831. doi: 10.1111/fwb.12755
Hawkins A., Bayne S., Bougrier S., Héral M., Iglesias J., Navarro E., et al. (1998). Some general relationships in comparing the feeding physiology of suspension-feeding bivalve molluscs. J. Exp. Mar. Biol. Ecol. 219, 87–103. doi: 10.1016/S0022-0981(97)00176-7
Hirayama K., Kusano T. (1972). Fundamental studies on physiology of the rotifer for its mass culture. II. “Influence of water temperature on population growth of rotifers”. Bull. Japanese Soc. Sci. Fisheries 38, 1357–1363. doi: 10.2331/suisan.38.1357
Jolivet A., Tremblay R., Olivier F., Gervaise C., Sonier R., Genard B., et al. (2016). Validation of trophic and anthropic underwater noise as settlement trigger in blue mussels. Sci. Rep. 6, 33829. doi: 10.1038/srep33829
Koehl M., Strickier J. R. (1981). Copepod feeding currents: food capture at low reynolds number. Limnol. Oceanogr. 26, 1062–1073. doi: 10.4319/lo.1981.26.6.1062
Lepage G., Roy C. C. (1984). Improved recovery of fatty acid through direct transesterification without prior extraction or purification. J. Lipid Res. 25, 1391–1396. doi: 10.1016/S0022-2275(20)34457-6
Lubzens E., Marko A., Tietz A. (1985). De novo synthesis of fatty acids in the rotifer, Brachionus plicatilis. Aquaculture 47, 27–37. doi: 10.1016/0044-8486(85)90005-5
Martinez-Silva M. A., Audet C., Winkler G., Tremblay R. (2018). Prey quality impact on the feeding behavior and lipid composition of winter flounder (Pseudopleuronectes americanus) larvae. Aquaculture Fisheries 3, 145–155. doi: 10.1016/j.aaf.2018.06.003
McCauley R. D., Day R. D., Swadling K. M., Fitzgibbon Q. P., Watson A., Semmens J. M. (2017). Widely used marine seismic survey air gun operations negatively impact zooplankton. Nat. Ecol. Evol. 1, 195. doi: 10.1038/s41559-017-0195
McDonald M. A., Hildebrand J. A., Wiggins S. M., Ross D. (2008). A 50-year comparison of ambient ocean noise near San clemente island: a bathymetrically complex coastal region off southern California. J. Acoustical Soc. America 124, 1985–1992. doi: 10.1121/1.2967889
McDonald J. I., Wilkens S. L., Stanley J. A., Jeffs A. G. (2014). Vessel generator noise as a settlement cue for marine biofouling species. Biofouling 30, 741–749. doi: 10.1080/08927014.2014.919630
Müller-Navarra D. C., Brett M. T., Liston A. M., Goldman C. R. (2000). A highly unsaturated fatty acid predicts carbon transfers between primary producers and consumers. Nature 403, 74–77. doi: 10.1038/47469
Mullin M. M. (1963). Some factors affecting the feeding of marine copepods of the genus Calanus. Limnology Oceanography 8, 239–250. doi: 10.4319/lo.1963.8.2.0239
Olivier F., Gigot M., Mathias D., Jezequel Y., Meziane T., L’Her C., et al. (2023). Assessing the impacts of anthropogenic sounds on early stages of benthic invertebrates: the ‘Larvosonic system’. Limnology Oceanogr. Methods 21, 53–68. doi: 10.1002/lom3.10527
Parrish C. C. (1987). Separation of aquatic lipid classes by chromarod thin-layer chromatography with measurement by latroscan flame ionization detection. Can. J. Fisheries Aquat. Sci. 44, 722–731. doi: 10.1139/f87-087
Popper A. N. (2003). Effects of anthropogenic sounds on fishes. Fisheries 28, 24–31. doi: 10.1577/1548-8446(2003)28[24:EOASOF]2.0.CO;2
Popper A. N., Hawkins A. (2016). The effects of noise on aquatic life II (New York, NY: Springer), 1243 p. doi: 10.1007/978-1-4939-2981-8
Ravet J. L., Brett M. T., Müller-Navarra D. C. (2003). A test of the role of polyunsaturated fatty acids in phytoplankton food quality for Daphnia using liposome supplementation. Limnol. Oceanogr. 48, 1938–1947. doi: 10.4319/lo.2003.48.5.1938
Rayssac N., Pernet F., Lacasse O., Tremblay R. (2010). Temperature effect on survival, growth, and triacylglycerol content during the early ontogeny of Mytilus edulis and M. trossulus. Mar. Ecol. Prog. Ser. 417, 183–191. doi: 10.3354/meps08774
Roberts L., Cheesman S., Breithaupt T., Elliott M. (2015). Sensitivity of the mussel Mytilus edulis to substrate−borne vibration in relation to anthropogenically generated noise. M Mar. Ecol. Prog. Ser. 538, 185–195. doi: 10.3354/meps11468
Rountree R. A., Gilmore R. G., Goudey C. A., Hawkins A. D., Luczkovich J. J., Mann D. A. (2006). Listening to fish: applications of passive acoustics to fisheries science. Fisheries 31, 433–446. doi: 10.1121/1.4786172
Runge J. A., Simard Y. (1990). “Zooplankton of the st. Lawrence estuary: the imprint of physical processes on its composition and distribution,” in Coastal and estuarine studies, vol. 39 . Eds. El-Sabh M. I., Silverberg N. (New York: Springer-Verlag), 296–320. Oceanography of a large-scale estuarine system, the St. Lawrence. doi: 10.1007/978-1-4615-7534-4
Sameoto D. D., Neilson J., Waldron D. (1994). Zooplankton prey selection by juvenile fish in Nova scotian shelf basins. J. Plankton Res. 16, 1003–1019. doi: 10.1093/plankt/16.8.1003
Sanna R., Siliani S., Melis R., Loi B., Baroli M., Roggio T., et al. (2017). The role of fatty acids and triglycerides in the gonads of Paracentrotus lividus from Sardinia: growth, reproduction and cold acclimatization. Mar. Environ. Res. 130, 113–121. doi: 10.1016/j.marenvres.2017.07.003
Seychelles L., Audet C., Tremblay R., Fournier R., Pernet F. (2009). Essential fatty acid enrichment of cultured rotifers (Brachionus plicatilis) using frozen-concentrated microalgae. Aquaculture Nutr. 15, 431–439. doi: 10.1111/j.1365-2095.2008.00608.x
Simard Y., Roy N., Gervaise C., Giard S. (2016). “A seaway acoustic observatory in action: the st. Lawrence seaway,” in The effects of noise on aquatic life II. Eds. Popper A. N., Hawkins A. (New York, NY: Springer), 1031–1040. doi: 10.1007/978-1-4419-7311-5
Solé M., Kaifu K., Mooney T. A., Nedelec S. L., Olivier F., Radford A. N., et al. (2023). Marine invertebrates and noise. Front. Mar. Sci. 10. doi: 10.3389/fmars.2023.1129057
Solé M., Lenoir M., Fontuño J. M., Durfort M., van der Schaar M., André M. (2016). Evidence of cnidarians sensitivity to sound after exposure to low-frequency noise underwater sources. Sci. Rep. 6, 37979. doi: 10.1038/srep37979
Solé M., Lenoir M., Fortuno J. M., De Vreese S., van der Schaar M., André M. (2021b). Sea Lice are sensitive to low frequency sounds. J. Mar. Sci. Eng. 9, 765. doi: 10.3390/jmse9070765
Sprung M. (1984). Physiological energetics of mussel larvae (Mytilus edulis). II. food uptake. Mar. Ecol. Prog. Ser. 17, 295–305.
Srivastava A., Hamre K., Stoss J., Chakrabarti R., Tonheim S. K. (2006). Protein content and amino acid composition of the live feed rotifer (Brachionus plicatilis): with emphasis on the water soluble fraction. Aquaculture 254, 534–543. doi: 10.1016/j.aquaculture.2005.11.014
Stanley-Samuelson D. W. (1994a). Assessing the significance of prostaglandins and other eicosanoids in insect physiology. J. Insect Physiol. 40, 3–11. doi: 10.1016/0022-1910(94)90106-6
Stanley-Samuelson D. W. (1994b). The biological significance of prostaglandins and related eicosanoids in invertebrates. Am. Zoology 34, 589–598. doi: 10.1093/icb/34.6.589
Tackx M., Herman P., Gasparini S., Irigoien X., Billiones R., Daro M. (2003). Selective feeding of Eurytemora affinis (Copepoda, calanoida) in temperate estuaries: model and field observations. Estuarine Coast. Shelf Sci. 56, 305–311. doi: 10.1016/S0272-7714(02)00182-8
Weatherby T. M., Lenz P. H. (2000). Mechanoreceptors in calanoid copepods: designed for high sensitivity Arthropod. Struct. Dev. 29, 4, 275–288. doi: 10.1016/S1467-8039(01)00011-1
Wilkens S., Stanley J., Jeffs A. (2012). Induction of settlements in mussel (Perna canaliculus) larvae by vessel noise. Biofouling 28, 65–72. doi: 10.1080/08927014.2011.651717
Keywords: bioacoustic, zooplankton, clearance rate, growth, vessel noise emission
Citation: Aspirault A, Winkler G, Jolivet A, Audet C, Chauvaud L, Juanes F, Olivier F and Tremblay R (2023) Impact of vessel noise on feeding behavior and growth of zooplanktonic species. Front. Mar. Sci. 10:1111466. doi: 10.3389/fmars.2023.1111466
Received: 29 November 2022; Accepted: 02 May 2023;
Published: 15 May 2023.
Edited by:
Marta Solé, Universitat Politècnica de Catalunya, BarcelonaTech (UPC), SpainCopyright © 2023 Aspirault, Winkler, Jolivet, Audet, Chauvaud, Juanes, Olivier and Tremblay. This is an open-access article distributed under the terms of the Creative Commons Attribution License (CC BY). The use, distribution or reproduction in other forums is permitted, provided the original author(s) and the copyright owner(s) are credited and that the original publication in this journal is cited, in accordance with accepted academic practice. No use, distribution or reproduction is permitted which does not comply with these terms.
*Correspondence: Réjean Tremblay, UmVqZWFuX1RyZW1ibGF5QHVxYXIuY2E=