- 1IFREMER, University of Brest, CNRS, IRD, LEMAR, F-29280 Plouzané, France
- 2MARBEC Université Montpellier, CNRS, Ifremer, INRAE, IRD, Palavas-les-Flots, France
Predator-prey interactions and, especially, the success of anti-predator responses are modulated by the sensory channels of vision, olfaction, audition and mechanosensation. If climate change alters fish sensory ability to avoid predation, community dynamics can be affected. We investigated whether mid-duration exposure to warming and/or acidification alters behavioural response to visual or mechano-acoustic predator cues in juvenile Dicentrarchus labrax. We measured kinematic variables before and after a visual or a mechano-acoustic challenge which mimicked an overflying bird shadow or a bird swoop attack, respectively. Due to large interindividual variability in responses before cue presentation, fish were categorized as slow and fast to account for baseline individual variability. Treatment did not impact kinematic variables as both slow and fast fish of every treatment elicited precautionary and escape responses. Interestingly, even slow fish swam as fast as fast fish after the cue, suggesting that regardless of initial category, fish managed to escape facing a danger. Anti-predator response varied according to the level of threat to survival with greater responses elicited after the swoop attack. Although wild juvenile sea bass aggregate in schools, school dynamics rely on single leaders which highlights the importance of the variability in individual behaviours. We demonstrated that anti-predator response in juvenile D. labrax is robust to mid-duration exposure to independent and combined effects of warming and acidification. If robustness is confirmed over long-duration, it could provide D. labrax with an evolutionary advantage in the future ocean, where cue transmission through changing environments can further modulate cue perception and predator-prey interactions.
1 Introduction
Due to anthropogenic activity, atmospheric CO2 has reached a current concentration of 422 ppm (Dlugokencky and Tans, 2021), and could reach ~1000 ppm by the end of this century (IPCC, 2021). This is causing ocean warming and acidification. According to the intermediate emission scenario (SSP2-4.5), an increase between 2.1 to 3.5°C in the global sea surface temperature as well as a decrease of up to 0.3 pH units in the global sea surface pH or up to 0.4 in the North Atlantic Ocean are expected by the end of the century compared to 1850-1900 (Caldeira and Wickett, 2003; Orr et al., 2005; IPCC, 2021).
Although most marine teleosts have long been considered efficient acid-base regulators, coping with high CO2 and elevated temperature may lead to downstream physiological defects (Munday et al., 2012; Heuer and Grosell, 2014; Esbaugh, 2018; Cominassi et al., 2019; Howald et al., 2019; Cohen-Rengifo et al., 2022a) including those related to sensory behaviour in some species (Clements and Hunt, 2015; Ashur et al., 2017; Tresguerres and Hamilton, 2017; Domenici et al., 2019). Several studies identified a range of behavioural traits that were severely impacted when the sensory channels of olfaction (Dixson et al., 2010; Devine et al., 2012; Devine and Munday, 2013; Munday et al., 2016; Porteus et al., 2018; Velez et al., 2019; Roggatz et al., 2022), vision (Ferrari et al., 2012; Chung et al., 2014; Steckbauer et al., 2018), audition (Simpson et al., 2011; Bignami et al., 2013; Rossi et al., 2016; Radford et al., 2021) or mechano-audition (Allan et al., 2014) were primarily stimulated under acidification alone or in combination with warming (Watson et al., 2018). These impacted traits may have ecological implications on recruitment (Munday et al., 2010; Stiasny et al., 2016), homing (Devine et al., 2012), habitat selection (Devine and Munday, 2013), learning (Chivers et al., 2014; Paula et al., 2019a), and interspecific relationships such as mutualism (Paula et al., 2019a; Paula et al., 2019b) and avoidance of a simulated predator (Ferrari et al., 2012; Allan et al., 2014; Munday et al., 2016; Steckbauer et al., 2018; Draper and Weissburg, 2019) or a real predator (Allan et al., 2013; Allan et al., 2015; Allan et al., 2017). Deleterious responses of acidification observed on olfaction (Nilsson et al., 2012; Hamilton et al., 2014; Jiahuan et al., 2018) and vision (Chung et al., 2014) result from brain dysfunction at the level of the GABAergic system where an inversion of the Cl−/HCO3− flux occurs across neuronal membranes. Conversely, other studies stimulating the sensory channels of olfaction (Manciocco et al., 2015; Sundin and Jutfelt, 2016; Sundin et al., 2017; Clark et al., 2020a), vision (Jarrold et al., 2020) and mechano-audition (Manciocco et al., 2015; Näslund et al., 2015; Nasuchon et al., 2016; Jarrold and Munday, 2018) under acidification (Näslund et al., 2015; Sundin and Jutfelt, 2016; Sundin et al., 2017; Clark et al., 2020a; Jarrold et al., 2020) and warming (Manciocco et al., 2015) either independently or interactively (Nasuchon et al., 2016; Jarrold and Munday, 2018) revealed no impacts, minor impacts or both. This highlights the complexity of interpretation as the impacts are trait-specific, even in closely related traits such as lateralization, an indicator of brain function. For instance, in both Gobiusculus flavescens (Sundin and Jutfelt, 2018) and Ctenolabrus rupestris (Sundin and Jutfelt, 2016), relative lateralisation was impacted by acidification whereas absolute lateralisation was not. The opposite occurred in Acanthochromis polyacanthus (Jarrold and Munday, 2018). In Ctenolabrus rupestris, even if lateralisation was impacted, most activity metrics and olfactory choice metrics were not impacted by acidification (Sundin and Jutfelt, 2016). A similar pattern was observed in Gasterosteus aculeatus exposed to acidification, as altered absolute lateralization was not accompanied by altered escape response (Näslund et al., 2015). Escape response to predator olfactory cues also varied within species of the same genus and showed overall conflicting results across studies (Dixson et al., 2010; Munday et al., 2010; Clark et al., 2020a). All these results evidence an underlying variation probably related to differences in the experimental conditions, system and methodology that may partially account for conflicting results. Furthermore, this puts forward the importance of standardised methods, data transparency, replicability and reproducibility (Clark et al., 2020a; Clark et al., 2020b; Munday et al., 2020; Williamson et al., 2020).
Sensory-mediated behaviours regulate inter-specific interactions including predator-prey interactions. Particularly, the escape response is considered a fundamental trait in neurobiology because when fish are confronted with a predator cue, they are able to display a wide array of behavioural and cognitive traits including decision-making (Domenici and Hale, 2019). While temperature is the primary environmental parameter governing sensory-mediated behaviours and interactions, littles is known concerning the effects of warming alone or its interactive effects with ocean acidification, notably on temperate pelagic fish of ecological and socio-economic importance (Domenici et al., 2014; Draper and Weissburg, 2019). Yet, the study of independent and interactive effects of warming and acidification provide understanding on how stressors can operate (i.e. additively, synergistically or antagonistically) in a near-future ocean where warming and acidification are expected to act simultaneously (Vinebrooke R et al., 2004; Nowicki et al., 2012; Pimentel et al., 2016; Allan et al., 2017; Domenici and Hale, 2019).
The European sea bass (Dicentrarchus labrax) is a large pelagic predatory fish of ecological relevance and has an established aquaculture value and a developing capture value in the north-eastern Atlantic Ocean (Vandeputte et al., 2019; FAO Yearbook, 2021). It is found in highly hydrodynamic habitats from Morocco though the Mediterranean and the Northeast Atlantic up to Norway (Jennings and Pawson, 1992). D. labrax juveniles inhabit nursery areas for their first four to five years (Jennings and Pawson, 1992). Habitat connectivity and complexity and high food availability make these areas ideal for growth and recruitment (Pawson et al., 2007; Sheaves et al., 2015) even if piscivorous fish and birds exert a certain degree of predation pressure (Baker and Sheaves, 2006; Sheaves et al., 2015). Juveniles must be able to recognize predators using their sensory systems in order to survive since overflying birds can suddenly undertake a swoop attack. For example, Gasterosteus aculeatus dart away and abruptly remain still to avoid being seen by an avian predator; a response that is believed to be innate, as it was observed in predator-naïve laboratory fish (Giles, 1984).
If warming and acidification affect the sensory ability of juvenile fish to detect a predator, the risk of being predated might be higher and consequently, the movement of fish from nursery areas to adjacent adult habitats may be reduced. Therefore, community-level impacts might be expected with carry over socio-economic implications. Understanding how warming and acidification may impact sensory behaviour-mediated predator-prey interactions is therefore of utmost importance. The aim of this study was to evaluate whether exposing juvenile D. labrax to independent and interactive warming and acidification for a mid-duration exposure (60-92 days) could alter their sensory behaviour, which is a mediator of predator detection and escape response. The sensory channels of vision and mechano-audition were assessed in separate behavioural tests, and for the first time both were evaluated under independent and combined warming and acidification. We used automated video tracking to calculate kinematic swimming variables and evaluate escape response.
2 Materials and methods
2.1 Husbandry
Fish husbandry and experiments were conducted at the PHYTNESS unit of IFREMER-Plouzané. Experiments were authorised (APAFIS#10745) by the ethics committee agreement and the Ministry of Higher Education and Research. Procedures involving animals followed the ethical standards of the institution and Directive 2010/63/EU recommendations and complies with ARRIVE guidelines.
In September 2020, D. labrax juveniles (208 dph, 8.6 ± 3.4 cm total body length) were transported from their hatchery at IFREMER Palavas-les-Flots to IFREMER Plouzané where they were held for 12 days in a 4000 L tank. On day 13, fish were evenly distributed into 12 flow-through tanks (1000 L, n= 64-72 fish per tank). pHT (total scale) in these tanks was already set either at pHT7.9 (control) or at pHT7.5 (-0.4 pH units, SSP2-4.5 (IPCC, 2014; IPCC, 2021)), whereas temperature was either maintained at natural seasonal values or was increased 1°C per day over three days to reach +3°C (SSP2-4.5). At day 16, four treatments were achieved: a control treatment (CT: seasonal temperature and pHT7.9), a warmed treatment (WT: +3°C and pHT7.9), an acidified treatment (AT: seasonal temperature and -0.4 pH units) and a warmed and acidified treatment (WAT: +3°C and -0.4 pH units). Each treatment was triplicated in one of the twelve 1000 L tanks. Fish were reared under these conditions for up to 92 days and were fed ad libitum with Le Gouessant (France) pellets of different size to match fish size.
Seawater was pumped at 20 m depth 500 m off the Dellec beach (Plouzané), passed through a sand filter (Daqua H4015101101506, France) and stored in a non-heated header tank from which it was delivered into three CT replica-tanks at a rate of 35 ± 5 L min-1. For the other treatments, seawater was warmed and/or acidified (Figure S1) before being delivered into 3 replica-tanks for each treatment. Seawater was warmed using a plate heat exchanger (Vicarb N° V4, France) and a universal controller (Landis & Staefa POLYGYR RWX62.7032, Switzerland). Seawater was acidified using a CO2 bottle (AirLiquide, France) plugged with a single flow tube rotameter (model P, Aalborg, USA) allowing constant CO2 injection (30 ± 6 ml min-1) into two mixing tanks. Both CO2 and seawater entered each mixing tank from the top and flowed down through a spiral column (JBL Proflora Taifun Spirale 10, Germany) which breaks CO2 into smaller bubbles and favours CO2 mixing efficiency.
Temperature and pH were measured daily in the rearing tanks with a pH meter (Multi3420, WTW, Germany) equipped with a SenTix940 pH electrode (WTW, Germany) which was calibrated with pH4.0 and pH7.0 buffers (WTW, Germany). Salinity and oxygen concentration were measured weekly with a LF340 salinity meter and Oxi340 (WTW, Germany), respectively. Total alkalinity (TA) was calculated based on weekly manual titrations of seawater following the adapted protocol of Strickland and Parsons (1972): a sample of 50 ml of seawater was mixed with 15 ml of 0.01M HCl; pH and temperature were measured before and after the HCl addition. TA (mol l−1) was calculated according to the following formula:
where V is the volume (l) and C the concentration (mol l−1) of either HCl or the sample, H+ is the hydrogen activity (10−pH) and УH+ is the hydrogen activity coefficient (here = 0.758). The software CO2SYS v2.1 together with constants from Mehrbach et al. refitted by Dickson and Millero (Mehrbach et al., 1973; Dickson and Millero, 1987; Lewis and Wallace, 2006) were used to calculate the concentration of the carbonate system components, as well as to calculate pHT based on measured pHNIST (National Institute of Standards and Technology scale) and pCO2. Photoperiod was progressively modified from 12hD:12hN in September to 8hD:16hN in December to follow a natural seasonal shift.
2.2 Behavioural setup
Two behavioural tests, either visual or mechano-acoustic, were performed separately in the same setup (Figure 1). The tests were performed in three arenas (24 L, 88x18x15 cm) with white non reflective walls and transparent bottoms. Above the arenas were placed 2 LED spots, a fisheye camera with an infrared filter (DMK31AU03, IR Filter 092, TheImagingSource, Germany) connected to a computer for video acquisitions (software IC Capture, v2.5.1525.3931) and an electromagnet device (Millot et al., 2009; Näslund et al., 2015; Munday et al., 2016). This device was used only for the mechano-acoustic tests and was composed of three magnets (EM 25 12V RS PRO, France), each holding a 50 mL Falcon tube filled of sand which represented a simplified bird model. An infrared floor (1 m2, Noldus, The Netherlands) was placed below the arenas to guarantee homogeneous visibility during recording. White frames surrounded the arenas to avoid fish stress during trials.
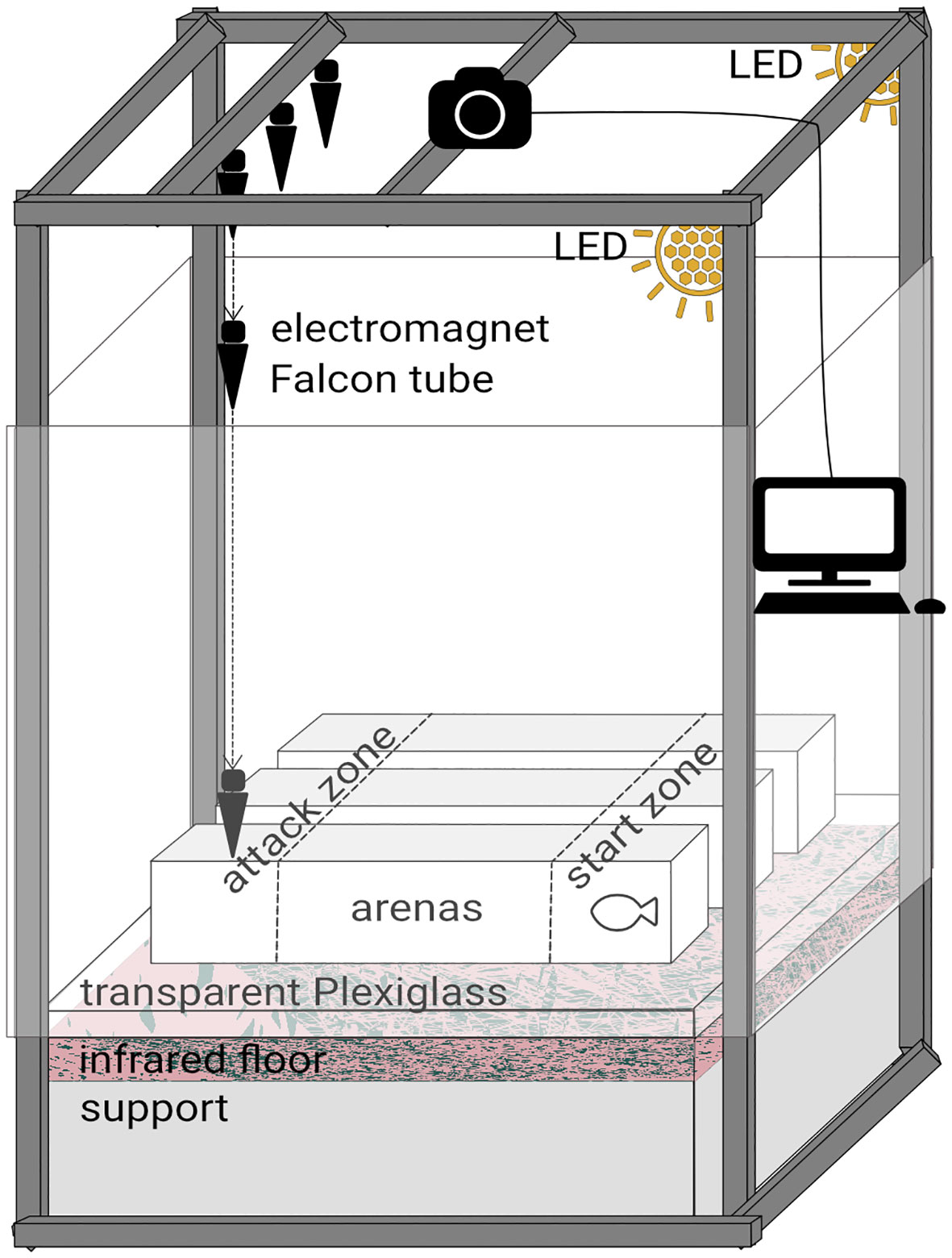
Figure 1 Setup for both visual and mechano-acoustic behavioural tests. The LED spots were kept for both tests but were turned off 30 s only for the visual tests. The electromagnet device was used only for the mechano-acoustic tests.
Behavioural rounds (BR) were conceived to use the same fish for both behavioural tests (i.e. fish were always naive to the test). A BR comprises one day dedicated to conduct the visual tests and the other day to conduct the mechano-acoustic tests (Figure 2). The choice of which behavioural test was run each day was random for each BR and was statistically included in our models as the factor floating cage (see section 2.4). After each fish’s first trial, they recovered one night in a floating cage back in their home tanks in order to be used the next day. After a fish’s second trial, fish were euthanized with an overdose of 2-Methoxy-4-(prop-2-en-1-yl) phenol (0.45 ml L-1; Fili@Vet Reseau Cristal, France). Each day was divided in three sessions and each session included four trials (12 trials per day). Because of technical constraints, only 3 arenas were used to receive three out of four treatments per trial, so four trials per session were needed to cover the four treatments along the 12 trials. A total of 36 fish were challenged per day (Figure 2).
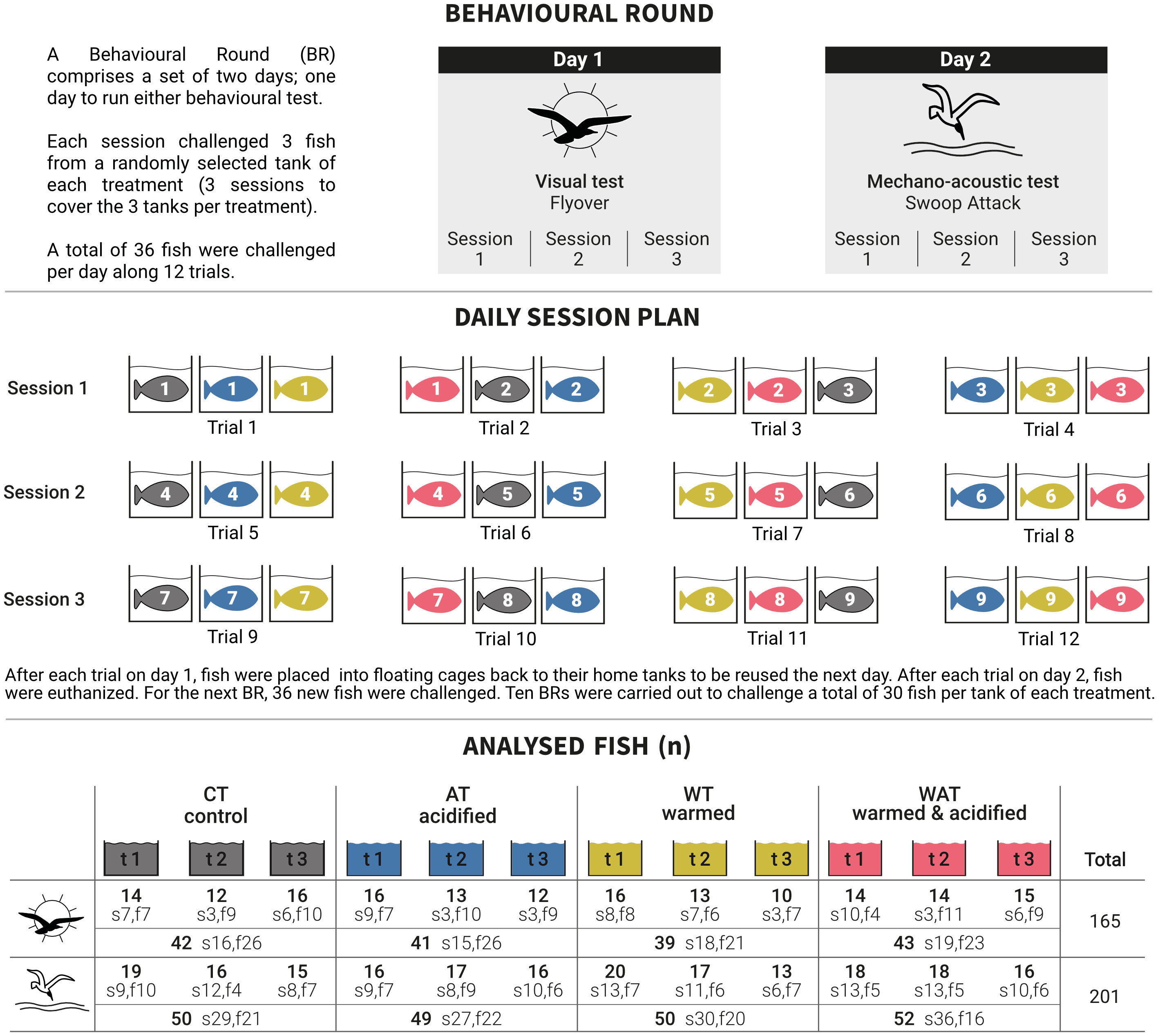
Figure 2 Experimental design arranged in “behavioural rounds” (BR). A BR comprises 2 working days and each day was segmented in 3 sessions. Due to technical and tracking issues the number of effective analysed fish (n) per tank (t) was lower than the number of challenged fish. Due to large interindividual variability fish were categorized as slow (s) and fast (f), for which n is also shown.
Per session, three fish per tank of each treatment were captured at once instead of one at the time in order to reduce fish stress due to constant fishing. The three fish were haphazardly captured from a randomly selected tank and placed in 20 L buckets (one per treatment) in a calm and lighted environment in the behavioural room. Fish remained in the buckets for the whole session (2.5 h) while waiting to be challenged. Because the first challenged fish waited only 0.5 h and the last challenged fish waited 2.5 h, the difference in acclimation duration in the bucket was accounted statistically as the categorical factor bucket acclimation (see section 2.4). Bucket seawater was aerated and replaced after every session. Arenas were filled with treatment seawater, which was randomly assigned. Each fish was gently transferred to its corresponding arena and allowed to recover from transfer for 13 min. Trials were recorded and were composed of the following periods: 2 min before the cue presentation (pre), 30 sec of visual cue (dark), and 2 min after the cue presentation (post). The visual cue consisted in turning the LED spots off during 30 s to mimic the shadow of an overflying bird. The mechano-acoustic cue consisted in turning the electromagnet off, letting the bird model fall into each arena (Schneider et al., 2012) to mimic a bird’s swoop attack. The Falcon tubes were moored to the device with a nylon thread, so that they remained plunged 5 cm below the water surface until the end of the trial, as we thought removal of the object would have added another level of stress. At the end of each trial, the camera was turned off and arena’s water was renewed.
A BR was repeated 10 times, allowing us to test n = 30 fish per tank of each treatment for each behavioural test (360 in total). Behavioural tests were conducted over 32 days, starting on day 60 (283 days post-hatching) and ending on day 92 (315 days post-hatching) of exposure to warming and/or acidification. On day 2 of each BR, total body length (cm) was measured on euthanized fish from nose tip to end of caudal fin.
2.3 Video analysis
Video analysis was performed using the software EthoVision®XT (v.15.0.1418, The Netherlands (Noldus. EthoVision, 2021)) which was set to detect a single fish and to track its position at a frame rate of 15 images s-1. If fish detection was between 85-95%, a person who was blind with respect to the treatments, visualised the videos and manually corrected tracking errors resulting from differences in contrast between the fish and the background. When a technical issue (fish being stuck in the net, falcon rebounding into another arena, camera or computer bug) or human error (wrong assignment of fish into the correct treatment, late/early presentation of the cue) occurred during a trial, or when fish detection was lower than 85%, the trial was dismissed. Thus, among the 360 challenged fish, videos of 165 fish from the visual tests and of 201 fish from the mechano-acoustic tests were used for tracking analysis. The effective number of fish analysed per tank is available in Figure 2.
To assess escape response, the following kinematic variables were calculated: cumulative distance travelled (Dtot) measured during the whole trial duration per treatment and mean swimming velocity (Vmean) measured per period and treatment. Dtot and Vmean were standardised by total body length and are expressed in body lengths units (bl) and in bl s-1, respectively.
2.4 Statistics
We used the software R-4.0.5 (R Core Team, 2015) and the packages car, matrix and lme4 to build generalized linear mixed-effects models with the function glmer, the package dplyr to plot residuals panels and the package ggplot2 for plots. For each model, several model variants were run using every possible combination of additive and interactive effects of the predictor factors. To calculate the deviance table, a type II Wald Chi Square test was performed with the function Anova. Tukey post hoc tests were performed with the function emmeans. Model diagnosis was carried out by comparing the model variants according to the AIC and BIC values, the residuals Vs. fitted plots (to verify residual’s linarity), and the QQ-plots and histograms (to residual’s normality) (Zuur et al., 2009). A model was selected if it showed a low BIC and if residuals were as linear and normal as possible.
To understand, from an overall perspective, how fish responded to both cues, Dtot was analysed along the whole trial duration. A first model sought to determine if Dtot was modulated by the fixed factors round (accounting for the differences in exposure duration or age between the beginning and the end of the behavioural tests on day 60 and day 92) and treatment, considering the following random factors: floating cage (accounting for the order of trial in a BR; 2 categorical levels), bucket acclimation (accounting for different acclimation duration in the buckets before a trial; 5 categorical levels), and tank (replica) nested in treatment.
To compare fish anti-predator response, Vmean was analysed before and after the predator cues. However, extensive interindividual variation in swimming speed (before presenting the cue) prevented us from fitting models to our data, therefore, we created an additional categorical factor rapidity to account for baseline variation in individual swimming speed. Our assumption was that initial individual response to transfer in the arena will modulate individual response to the predator cues. Rapidity ponders individual Vmean before cue presentation, and categorizes fish in two levels: slow (Vmean < 0.04 bl cm-1) and fast (Vmean ≥ 0.04 bl cm-1). The threshold of 0.04 bl cm-1 was set arbitrarily based on movement observations. Thus, a second model sought to determine if Vmean was modulated by the fixed factors round, treatment, period and rapidity considering the following random factors: floating cage, bucket acclimation, the repeated-measures factor id (individual fish) repeated in rapidity nested in period nested in tank. It is worth noting that because fish were categorized along rapidity, we expected to find differences between slow and fast fish in the pre-cue period, but this should not be considered an experimental result.
3 Results
3.1 Rearing
Fish were reared under stable physico-chemical seawater conditions (Table 1). The mean difference (± standard deviation (sd)) in temperature between the current (CT, AT) and the high temperature (WT, WAT) treatments was 3.98 ± 0.69°C, while the mean difference in pHT between the current (CT, WT) and the low pH (AT, WAT) treatments was 0.40 ± 0.04. Raw datasets including for the seawater parameters are available in the SEANOE public repository (Cohen-Rengifo et al., 2022b).
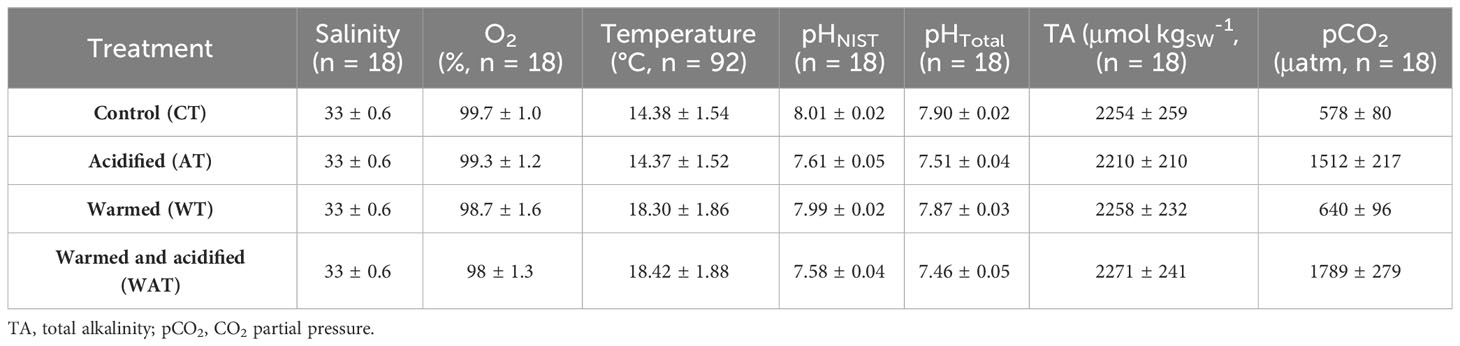
Table 1 Seawater parameters (mean ± sd) averaged daily (n = 92) or weekly (n = 18), according to treatment.
3.2 Body length
Mean body length ± sd of fish for which videos were analysed for the visual tests was 11.1 ± 1.0 cm in CT (42 fish), 11.7 ± 1.2 cm in AT (41 fish), 12.7 ± 1.1 cm in WT (39 fish) and 13.1 ± 1.3 cm in WAT (43 fish). Minimal (8.8 cm) and maximal (16 cm) body length were observed in AT. These values slightly changed for those videos analysed for the mechano-acoustic tests as sample size was higher (Table S1).
3.3 Cumulative distance travelled (Dtot)
Overall, we observed a large variability of responses to both visual and mechano-acoustic cues (i.e. some fish moved a lot, others moved very little and others did not move at all). Figure 3 shows a greater density of observation around 25 bl. The proportion of CT fish travelling more than 25 bl was higher during the mechano-acoustic tests (Mat: 72%) than the visual tests (Vit: 55%) and this was also valid for the other treatments (Table S1). Likewise, fish travelled longer distances in Mat than Vit in CT (mean ± standard error (se): DtotMat = 52.4 ± 5.7 bl s-1; DtotVit = 34.5 ± 4.5 bl s-1) and AT (DtotMat = 50.9 ± 5.6 bl s-1; DtotVit = 41.4 ± 4.6 bl s-1) but not in WT (DtotMat = 45.8 ± 4.1 bl s-1; DtotVit = 43.9 ± 5.2 bl s-1) and WAT (DtotMat = 39.4 ± 4 bl s-1; DtotVit = 38.2 ± 4 bl s-1) (Figure 3, Table S1).
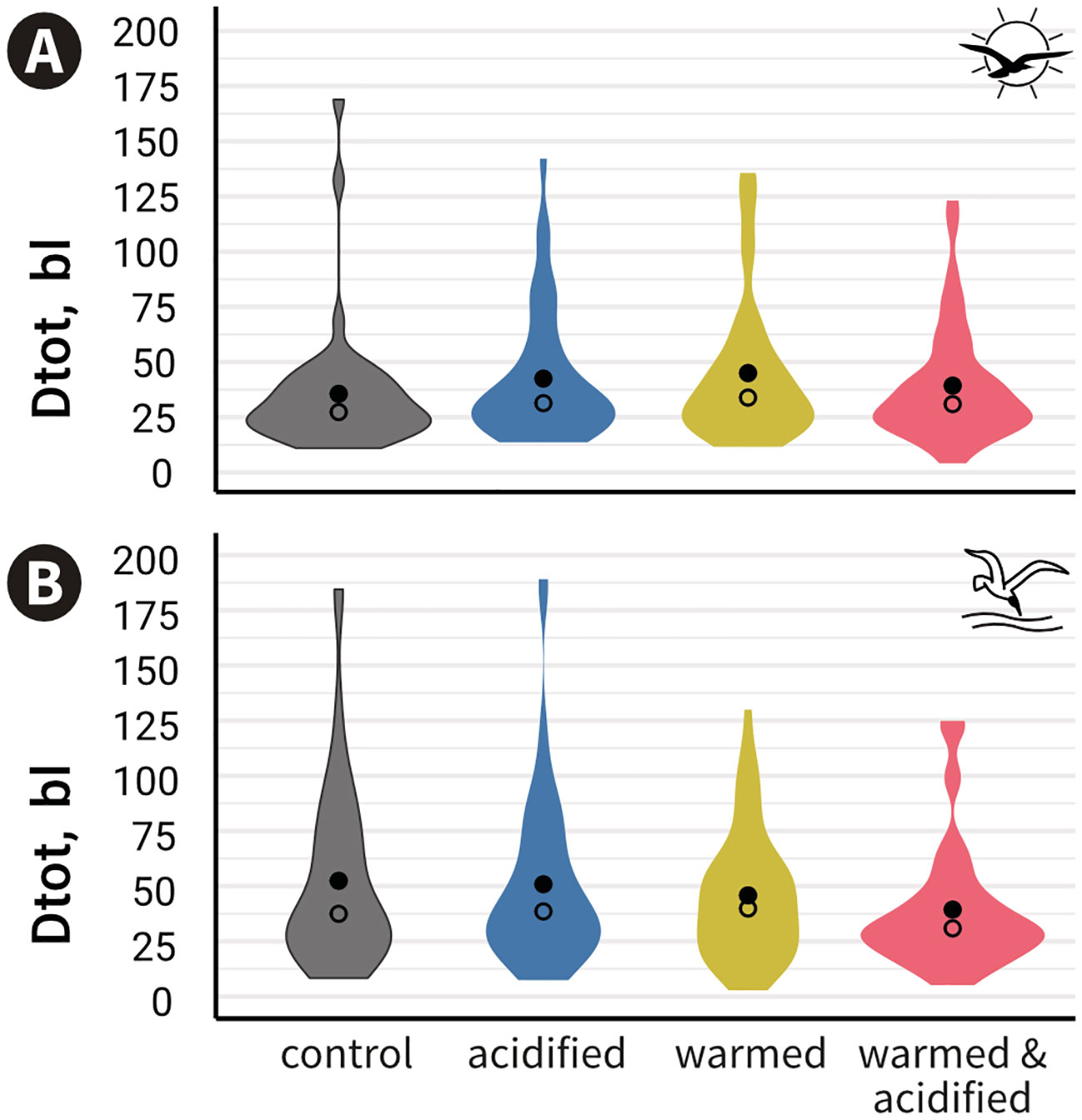
Figure 3 Cumulated distance travelled standardised by individual total body length (Dtot) measured during the whole trial duration per treatment (mean Dtot ± standard error are shown) for the visual (A) or the mechano-acoustic (B) behavioural tests. •: means; ○: medians; No significant differences between treatments were found.
Fish travelled the shortest distance in CT and the longest in WT during Vit, whereas fish travelled the longest distance in CT and the shortest in WAT during Mat (Figure 3). However, according to the first model, Dtot was not modulated by treatment (p = 0.555) or round (p = 0.058) in Vit, whereas in Mat, Dtot was not modulated by treatment (p = 0.084) but by the interaction round:treatment (p = 0.013), though no comparatively relevant contrasts were significantly different. Comparatively relevant refers to different Dtot between rounds within either level of treatment. Model outcomes and residual panels for Vit and Mat are available in Table S2 and Figure S2.
3.4 Mean swimming velocity (Vmean)
According to the second model for both behavioural tests, Vmean was not modulated by treatment alone or in interaction with another factor, but by the interactions round:period (pVit ≤ 0.001; pMat = 0.010), round:rapidity (pVit = 0.017) and period:rapidity (pVit&Mat ≤ 0.001) (Table S3, Figure S3).
For Vit, the interaction round:rapidity showed no comparatively relevant contrasts that were significantly different. The interaction round:period, showed that Vmean was marginally different in the post-cue period between round5 and round8 (p = 0.049). The interaction period:rapidity revealed that for every treatment fast fish swam faster than slow fish in the pre-cue period (p ≤ 0.001; mean ± se: example for CT Vmeanslow = 0.04 ± 0.004 bl s-1; Vmeanfast = 0.14 ± 0.01 bl s-1) but not in the dark- (Vmeanslow = 0.13 ± 0.02 bl s-1; Vmeanfast = 0.16 ± 0.04 bl s-1) and post-cue periods (Vmeanslow = 0.15 ± 0.02 bl s-1; Vmeanfast = 0.14 ± 0.03 bl s-1). Slow fish swam faster in the dark- and post-cue periods than in the pre-cue period, but they did not change their swimming between the dark- and post-cue periods; fast fish swam similarly across periods (Figure 4A, Table S4).
For Mat, the interaction round:period (p ≤ 0.001) showed no comparatively relevant contrasts (Table S3). The interaction period:rapidity revealed that for every treatment fast fish swam faster than slow fish in both periods (p ≤ 0.001; mean ± se: example for CT Vmeanslow-pre = 0.03 ± 0.003 bl s-1; Vmeanfast-pre = 0.22 ± 0.04 bl s-1; Vmeanslow-post = 0.27 ± 0.04 bl s-1; Vmeanfast-post = 0.43 ± 0.05 bl s-1) and that slow and fast fish swam faster in the post-cue period than in the pre-cue period (Figure 4B; Table S4).
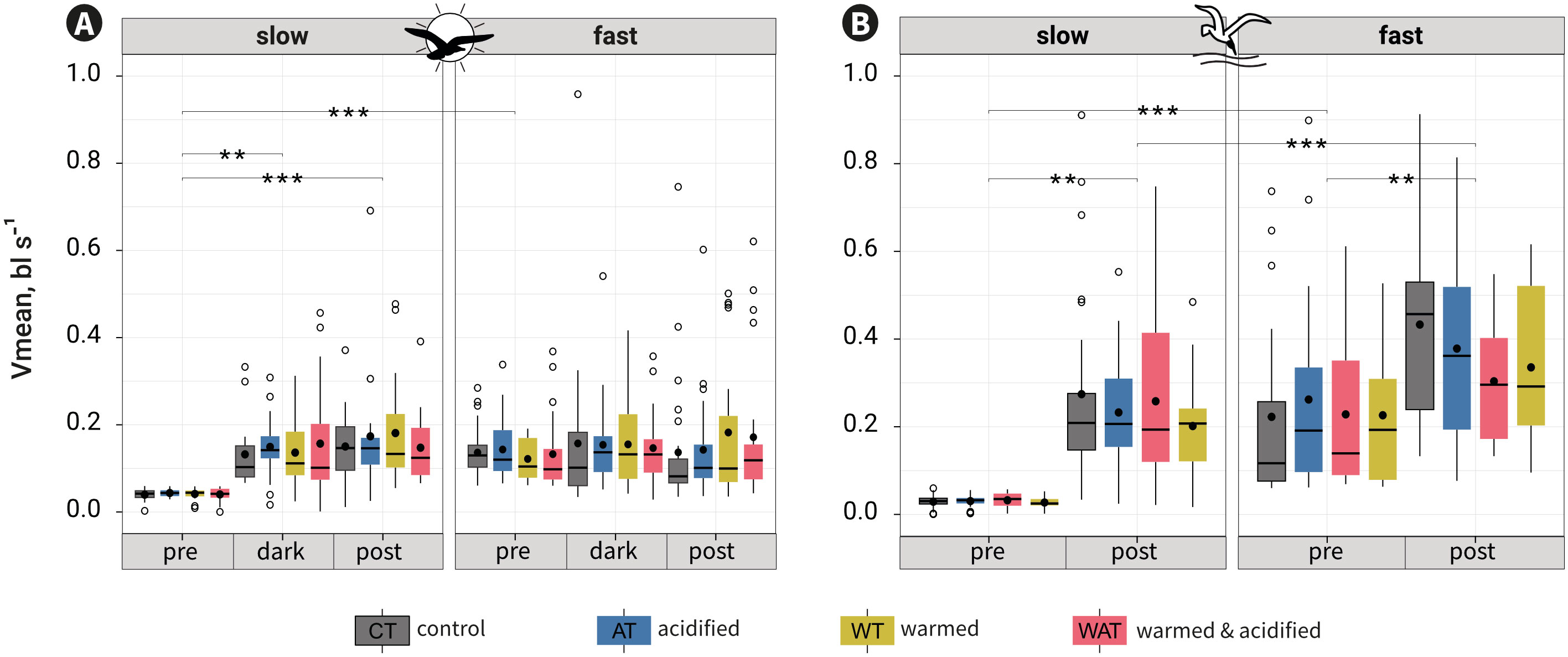
Figure 4 Mean swimming velocity (Vmean) per category of rapidity, period and treatment for the visual (A) or the mechano-acoustic (B) tests. •: means; ▬: medians. Tukey contrasts for the interaction period:rapidity are shown as: ** p-value = 0.001-0.01; ***: p-value ≤ 0.001. Contrasts shown between slow and fast fish in the pre-cue period reflect the division of fish along rapidity and not an experimental result.
4 Discussion
The present study highlights a robust perception and escape response to independent and combined effects of warming and acidification in Dicentrarchus labrax in the face of danger, such as that mimicked by either the shadow of an overflying bird (visual cue) or the attack of a swooping bird (mechano-acoustic cue). Behavioural resilience to OA has been previously observed in ~60 days old juveniles D. labrax (Duteil et al., 2016) and the present study extends escape response resilience to late juveniles of 9-10 months. Our study also highlights a large variability in individual responses observed before and after either cue.
Some of the observed variability may arise from technical variability (Hecksteden et al., 2015) such as the differences in acclimation time in the bucket, fish being familiar with the arena upon their second challenge, different exposure duration to stressors or short acclimation period in the arenas. Regarding acclimation duration in D. labrax of similar body lengths when exposed to a mechano-acoustic challenge, it seems that if we had granted a longer acclimation duration before the trials, variability would not necessarily have been reduced. Indeed, 15 min acclimation was accompanied by 75 to 90% of reactivity (Benhaïm et al., 2012) whereas 2 h acclimation was accompanied by 50% of reactivity (Millot et al., 2009). In addition, although we conducted the experiment under apparently similar conditions, the environment of the experimental infrastructure changes every day, and it was technically difficult to identified every source of variability. We should therefore not neglect the importance of unidentified background noise as this can modulate fish environment and translate into differences in fish behaviour on a daily basis. Another portion of the observed variability can be explained by intra- or interindividual variability (Hecksteden et al., 2015) whether it is induced by the environmental and technical parameters or by genetic endowment. Within-subject variability manifested as single fish showing different activities that ranged from immobility, sustained swimming to erratic fast one-off movements. These activities varied in frequency, duration and layout between fish on a daily basis and likely contribute to greater between-subject variability.
We included in our model both technical variability as the random factors bucket acclimation and floating cage, and interindividual variability as the categorical factor rapidity, assuming that pre-cue swimming velocity will modulate post-cue swimming velocity. Contrary to our assumption, even slow fish were able to swim as fast as fast fish after either cue, suggesting that regardless of initial responsiveness, fish were able to escape from dangerous situations where their survival could be threatened. Although rapidity improved model fit, there is yet a part of the variance that is not fully explained by our models, and together with the large behavioural variability, perhaps precluded statistical identification of a potential impact of treatment. Indeed, round or treatment had no statistical impact on the cumulative distance travelled or the mean swimming velocity indicating that differences in 32-day exposure to warming and/or ocean acidification had no impact on the escape response to simulated predator cues in juvenile D. labrax.
Concerning the visual test, swimming velocity was significantly different during than before the cue, indicating that the shadow elicited a response. In addition, swimming velocity did not vary between the dark and post-cue periods denoting a sustained shadow-induced response that can last a few minutes after the danger has passed. Rather than a strong escape response, the shadow cue elicited the maintenance of a precautionary response where fish swam conservatively faster, regardless of rapidity and treatment. The few studies addressing the response to a visual cue under acidification revealed negative impacts. In early juvenile Pomacentrus amboinensis, anti-predator response was reduced after 6 days of exposure (Ferrari et al., 2012), while in juvenile Acanthochromis polyancanthus retinal flickering was reduced after 7-day exposure (Chung et al., 2014), though it is unknown if this can alter fish ability to react to visual cues. On the other hand, the only study addressing both warming and acidification found no impact on lateralization and visual response in early juvenile Seriola lalandi, though vision was only tested under acidification because fish exposed to warming were too fast (Jarrold et al., 2020).
Concerning the mechano-acoustic test, swimming velocity significantly increased after the swoop attack in fish of every treatment. After the cue, slow fish swam slower that fast fish, yet their swimming velocities were still highly indicative of a strong escape response. However, it is necessary to experimentally validate whether the lower swimming velocity of slow fish leads to reduced escape success. Our results suggest a robust response to the predator swoop attack under warming and acidification. This is consistent with related work in which late juvenile D. labrax exposed 21 days to +4°C (Manciocco et al., 2015) and adult Gasterosteus aculeatus exposed 20 days to acidification (Näslund et al., 2015) showed unaltered overall anti-predator response. However, signs of neuronal dysfunction were found in both species and a longer latency to escape was observed in D. labrax. Compared to these studies, the absence of effect we observed might partially be explained by a longer exposure duration to both stressors that might allow our fish to acclimate over 3 months. In adult Engraulis japonicus, 1-month exposure to both warming and acidification, did not impact a range of escape response traits (Nasuchon et al., 2016). However, longer transgenerational exposure to acidification in the coral reef species Amphiprion melanopus (15 dph) restore only a few escape response traits that were impacted by acidification (Allan et al., 2014). Interestingly, multi-stressor experiments performed on early life stages revealed that a range of escape traits are more impacted by warming than by acidification in 25-dph Seriola lalandi (Watson et al., 2018) and 28-49-dph Acanthochromis polyacanthus (Jarrold and Munday, 2018). On the other hand, the auditory sensory channel alone, is also sensitive in early life stages exposed to acidification, as observed in 17-20-dph Amphiprion percula that did not avoid recordings of daytime predator-rich reef noise (Simpson et al., 2011) and in 42-dph Chrysophyrs auratus that showed diminished hearing sensitivity (Radford et al., 2021).
Escape response is known to vary according to the perceived level of threat (Domenici and Batty, 1997; Domenici and Hale, 2019). We observed that perception of the predator cue elicited different escape responses in fish according to the sensory channel stimulated. Stimulation of the mechano-acoustic sensory channel led to exacerbated distance travelled and swimming velocity, indicating a stronger escape behaviour after the swoop attack than after the shadow. Likewise, the proportion of CT fish travelling distances greater than 25 bl was higher in the mechano-acoustic tests (72%), than in the visual tests (55%), and this was also valid in the other treatments (Table S1). This probably reflects a higher sensitivity of the mechano-acoustic sensory channel related to the higher threat to survival of a direct bird attack compared to that of an overflying bird shadow. Also, fish exposed to the visual cue probably slowed down to reduce the chances of being perceived by a bird, a behaviour observed in both wild and captive three-spined sticklebacks (Giles, 1984). Furthermore, the mechano-acoustic test stimulated simultaneously three sensory channels: mechanosensation and audition, but also vision, and fish responses were likely stronger than if the sensory channels had been stimulated separately. It was not intended to separate these sensory channels, since during a swoop attack, fish are able to hear the bird hitting the water, feel the vibrations of the hit, and see the bird in the water. Besides, it is technically difficult to evaluate separately mechanosensation or audition, because sound, in addition to being detected by the inner ears, is also detected by the same organ that detects vibrations, the lateral line (Braun and Sand, 2014).
In the wild, D. labrax juveniles aggregate in schools; a behaviour known to benefit anti-predator performance in other species, and which is further enhanced by the level of social recognition between members of a school (Domenici and Batty, 1997; Nadler et al., 2021). Although escape response varied whether measured in a single-fish or group situation (Domenici and Batty, 1997; Domenici and Hale, 2019), our single-fish approach puts forward the individual responsiveness to a danger which is ecologically relevant for schooling dynamics. Indeed, anti-predator responses of the entire school is governed by individual responses of the leader fish that initiates the escape (Marras and Domenici, 2013). Individual responses are therefore critical for the success of anti-predator response and consequently for survival and fitness (Fuiman et al., 2006). Thus, the variation of responses between individuals is crucial to understand different ways of responding to a predator and drives natural selection and population persistence across generations.
Predator detection in the wild is mediated by the use of multiple senses, so that if a given sensory channel is compromised by an environmental stressor, another less sensitive sensory channel can mitigate negative effects through trade-offs or sensory redundancy (Lönnstedt et al., 2013). However, in experiments in which all the sensory channels of both predatory fish (Pseudochromis fuscus) and prey (Pomacentrus wardi) were stimulated simultaneously under warming and acidification (Allan et al., 2015; Allan et al., 2017), a range of kinematic escape traits were impacted in a greater way by warming than by acidification, suggesting that P. wardi was not able to compensate (Allan et al., 2017). These experiments highlight that predator’s behaviour may also be impacted by climate change which can regulate the success of prey escape response in complex ways. Even if warming and acidification did not directly impact escape response in juvenile D. labrax after stimulation of the visual and mechano-auditory sensory channels, it in unknown how D. labrax would react in a real-predator situation where all the senses are stimulated simultaneously, and if predators are also exposed to the stressors.
As for the near-future ocean, facilitation of cue transmission through the natural environment, also depends on the quality of the transmission medium in which predator-prey sensory processes operate. For example, warming-induced algal blooms can reduce prey visual cues through increased turbidity that impeds predation (Strod et al., 2008), while habitat fragmentation may enhance the transduction of visual cues and facilitates predation (McCormick and Löonnstedt, 2013). The impacts of warming and acidification on the sensory transduction pathway including cue emission, perception and processing by fish may be modulated by the interaction with other natural or anthropogenic factors as well as by the organism adaptive response after transgenerational acclimation (Allan et al., 2014). Whether this may promote resiliency or plasticity of predator-prey interactions, is still an open question that deserves ideally head-to-head predator-prey challenges in a climate change mesocosm using both single-fish and school approach.
Data availability statement
The datasets generated and analysed in this article are available in the SEANOE public repository https://doi.org/10.17882/87244.
Ethics statement
The animal study was approved by Commission nationale pour la protection des animaux utilisés à des fins scientifiques (CNEA). The study was conducted in accordance with the local legislation and institutional requirements (authorization number APAFIS#10745).
Author contributions
All authors conceived the scientific questions. MC-R & M-LB designed the behavioural experiments. MC-R conditioned fish to warming and acidification, performed the behavioural tests, analysed the videos and conceived and run the statistical models. M-LB & DM provided valuable advice and supervision. All authors contributed significantly to the revision of the manuscript and approved the submitted version.
Funding
This project was supported by IFREMER and the Regional Council of Brittany (SAD n°1540).
Acknowledgments
We thank the PHYTNESS unit: to the whole fish team and the mollusc team members Philippe Miner, Pierrick Le Souchou, Christophe Lebigre. Thanks to Sébastien Hervé from LEMAR, the intern Laura Buchet and the IFREMER members Xavier Cousin, Cyril Noël, Aurélien Lledo and Jean-Pierre Lafontaine.
Conflict of interest
The authors declare that the research was conducted in the absence of any commercial or financial relationships that could be construed as a potential conflict of interest.
Publisher’s note
All claims expressed in this article are solely those of the authors and do not necessarily represent those of their affiliated organizations, or those of the publisher, the editors and the reviewers. Any product that may be evaluated in this article, or claim that may be made by its manufacturer, is not guaranteed or endorsed by the publisher.
Supplementary material
The Supplementary Material for this article can be found online at: https://www.frontiersin.org/articles/10.3389/fmars.2023.1108968/full#supplementary-material
References
Allan B. J. M., Domenici P., McCormick M. I., Watson S.-A., Munday P. L. (2013). Elevated CO2 affects predator-prey interactions through altered performance. PloS One 8, e58520. doi: 10.1371/journal.pone.0058520
Allan B. J. M., Domenici P., Munday P. L., McCormick M. I. (2015). Feeling the heat: the effect of acute temperature changes on predator–prey interactions in coral reef fish. Conserv. Physiol. 3, cov011. doi: 10.1093/conphys/cov011
Allan B. J. M., Domenici P., Watson S. A., Munday P. L., McCormick M. I. (2017). Warming has a greater effect than elevated CO2 on predator–prey interactions in coral reef fish. Proc. R. Soc. B.: Biol. Sci. 284, 1–9. doi: 10.1098/rspb.2017.0784
Allan B. J. M., Miller G. M., McCormick M. I., Domenici P., Munday P. L. (2014). Parental effects improve escape performance of juvenile reef fish in a high-CO 2 world. Proc. R. Soc. B. 281, 20132179. doi: 10.1098/rspb.2013.2179
Ashur M. M., Johnston N. K., Dixson D. L. (2017). Impacts of ocean acidification on sensory function in marine organisms. Integr. Comp. Biol. 57, 63–80. doi: 10.1093/icb/icx010
Baker R., Sheaves M. (2006). Visual surveys reveal high densities of large piscivores in shallow estuarine nurseries. Mar. Ecol. Prog. Ser. 323, 75–82. doi: 10.3354/meps323075
Benhaïm D., Péan S., Lucas G., Blanc N., Chatain B., Bégout M.-L. (2012). Early life behavioural differences in wild caught and domesticated sea bass (Dicentrarchus labrax). Appl. Anim. Behav. Sci. 141, 79–90. doi: 10.1016/j.applanim.2012.07.002
Bignami S., Enochs I. C., Manzello D. P., Sponaugle S., Cowen R. K. (2013). Ocean acidification alters the otoliths of a pantropical fish species with implications for sensory function. Proc. Natl. Acad. Sci. 110, 7366–7370. doi: 10.1073/pnas.1301365110
Braun C. B., Sand O. (2014). ““Functional Overlap and Nonoverlap Between Lateral Line and Auditory Systems.,”,” in The Lateral Line System. Springer Handbook of Auditory Research. Eds. Coombs S., Bleckmann H., Fay R. R., Popper A. N. (New York, NY: Springer), 281–312. doi: 10.1007/2506_2013_19
Caldeira K., Wickett M. E. (2003). Anthropogenic carbon and ocean pH. Nature 425, 365–365. doi: 10.1038/425365a
Chivers D. P., Mccormick M. I., Nilsson G. E., Munday P. L., Watson S. A., Meekan M. G., et al. (2014). Impaired learning of predators and lower prey survival under elevated CO2: A consequence of neurotransmitter interference. Global Change Biol. 20, 515–522. doi: 10.1111/gcb.12291
Chung W.-S., Marshall N. J., Watson S.-A., Munday P. L., Nilsson G. E. (2014). Ocean acidification slows retinal function in a damselfish through interference with GABAA receptors. J. Exp. Biol. 217, 323–326. doi: 10.1242/jeb.092478
Clark T. D., Raby G. D., Roche D. G., Binning S. A., Speers-Roesch B., Jutfelt F., et al. (2020a). Ocean acidification does not impair the behaviour of coral reef fishes. Nature 577, 370–375. doi: 10.1038/s41586-019-1903-y
Clark T. D., Raby G. D., Roche D. G., Binning S. A., Speers-Roesch B., Jutfelt F., et al. (2020b). Reply to: Methods matter in repeating ocean acidification studies. Nature 586, E25–E27. doi: 10.1038/s41586-020-2804-9
Clements J. C., Hunt H. L. (2015). Marine animal behaviour in a high CO2 ocean. Mar. Ecol. Prog. Ser. 536, 259–279. doi: 10.3354/meps11426
Cohen-Rengifo M., Danion M., Gonzalez A.-A., Bégout M.-L., Madec L., Cormier A., et al. (2022a). The extensive intergenerational molecular effects of ocean acidification on the olfactory epithelium transcriptome of a marine fish are associated with a better viral resistance. BMC Genomics 23. doi: 10.21203/rs.3.rs-1197163/v1
Cohen-Rengifo M., Mazurais D., Bégout M.-L. (2022b). Raw data concerning the carbonate system and the sensory behaviour of juvenile Dicentrarchus labrax in response to mechano-acoustic and visual cues under ocean warming and acidification. SEANOE. doi: 10.17882/87244
Cominassi L., Moyano M., Claireaux G., Howald S., Mark F. C., Zambonino-Infante J.-L., et al. (2019). Combined effects of ocean acidification and temperature on larval and juvenile growth, development and swimming performance of European sea bass (Dicentrarchus labrax). PloS One 14, e0221283. doi: 10.1371/journal.pone.0221283
Devine B. M., Munday P. L. (2013). Habitat preferences of coral-associated fishes are altered by short-term exposure to elevated CO2. Mar. Biol. 160, 1955–1962. doi: 10.1007/s00227-012-2051-1
Devine B. M., Munday P. L., Jones G. P. (2012). Homing ability of adult cardinalfish is affected by elevated carbon dioxide. Oecologia 168, 269–276. doi: 10.1007/s00442-011-2081-2
Dickson A. G., Millero F. J. (1987). A comparison of the equilibrium constants for the dissociation of carbonic acid in seawater media. Deep. Sea. Res. Part A. Oceanogr. Res. Papers. 34, 1733–1743. doi: 10.1016/0198-0149(87)90021-5
Dixson D. L., Munday P. L., Jones G. P. (2010). Ocean acidification disrupts the innate ability of fish to detect predator olfactory cues. Ecol. Lett. 13, 68–75. doi: 10.1111/j.1461-0248.2009.01400.x
Dlugokencky E., Tans P. (2021) Trends in atmospheric carbon dioxide (NOAA/ESRL). Available at: https://www.esrl.noaa.gov/gmd/ccgg/trends/mlo.html (Accessed August 18, 2023).
Domenici P., Allan B. J. M., Lefrançois C., McCormick M. I. (2019). The effect of climate change on the escape kinematics and performance of fishes: implications for future predator–prey interactions. Conserv. Physiol. 7, coz078. doi: 10.1093/conphys/coz078
Domenici P., Allan B. J. M., Watson S.-A., McCormick M. I., Munday P. L. (2014). Shifting from right to left: the combined effect of elevated CO2 and temperature on behavioural lateralization in a coral reef fish. PloS One 9, e87969. doi: 10.1371/journal.pone.0087969
Domenici P., Batty R. S. (1997). Escape behaviour of solitary herring ( Clupea harengus ) and comparisons with schooling individuals. Mar. Biol. 128, 29–38. doi: 10.1007/s002270050065
Domenici P., Hale M. E. (2019). Escape responses of fish: A review of the diversity in motor control, kinematics and behaviour. J. Exp. Biol. 222, jeb166009. doi: 10.1242/jeb.166009
Draper A. M., Weissburg M. J. (2019). Impacts of global warming and elevated CO2 on sensory behavior in predator-prey interactions: A review and synthesis. Front. Ecol. Evol. 7. doi: 10.3389/fevo.2019.00072
Duteil M., Pope E. C., Pérez-Escudero A., de Polavieja G. G., Fürtbauer I., Brown M. R., et al. (2016). European sea bass show behavioural resilience to near-future ocean acidification. R. Soc. Open Sci. 3, 160656. doi: 10.1098/rsos.160656
Esbaugh A. J. (2018). Physiological implications of ocean acidification for marine fish: emerging patterns and new insights. J. Comp. Physiol. B. 188, 1–13. doi: 10.1007/s00360-017-1105-6
FAO Yearbook (2021). Fishery and Aquaculture Statistics 2019/FAO annuaire. Statistiques des pêches et de l’aquaculture 2019/FAO anuario. Estadísticas de pesca y acuicultura 2019 (Rome, Italy: FAO). doi: 10.4060/cb7874t
Ferrari M. C. O., McCormick M. I., Munday P. L., Meekan M. G., Dixson D. L., Lönnstedt O., et al. (2012). Effects of ocean acidification on visual risk assessment in coral reef fishes. Funct. Ecol. 26, 553–558. doi: 10.1111/j.1365-2435.2011.01951.x
Fuiman L. A., Rose K. A., Cowan J. H., Smith E. P. (2006). Survival skills required for predator evasion by fish larvae and their relation to laboratory measures of performance. Anim. Behav. 71, 1389–1399. doi: 10.1016/j.anbehav.2005.11.013
Giles N. (1984). Development of the overhead fright response in wild and predator-naive three-spined sticklebacks, Gasterosteus aculeatus L. Anim. Behav. 32, 276–279. doi: 10.1016/S0003-3472(84)80347-4
Hamilton T. J., Holcombe A., Tresguerres M. (2014). CO 2 -induced ocean acidification increases anxiety in Rockfish via alteration of GABA A receptor functioning. Proc. R. Soc. B.: Biol. Sci. 281, 20132509. doi: 10.1098/rspb.2013.2509
Hecksteden A., Kraushaar J., Scharhag-Rosenberger F., Theisen D., Senn S., Meyer T. (2015). Individual response to exercise training - a statistical perspective. J. Appl. Physiol. 118, 1450–1459. doi: 10.1152/japplphysiol.00714.2014
Heuer R. M., Grosell M. (2014). Physiological impacts of elevated carbon dioxide and ocean acidification on fish. Am. J. Physiology-Regulatory. Integr. Comp. Physiol. 307, R1061–R1084. doi: 10.1152/ajpregu.00064.2014
Howald S., Cominassi L., LeBayon N., Claireaux G., Mark F. C. (2019). Future Ocean warming may prove beneficial for the northern population of European seabass, but ocean acidification does not. J. Exp. Biol. jeb213017, 1–10. doi: 10.1242/jeb.213017
IPCC (2014). Climate change 2014: synthesis report. Contribution of working groups I, II and III to the fifth assessment report of the intergovernmental panel on climate change. IPCC. Eds. Pachauri R., Meyer L. (Geneva: IPCC), 151.
IPCC (2021). “Summary for policymakers”, in Climate Change 2021: The Physical Science Basis. Contribution of Working Group I to the Sixth Assessment Report of the Intergovernmental Panel on Climate Change. Eds. Masson-Delmotte V., Zhai P., Pirani A., Connors S., Péan C., Berger S., et al. (Cambridge, United Kingdom and New York, NY, USA: Cambridge University Press), 3–32. doi: 10.1017/9781009157896.001
Jarrold M. D., Munday P. L. (2018). Elevated temperature does not substantially modify the interactive effects between elevated CO2 and diel CO2 cycles on the survival, growth and behavior of a coral reef fish. Front. Mar. Sci. 5. doi: 10.3389/fmars.2018.00458
Jarrold M. D., Welch M. J., McMahon S. J., McArley T., Allan B. J. M., Watson S.-A., et al. (2020). Elevated CO2 affects anxiety but not a range of other behaviours in juvenile yellowtail kingfish. Mar. Environ. Res. 157, 104863. doi: 10.1016/j.marenvres.2019.104863
Jennings S., Pawson M. G. (1992). The origin and recruitment of bass, Dicentrarchus labrax , larvae to nursery areas. J. Mar. Biol. Ass. 72, 199–212. doi: 10.1017/S0025315400048888
Jiahuan R., Wenhao S., Xiaofan G., Wei S., Shanjie Z., Maolong H., et al. (2018). Ocean acidification impairs foraging behavior by interfering with olfactory neural signal transduction in black sea bream, acanthopagrus schlegelii. Front. Physiol. 9. doi: 10.3389/fphys.2018.01592
Lewis P. D. E., Wallace D. W. R. (2006). MS Excel Program Developed for CO2 System Calculations. (Tennessee: Carbon Dioxide Information Analysis Center, Oak Ridge National Laboratory, U.S. Department of Energy, Oak Ridge). ORNL/CDIAC-105a p. doi: 10.3334/CDIAC/otg.CO2SYS_XLS_CDIAC105a
Lönnstedt O. M., Munday P. L., McCormick M. I., Ferrari M. C. O., Chivers D. P. (2013). Ocean acidification and responses to predators: can sensory redundancy reduce the apparent impacts of elevated CO 2 on fish? Ecol. Evol. 3, n/a–n/a. doi: 10.1002/ece3.684
Manciocco A., Toni M., Tedesco A., Malavasi S., Alleva E., Cioni C. (2015). The acclimation of european sea bass (dicentrarchus labrax) to temperature: Behavioural and neurochemical responses. Ethology 121, 68–83. doi: 10.1111/eth.12315
Marras S., Domenici P. (2013). Schooling Fish Under Attack are not all equal: some lead, others follow. PloS One 8, e65784. doi: 10.1371/journal.pone.0065784
McCormick M. I., Löonnstedt O. M. (2013). Degrading habitats and the effect of topographic complexity on risk assessment. Ecol. Evol. 3, 4221–4229. doi: 10.1002/ece3.793
Mehrbach C., Culberson C. H., Hawley J. E., Pytkowicx R. M. (1973). MEASUREMENT OF THE APPARENT DISSOCIATION CONSTANTS OF CARBONIC ACID IN SEAWATER AT ATMOSPHERIC PRESSURE1. Limnol. Oceanogr. 18, 897–907. doi: 10.4319/lo.1973.18.6.0897
Millot S., Bégout M.-L., Chatain B. (2009). Exploration behaviour and flight response toward a stimulus in three sea bass strains (Dicentrarchus labrax L.). Appl. Anim. Behav. Sci. 119, 108–114. doi: 10.1016/j.applanim.2009.03.009
Munday P. L., Dixson D. L., McCormick M. I., Meekan M., Ferrari M. C. O., Chivers D. P. (2010). Replenishment of fish populations is threatened by ocean acidification. Proc. Natl. Acad. Sci. 107, 12930–12934. doi: 10.1073/pnas.1004519107
Munday P. L., Dixson D. L., Welch M. J., Chivers D. P., Domenici P., Grosell M., et al. (2020). Methods matter in repeating ocean acidification studies. Nature 586, E20–E24. doi: 10.1038/s41586-020-2803-x
Munday P. L., McCormick M. I., Nilsson G. E. (2012). Impact of global warming and rising CO2 levels on coral reef fishes: what hope for the future? J. Exp. Biol. 215, 3865–3873. doi: 10.1242/jeb.074765
Munday P. L., Welch M. J., Allan B. J. M., Watson S. A., McMahon S. J., McCormick M. I. (2016). Effects of elevated CO2 on predator avoidance behaviour by reef fishes is not altered by experimental test water. PeerJ 2016, 1–18. doi: 10.7717/peerj.2501
Nadler L. E., McCormick M. I., Johansen J. L., Domenici P. (2021). Social familiarity improves fast-start escape performance in schooling fish. Commun. Biol. 4, 897. doi: 10.1038/s42003-021-02407-4
Näslund J., Lindström E., Lai F., Jutfelt F. (2015). Behavioural responses to simulated bird attacks in marine three-spined sticklebacks after exposure to high CO2 levels. Mar. Freshw. Res. 66, 877–885. doi: 10.1071/MF14144
Nasuchon N., Yagi M., Kawabata Y., Gao K., Ishimatsu A. (2016). Escape responses of the Japanese anchovy Engraulis japonicus under elevated temperature and CO2 conditions. Fisheries. Sci. 82, 435–444. doi: 10.1007/s12562-016-0974-z
Nilsson G. E., Dixson D. L., Domenici P., Mccormick M. I., Sørensen C., Watson S., et al. (2012). Near-future carbon dioxide levels alter fish behaviour by interfering with neurotransmitter function. Nat. Climate Change 2, 201–204. doi: 10.1038/nclimate1352
Nowicki J. P., Miller G. M., Munday P. L. (2012). Interactive effects of elevated temperature and CO 2 on foraging behavior of juvenile coral reef fish. J. Exp. Mar. Biol. Ecol. 412, 46–51. doi: 10.1016/j.jembe.2011.10.020
Orr J. C., Fabry V. J., Aumont O., Bopp L., Doney S. C., Feely R. A., et al. (2005). Anthropogenic ocean acidification over the twenty-first century and its impact on calcifying organisms. Nature 437, 681–686. doi: 10.1038/nature04095
Paula J. R., Baptista M., Carvalho F., Repolho T., Bshary R., Rosa R. (2019a). The past, present and future of cleaner fish cognitive performance as a function of CO 2 levels. Biol. Lett. 15, 20190618. doi: 10.1098/rsbl.2019.0618
Paula J. R., Repolho T., Pegado M. R., Thörnqvist P.-O., Bispo R., Winberg S., et al. (2019b). Neurobiological and behavioural responses of cleaning mutualisms to ocean warming and acidification. Sci. Rep. 9, 12728. doi: 10.1038/s41598-019-49086-0
Pawson M. G., Pickett G. D., Leballeur J., Brown M., Fritsch M. (2007). Migrations, fishery interactions, and management units of sea bass (Dicentrarchus labrax) in Northwest Europe. ICES. J. Mar. Sci. 64, 332–345. doi: 10.1093/icesjms/fsl035
Pimentel M. S., Faleiro F., Marques T., Bispo R., Dionísio G., Faria A. M., et al. (2016). Foraging behaviour, swimming performance and malformations of early stages of commercially important fishes under ocean acidification and warming. Clim. Change 137, 495–509. doi: 10.1007/s10584-016-1682-5
Porteus C. S., Hubbard P. C., Uren Webster T. M., van Aerle R., Canário A. V. M., Santos E. M., et al. (2018). Near-future CO2 levels impair the olfactory system of a marine fish. Nat. Climate Change 8, 737–743. doi: 10.1038/s41558-018-0224-8
Radford C. A., Collins S. P., Munday P. L., Parsons D. (2021). Ocean acidification effects on fish hearing. Proc. R. Soc. B.: Biol. Sci. 288, 20202754. doi: 10.1098/rspb.2020.2754
R Core Team (2015). R: A language and environment for statistical computing. R. Foundation. Stat. Computing.
Roggatz C. C., Saha M., Blanchard S., Schirrmacher P., Fink P., Verheggen F., et al. (2022). Becoming nose-blind—Climate change impacts on chemical communication. Global Change Biol. 28, 4495–4505. doi: 10.1111/gcb.16209
Rossi T., Nagelkerken I., Pistevos J. C. A., Connell S. D. (2016). Lost at sea: ocean acidification undermines larval fish orientation via altered hearing and marine soundscape modification. Biol. Lett. 12, 20150937. doi: 10.1098/rsbl.2015.0937
Schneider C. A., Rasband W. S., Eliceiri K. W. (2012). NIH Image to ImageJ: 25 years of image analysis. Nat. Methods 9, 671–675. doi: 10.1038/nmeth.2089
Sheaves M., Baker R., Nagelkerken I., Connolly R. M. (2015). True value of estuarine and coastal nurseries for fish: incorporating complexity and dynamics. Estuaries. Coasts. 38, 401–414. doi: 10.1007/s12237-014-9846-x
Simpson S. D., Munday P. L., Wittenrich M. L., Manassa R., Dixson D. L., Gagliano M., et al. (2011). Ocean acidification erodes crucial auditory behaviour in a marine fish. Biol. Lett. 7, 917–920. doi: 10.1098/rsbl.2011.0293
Steckbauer A., Díaz-Gil C., Alós J., Catalán I. A., Duarte C. M. (2018). Predator avoidance in the european seabass after recovery from short-term hypoxia and different CO2 conditions. Front. Mar. Sci. 5. doi: 10.3389/fmars.2018.00350
Stiasny M. H., Mittermayer F. H., Sswat M., Voss R., Jutfelt F., Chierici M., et al. (2016). Ocean acidification effects on atlantic cod larval survival and recruitment to the fished population. PloS One 11, e0155448. doi: 10.1371/journal.pone.0155448
Strickland J., Parsons A. (1972). A Practical Handbook of Seawater Analysis. Ottawa, Vol. 293. doi: 10.1002/iroh.19700550118
Strod T., Izhaki I., Arad Z., Katzir G. (2008). Prey detection by great cormorant (Phalacrocorax carbo sinensis)in clear and in turbid water. J. Exp. Biol. 211, 866–872. doi: 10.1242/jeb.014324
Sundin J., Amcoff M., Mateos-González F., Raby G. D., Jutfelt F., Clark T. D. (2017). Long-term exposure to elevated carbon dioxide does not alter activity levels of a coral reef fish in response to predator chemical cues. Behav. Ecol. Sociobiol. 71, 108. doi: 10.1007/s00265-017-2337-x
Sundin J., Jutfelt F. (2016). 9–28 d of exposure to elevated pCO2 reduces avoidance of predator odour but had no effect on behavioural lateralization or swimming activity in a temperate wrasse (Ctenolabrus rupestris). ICES. J. Mar. Sci. 73, 620–632. doi: 10.1093/icesjms/fsv101
Sundin J., Jutfelt F. (2018). Effects of elevated carbon dioxide on male and female behavioural lateralization in a temperate goby. R. Soc. Open Sci. 5, 171550. doi: 10.1098/rsos.171550
Tresguerres M., Hamilton T. J. (2017). Acid-base physiology, neurobiology and behaviour in relation to CO2-induced ocean acidification. J. Exp. Biol. 220, 2136–2148. doi: 10.1242/jeb.144113
Vandeputte M., Gagnaire P -A., Allal F. (2019). The European sea bass: a key marine fish model in the wild and in aquaculture. Anim. Genet. 50, 195–206. doi: 10.1111/age.12779
Velez Z., Roggatz C. C., Benoit D. M., Hardege J. D., Hubbard P. C. (2019). Short- and medium-term exposure to ocean acidification reduces olfactory sensitivity in gilthead seabream. Front. Physiol. 10. doi: 10.3389/fphys.2019.00731
Vinebrooke R D., Cottingham K L., Norberg Marten Scheffer J., I. Dodson S., C. Maberly S., Sommer U. (2004). Impacts of multiple stressors on biodiversity and ecosystem functioning: the role of species co-tolerance. Oikos 104, 451–457. doi: 10.1111/j.0030-1299.2004.13255.x
Watson S., Allan B. J. M., McQueen D. E., Nicol S., Parsons D. M., Pether S. M. J., et al. (2018). Ocean warming has a greater effect than acidification on the early life history development and swimming performance of a large circumglobal pelagic fish. Glob. Change Biol. 24, 4368–4385. doi: 10.1111/gcb.14290
Williamson P., Pörtner H., Widdicombe S., Gattuso J. (2020). Ideas and Perspectives: When ocean acidification experiments are not the same, reproducibility is not tested. Biogeosci. Discussions. 18, 1–7. doi: 10.5194/bg-2020-394
Keywords: sensory ecology, anti-predator response, vision, mechano-audition, ocean warming and acidification, European sea bass
Citation: Cohen-Rengifo M, Mazurais D and Bégout M-L (2023) Response to visual and mechano-acoustic predator cues is robust to ocean warming and acidification and is highly variable in European sea bass. Front. Mar. Sci. 10:1108968. doi: 10.3389/fmars.2023.1108968
Received: 26 November 2022; Accepted: 04 October 2023;
Published: 18 October 2023.
Edited by:
Jeff Shimeta, RMIT University, AustraliaReviewed by:
Philip Munday, James Cook University, AustraliaKenneth Zillig, University of California, Davis, United States
Copyright © 2023 Cohen-Rengifo, Mazurais and Bégout. This is an open-access article distributed under the terms of the Creative Commons Attribution License (CC BY). The use, distribution or reproduction in other forums is permitted, provided the original author(s) and the copyright owner(s) are credited and that the original publication in this journal is cited, in accordance with accepted academic practice. No use, distribution or reproduction is permitted which does not comply with these terms.
*Correspondence: Mishal Cohen-Rengifo, bWlzaGFsLmNvaGVuLnJAZ21haWwuY29t