- 1Department of Environment and Geography, Wentworth Way, University of York, York, United Kingdom
- 2School of Geographical and Earth Sciences, University of Glasgow, Glasgow, United Kingdom
- 3UK Centre for Ecology and Hydrology, Bangor, United Kingdom
- 4Department of Biology, Wentworth Way, University of York, York, United Kingdom
- 5Institute of Science and Environment, University of Cumbria, Ambleside, United Kingdom
Political discourse around coastal wetland restoration and blue carbon management strategies has increased in the past decade, yet carbon storage has neither been a reason for restoration, nor a criterion to measure the success of current saltmarsh restoration schemes in the UK. To maximise climate change mitigation through saltmarsh restoration, knowledge on the key drivers of carbon stock variability is required. We use restored saltmarshes of similar age, paired with adjacent natural marshes as references, to identify drivers of carbon stocks following managed realignment within an estuary in southeastern England. From surficial soil cores (top 30 cm), we measured carbon stock alongside environmental characteristics. Carbon stock between natural and restored sites were similar after ~ 30 years when restored sites were above mean high water neap (MHWN) tidal levels. Elevated marsh platforms likely provide suitable conditions for the development of mature plant communities associated with greater capture and production of organic carbon. The restored site at Tollesbury (Essex, UK) had a 2-fold lower carbon stock than other restored sites in the estuary. We attribute this to the site’s low position in the tidal frame, below MHWN tidal levels, coupled with low sediment supply and the dominance of pioneer plant communities. As blue carbon is anticipated to become an important facet of saltmarsh restoration, we recommend that sites above MHWN tidal levels are selected for managed realignment or that preference is given to coastlines with a high sediment supply that may rapidly elevate realignment sites above MHWN. Alternatively, elevation could be artificially raised prior to realignment. Restoration schemes aiming to maximise climate change mitigation should also encourage the establishment of key plant species (e.g., Atriplex portulacoides in our study) to enhance carbon stocks. However, the overall goal of restoration ought to be carefully considered as trade-offs in ecosystem services may ensue if restoration for climate change mitigation alone is pursued.
1 Introduction
The capture and storage of blue carbon (organic carbon found within marine and coastal ecosystems) is increasingly recognized by governments, industries, and scientists around the world as a nature-based solution to help mitigate climate change (Lovelock and Duarte, 2019; Macreadie et al., 2019; Bertram et al., 2021). Coastal wetlands, including saltmarshes, store more carbon per unit area than terrestrial forests, and represent a globally significant carbon sink (Duarte et al., 2005; Nellemann and Corcoran, 2009; Mcleod et al., 2011; Temmink et al., 2022). In addition to their climate change mitigation capacities (via carbon capture and subsequent burial), coastal wetlands provide numerous other ecosystem services and benefits, including fisheries support, coastal flood protection, and biodiversity enhancement (Barbier et al., 2011; Möller et al., 2014; Unsworth et al., 2019). Coastal wetlands are also amongst the most threatened habitats in the world. Although global assessments of saltmarsh extent change are lacking, saltmarsh habitat loss occurs by approximately 1 – 2 % per year (Duarte et al., 2008), with large-scale losses to sea-level rise anticipated (Saintilan et al., 2022). Recent restoration efforts have successfully offset a large proportion of tidal wetland loss globally (Murray et al., 2022). However, in the UK, the area of saltmarsh created via restoration is considered to be behind the pace required to compensate for current and historic losses in extent (Rupp-Armstrong and Nicholls, 2007; Lawrence, 2018).
Saltmarsh loss and degradation not only reduces future carbon sequestration potential (Howard et al., 2017; Lovelock et al., 2017), but can result in the ecosystem switching from a carbon sink to a carbon source (Macreadie et al., 2013). This occurs when soil disturbance leads to the remineralization of particulate organic matter, whereby carbon stored in biomass and soil is oxidized and released back into the atmosphere as CO2 (Pendleton et al., 2012; Lovelock et al., 2017). The conservation and restoration of blue carbon habitats can therefore enhance CO2 capture, as well as avoid CO2 emissions from habitat degradation (Duarte et al., 2013; Macreadie et al., 2013; Kelleway et al., 2020). Saltmarshes are therefore part of the solution to limiting global warming to below the 1.5°C threshold set out in the Paris Agreement, and enhancing the removal of excess CO2 from the atmosphere (Chausson et al., 2020; Macreadie et al., 2021). Political discourse around coastal wetland restoration and blue carbon strategies has exponentially increased in the past decade, with 46 countries now mentioning coastal and marine ecosystems as mitigation solutions within their Nationally Determined Contributions – the country-level plans to address climate change as part of the Paris Agreement (Lecerf et al., 2021). To maximize climate change mitigation through coastal wetland restoration, an understanding of the environmental drivers that influence carbon storage in restored coastal wetlands is required.
Nations around the globe are investing in coastal wetland restoration and there is international interest in the inclusion of coastal wetlands in climate change mitigation policy through carbon financing (Wylie et al., 2016; Vanderklift et al., 2019). Numerous approaches to restoring saltmarsh habitat exist (MacDonald et al., 2020; Hudson et al., 2021); however, the predominant method of saltmarsh restoration in the UK and Europe is managed realignment (MR), defined as the deliberate breaching of coastal defenses to reinstate tidal inundation and recreate intertidal habitat (Luisetti et al., 2011; Burden et al., 2019). The capacity of healthy saltmarshes to keep pace with sea-level rise (Kirwan et al., 2016) and to naturally dissipate wave energy (Möller et al., 2014), makes their restoration an attractive option in reducing maintenance costs of coastal defenses and improving flood management (Shepherd et al., 2007). In line with the EU Habitats Directive, MR also compensates for historic losses of intertidal habitat and supports biodiversity (Hudson et al., 2021). Of the 48 MR schemes in the UK where saltmarsh habitat has been restored, cost-effective flood protection, compensation for natural habitat loss, and habitat creation were listed as the primary reasons for restoration (ABPmer, 2022).
Although saltmarsh carbon storage is now frequently highlighted as a co-benefit of restoration, blue carbon has neither been a reason for restoration, nor a criterion against which to measure the success of current MR schemes in the UK (Burden et al., 2013; Austin et al., 2022). Despite this, coastal wetland restoration for blue carbon benefits has become a recognized tool in climate change mitigation (Wylie et al., 2016; Duarte et al., 2020). The increasing interest in using nature-based solutions for climate change mitigation, complemented by the United Nations Decade on Ecosystem Restoration (2021 – 2030), provides an opportunity for blue carbon and the maximization of climate change mitigation benefits to be an important facet of future MR schemes (Seddon et al., 2020; Waltham et al., 2020; Austin et al., 2022). There have already been calls for blue carbon habitat restoration to be a key focus of the UN Decade on Ecosystem Restoration (Macreadie et al., 2021).
Initial carbon accumulation rates in MR saltmarshes can be high. The large accommodation space created by MR rapidly fills with riverine and/or marine sediment (if available) that can be rich in allochthonous carbon (Wollenberg et al., 2018; Drexler et al., 2020). However, the timescales involved in the development of carbon stocks equivalent to adjacent natural sites is unclear. Previous studies have reported carbon stocks in restored saltmarshes become comparable to natural marshes over decadal to centennial timescales, with estimates up to 100 years (Burden et al., 2013; Burden et al., 2019). Conservation of existing carbon stocks in natural marshes is therefore often prioritized as a climate change mitigation strategy over restoration (Macreadie et al., 2017). Recent evidence from the USA, however, found carbon stocks in the top 30 cm of a MR site were only marginally lower than adjacent natural sites in the 4 years following restoration (4.43 and 5.95 kg C m-2 respectively; Poppe and Rybczyk, 2021). The various timescales reported for the development of carbon stocks in restored saltmarshes are highly site-specific - an important consideration for MR as a technique to enhance climate change mitigation. For example, Nightingale et al. (2022) recommended choosing optimal regional and ecological factors that enhance carbon accumulation rates in realigned saltmarshes, such as higher latitudes, when focusing on the climate change mitigation potential of future MR schemes.
Whilst advances have been made on a global (Rogers et al., 2019a) and regional (Ford et al., 2019) scale, few studies have examined saltmarsh blue carbon variability at the estuarine scale (Broek et al., 2016) and even fewer for MR sites. Identifying local scale controls on blue carbon variability within MR sites will ascertain how the increasing number of MR schemes globally contribute to addressing the Climate Crisis. Drivers of blue carbon stock in saltmarshes include environmental characteristics such as elevation and local hydrology, that influence the supply of sediment and allochthonous carbon into a site (Wollenberg et al., 2018; Rogers et al., 2019a; Mossman et al., 2022), sedimentary characteristics such as particle size that affect the preservation of carbon stocks (Kelleway et al., 2016; Ford et al., 2019), and vegetation characteristics that include vegetation community composition and diversity, which affect both the supply of autochthonous carbon through primary production as well as the trapping of allochthonous carbon (Ouyang and Lee, 2014).
To our knowledge, no study has yet compared a range of environmental drivers against blue carbon stocks in MR saltmarshes. Here, we investigate which drivers enhance blue carbon capture of saltmarsh MR sites within an estuary in southeastern England to inform future schemes seeking to maximise the potential blue carbon benefits of MR. The objectives of this study are to: (1) quantify blue carbon stocks for the top 30 cm of soil for saltmarshes with different management histories (managed realigned and adjacent natural sites) within the same estuary; (2) evaluate the relationship between environmental variables and blue carbon stock to determine the greatest drivers of spatial variation, and; (3) provide management recommendations to maximize blue carbon stocks in future MR schemes.
2 Materials and methods
2.1 Study sites and sampling design
We studied six saltmarshes (three managed realigned sites and three adjacent natural sites) in the Blackwater Estuary, Essex, UK (Figure 1). The Blackwater Estuary is of high biological and conservation importance, as reflected in numerous conservation designations: Site of Special Scientific Interest, Ramsar site, Special Area of Conservation, and Special Protection Area. Alongside neighboring estuaries (Crouch, Roach, and Colne), the area forms part of a designated inshore marine conservation zone. The estuary is also home to the first MR scheme in the UK at Northey Island, which was undertaken in 1991 as a pilot project to improve coastal defenses (Doody, 2013; Ladd, 2021).
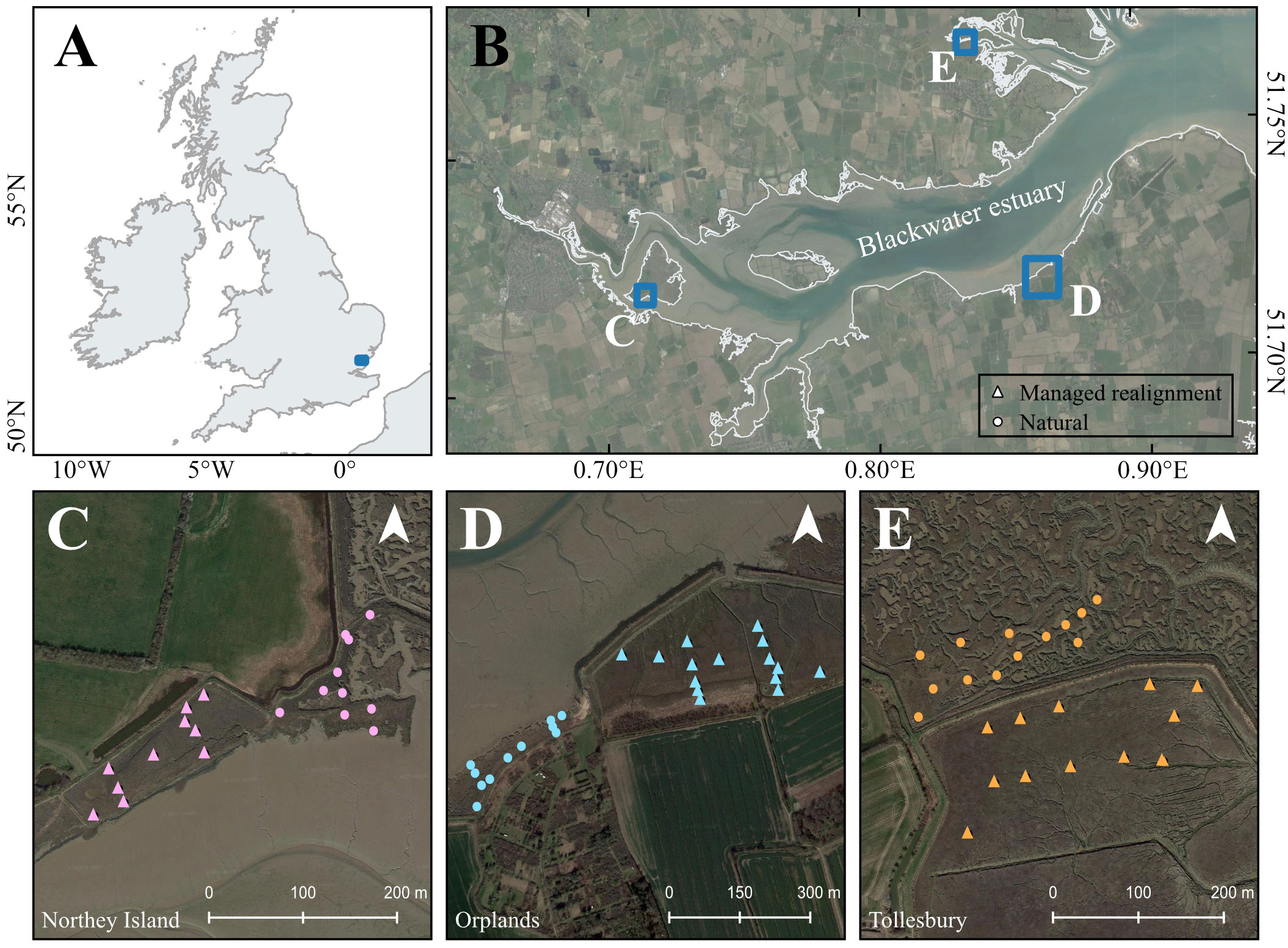
Figure 1 Location of Blackwater Estuary, Essex within southeastern England (A) and saltmarsh study sites within the Blackwater Estuary (B). Sampling transects and plot locations are shown for managed realigned (triangle) and natural (circle) sites at Northey Island (C), Orplands (D), and Tollesbury (E).
Three managed realigned sites in the Blackwater Estuary were chosen for this study: Northey Island, Orplands and Tollesbury (Table 1), all of which were restored primarily for the purposes of improving flood protection and creating habitat (ABPmer, 2022). Sites were selected due to similar timing of realignment in the early to mid-1990s (Table 1) and the presence of adjacent natural saltmarshes that could act as controls for the study. All realignment sites were restored by breaches to existing coastal defenses (Table 1). A single breach was implemented at Northey Island and Tollesbury MR sites during construction, whereas multiple breaches were implemented at Orplands MR (ABPmer, 2022; Table 1). The Blackwater estuary is macrotidal (tidal range: 5.2 – 5.8 m) and ebb-dominant, with a net export of sediment and material from the mouth of the estuary (Ladd et al., 2019).
To gain insights into site-specific environmental setting, a range of tidal, vegetation and soil characteristics shown to influence blue carbon stocks were sampled at each site along three transects: two perpendicular to the marsh edge and one passing diagonally across (Figure 1). Multiple sampling plots (1 m × 1 m quadrat) were placed along each transect to capture heterogeneity in saltmarsh vegetation zones and elevation. The number of sampling plots varied between 10 and 14. All fieldwork was carried out in July 2019.
2.2 Data collection
2.2.1 Tidal characteristics
Site-specific hydrology can provide insights on potential allochthonous carbon supply, as well as the development of vegetation communities and plant zones. Elevation was recorded at each sampling plot using a Trimble Catalyst differential global positioning system with an accuracy of<1 cm. To standardize sampling plot elevation to the respective height in the tidal frame and to allow for comparisons across sites, relative tidal height was calculated, where 0 = mean high water neap (MHWN) and 1 = mean high water spring (MHWS) tidal levels (Mossman et al., 2012a; Mossman et al., 2020):
MHWN and MHWS tidal data were collated for each site from Mossman et al. (2012b). Tidal data was available for Orplands, Tollesbury (natural) and Tollesbury (MR) sites. There was no available data for Northey Island, so data was used from the next closest site, Steeple, less than 5 km away.
2.2.2 Vegetation characteristics
Vegetation characteristics were recorded as a proxy for potential autochthonous and allochthonous (via trapping) carbon supply (Ford et al., 2019). Above-ground vegetation characteristics, which are best described by species identity, vegetation height and plant density (Owers et al., 2018) were measured within each sampling plot (1 m × 1 m quadrat). Average vegetation height was taken at 10 representative positions within each plot. Plant cuttings were removed from a 25 cm2 area within each plot and oven-dried (60°C, 72 hrs) for above-ground biomass calculations. Saltmarsh plants were identified to species level and percentage cover of individual species was recorded to give an indication of community composition. Each quadrat/sampling point was then categorized following National Vegetation Classification (NVC) and corresponding marsh zone (Rodwell, 2000; Supplementary Table 1). Species richness and Shannon-Wiener’s Diversity Index (H’) were also calculated per plot:
where:
2.2.3 Soil characteristics
Soil cores (diameter: 5 cm; depth: 30 cm) were extracted using a gouge auger and sub-sampled in-situ at 0 – 10 cm, 10 – 20 cm and 20 – 30 cm depth intervals per sampling point. A depth of 30 cm was chosen in line with other studies that have quantified carbon stocks in MR sites (Burden et al., 2019). Regular monitoring at Tollesbury MR has shown mean annual sedimentation rates were 2.3 cm between 1995 and 2001 (Garbutt et al., 2006). When the sampling for this study took place in 2019, it would have been 24 years post-breach at Orplands and Tollesbury MRs, and 28 years post-breach at Northey Island MR. The top 30 cm of soil for all MR sites in this study therefore likely represents post-realignment accumulation. Attempts to visually identify boundaries in the facies between pre- and post-restoration deposits per soil core were inconclusive.
Subsamples from each core were oven-dried (60°C, minimum 24 hrs). At temperatures exceeding 60°C, fractions of soil organic matter may oxidize and cause an under-estimation of organic carbon (Howard et al., 2014). Dry bulk density was calculated by dividing the dry weight of soil by sample volume. Dried samples were then ground, sieved (1 mm mesh size) to remove large inorganic particles, and sub-sampled to provide material for total organic carbon and particle size analysis.
Total organic carbon (TOC) was measured using a Carbon and Nitrogen elemental analyzer (Flash, 2000, Thermo Fisher Scientific). The instrument was calibrated using aspartic acid as a standard. Prior to TOC analysis, samples were homogenized to a fine powder using a ball mill (Retsch MM200; 250 µm, 2 mins). Between 10 – 15 mg of sample was placed into silver capsules and treated with 10 % HCl acid to remove carbonates (CaCO3) (Smeaton et al., 2022). Following acidification, samples were not observed to effervesce, suggesting that inorganic carbon represented a minimal component of total sediment weight (Howard et al., 2014). The acidified samples were then dried overnight at 50°C and sealed (Smeaton et al., 2022). A replicate was taken every 5 samples to assess the reliability of %TOC data.
Prior to particle size analysis, dried soil samples (~2 g) were digested in 10 ml 30 % hydrogen peroxide (H2O2) and heated on a hotplate (50°C). To remove all residual organic material, H2O2 was continuously added until reactions had ceased. Samples were then heated to reduce liquid content and left to cool prior to centrifuging (3 times: 3,500 rpm; 8 minutes). To prevent flocculation, 2 ml sodium hexametaphosphate [(NaPO3)6] was added to samples. Particle size was measured using a laser granulometer (Malvern Mastersizer Hydro, 2000), and test sand was used to calibrate the instrument. Outputs from the laser granulometer were given as percentages of different size categories, and then grouped into 3 main categories (clay, silt, and sand) using the Wentworth (1922) classification scheme. Clay particles were not detected in any samples during particle size analysis. A sand:silt ratio was then calculated for data analysis:
2.2.4 Calculation of organic carbon stock
Organic carbon soil stocks were calculated using soil core depth (30 cm), depth interval of subsamples (10 cm), dry bulk density (DBD) and total organic carbon (TOC). Soil organic carbon density (SOC) of each soil core was calculated using the approach outlined in the Coastal Blue Carbon Manual (The International Blue Carbon Initiative; Howard et al., 2014) as follows:
Organic carbon content (g C cm-3) for each subsample was multiplied by the depth interval of the subsample (10 cm) and summed across the three subsamples (0 – 10 cm, 10 – 20 cm, and 20 – 30 cm). Organic carbon stocks were converted to Mg C ha-1 to 30 cm for each sampling point. Mean organic carbon stock and standard deviation for the top 30 cm for each site was then calculated using each sampling point within a site.
2.3 Data analysis
Statistical analysis was conducted using R software (version 4.1.2). Site differences between each variable were tested using ANOVA. If present, significantly different means were identified using post-hoc Tukey tests. For categorical variables, including those with unequal sample sizes, non-parametric Kruskal-Wallis and post-hoc Dunn tests were used instead. When variables did not meet the assumption of normality, log and exponential transformations were applied and retested using Shapiro-Wilk and diagnostic plots. All models met the assumptions of normality, homoscedasticity, and leverage.
Multivariate Generalized Linear Models (“MASS” package; Venables and Ripley, 2002) were used to determine the relationship between organic carbon stock and a set of predictor variables. A priori knowledge about the data was used to determine the error distribution and link function for models. Model residuals were checked for normality, homoscedasticity, and bias by unduly influential observations using diagnostic plots, supported by the Shapiro-Wilk test for normality, and Breusch-Pagan test for heteroscedasticity. A log link function was used with a gaussian error family to reduce high leverage values observed in model residuals. When the response variable (carbon stock) was positively skewed, a Gamma error distribution and log link function was used to meet model assumptions. When heteroscedasticity was present and/or model residuals were non-normally distributed after changing the error family or link function, the dependent variable (carbon stock) was log transformed. Diagnostic plots and Akaike Information Criterion (AIC) were used to confirm error family and link function choices during model selection. Models with the lowest AIC value were deemed best fit for the data. Model residuals were also tested for serial autocorrelation to determine whether a mixed effects model with a random component was necessary. Auto-Correlation Function plots and Durbin-Watson tests showed model residuals did not contain serial autocorrelation and therefore Multivariate Generalized Linear Models were an appropriate method for analysis.
Predictor variables were tested for correlation using Pearson’s coefficient (r) and Variance Inflation Factors (VIF). Correlation was considered high if r ≥ 0.6 and/or VIF ≥ 3 (Zuur et al., 2010). Predictor variables that displayed high levels of collinearity were not included in the same model (Supplementary Figure 1). The stepAIC function (“MASS” package; Venables and Ripley, 2002), based on Akaike information criterion (AIC), was used in model selection to produce an optimal model. Analysis of variance was used to test the probability of decreased deviance between the optimal and full model. Finally, for each optimal model, the deviance explained by the model was calculated [1 – Residual deviance/Null deviance]. Where multiple predictors were retained in the optimal model, hierarchical partitioning using the “hier.part” package (Mac Nally and Walsh, 2004) was used to identify independent effects (%) that each predictor contributed to the optimal model. All figures were created using the “ggplot2” package (Wickham, 2016).
3 Results
Variation in carbon stock and environmental variables were compared between sites, and management history (managed realigned and natural saltmarshes). Environmental variables were then analyzed against carbon stock to determine the key drivers of variation.
3.1 Variation among sites
3.1.1 Carbon stock
Mean carbon stock within saltmarsh soil (top 30 cm) of all six sites was 78.9 ± 27.5 Mg C ha-1 (n = 70). Natural sites had a mean carbon stock (87.7 ± 8.4 Mg C ha-1) greater than MR sites (72.1 ± 25.8 Mg C ha-1). Among all six sites, carbon stock ranged between 43.3 and 94.5 Mg C ha-1 (Table 2). Mean carbon stock was highest in the natural site at Orplands (Table 2; Figure 2). Tollesbury MR had the lowest mean carbon stock which was 36.3 Mg C ha-1 less than the MR site at Orplands, and 50 Mg C ha-1 less than the MR site at Northey Island (Table 2; Figure 2). The natural site at Tollesbury had a lower carbon stock than the MR and natural sites at Northey Island and Orplands (Table 2; Figure 2).
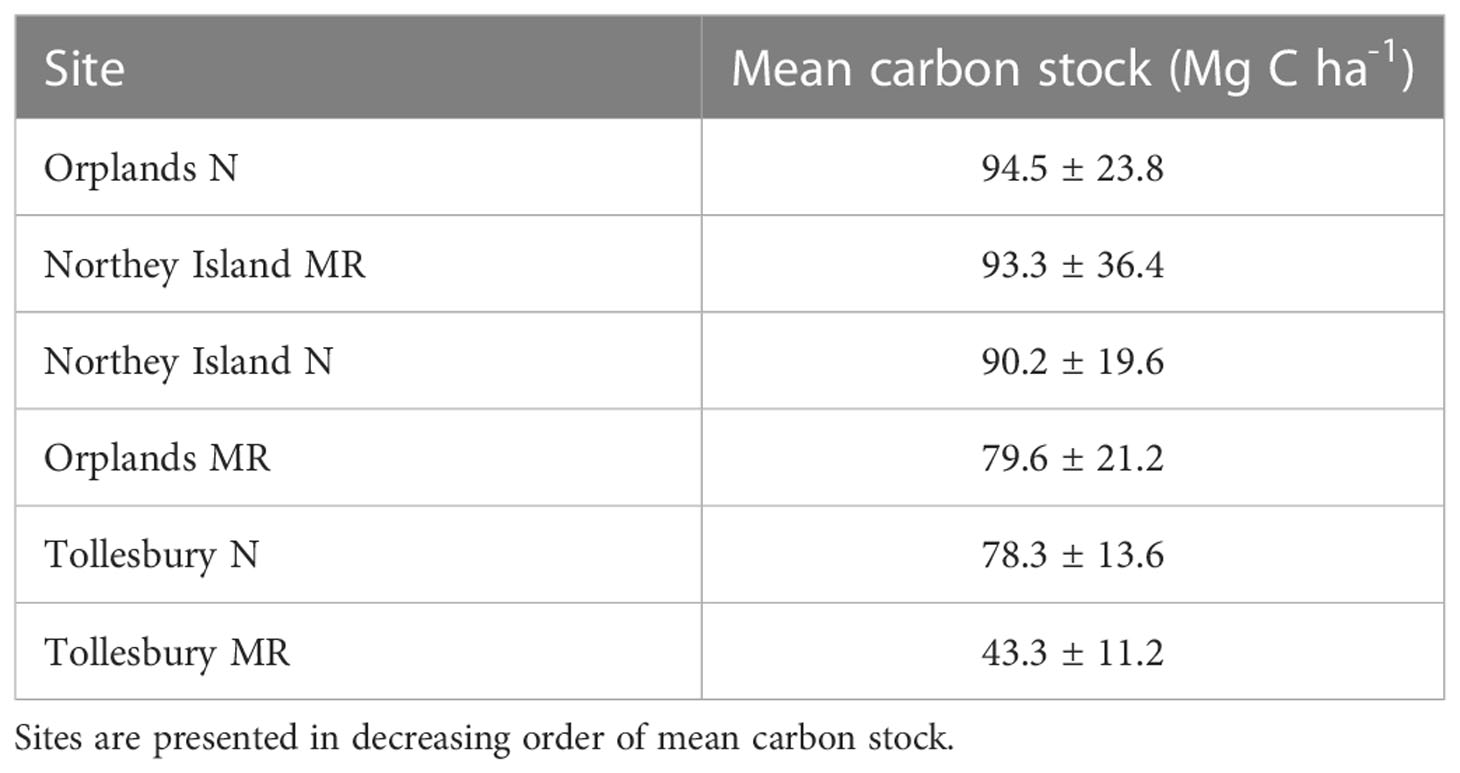
Table 2 Mean carbon stock (Mg C ha-1) in the top 30 cm of saltmarsh soil at managed realigned (MR) and natural (N) sites ± standard deviation.
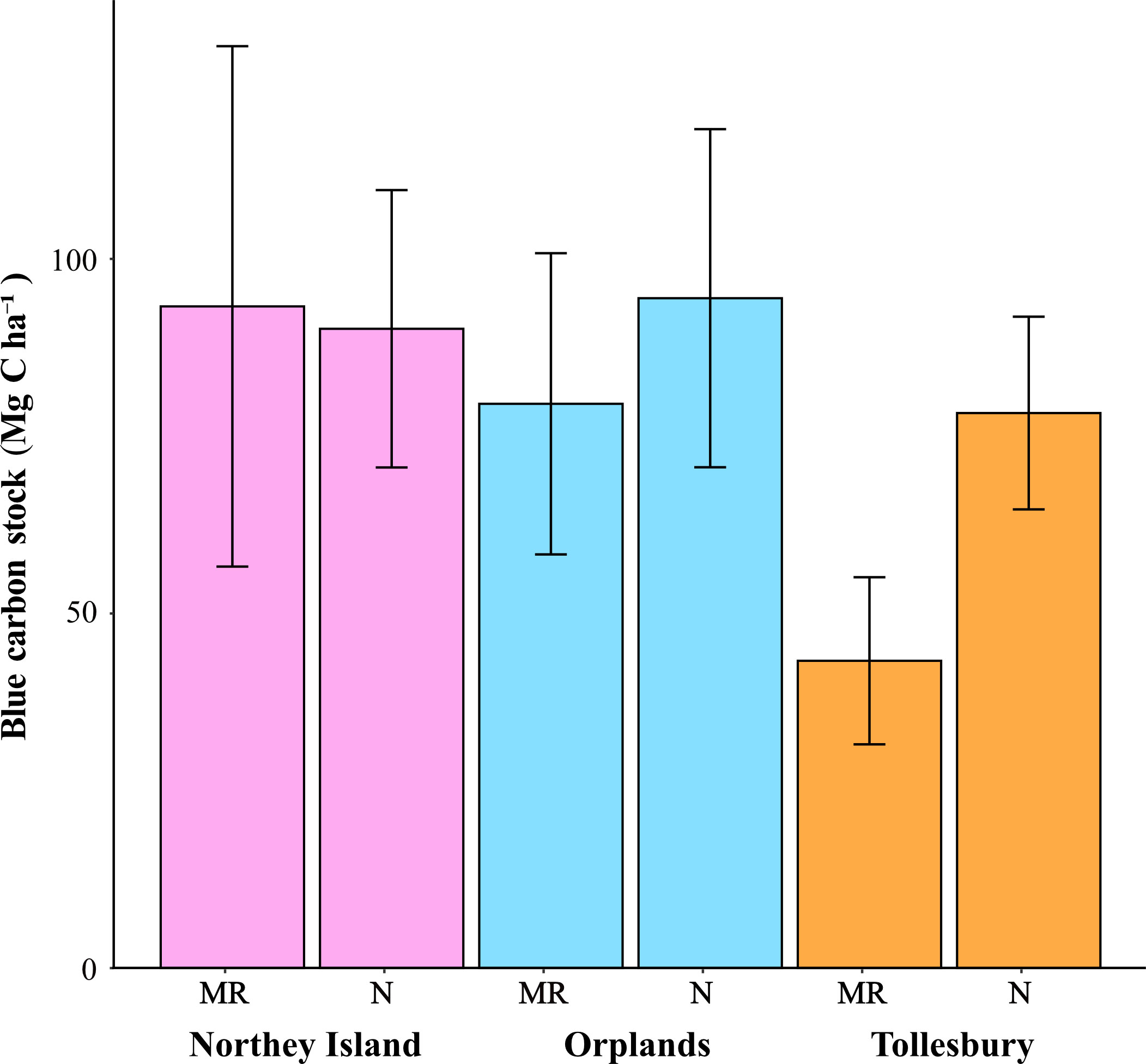
Figure 2 Mean carbon stock ± standard deviation in the top 30cm of soil for managed realigned (MR) and natural (N) saltmarsh sites at Northey Island (pink), Orplands (blue) and Tollesbury (orange) in the Blackwater Estuary, Essex, UK.
Among all sites, carbon stock was significantly different (N = 70; F = 8.796; p< 0.001). Mean carbon stock at Tollesbury MR was significantly lower than the MR sites at Northey Island and Orplands (p< 0.001 and p = 0.001 respectively), and the natural sites at Northey Island (p< 0.001), Orplands (p< 0.001), and Tollesbury (p = 0.02). When excluding Tollesbury MR, there was no significant difference in mean carbon stock between sites (N = 58; F = 1.286; p = 0.29; Figure 2) and mean carbon stocks between natural and MR sites were similar (87.7 ± 8.4 and 86.5 ± 9.7 Mg C ha-1, respectively).
3.1.2 Environmental variables
Full statistical results for ANOVA and Kruskal-Wallis tests, and subsequent post-hoc tests, for environmental variables can be found in (Supplementary Tables 2A–H). Our main findings are outlined below.
The mean dry bulk density (DBD) of all samples in this study was 0.64 ± 0.19 g cm-3. However, the DBD at Northey Island and Orplands MR sites (0.82 ± 0.23 g cm-3 and 0.79 ± 0.20 g cm-3, respectively; Table 3) was significantly higher than the three natural sites. DBD did not differ significantly between Tollesbury MR and any other site, nor between natural sites. Soil samples from all sites were predominantly composed of silt particles, according to classifications from the Wentworth scale. The percentage of silt recorded in samples ranged from 63.1 – 97.5%, with the remainder of samples comprised of sand particles. However, the natural site at Orplands had a significantly higher sand:silt ratio (Table 3), and thus a significantly higher proportion of sand particles than all other sites. The MR and natural sites at Northey Island and Tollesbury had a similar sand:silt ratio (ranging between 0.12 ± 0.07 and 0.14 ± 0.11; Table 3). The sand:silt ratio at Orplands MR was 2-fold lower than the site with the next lowest value, whilst the natural site at Orplands was over 2 times greater than the next highest site (Table 3). Dry bulk density and particle size were not highly correlated with each other or any other variable (Supplementary Figure 1).
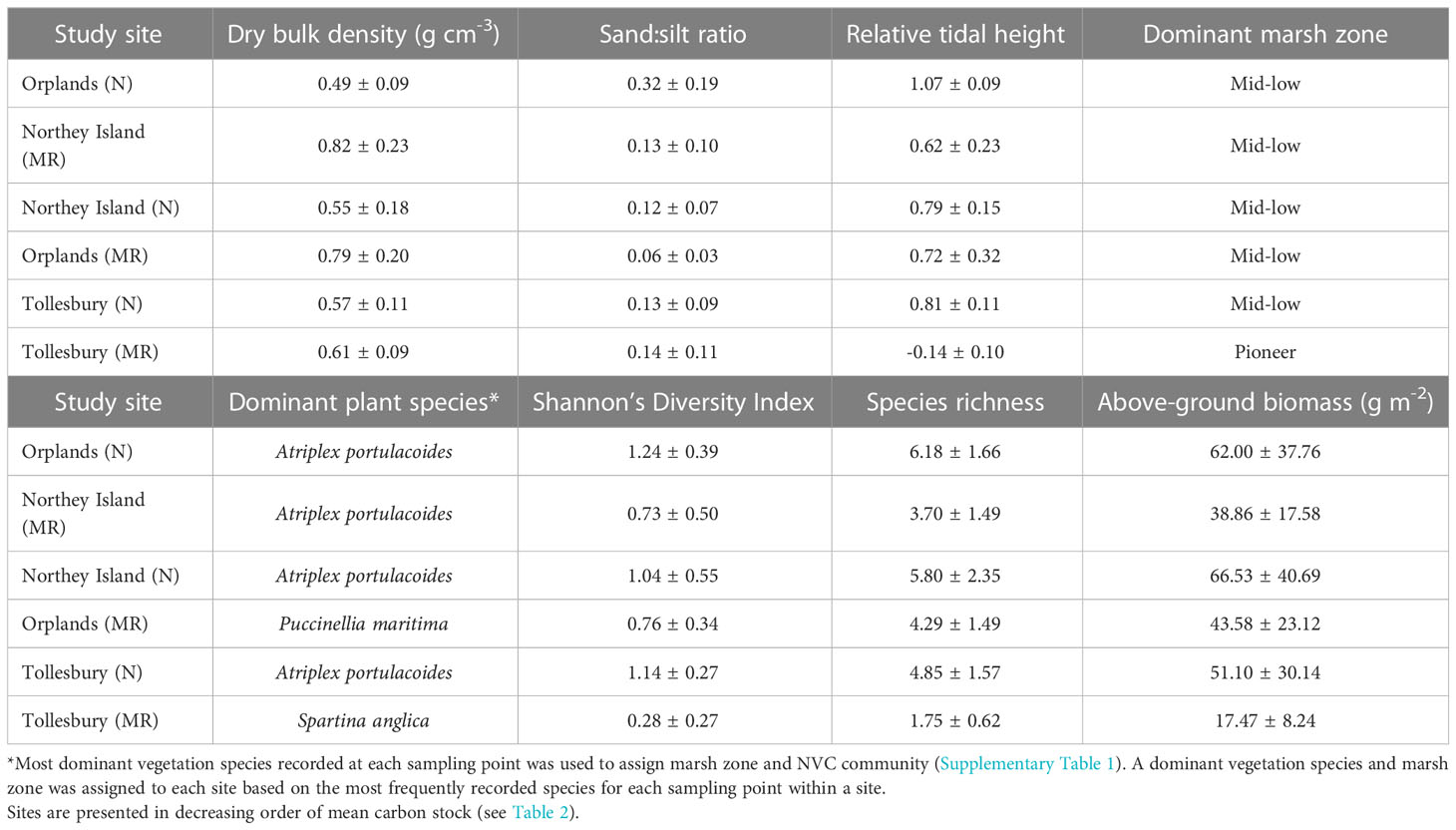
Table 3 Mean environmental variables at each natural (N) and managed realignment (MR) site ± standard deviation.
The mean relative tidal height of saltmarshes, where 0 represents MHWN and 1 represents MHWS tidal level, of natural sites was 0.89 ± 0.12. This was over 2-fold higher than the value obtained for MR sites (0.40 ± 0.22). Of our sites, only Tollesbury MR had a mean relative tidal height below the MHWN tidal level (- 0.14 ± 0.10; Table 3), and this was significantly lower in the tidal frame than all other sites. The natural site at Orplands was the only site with a mean relative tidal height above MHWS tidal level (1.07 ± 0.09; Table 3) and was significantly higher than all other sites. Relative tidal height was positively correlated with marsh plant zone (r = 0.61; t = 6.32; p< 0.001; Supplementary Figure 1) and NVC community (r = 0.62; t = 6.40; p< 0.001; Supplementary Figure 1).
With the exception of Tollesbury MR, there was no significant difference in marsh zone or NVC community between sites or management history. Marsh zone at Tollesbury MR was significantly different to all other sites, and NVC community was significantly different between Tollesbury MR and all other sites except for Orplands MR. The most dominant vegetation species recorded at all natural sites and Northey Island MR was Atriplex portulacoides (NVC code: SM14), representative of the mid-low marsh zone (Table 3; Supplementary Table 1). Another mid-low marsh species, Puccinellia maritima (NVC code: SM13), was the most dominant vegetation at Orplands MR (Table 3; Supplementary Table 1). Tollesbury MR was the only site where the vegetation community composition was predominantly pioneer species (Spartina anglica, NVC code: SM8; Table 3; Supplementary Table 1). Marsh zone and NVC community were highly positively correlated with each other (r = 0.86; t = 14.10; p< 0.001), and with relative tidal height.
The vegetation community at Tollesbury MR, described by the Shannon-Weiner Diversity Index, was the least diverse (Table 3) and significantly lower in diversity than all other sites, except for Northey Island MR. The natural site at Orplands had the highest vegetation diversity (Table 3) which was significantly higher than all MR sites. Mean species richness recorded at Tollesbury MR (1.75 ± 0.62; Table 3) was significantly lower than all three natural sites. Tollesbury MR was the only MR site to differ significantly to natural sites, and otherwise, there was no significant difference between natural sites or MR sites. The MR site at Northey Island had the next lowest mean species richness (3.70 ± 1.49; Table 3), yet this was still more than 2-fold higher than Tollesbury MR. Shannon-Weiner Diversity Index and species richness were highly correlated parameters (r = 0.85; t = 13.50; p< 0.001; Supplementary Figure 1).
Above-ground biomass at Tollesbury MR (17.47 ± 8.24 g m-2; Table 3), the only site predominantly composed of pioneer species, was significantly lower than all other sites. The greatest difference was observed between Tollesbury MR and the natural sites, particularly at Northey Island and Orplands which had the greatest above-ground biomass (66.53 ± 40.69 and 62.00 ± 37.76 g m-2, respectively; Table 3). The MR sites at Northey Island and Orplands did not differ significantly to any of the three natural sites, although above-ground biomass was higher for all three natural sites when compared to MR sites. Above-ground biomass showed little correlation with any other variable (Supplementary Figure 1).
3.2 Drivers of carbon stock
Four models were run to determine the key environmental drivers of carbon stock for all sites. First, drivers of carbon stock were examined across all sites. Given that Tollesbury MR had a significantly lower carbon stock than other MR sites, and so may account for the majority of variance in carbon stock, we also investigated whether drivers were consistent across all sites excluding Tollesbury MR. Drivers of carbon stock were then compared between natural and MR sites, with the exclusion of Tollesbury MR. A summary of model outputs can be seen below in Table 4.
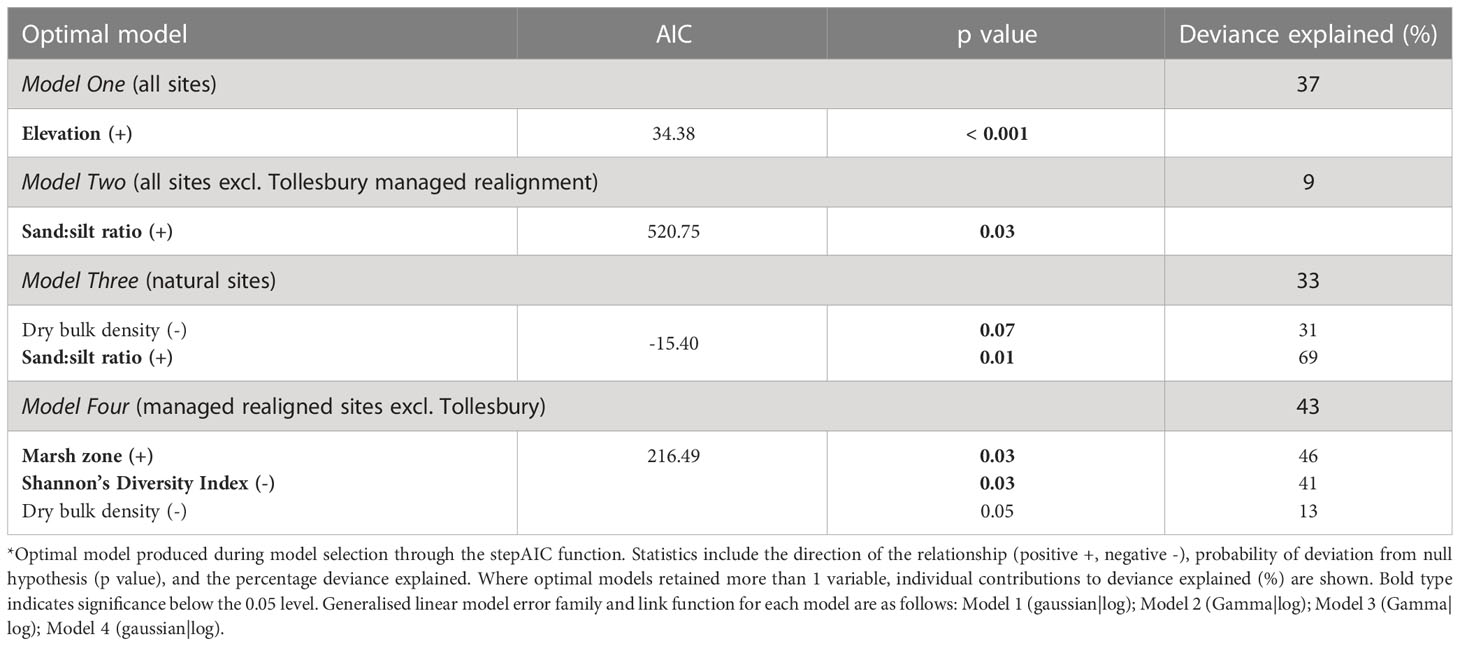
Table 4 Multivariate general and generalized linear models of carbon stock vs environmental predictor variables.
3.2.1 All sites
For all sites and management history, only relative tidal height was found to be a positive significant predictor of carbon stock (AIC = 34.38; p< 0.001; Table 4): carbon stock was lower when sampling points were lower in the tidal frame (Figure 3). The optimal model retained only relative tidal height as a variable, and explained 37 % of the observed variation in carbon stock (Table 4). In Tollesbury MR, all sampling points bar one were below Mean High Water Neap (Figure 3). Carbon stock for sites higher in the tidal frame showed no apparent relationship with relative tidal height (Figure 3). For this reason, another model was run for all sites excluding Tollesbury MR to see if other important relationships were masked.
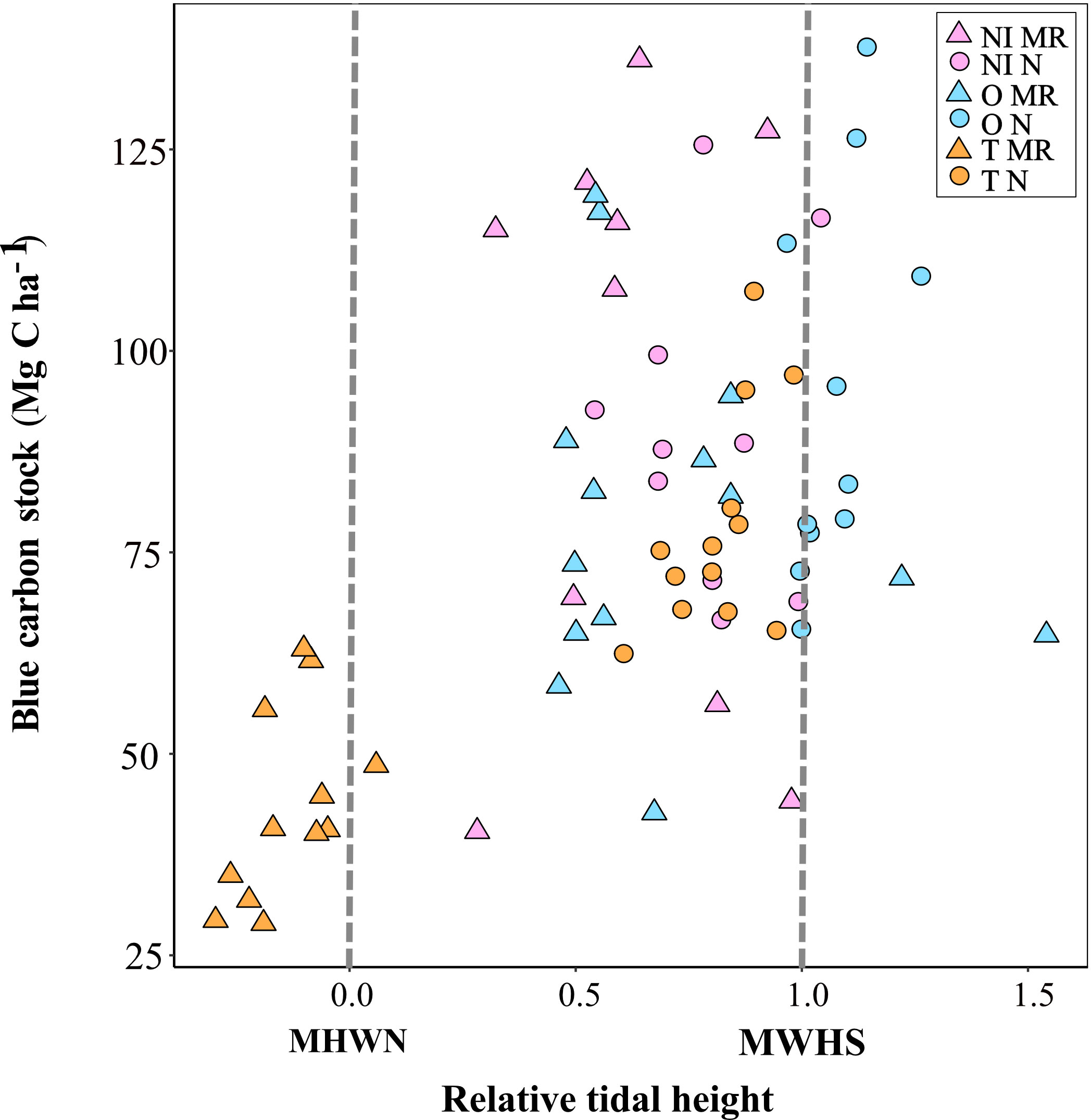
Figure 3 Carbon stock against relative tidal height for managed realigned (MR; triangle) and natural (N; circle) sites [NI, Northey Island; O, Orplands; T, Tollesbury]. Saltmarshes generally occur between mean high water neap (MHWN) and mean high water spring (MHWS) tidal levels (dashed grey lines; Lawrence et al., 2018).
Relative tidal height was positively correlated with marsh zone and NVC community (Supplementary Figure 1), so that pioneer species (e.g. Salicornia europaea and Spartina anglica) were found lower in the tidal frame than vegetation communities associated with the mid-low marsh zone (Supplementary Table 1). The highest carbon stocks were therefore found in the mid-low marsh zone. Tollesbury MR was the only site without mid-low plant communities recorded in sampling. Instead, vegetation communities recorded at all sampling points in Tollesbury MR were exclusively pioneer species Spartina anglica and Salicornia europaea.
3.2.2 Excluding Tollesbury MR
To determine drivers of carbon stock for sites above MHWN, Tollesbury MR was excluded in a second model. Relative tidal height was dropped as a predictor of carbon stock variability, whilst sand:silt ratio was retained and had a marginally significant and positive relationship with carbon stock (AIC = 520.75; p = 0.03). ratioSamples with a higher percentage of sand particles generally had higher carbon stock. All samples across sites included in this model were predominantly composed of silt (between 63 to 97 %), with the remainder composed of sand particles. The optimal model, retaining only sand:silt ratio, explained very little of the variation in carbon stock (9 %), meaning 91 % of the observed variation in carbon stock for all sites, excluding Tollesbury MR, was unaccounted for.
3.2.3 Natural versus managed realignment sites (excluding Tollesbury MR)
Excluding Tollesbury MR, we also investigated whether predictors of carbon stock differed between natural and MR sites. For natural sites, dry bulk density and sand:silt ratio were retained in the optimal model (AIC = -15.40). Of the retained variables, only sand:silt ratio was identified as a significant predictor, showing a positive relationship with carbon stock (p = 0.01). Whilst the sand:silt ratio at Northey Island and Tollesbury natural sites were similar, and the soil in all 3 natural sites was predominantly composed of silt particles, the mean sand:silt ratio at Orplands was over 2-fold greater (Table 3; Figure 4) than the other natural sites. The mean percentage of silt in the samples from Northey Island and Tollesbury natural sites was 89 % and 88 %, respectively, with the remainder composed of sand particles, whereas the mean percentage of silt for the natural site at Orplands was 77 %.
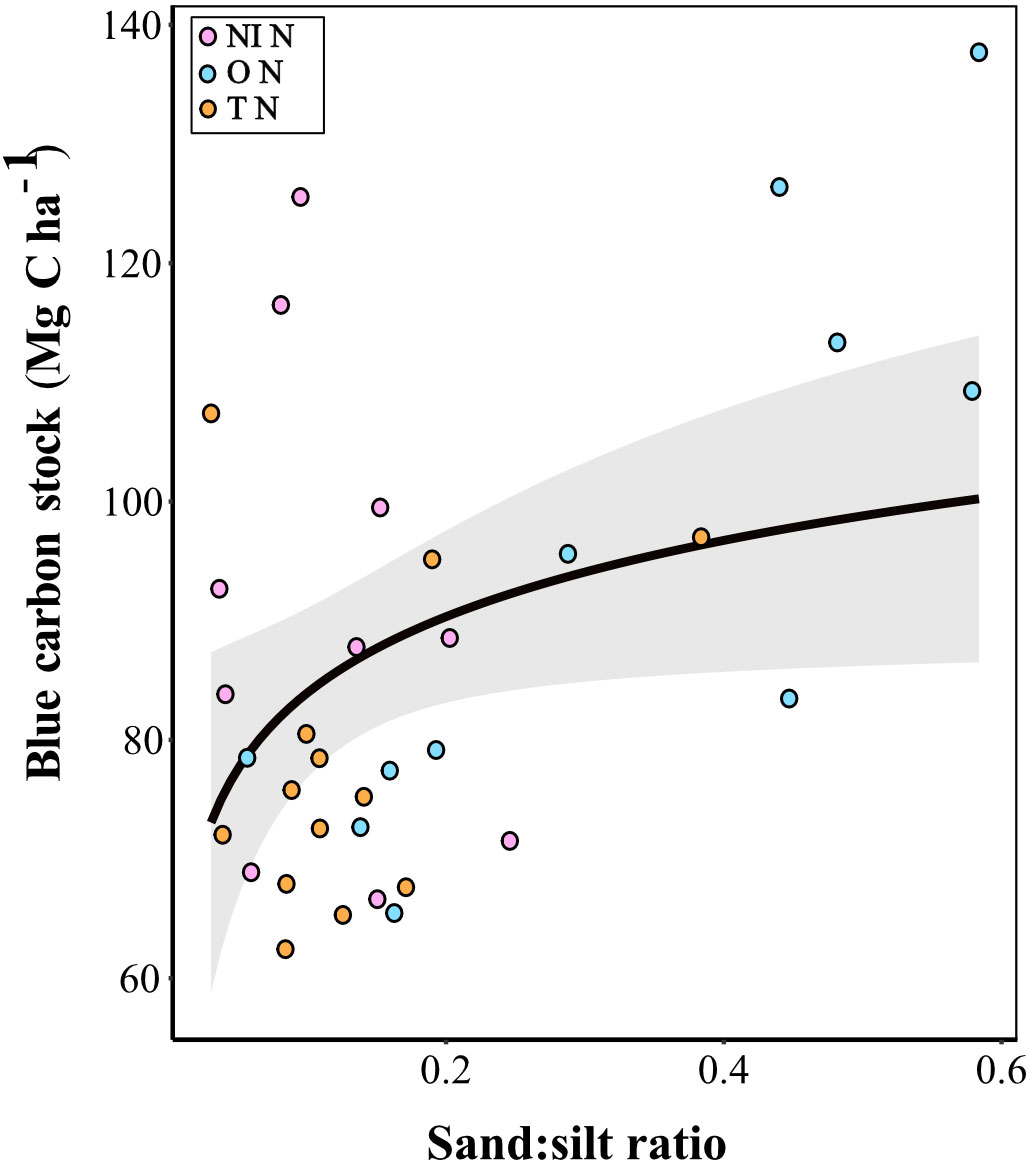
Figure 4 Carbon stock against Sand:silt ratio for natural (N) sites at Northey Island (NI; pink), Orplands (O; blue), and Tollesbury (T; orange) with a logarithmic curved line and 95% confidence interval (grey bar). The higher the sand:silt ratio, the greater the proportion of sand particles in the predominantly silty sediment.
For natural sites, sand:silt ratio was positively correlated with relative tidal height, whereby samples with a lower proportion of sand particles in the predominantly silty sediment were found lower in the tidal frame. The natural site at Orplands was the only site with a mean relative tidal height above MHWS tidal levels (Table 3; Figure 3).
The optimal model explained 33 % of the total variation in carbon stock, of which hierarchical partitioning showed that sand:silt ratio accounted for the most variation (69 %). DBD accounted for the remaining explained variation (31 %) but was an insignificant predictor of carbon stock.
Across MR sites (excluding Tollesbury), marsh zone (p = 0.03) and Shannon’s Diversity Index (p = 0.03) were significant predictors of carbon stock in the optimal model (AIC = 216.49). Soil carbon stock was higher in sampling points with a less diverse vegetation community composition, as described by Shannon’s Diversity Index (Figure 5B). Conversely, vegetation communities associated with the mid-low marsh had higher carbon stock than pioneer zones (Figure 5A). However, associated soil carbon stock within both the mid-low and pioneer marsh zones varied depending on NVC communities (Figure 5A).
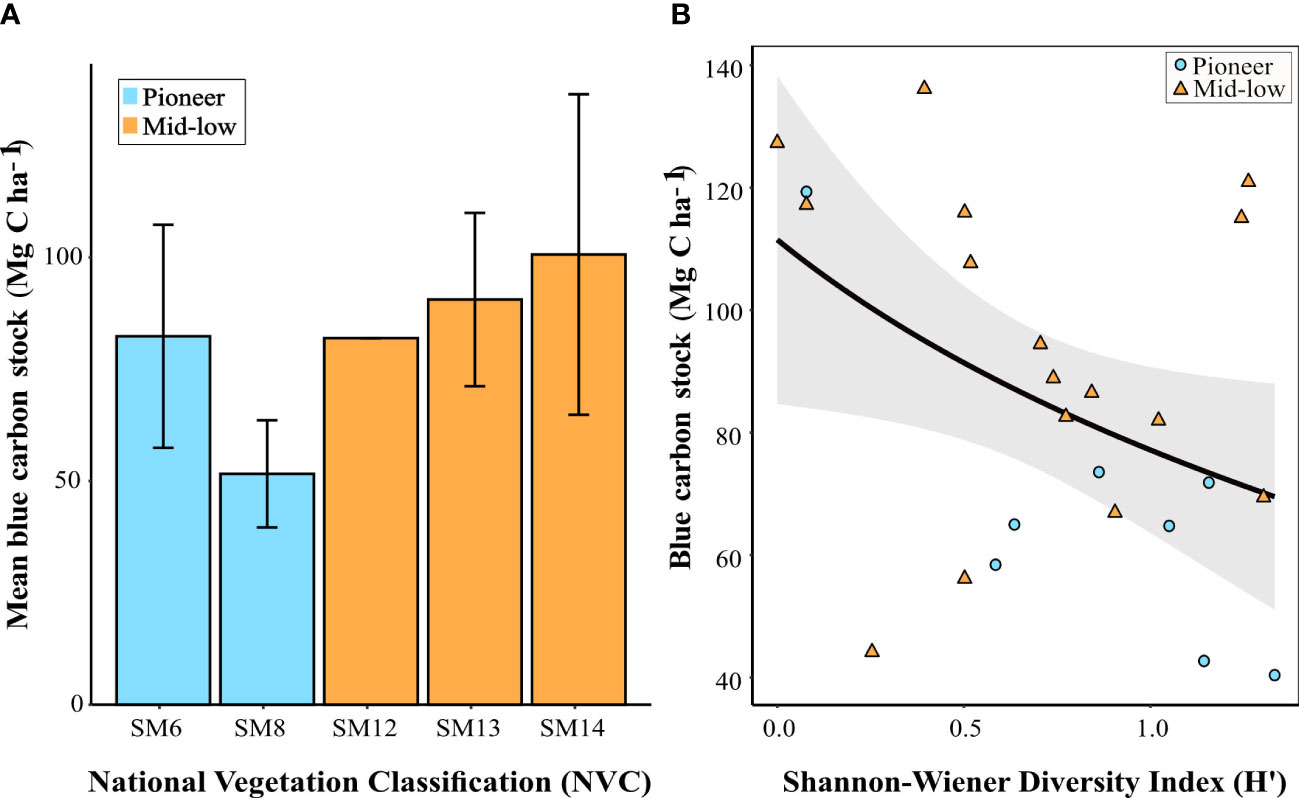
Figure 5 (A) Mean carbon stock ± standard deviation for each dominant National Vegetation Classification (NVC) community found in the managed realigned sites at Northey Island and Orplands (pioneer communities = blue; mid-low communities = orange). Number of observations varied across NVC groupings: SM6 (n = 4), SM8 (n = 4), SM12 (n = 1), SM13 (n = 8), and SM14 (n = 7). See Supplementary Table 1 for NVC categories and corresponding marsh zone; and (B) Carbon stock against Shannon-Wiener Diversity Index for Northey Island and Orplands managed realigned sites, with a logarithmic curved line and 95% confidence interval (grey bar). Data points correspond to marsh zones (pioneer = blue circle; and mid-low = orange triangle). The higher the H’ value, the more diverse the vegetation community.
At Northey Island and Orplands MR sites, the following NVC communities were recorded: SM6, SM8, SM12, SM13, and SM14 (Figure 5A; Supplementary Table 1). Of these, SM6 and SM8 communities dominated by Spartina anglica and Salicornia europaea, respectively, are pioneer communities. The remaining communities (SM12, SM13, and SM14) are associated with the mid-low marsh zone (Figure 5A; Supplementary Table 1). The mean carbon stock in pioneer communities (66.98 ± 24.45 Mg C ha-1) was lower than in vegetation communities associated with the mid-low marsh (94.44 ± 26.92 Mg C ha-1) at these sites (Figure 5A; Supplementary Table 1). SM14 communities, dominated by Atriplex portulacoides (Supplementary Table 1), had the lowest mean diversity index value (Figure 5B) of all communities present in Northey Island and Orplands MR sites, but were associated with the greatest mean soil carbon stock (100.64 ± 35.82 Mg C ha-1; Figure 5A). Above-ground biomass showed a marginally significant correlation with NVC community (r = 0.42; t = 2.14; p = 0.04) for the MR model excluding Tollesbury (Table 4), but was dropped from the optimal model with no loss in explanatory power.
Dry bulk density was also retained in the optimal model but had a marginally insignificant, negative relationship with carbon stock (p = 0.05). The optimal model explained 43 % of the variation in carbon stock. Biological characteristics relating to plant community composition and diversity were responsible for 87 % of the variation (marsh zone: 46 %; Shannon’s Diversity Index: 41 %), whilst dry bulk density explained 13 %.
4 Discussion
This study demonstrates that blue carbon stocks in managed realigned sites exhibit low spatial variability at an estuarine scale, with important implications for the inclusion of restoration schemes in climate change mitigation strategies. However, carbon stocks are significantly higher in MR sites elevated above MHWN tidal levels, which are associated with more mature saltmarsh plant communities. Sedimentary drivers appear more influential in explaining the carbon stock variability between natural saltmarshes. These findings provide a useful insight into environmental drivers that control blue carbon stocks in both natural and MR saltmarshes, which can be used to inform future restoration schemes that aim to maximise climate change mitigation.
We found that surficial (top 30 cm) soil carbon stocks in our MR sites become equivalent to natural saltmarsh stocks after 25 – 29 years post-breaching, but importantly only when sites were above MHWN tidal levels. A threshold relationship existed where mean carbon stocks almost doubled beyond MHWN elevations. The timescale to develop equivalent carbon stocks to natural saltmarshes is faster than reported in other studies for MR sites (Burden et al., 2013; Burden et al., 2019) and restored wetlands in general (Moreno-Mateos et al., 2012). We attribute this to the vegetation community composition, an important biological indicator of the blue carbon capacity of saltmarshes (Rogers et al., 2019b). Even the presence of a single key species is known to enhance soil carbon stocks in restored marshes (Zhao et al., 2018). Specifically, at MR sites above MHWN tidal levels, we observed the successful colonization and establishment of a considerable proportion of late-successional plant species from the local species pool, and site dominance by key mid-low plant species, such as Atriplex portulacoides, in comparison to the dominance of pioneer communities that often characterise MR sites (Garbutt et al., 2006; Mossman et al., 2012a; Brooks et al., 2015). Key plant species (e.g., Atriplex portulacoides) therefore appear to rapidly enhance the carbon storage value of MR sites once suitable inundation characteristics are reached.
The lowest carbon stocks were found at Tollesbury MR, the only site with a mean relative tidal height below MHWN tidal levels (Figure 3) at the lower limit of pioneer marsh establishment (Adam, 2002; Balke et al., 2016; Lawrence et al., 2018; Hudson et al., 2021). Over a decade prior to our research, Adams et al. (2012) also observed Tollesbury MR to be dominated by pioneer communities of Spartina anglica and Salicornia europaea, indicative of the site’s low position in the tidal frame. Across Great British saltmarshes, Smeaton et al. (2022) reported lower soil organic carbon stocks for pioneer communities when compared to communities associated with higher elevations, such as mid-low and high marsh zones. For MR sites higher in the tidal frame than MHWN tidal levels, saltmarsh plant species from the local species pool are expected to quickly colonize following pioneer establishment (Adams et al., 2012) – on timescales as short as 4 years (Davy et al., 2011; Mossman et al., 2012c). Even 25 years post-restoration, Tollesbury MR remains below MHWN tidal levels. Time since restoration therefore is not necessarily a strong predictor of carbon stock in MR sites (Adams et al., 2012; Abbott et al., 2019; Burden et al., 2019). The blue carbon potential of proposed MR schemes thus need to account for local scale controls of carbon variability in MR sites, which is of particular importance when including coastal wetland restoration in climate change mitigation strategies through robust blue carbon estimates (Hayes et al., 2017).
Unless action is taken to increase the ‘elevation capital’ (Cahoon et al., 2019; Langston et al., 2021) of planned MR sites prior to breaching, such as through the use of dredged estuarine material (e.g. French, 2006; Stagg and Mendelssohn, 2010; Kadiri et al., 2011), MR sites are often lower in the tidal frame than natural saltmarshes due to historical subsidence and inhibited sediment supply from the tide (Oosterlee et al., 2018). The relationship between elevation in the tidal frame and redox potential is well established for MR sites across the UK (Davy et al., 2011; Mossman et al., 2012a; Mossman et al., 2020). More frequent and prolonged inundation of low elevation sites, coupled with poor drainage of anoxic sediments, means reduced gas exchange, and subsequent low sediment redox potential can characterise MR sites (Castillo et al., 2000; Garbutt et al., 2006; Davy et al., 2011; Mossman et al., 2012a; Mossman et al., 2020). Such conditions can inhibit the colonization of plant communities associated with mid-low and high marsh zones, favoring more tolerant pioneer communities (French, 2006; Wolters et al., 2008; Mossman et al., 2012c), as is the case for Tollesbury MR. If the elevation of Tollesbury MR is unable to increase, whether naturally through sediment trapping and accretion or artificially, pioneer communities are likely to persist (Garbutt et al., 2006). Sediment additions that raise elevations of MR sites comparable with mid to high marsh zones have been successful in restoring ecosystem functionality, and may also encourage long-term resilience to accelerated sea-level rise, particularly in regions with low sediment supply (Stagg and Mendelssohn, 2010; Langston et al., 2021).
The capacity of a newly restored saltmarsh to increase elevation and facilitate vegetation development is usually a key aspect of restoration (Davy et al., 2011), yet often relies on a suitable elevation of the tidal flat for pioneer vegetation to establish and for the positive feedback of elevation gain to commence (D'Alpaos, 2011). For MR sites low in the tidal frame, considerable sediment accretion is needed to raise the surface above MHWN tidal levels to elevations suited for vegetation colonization (Garbutt et al., 2006; Davy et al., 2011). When hydrology is restored through breaching at MR sites, the resulting accommodation space creates a settling basin for sediments. Rapid elevation gain can ensue provided a sufficient sediment supply exists (Liu et al., 2021) and that shallow compaction does not offset elevation gain (Saintilan et al., 2022). Soil carbon stocks in the early stages of MR are also developed if the deposited sediment is rich in organic material (Friess et al., 2012; Hansen et al., 2017; Wollenberg et al., 2018). Sediment accumulation helps to ameliorate redox conditions via elevation gain and allows the colonization of mid-low and high marsh species associated with a higher carbon stock potential (Wollenberg et al., 2018; Mossman et al., 2020; Smeaton et al., 2022). Sediment supply, alongside other parameters, such as breach width, may also account for some of the unexplained variance in the data.
Saltmarshes in southeastern England (the study region for this research) appear to have limited sediment supply (Ladd et al., 2019). In the Blackwater Estuary, suspended sediment concentrations (~ 50 – 150 mg/l), and thus sediment supply, are relatively low in comparison to other UK estuaries such as the Parrett Estuary in southwestern England which has a suspended sediment concentration in the range of 1,000 – 10,000 mg/l (Manning et al., 2010; Spearman, 2011; Mossman et al., 2022). Tollesbury MR was implemented at initial elevations lower in the tidal frame than Northey Island and Orplands MR (Wolters et al., 2005). The lower sediment supply experienced in the region (Ladd et al., 2019), exacerbated by the construction of a counter sea wall which restricted tidal inundation and further dampened sediment supply (Garbutt et al., 2008), likely explains why Tollesbury MR remains in a low-elevation state despite 25 years since restoration. When saltmarsh restoration occurs at extremely low elevations in the tidal frame, such as Tollesbury MR, sediment supply should thus be a key consideration in forecasting the likely development of the MR site and carbon stocks. Furthermore, reliable sediment supply is required to ensure the restoration success of saltmarshes under projected sea-level rise (Liu et al., 2021), regardless of initial elevation, which is vital in securing the longevity of vegetation communities and associated carbon storage.
Studies on natural blue carbon habitats (saltmarshes, seagrasses, and mangroves) report high spatial heterogeneity at the estuarine level (Broek et al., 2016; Ricart et al., 2020; Suello et al., 2022). Conversely, we observed relatively low spatial variability in carbon stock among natural and MR saltmarshes in the Blackwater Estuary, with the exception of Tollesbury MR. We highlight the variability seen between Tollesbury MR and the other MR sites in the estuary is mostly linked to the vegetation community composition, whereas sediment properties are a more influential driver for natural saltmarshes.
Numerous studies have reported higher carbon stocks associated with fine-grained sediments, such as clay and silt, due to their enhanced preservation capacity over coarser sediments (Kelleway et al., 2016; Zhao et al., 2018; Ford et al., 2019; Rogers et al., 2019b). However, we found an opposite relationship where a greater proportion of sand particles in the soil were associated with higher carbon stocks in natural saltmarshes. It is important to note that soil samples across all sites were predominantly composed of silt, reflective of the wider Essex region (Ford et al., 2016). Sand particles are coarser than silt particles, with higher hydraulic conductivity and drainage capacity (Crooks et al., 2002). These factors are associated with better aerated sediment and are likely to enhance redox potential (Mossman et al., 2012a). This could potentially create optimal conditions for greater above-ground biomass whereby greater carbon sequestration is supported mostly by autochthonous contributions (Kelleway et al., 2016). Indeed, we found the highest carbon stocks in the natural saltmarsh at Orplands, which not only had the greatest proportion of sand particles, but also exhibited among the greatest above-ground biomass and was the only site above MHWS tidal levels. The high elevation of this site suggests a lower opportunity for allochthonous carbon inputs, coupled with a lower capacity for tidal export of autochthonous carbon, due to reduced inundation frequency at high elevations (Kelleway et al., 2016). Similarly, in seagrass habitats, the expected positive relationship between fine-grained sediment and carbon stocks was poor in meadows of higher biomass when autochthonous contributions were high (Serrano et al., 2016).
The highest carbon stocks in MR sites were associated with mid-low plant communities, specifically Atriplex portulacoides, which was the dominant plant community at Northey Island, the MR site with the greatest carbon stock and a vegetation assemblage most similar to natural saltmarshes in the estuary. These findings corroborate a previous study (Adams et al., 2012) which attributed the variability in carbon stock among MR sites in the Blackwater Estuary to the vegetation community composition. Communities of A. portulacoides form dominant, almost monospecific stands (Penk et al., 2020), explaining why higher carbon stocks were associated with lower plant diversities for Northey Island and Orplands MR sites. Long-term monitoring at a restored saltmarsh site showed dominant plant species controlled and enhanced biomass, whilst reducing overall species richness, supporting the concept that greater diversity does not necessarily equate to enhanced ecosystem functioning (Doherty et al., 2011). Furthermore, the shrub A. portulacoides has a complex branched and deep root system (Decuyper et al., 2014), characteristics associated with greater belowground productivity and root biomass (Adams et al., 2012) which contribute to enhanced carbon stocks (Chmura et al., 2003; Ford et al., 2016). For example, Puccinellia maritima, a shallow-rooted grass which forms mid-low plant communities together with other simple-rooted saltmarsh vegetation (Ford et al., 2019), was the dominant species at Orplands MR which had a lower carbon stock than Northey Island MR. As such, the role of belowground root biomass may represent an important environmental driver of spatial variability in carbon stocks between MR saltmarshes and should be explored in further studies. Over half of organic matter production in saltmarsh plants occurs in roots and rhizomes that are buried within the soil (Duarte et al., 2013) and lower dry bulk densities have been associated with high biomass and abundance of roots in saltmarsh plant species (Santini et al., 2019), hence the inverse relationship when carbon stock is higher at lower bulk densities observed across coastal blue carbon habitats (Dahl et al., 2016; Barry et al., 2018; Gao et al., 2019; Lima et al., 2020). In turn, root biomass is known to contribute significantly to carbon stocks (Jones and Donnelly, 2004; Ford et al., 2019; Santini et al., 2019; Chen et al., 2022) and has been shown to be significantly lower in pioneer communities than for plant communities at higher elevations across UK saltmarshes (Harvey et al., 2019).
It has been reported that plant communities in restored marshes differ from those found in natural saltmarshes, even after decades (Garbutt and Wolters, 2008; Moreno-Mateos et al., 2012; Mossman et al., 2012a). Such differences are thought to hinder the ability of restored marshes to reach functional equivalence with natural marshes (Wolters et al., 2005; Mossman et al., 2012a; Burden et al., 2019). However, we found that although the vegetation communities at MR sites had a lower species diversity, species richness, and above-ground biomass than natural sites, these biological parameters did not differ significantly between natural and MR saltmarshes, nor did carbon stock, with the exception of Tollesbury MR. Our findings support those of Santini et al. (2019) who reported soil carbon stocks for the top 30 cm were similar between natural and regenerated saltmarshes in New South Wales, Australia, after 20 years, despite above-ground biomass being higher in natural marshes. The presence of key plant species, or dominant species within a community (e.g., Atriplex portulacoides in this study), is highly influential for productivity, and consequently carbon stocks, in restored marshes (Doherty et al., 2011), and may hold more value than developing equivalent vegetation communities to nearby natural saltmarshes, depending on the overall goal of restoration schemes. However, A. portulacoides is restricted to areas of high redox potential and elevation with well-drained soils (Garbutt et al., 2008; Davy et al., 2011; Cott et al., 2013; Mossman et al., 2020) as it lacks the structural adaptations for flooding (Armstrong et al., 1985), explaining its absence from Tollesbury MR which sits too low in the tidal frame. Experimental manipulations of topography at MR sites in southeastern England found A. portulacoides to respond well to raised elevations (Mossman et al., 2020). Slight increases in elevation were sufficient to ameliorate redox conditions for the colonization and survival of mid-marsh communities (Mossman et al., 2020). Enhanced soil drainage, whether from increased elevation, or the creation of small creeks (Wolters et al., 2005; Lawrence et al., 2018), will improve redox conditions (Mossman et al., 2020). In turn, primary productivity will increase (Stagg and Mendelssohn, 2010), boosting autochthonous carbon inputs, and providing a source of plant litter that contributes to carbon stocks (Kadiri et al., 2011). Such interventions (e.g. enhanced elevation and/or the creation of creeks/shallow lagoons) have been shown to be cost-effective and efficient in altering plant communities in MR sites (Lawrence et al., 2022).
In addition, wave attenuation for coastal protection and the provision of heterogeneous habitat for biodiversity enhancement, the primary goals of managed realignment in the UK, benefit from dense vegetation canopies which are more associated with mid-low and high marsh plant communities (Levin and Talley, 2002; Möller and Spencer, 2002). A. portulacoides is a prominent species for saltmarshes in southern UK (Mossman et al., 2012a), where our study sites are located. It represents a key species for MR schemes in the region to enhance carbon storage and supports the theory that ecosystem functioning may not be solely driven by diversity, but instead dominant species (Keer and Zedler, 2002). A. portulacoides is also an important species for promoting saltmarsh stabilisation and protection as the root systems provide high resistance to erosion (Chen et al., 2019). However, Ford et al. (2016) demonstrated that saltmarsh plant diversity enhances sediment stability and erosion control, particularly in areas of sandy soils which are more prone to erosion, therefore trade-offs in ecosystem services must be considered in respect to the aim of restoration.
5 Conclusion and recommendations
Climate change mitigation has become a key goal for coastal wetland restoration projects, and blue carbon represents an important facet of future managed realignment schemes. We found carbon stocks in MR and natural saltmarsh sites in the Blackwater Estuary exhibited a relatively low spatial variability, with the exception of Tollesbury MR. Investigating a range of environmental drivers, we consider the comparatively low carbon stock at Tollesbury MR to be governed by local-scale controls including relative tidal height and vegetation community composition. The homogeneity of soil carbon stocks across MR sites has implications for estimating the climate change mitigation potential of proposed MR schemes.
Overall, we found relative tidal height to be a key driver of the capacity for MR sites to develop equivalent carbon stocks to natural marshes. We attributed this to sediment redox potentials and plant community composition, an important indicator for blue carbon capacity in saltmarshes (Rogers et al., 2019b). The MR site at Tollesbury sits too low in the tidal frame (below MHWN tidal levels) and we consider the corresponding low redox potential to have created unsuitable conditions for mid-low marsh communities, and key species for carbon storage such as A. portulacoides, to establish. The dominant presence of pioneer communities at this site 25 years after restoration has negative consequences for saltmarsh development and carbon storage. Biological characteristics explained the majority of the observed variability in carbon stocks between MR sites, whilst sedimentary characteristics appear to be more influential for carbon stocks in natural saltmarshes, although we consider these to have been mediated by plant dynamics.
In agreement with the recently published ‘Saltmarsh restoration handbook’ for the UK and Ireland (Hudson et al., 2021), we propose that relative tidal height is one of the most important design considerations for MR schemes. Our findings suggest that the elevation of MR sites should surpass mean high water neap (MHWN) tidal levels for significant carbon stocks, comparable to that of natural saltmarshes, to develop. We recommend that future managed realigned schemes, particularly those that aim to maximise blue carbon stocks, should restore saltmarsh habitat above MHWN tidal levels, where possible, or select restoration sites that can be raised to MHWN elevations, whether naturally through sedimentary processes that rely on levels of high sediment supply or artificially (e.g., via dredged material). This will also provide benefits for coastal protection and biodiversity enhancement. Careful consideration of local sediment supply and elevation capital should also be taken into account to ensure the longevity of restored saltmarshes and their carbon stocks, particularly under projected sea-level rise. Finally, topographic manipulations may also provide an alternative to enhance drainage conditions and improve sediment redox potentials to create suitable conditions for the establishment of successional saltmarsh plant species. If climate change mitigation is the aim of restoration, schemes should pay closer attention to the establishment of key species (e.g. Atriplex portulacoides in our study area) and communities representative of the area, with measures to encourage their establishment.
Data availability statement
The datasets presented in this study can be found in online repositories. The names of the repository/repositories and accession number(s) can be found below: https://doi.org/10.6084/m9.figshare.21587940 [figshare].
Author contributions
LM came up with the concept of the research, and all authors contributed to discussions on the research approach throughout. LM and CL collected the field data. LM analyzed all laboratory samples and carried out calculations to estimate soil organic carbon stocks with the guidance of AB. LM undertook data analysis with support from CL. LM wrote the first draft of the manuscript, and all authors contributed to revisions and approved the submitted version.
Funding
This research forms part of an Adapting to the Challenges of a Changing Environment (ACCE) Doctoral Training Partnership funded by the Natural Environment Research Council (NERC), part of United Kingdom Research and Innovation (UKRI) (NE/L002450/1). CL, AB and RG were funded by NERC grant NE/R010846/1 (Carbon Storage in Intertidal Environments; C-SIDE).
Acknowledgments
We thank the National Trust, Natural England, and Richard Playle for granting access to the study sites. We are grateful to Hannah Mossman, Kate Spencer and Angus Garbutt for providing contacts for site permission. We would like to thank Mike Beckwith (University of York) for technical support in field data collection, Matt Pickering (University of York) and Maria Gehrels (University of York) for technical support with the running of the elemental and particle size analyzers. We would also like to thank Shannon Burke (University College Dublin) for guidance in carbon stock calculations and Shane Goulding for helping on fieldwork. Finally, we thank the Editor and reviewers for providing helpful comments which have improved the manuscript.
Conflict of interest
The authors declare that the research was conducted in the absence of any commercial or financial relationships that could be construed as a potential conflict of interest.
Publisher’s note
All claims expressed in this article are solely those of the authors and do not necessarily represent those of their affiliated organizations, or those of the publisher, the editors and the reviewers. Any product that may be evaluated in this article, or claim that may be made by its manufacturer, is not guaranteed or endorsed by the publisher.
Supplementary material
The Supplementary Material for this article can be found online at: https://www.frontiersin.org/articles/10.3389/fmars.2023.1106607/full#supplementary-material
References
Abbott K. M., Elsey-Quirk T., DeLaune R. D. (2019). Factors influencing blue carbon accumulation across a 32-year chronosequence of created coastal marshes. Ecosphere 10 (8), e02828. doi: 10.1002/ecs2.2828
ABPmer (2022) Online managed realignment guide (OMRG). Available at: https://www.abpmer.net/omreg/ (Accessed October 23, 2022).
Adam P. (2002). Saltmarshes in a time of change. Environ. Conserv. 29 (1), 39–61. doi: 10.1017/S0376892902000048
Adams C. A., Andrews J. E., Jickells T. (2012). Nitrous oxide and methane fluxes vs. carbon, nitrogen and phosphorous burial in new intertidal and saltmarsh sediments. Sci. Total Environ. 434, 40–251. doi: 10.1016/j.scitotenv.2011.11.058
Armstrong W., Wright E. J., Lythe S., Gaynard T. J. (1985). Plant zonation and the effects of the spring-neap tidal cycle on soil aeration in a Humber salt marsh. J. Ecol. 73 (1), 323–339. doi: 10.2307/2259786
Austin W. E. N., Smeaton C., Ruranska P., Paterson D. M., Skov M. W., Ladd C. J. T., et al. (2022). “Carbon storage in UK intertidal environments,” in Challenges in estuarine and coastal science: Estuarine and Coastal Sciences Association 50th Anniversary Volume. Eds. Humphreys J., Little S. (Pelagic Publishing). doi: 10.53061/STPP2268
Balke T., Stock M., Jensen K., Bouma T. J., Kleyer M. (2016). A global analysis of the seaward salt marsh extent: The importance of tidal range. Water Resour. Res. 52 (5), 3775–3786. doi: 10.1002/2015WR018318
Barbier E. B., Hacker S. D., Kennedy C., Koch E. W., Stier A. C., Silliman B. R. (2011). The value of estuarine and coastal ecosystem services. Ecol. Monogr. 81 (2), 169–193. doi: 10.1890/10-1510.1
Barry S. C., Bianchi T. S., Shields M. R., Hutchings J. A., Jacoby C. A., Frazer T. K. (2018). Characterizing blue carbon stocks in thalassia testudinum meadows subjected to different phosphorus supplies: A lignin biomarker approach. Limnology Oceanography 63 (6), 2630–2646. doi: 10.1002/lno.10965
Bertram C., Quaas M., Reusch T. B., Vafeidis A. T., Wolff C., Rickels W. (2021). The blue carbon wealth of nations. Nat. Climate Change 11, 704–709. doi: 10.1038/s41558-021-01089-4
Broek M. V. D., Temmerman S., Merckx R., Govers G. (2016). Controls on soil organic carbon stocks in tidal marshes along an estuarine salinity gradient. Biogeosciences 13 (24), 6611–6624. doi: 10.5194/bg-13-6611-2016
Brooks K. L., Mossman H. L., Chitty J. L., Grant A. (2015). Limited vegetation development on a created salt marsh associated with over-consolidated sediments and lack of topographic heterogeneity. Estuaries Coasts 38 (1), 325–336. doi: 10.1007/s12237-014-9824-3
Burden A., Garbutt A., Evans C. D. (2019). Effect of restoration on saltmarsh carbon accumulation in Eastern England. Biol. Lett. 15 (1). doi: 10.1098/rsbl.2018.0773
Burden A., Garbutt R. A., Evans C. D., Jones D. L., Cooper D. M. (2013). Carbon sequestration and biogeochemical cycling in a saltmarsh subject to coastal managed realignment. Estuarine Coast. Shelf Sci. 120, 12–20. doi: 10.1016/j.ecss.2013.01.014
Cahoon D. R., Lynch J. C., Roman C. T., Schmit J. P., Skidds D. E. (2019). Evaluating the relationship among wetland vertical development, elevation capital, sea-level rise, and tidal marsh sustainability. Estuaries Coasts 42, 1–15. doi: 10.1007/s12237-018-0448-x
Castillo J. M., Fernández-Baco L., Castellanos E. M., Luque C. J., Figueroa M. E., Davy A. J. (2000). Lower limits of spartina densiflora and s. maritima in a Mediterranean salt marsh determined by different ecophysiological tolerances. J. Ecol. 88 (5), 801–812. doi: 10.1046/j.1365-2745.2000.00492.x
Chausson A., Turner B., Seddon D., Chabaneix N., Girardin C. A., Kapos V., et al. (2020). Mapping the effectiveness of nature-based solutions for climate change adaptation. Global Change Biol. 26 (11), 6134–6155. doi: 10.1111/gcb.15310
Chen G., Bai J., Yu L., Chen B., Zhang Y., Liu G., et al. (2022). Effects of ecological restoration on carbon sink and carbon drawdown of degraded salt marshes with carbon-rich additives application. Land Degradation Dev. 33 (12), 2103–2114. doi: 10.1002/ldr.4306
Chen Y., Thompson C., Collins M. (2019). Controls on creek margin stability by the root systems of saltmarsh vegetation, beaulieu estuary, southern England. Anthropocene Coasts 2 (1), 21–38. doi: 10.1139/anc-2018-0005
Chmura G. L., Anisfeld S. C., Cahoon D. R., Lynch J. C. (2003). Global carbon sequestration in tidal, saline wetland soils. Global biogeochemical cycles 17 (4). doi: 10.1029/2002GB001917
Cott G. M., Reidy D. T., Chapman D. V., Jansen M. A. (2013). Waterlogging affects the distribution of the saltmarsh plant atriplex portulacoides (L.) aellen. Flora-Morphology Distribution Funct. Ecol. Plants 208 (5-6), 336–342. doi: 10.1016/j.flora.2013.04.006
Crooks S., Schutten J., Sheern G. D., Pye K., Davy A. J. (2002). Drainage and elevation as factors in the restoration of salt marsh in Britain. Restor. Ecol. 10 (3), 591–602. doi: 10.1046/j.1526-100X.2002.t01-1-02036.x
D'Alpaos A. (2011). The mutual influence of biotic and abiotic components on the long-term ecomorphodynamic evolution of salt-marsh ecosystems. Geomorphology 126 (3-4), 269–278. doi: 10.1016/j.geomorph.2010.04.027
Dahl M., Deyanova D., Gütschow S., Asplund M. E., Lyimo L. D., Karamfilov V., et al. (2016). Sediment properties as important predictors of carbon storage in zostera marina meadows: A comparison of four European areas. PLoS One 11 (12), e0167493. doi: 10.1371/journal.pone.0167493
Davy A. J., Brown M. J., Mossman H. L., Grant A. (2011). Colonization of a newly developing salt marsh: Disentangling independent effects of elevation and redox potential on halophytes. J. Ecol. 99 (6), 1350–1357. doi: 10.1111/j.1365-2745.2011.01870.x
Decuyper M., Slim P. A., Loon-Steensma V., Jantsje M. (2014). Dendrochronology of atriplex portulacoides and artemisia maritima in wadden Sea salt marshes. J. Coast. Conserv. 18 (3), 279–284. doi: 10.1007/s11852-014-0317-0
Doherty J. M., Callaway J. C., Zedler J. B. (2011). Diversity–function relationships changed in a long-term restoration experiment. Ecol. Appl. 21 (6), 2143–2155. doi: 10.1890/10-1534.1
Doody J. P. (2013). Coastal squeeze and managed realignment in southeast England, does it tell us anything about the future? Ocean Coast. Manage. 79, 34–41. doi: 10.1016/j.ocecoaman.2012.05.008
Drexler J. Z., Davis M. J., Woo I., de la Cruz S. (2020). Carbon sources in the sediments of a restoring vs. historically unaltered salt marsh. Estuaries Coasts 43 (6), 1345–1360. doi: 10.1007/s12237-020-00748-7
Duarte C. M., Agusti S., Barbier E., Britten G. L., Castilla J. C., Gattuso J. P., et al. (2020). Rebuilding marine life. Nature 580, 39–51. doi: 10.1038/s41586-020-2146-7
Duarte C. M., Dennison W. C., Orth R. J., Carruthers T. J. (2008). The charisma of coastal ecosystems: Addressing the imbalance. Estuaries Coasts 31 (2), 233–238. doi: 10.1007/s12237-008-9038-7
Duarte C. M., Losada I. J., Hendriks I. E., Mazarrasa I., Marbà N. (2013). The role of coastal plant communities for climate change mitigation and adaptation. Nat. Climate Change 3 (11), 961–968. doi: 10.1038/nclimate1970
Duarte C. M., Middelburg J. J., Caraco N. (2005). Major role of marine vegetation on the oceanic carbon cycle. Biogeosciences 2 (1), 1–8. doi: 10.5194/bg-2-1-2005
Ford H., Garbutt A., Duggan-Edwards M., Harvey R., Ladd C., Skov M. W. (2019). Large-Scale predictions of salt-marsh carbon stock based on simple observations of plant community and soil type. Biogeosciences 16 (2), 425–436. doi: 10.5194/bg-16-425-2019
Ford H., Garbutt A., Ladd C., Malarkey J., Skov M. W. (2016). Soil stabilization linked to plant diversity and environmental context in coastal wetlands. J. vegetation Sci. 27 (2), 259–268. doi: 10.1111/jvs.12367
French P. W. (2006). Managed realignment–the developing story of a comparatively new approach to soft engineering. Estuarine Coast. Shelf Sci. 67 (3), 409–423. doi: 10.1016/j.ecss.2005.11.035
Friess D. A., Spencer T., Smith G. M., Möller I., Brooks S. M., Thomson A. G. (2012). Remote sensing of geomorphological and ecological change in response to saltmarsh managed realignment, the wash, UK. Int. J. Appl. Earth Observation Geoinformation 18, 57–68. doi: 10.1016/j.jag.2012.01.016
Gao Y., Zhou J., Wang L., Guo J., Feng J., Wu H., et al. (2019). Distribution patterns and controlling factors for the soil organic carbon in four mangrove forests of China. Global Ecol. Conserv. 17, e00575. doi: 10.1016/j.gecco.2019.e00575
Garbutt R. A., Reading C. J., Turk A., Yates M., Rothery P. (2008). Botanical and sediment monitoring of the tollesbury managed realignment site and adjacent saltmarshes. Managed realignment at Tollesbury Centre Ecol. Hydrology Rep., 47.
Garbutt R. A., Reading C. J., Wolters M., Gray A. J., Rothery P. (2006). Monitoring the development of intertidal habitats on former agricultural land after the managed realignment of coastal defences at tollesbury, Essex, UK. Mar. pollut. Bull. 53 (1-4), 155–164. doi: 10.1016/j.marpolbul.2005.09.015
Garbutt A., Wolters M. (2008). The natural regeneration of salt marsh on formerly reclaimed land. Appl. Vegetation Sci. 11 (3), 335–344. doi: 10.3170/2008-7-18451
Hansen K., Butzeck C., Eschenbach A., Gröngröft A., Jensen K., Pfeiffer E. M. (2017). Factors influencing the organic carbon pools in tidal marsh soils of the Elbe estuary (Germany). J. Soils Sediments 17 (1), 47–60. doi: 10.1007/s11368-016-1500-8
Harvey R. J., Garbutt A., Hawkins S. J., Skov M. W. (2019). No detectable broad-scale effect of livestock grazing on soil blue-carbon stock in salt marshes. Front. Ecol. Evol. 7. doi: 10.3389/fevo.2019.00151
Hayes M. A., Jesse A., Hawke B., Baldock J., Tabet B., Lockington D., et al. (2017). Dynamics of sediment carbon stocks across intertidal wetland habitats of moreton bay, Australia. Global Change Biol. 23 (10), 4222–4234. doi: 10.1111/gcb.13722
Howard J., Hoyt S., Isensee K., Telszewski M., Pidgeon E. (Eds.) (2014). “Coastal blue carbon: Methods for assessing carbon stocks and emissions factors in mangroves, tidal salt marshes, and seagrasses,” in Conservation international, intergovernmental oceanographic commission of UNESCO (Arlington, Virginia, USA: International Union for Conservation of Nature).
Howard J., Sutton-Grier A., Herr D., Kleypas J., Landis E., Mcleod E., et al. (2017). Clarifying the role of coastal and marine systems in climate mitigation. Front. Ecol. Environ. 15 (1), 42–50. doi: 10.1002/fee.1451
Hudson R., Kenworthy J., Best M. (Eds.) (2021). Saltmarsh restoration handbook: UK and Ireland (Bristol, UK: Environment Agency).
Jones M. B., Donnelly A. (2004). Carbon sequestration in temperate grassland ecosystems and the influence of management, climate and elevated CO2. New Phytol. 164 (3), 423–439. doi: 10.1111/j.1469-8137.2004.01201.x
Kadiri M., Spencer K. L., Heppell C. M., Fletcher P. (2011). Sediment characteristics of a restored saltmarsh and mudflat in a managed realignment scheme in southeast England. Hydrobiologia 672 (1), 79–89. doi: 10.1007/s10750-011-0755-8
Keer G. H., Zedler J. B. (2002). Salt marsh canopy architecture differs with the number and composition of species. Ecol. Appl. 12 (2), 456–473. doi: 10.1890/1051-0761(2002)012[0456:SMCADW]2.0.CO;2
Kelleway J. J., Saintilan N., Macreadie P. I., Ralph P. J. (2016). Sedimentary factors are key predictors of carbon storage in SE Australian saltmarshes. Ecosystems 19 (5), 865–880. doi: 10.1007/s10021-016-9972-3
Kelleway J. J., Serrano O., Baldock J. A., Burgess R., Cannard T., Lavery P. S., et al. (2020). A national approach to greenhouse gas abatement through blue carbon management. Global Environ. Change 63, 102083. doi: 10.1016/j.gloenvcha.2020.102083
Kirwan M. L., Temmerman S., Skeehan E. E., Guntenspergen G. R., Fagherazzi S. (2016). Overestimation of marsh vulnerability to sea level rise. Nat. Climate Change 6 (3), 253–260. doi: 10.1038/nclimate2909
Ladd C. J. (2021). Review on processes and management of saltmarshes across great Britain. Proc. Geologists' Assoc. 132 (3), 269–283. doi: 10.1016/j.pgeola.2021.02.005
Ladd C. J., Duggan-Edwards M. F., Bouma T. J., Pages J. F., Skov M. W. (2019). Sediment supply explains long-term and large-scale patterns in salt marsh lateral expansion and erosion. Geophysical Res. Lett. 46 (20), 11178–11187. doi: 10.1029/2019GL083315
Langston A. K., Alexander C. R., Alber M., Kirwan M. L. (2021). Beyond 2100: Elevation capital disguises salt marsh vulnerability to sea-level rise in Georgia, USA. Estuarine Coast. Shelf Sci. 249, 107093. doi: 10.1016/j.ecss.2020.107093
Lawrence P. J. (2018). How to create a saltmarsh: Understanding the roles of topography, redox and nutrient dynamics. Doctoral thesis (PhD), Manchester Metropolitan University.
Lawrence P. J., Smith G. R., Sullivan M. J., Mossman H. L. (2018). Restored saltmarshes lack the topographic diversity found in natural habitat. Ecol. Eng. 115, 58–66. doi: 10.1016/j.ecoleng.2018.02.007
Lawrence P. J., Sullivan M. J., Mossman H. L. (2022). Restored saltmarshes have low beta diversity due to limited topographic variation, but this can be countered by management. J. Appl. Ecol. 59 (7), 1709–1720. doi: 10.1111/1365-2664.14179
Lecerf M., Herr D., Thomas T., Elverum C., Delrieu E., Picourt L. (2021). Coastal and marine ecosystems as nature-based solutions in new or updated nationally determined contributions, ocean and climate platform, conservation international, IUCN, GIZ, rare. Nat. Conservancy Wetlands Int. WWF.
Levin L. A., Talley T. S. (2002). Natural and manipulated sources of heterogeneity controlling early faunal development of a salt marsh. Ecol. Appl. 12 (6), 1785–1802. doi: 10.1890/1051-0761(2002)012[1785:NAMSOH]2.0.CO;2
Lima M. D. A. C., Ward R. D., Joyce C. B. (2020). Environmental drivers of sediment carbon storage in temperate seagrass meadows. Hydrobiologia 847 (7), 1773–1792. doi: 10.1007/s10750-019-04153-5
Liu Z., Fagherazzi S., Cui B. (2021). Success of coastal wetlands restoration is driven by sediment availability. Commun. Earth Environ. 2 (1), 1–9. doi: 10.1038/s43247-021-00117-7
Lovelock C. E., Atwood T., Baldock J., Duarte C. M., Hickey S., Lavery P. S., et al. (2017). Assessing the risk of carbon dioxide emissions from blue carbon ecosystems. Front. Ecol. Environ. 15 (5), 257–265. doi: 10.1002/fee.1491
Lovelock C. E., Duarte C. M. (2019). Dimensions of blue carbon and emerging perspectives. Biol. Lett. 15 (3), 20180781. doi: 10.1098/rsbl.2018.0781
Luisetti T., Turner R. K., Bateman I. J., Morse-Jones S., Adams C., Fonseca L. (2011). Coastal and marine ecosystem services valuation for policy and management: Managed realignment case studies in England. Ocean and Coastal Management 54, 3, 212–224. doi: 10.1016/j.ocecoaman.2010.11.003
Mac Nally R., Walsh C. J. (2004). Hierarchical partitioning public-domain software. Biodiversity Conserv. 13 (3), 659–660. doi: 10.1023/B:BIOC.0000009515.11717.0b
MacDonald M.A., de Ruyck C., Field R.H., Bedford A., Bradbury R.B. (2020). Benefits of coastal managed realignment for society: Evidence from ecosystem service assessments in two UK regions. Estuarine, Coastal and Shelf Science 244, 1044609. doi: 10.1016/j.ecss.2017.09.007
Macreadie P. I., Anton A., Raven J. A., Beaumont N., Connolly R. M., Friess D. A., et al. (2019). The future of blue carbon science. Nat. Commun. 10 (1), 1–13. doi: 10.1038/s41467-019-11693-w
Macreadie P. I., Costa M. D., Atwood T. B., Friess D. A., Kelleway J. J., Kennedy H., et al. (2021). Blue carbon as a natural climate solution. Nat. Rev. Earth Environ. 2 (12), 826–839. doi: 10.1038/s43017-021-00224-1
Macreadie P. I., Hughes A. R., Kimbro D. L. (2013). Loss of ‘blue carbon’ from coastal salt marshes following habitat disturbance. PLoS One 8 (7), e69244. doi: 10.1371/journal.pone.0069244
Macreadie P. I., Nielsen D. A., Kelleway J. J., Atwood T. B., Seymour J. R., Petrou K., et al. (2017). Can we manage coastal ecosystems to sequester more blue carbon? Front. Ecol. Environ. 15 (4), 206–213. doi: 10.1002/fee.1484
Manning A. J., Langston W. J., Jonas P. J. C. (2010). A review of sediment dynamics in the Severn estuary: Influence of flocculation. Mar. Pollut. Bull. 61 (1-3), 37–51. doi: 10.1016/j.marpolbul.2009.12.012
Mcleod E., Chmura G. L., Bouillon S., Salm R., Björk M., Duarte C. M., et al. (2011). A blueprint for blue carbon: Toward an improved understanding of the role of vegetated coastal habitats in sequestering CO2. Front. Ecol. Environ. 9 (10), 552–560. doi: 10.1890/110004
Möller I., Kudella M., Rupprecht F., Spencer T., Paul M., Van Wesenbeeck B. K., et al. (2014). Wave attenuation over coastal salt marshes under storm surge conditions. Nat. Geosci. 7 (10), 727–731. doi: 10.1038/ngeo2251
Möller I., Spencer T. (2002). Wave dissipation over macro-tidal saltmarshes: Effects of marsh edge typology and vegetation change. J. Coast. Res. 36, 506–521. doi: 10.2112/1551-5036-36.sp1.506
Moreno-Mateos D., Power M. E., Comín F. A., Yockteng R. (2012). Structural and functional loss in restored wetland ecosystems. PLoS Biol. 10 (1), e1001247. doi: 10.1371/journal.pbio.1001247
Mossman H. L., Brown M. J., Davy A. J., Grant A. (2012c). Constraints on salt marsh development following managed coastal realignment: Dispersal limitation or environmental tolerance? Restor. Ecol. 20 (1), 65–75. doi: 10.1111/j.1526-100X.2010.00745.x
Mossman H. L., Davy A. J., Grant A. (2012a). Does managed coastal realignment create saltmarshes with ‘equivalent biological characteristics’ to natural reference sites? J. Appl. Ecol. 49 (6), 1446–1456. doi: 10.1111/j.1365-2664.2012.02198.x
Mossman H. L., Davy A. J., Grant A. (2012b). Quantifying local variation in tidal regime using depth-logging fish tags. Estuarine Coast. Shelf Sci. 96, 122–128. doi: 10.1016/j.ecss.2011.10.019
Mossman H. L., Grant A., Davy A. J. (2020). Manipulating saltmarsh microtopography modulates the effects of elevation on sediment redox potential and halophyte distribution. J. Ecol. 108 (1), 94–106. doi: 10.1111/1365-2745.13229
Mossman H. L., Pontee N., Born K., Hill C., Lawrence P. J., Rae S., et al. (2022). Rapid carbon accumulation at a saltmarsh restored by managed realignment exceeded carbon emitted in direct site construction. PLoS One 17 (11), e0259033. doi: 10.1371/journal.pone.0259033
Murray N. J., Worthington T. A., Bunting P., Duce S., Hagger V., Lovelock C. E., et al. (2022). High-resolution mapping of losses and gains of earth’s tidal wetlands. Science 376 (6594), 744–749. doi: 10.1126/science.abm9583
Nellemann C., Corcoran E. (Eds.) (2009). Blue carbon: In A rapid response assessment (GRID-Arendal: United Nations Environment Programme/ Earthprint).
Nightingale J., McMahon L., Steinke M., McGenity T. J., Gehrels W., Dumbrell A., et al. (2022)Carbon neutrality does not equal climate neutrality in saltmarsh restoration. In: Research square. Available at: https://www.researchsquare.com/article/rs-1731723/v1 (Accessed October 23, 2022). [Preprint].
Oosterlee L., Cox T. J., Vandenbruwaene W., Maris T., Temmerman S., Meire P. (2018). Tidal marsh restoration design affects feedbacks between inundation and elevation change. Estuaries coasts 41 (3), 613–625. doi: 10.1007/s12237-017-0314-2
Ouyang X., Lee S. Y. (2014). Updated estimates of carbon accumulation rates in coastal marsh sediments. Biogeosciences 11 (18), 5057–5071. doi: 10.5194/bg-11-5057-2014
Owers C. J., Rogers K., Woodroffe C. D. (2018). Spatial variation of above-ground carbon storage in temperate coastal wetlands. Estuarine Coast. Shelf Sci. 210, 55–67. doi: 10.1016/j.ecss.2018.06.002
Pendleton L., Donato D. C., Murray B. C., Crooks S., Jenkins W. A., Sifleet S., et al. (2012). Estimating global “blue carbon” emissions from conversion and degradation of vegetated coastal ecosystems. PLoS One 7 (9), e43542. doi: 10.1371/journal.pone.0043542
Penk M. R., Perrin P. M., Kelly R., O’Neill F., Waldren S. (2020). Plant diversity and community composition in temperate northeast Atlantic salt marshes are linked to nutrient concentrations. Applied Vegetation Science 23, 1, 3–13. doi: 10.1111/avsc.12459
Poppe K. L., Rybczyk J. M. (2021). Tidal marsh restoration enhances sediment accretion and carbon accumulation in the stillaguamish river estuary, Washington. PLoS One 16 (9), e0257244. doi: 10.1371/journal.pone.0257244
Ricart A. M., York P. H., Bryant C. V., Rasheed M. A., Ierodiaconou D., Macreadie P. I. (2020). High variability of blue carbon storage in seagrass meadows at the estuary scale. Sci. Rep. 10 (1), 1–12. doi: 10.1038/s41598-020-62639-y
Rodwell J. S. (2000). British Plant communities, maritime communities and vegetation of open habitats Vol. 5 (Cambridge, UK: Cambridge University Press).
Rogers K., Kelleway J. J., Saintilan N., Megonigal J. P., Adams J. B., Holmquist J. R., et al. (2019a). Wetland carbon storage controlled by millennial-scale variation in relative sea-level rise. Nature 567 (7746), 91–95. doi: 10.1038/s41586-019-0951-7
Rogers K., Macreadie P. I., Kelleway J. J., Saintilan N. (2019b). Blue carbon in coastal landscapes: A spatial framework for assessment of stocks and additionality. Sustainability Sci. 14 (2), 453–467. doi: 10.1007/s11625-018-0575-0
Rupp-Armstrong S., Nicholls R. J. (2007). Coastal and estuarine retreat: A comparison of the application of managed realignment in England and Germany. J. Coast. Res. 23 (6), 1418–1430. doi: 10.2112/04-0426.1
Saintilan N., Kovalenko K. E., Guntenspergen G., Rogers K., Lynch J. C., Cahoon D. R., et al. (2022). Constraints on the adjustment of tidal marshes to accelerating sea level rise. Science 377 (6605), 523–527. doi: 10.1126/science.abo7872
Santini N. S., Lovelock C. E., Hua Q., Zawadzki A., Mazumder D., Mercer T. R., et al. (2019). Natural and regenerated saltmarshes exhibit similar soil and belowground organic carbon stocks, root production and soil respiration. Ecosystems 22 (8), 1803–1822. doi: 10.1007/s10021-019-00373-x
Seddon N., Chausson A., Berry P., Girardin C. A., Smith A., Turner B. (2020). Understanding the value and limits of nature-based solutions to climate change and other global challenges. Philos. Trans. R. Soc. B. 375 (1794), 20190120. doi: 10.1098/rstb.2019.0120
Serrano O., Lavery P. S., Duarte C. M., Kendrick G. A., Calafat A., York P. H., et al. (2016). Can mud (silt and clay) concentration be used to predict soil organic carbon content within seagrass ecosystems? Biogeosciences 13 (17), 4915–4926. doi: 10.5194/bg-13-4915-2016
Shepherd D., Burgess D., Jickells T., Andrews J., Cave R., Turner R. K., et al. (2007). Modelling the effects and economics of managed realignment on the cycling and storage of nutrients, carbon and sediments in the blackwater estuary UK. Estuarine Coast. Shelf Sci. 73 (3-4), 355–367. doi: 10.1016/j.ecss.2007.01.019
Smeaton C., Burden A., Ruranska P., Ladd C. J., Garbutt A., Jones L., et al. (2022). Using citizen science to estimate surficial soil blue carbon stocks in great British saltmarshes. Front. Mar. Sci. 9. doi: 10.3389/fmars.2022.959459
Spearman J. (2011). The development of a tool for examining the morphological evolution of managed realignment sites. Continental Shelf Res. 31 (10), 199–210. doi: 10.1016/j.csr.2010.12.003
Stagg C. L., Mendelssohn I. A. (2010). Restoring ecological function to a submerged salt marsh. Restor. Ecol. 18, 10–17. doi: 10.1111/j.1526-100X.2010.00718.x
Suello R. H., Hernandez S. L., Bouillon S., Belliard J. P., Dominguez-Granda L., Van de Broek M., et al. (2022). Mangrove sediment organic carbon storage and sources in relation to forest age and position along a deltaic salinity gradient. Biogeosciences 19 (5), 1571–1585. doi: 10.5194/bg-19-1571-2022
Temmink R. J., Lamers L. P., Angelini C., Bouma T. J., Fritz C., van de Koppel J., et al. (2022). Recovering wetland biogeomorphic feedbacks to restore the world’s biotic carbon hotspots. Science 376 (6593), 1479. doi: 10.1126/science.abn1479
Unsworth R. K., Nordlund L. M., Cullen-Unsworth L. C. (2019). Seagrass meadows support global fisheries production. Conserv. Lett. 12 (1), e12566. doi: 10.1111/conl.12566
Vanderklift M. A., Marcos-Martinez R., Butler J. R., Coleman M., Lawrence A., Prislan H., et al. (2019). Constraints and opportunities for market-based finance for the restoration and protection of blue carbon ecosystems. Mar. Policy 107, 103429. doi: 10.1016/j.marpol.2019.02.001
Venables W. N., Ripley B. D. (2002). Modern applied statistics with s. 4th ed. (New York: Springer).
Waltham N. J., Elliott M., Lee S. Y., Lovelock C., Duarte C. M., Buelow C., et al. (2020). UN Decade on ecosystem restoration 2021–2030—what chance for success in restoring coastal ecosystems? Front. Mar. Sci. 7. doi: 10.3389/fmars.2020.00071
Wentworth C. K. (1922). A scale of grade and class terms for clastic sediments. J.geology 30 (5), 377–392. doi: 10.1086/622910
Wollenberg J. T., Ollerhead J., Chmura G. L. (2018). Rapid carbon accumulation following managed realignment on the bay of fundy. PLoS One 13 (3), e0193930. doi: 10.1371/journal.pone.0193930
Wolters M., Bakker J. P., Bertness M. D., Jefferies R. L., Möller I. (2005). Saltmarsh erosion and restoration in south-east England: Squeezing the evidence requires realignment. J. Appl. Ecol. 42 (5), 844–851. doi: 10.1111/j.1365-2664.2005.01080.x
Wolters M., Garbutt A., Bekker R. M., Bakker J. P., Carey P. D. (2008). Restoration of salt-marsh vegetation in relation to site suitability, species pool and dispersal traits. J. Appl. Ecol. 45 (3), 904–912. doi: 10.1111/j.1365-2664.2008.01453.x
Wylie L., Sutton-Grier A. E., Moore A. (2016). Keys to successful blue carbon projects: Lessons learned from global case studies. Mar. Policy 65, 76–84. doi: 10.1016/j.marpol.2015.12.020
Zhao Q., Bai J., Zhang G., Jia J., Wang W., Wang X. (2018). Effects of water and salinity regulation measures on soil carbon sequestration in coastal wetlands of the yellow river delta. Geoderma 319, 219–229. doi: 10.1016/j.geoderma.2017.10.058
Keywords: blue carbon, saltmarsh habitat, ecosystem restoration, relative tidal height, vegetation zonation
Citation: McMahon L, Ladd CJT, Burden A, Garrett E, Redeker KR, Lawrence P and Gehrels R (2023) Maximizing blue carbon stocks through saltmarsh restoration. Front. Mar. Sci. 10:1106607. doi: 10.3389/fmars.2023.1106607
Received: 23 November 2022; Accepted: 16 March 2023;
Published: 30 March 2023.
Edited by:
Heliana Teixeira, University of Aveiro, PortugalReviewed by:
Mark Schuerch, University of Lincoln, United KingdomJunhong Bai, Beijing Normal University, China
Copyright © 2023 McMahon, Ladd, Burden, Garrett, Redeker, Lawrence and Gehrels. This is an open-access article distributed under the terms of the Creative Commons Attribution License (CC BY). The use, distribution or reproduction in other forums is permitted, provided the original author(s) and the copyright owner(s) are credited and that the original publication in this journal is cited, in accordance with accepted academic practice. No use, distribution or reproduction is permitted which does not comply with these terms.
*Correspondence: Lucy McMahon, bHVjeS5tY21haG9uQHlvcmsuYWMudWs=