- 1Royal Belgian Institute of Natural Sciences (RBINS), Operational Directorate Natural Environment, Marine Ecology and Management, Brussels, Belgium
- 2Marine Biology Research Group, Department of Biology, Ghent University, Ghent, Belgium
- 3Department of Estuarine and Delta Systems, Royal Netherlands Institute for Sea Research (NIOZ), Yerseke, Netherlands
- 4Isotope Bioscience Laboratory (ISOFYS), Department of Green Chemistry and Technology, Ghent University, Ghent, Belgium
Blue mussels (Mytilus edulis) are an abundant and economically important species across the North Sea. Partly because of their potent filter feeding and associated shell biofilm, they are able to influence and alter the surrounding marine ecosystem. As a result of proliferating offshore wind farms (OWFs), whose turbine foundations are rapidly colonised by suspension feeding artificial hard substrate communities dominated by M. edulis, as well as planned co-location strategies of these OWFs with mussel mariculture, their numbers will only increase towards the future. On top of these local stressors, global climate change is exerting additional pressure on the marine environment. This study focusses on the link between M. edulis, its microbial shell biofilm and the local nitrogen cycling by quantifying the magnitude and underlying pathways of mussel-associated nitrous oxide (N2O) production. A set of closed-core incubations established nitrifier denitrification as the main chemical pathway of M. edulis related N2O production, although ammonium, nitrite and nitrate all acted as possible precursors. Additional future-climate experiments revealed that blue mussel’s total N2O production, as well as its metabolic activity and the relative contribution of its shell biofilm, were affected by warming (+ 3°C), acidification (- 0.3 pH units), or the combination of both. Because the effects of temperature and acidity were often of an antagonistic nature, the results suggest a relatively small net effect on local N2O production in future-climate marine environments. However, N2O production rates were several orders of magnitude lower than other measured N species (, and ), making substantial mussel-associated N2 production likely. This would greatly affect the local eutrophication levels or even bioavailable nitrogen concentrations.
1. Introduction
After carbon dioxide (CO2) and methane (CH4), nitrous oxide (N2O) is an impactful long-lived greenhouse gasses (GHGs), substantially contributing to global warming (estimated 6 %) and to the depletion of the stratospheric ozone layer (IPCC, 2021). It has a long atmospheric lifetime (average of 114 years) and its warming effect on the global climate through radiative forcing (Global Warming Potential of 273 for a 100-year timescale) therefore persists for over a century after its emission (Smith and Sharp, 2012; Myhre et al., 2013; IPCC, 2021). N2O is produced by (de)nitrifying bacteria and archaea in both terrestrial and aquatic environments, mainly associated with agricultural practices and invertebrate presence or activities (Mosier et al., 1998; Stein and Yung, 2003; Heisterkamp et al., 2010). The biogenic emission of N2O by freshwater invertebrates is correlated with their feeding type, due to the denitrifying activity of ingested bacteria in the anoxic digestive system (Stief et al., 2009). In the marine environment, N2O is emitted by denitrifying gut bacteria as well, but also by microbial biofilms on the surfaces of e.g. bivalves and gastropods (Heisterkamp et al., 2010; Heisterkamp et al., 2013). These shell biofilms can generate N2O through three main pathways (Figure 1): (1) as a by-product of ammonium oxidation, the first step in (oxic) nitrification by ammonium-oxidising bacteria (AOB) and archaea (AOA), (2) as an intermediate in the reduction of nitrite during AOB- and AOA-mediated suboxic nitrifier denitrification and (3) as an intermediate in the reduction of nitrate during anoxic denitrification by heterotrophic bacteria. The latter comprises the final steps in the pathway of coupled nitrification-denitrification (CND), in which (heterotrophic) denitrification is carried out by a different set of actors compared to (autotrophic) nitrifier denitrification and nitrification (Figure 1).
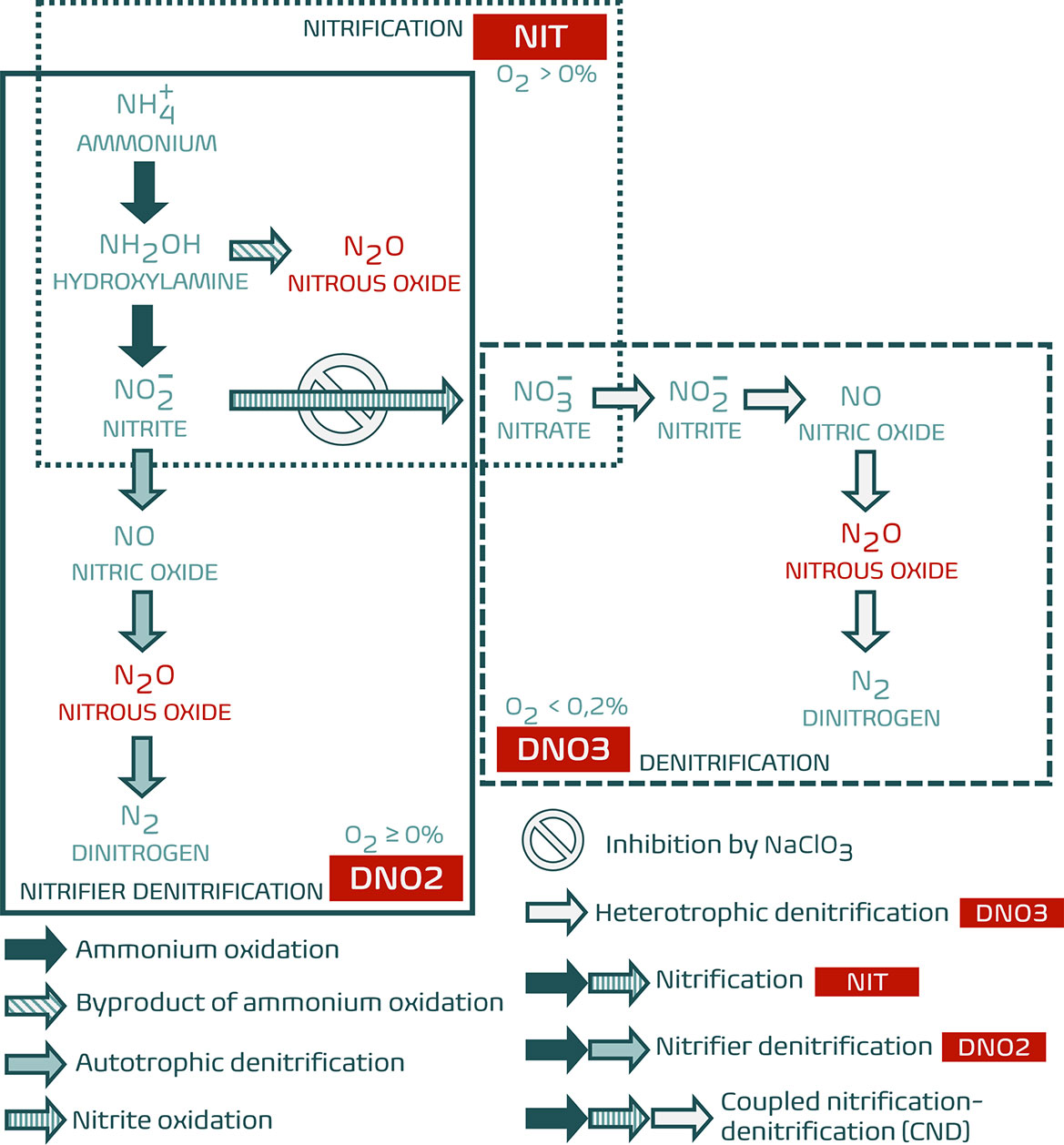
Figure 1 Relevant pathways in the marine nitrogen cycle with possible sources of N2O emission and oxygenation demands. The result of a targeted inhibition by NaClO3 is indicated. NIT: oxic nitrification (autotrophic); DNO2: suboxic nitrifier denitrification (autotrophic); DNO3: anoxic denitrification (heterotrophic) coupled to nitrification. Adapted from Zhu et al. (2013).
An important shell-bearing organism in coastal ecosystems is the blue mussel Mytilus edulis. It has an important ecological relevance through its high filtration capacity and ‘bio-engineering’ role in habitat creation and food provision (Cranford, 2019; Degraer et al., 2020). In the North Sea, it is one of the main colonising species on artificial hard substrate [AHS] (De Mesel et al., 2015), readily available due to the proliferating European offshore wind farm [OWF] industry (IEA, 2019; GWEC, 2021). Partly due to the blue mussel’s presence, these AHSs act as an artificial reef and as a biofilter, redirecting organic material from the water column towards the benthic environment (Slavik et al., 2019; Ivanov et al., 2021). Furthermore, this species has a high economical value because of its prominent role in aquaculture (Costello et al., 2020), a practice that might progressively be combined with OWF operations (Schupp et al., 2019; MSP, 2020) as a favourable way of minimising the impacted seafloor footprint (Buck and Langan, 2017; Steins et al., 2021).
All three of the N2O-producing pathways (Figure 1) could potentially occur in and on the blue mussel. Firstly, M. edulis accommodates a selection of ingested denitrifying bacteria in its anoxic digestive system, where organic carbon and different N-species are readily available (Stief et al., 2009). Additionally, it has a microbial biofilm on the outer surface of its shell, within which a variable oxygenation allows different pathways of N2O emission (Heisterkamp et al., 2010; Heisterkamp et al., 2013). Furthermore, these biofilm emissions can be sustained through the pumping activity of the mussel replenishing its surroundings with nutrient-enriched waters (Heisterkamp et al., 2013). This could potentially decouple the shell biofilm’s N2O production from (outside) environmental nitrogen cycling and fluctuations, establishing a relatively stable capacity for N2O emission. In an OWF or aquaculture environment, where mussels are typically present in high densities, this could potentially generate high local N2O emissions (Voet et al., manuscript in preparation)1.
The abundance of mussels could well rise towards the future due to their potential in offshore and integrated multi-trophic aquaculture projects (Avdelas et al., 2021). When investigating the production of a potent greenhouse gas by such a marine species, the link with marine climate change and its global effects on the oceanic environment needs to be taken into account. The IPCC predicts a sea surface temperature rise of ca. 3 °C and a drop in oceanic pH of ca. 0.3 by the end of this century (Bindoff et al., 2019; Fox-Kemper et al., 2021; IPCC, 2021), changing the biochemistry in marine environments and potentially affecting the N2O emissions by mussel aggregations. The objective of this study was therefore to investigate the production of N2O by M. edulis and its associated shell biofilm in current conditions and to describe the potential effects of seawater temperature and pH on both. This was done by experimentally (1) quantifying the relative contribution of the microbial shell biofilm to M. edulis’ entire N2O production and (2) unravelling the different chemical pathways and precursors of M. edulis’ N2O production. Experiments were performed in a fully-crossed design of temperature and pH, representing current and future climate conditions.
2. Methodology
2.1. Organism collection and incubation set-up
In summer 2018 and 2020, a respective total of 800 and 240 adult blue mussels (Mytilus edulis) with a mean soft tissue dry weight (± SD) of 0.79 ± 0.28 g were sampled from a M. edulis longline in an offshore aquaculture pilot project approximately 10 km off the Belgian coast (51°11.02’N - 02°39.88’E). All samples were stored in aerated seawater and transported to the experimental facilities within 4 hours.
On both occasions, organisms were evenly and randomly distributed across four identical aquaria (100 × 5 × 0 cm). These were equipped with a continuous flow-through mechanism, allowing the homogenisation of approximately 400 L natural seawater in circulation per system. All aquaria were continuously aerated and pre-set at laboratory conditions mimicking the in situ seawater salinity, temperature and pH at the time of sampling (34 PSU, 20°C and pH 7.96; LifeWatch Belgium, 2015). After an initial acclimatisation period of minimum 48 h under ambient conditions, both seawater temperature and pH were manipulated individually across the aquaria. This resulted in a 2 × 2 factorial design with four experimental treatments: a control treatment [CTRL: in situ temperature and pH], an ocean acidification treatment [OA: in situ temperature and lowered pH], an ocean warming treatment [OW: elevated temperature and in situ pH] and a climate change treatment [CC: combined elevated temperature and lowered pH].
For each treatment, stepwise manipulations were imposed over the course of three days, with a daily seawater temperature increase of 1°C and/or pH decrease of 0.1 pH unit. Seawater temperature was regulated using TECO TK2000 heaters and pH was manipulated through the controlled bubbling of 100 % CO2 in the OA and CC treatment tanks using the IKS AquaStar aquaristic computer system, simultaneously logging temperature and pH records every 15 minutes throughout the six-week experiments (Table 1; Appendix A-Appendix B). This resulted in environmental conditions of +3°C and/or -0.3 pH units in the corresponding treatments compared to the control settings, reflecting the IPCC RCP-SSP8.5 projections for ocean warming and acidification towards the end of this century (Hoegh-Guldberg et al., 2014; IPCC, 2021). These conditions were maintained for six weeks and organisms were fed three times a week by adding 5 mL Shellfish Diet 1800® (Instant Algae® mix by Reed Mariculture Inc.) to each aquarium. Glass pH electrodes were calibrated weekly using Hanna Instruments™ NIST Reference Buffer Solutions (4.01 and 7.01) and each aquarium was sampled weekly to determine Total Alkalinity (TA) using a CONTROS HydroFIA™TA alkalinity system. One third of each flow-through system’s water was renewed weekly. The carbonate chemistry of the seawater was calculated using CO2SYS software (Pierrot et al., 2006) with the thermodynamic constants of Mehrbach et al. (1973).
2.2. Experimental procedures
2.2.1. Relative contribution of shell biofilm to N2O production
Weekly, triplicated individual closed-core incubations were set up in each experimental treatment (CTRL, OA, OW and CC) to measure N2O production by the mussel itself and its shell biofilm (see Appendix C: Experiment 1). Whole mussels [WHOLE] and dissected mussel shells [SHELL] were incubated separately, along with a control incubation without mussels or shells [EMPTY] to correct for background N2O presence. An additional control consisted of whole mussels in a 1 % ZnCl2 seawater solution inhibiting all biological activity [ZNCL] (Heisterkamp et al., 2013). Each incubation core of 1.5 L held one individual or dissected shell of one individual (except EMPTY), along with manipulated seawater from the respective treatment, and was kept at the correct temperature throughout 3h the incubations. A magnetic stirrer ensured an evenly mixed water column in the cores. Discrete 30 mL water samples were taken at the start and end of the closed-core incubations and stored at room temperature in dark conditions. Each sample filled, to overflowing, a 30 mL airtight serum bottle and was fixed with 100 µL saturated HgCl2 solution for N2O analysis. Quantification of dissolved N2O was done by gas chromatography (SRI Instruments, ECD) and the N2O production rates (PR) of whole organisms and dissected shells were calculated according to Equation 1:
with V the volume (L) of the incubation core corrected for the biovolume of the whole organism or shell and with C0 and C1 the concentrations of N2O (nmol L-1) at the start and finish of the closed-core incubation, t0 and t1 (h), respectively. The relative contribution of the shell biofilm to whole animal N2O production was calculated by dividing each SHELL PR by the corresponding WHOLE PR in each week and treatment.
2.2.2. Pathways of N2O production: Nutrient flux and inhibition by NaClO3
After three and six weeks of manipulations [WK3 and WK6, respectively], triplicated closed-core incubations with individual whole organisms were set up and sampled as described above (see Appendix C: Experiment 2). Along with biomass-specific N2O production rates [PRB] (calculated according to Equation 2), , and concentrations were measured through discrete sampling (25 mL; automated colorimetric analyses performed on SKALAR SAN++ CFA) at the start and finish of closed-core incubations, with and without the addition of 20 mM NaClO3 to inhibit CND (Belser and Mays, 1980; see Figure 1). NaClO3 inhibits the oxidation of nitrite () to nitrate () in the nitrification pathway (Figure 1), meaning N2O is produced either though nitrification (as a by-product during ammonium oxidation) or through nitrifier denitrification (as result of denitrification of nitrite; Tallec et al., 2008), as well as through denitrification of nitrate already present in the incubation core (Figure 1).
Nutrient flux was calculated according to Equation 3;
with parameters identical as in Equation 1, C the concentration of N2O (nmol L-1) and nutrients , or (µmol L-1) in Equation 2 and Equation 3, respectively, and DW the dry weight (g) of the mussel’s soft tissue.
2.2.3. Pathways of N2O production: 15N stable isotope tracer experiments
Three different labelled N-tracer treatments were designed to distinguish the production of double-15N-labelled N2O (46N2O) from either nitrification [NIT], nitrifier denitrification [DNO2] or CND [DNO3], in which , or act as a precursor to N2O production, respectively (Table 2 and Figure 1). The composition of these N-tracer treatments, in which 15N was introduced using either 15NH4Cl [NIT], Na15NO2 [DNO2] or Na15NO3 [DNO3], and the resulting concentrations in the incubation cores were identical to those in Heisterkamp et al (2013; adapted in Table 2).
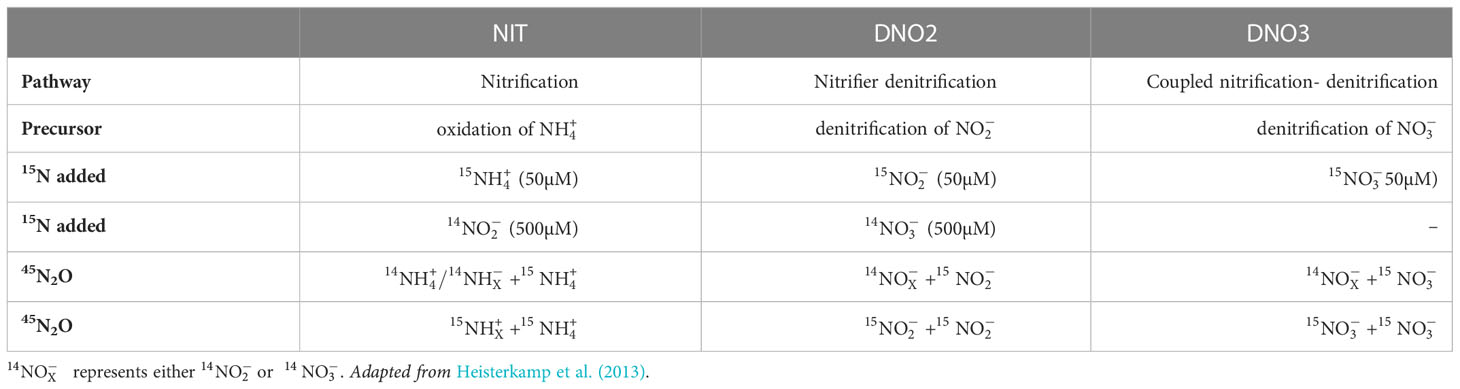
Table 2 Nitrogen tracer treatments NIT, DNO2 and DNO3 with the targeted N2O-producing pathway and precursor, the concentrations of 15N and 14N added to the tracer treatments and the possible combinations of 14N and 15N to form 45N2O and 46N2O, respectively.
Before manipulations took place [WK0] and at WK3 and WK6, triplicated closed-core incubations for all three N-tracers were set up in all four experimental treatments (CTRL, OA, OW and CC; see Appendix C: Experiment 3). The 125mL incubation cores held one individual in seawater from its respective experimental treatment amended with the respective N-tracer. Cores were incubated for 4 hours on a shaking table to ensure adequate mixing. Hourly, 1mL samples were taken with a surgical syringe and transferred to 12mL He-flushed exetainers. These were prefilled with 100µL saturated HgCl2 and 20 nmol natural N2O with known isotopic composition. The HgCl2 was added to fixate the N2O, while the addition of unlabelled N2O ensured the detection limit of the isotope ratio mass spectrometer was reached. Since only 4 % of the core volume was sampled by the end of the incubation, under-pressure was not considered to be an issue, and the withdrawn volume was not replaced. The excess 45N2O and 46N2O content was determined from the 45N2O/44N2O and 46N2O/44N2O ratio of the samples compared to a reference N2O spiked blank sample using a ANCA-TGII interfaced with a SerCon 20-20 IRMS (SysCon electronics) with cryogenic trapping and focusing of samples. Production of 44N2O could not be determined in this assay due to the presence of the added 20 nmol N2O spike. The linear increase of 45N2O and 46N2O over time was used to calculate net PRB (nmol g-1DW h-1). The contribution of the different pathways to the total 15N-labelled N2O production was calculated by dividing the respective 46N2O PRB by the sum of 45N2O and 46N2O PRB in each N-tracer experiment.
2.2.4. Shell biofilm oxygen microprofile
As a proxy for metabolic activity of shell biofilms, a vertical oxygen profile of the microbial biofilm on three replicate M. edulis shells was measured before seawater manipulations started [WK0] and in each experimental treatment after three and six weeks of manipulations [WK3 and WK6, respectively]. Individuals were carefully dissected with a scalpel to isolate the mussel shell without damaging the associated biofilm and dissected shells were placed in aerated seawater from the respective experimental treatments. A Unisense™ MicroProfiling System was used to position the sensor tip of a PyroScience™ retractable fiber oxygen microsensor on the shell surface and a vertical dissolved oxygen concentration profile was recorded in increments of 50 or 100 µm through the biofilm between 0-3000 µm above the shell surface. Oxygen microprofiles were measured at three random positions on the shell of each replicate organism and conducted in dark conditions to avoid net oxygen production.
2.3. Data analysis
The effect of temperature (current or elevated) and pH (current or lowered) on the production of N2O in the WHOLE, SHELL, NaClO3-inhibited and non-inhibited incubations was investigated using linear mixed effects models, with incubation core and/or experimental week added as a random factor in the models. If none of the random factors could explain left-over variance, a linear regression model was fitted. The effect of temperature, pH and experimental week (WK3 or WK6) on nutrient flux was analysed using linear regression models. Significance of the two-way interaction ‘temperature x pH’ and post-hoc pairwise comparison of the group means was used to identify possible combination effects of increased temperature and lowered pH. Normality of the residuals and model assumptions were checked using Shapiro-Wilk, Gaussian error distributions were used and model selection was based on the parametric bootstrap and Kenward Roger methods for mixed model comparison (Halekoh and Højsgaard, 2014) or on the backwards selection procedure for linear regression models. Statistical analyses were conducted using R v3.6.1 (R Core Team, 2019), linear mixed effects models were built using the R package lme4 (Bates et al., 2015) and linear regression models were fitted using the stats package (R Core Team, 2019).
To test for differences between oxygen microprofiles between experimental treatments in WK3 and WK6 (to keep a balanced design), a multivariate matrix was constructed with the oxygen concentrations at each distance above the shell (Widdicombe et al., 2013). Differences in the shell biofilm oxygen microprofiles were tested using permutational ANOVA (PERMANOVA) with experimental treatment and week as fully-crossed factors and with all profiles on all shells considered as replicates within the Euclidian distance matrix. For all significant PERMANOVA factors, post-hoc pairwise testing was used and homogeneity of multivariate dispersion was tested using PERMDISP. The SIMPER routine was used to identify which distances from the shell contributed most to any observed differences. Multivariate analyses were carried out in Primer v6.0 with PERMANOVA+ add-on software (Clarke and Gorley, 2006; Anderson et al., 2008).
3. Results
Mean seawater temperature (± SD) in CTRL treatments was 19.97 ± 0.20°C and ranged between 23.07 ± 0.36°C and 23.15 ± 0.24°C in the warmed treatments (OW and CC). Mean seawater pH (± SD) in acidified treatments (OA and CC) ranged between 7.63 ± 0.05 and 7.65 ± 0.02, compared to an average CTRL seawater pH of 7.96 ± 0.02 (Table 1; Appendix A - Appendix B). Seawater salinity differed slightly between the treatments but was well within the natural occurring salinity range. The total alkalinity (TA) fluctuated according to the pH levels (1985-2123 µmol kg-1) and the average aragonite/calcite seawater saturation states (Ω; ± SD) ranged between 1.70 ± 0.25 – 2.97 ± 0.52 in the non-acidified treatments and between 0.82 ± 0.16 – 1.51 ± 0.24 in the acidified treatments (Table 3).
For an overview of ecophysiological effects of increased temperature and lowered seawater pH on M. edulis, see Voet et al. (2021). In this study, the same experimental treatments (CTRL, OW, OA and CC) were imposed for six weeks. Significant results included a decreased survival rate and growth in acidified treatments, enhanced growth rates in warmed treatments and an additive effect of higher temperatures and lower pH levels on respiration and clearance rates (Voet et al., 2021).
3.1. Relative contribution of shell biofilm to N2O production
In all treatments, N2O was produced in both the WHOLE and SHELL incubations, whereas no N2O production was measured in the poisoned ZNCL incubations. In CTRL, whole animals produced on average (± SD) 4.55 ± 0.18 nmol N2O ind-1 h-1 and throughout the experiment, the shell biofilm contribution ranged between 65-75% (Figure 2). In OW, the average WHOLE production rate gradually increased from 5.47 ± 0.23 to 15.84 ± 0.48 nmol N2O ind-1 h-1 over six weeks, while the shell biofilm contribution remained stable at 68% (Figure 2). In both acidified treatments, the relative contribution of the shell biofilm to whole organism N2O production decreased over time. In OA, the WHOLE N2O production rate was stable throughout the experiment (mean 5.79 ± 0.33 nmol N2O ind-1 h-1), but the contribution of the shell biofilm decreased from 63% to 23% over the course of six weeks (Figure 2). Whole animals in CC produced on average 9.10 ± 1.82 nmol N2O ind-1 h-1, while the associated shell biofilm contribution decreased from 60% to 12% over time, after an initial rise to 65% in the second week of experiments. Overall, temperature and pH had a significant, antagonistic effect on the parts of the animal that emitted N2O, with an increasing production in warmer environments and a decreasing production in acidified or combined climate change conditions (Table 4).
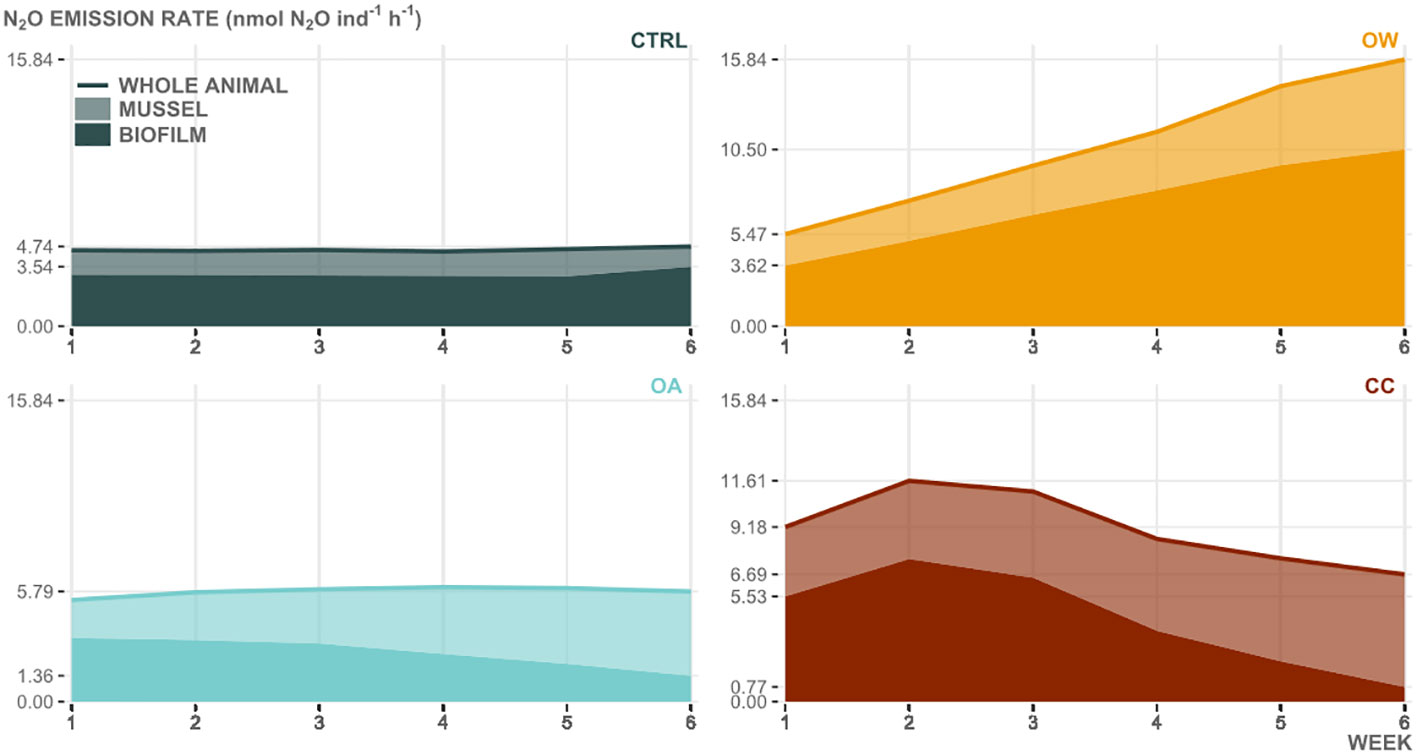
Figure 2 Estimated mean relative contribution of dissected Mytilus edulis shells [SHELL] to whole animal [WHOLE] N2O emission rates (nmol N2O ind-1 h-1) in four experimental treatments [CTRL, control; OA, ocean acidification; OW, ocean warming and CC: climate change]. Relative contribution of M. edulis itself [MUSSEL] represents the calculated difference between the two.
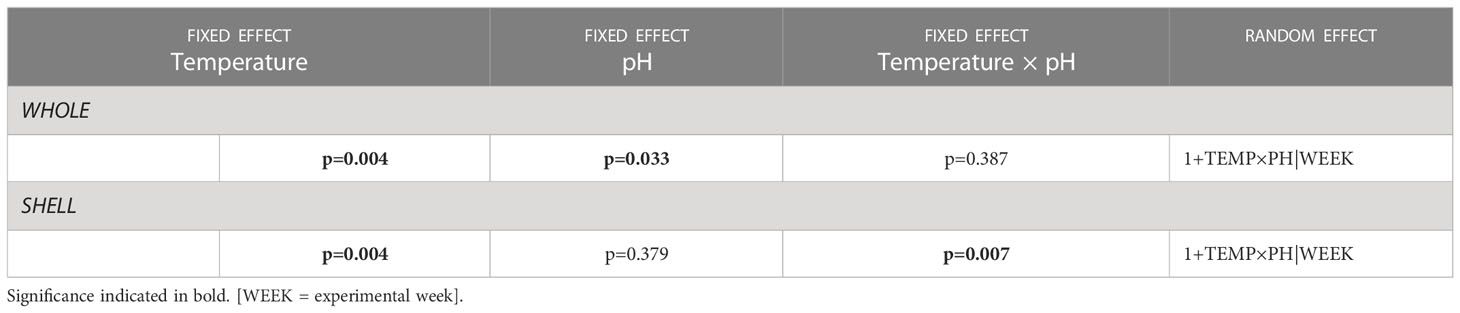
Table 4 Structure of final linear mixed effects models for N2O production [nmol N2O ind-1 h-1] by whole animals [WHOLE] and shell biofilms [SHELL]: significance of fixed effects Temperature (TEMP), pH (PH) and two-way interaction (TEMP x PH), and identity of random effects, including random intercept (format = 1|random) and potential random slope (format = 1+fixed|random).
3.2. Pathways of N2O production
3.2.1. Nutrient flux and inhibition of coupled nitrification-denitrification
When the CND pathway was inhibited by the addition of NaClO3, average N2O production rates were 4 to 16 times higher compared to incubations where the inhibitor was absent (Figure 3). Regardless of the presence of the inhibitor, temperature and pH had an antagonistic effect on the production of N2O by M. edulis and the highest average N2O production rates were measured in OW (Figure 3 and Table 5).
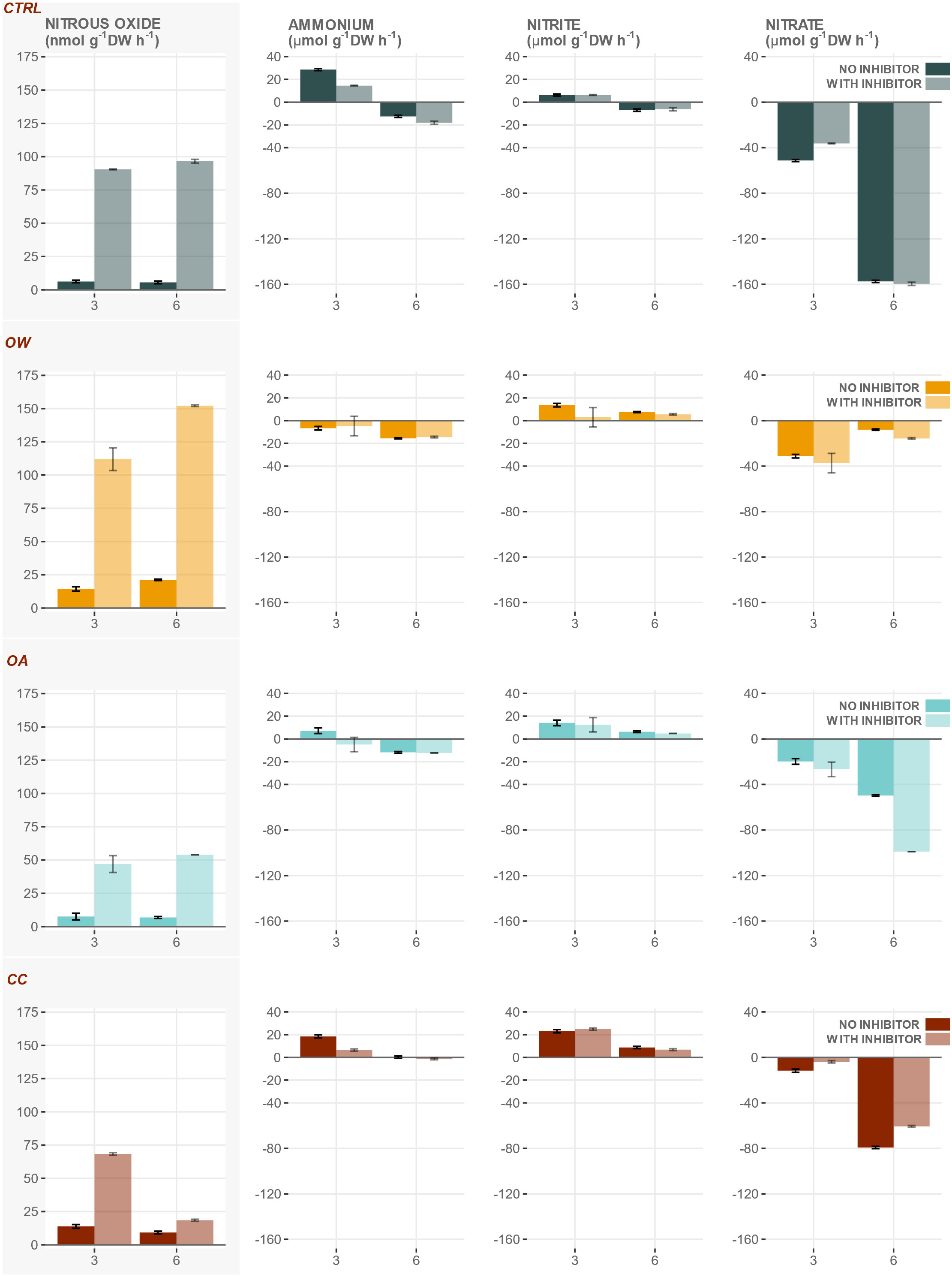
Figure 3 N2O production rates (nmol g-1DW h-1 ± SE) and nutrient flux (µmol g-1DW h-1 ± SE) of whole animals incubated with and without the addition of NaClO3, inhibiting the oxidation of nitrite to nitrate, in four experimental treatments [CTRL, control; OW, ocean warming; OA, ocean acidification and CC,: climate change]. Note different scale and unit in left panels.
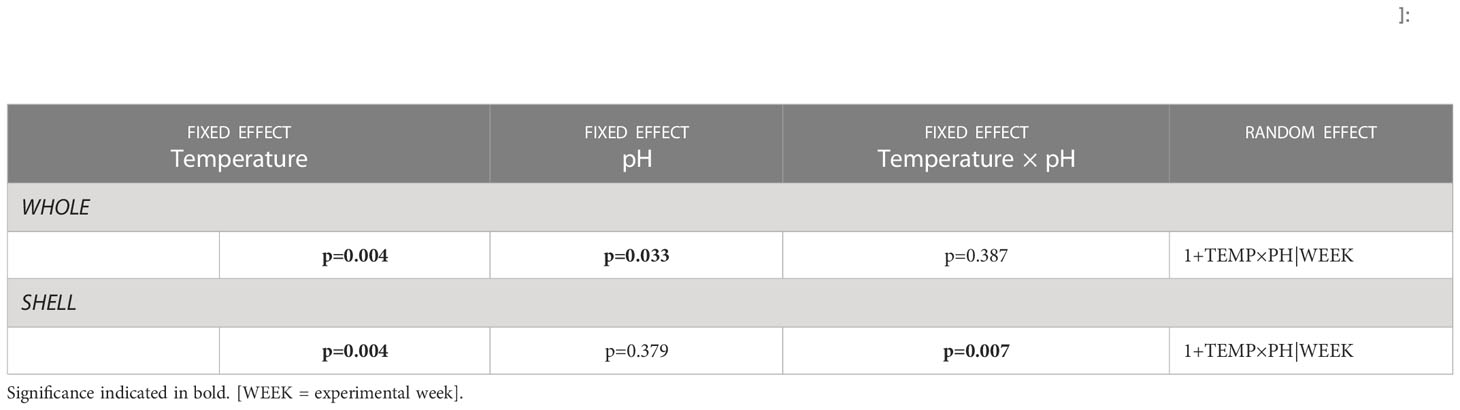
Table 5 R2 of final linear regression models with significance of Temperature (TEMP), pH (PH), two-way interaction (TEMP x PH) and week (WEEK) in incubations with and without inhibitor NaClO3 for production of N2O [nmol g-1DW h-1].
Inhibition by NaClO3 significantly lowered ammonium flux, which additionally decreased significantly in WK6 compared to WK3 (Figure 3 and Table 6). Lowered pH and elevated temperature antagonistically affected ammonium flux (production or oxidation rates; Table 6), with the lowest average flux in OW and a significantly higher flux when combined with lowered pH in CC (Figure 3).
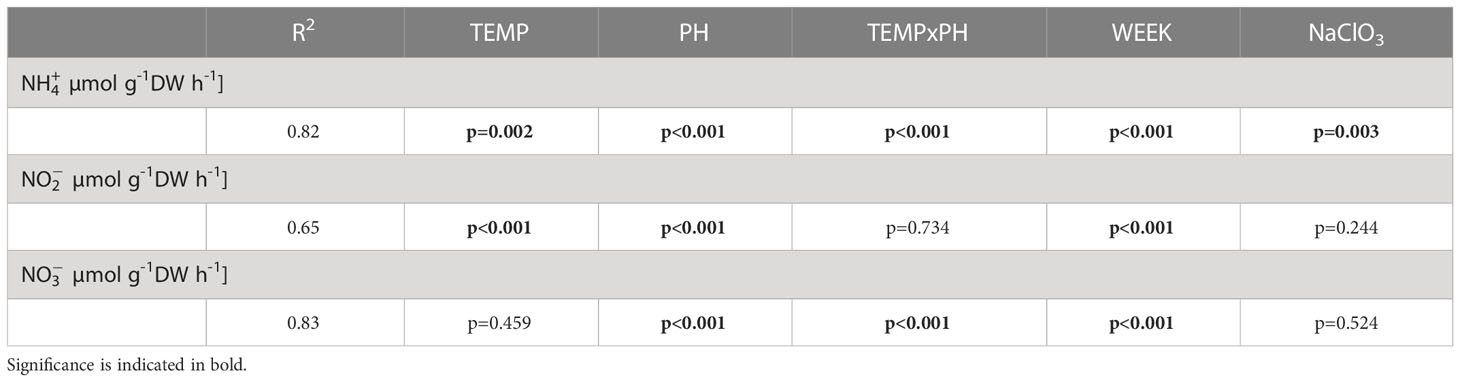
Table 6 R2 of final linear regression models with significance of Temperature (TEMP), pH (PH), two-way interaction (TEMP x PH), week (WEEK) and addition of inhibitor sodium chlorate (NaClO3) for nutrient flux (ammonium , nitrite NO2 and nitrate NO3) [µmol g-1 DW h-1].
There was no significant effect of the inhibitor NaClO3 on nitrite flux and in general, nitrite flux decreased with time (Figure 3 and Table 6). Elevated temperature and lowered pH significantly increased flux (Table 6).
Nitrate flux was negative across all experimental treatments and not affected by the presence of the inhibitor (Figure 3 and Table 6). Nitrate consumption (negative flux) significantly increased with time and the significant decreasing effect of a lowered pH was further amplified when combined with an elevated temperature in CC (Table 6).
3.2.2. N2O precursors: 15N stable isotope tracers
45N2O and 46N2O was detected in all 15N-tracer experiments across all treatments and weeks, showing that , and all serve as potential precursors and nitrification, nitrifier denitrification and coupled nitrification-denitrification (respectively) all serve as potential pathways of N2O production by M. edulis and its shell biofilm (Table 7 and Figure 4).
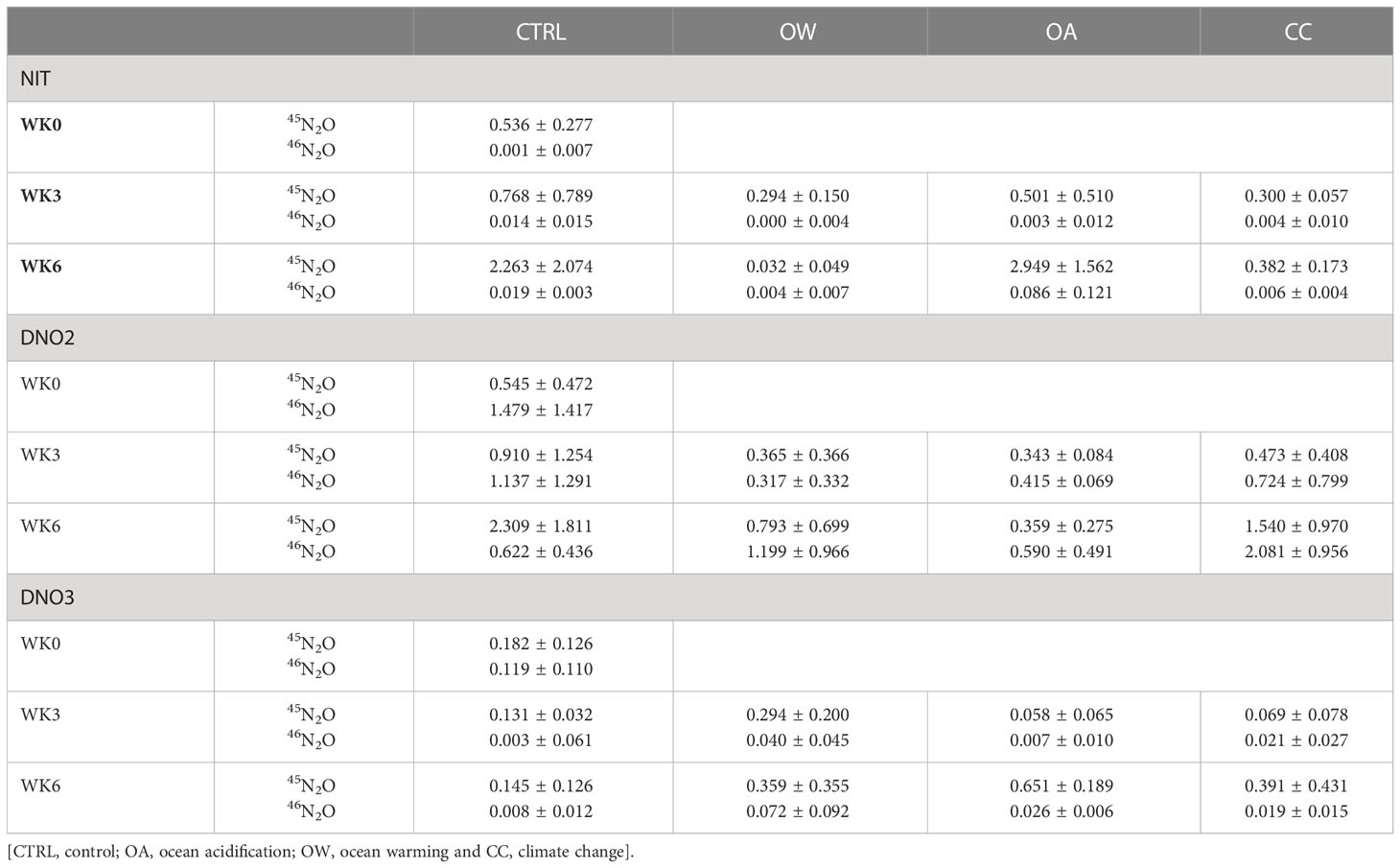
Table 7 45N2O and 46N2O production rate (nmol g-1DW h-1 ± SD) of whole animals before seawater manipulations started [WK0] and after three [WK3] and six [WK6] weeks of manipulations with three N-tracer targeting nitrification [NIT], nitrifier denitrification [DNO2] or coupled nitrification-denitrification [DNO3], in which , or act as a precursor to N2O emission, respectively, in four experimental treatments.
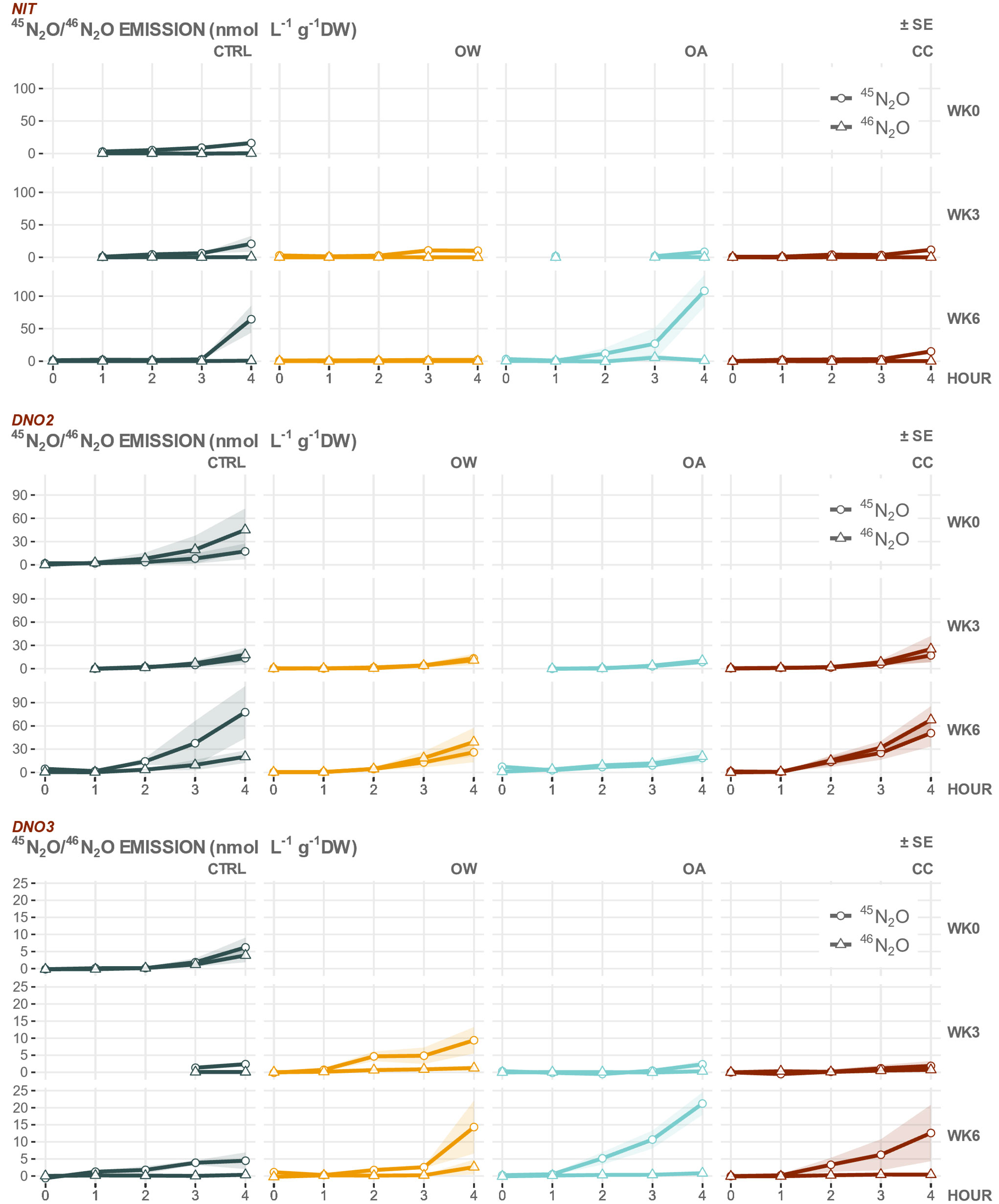
Figure 4 45N2O (circles) and 46N2O (triangles) emission (nmol L-1 g-1DW ± SE shaded area) of M. edulis before seawater manipulations [WK0] and after three [WK3] or six [WK6] weeks of manipulations with 3 N-tracers targeting nitrification [NIT], nitrifier denitrification [DNO2] or coupled nitrification-denitrification [DNO3], in which , or act as a precursor to N2O emission, respectively, in 4 experimental treatments [CTRL: control, OA: ocean acidification, OW: ocean warming and CC: climate change]. Missing data due to faulty GC-IRMS. Note difference in scale.
In the NIT incubations, targeting the production of N2O through nitrification (precursor ), no or very low amounts of 46N2O (0.00 – 0.09 nmol g-1DW h-1) were produced across all experimental treatments and weeks (Table 7 and Figure 4). The contribution of nitrification to the total 15N-labelled N2O production (46N2O + 45N2O) by M. edulis and its shell biofilm in this series of experiments was very low, ranging between 1 % in CTRL to 3 % in OA. The production rate of 45N2O, on the other hand, was ± 10 to 500 times higher (0.03 – 2.95 nmol g-1DW h-1), started after an initial lag phase of 3 hours (Figure 4 and Table 7) and was the result of either random isotope paring, nitrifier denitrification or CND (Table 2).
The DNO2 incubations, targeting nitrifier denitrification (precursor ), showed the highest average 46N2O production rates of all 15N-tracer treatments, with highest average values (2.08 ± 0.96 nmol g-1DW h-1) recorded in CC after 6 weeks (Table 7). Nitrifier denitrification contributed, on average, 46 % to the total labelled N2O production of whole animals in CTRL and up to 59 % the OA treatment. In general, the 45N2O production rates were ± 1.2 to 3 times lower compared to 46N2O and produced by the combination of with or present or produced during the incubation (Table 7 and Figure 4).
Average 46N2O production rates in DNO3 incubations, targeting the CND pathway (precursor ), was relatively high at the start of the experiment (WK0; 0.12 ± 0.18 nmol g-1DW h-1) and contributed approximately 40 % of the total labelled N2O production in that week. In subsequent weeks, 46N2O production rates decreased 2- to 42-fold and all manipulated treatments had higher average 46N2O production rates compared to CTRL (Table 7 and Figure 4). On average, CND contributed between 4 % in CRTL up to 15 % in OW in the subsequent weeks. Average 45N2O production rates were 2 to 46 times higher across all experimental treatments compared to 46N2O and occurred in the DNO3 tracer treatment as a result of the random pairing of with or that were present or produced during the incubation (Tables 2, 7).
3.2.3. Oxygen microprofile
The biofilm oxygen microprofiles differed significantly between experimental treatments (PERMANOVA; pseudo-F=93.98; p=0.001), while the effect of week or the 2-way interaction of both was not significant (PERMANOVA; pseudo-F=0.92 and 1.23, respectively; both p>0.29). Pairwise testing revealed significant differences in oxygen microprofiles between all experimental treatments (p=0.001), except for the profiles measured in OA and CC (p=0.24). SIMPER revealed that at least 90% of the differences between CTRL and the other experimental treatments could be attributed to the first 600µm above the shell surface. In OW, oxygen concentrations above the shell declined faster and stronger, while in both acidified treatments (OA and CC), the onset of the decline in oxygenation was closer to the shell surface (biofilm thickness declined) and the magnitude of the decline was lower (net biofilm oxygen consumption decreased) (Figure 5).
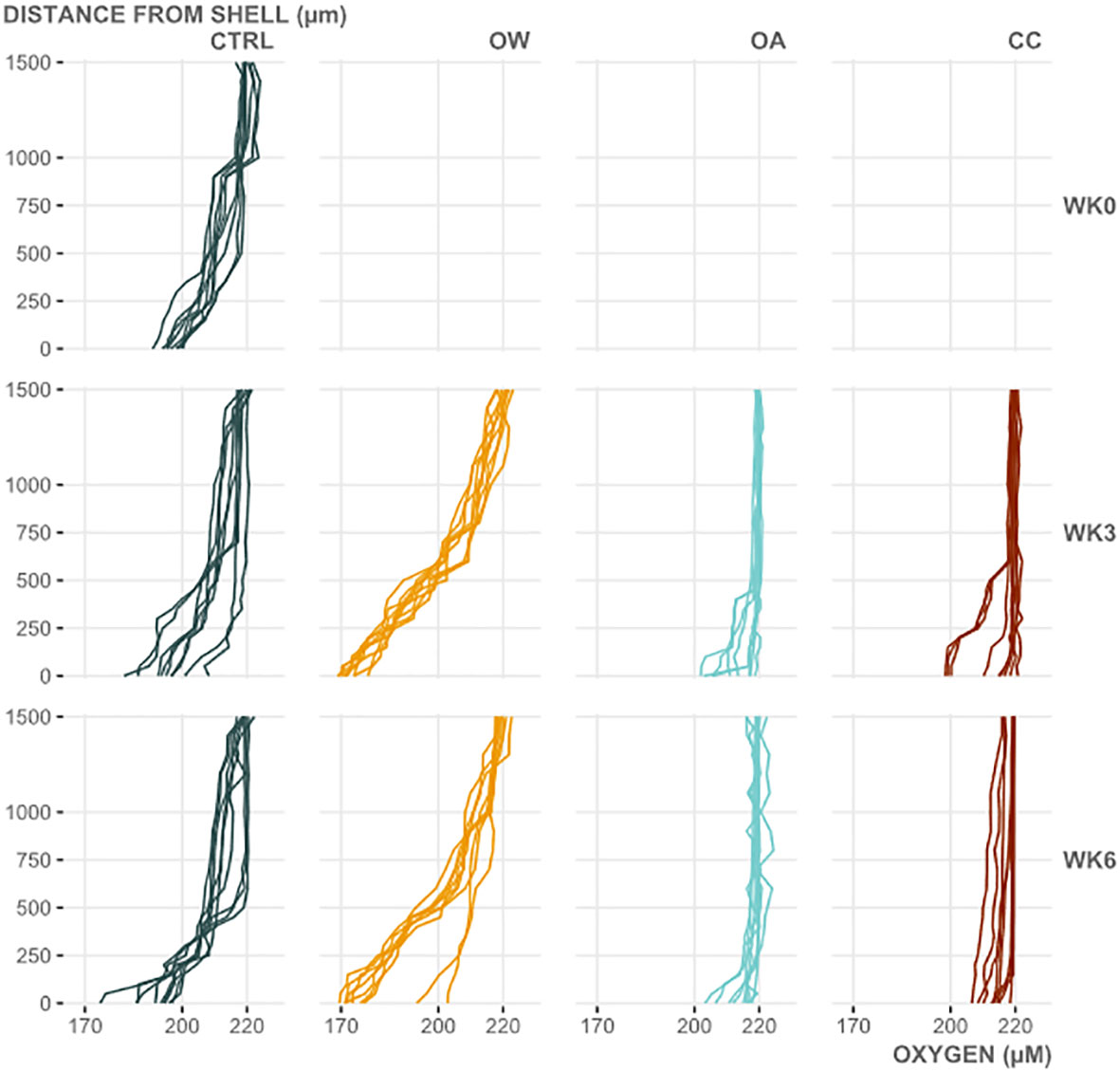
Figure 5 Vertical oxygen profiles of the microbial biofilm on Mytilus edulis shells, 1500µm above the shell surface, before seawater manipulations started (WK0) and after three and six weeks of manipulations (WK3 and WK6, respectively) in each experimental treatment [CTRL: control, OA: ocean acidification, OW: ocean warming and CC: climate change]. Profiles were measured on three random locations per replicate M. edulis shell (n=3) per timepoint and treatment.
4. Discussion
This study confirmed the contribution by the abundant and economically important blue mussel Mytilus edulis to marine N2O production (Stief et al., 2009; Heisterkamp et al., 2010; Bonaglia et al., 2017), as well as the relative importance of shell biofilms in this process (Heisterkamp et al., 2013). Our results on the magnitude and underlying processes of this N2O production show that nitrification, nitrifier denitrification and coupled nitrification-denitrification all served as potential pathways in N2O production. In contrast to earlier research by Heisterkamp et al. (2013) on dissected shell biofilms, nitrification of was not an important contributor to the total production by both the mussels and its shell biofilm. Additionally, we suggest that future climate change will not substantially affect total N2O production by blue mussels as the increase caused by higher temperatures is counteracted by a decreased shell biofilm activity due to ocean acidification and overall, N2O production declined over time in the climate change environment.
4.1. Pathways and contributions to N2O production
The current climate [CTRL] N2O production of blue mussels (including their associated shell biofilm) was in line with other studies (e.g. Heisterkamp et al., 2010; Heisterkamp et al., 2013; Gárate et al., 2019) and exclusively biological, as ZnCl2 poisoning fully inhibited N2O production. The shell biofilm contribution to total N2O production by M. edulis was important (± 70 %) and remained stable through time, as did the total N2O production rate. Although still a considerable contribution, the partitioning measured in this study was lower than previously reported by Heisterkamp et al. (2013), where N2O production originated almost exclusively from the shell biofilm (± 94 %). Possibly, methodological differences such as the use of natural seawater and a regular feeding regime in our experiments could have prompted the adept filter feeder M. edulis, with an established rich intestinal microbial system capable of considerable N2O production, to continue to do so throughout the duration of this experiment.
Nitrifiers, a combination of ammonium-oxidising bacteria and archaea, produce N2O during nitrification in oxic environments and during nitrifier denitrification in suboxic environments. Another set of bacteria, denitrifiers, produce N2O during anaerobic denitrification in anoxic environments, comprising the final steps of CND. These three pathways are considered to be the main oceanic sources of N2O production, while the main sink is the further reduction of N2O to N2 by (nitrifier) denitrification in sub- or anoxic environments (Bange et al., 2010). In this study, each of these three N2O producing pathways were observed. Contrary to Heisterkamp et al. (2013), where only dissected shells were used in a similar experiment, nitrification apparently did not play as an important role when whole animals (incl. shell biofilm) were incubated. Most likely, the presence of the living and digesting animal itself caused the resulting total N2O production to lean towards a more prominent inclusion of anoxic or suboxic pathways.
Denitrification of nitrite, targeted by the DNO2 precursor incubations, was the most dominant pathway of N2O production by M. edulis in the current climate scenario, corroborating the findings of Heisterkamp et al. (2013). Production of N2O in the presence of NaClO3, actively preventing the oxidation of nitrite to nitrate, was on average an order of magnitude higher than without the inhibitor present. As such, we suggest that the availability of nitrite might be a limiting factor in the production of N2O and that incomplete (nitrifier) denitrification of nitrite, where further reduction to N2 has not (yet) happened, is indeed a major pathway of N2O production by M. edulis and its biofilm. An overestimation of said denitrification due to anaerobic ammonium oxidation (anammox), where ammonium oxidises to N2 using nitrite under anoxic conditions, was assumed negligible since no anammox activity has been associated with mussel biofilms before (Marzocchi et al., 2021).
Denitrification of nitrite can be associated with the presence of M. edulis in different ways, both in and on the organism. The incomplete denitrification of nitrite to N2O by denitrifiers in the mussel’s anoxic digestive system could be the result of a high lysozyme activity, cutting short the denitrification pathway (Birkbeck and McHenery, 1982; Heisterkamp et al., 2013; Gárate et al., 2019). Additionally, this last step in the nitrifier denitrification pathway has only be attributed to a certain genus of nitrifying bacteria, Nitrosomonas sp., whose biofilm presence and/or (differential) activity is related to oxygen and nutrient availability (Schramm et al., 2000; Shrestha et al., 2002) and substrate affinity and availability (Foesel et al., 2008; Zhu et al., 2013). With the variable oxygenation of the shell biofilm measured in this study in mind, this could further explain why the produced N2O is not always readily reduced to N2 in a heterogeneous microbial biofilm on M. edulis’s outer shell. However, as the N2O flux was several orders of magnitude lower than that of the other measured N species (, and ), reduction of N2O to N2 must still have occurred to close the nitrogen budget in the experimental incubations. Research by Marzocchi et al. (2021) suggests that mussel-associated N2 production, although currently often overlooked, might be substantial. The high CTRL nitrate consumption rates observed in this study comply with this assumption.
4.2. Climate change effects on N2O production
By nature, adult blue mussels have a wide thermal tolerance window (e.g. Seuront et al., 2019; Kamermans and Saurel, 2022). Since this species has adapted to survive in such variable conditions, this study opted to push towards the boundaries of its natural tolerances in order to get the most realistic stress response to variable climate scenarios. In the North Sea, the highest mean temperatures occur over the summer period (when organisms were sampled) when the mean seawater pH is fairly stable (LifeWatch Belgium, 2015), meaning the adopted climate manipulations should elicit a relatively accurate physiological response outside M. edulis’ natural tolerances. Results show that the effects of seawater temperature rise and ocean acidification were generally of an antagonistic nature. N2O production rates of whole animals, as well as the production rate of dissected shells with an intact microbial biofilm, significantly increased with a higher temperature and significantly decreased in an acidified environment (whole animals) or a combined high temperature/low pH climate change environment (biofilm).
These experiments were set up to mimic North Sea summer conditions in CTRL, with additional manipulations in terms of temperature and/or pH levels according to the experimental treatments. Although this summer setting is the most relevant in terms of studying potential stress responses due to climate change, the temperature-dependent nature of physiological and microbial processes suggest that the absolute values of these responses would differ between the seasons (Lesser et al., 2010; Boulêtreau et al., 2012; Múgica et al., 2015). However, whether the observed patterns and nature of the interaction between ocean warming and acidification varies seasonally remains to be confirmed.
The increasing effect of elevated temperature on N2O production rates by M. edulis and its associated shell biofilm are most likely linked to the mussel’s concomitantly increasing filtration rates in combination with temperature-induced increased biofilm activity. As bivalve filter feeding increases with temperature (Kittner and Riisgård, 2005; Ong et al., 2017; Voet et al., 2021), more denitrifying microorganisms might be ingested and/or the concentration of available N precursors in the digestive system might increase. In addition, general microbial activity increases with warmer temperatures (Kroeze and Seitzinger, 1998; Boulêtreau et al., 2012). This study showed that even though the N2O production by whole animals increased with temperature, the relative contribution of the microbial shell biofilm remained stable over time in the ocean warming [OW] scenario. Indeed, this would suggest a similar positive effect of warmer environments on both the animal’s and the shell biofilm’s N2O producing capacities. Moreover, increased feeding rates will result in higher biodeposition rates and in turn, a higher nutrient load, potentially further stimulating (de)nitrification activities in the microbial shell biofilm.
On the other hand, the relative contribution of the shell biofilm to N2O production evidently decreased over time in both acidified scenarios, on top of the overall decrease in whole animal N2O production rates. A negative effect of ocean acidification on the marine nitrogen cycle was previously observed by Beman et al. (2011), reporting reduced oceanic nitrification at even relatively small experimental pH reductions (0.05-0.14). Together with a profound effect of reduced pH on the community composition of marine biofilms (Espinel-Velasco et al., 2021), this could potentially explain the observed lower N2O production rates under acidified conditions, as well as the decreasing relative contribution of the microbial biofilm. The latter is most likely also tied to a thinner, patchier or even disappearing shell biofilm, as is suggested by this study’s significantly affected oxygen microprofiles in both acidified conditions. The microprofile analysis indeed confirmed an adverse effect of pH on the metabolic activity (respiration) and composition of the shell biofilm. In both acidified treatments, perceived biofilm thickness considerably decreased, as well as the net biofilm oxygen consumption. Furthermore, net oxygen consumption at the shell surface sometimes dropped to (near) zero under acidified conditions, indicating either an incomplete biofilm cover or a profound shift in functional metabolism and/or the biofilm’s microbial makeup. A more in-depth functional analysis of M. edulis’ shell biofilm under climate change conditions is described in more detail by Dairain et al. (manuscript in preparation)2. Nitrifier denitrification, shown to be the dominant N2O producing pathway for M. edulis (e.g. this study and Heisterkamp et al., 2013), gained even more importance in the OA treatment. Affirmingly, this could (in part) be a result of the increasing prevalence of suboxic conditions provided within a thinning and/or receding shell biofilm.
4.3. Towards ecosystem-level effects
The blue mussel is by far the most abundant mollusc species colonising North Sea artificial hard substrates (Coolen et al., 2020) and these numbers will multiply even further by planned co-location strategies involving offshore wind farms and bivalve mariculture (Schupp et al., 2019; MSP, 2020; Steins et al., 2021). Considering the prevalence of these organisms and the potential biofilm-substrate they provide are on the rise, their role in the local emission of the potent GHG nitrous oxide could be noteworthy and a valuable research topic. On the other hand, this study also suggested that reduction of this GHG to inert N2 could be prevalent in or around these mussels, as was the case in other recent mussel microbiome research (Marzocchi et al., 2021). This would suggest that colonising and aquaculture mussel communities could play a role in the removal of nitrogen from the water column, actively counteracting marine eutrophication. Contrary to climate change-induced changes in (i.a.) survival or ecophysiology of blue mussels (Voet et al., 2021), the antagonistic effects of temperature and pH on N2O production described in this study would most likely not amount to large-scale enhancements of future mussel-associated N2O emissions. With the expansion of the offshore renewable energy effort in a necessary attempt to abate GHG emissions causing global climate change, insights such as described in this study are paramount. Complementary, marine climate science would benefit greatly from further research into the link between impacts of future climate effects on current marine communities and the potential effects of adaptation and ecophysiological acclimatisation over longer time periods.
5. Conclusion
The blue mussel Mytilus edulis and its microbial shell biofilm produce N2O through three potential pathways, i.e. nitrification, denitrification (CND) and nitrifier denitrification (using ammonium, nitrate and nitrite as a precursor, respectively), but nitrifier denitrification proved to be the main pathway. The relative importance of these pathways and the relative contribution of the shell biofilm to the blue mussel’s total N2O production, as well as the biofilm thickness, metabolic activity and coverage are all affected by warming, acidification, or the combination of both. The effects of temperature and pH were often of an antagonistic nature, predicting a relatively small net effect on local N2O production in future climate environments. In a future setting with increasing blue mussel densities, such as proliferating offshore wind farms in possible multifunctional co-use with bivalve mariculture, animal-associated N2O and N2 production will play a major role in local nitrogen cycling and have potential knock-on effects on local greenhouse gas emissions, eutrophication levels and bioavailable nitrogen concentrations. An understanding of the underlying pathways and inner dynamics of the OWF- and aquaculture-bound N cycling will therefore be indispensable to face and mitigate the additional impact of a changing marine climate.
Data availability statement
The raw data supporting the conclusions of this article will be made available by the authors, without undue reservation.
Author contributions
HV: Conceptualization, Methodology, Validation, Formal analysis, Investigation, Resources, Data curation, Writing – original draft, Writing – review and editing, visualization. KS: Conceptualization, Methodology, Validation, Resources, Writing – review and editing, supervision, funding acquisition. TM: Methodology, Validation, Resources, Writing – review and editing, supervision, project administration. SB: Methodology, Formal analysis, Writing – review and editing. PB: Funding acquisition, Resources, Writing – review and editing. CC: Conceptualization, Methodology, Validation, Resources, Writing – review and editing, supervision, funding acquisition. JV: Conceptualization, Methodology, Validation, Resources, Writing – review and editing, supervision, project administration, funding acquisition. All authors contributed to the article and approved the submitted version.
Funding
The research leading to results presented in this publication was funded through BELSPO BRAIN-be project BR/175/A1/PERSUADE, additional funding obtained from Ghent University’s Special Research Fund (BOF) through GOA project 01G02617 and carried out with infrastructure funded by EMBRC Belgium FWO project GOH3817N. This work was further supported by logistic support from the Research Vessel Simon Stevin, the vessel Stream (Brevisco), the scientific diving teams of VLIZ and RBINS and by access to the ILVO aquaculture pilot project Value@Sea (with partners Belwind, Brevisco, Sioen Industries, Colruyt Group, C-Power, DEME, RBINS – OD Nature, Lobster Fish and Ghent University). Practical and analytical support by the MarBiol Research Group (UGent), VLIZ, the Chemical Oceanography Unit (COU; University of Liège) and the Isotope Bioscience Laboratory (ISOFYS; UGent) was indispensable in the realisation of this study.
Conflict of interest
The authors declare that the research was conducted in the absence of any commercial or financial relationships that could be construed as a potential conflict of interest.
Publisher’s note
All claims expressed in this article are solely those of the authors and do not necessarily represent those of their affiliated organizations, or those of the publisher, the editors and the reviewers. Any product that may be evaluated in this article, or claim that may be made by its manufacturer, is not guaranteed or endorsed by the publisher.
Supplementary material
The Supplementary Material for this article can be found online at: https://www.frontiersin.org/articles/10.3389/fmars.2023.1101469/full#supplementary-material
Footnotes
- ^ Voet, H. E. E., De Luca, L. V., Vanaverbeke, J., and Soetaert, K. (manuscript in preparation). Modelling the combined effects of climate change on an offshore wind farm ecosystem with blue mussel (Mytilus edulis) aquaculture in multifunctional co-use.
- ^ Dairain, A., Voet, H. E. E., Vafeiadou, A.-M., de Meester, N., Rigaux, A., van Colen, C., et al. (manuscript in preparation). Structurally stable but functionally disrupted marine epi-microbial communities under a future climate change scenario: impact on the nitrogen cycle.
References
Anderson M. J., Gorley R. N., Clarke K. R. (2008). PERMANOVA+ for PRIMER: guide to software and statistical methods. Primer-E Plymouth.
Avdelas L., Avdic-Mravlje E., Borges Marques A. C., Cano S., Capelle J. J., Carvalho N., et al. (2021). The decline of mussel aquaculture in the European union: causes, economic impacts and opportunities. Rev. Aquacult. 13, 91–118. doi: 10.1111/raq.12465
Bange H. W., Freing A., Kock A., Löscher C. R. (2010). “Marine pathways to nitrous oxide,” in Nitrous oxide and climate change. Ed. Smith K. (London: Earthscan), 36–62.
Bates D., Maechler M., Bolker W., Walker S. (2015). Fitting linear mixed-effects models using lme4. J. Stat. Softw. 67 (1), 1–48. doi: 10.18637/jss.v067.i01
Belser L. W., Mays E. L. (1980). Specific inhibition of nitrite oxidation by chlorate and its use in assessing nitrification in soils and sediments. Appl. Environ. Microbiol. 39, 505–510. doi: 10.1128/aem.39.3.505-510.1980
Beman J. M., Chow C.-E., King A. L., Feng Y., Fuhrman J. A., Andersson A., et al. (2011). Global declines in ocean nitrification rates as a consequence of ocean acidification. Proc. Natl. Acad. Sci. 108 (1), 208–213. doi: 10.1073/pnas.1011053108
Bindoff N. L., Cheung W. W. L., Kairo J. G., Arístegui J., Guinder V. A., Hallberg R., et al (2019) “Changing ocean, marine ecosystems, and dependent communities” in IPCC special report on the ocean and cryosphere in a changing climate. Eds. Pörtner H. O., Roberts D. C., Masson-Delmotte V., Zhai P., Tignor M., Poloczanska E., Mintenbeck K., Alegría A., Nicolai M., Okem A., Petzold J., Rama B., Weyer N. M. Cambridge University Press, Cambridge, UK and New York, NY, USA, 447–587. doi: 10.1017/9781009157964.007
Birkbeck T. H., McHenery J. G. (1982). Degradation of bacteria by Mytilus edulis. Mar. Biol. 72, 7–15. doi: 10.1016/0305-0491(89)90174-0
Bonaglia S., Brüchert V., Callac N., Vicenzi A., Chi Fru E., Nascimento F. J. A. (2017). Methane fluxes from coastal sediments are enhanced by macrofauna. Nat. Sci. Rep. 7 (1), 13145. doi: 10.1038/s41598-017-13263-w
Boulêtreau S., Salvo E., Lyautey E., Mastrorillo S., Garabetian F. (2012). Temperature dependence of denitrification in phototrophic river biofilms. Sci. Total Environ. 416, 323–328. doi: 10.1016/j.scitotenv.2011.11.066
Buck B. H., Langan R. (2017). “Chapter 14: Epilogue–pathways towards sustainable ocean food production,” in Aquaculture perspective of multi-use sites in the open ocean. Eds. Buck B. H., Langan R., Springer International Publishing, ISBN: 978-3-319-51159-7 395–404.doi: 10.1007/978-3-319-51159-7
Coolen J. W. P., van der Weide B., Cuperus J., Blomberg M., Van Moorsel G. W. N. M., Faasse M. A. A., et al. (2020). Benthic biodiversity on old platforms, young wind farms and rocky reefs. ICES J. Mar. Sci. 77 (3), 1250–1265. doi: 10.1093/icesjms/fsy092
Costello C., Cao L., Gelcich S., Cisneros-Mata M. A., Free C. M., Froehlich H. E., et al. (2020). The future of food from the sea. Nature 588, 95–100. doi: 10.1038/s41586-020-2616-y
Cranford P. J. (2019). “Chapter 8: Magnitude and extent of water clarification services provided by bivalve suspension feeding,” in Goods and services of marine bivalves. Eds. Smaal A. C., Ferreira J. G., Grant J., Petersen J. K., Strand Ø, Springer Cham 119–141. doi: 10.1007/978-3-319-96776-9
Degraer S., Carey D. A., Coolen J. W. P., Hutchison Z. L., Kerckhof F., Rumes B., et al. (2020). Offshore wind farm artificial reefs affect ecosystem structure and functioning: A synthesis. in special issue on understanding the effects of offshore wind energy development on fisheries. Oceanography 33 (4), 48–57. doi: 10.5670/oceanog.2020.405
De Mesel I., Kerckhof F., Norro A., Rumes B., Degraer S. (2015). Succession and seasonal dynamics of the epifauna community on offshore wind farm foundations and their role as stepping stones for non-indigenous species. Hydrobiologia 756, 37–50. doi: 10.1007/s10750-014-2157-1
Espinel-Velasco N., Tobias-Hünefeldt S. P., Karelitz S., Hoffmann L. J., Morales S. E., Lamare M. D. (2021). Reduced seawater pH alters marine biofilms with impacts for marine polychaete larval settlement. Mar. Env. Res. 167, 105291. doi: 10.1016/j.marenvres.2021.105291
Foesel B. U., Gieseke A., Schwermer C., Stief P., Koch L., Cytryn E., et al. (2008). Nitrosomonas Nm143-like ammonia oxidizers and nitrospira marina-like nitrite oxidizers dominate the nitrifier community in a marine aquaculture biofilm. FEMS Microbiol. Ecol. 63 (2), 192–204. doi: 10.1111/j.1574-6941.2007.00418.x
Fox-Kemper B., Hewitt H. T., Xiao C., GAðalgeirsdóttir G., Drijfhout S. S., Edwards T. L., et al. (2021). “Ocean, cryosphere and Sea level change,” in Climate change 2021: The physical science basis. contribution of working group I to the sixth assessment report of the intergovernmental panel on climate change. Eds. Masson-Delmotte V., Zhai P., Pirani A., Connors S. L., Péan C., Berger S., Caud N., Chen Y., Goldfarb L., Gomis M. I., Huang M., Leitzell K., Lonnoy E., Matthews J. B. R., Maycock T. K., Waterfield T., Yelekçi O., Yu R., Zhou B. (Cambridge University Press, Cambridge, United Kingdom and New York, NY, USA), 1211–1362. doi: 10.1017/9781009157896.011
Gárate M., Moseman-Valtierra S., Moen A. (2019). Potential nitrous oxide production by marine shellfish in response to warming and nutrient enrichment. Mar. pollut. Bull. 146, 236–246. doi: 10.1016/j.marpolbul.2019.06.025
GWEC (Global Wind Energy Council) (2021). Global wind report 2021. Eds. Lee J., Zhao F., Dutton A., Backwell B., Fiestas R., Qiao L., Balachandran N., Lim S., Liang W., Clarke E., Lathigara A., Younger D. R., 80.
Halekoh U., Højsgaard S. (2014). A kenward-roger approximation and parametric bootstrap methods for tests in linear mixed models - the r package pbkrtest. J. Stat. Softw. 59 (9).
Heisterkamp I. M., Schramm A., de Beer D., Stief P. (2010). Nitrous oxide production associated with coastal marine invertebrates. Mar. Ecol. Prog. Ser. 415, 1–9. doi: 10.3354/meps08727
Heisterkamp I. M., Schramm A., Larsen L. H., Svenningsen N. B., Lavik G., de Beer D., et al. (2013). Shell biofilm-associated nitrous oxide production in marine molluscs: processes, precursors and relative importance. Environ. Microbiol. 15 (7), 1943–1955. doi: 10.1111/j.1462-2920.2012.02823.x
Hoegh-Guldberg O., Cai R., Poloczanska E. S., Brewer P. G., Sundby S., Hilmi K., et al. (2014). “The ocean,” in Climate change 2014: Impacts, adaptation, and vulnerability. part b: Regional aspects. contribution of working group II to the fifth assessment report of the intergovernmental panel on climate change. Eds. Barros V. R., Field C. B., Dokken D. J., Mastrandrea M. D., Mach K. J., Bilir T. E., Chatterjee M., Ebi K. L., Estrada Y. O., Genova R. C., Girma B., Kissel E. S., Levy A. N., MacCracken S., Mastrandrea P. R., White L. L. (Cambridge, United Kingdom and New York, NY, USA: Cambridge University Press), 1655–1731.
IPCC (2021). “Summary for policymakers,” in Climate change 2021: The physical science basis. contribution of working group I to the sixth assessment report of the intergovernmental panel on climate change. Eds. Masson-Delmotte V., Zhai P., Pirani A., Connors S. L., Péan C., Berger S., Caud N., Chen Y., Goldfarb L., Gomis M. I., Huang M., Leitzell K., Lonnoy E., Matthews J. B. R., Maycock T. K., Waterfield T., Yelekçi O., Yu R., Zhou B. (Cambridge University Press, United Kingdom and New York, NY, USA, In press). doi: 10.1017/9781009157896
Ivanov E., Capet A., De Borger E., Degraer S., Delhez E. J. M., Soetaert K., et al. (2021). Offshore wind farm footprint on organic and mineral particle flux to the bottom. Front. Mar. Sci. 8, 631799. doi: 10.3389/fmars.2021.631799
Kamermans P., Saurel C. (2022). Interacting climate change effects on mussels (Mytilus edulis and m. galloprovincialis) and oysters (Crassostrea gigas and ostrea edulis): experiments for bivalve individual growth models. Aquat. Living Resour. 35 (1). doi: 10.1051/alr/2022001
Kittner C., Riisgård H. U. (2005). Effect of temperature on filtration rate in the mussel Mytilus edulis: no evidence for temperature compensation. Mar. Ecol. Prog. Ser. 305, 147–152. doi: 10.3354/meps305147
Kroeze C., Seitzinger S. P. (1998). Nitrogen inputs to rivers, estuaries and continental shelves and related nitrous oxide emissions in 1990 and 2050: a global model. Nutr. Cycl. Agroecosyst. 52, 195–212. doi: 10.1023/A:1009780608708
Lesser M., Bailey M., Merselis D., Morrison J. (2010). Physiological response of the blue mussel mytilus edulis to differences in food and temperature in the gulf of Maine. Comp. Biochem. Physiol. Part A Mol. Integr. Physiol. 156, 541–551. doi: 10.1016/j.cbpa.2010.04.012
LifeWatch Belgium (2015) Flanders Marine institute. Thornton measuring buoy (C-power). Available at: https://rshiny.lifewatch.be/buoy-data/ (Accessed 30 June 2018).
Marzocchi U., Bonaglia S., Zaiko A., Quero G. M., Vybernaite-Lubiene I., Politi T., et al. (2021). Zebra mussel holobionts fix and recycle nitrogen in lagoon sediments. Front. Microbiol. 11. doi: 10.3389/fmicb.2020.610269
Mehrbach C., Culberson C. H., Hawley J. E., Pytkowicz R. M. (1973). Measurement of the apparent dissociation constants of carbonic acid in seawater at atmospheric pressure. Limnol. Ocean 18, 897–907. doi: 10.4319/lo.1973.18.6.0897
Mosier A., Kroeze C., Nevison C., Oenema O., Seitzinger S., Van Cleemput O. (1998). Closing the global N2O budget: nitrous oxide emissions through the agricultural nitrogen cycle — OECD/IPCC/IEA phase II development of IPCC guidelines for national greenhouse gas inventory methodology. Nutr. Cycl. Agroecosyst. 52, 225–248. doi: 10.1023/A:1009740530221
MSP – Marine Spatial Plan 2020-2026 (2020) Marine environment service, federal public service – health, food chain safety and environment. Available at: https://www.health.belgium.be/en/environment/seas-oceans-and-antarctica/north-sea-and-oceans/marine-spatial-plan (Accessed 1 June 2021).
Múgica M., Sokolova I. M., Izagirre U., Marigómez I. (2015). Season-dependent effects of elevated temperature on stress biomarkers, energy metabolism and gamete development in mussels. Mar. Environ. Res. 103, 1–10. doi: 10.1016/j.marenvres.2014.10.005
Myhre G., Shindell D., Bréon F.-M., Collins W., Fuglestvedt J., Huang J., et al. (2013). “Anthropogenic and natural radiative forcing,” in Climate change 2013: The physical science basis. contribution of working group I to the fifth assessment report of the intergovernmental panel on climate change. Eds. Stocker T. F., Qin D., Plattner G.-K., Tignor M., Allen S. K., Boschung J., Nauels A., Xia Y., Bex V., Midgley P. M. (Cambridge, United Kingdom and New York, NY, USA: Cambridge University Press), 659–740.
Ong E. Z., Briffa M., Moens T., Van Colen C. (2017). Physiological responses to ocean acidification andwarming synergistically reduce condition of the common cockle Cerastoderma edule. Marine Environmental Research. doi: 10.1016/j.marenvres.2017.07.001
Pierrot D., Lewis E., Wallace D. W. R. (2006). MS Excel Program Developed for CO2System Calculations. ORNL/CDIAC-105a. Carbon Dioxide Inf. Anal. Center, Oak Ridge Natl. Lab. US Dep. Energy, Oak Ridge, Tennessee.
R Core Team (2019). R: A language and environment for statistical computing. r foundation for statistical computing (Vienna, Austria).
Schramm A., De Beer D., Gieseke A., Amann R. (2000). Microenvironments and distribution of nitrifying bacteria in a membrane-bound biofilm. Environ. Microbiol. 6), 680–686. doi: 10.1046/j.1462-2920.2000.00150.x
Schupp M. F., Bocci M., Depellegrin D., Kafas A., Kyriazi Z., Lukic I., et al. (2019). Towards a common understanding of ocean multi-use. Front. Mar. Sci. 6, 165. doi: 10.3389/fmars.2019.00165
Seuront L., Nicastro K. R., Zardi G. I., Goberville E. (2019). Decreased thermal tolerance under recurrent heat stress conditions explains summer mass mortality of the blue mussel Mytilus edulis. Sci. Rep. 9, 17498. doi: 10.1038/s41598-019-53580-w
Shrestha N. K., Hadano S., Kamachi T., Okura I. (2002). Dinitrogen production from ammonia by nitrosomonas europaea. Appl. Catal. A: Gen. 237 (1-2), 33–39. doi: 10.1016/S0926-860X(02)00279-X
Slavik K., Lemmen C., Zhang W., Kerimoglu O., Klingbeil K., Wirt K. W. (2019). The large-scale impact of offshore wind farm structures on pelagic primary productivity in the southern north Sea. Hydrobiologia 845, 35–53. doi: 10.1007/s10750-018-3653-5
Smith H. A., Sharp K. (2012). Indigenous climate knowledges. Wiley Interdiscip. Rev.: Climate Change 3 (5), 467–476. doi: 10.1002/wcc.185
Stein L. Y., Yung Y. L. (2003). Production, isotopic composition, and atmospheric fate of biologically produced nitrous oxide. Annu. Rev. Earth Planet Sci. 31, 329–356. doi: 10.1146/annurev.earth.31.110502.080901
Steins N. A., Veraart J. A., Klostermann J. E. M., Poelman M. (2021). Combining offshore wind farms, nature conservation and seafood: Lessons from a Dutch community of practice. Mar. Policy 126, 104371. doi: 10.1016/j.marpol.2020.104371
Stief P., Poulsen M., Nielsen L. P., Brix H., Schramm A. (2009). Nitrous oxide emission by aquatic macrofauna. Proc. Natl. Acad. Sci. U.S.A. 106, 4296–4300. doi: 10.1073/pnas.0808228106
Tallec G., Garnier J., Billen G., Gousailles M. (2008). Nitrous oxide emissions from denitrifying activated sludge of urban wastewater treatment plants, under anoxia and low oxygenation. Bioresour. Technol. 99 (7), 2200–2209. doi: 10.1016/j.biortech.2007.05.025
Voet H. E. E., Van Colen C., Vanaverbeke J. (2021). Climate change effects on the ecophysiology and ecological functioning of an offshore wind farm artificial hard substrate community. Sci. Total Environ. 810, 152194. doi: 10.1016/j.scitotenv.2021.152194
Widdicombe S., Beesley A., Berge J. A., Dashfield S. L., McNeill C. L., Needham H. R., et al. (2013). Impact of elevated levels of CO2 on animal mediated ecosystem function: The modification of sediment nutrient fluxes by burrowing urchins. Mar. pollut. Bull. 73 (2), 416–427. doi: 10.1016/j.marpolbul.2012.11.008
Keywords: nitrous oxide - N2O, Mytilus edulis, climate change, biofilm, production pathways
Citation: Voet H, Soetaert K, Moens T, Bodé S, Boeckx P, Van Colen C and Vanaverbeke J (2023) N2O production by mussels: Quantifying rates and pathways in current and future climate settings. Front. Mar. Sci. 10:1101469. doi: 10.3389/fmars.2023.1101469
Received: 17 November 2022; Accepted: 30 January 2023;
Published: 16 February 2023.
Edited by:
Inna Sokolova, University of Rostock, GermanyReviewed by:
Bo Gustafsson, Stockholm University, SwedenSonglin Liu, South China Sea Institute of Oceanology (CAS), China
Copyright © 2023 Voet, Soetaert, Moens, Bodé, Boeckx, Van Colen and Vanaverbeke. This is an open-access article distributed under the terms of the Creative Commons Attribution License (CC BY). The use, distribution or reproduction in other forums is permitted, provided the original author(s) and the copyright owner(s) are credited and that the original publication in this journal is cited, in accordance with accepted academic practice. No use, distribution or reproduction is permitted which does not comply with these terms.
*Correspondence: Jan Vanaverbeke, anZhbmF2ZXJiZWtlQG5hdHVyYWxzY2llbmNlcy5iZQ==