- Marine Evolutionary Biology, Christian-Albrechts-University, Kiel, Germany
The syngnathid fish group (seahorses, pipefishes and seadragons) is a fascinating lineage associated with an array of evolutionary peculiarities that include diverse morphologies and their unique male pregnancy. These oddities also extend to their immune systems, with a growing body of research highlighting a range of intriguing immunological characteristics and genomic rearrangements, which pose questions regarding their evolutionary history and immune strategies. The functional loss of the major histocompatibility complex class II pathway (MHC II) in the Syngnathus genus and related pathway components in the seahorse (Hippocampus) were two discoveries that initially piqued interest. This sparked discussions concerning immune capabilities, possible facilitative roles in advanced male pregnancy evolution through means of evoking immunological tolerance, as well as a general re-evaluation of how we interpret vertebrate immunological plasticity. Experimental approaches have attempted to clarify further the impact of immune repertoire loss on the efficacy of the syngnathid immune response, specificities regarding the pathways in play during pregnancy as well as the concept of immunological inheritance. The first characterization of the immune cell repertoire of Syngnathus typhle using scRNA-seq represents the latest step to understanding the immune dynamics of these enigmatic fish. This report serves as a review for the immunological insights into the fascinating syngnathid fish group; encompassing their evolutionary history, immune cell populations, links to male pregnancy, and sex specificity, in addition to highlighting future research opportunities in need of investigation.
1 Introduction
Facilitated by the evolution of self-non-self recognition mechanisms, the immune system provides crucial protection against harmful pathogens as well as maintaining immune homeostasis. Over time, the immune system has evolved into a diverse set of specific, rapid and modulatory pathways facilitated by multifunctional cell types and chemical signals. Innate immunity represents the first line of defence, a rapid, generally non-specific response that initiates antigen-presentation, inflammation and activation of the complement and adaptive immune systems (Medzhitov and Janeway, 2000). The adaptive immune system provides highly specific responses upon pathogen re-exposure and is chief orchestrator of immune memory (Bonilla and Oettgen, 2010). Assisted by the major histocompatibility complexes (MHC I and II), antigen presentation processes must equally be able to determine self from non-self in order to avoid autoimmune related responses (Ljunggren and Kärre, 1990; Edwards and Hedrick, 1998). Forms of the evolutionary conserved innate immunity are found in all vertebrates and most invertebrates, evolving prior to the adaptive branch, which first emanated in primordial jawed vertebrates and has since become a hallmark of vertebrate evolution (Flajnik and Kasahara, 2010). Adaptive immune components are well conserved among gnathostomes from sharks to mammals, and cases of genomic immune system plasticity were deemed rare among this lineage. This has since been refuted with marine species such as the elephant shark (Venkatesh et al., 2014) and coelacanth (Amemiya et al., 2013), as well as teleost fishes including anglerfish (Dubin et al., 2019; Swann et al., 2020), Gadiformes (Star et al., 2011; Malmstrøm et al., 2016) and several representatives of syngnathids (Haase et al., 2013; Roth et al., 2020) exhibiting remarkable cases of adaptive immune system remodelling. These cases raise questions concerning the conventions of vertebrate immunity and the underlying requirements for a functional immune system.
One group in particular that has attracted significant interest is the syngnathid fish group comprising seahorses, pipefishes, pipehorses and seadragons (Herald, 1959; Dawson, 1986). The bizarre and diverse morphologies held among syngnathid teleosts are emblematic of their peculiar evolutionary path, having also evolved the sole instance of male pregnancy in the animal kingdom (Stölting and Wilson, 2007). Recent discoveries have highlighted the occurrence of adaptive immune system remodelling in some pipefish and seahorse species, giving rise to a convoluted and drastically alternative set of immune defences (Bahr and Wilson, 2011; Haase et al., 2013; Roth et al., 2020). Alongside and succeeding these studies, research has delved further into the molecular underpinnings that shaped these enigmatic fish defences; exploring the links with male pregnancy evolution, immunological tolerance, transgenerational immune priming and alternative immune strategies (Figure 1) (Roth et al., 2012; Whittington et al., 2015; Beemelmanns and Roth, 2016a; Keller and Roth, 2020; Whittington and Friesen, 2020; Parker et al., 2022). This review attempts to summarise the growing body of research concerning the syngnathid immune system, its evolution, and associations with other facets of their physiology and reproduction.
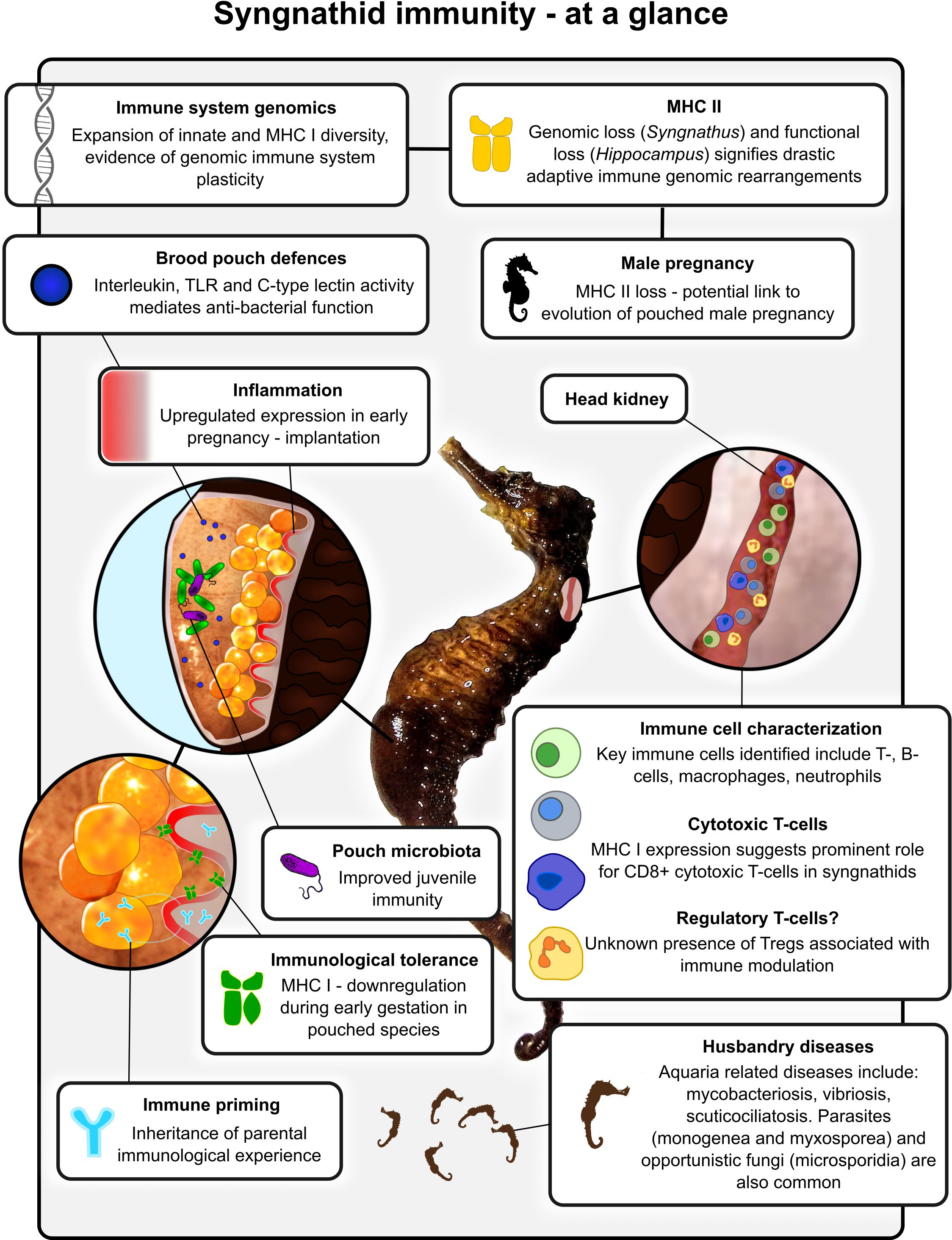
Figure 1 Schematic diagram highlighting some of the key immunological characteristics and research findings associated with syngnathid fishes.
2 Genomics of the immune system
The last decade has seen the release of several high quality syngnathid genomes. Most of the studies focused around the genetics underlying syngnathid unique morphology and male pregnancy evolution (Small et al., 2016; Roth et al., 2020; Zhang et al., 2020; He et al., 2021; Li et al., 2021; Qu et al., 2021; Small et al., 2022). The genomes were fundamental to linking the loss of particular genes to a number of morphological traits such as the loss of mineralized teeth (loss of P/Q-rich scpp genes), pelvic fins (loss of tbx4) and ribs (HOX gene losses) (Lin et al., 2016; Small et al., 2016; Zhang et al., 2020; Qu et al., 2021). This research also paved the way towards the realization that the genetics of male pregnancy are highly complex. The diversity of intricate brooding structures, such as the Hippocampus pouch and Syngnathus skin folds, as well as behaviour and immune system adaptations make it difficult to disentangle the evolutionary path and genetic foundations of male pregnancy. Nonetheless, some advancements in the understanding of male pregnancy evolution have been made. For example, syngnathid genome studies reported expansions of patristacin (pastn) genes, metalloproteases that are involved in egg hatching (Small et al., 2016; Lin et al., 2016). This, together with pregnancy-related expression patterns suggests a role of pastn genes in male pregnancy evolution. The immune gene repertoire of syngnathids also appears to be modified when compared to other teleost fish, showing expansions and contractions of certain crucial gene families and pathways, as well as the loss of some key adaptive immune system components (Roth et al., 2020).
2.1 The major histocompatibility complex
The major histocompatibility complex (MHC) represents a group of adaptive immune system genes. In tetrapods and chondrichthyes, the MHC genes are closely linked on the same chromosomal region. However, in teleosts this region is split between different chromosomes and lacks defined synteny. The MHC is usually divided into two major classes that represent two arms of the adaptive immune system - I and II. The MHC class I pathway is responsible for protection against viruses and malfunctioning cells, while the MHC class II pathway defends against extracellular threats, like bacteria and parasites. Both are also crucial mediators of self-non-self recognition and maintenance of self-tolerance (Cooper and Alder, 2006; Neefjes et al., 2011; Grimholt, 2016; Roth et al., 2020). The genes that encode MHC class I and II molecules are present in multiple copies within a genome and often are highly polymorphic. These genes are further grouped into classical and non-classical subsets, depending on the structure and binding capacities of the encoded proteins. The latter is often deduced based on homology. Classical molecules are highly expressed and polymorphic; they bind peptide antigens and present them to immune cells. Non-classical molecules vary in their roles, from binding of non-peptide antigens to accessory molecules (Dijkstra et al., 2013; Grimholt et al., 2015; Grimholt, 2016). Five MHC class I lineages exist in teleosts - U, Z, S, L, and P, with all classical MHC I molecules in teleosts belonging to the U lineage (Grimholt et al., 2015; Grimholt, 2016). Teleost MHC class II molecules are divided into three lineages - A, B and E, with classical teleost MHC II molecules belonging to the A lineage, which seem to be present in all species (with a few exceptions) (Dijkstra et al., 2013; Grimholt, 2016).
Following the report in cod (Star et al., 2011) it was discovered that the pipefish Syngnathus typhle lacks expression of the mhc II α/β, cd4 and the MHC class II transactivator (ciita) genes, and express a truncated version of MHC II invariant chain gene (cd74) (Haase et al., 2013). Consequently, it was hypothesized that like cod, S. typhle had lost the MHC II adaptive immune system component. The loss of these genes along with the activation induced cytidine deaminase (aicda) gene was then confirmed with genome studies, first in Syngnathus scovelli (Small et al., 2016) and then in other Syngnathus species (Roth et al., 2020). To date, there are only four vertebrate clades that have lost the conventional MHC class II pathway. The Callorhinchus milii (elephant shark) genome lacks cd4 and related transcription factors, but contains polymorphic MHC II genes (Venkatesh et al., 2014). The Syngnathus genus, the entire Gadiformes order, Lophius piscatorius and some ceratioid species, all appear to have lost key MHC II pathway genes rendering it non-functional (Star et al., 2011; Haase et al., 2013; Malmstrøm et al., 2016; Small et al., 2016; Dubin et al., 2019; Swann et al., 2020; Roth et al., 2020).
The Hippocampus species have modifications in the autoimmune regulator (aire), cd74, and ciita genes, which also suggests an altered adaptive immune system. CIITA regulates the expression of MHC I and MHC II genes in antigen-presenting cells, and AIRE is responsible for negative selection on self-recognizing T cells. Genes encoding for AIRE and CIITA in Hippocampus have highly divergent exons compared to other teleost species, while CD74 has modifications in the CLIP (class II-associated invariant chain peptide) region (Roth et al., 2020). In the mammalian adaptive immune system, the CLIP region blocks the peptide-binding groove of a classical MHC class II molecule until it reaches the MHC class II compartment (MIIC) of the late endosome. The CLIP then is removed from the peptide-binding groove through the interaction with the non-classical MHC II DM molecule, allowing other peptides (e.g. antigens) to bind to it. In mammals, both the CD74 with intact CLIP and the MHC II DM are crucial for normal MHC II pathway function. Curiously, all teleosts appear to lack the aforementioned MHC II DM molecule (Neefjes et al., 2011; Dijkstra et al., 2013). To date the mechanism with which CLIP dissociates from the MHC II peptide-binding groove in teleosts is unknown. Dijkstra and colleagues suggested that the accessory molecule might not be needed at all, since some MHC II molecules bind CLIP with low affinity and that CLIP was observed to disassociate rapidly at low pH, or other non-classical MHC II molecules could functionally replace the DM (Dijkstra et al., 2013). However, the fact that CD74 with intact CLIP is preserved in most sequenced teleost species suggests that either it is homologous in function to mammalian CD74, and thus species that lost it have a modified MHC II pathway, or it has functions outside of the pathway or even the immune system.
In syngnathids, the MHC I pathway also appears to be modified. When compared to Syngnathiformes without male pregnancy, species with pregnancy (Nerophinae, Hippocampus, and Syngnathus) were estimated to have an expansion of genes encoding the MHC I U lineage molecules (Roth et al., 2020), though another study reports smaller numbers in some Syngnathus and Hippocampus species (11/30 vs 7/11) (Qu et al., 2021). Surprisingly, these expanded genes form distinct clusters on a gene tree. Such clustering might represent sub- or neofunctionalization, serving as an adaptation to male pregnancy and a compensatory mechanism for the MHC II loss, where certain MHC I lineages specialise on the cross-presentation pathway or perform an entirely different immune function. Similar patterns of MHC I expansions and clustering can be observed within the Gadiformes order (Star et al., 2011; Malmstrøm et al., 2016).
2.2 Alternative immune pathways
Despite high metabolic costs, the gnathostome adaptive immune system offers a tremendous advantage in a form of highly specific immune responses and immune memory. The key components of the system are highly conserved throughout the gnathostome lineage and thus it seems highly unusual for a taxon to lose a core part of its functionality (Flajnik, 2018).
Since the first report in cod there was a debate concerning the reasons and mechanisms of the MHC II loss in certain teleost clades. Two broad scenarios have been proposed so far. The first scenario simply proposes that the MHC II pathway is dispensable in teleosts and was lost through genetic drift. The second suggests that the loss was mediated by directional selection. The authors then proposed two hypotheses for the second scenario: the metabolic shift hypothesis and the functional shift hypothesis (Star and Jentoft, 2012). Though originally discussed for cod, these hypotheses can be applied to all clades that have lost the MHC II pathway.
The metabolic shift hypothesis describes a situation where under particular environmental conditions the metabolic gains of losing the MHC II pathway would outweigh the protection it provides. The mutations that hamper expression would then be favoured, resulting in the gradual loss of the pathway and its core genes. Here the loss of MHC II pathway is independent of any other potential modifications to the immune system (e.g. expansion of innate immune receptors). On the contrary, the functional shift hypothesis suggests that certain environments could favour the development of alternative adaptive or innate immune pathways that make the MHC II pathway redundant. Selection on the pathway would then be relaxed and through genetic drift, it would slowly degenerate. In a functional shift hypothesis, the emergence of these alternative immune pathways would predate or occur concurrently with the loss of MHC II. Thus, if the hypothesis is supported, in the species that lost MHC II pathway we can expect to observe an alternative immune gene profile (e.g. via MHC I cross-presentation). It is important to note that these hypotheses are not mutually exclusive and could act on the immune system simultaneously (Star and Jentoft, 2012).
Since then evidence supporting the metabolic cost hypothesis was obtained in cod-like fishes demonstrating that the loss of MHC II predated expansions of MHC I genes, hinting that the latter might be a compensatory response rather than a competing alternative immune strategy (Malmstrøm et al., 2016). The Atlantic cod also shows a distinct Toll-like receptor (TLR) profile. TLRs represent a large family of Pattern Recognition Receptors (PRRs) and are one of the key components of the innate system (Brubaker et al., 2015). The expansion of tlr7, 8, 9, 22 and 25, and contractions of tlr2 and 5 in cod is hypothesized to be a consequence of the MHC II loss and greater reliance on the innate immune system (Solbakken et al., 2016).
In syngnathids, only one genome study has mentioned the TLR repertoire and related pathways (He et al., 2021). The researchers reported species-specific contractions of TLR 2, 4 and 5 cascades in Hippocampus abdominalis using Gene ontology (GO) analysis approach. The expanded genes that belong to the aforementioned GO terms are mapk14a, mapk3, s100b, tab2, ikbkg, peli1b, irak1 and dusp3a (He et al., 2021). While these genes do indeed belong to the TLR signalling pathways, the results have to be treated with caution as they represent only a small fraction of the GO term size (~50 genes) and at least some of these genes also belong to other pathways that are unrelated to the immune system. Strangely enough, the TLR10 cascade term is not mentioned in the manuscript, but it also appears to be enriched because of the same eight genes. However, the TLR1, TLR2, and TLR9 related genes that appear to be contracted within the whole Syngnathidae lineage were only referred to in the supplementary materials. Tlr18 is reported to be expanded in the ancestor of Syngnathidae, yet no additional information is provided (i.e. copy number information) (He et al., 2021). In turn, investigating the diversity of TLRs in syngnathids is crucial as sub/neofunctionalization of the expanded TLR lineages could serve as an alternative to the lost MHC II pathway.
A number of genome studies have used similar approaches, casting a wide net and looking at enriched GO terms or expanded protein families, rather than at selected individual genes or groups of genes (Small et al., 2016; Zhang et al., 2020; Qu et al., 2021; Small et al., 2022). Such an approach helps to look at a broader picture of syngnathid genome evolution, but cannot substitute a more detailed investigation of immune system components. In order to trace the immune system evolution alongside the male pregnancy gradient a thorough genome scan of all sequenced syngnathids is needed. The genes encoding innate and adaptive immune system receptors, co-receptors, accessory, regulatory and signalling molecules should be evaluated for presence-absence and copy number variations. So far, only a fraction of immune pathways have been evaluated.
In the genomes of the leafy seadragon (Phycodurus eques) and “weedy” seadragon (Phyllopteryx taeniolatus) seven gene families related to innate immunity experienced contractions, among them contractions in tripartite motif-containing (TRIM), immune-associated nucleotide-binding (IAN), and mannose receptor (MRC) gene families were identified, confirming previous reports in syngnathids (Small et al., 2022). The TRIM family of proteins are involved in many cellular processes, including within the immune system. Expansions of certain genes encoding for TRIM sub-families have been described in teleosts and shown to have strong antiviral activities (Ozato et al., 2008; Van Der Aa et al., 2009; Langevin et al., 2017). Mannose receptor family (C-type lectin superfamily) is a multifunctional protein family with roles within adaptive and innate immune systems (Vasta et al., 2011). Interestingly, a Manado pipefish (Microphis manadensis) genome study also reports contractions within nine C-type lectin-domain containing families (Zhang et al., 2020). The IAN/GTPase of the immunity-associated protein (IAN/GIMAP) family genes were shown to be upregulated in zebrafish during viral infections (Balla et al., 2020). GIMAP and GIMAP-like genes have also been identified in invertebrates and hypothesized to have immune functions (Weiss et al., 2013; Milan et al., 2018; Lu et al., 2020; Limoges et al., 2021). The gimap4 gene was shown to be upregulated during pouch development and late pregnancy in S. typhle, which is suggested to suppress lymphocyte maturation and proliferation protecting the eggs (Roth et al., 2020). Despite the overall IAN/GIMAP family contraction, gimap4 seems to remain intact throughout the Syngnathidae lineage (Small et al., 2022). In addition to the aforementioned C-type lectin family contraction, contractions of NACHT-domain and immunoglobulin-domain containing families in M. manadensis have been reported (Zhang et al., 2020).
Alternative immune strategies could develop not only via expansions/contractions of certain receptor molecules, but also via modifications of regulatory pathways. Interleukins are a group of short protein cytokines that represent promising candidates for such investigation, owing to their important involvement in innate and adaptive immune system regulation (Secombes et al., 2011).
3 Male pregnancy
3.1 Immunological tolerance
The evolution of the unique male pregnancy can only be attributed to the syngnathid fish group, of which there are a number of varying brooding strategies and physiological adaptations, some of which are similar to female amniotes (Stölting and Wilson, 2007; Whittington and Friesen, 2020). Syngnathid brooding forms range from simplified external egg-attaching integument tissue (Nerophinae), egg-retaining inverted skin flap extensions, to the advanced fully enclosed marsupium-like brood pouches with placenta-like structures (Wilson et al., 2001; Carcupino et al., 2002; Ripley et al., 2010). These brooding differences between closely related species allow for the examination of evolutionary change and the potential drivers or crucial adaptations that culminate in advanced forms of pregnancy. In turn, pregnancy evolution is heavily linked with the evolution of the adaptive immune system, and therefore syngnathids provide scope to understanding immune system evolution and its relevance within the realm of pregnancy. Currently, it is generally accepted that the expansion of a number of vertebrate systems accommodating organism physical growth, likely coincided with adaptive immune system evolution (Kasahara, 2000).
The evolution of gestation requires specialized morphological and immune gene expression changes (Moffett-King, 2002; Zenclussen et al., 2006; Hedlund et al., 2009). However, the co-evolution of gestation and the immune system creates a dilemma, regarding the avoidance of embryonic rejection via immune modulation and still maintaining maternal immune vigilance (La Rocca et al., 2014). In mammals, these problems have been solved through gene expression changes during pregnancy and at its onset, specific immune cell activities and specialized uterine/placental tissues (Moffett and Loke, 2006; Hedlund et al., 2009). In a general sense, immunological function in syngnathids is found to be disparate between pregnant and non-gravid individuals (Small et al., 2016; Roth et al., 2020; Parker et al., 2022). Similar suppression of the adaptive immune system has been noted in syngnathid pregnancy. This is through the diversity downregulation of MHC I genes and the functional (Hippocampus spp.) and complete genomic loss of MHC II (Syngnathus spp.), which appears a striking potential solution to immune regulation in pouched syngnathids when compared with the less drastic gene downregulation (Roth et al., 2020; Parker et al., 2022). MHC I pathway related downregulation was found to occur specifically during early gestation in syngnathids with a defined brood pouch, contrary to pouchless species (Parker et al., 2022). Immunological activity differences between brooding strategies have also been observed in S. typhle (inverted brood pouch), which exhibited a greater immune investment during pregnancy compared with Nerophis ophidion (pouchless) (Keller and Roth, 2020). These reports suggest that the evolution of the more ‘intimate’ brooding strategies required the coevolution of immune suppressive measures to accommodate the progeny.
Following mammalian coitus, sperm containing seminal fluid enters the female reproductive tract (Poiani, 2006). Seminal plasma is enriched with signalling molecules that have been shown to influence successful pregnancy establishment and implantation (Robertson et al., 2011; Robertson et al., 2013). It is also posited to act as an immunological tolerance primer for the receiving female to avoid embryo rejection (Tremellen and Robertson, 1999; Robertson et al., 2018). Mucus-like fluid has been reported to surround deposited syngnathid eggs (Carcupino et al., 1997; Watanabe, 1999), however, its significance or function is unknown. Whittington and Friesen (2020) have posited that the fluid could be a female equivalent to seminal fluid that potentially influences the onset and immunological homeostasis of male pregnancy. This is a deeply interesting concept that should be explored in more depth in the future.
3.2 Brood pouch defences
Unlike mammals, pouched syngnathids have had to overcome another immunological quandary when it comes to internal gestation relating to the inner pouches and progeny exposure to environmental water and pathogens (Fiedler, 1954; Whittington and Friesen, 2020; Parker et al., 2022). Balancing the activity of immune system defences and concurrent immunological tolerance measures is a challenging proposition and is yet to be fully understood. Immunological function during male pregnancy is found to be disparate to non-gravid individuals (Small et al., 2016; Roth et al., 2020; Parker et al., 2022), with immunological factor concentrations at their greatest during pregnancy in seahorses (Lin et al., 2017). Bacterial activity and growth is thought to be facilitated in the sealed brood pouch particularly during the later gestation stages (Whittington and Friesen, 2020) and are likely a key driver of these immune disparities between pregnancy statuses. Uterine flushing in some gestating shark species is believed to assist with gas exchange and waste disposal around the time of parturition (Burger, 1967; Evans et al., 1982; Tomita et al., 2016; Tomita et al., 2017). A consequential upregulation of immune processes is also suggested to counter the influx of harmful pathogens (Sunyem and Vooren, 1997; Ellis and Otway, 2011; Buddle et al., 2020). Related upregulated expression of immune genes during parturition have also been described in seahorses with studies advocating the occurrence of similar brood pouch flushing (Whittington et al., 2015; Parker et al., 2022). Brood pouch flushing and its role in immune defence is an intriguing concept that will require further experimental studies.
A number of specific immunological defence components within syngnathid brooding structures have been proposed. Transcriptomic evidence of interleukin release (Whittington et al., 2015; Jiang et al., 2022), TLR gene expression (Whittington et al., 2015; Zhang et al., 2019; Wu et al., 2021) and C-type lectin activity (Melamed et al., 2005; Small et al., 2013; Whittington et al., 2015) in syngnathid brood pouches pertain to anti-bacterial function. The co-option of the antifungal hepcidin genes in seahorses is believed to have dampened their antimicrobial potential in the brood pouch to assist with immune homeostasis, but could still play a minor defence role against pouch dwelling pathogens (Whittington et al., 2015; Xiao et al., 2022). Bulk RNA-seq studies have reported the upregulated expression of genes coding for phospholipase sPLA2-IB and the macroglobulin A2M in the brood pouch during pregnancy in Hippocampus species (Wu et al., 2021; Parker et al., 2022; Xiao et al., 2022). Both are suggested to provide antimicrobial assistance, however, their presence and specific function within the brood pouch would benefit from further functional experimentation. Retinoic acid has a number of physiological functions, many of which revolve around immune system regulation (Larange and Cheroutre, 2016). In turn, retinoic acid concentration stability was suggested to be important for avoiding oxidative stress during male pregnancy (Li et al., 2020). Prolactin has been shown to influence growth, skin secretion regulation and immunological function in teleost fishes (Páll et al., 2004; Richards et al., 2009). It has also been isolated in the seahorse brood pouch during pregnancy and is thought to contribute to pouch osmoregulation; however, its potential immunological role is yet to be properly defined (Boisseau, 1967; Patron et al., 2008; Scobell and MacKenzie, 2011; Whittington and Wilson, 2013; Clarke and Bern, 2012). Upregulated seahorse pouch-derived genes with implicated immune roles are regularly identified, however, a clear understanding of the functional relationships connecting most of the aforementioned components is still lacking. In time, condensing molecular and gene expression findings into a comprehensible network should help discern pregnancy immune modulation processes from pathogen protective measures.
3.3 Inflammation
The inflammatory function of the uterine tissues during early mammalian pregnancy is associated with tissue swelling which supports egg implantation (Mor and Abrahams, 2002; Dekel et al., 2010; Chavan et al., 2017). The fleshiness of mature male syngnathid brood pouches has been observed, in preparation for the deposition of eggs (Harlin-Cognato et al., 2006; Whittington and Friesen, 2020; Parker et al., 2022). These visual observations were recently corroborated by the upregulation of inflammation-related genes during the early stages of gestation in syngnathids of external, inverted brooding and advanced brooding forms (Parker et al., 2022), and during pouch development (Roth et al., 2020). Inflammatory pathway induction stimulates immune cell recruitment, which consequently causes cytokine release leading to tissue reshaping/remodelling (Granot et al., 2012). Therefore, it is conceivable that the extension of tissue folds in the syngnathid pouch is influenced by inflammatory pathways, and in turn aids egg immersion in pouched syngnathids. The molecular triggers for this inflammation are unknown; however, it could in part be induced by a seminal-like substance coating the deposited eggs. Seminal fluid is known to trigger inflammation upon entering the female reproductive tract in mammals (Robertson, 2005). A similar function may be found in syngnathids with female egg-coating fluid serving as the stimulant, however, this would require extensive work to clarify. Inflammation exhibited in the integument tissue of pouchless syngnathid species such as N. ophidion is suggested to be representative of the evolutionary root of this form of egg retention assistance (Parker et al., 2022). Similarities drawn with reproductive strategies in ricefishes (Hilgers et al., 2022), support the idea of inflammation assisting with the instigation of evolutionary modification and tissue specialisation. A number of brooding strategies have evolved within the lineage that occupy morphological gaps between the inverted dual skin-flapped pouch of S. typhle and the pouchless N. ophidion. For example, Stigmatopora pipefish species have pouch extensions akin to S. typhle, but without complete egg envelopment, while Doryrhamphus dactyliophorus have evolved thinner membranous egg capsules to retain the growing embryos (Wilson et al., 2001). Exploring the expression profiles of such phylogenetic representatives could provide a clearer understanding of the influence of inflammatory processes on the evolution of brood retention.
4 Sex specificity
Across a number of species, distinct differences have been documented concerning the immune capabilities of the respective sexes, with males generally possessing a less efficient immune system compared with females (Hamilton, 1948; Møller et al., 1998; Kurtz et al., 2000; Falagas et al., 2007; Roth et al., 2008; Abdullah et al., 2012). In humans, this evolutionary disparity is in part associated with female pregnancy, with an increase in immune potential linked to the parent providing the highest degree of investment (Rolff, 2002). Therefore, the strength of parental immunity appears to depend on the life-history strategy; however, within the syngnathid lineage changes in sex roles and the degrees of parental investment vary depending on the species, rendering the immunological activity and concept of sex-role reversal difficult to disentangle. Despite this, in some cases of induced parental care, sex role reversal in syngnathids appears to have potentially led to distinct sexual immune dimorphism, with males adopting the role with greatest immunological and parental responsibility, while females are tasked with attracting mates. Studies on Hippocampus comes and S. typhle support this difference, with paternal immune response efficiencies appearing greater than in females (Roth et al., 2011; Lin et al., 2016a). These reports also suggest that competition for mates reduced immunity and that the adoption of parental care during pregnancy likely has a positive effect on the parent’s immunity. Experimental exposure to water contaminants further support this sex distinction with immunocompetence in males greatly exceeding that of females (Jiang et al., 2019).
Hormones represent a complicated but compelling set of factors charged with mediating many important steps in mammalian pregnancy. Hormonal dynamics are intrinsically different between males and females in humans, and endocrine processes dictate immune shifts in females during pregnancy (Robinson and Klein, 2012). A number of endocrine-related studies in syngnathids have been conducted to date, highlighting their importance in syngnathid pregnancy, parturition and pouch development (Boisseau, 1967; Mayer et al., 1993; Ripley and Foran, 2010; Scobell and MacKenzie, 2011; Whittington and Wilson, 2013; Paul et al., 2020; Dudley et al., 2021). Others have identified sexual dimorphism in the pipefish liver, suggesting that estrogen in pipefish regulates reproductive physiology similarly to fish without reversed sex roles (Rose et al., 2015). However, the significance of sex specific hormonal activity in the realm of syngnathid male pregnancy, sex role reversal and specifically immune function still remains relatively unknown, but is an interesting proposition for future investigation.
5 Immune priming
5.1 Transgenerational immune priming
Transgenerational immune priming (TGIP) describes the transfer of parentally derived immunological experience to the progeny (Grindstaff et al., 2003). TGIP has a crucial influence on offspring survival and via the maternal line is a phenomenon well reported across the animal kingdom (Roth et al., 2018). The unique male pregnancy provided the mechanistic opportunity for a transfer of maternal experience via the egg in combination with a transfer of paternal experiences provided via the paternal brood pouch during male pregnancy (Roth et al., 2012; Beemelmanns and Roth, 2016a). The parental investment dynamics are found to be asymmetric with maternal immune benefits only persisting during the early life stages, compared with the paternal immune influence which was suggested to be long-lasting (Roth et al., 2012; Beemelmanns and Roth, 2016a). In addition, maternal priming is suggested to benefit the offspring’s adaptive immune system, while the paternal influence rather influences the innate branch (Roth et al., 2012; Beemelmanns and Roth, 2016a). This is in contrast to the investment dynamics of conventional mammalian sex roles and is one of the very few instances of paternally derived TGIP in the animal kingdom. Sex-specific grandparental immune priming has also been determined in concert with the male pregnancy system, with F2 offspring benefitting from the immunological experiences of the grandparents (Beemelmanns and Roth, 2017). Both these reports further support the influence of TGIP on the co-evolutionary arms race between pathogens and their hosts and that sex-role reversal still maintains the typical immune priming customary to mammals where the female primarily supports the offspring. Under changing environmental conditions, when parents are exposed to an additional environmental stressor (i.e., a temperature shift), the transfer of immunity from parents to offspring is hampered, implying that trans-generational plasticity reaches its limits when multiple stressors occur during the parental generation and offspring environments become unpredictable (Roth and Landis, 2017). TGIP might be influenced or partly maintained by a specific community of maternal and paternal microbes (Beemelmanns et al., 2019).
5.2 Brood pouch microbiota
Host-associated microbiota are integral for a number of physiological processes, including nutritional uptake, development and immunity; colonizing vulnerable regions such as the skin and gut in many vertebrates (Robinson et al., 2010; Hooper et al., 2012; Hacquard et al., 2015). Recently there has been a surge in research relating to the composition, evolutionary characteristics and function of the syngnathid brood pouch microbiota (Beemelmanns and Roth, 2016b; Beemelmanns et al., 2019; Wagner, 2019). It has been observed that upon immune system activation in pregnant males, there is an upward turn in microbial community richness (Beemelmanns et al., 2019). This is proposed to coincide with larval mouth opening and consequent microbial colonisation of the progeny. The establishment of a cohesive, functional microbiome is widely recognized as a crucial player in immune system development and efficiency (Gómez and Balcázar, 2008; Belkaid and Hand, 2014). Pouch microbial community changes during pregnancy, environmental influences, as well as diversity differences between pouch types and species are all topics that would benefit from further investigation. These along with future functional experimentation should improve the understanding of the functional relationships that exist between microbes, male pregnancy and immunity.
6 Immune cells
6.1 Syngnathid immune organs
The major immune organ and the first to develop in syngnathids is the head kidney (Tort et al., 2003; Falk-Petersen, 2005). Splenic presence and functionality among syngnathids is largely unsubstantiated with no spleen identifications in pipefish, while in seahorses its presence is contentious, with a number of contrasting reports (Matsunaga and Rahman, 1998; Novelli et al., 2015; Luo et al., 2016; Ofelio et al., 2018; Wijerathna et al., 2022). In seahorses, developmental studies report the presence of a spleen during early juvenile development (Novelli et al., 2015; Ofelio et al., 2018), but adult spleens are seldom reported. This could be an indication of splenic shrinkage during adulthood, which in turn could render them functional redundant, however, this speculation would require further experimental clarification. The gut-associated lymphoid tissue (GALT) is a mucosal region located in the intestines, commonly found in humans and other animals, performing an important immunological role in the gut maintaining and developing immune cells in preparation for a response (Haley, 2017). In seahorses and pipefish, the GALT has been deduced missing or at the very least reduced to a vestigial level, with immune cells primarily stemming from the main head kidney (Matsunaga and Rahman, 1998; Roth et al., 2020). This loss was proposed to be an ancestral change in predatory activity, reducing the need for gut related immunological reserves (Matsunaga and Rahman, 1998). As with other teleost species, syngnathids likely possess gill-associated lymphoid tissue (GIALT) that offers mucus derived immunological protection from external pathogens encountered during oxygen uptake (Salinas, 2015). This is a clear sign of immunological activity in the tissue (Roth et al., 2012; Birrer et al., 2012; Luo et al., 2016). Nasopharynx-associated lymphoid tissue (NALT) and skin-associated lymphoid tissue (SALT) are equally important mucosal immune hubs, serving to protect the skin and olfactory organs, respectively (Salinas, 2015). Interestingly, research pertaining to the presence of SALT and NALT in syngnathids is yet to materialise but should be encouraged as it could hold the answers for many immune related knowledge gaps across the lineage.
6.2 Immune cell populations
The identification of immune cell types in teleost fishes in recent years has been assisted by the development of efficient cell sorting machinery and the advent of single-cell RNA sequencing methodologies (Islam et al., 2014; Chen et al., 2019). These methods are at the forefront of transcriptome research, providing a high-resolution investigative assessment of cell types that transcends traditional bulk-RNA sequencing techniques. Among the fish species that have so far undergone immune cell characterizations or specific cell isolations are zebrafish (Danio rerio) (Dee et al., 2016; Athanasiadis et al., 2017; Carmona et al., 2017; Tang et al., 2017; Hernández et al., 2018; Ferrero et al., 2020; Loes et al., 2021), Atlantic cod (Gadus morhua) (Guslund et al., 2020; Guslund et al., 2022), Atlantic salmon (Salmo salar) (Smith et al., 2021), Nile tilapia (Oreochromis niloticus) (Niu et al., 2020; Wu et al., 2021), Mexican tetra (Astyanax mexicanus) (Peuß et al., 2020) and rainbow trout (Oncorhynchus mykiss) (Perdiguero et al., 2021). Recently, the first syngnathid immune cell repertoire was characterized in S. typhle providing a crucial baseline for future immune cell studies within the lineage (Parker et al., 2022). This report described the presence of a number of key immune cell types and their associated gene identifiers including macrophages (mrc1, mpeg1), neutrophils (cebpe, lce, ncf4), B- (iglc1, cd53, cd79b) and T-cell lymphocytes (cd2, cd3e, v-tcr). Interestingly, no signs of CD4+ T-cell types were observed, which is in line with the loss of MHC II in the species (Haase et al., 2013; Roth et al., 2020). However, perhaps the most interesting discoveries concerned two genes within the T-cell cluster, ilr2rb and gzma, suggesting the potential presence of regulatory T-cells (Tregs) and cytotoxic T-lymphocytes (CTLs), respectively. Immunosuppressive Treg populations and their function remain elusive in syngnathids but if their identification can be confirmed, it would be a fascinating addition to the immunological tolerance discussion surrounding the lineage. The identification of CTLs in this study, along with their prominence in another recent study in the same species, suggest that they may be at the forefront of the syngnathid immune response (Parker and Roth, 2022). Elevated CTL activity in the MHC II/CD4+ cell devoid S. typhle could also hint at a potential compensatory measure that has evolved, however, these deductions likely require further substantiation.
Transcriptome assessments of the leafy appendages possessed by the seadragon, P. taeniolatus, uncovered inflammation-related gene expression, suggesting a potential immunological role (Qu et al., 2021). The defensive addition of highly upregulated MHC I gene expression in the leafy extensions supports the theory that due to the appendages being crucial to seadragon camouflage and therefore survival, there is added importance in its protection and regeneration.
7 Husbandry pathology
The demand for syngnathid species, in particular seahorses, has increased drastically over the last decade as they have become a prominent feature in traditional Chinese medicine and ornamental fish collections (Vincent, 1996). These practices rely on prolific husbandry set-ups, which in turn increase the demand for information on efficient aquaculture rearing methods (Koldewey and Martin-Smith, 2010). Syngnathid fish husbandry is associated with elevated infection risks due to excessive fish handling, lower water quality and higher stock densities than those found in the natural environment (Prosser et al., 2011). Even factors such as ambient aquaria noise are believed to induce stress and impact immunological efficiency in syngnathids (Anderson et al., 2011). Syngnathid fishes raised in aquaria systems are subjected to numerous pathogenic challenges which are often exacerbated due to imperfections in husbandry rearing conditions (Sanaye et al., 2013). Here is a brief overview of some of the more common pathogens encountered by syngnathids within the aquaria trade, and their related diseases. A more comprehensive list of harmful pathogens and health issues is also included (Table 1).
Mycobacteria related infections can lead to mycobacteriosis, a disease that reared seahorses are particularly susceptible to (Koldewey, 2005). Infection has been shown to stimulate atypical lesions on a number of organs and body parts such as the tail, spleen, liver and kidney in seahorse cultures (Bombardini et al., 2006; Balcázar et al., 2011; Fogelson et al., 2017; Fogelson et al., 2018), while infections in seadragon and pipefish species have also been cited (Bombardini et al., 2006; Bonar et al., 2013).
A number of flavobacteria strains such as Tenacibaculum spp., Cellulophaga fucicola and Flavobacterium columnare have all been isolated previously from pipefish, seahorse and seadragon aquaria stocks (Bombardini et al., 2006; LePage, 2012; Declercq et al., 2014; LePage et al., 2015). Targeted tissues can vary greatly among fish species, with these syngnathid studies highlighting signs of necrosis in the gills, tail, skin and muscle.
Vibrio strains are the most abundant, diverse opportunistic marine pathogens and regularly used experimentally to assess syngnathid fish immunity (Thompson et al., 2004; Birrer et al., 2012; Landis et al., 2012; Roth et al., 2012; Goehlich et al., 2021). When conditions suit, they are a common cause of disease in aquaria raised syngnathids (Alcaide et al., 2001; Balcázar et al., 2010; Balcázar et al., 2010; Wang et al., 2016). Symptoms can include lethargy, skin spots, loss of appetite and tail necrosis (Balcázar et al., 2010). Vibrio are also implicated in opportunistic secondary infections, with recent findings suggesting that gas bubble disease (GBD) associated with syngnathid husbandry (Lin et al., 2010; LePage et al., 2015; Zhang et al., 2015), renders fish susceptible to vibrionic invasion (Kang et al., 2022). Moreover, juvenile seahorses are particularly susceptible to Vibrio strains, which can lead to a number of physiological and developmental issues (Lin et al., 2016b; Shao et al., 2019).
Scuticociliates are a group of parasitic unicellular marine organisms and renowned causative agents of scuticociliatosis, another common disease among aquaria fish species such as seadragons, seahorses and pipefishes (Marcer et al., 2005; Garner et al., 2008; Rossteuscher et al., 2008; Sang et al., 2011; Bonar et al., 2013; Di Cicco et al., 2013; Ofelio et al., 2014; Armwood et al., 2021). Often scuticociliatosis leads to severe skin lesions and necrosis, while internal organs, blood vessels and gills are also regularly affected (Cheung et al., 1980; Woo and Buchmann, 2012; Bonar et al., 2013; Ofelio et al., 2014).
Monogenean flatworms are another common parasite often reported among syngnathids including many from the Syngnathus pipefish group (Bombardini et al., 2006; Williams et al., 2008; Vaughan et al., 2010; Paladini et al., 2010). They have been shown to parasitize a number for anatomical regions including the brood pouch, skin and gills (Williams et al., 2008; Paladini et al., 2010; Cone et al., 2013). Myxospora are microscopic parasites from the cnidaria phylum that commonly infiltrate seahorses (Vincent and Clifton-Hadley, 1989; Sears et al., 2011), seadragons (Garner et al., 2008; Bonar et al., 2013) and pipefish (Longshaw et al., 2004). The parasites often reside in the gall bladder of the infected individuals (Vincent and Clifton-Hadley, 1989; Longshaw et al., 2004; Bombardini et al., 2006).
A number of fungal pathogens including microsporidia, primarily of the genus Glugea, and melanized fungi, have been isolated from syngnathid fishes (Bombardini et al., 2006; Nyaoke et al., 2009). Glugea strains are spore-forming organisms capable of transforming infected fish cells into proliferating masses known as xenomas a key symptom of microsporidiosis (Lom and Dyková, 2005; Dyková and Lom, 2007). In Hippocampus erectus, Glugea had a particular tendency to corrupt skin and connective tissues (Blasiola, 1979; Vincent and Clifton-Hadley, 1989; Bombardini et al., 2006). Phaeohyphomycosis stems from the infection of opportunistic melanized fungi such as those from the Exaphiala genus, and has been documented a number of times, particularly in weedy and leafy seadragons where they had a tendency to infect vascular tissues (Nyaoke et al., 2009; Bonar et al., 2013).
Viral pathogens and their aquacultural impacts are under-researched in syngnathids, with very little information documented on the topic. Suspected viral induced lesions have been identified in H. abdominalis previously without conclusively identifying the specific culprit (LePage et al., 2015), while a more recent paper has isolated and characterized a new strain of virus called the seahorse nervous necrosis virus (SHNNV) (Chen et al., 2022). Extracted from the brain and eye, SHNNV is deduced to cause harmful vacuolations in the organs and based on infection experiments is more virulent among juveniles. In H. abdominalis, viperin, an antiviral related gene was identified and upregulated in intestinal and kidney tissues when exposed to infection, suggesting it could have a role in modulating syngnathid antiviral responses (Tharuka et al., 2019). These limited findings strengthen the need for further research focused on elucidating the pathology, diversity and general relevance of viruses in the syngnathid aquaculture trade.
8 Conclusion
Syngnathids are some of the most fascinating subjects for evolutionary and immunological research due to their unique male pregnancy and intriguing immunological rearrangements. Molecular based studies highlighted here provide an ideal platform for future experimental work, which should focus on understanding the functional properties and mechanisms at play and how they relate to syngnathid evolution and physiology. By sharing a recent common ancestor and exhibiting diverse brooding strategies, syngnathids are useful candidates for comparative work and interpreting the nuances of evolutionary adaptation. Understanding the intricate inner workings of syngnathid immune function, immunological tolerance and pregnancy, should provide a useful alternative perspective to model species research and could prove vital for the development of applied autoimmune and other medical related practices.
Author contributions
JP, AD and OR contributed to the conception of the manuscript. JP wrote the first draft of the manuscript, JP, AD and OR wrote sections of the manuscript. All authors contributed to the manuscript review, and read and approved the final submitted version.
Funding
Funding support was provided by the German Research Foundation (RO-4628/4-2) and the European Research Council (ERC) the European Union´s Horizon research and innovation program (MALEPREG: eu-repo/grantAgreement/EC/H2020/755659) to OR.
Acknowledgments
Thanks are given to all members of the Marine Evolutionary Biology group at Kiel University for their scientific advice and discussions.
Conflict of interest
The authors declare that the research was conducted in the absence of any commercial or financial relationships that could be construed as a potential conflict of interest.
Publisher’s note
All claims expressed in this article are solely those of the authors and do not necessarily represent those of their affiliated organizations, or those of the publisher, the editors and the reviewers. Any product that may be evaluated in this article, or claim that may be made by its manufacturer, is not guaranteed or endorsed by the publisher.
References
Abdullah M., Chai P.-S., Chong M.-Y., Tohit E. R. M., Ramasamy R., Pei C. P., et al. (2012). Gender effect on in vitro lymphocyte subset levels of healthy individuals. Cell Immunol. 272 (2), 214–219. doi: 10.1016/j.cellimm.2011.10.009
Alcaide E., Gil-Sanz C., Sanjuan E., Esteve D., Amaro C., Silveira L. (2001). Vibrio harveyi causes disease in seahorse, hippocampus sp. J. Fish Dis. 24 (5), 311–313. doi: 10.1046/j.1365-2761.2001.00297.x
Amemiya C. T., Alföldi J., Lee A. P., Fan S., Philippe H., MacCallum I., et al. (2013). The African coelacanth genome provides insights into tetrapod evolution. Nature 496 (7445), 311–316. doi: 10.1038/nature12027
Anderson P. A., Berzins I. K., Fogarty F., Hamlin H. J., Guillette L. J. Jr. (2011). Sound, stress, and seahorses: the consequences of a noisy environment to animal health. Aquaculture. 311 (1-4), 129–138. doi: 10.1016/j.aquaculture.2010.11.013
Anderson P. A., Petty B. D. (2013). Mixed metazoan and bacterial infection of the gas bladder of the lined seahorse–a case report. J. Aquat Anim. Health 25 (1), 42–52. doi: 10.1080/08997659.2012.743932
Appleby C. (1996). Gyrodactylus syngnathi n. sp.(Monogenea: gyrodactylidae) from the pipefish syngnathus rostellatus Nilsson, 1855 (Syngnathiformes: Syngnathidae) from the Oslo fjord, Norway. Syst. Parasitol. 33 (2), 131–134. doi: 10.14411/fp.2008.034
Armwood A. R., Cañete-Gibas C. F., Dill-Okubo J. A., Wiederhold N. P., Camus A. C. (2021). Retrospective study of phaeohyphomycosis in aquarium-housed fish, with first descriptions of exophiala lecanii-corni and neodevriesia cladophorae in fish. J. Fish Dis. 44 (10), 1563–1577. doi: 10.1111/jfd.13477
Athanasiadis E. I., Botthof J. G., Andres H., Ferreira L., Lio P., Cvejic A. (2017). Single-cell RNA-sequencing uncovers transcriptional states and fate decisions in haematopoiesis. Nat. Commun. 8 (1), 1–11. doi: 10.1038/s41467-017-02305-6
Bahr A., Wilson A. (2011). The impact of sex-role reversal on the diversity of the major histocompatibility complex: insights from the seahorse (Hippocampus abdominalis). BMC Evol. Biol. 11 (1), 121. doi: 10.1186/1471-2148-11-121
Balcázar J. L., Gallo-Bueno A., Planas M., Pintado J. (2010). Isolation of vibrio alginolyticus and vibrio splendidus from captive-bred seahorses with disease symptoms. Anton Leeuw Int. J. G 97 (2), 207–210. doi: 10.1007/s10482-009-9398-4
Balcázar J. L., Lee N. M., Pintado J., Planas M. (2010). Phylogenetic characterization and in situ detection of bacterial communities associated with seahorses (Hippocampus guttulatus) in captivity. Syst. Appl. Microbiol. 33 (2), 71–77. doi: 10.1016/j.syapm.2009.11.005
Balcázar J. L., Planas M., Pintado J. (2011). Novel mycobacterium species in seahorses with tail rot. Emerg. Infect. Dis. 17 (9), 1770. doi: 10.3201/eid1709.101289
Balla K. M., Rice M. C., Gagnon J. A., Elde N. C. (2020). Linking virus discovery to immune responses visualized during zebrafish infections. Curr. Biol. 30 (11), 2092–2103. doi: 10.1016/j.cub.2020.04.031
Beemelmanns A., Poirier M., Bayer T., Kuenzel S., Roth O. (2019). Microbial embryonal colonization during pipefish male pregnancy. Sci. Rep. 9 (1), 1–14. doi: 10.1038/s41598-018-37026-3
Beemelmanns A., Roth O. (2016a). Biparental immune priming in the pipefish syngnathus typhle. Zoology. 119 (4), 262–272. doi: 10.1016/j.zool.2016.06.002
Beemelmanns A., Roth O. (2016b). Bacteria-type-specific biparental immune priming in the pipefish syngnathus typhle. Ecol. Evol. 6 (18), 6735–6757. doi: 10.1002/ece3.2391
Beemelmanns A., Roth O. (2017). Grandparental immune priming in the pipefish syngnathus typhle. BMC Evol. Biol. 17 (1), 1–15. doi: 10.1186/s12862-017-0885-3
Belkaid Y., Hand T. W. (2014). Role of the microbiota in immunity and inflammation. Cell. 157 (1), 121–141. doi: 10.1016/j.cell.2014.03.011
Bellomy M. (1969). Encyclopaedia of seahorses (Reigate, Surrey, England: Tropical Fish Hobbyist Publications).
Binh D. T., Quyen V., Sang T. Q., Oanh T. T. (2016). Vibriosis in cultured seahorse (Hippocampus spp.) in khanh hoa province, Vietnam. Int. J. Innov. Stud. Aquat Biol. Fish 2, 43–50. doi: 10.20431/2455-7670.0202005
Birrer S. C., Reusch T. B., Roth O. (2012). Salinity change impairs pipefish immune defence. Fish Shellfish Immunol. 33 (6), 1238–1248. doi: 10.1016/j.fsi.2012.08.028
Blasiola G. (1979). Glugea heraldi n. sp.(Microsporida, glugeidae) from the seahorse hippocampus erectus perry. J. Fish Dis. 2 (6), 493–500. doi: 10.1111/j.1365-2761.1979.tb00410.x
Blasiola G. (1983). A review of brooklynella parasitization in marine fishes: diagnosis, pathology and chemotherapy. Proc. Int. Assoc. Aquat. Med. 12, 1.
Blazer V. S., Wolke R. E. (1979). An exophiala-like fungus as the cause of a systemic mycosis of marine fish. J. Fish Dis. 2 (2), 145–152. doi: 10.1111/j.1365-2761.1979.tb00151.x
Boisseau J. (1967). Recherche sur le controle hormonal de l’incubation chez l’hippocampe. Rev. Européen d'Endocrinologie 4 (3), 197–234.
Bombardini C., Florio D., Fichtel L., Fioravanti M. (2006). The main disease of syngnathidae in captivity. Ittiopatologia. 3 (3), 205–211.
Bonar C., Garner M., Weber I. I. I. E., Keller C., Murray M., Adams L., et al. (2013). Pathologic findings in weedy (Phyllopteryx taeniolatus) and leafy (Phycodurus eques) seadragons. Vet. Pathol. 50 (3), 368–376. doi: 10.1177/0300985813482337
Bonilla F. A., Oettgen H. C. (2010). Adaptive immunity. J. Allergy Clin. Immunol. 125 (2), S33–S40. doi: 10.1016/j.jaci.2009.09.017
Boylan S., Camus A., Waltzek T., Yarbrough L., Miller S., Howard S. (2015). Liquid nitrogen cryotherapy for fibromas in tarpon, megalops atlanticus, valenciennes 1847, and neoplasia in lined sea horse, hippocampus erectus, perry 1810. J. Fish Dis. 38 (7), 681–685. doi: 10.1111/jfd.12276
Braicovich P. E., González R. A., Tanzola R. D. (2005). First record of corynosoma australe (Acanthocephala, polymorphidae) parasitizing seahorse, hippocampus sp.(Pisces, syngnathidae) in Patagonia (Argentina). Acta Parasitologica 50 (2), 145–149.
Brown T., Millar Z., Evans D., Pham P. H., LePage V., Lumsden J. S. (2020). Fusarium solani haplotype 12-b and aortic and branchial arteritis in hippocampus erectus perry. J. Fish Dis. 43 (2), 301–304. doi: 10.1111/jfd.13099
Brubaker S. W., Bonham K. S., Zanoni I., Kagan J. C. (2015). Innate immune pattern recognition: a cell biological perspective. Annu. Rev. Immunol. 33, 257. doi: 10.1146/annurev-immunol-032414-112240
Buddle A. L., Otway N. M., Van Dyke J. U., Thompson M. B., Murphy C. R., Dowland S. N., et al. (2020). Structural changes to the uterus of the dwarf ornate wobbegong shark (Orectolobus ornatus) during pregnancy. J. Morphol 281 (4-5), 428–437. doi: 10.1002/jmor.21109
Burger J. (1967). Problems in the electrolyte economy of the spiny dogfish, squalus acanthias. Sharks Skates Rays. (Baltimore: Johns Hopkins Press), 177–185.
Carcupino M., Baldacci A., Mazzini M., Franzoi P. (1997). Morphological organization of the male brood pouch epithelium of syngnathus abaster risso (Teleostea, syngnathidae) before, during, and after egg incubation. Tissue Cell. 29 (1), 21–30. doi: 10.1016/S0040-8166(97)80068-7
Carcupino M., Baldacci A., Mazzini M., Franzoi P. (2002). Functional significance of the male brood pouch in the reproductive strategies of pipefishes and seahorses: a morphological and ultrastructural comparative study on three anatomically different pouches. J. Fish Biol. 61 (6), 1465–1480. doi: 10.1111/j.1095-8649.2002.tb02490.x
Carmona S. J., Teichmann S. A., Ferreira L., Macaulay I. C., Stubbington M. J., Cvejic A., et al. (2017). Single-cell transcriptome analysis of fish immune cells provides insight into the evolution of vertebrate immune cell types. Genome Res. 27 (3), 451–461. doi: 10.1101/gr.207704.116
Chavan A. R., Griffith O. W., Wagner G. P. (2017). The inflammation paradox in the evolution of mammalian pregnancy: turning a foe into a friend. Curr. Opin. Genet. Dev. 47, 24–32. doi: 10.1016/j.gde.2017.08.004
Chen G., Ning B., Shi T. (2019). Single-cell RNA-seq technologies and related computational data analysis. Front. Genet. 10, 317. doi: 10.3389/fgene.2019.00317
Chen X., Qi J., He L., Luo H., Lin J., Qiu F., et al. (2022). Isolation and identification of a new strain of nervous necrosis virus from the big-belly seahorse hippocampus abdominalis. Virol. J. 19 (1), 1–9. doi: 10.1186/s12985-022-01837-8
Cheung P., Nigrelli R., Ruggieri G. (1980). Studies on the morphology of uronema marinum dujardin (Ciliatea: Uronematidae) with a description of the histopathology of the infection in marine fishes. J. Fish Dis. 3 (4), 295–303. doi: 10.1111/j.1365-2761.1980.tb00400.x
Clarke W. C., Bern H. A. (2012). Comparative endocrinology of prolactin. Hormonal Proteins Peptides 8, 105–197. doi: 10.1016/B978-0-12-447208-2.50010-1
Cone D. K., Appy R., Baggett L., King S., Gilmore S., Abbott C. (2013). A new gyrodactylid (Monogenea) parasitizing bay pipefish (Syngnathus leptorhynchus) from the pacific coast of north America. J. Parasitol. 99 (2), 183–188. doi: 10.1645/GE-3224.1
Cooper M. D., Alder M. N. (2006). The evolution of adaptive immune systems. Cell. 124 (4), 815–822. doi: 10.1016/j.cell.2006.02.001
Dawson C. E. (1986). “Syngnathidae,” in Fishes of the north-eastern Atlantic and Mediterranean II. Eds. Whitehead P., Bauchot M. L., Hureau J. C., Nielsen J. E. T. (Paris: UNESCO), 628–639.
Declercq A., Chiers K., Van Den Broeck W., Rekecki A., Teerlinck S., Adriaens D., et al. (2014). White necrotic tail tips in estuary seahorses, hippocampus kuda, bleeker. J. Fish Dis. 37 (5), 501–504. doi: 10.1111/jfd.12138
Dee C. T., Nagaraju R. T., Athanasiadis E. I., Gray C., Del Ama L. F., Johnston S. A., et al. (2016). CD4-transgenic zebrafish reveal tissue-resident Th2-and regulatory T cell–like populations and diverse mononuclear phagocytes. J. Immunol. 197 (9), 3520–3530. doi: 10.4049/jimmunol.1600959
Dekel N., Gnainsky Y., Granot I., Mor G. (2010). Inflammation and implantation. Am. J. Reprod. Immunol. 63 (1), 17–21. doi: 10.1111/j.1600-0897.2009.00792.x
Di Cicco E., Paradis E., Stephen C., Turba M. E., Rossi G. (2013). Scuticociliatid ciliate outbreak in australian pot-bellied seahorse, hippocampus abdominalis (Lesson, 1827): clinical signs, histopathologic findings, and treatment with metronidazole. J. Zoo Wildl Med. 44 (2), 435–440. doi: 10.1638/2012-127R1.1
Dijkstra J. M., Grimholt U., Leong J., Koop B. F., Hashimoto K. (2013). Comprehensive analysis of MHC class II genes in teleost fish genomes reveals dispensability of the peptide-loading DM system in a large part of vertebrates. BMC Evol. Biol. 13 (1), 1–14. doi: 10.1186/1471-2148-13-260
Dill J., Sanchez S., McDermott A., Camus A. (2017). Disseminated nocardiosis associated with the isolation of nocardia nova in a longsnout seahorse hippocampus reidi (Ginsburg). J. Fish Dis. 40 (9), 1235–1239. doi: 10.1111/jfd.12589
Dubin A., Jørgensen T. E., Moum T., Johansen S. D., Jakt L. M. (2019). Complete loss of the MHC II pathway in an anglerfish, lophius piscatorius. Biol. Lett. 15 (10), 20190594. doi: 10.1098/rsbl.2019.0594
Dudley J., Hannaford P., Dowland S., Lindsay L., Thompson M., Murphy C., et al. (2021). Structural changes to the brood pouch of male pregnant seahorses (Hippocampus abdominalis) facilitate exchange between father and embryos. Placenta 114, 115–123. doi: 10.1016/j.placenta.2021.09.002
Dyková I., Lom J. (2007). Histopathology of protistan and myxozoan infections in fishes: An atlas. (Praha: Academia), 219.
Edwards S. V., Hedrick P. W. (1998). Evolution and ecology of MHC molecules: from genomics to sexual selection. Trends Ecol. Evol. 13 (8), 305–311. doi: 10.1016/S0169-5347(98)01416-5
Ellis M. T., Otway N. M. (2011). Uterine fluid composition of the dwarf ornate wobbegong shark (Orectolobus ornatus) during gestation. Mar. Freshw. Res. 62 (6), 576–582. doi: 10.1071/MF10138
Evans D. H., Oikari A., Kormanik G. A., Mansberger L. (1982). Osmoregulation by the prenatal spiny dogfish, squalus acanthias. J. Exp. Biol. 101 (1), 295–305. doi: 10.1242/jeb.101.1.295
Falagas M. E., Mourtzoukou E. G., Vardakas K. Z. (2007). Sex differences in the incidence and severity of respiratory tract infections. Respir. Med. 101 (9), 1845–1863. doi: 10.1016/j.rmed.2007.04.011
Falk-Petersen I. (2005). Comparative organ differentiation during early life stages of marine fish. Fish Shellfish Immunol. 19 (5), 397–412. doi: 10.1016/j.fsi.2005.03.006
Ferrero G., Gomez E., Lyer S., Rovira M., Miserocchi M., Langenau D. M., et al. (2020). The macrophage-expressed gene (mpeg) 1 identifies a subpopulation of b cells in the adult zebrafish. J. Leukoc. Biol. 107 (3), 431–443. doi: 10.1002/JLB.1A1119-223R
Fiedler K. (1954). Vergleichende verhaltensstudien an seenadeln, schlangen nadeln und seepferdchen (Syngnathidae) 1. Z Tierpsychol 11 (3), 358–416. doi: 10.1111/j.1439-0310.1954.tb02165.x
Flajnik M. F. (2018). A cold-blooded view of adaptive immunity. Nat. Rev. Immunol. 18 (7), 438–453. doi: 10.1038/s41577-018-0003-9
Flajnik M. F., Kasahara M. (2010). Origin and evolution of the adaptive immune system: genetic events and selective pressures. Nat. Rev. Genet. 11 (1), 47. doi: 10.1038/nrg2703
Florio D., Marcer F., Fichtel L. (2004). Isolamento di tsukamurellapaurometabola e vibrio alginolyticus da hippocampus barbouri (Barbour’s seahorse) stabulati inacquario. Atti XI Convegno Nazionale S.I.P.I. 27.
Fogelson S. B., Camus A. C., Lorenz W., Phillips A., Bartlett P., Sanchez S. (2018). Mycobacterium syngnathidarum sp. nov., a rapidly growing mycobacterium identified in syngnathid fish. Int. J. Syst. Evol. Microbiol. 68 (12), 3696–3700. doi: 10.1099/ijsem.0.002978
Fogelson S., Fast M., Leary J., Camus A. (2017). Pathologic features of mycobacteriosis in naturally infected syngnathidae and novel transcriptome assembly in association with disease. J. Fish Dis. 40 (11), 1681–1694. doi: 10.1111/jfd.12634
Garner M., Atkinson S., Hallett S., Bartholomew J., Nordhausen R., Reed H., et al. (2008). Renal myxozoanosis in weedy sea dragons, phyllopteryx taeniolatus (Lacepede), caused by sinuolinea phyllopteryxa n. sp. J. Fish Dis. 31 (1), 27–35. doi: 10.1111/j.1365-2761.2007.00862.x
Goehlich H., Sartoris L., Wagner K.-S., Wendling C. C., Roth O. (2021). Pipefish locally adapted to low salinity in the Baltic Sea retain phenotypic plasticity to cope with ancestral salinity levels. Front. Ecol. Evol. 9, 93. doi: 10.3389/fevo.2021.626442
Gómez G. D., Balcázar J. L. (2008). A review on the interactions between gut microbiota and innate immunity of fish. FEMS Immunol. Med. Microbiol. 52 (2), 145–154. doi: 10.1111/j.1574-695X.2007.00343.x
Granot I., Gnainsky Y., Dekel N. (2012). Endometrial inflammation and effect on implantation improvement and pregnancy outcome. Reproduction. 144 (6), 661–668. doi: 10.1530/REP-12-0217
Grimholt U., Tsukamoto K., Azuma T., Leong J., Koop B. F., Dijkstra J. M. (2015). A comprehensive analysis of teleost MHC class I sequences. BMC Evol. Biol. 15 (1), 1–17. doi: 10.1186/s12862-015-0309-1
Grindstaff J. L., Brodie Iii E. D., Ketterson E. D. (2003). Immune function across generations: Integrating mechanism and evolutionary process in maternal antibody transmission. Proc. R Soc. Lond B Biol. Sci. 270 (1531), 2309–2319. doi: 10.1098/rspb.2003.2485
Guslund N., Krabberød A., Nørstebø S., Solbakken M., Jakobsen K., Johansen F.-E., et al. (2022). Lymphocyte subsets in Atlantic cod (Gadus morhua) interrogated by single-cell sequencing. Commun. Biol. 5, 689. doi: 10.21203/rs.3.rs-1342445/v1
Guslund N. C., Solbakken M. H., Brieuc M. S., Jentoft S., Jakobsen K. S., Qiao S.-W. (2020). Single-cell transcriptome profiling of immune cell repertoire of the Atlantic cod which naturally lacks the major histocompatibility class II system. Front. Immunol. 11, 2602. doi: 10.3389/fimmu.2020.559555
Haase D., Roth O., Kalbe M., Schmiedeskamp G., Scharsack J. P., Rosenstiel P., et al. (2013). Absence of major histocompatibility complex class II mediated immunity in pipefish, syngnathus typhle: evidence from deep transcriptome sequencing. Biol. Lett. 9 (2), 20130044. doi: 10.1098/rsbl.2013.0044
Hacquard S., Garrido-Oter R., González A., Spaepen S., Ackermann G., Lebeis S., et al. (2015). Microbiota and host nutrition across plant and animal kingdoms. Cell Host Microbe 17 (5), 603–616. doi: 10.1016/j.chom.2015.04.009
Haley P. J. (2017). The lymphoid system: a review of species differences. J. Toxicol. Pathol. 30 (2), 111–123. doi: 10.1293/tox.2016-0075
Hamilton J. (1948). “The role of testicular secretions as indicated by the effects of castration in man and by studies of pathological conditions and the short lifespan associated with maleness,” in Recent progress in hormone research: The proceedings of the laurentian hormone conference. 3 (New York: Academic Press), 257–322.
Harlin-Cognato A., Hoffman E. A., Jones A. G. (2006). Gene cooption without duplication during the evolution of a male-pregnancy gene in pipefish. PNAS. 103 (51), 19407–19412. doi: 10.1073/pnas.0603000103
Hedlund M., Stenqvist A.-C., Nagaeva O., Kjellberg L., Wulff M., Baranov V., et al. (2009). Human placenta expresses and secretes NKG2D ligands via exosomes that down-modulate the cognate receptor expression: evidence for immunosuppressive function. J. Immunol. 183 (1), 340–351. doi: 10.4049/jimmunol.0803477
He L., Long X., Qi J., Wang Z., Huang Z., Wu S., et al. (2021). Genome and gene evolution of seahorse species revealed by the chromosome-level genome of hippocampus abdominalis. Mol. Ecol. Resour 22 (4), 1465–1477. doi: 10.1111/1755-0998.13541
Herald E. S. (1959). From pipefish to seahorse — a study of phylogenetic relationships. Proc. Calif Acad. Sci. 29, 465–473.
Hernández P. P., Strzelecka P. M., Athanasiadis E. I., Hall D., Robalo A. F., Collins C. M., et al. (2018). Single-cell transcriptional analysis reveals ILC-like cells in zebrafish. Sci. Immunol. 3 (29), 29. doi: 10.1126/sciimmunol.aau5265
Hilgers L., Roth O., Nolte A. W., Schüller A., Spanke T., Flury J. M., et al. (2022). Inflammation and convergent placenta gene co-option contributed to a novel reproductive tissue. Curr. Biol. 32 (3), 715–24. e4. doi: 10.1016/j.cub.2021.12.004
Holliman R. B. (1963). Gyrodactylus shorti, a new species of monogenetic trematode from the brood pouch of the southern pipefish, Syngnathus scovelli (Evermann and Kendall). Tulane Stud. Zool. 10, 83–86. doi: 10.5962/bhl.part.4639
Hooper L. V., Littman D. R., Macpherson A. J. (2012). Interactions between the microbiota and the immune system. Science. 336 (6086), 1268–1273. doi: 10.1126/science.1223490
Islam S., Zeisel A., Joost S., La Manno G., Zajac P., Kasper M., et al. (2014). Quantitative single-cell RNA-seq with unique molecular identifiers. Nat. Methods 11 (2), 163–166. doi: 10.1038/nmeth.2772
Jiang H., Li C., Zhang B., Wu Y., Lin Q. (2022). Roles of interleukins in antibacterial immune defense of the brood pouch in the lined seahorse hippocampus erectus. J. Oceanol Limnol 40 (1), 235–244. doi: 10.1007/s00343-021-0310-z
Jiang H., Wang K., Fang Y., Chen J., Li Y., Xia G., et al. (2019). Sex-biased regulation of respiratory burst, phagocytic activity and plasma immune factors in lined seahorse (Hippocampus erectus) after subchronic benzo [a] pyrene exposure. Fish Shellfish Immunol. 86, 1162–1168. doi: 10.1016/j.fsi.2018.12.068
Jiang F., Yang N., Huang H., Feng H., Li Y., Han B. (2020). Recovery of vibrio vulnificus from head ulceration in seahorse (Hippocampus kuda). Aquac Int. 28 (2), 653–660. doi: 10.1007/s10499-019-00486-z
Kang G., Choi K.-M., Joo M.-S., Woo W.-S., Park C.-I. (2022). A case report of secondary infection by vibrio splendidus associated with gas bubble disease in syngnathid fishes (Syngnathus schlegeli and hippocampus haema). Fish Aquat Sci. 25 (1), 40–48. doi: 10.47853/FAS.2022.e5
Kasahara M. (2000). Major histocompatibility complex: evolution, structure, and function (Japan: Springer).
Keller I. S., Roth O. (2020). Parental investment and immune dynamics in sex-role reversed pipefishes. PLos One 15 (9), e0228974. doi: 10.1371/journal.pone.0228974
Koldewey H. (2005). Syngnathid husbandry in public aquariums 2005 manual (London, UK: Project Seahorse).
Koldewey H. J., Martin-Smith K. M. (2010). A global review of seahorse aquaculture. Aquaculture. 302 (3-4), 131–152. doi: 10.1016/j.aquaculture.2009.11.010
Kurtz J., Wiesner A., Götz P., Sauer K. P. (2000). Gender differences and individual variation in the immune system of the scorpionfly panorpa vulgaris (Insecta: Mecoptera). Dev. Comp. Immunol. 24 (1), 1–12. doi: 10.1016/S0145-305X(99)00057-9
Landis S. H., Sundin J., Rosenqvist G., Roth O. (2012). Behavioral adjustments of a pipefish to bacterial vibrio challenge. Behav. Ecol. Sociobiol 66 (10), 1399–1405. doi: 10.1007/s00265-012-1395-3
Langdon J., Elliott K., Mackay B. (1991). Epitheliocystis in the leafy sea-dragon. Aust. Vet. J. 68, 244. doi: 10.1111/j.1751-0813.1991.tb03219.x
Langevin C., Aleksejeva E., Houel A., Briolat V., Torhy C., Lunazzi A., et al. (2017). FTR83, a member of the large fish-specific finTRIM family, triggers IFN pathway and counters viral infection. Front. Immunol. 8, 617. doi: 10.3389/fimmu.2017.00617
Larange A., Cheroutre H. (2016). Retinoic acid and retinoic acid receptors as pleiotropic modulators of the immune system. Annu. Rev. Immunol. 34, 369–394. doi: 10.1146/annurev-immunol-041015-055427
La Rocca C., Carbone F., Longobardi S., Matarese G. (2014). The immunology of pregnancy: regulatory T cells control maternal immune tolerance toward the fetus. Immunol. Lett. 162 (1), 41–48. doi: 10.1016/j.imlet.2014.06.013
LePage V. (2012). A study of syngnathid diseases and investigation of ulcerative dermatitis [Masters thesis] (Ontario, Canada: University of Guelph).
LePage V., Dutton C. J., Kummrow M., McLelland D. J., Young K., Lumsden J. S. (2012). Neoplasia of captive yellow sea horses (Hippocampus kuda) and weedy sea dragons (Phyllopteryx taeniolatus). J. Zoo Wildl Med. 43 (1), 50–58. doi: 10.1638/2010-0236.1
LePage V., Young J., Dutton C., Crawshaw G., Paré J., Kummrow M., et al. (2015). Diseases of captive yellow seahorse hippocampus kuda b leeker, pot-bellied seahorse hippocampus abdominalis l esson and weedy seadragon phyllopteryx taeniolatus (L acépède). J. Fish Dis. 38 (5), 439–450. doi: 10.1111/jfd.12254
Li C., Li Y., Qin G., Chen Z., Qu M., Zhang B., et al. (2020). Regulatory role of retinoic acid in Male pregnancy of the seahorse. Innovation 1 (3), 100052. doi: 10.1016/j.xinn.2020.100052
Limoges M.-A., Cloutier M., Nandi M., Ilangumaran S., Ramanathan S. (2021). The GIMAP family proteins: an incomplete puzzle. Front. Immunol. 12, 679739. doi: 10.3389/fimmu.2021.679739
Lin Q., Fan S., Zhang Y., Xu M., Zhang H., Yang Y., et al. (2016). The seahorse genome and the evolution of its specialized morphology. Nature 540 (7633), 395. doi: 10.1038/nature20595
Lin Q., Lin J., Huang L. (2010). Effects of light intensity, stocking density and temperature on the air-bubble disease, survivorship and growth of early juvenile seahorse hippocampus erectus perry, 1810. Aquac Res. 42 (1), 91–98. doi: 10.1111/j.1365-2109.2010.02573.x
Lin T., Liu X., Xiao D., Zhang D. (2017). Plasma levels of immune factors and sex steroids in the male seahorse hippocampus erectus during a breeding cycle. Fish Physiol. Biochem. 43 (3), 889–899. doi: 10.1007/s10695-017-0343-6
Lin T., Zhang D., Liu X., Xiao D. (2016a). Parental care improves immunity in the seahorse (Hippocampus erectus). Fish Shellfish Immunol. 58, 554–562. doi: 10.1016/j.fsi.2016.09.065
Lin T., Zhang D., Liu X., Xiao D. (2016b). Variations of immune parameters in the lined seahorse hippocampus erectus after infection with enteritis pathogen of vibrio parahaemolyticus. Fish Shellfish Immunol. 50, 247–254. doi: 10.1016/j.fsi.2016.01.039
Li C., Olave M., Hou Y., Qin G., Schneider R. F., Gao Z., et al. (2021). Genome sequences reveal global dispersal routes and suggest convergent genetic adaptations in seahorse evolution. Nat. Commun. 12 (1), 1–11. doi: 10.1038/s41467-021-21379-x
Li Y., Wang Z., Zheng F., Wang B., Liu H., Zhang P., et al. (2020). Identification and characterization of the pathogen associated with skin ulcer syndrome in lined seahorse, hippocampus erectus. Aquac Res. 51 (3), 989–999. doi: 10.1111/are.14445
Ljunggren H.-G., Kärre K. (1990). In search of the ‘missing self’: MHC molecules and NK cell recognition. Immunol. Today 11, 237–244. doi: 10.1016/0167-5699(90)90097-S
Loes A. N., Hinman M. N., Farnsworth D. R., Miller A. C., Guillemin K., Harms M. J. (2021). Identification and characterization of zebrafish Tlr4 coreceptor md-2. J. Immunol. 206 (5), 1046–1057. doi: 10.4049/jimmunol.1901288
Lom J., Dyková I. (2005). Microsporidian xenomas in fish seen in wider perspective. Folia Parasitol. (Praha) 52 (1-2), 69. doi: 10.14411/fp.2005.010
Longshaw M., Green M., Feist S. (2004). Histopathology of parasitic infections in greater pipefish, syngnathus acus l., from an estuary in the UK. J. Fish Dis. 27 (4), 245–248. doi: 10.1111/j.1365-2761.2004.00533.x
Lu L., Loker E. S., Zhang S.-M., Buddenborg S. K., Bu L. (2020). Genome-wide discovery, and computational and transcriptional characterization of an AIG gene family in the freshwater snail biomphalaria glabrata, a vector for schistosoma mansoni. BMC Genomics 21 (1), 1–20. doi: 10.1186/s12864-020-6534-z
Luo W., Wang X., Qu H., Qin G., Zhang H., Lin Q. (2016). Genomic structure and expression pattern of MHC IIα and IIβ genes reveal an unusual immune trait in lined seahorse hippocampus erectus. Fish Shellfish Immunol. 58, 521–529. doi: 10.1016/j.fsi.2016.09.057
Møller A., Sorci G., Erritzøe J. (1998). Sexual dimorphism in immune defense. Am. Nat. 152 (4), 605–619. doi: 10.1086/286193
Malmstrøm M., Matschiner M., Tørresen O. K., Star B., Snipen L. G., Hansen T. F., et al. (2016). Evolution of the immune system influences speciation rates in teleost fishes. Nat. Genet. 48 (10), 1204–1210. doi: 10.1038/ng.3645
Marcer F., Florio D., Galuppi R., Gustinelli A., Fichtel L., Fioravantil M. (2005). Pathological findings in Syngnathids kept in an Italian public aquarium. Abstract book EAFP 12th International Conference; (Copenhagen, Denmark), pp. 172.
Martins M., Mouriño J., Fezer G., Buglione Neto C., Garcia P., Silva B., et al. (2010). Isolation and experimental infection with vibrio alginolyticus in the sea horse, hippocampus reidi ginsburg, 1933 (Osteichthyes: Syngnathidae) in Brazil. Braz. J. Biol. 70, 205–209. doi: 10.1590/S1519-69842010000100028
Matsunaga T., Rahman A. (1998). What brought the adaptive immune system to vertebrates?-the jaw hypothesis and the seahorse. Immunol. Rev. 166 (1), 177–186. doi: 10.1111/j.1600-065X.1998.tb01262.x
Mayer I., Rosenqvist G., Borg B., Ahnesjö I., Berglund A., Schulz R. W. (1993). Plasma levels of sex steroids in three species of pipefish (Syngnathidae). Can. J. Zool. 71 (9), 1903–1907. doi: 10.1139/z93-272
Medzhitov R., Janeway C. (2000). Innate immunity. N Engl. J. Med. 343 (5), 338–344. doi: 10.1056/NEJM200008033430506
Meijer A., Roholl P. J., Ossewaarde J. M., Jones B., Nowak B. F. (2006). Molecular evidence for association of chlamydiales bacteria with epitheliocystis in leafy seadragon (Phycodurus eques), silver perch (Bidyanus bidyanus), and barramundi (Lates calcarifer). Appl. Environ. Microbiol. 72 (1), 284–290. doi: 10.1128/AEM.72.1.284-290.2006
Melamed P., Xue Y., Poon J. F. D., Wu Q., Xie H., Yeo J., et al. (2005). The male seahorse synthesizes and secretes a novel c-type lectin into the brood pouch during early pregnancy. FEBS J. 272 (5), 1221–1235. doi: 10.1111/j.1742-4658.2005.04556.x
Meng Q., Yu K. (1985). A new species of ciliata, licnophora hippocampi sp. nov., from the seahorse hippocampus trimaculatus leach, with considerations of its control in the host. Acta Zoologica Sinica 31 (1), 65–69.
Milan M., Dalla Rovere G., Smits M., Ferraresso S., Pastore P., Marin M., et al. (2018). Ecotoxicological effects of the herbicide glyphosate in non-target aquatic species: Transcriptional responses in the mussel mytilus galloprovincialis. Environ. pollut. 237, 442–451. doi: 10.1016/j.envpol.2018.02.049
Moffett-King A. (2002). Natural killer cells and pregnancy. Nat. Rev. Immunol. 2 (9), 656–663. doi: 10.1038/nri886
Moffett A., Loke C. (2006). Immunology of placentation in eutherian mammals. Nat. Rev. Immunol. 6 (8), 584–594. doi: 10.1038/nri1897
Mor G., Abrahams V. M. (2002). Immunology of implantation. Immunol. Allergy Clin. North Am. 22 (3), 545–565. doi: 10.1016/S0889-8561(02)00009-7
Neefjes J., Jongsma M. L., Paul P., Bakke O. (2011). Towards a systems understanding of MHC class I and MHC class II antigen presentation. Nat. Rev. Immunol. 11 (12), 823–836. doi: 10.1038/nri3084
Niu J., Huang Y., Liu X., Zhang Z., Tang J., Wang B., et al. (2020). Single-cell RNA-seq reveals different subsets of non-specific cytotoxic cells in teleost. Genomics 112 (6), 5170–5179. doi: 10.1016/j.ygeno.2020.09.031
Novelli B., Socorro J., Caballero M., Otero-Ferrer F., Segade-Botella A., Molina Domínguez L. (2015). Development of seahorse (Hippocampus reidi, ginsburg 1933): histological and histochemical study. Fish Physiol. Biochem. 41 (5), 1233–1251. doi: 10.1007/s10695-015-0082-5
Nyaoke A., Weber E. S., Innis C., Stremme D., Dowd C., Hinckley L., et al. (2009). Disseminated phaeohyphomycosis in weedy seadragons (Phyllopteryx taeniolatus) and leafy seadragons (Phycodurus eques) caused by species of exophiala, including a novel species. J. Vet. Diagn. Invest. 21 (1), 69–79. doi: 10.1177/104063870902100111
Ofelio C., Blanco A., Roura Á, Pintado J., Pascual S., Planas M. (2014). Isolation and molecular identification of the scuticociliate p orpostoma notata moebius, 1888 from moribund reared h ippocampus hippocampus (L.) seahorses, by amplification of the SSU rRNA gene sequences. J. Fish Dis. 37 (12), 1061–1065. doi: 10.1111/jfd.12207
Ofelio C., Díaz A. O., Radaelli G., Planas M. (2018). Histological development of the long-snouted seahorse hippocampus guttulatus during ontogeny. J. Fish Biol. 93 (1), 72–87. doi: 10.1111/jfb.13668
Osborn A., Stamper M., Reimschuessel R., Greenwell M., Zwick L., Kinsel M. (1999). Coccidiosis in the weedy seadragon (Phyllopteryx taeniolatus). Proc. Int. Assoc. Aquat Med. 30, 77–80.
Ozato K., Shin D.-M., Chang T.-H., Morse H. C. (2008). TRIM family proteins and their emerging roles in innate immunity. Nat. Rev. Immunol. 8 (11), 849–860. doi: 10.1038/nri2413
Paladini G., Fioravanti M. L., Cable J., Shinn A. (2010). The description of gyrodactylus corleonis sp. n. and g. neretum sp. n.(Platyhelminthes: Monogenea) with comments on other gyrodactylids parasitizing pipefish (Pisces: Syngnathidae). Folia Parasitol. (Praha) 57 (1), 17–30. doi: 10.14411/fp.2010.004
Páll M., Liljander M., Borg B. (2004). Prolactin diminishes courtship behaviour and stimulates fanning in nesting male three-spined sticklebacks, gasterosteus aculeatus. Behaviour. 141 (11-12), 1511–1519. doi: 10.1163/1568539042948088
Parker J., Dubin A., Schneider R., Wagner K., Jentoft S., Böhne A., et al. (2022). Immunological tolerance in the evolution of male pregnancy. Mol. Ecol. 00, 1–22. doi: 10.1111/mec.16333
Parker J., Guslund N S. J., Roth O. (2022). Characterization of pipefish immune cell populations through single-cell transcriptomics. Front. Immunol. 13, 820152. doi: 10.3389/fimmu.2022.820152
Parker J., Roth O. (2022). Comparative assessment of immunological tolerance in fish with natural immunodeficiency. Dev. Comp. Immunol. 132, 104393. doi: 10.1016/j.dci.2022.104393
Patron J. J., Herrera A. A., Oconer E. P. (2008). Prolactin and growth hormone levels in the pouch fluid of gravid male seahorse, hipocampus barbouri Jordan and Richardson 1908. Asia Life Sci. 17 (2), 261–269.
Paul J. W., Kemsley J. O., Butler T. A., Tolosa J. M., Thompson M. B., Smith R., et al. (2020). A comparison of uterine contractile responsiveness to arginine vasopressin in oviparous and viviparous lizards. J. Comp. Physiol B 190 (1), 49–62. doi: 10.1007/s00360-019-01254-4
Perdiguero P., Morel E., Tafalla C. (2021). Diversity of rainbow trout blood b cells revealed by single cell RNA sequencing. Biology. 10 (6), 511. doi: 10.3390/biology10060511
Peuß R., Box A. C., Chen S., Wang Y., Tsuchiya D., Persons J. L., et al. (2020). Adaptation to low parasite abundance affects immune investment and immunopathological responses of cavefish. Nat. Ecol. Evol. 4 (10), 1416–1430. doi: 10.1038/s41559-020-1234-2
Poiani A. (2006). Complexity of seminal fluid: a review. Behav. Ecol. Sociobiol 60 (3), 289–310. doi: 10.1007/s00265-006-0178-0
Prosser C. M., Unger M. A., Vogelbein W. K. (2011). Multistressor interactions in the zebrafish (Danio rerio): Concurrent phenanthrene exposure and mycobacterium marinum infection. Aquat Toxicol. 102 (3-4), 177–185. doi: 10.1016/j.aquatox.2011.01.011
Qin G., Wang X., Tan S., Lin Q. (2017). A bacterial infection by vibrio harveyi causing heavy reduction of cultured lined seahorse hippocampus erectus. J. Fish Dis. 40 (4), 601–605. doi: 10.1111/jfd.12533
Qu M., Liu Y., Zhang Y., Wan S., Ravi V., Qin G., et al. (2021). Seadragon genome analysis provides insights into its phenotype and sex determination locus. Sci. Adv. 7 (34), eabg5196. doi: 10.1126/sciadv.abg5196
Raj S. T., Lipton A., Chauhan G. (2010). Characterization and infectivity evaluation of vibrio harveyi causing white patch disease among captive reared seahorses, hippocampus kuda. Indian J. Mar. Sci. 39 (1), 151–156.
Richards J. G., Farrell A. P., Brauner C. J. (2009). Fish physiology: Hypoxia (New York: Academic Press).
Ripley J. L., Foran C. M. (2010). Quantification of whole brain arginine vasotocin for two syngnathus pipefishes: elevated concentrations correlated with paternal brooding. Fish Physiol. Biochem. 36 (4), 867–874. doi: 10.1007/s10695-009-9361-3
Ripley J., Williams P., Foran C. (2010). Morphological and quantitative changes in paternal brood-pouch vasculature during embryonic development in two syngnathus pipefishes. J. Fish Biol. 77 (1), 67–79. doi: 10.1111/j.1095-8649.2010.02659.x
Robertson S. A. (2005). Seminal plasma and male factor signalling in the female reproductive tract. Cell Tissue Res. 322 (1), 43–52. doi: 10.1007/s00441-005-1127-3
Robertson S. A., Care A. S., Moldenhauer L. M. (2018). Regulatory T cells in embryo implantation and the immune response to pregnancy. J. Clin. Invest 128 (10), 4224–4235. doi: 10.1172/JCI122182
Robertson S. A., Chin P. Y., Glynn D. J., Thompson J. G. (2011). Peri-conceptual cytokines–setting the trajectory for embryo implantation, pregnancy and beyond. Am. J. Reprod. Immunol. 66, 2–10. doi: 10.1111/j.1600-0897.2011.01039.x
Robertson S. A., Prins J. R., Sharkey D. J., Moldenhauer L. M. (2013). Seminal fluid and the generation of regulatory T cells for embryo implantation. Am. J. Reprod. Immunol. 69 (4), 315–330. doi: 10.1111/aji.12107
Robinson C. J., Bohannan B. J., Young V. B. (2010). From structure to function: the ecology of host-associated microbial communities. Microbiol. Mol. Biol. Rev. 74 (3), 453–476. doi: 10.1128/MMBR.00014-10
Robinson D. P., Klein S. L. (2012). Pregnancy and pregnancy-associated hormones alter immune responses and disease pathogenesis. Horm. Behav. 62 (3), 263–271. doi: 10.1016/j.yhbeh.2012.02.023
Rolff J. (2002). Bateman's principle and immunity. Proc. R Soc. Lond B Biol. Sci. 269 (1493), 867–872. doi: 10.1098/rspb.2002.1959
Rose E., Flanagan S. P., Jones A. G. (2015). The effects of synthetic estrogen exposure on the sexually dimorphic liver transcriptome of the sex-role-reversed gulf pipefish. PLosOne 10 (10), e0139401. doi: 10.1371/journal.pone.0139401
Rossteuscher S., Wenker C., Jermann T., Wahli T., Oldenberg E., Schmidt-Posthaus H. (2008). Severe scuticociliate (Philasterides dicentrarchi) infection in a population of sea dragons (Phycodurus eques and phyllopteryx taeniolatus). Vet. Pathol. 45 (4), 546–550. doi: 10.1354/vp.45-4-546
Roth O., Beemelmanns A., Barribeau S. M., Sadd B. M. (2018). Recent advances in vertebrate and invertebrate transgenerational immunity in the light of ecology and evolution. Heredity. 121 (3), 225–238. doi: 10.1038/s41437-018-0101-2
Roth O., Ebert D., Vizoso D. B., Bieger A., Lass S. (2008). Male-Biased sex-ratio distortion caused by octosporea bayeri, a vertically and horizontally-transmitted parasite of daphnia magna. Int. J. Parasitol. 38 (8-9), 969–979. doi: 10.1016/j.ijpara.2007.11.009
Roth O., Keller I., Landis S. H., Salzburger W., Reusch T. B. (2012). Hosts are ahead in a marine host–parasite coevolutionary arms race: innate immune system adaptation in pipefish syngnathus typhle against vibrio phylotypes. Evolution. 66 (8), 2528–2539. doi: 10.1111/j.1558-5646.2012.01614.x
Roth O., Klein V., Beemelmanns A., Scharsack J. P., Reusch T. B. (2012). Male Pregnancy and biparental immune priming. Am. Nat. 180 (6), 802–814. doi: 10.1086/668081
Roth O., Landis S. H. (2017). Trans-generational plasticity in response to immune challenge is constrained by heat stress. Evol. Appl. 10 (5), 514–528. doi: 10.1111/eva.12473
Roth O., Scharsack J., Keller I., Reusch T. B. (2011). Bateman’s principle and immunity in a sex-role reversed pipefish. J. Evol. Biol. 24 (7), 1410–1420. doi: 10.1111/j.1420-9101.2011.02273.x
Roth O., Solbakken M. H., Tørresen O. K., Bayer T., Matschiner M., Baalsrud H. T., et al. (2020). Evolution of male pregnancy associated with remodeling of canonical vertebrate immunity in seahorses and pipefishes. PNAS 117 (17), 9431–9439. doi: 10.1073/pnas.1916251117
Salinas I. (2015). The mucosal immune system of teleost fish. Biology 4 (3), 525–539. doi: 10.3390/biology4030525
Salter C. E., Donnell K. O., Sutton D. A., Marancik D. P., Knowles S., Clauss T. M., et al. (2012). Dermatitis and systemic mycosis in lined seahorses hippocampus erectus associated with a marine-adapted fusarium solani species complex pathogen. Dis. Aquat Organ 101 (1), 23–31. doi: 10.3354/dao02506
Sanaye S., Pawar H., Murugan A., Sreepada R., Singh T., Ansari Z. (2013). Diseases and parasites in cultured yellow seahorse, hippocampus kuda (Bleeker, 1852). Fish Chimes 32, 65–67.
Sang P. S., Jee E. H., Dennis K. G., Ji H. K., Casiano H. C. J., Jin W. J., et al. (2011). Identification of scuticociliate philasterides dicentrarchi from indo-pacific seahorses hippocampus kuda. Afr J. Microbiol. Res. 5 (7), 738–741. doi: 10.5897/AJMR10.294
Scobell S., MacKenzie D. (2011). Reproductive endocrinology of syngnathidae. J. Fish Biol. 78 (6), 1662–1680. doi: 10.1111/j.1095-8649.2011.02994.x
Sears B., Anderson P., Greiner E. (2011). A new species of myxosporean (Sphaeromyxidae), a parasite of lined seahorses, hippocampus erectus, from the gulf of Mexico. J. Parasitol. 97 (4), 713–716. doi: 10.1645/GE-2565.1
Secombes C. J., Wang T., Bird S. (2011). The interleukins of fish. Dev. Comp. Immunol. 35 (12), 1336–1345. doi: 10.1016/j.dci.2011.05.001
Shao P., Yong P., Wang X., Xie S., Fan Y., Zang L., et al. (2019). Isolation, identification, and histopathological analysis of vibrio tubiashii from lined seahorse hippocampus erectus. Dis. Aquat Organ 133 (3), 195–205. doi: 10.3354/dao03350
Small C. M., Bassham S., Catchen J., Amores A., Fuiten A. M., Brown R. S., et al. (2016). The genome of the gulf pipefish enables understanding of evolutionary innovations. Genome Biol. 17 (1), 258. doi: 10.1186/s13059-016-1126-6
Small C. M., Harlin-Cognato A. D., Jones A. G. (2013). Functional similarity and molecular divergence of a novel reproductive transcriptome in two male-pregnant syngnathus pipefish species. Ecol. Evol. 3 (12), 4092–4108. doi: 10.1002/ece3.763
Small C. M., Healey H. M., Currey M. C., Beck E. A., Catchen J., Lin A. S., et al. (2022). Leafy and weedy seadragon genomes connect genic and repetitive DNA features to the extravagant biology of syngnathid fishes. PNAS 119 (26), e2119602119. doi: 10.1073/pnas.2119602119
Smith N. C., Umasuthan N., Kumar S., Woldemariam N. T., Andreassen R., Christian S. L., et al. (2021). Transcriptome profiling of Atlantic salmon adherent head kidney leukocytes reveals that macrophages are selectively enriched during culture. Front. Immunol. 12. doi: 10.3389/fimmu.2021.709910
Solbakken M. H., Tørresen O. K., Nederbragt A. J., Seppola M., Gregers T. F., Jakobsen K. S., et al. (2016). Evolutionary redesign of the Atlantic cod (Gadus morhua l.) toll-like receptor repertoire by gene losses and expansions. Sci. Rep. 6 (1), 1–14. doi: 10.1038/srep25211
Star B., Jentoft S. (2012). Why does the immune system of Atlantic cod lack MHC II? Bioessays. 34 (8), 648–651. doi: 10.1002/bies.201200005
Star B., Nederbragt A. J., Jentoft S., Grimholt U., Malmstrøm M., Gregers T. F., et al. (2011). The genome sequence of Atlantic cod reveals a unique immune system. Nature 477 (7363), 207. doi: 10.1038/nature10342
Stölting K. N., Wilson A. B. (2007). Male Pregnancy in seahorses and pipefish: beyond the mammalian model. Bioessays. 29 (9), 884–896. doi: 10.1002/bies.20626
Sunyem P., Vooren C. (1997). On cloacal gestation in angel sharks from southern Brazil. J. Fish Biol. 50 (1), 86–94. doi: 10.1111/j.1095-8649.1997.tb01341.x
Swann J. B., Holland S. J., Petersen M., Pietsch T. W., Boehm T. (2020). The immunogenetics of sexual parasitism. Science. 369 (6511), 1608–1615. doi: 10.1126/science.aaz9445
Tang Q., Iyer S., Lobbardi R., Moore J. C., Chen H., Lareau C., et al. (2017). Dissecting hematopoietic and renal cell heterogeneity in adult zebrafish at single-cell resolution using RNA sequencing. J. Exp. Med. 214 (10), 2875–2887. doi: 10.1084/jem.20170976
Tendencia E. A. (2004). The first report of vibrio harveyi infection in the sea horse hippocampus kuda bleekers 1852 in the Philippines. Aquac Res. 35 (13), 1292–1294. doi: 10.1111/j.1365-2109.2004.01109.x
Tharuka M. N., Priyathilaka T. T., Yang H., Pavithiran A., Lee J. (2019). Molecular and transcriptional insights into viperin protein from big-belly seahorse (Hippocampus abdominalis), and its potential antiviral role. Fish Shellfish Immunol. 86, 599–607. doi: 10.1016/j.fsi.2018.12.006
Thompson F. L., Iida T., Swings J. (2004). Biodiversity of vibrios. Microbiol. Mol. Biol. Rev. 68 (3), 403–431. doi: 10.1128/MMBR.68.3.403-431.2004
Thompson J., Moewus L. (1964). Miamiensis avidus ng, n. sp., a marine facultative parasite in the ciliate order hymenostomatida. J. Protozool 11 (3), 378–381. doi: 10.1111/j.1550-7408.1964.tb01766.x
Tomita T., Cotton C. F., Toda M. (2016). Ultrasound and physical models shed light on the respiratory system of embryonic dogfishes. Zoology. 119 (1), 36–41. doi: 10.1016/j.zool.2015.09.002
Tomita T., Nozu R., Nakamura M., Matsuzaki S., Miyamoto K., Sato K. (2017). Live-bearing without placenta: Physical estimation indicates the high oxygen-supplying ability of white shark uterus to the embryo. Sci. Rep. 7 (1), 1–7. doi: 10.1038/s41598-017-11973-9
Tort L., Balasch J., Mackenzie S. (2003). Fish immune system. a crossroads between innate and adaptive responses. Inmunología. 22 (3), 277–286.
Tremellen K. P., Robertson S. A. (1999). "Seminal ‘priming’ for successful mammalian pregnancy." in Reproductive immunology. Eds. S. K. Gupta (Dordrecht: Springer) 88–98. doi: 10.1007/978-94-011-4197-0_9
Umehara A., Kosuga Y., Hirose H. (2003). Scuticociliata infection in the weedy sea dragon phyllopteryx taeniolatus. Parasitol. Int. 52 (2), 165–168. doi: 10.1016/S1383-5769(02)00080-6
Upton S. J., Stamper M. A., Osborn A. L., Mumford S. L., Zwick L., Kinsel M. J., et al. (2000). A new species of eimeria (Apicomplexa, eimeriidae) from the weedy sea dragon phyllopteryx taeniolatus (Osteichthyes: Syngnathidae). Dis. Aquat Organ 43 (1), 55–59. doi: 10.3354/dao043055
Van Der Aa L. M., Levraud J.-P., Yahmi M., Lauret E., Briolat V., Herbomel P., et al. (2009). A large new subset of TRIM genes highly diversified by duplication and positive selection in teleost fish. BMC Biol. 7 (1), 1–23. doi: 10.1186/1741-7007-7-7
Vasta G. R., Nita-Lazar M., Giomarelli B., Ahmed H., Du S., Cammarata M., et al. (2011). Structural and functional diversity of the lectin repertoire in teleost fish: relevance to innate and adaptive immunity. Dev. Comp. Immunol. 35 (12), 1388–1399. doi: 10.1016/j.dci.2011.08.011
Vaughan D., Christison K., Hansen H., Shinn A. (2010). Gyrodactylus eyipayipi sp. n.(Monogenea: gyrodactylidae) from syngnathus acus (Syngnathidae) from south Africa. Folia Parasitol. (Praha) 57 (1), 11–15. doi: 10.14411/fp.2010.002
Venkatesh B., Lee A. P., Ravi V., Maurya A. K., Lian M. M., Swann J. B., et al. (2014). Elephant shark genome provides unique insights into gnathostome evolution. Nature 505 (7482), 174–179. doi: 10.1038/nature12826
Vincent A. C., Clifton-Hadley R. S. (1989). Parasitic infection of the seahorse (Hippocampus erectus)–a case report. J. Wildl Dis. 25 (3), 404–406. doi: 10.7589/0090-3558-25.3.404
Wagner K.-S. (2019). Cultivation and characterization of sex-specific microbiota in the broadnosed pipefish syngnathus typhle [Masters thesis] (Kiel, Germany: Christian-Albrechts-Universität Kiel).
Wang Y., Ying N., Huang Y., Zou X., Liu X., Li L., et al. (2022). Nucleospora hippocampi n. sp., an intranuclear microsporidian infecting the seahorse hippocampus erectus from China. Front. Cell Infect. 12, 882843. doi: 10.3389/fcimb.2022.882843
Wang X., Zhang Y., Qin G., Luo W., Lin Q. (2016). A novel pathogenic bacteria (Vibrio fortis) causing enteritis in cultured seahorses, hippocampus erectus perry, 1810. J. Fish Dis. 39 (6), 765–769. doi: 10.1111/jfd.12411
Watanabe S. (1999). The role of male brood pouch in the reproduction of the seaweed pipefish, syngnathus schlegeli (Tokyo: University of Tokyo).
Weiss Y., Forêt S., Hayward D. C., Ainsworth T., King R., Ball E. E., et al. (2013). The acute transcriptional response of the coral acropora millepora to immune challenge: expression of GiMAP/IAN genes links the innate immune responses of corals with those of mammals and plants. BMC Genomics 14 (1), 1–13. doi: 10.1186/1471-2164-14-400
Whittington C. M., Friesen C. R. (2020). The evolution and physiology of male pregnancy in syngnathid fishes. Biol. Rev. 95 (5), 1252–1272. doi: 10.1111/brv.12607
Whittington C. M., Griffith O. W., Qi W., Thompson M. B., Wilson A. B. (2015). Seahorse brood pouch transcriptome reveals common genes associated with vertebrate pregnancy. Mol. Biol. Evol. 32 (12), 3114–3131. doi: 10.1093/molbev/msv177
Whittington C. M., Wilson A. B. (2013). The role of prolactin in fish reproduction. Gen. Comp. Endocrinol. 191, 123–136. doi: 10.1016/j.ygcen.2013.05.027
Wijerathna H., Nadarajapillai K., Udayantha H., Kasthuriarachchi T., Shanaka K., Kwon H., et al. (2022). Molecular delineation, expression profiling, immune response, and anti-apoptotic function of a novel clusterin homolog from big-belly seahorse (Hippocampus abdominalis). Fish Shellfish Immunol. 124, 289–299. doi: 10.1016/j.fsi.2022.04.015
Willens S., Dunn J. L., Frasca S. (2004). Fibrosarcoma of the brood pouch in an aquarium-reared lined seahorse (Hippocampus erectus). J. Zoo Wildl Med. 35 (1), 107–109. doi: 10.1638/02-085
Williams S. R., Kritsky D. C., Dunnigan B., Lash R., Klein P. (2008). Gyrodactylus pisculentus sp. n.(Monogenoidea: Gyrodactylidae) associated with mortality of the northern pipefish, syngnathus fuscus (Syngnathiformes: Syngnathidae) at the woods hole science aquarium. Folia Parasitol. (Praha) 55 (4), 265. doi: 10.14411/fp.2008.034
Wilson A. B., Vincent A., Ahnesjö I., Meyer A. (2001). Male Pregnancy in seahorses and pipefishes (family syngnathidae): rapid diversification of paternal brood pouch morphology inferred from a molecular phylogeny. J. Hered. 92 (2), 159–166. doi: 10.1093/jhered/92.2.159
Woo P. T., Buchmann K. (2012). Fish parasites: pathobiology and protection (Wallingford, UK: CAB International).
Woods C. M. (2000). Improving initial survival in cultured seahorses, hippocampus abdominalis leeson, 1827 (Teleostei: Syngnathidae). Aquaculture 190 (3-4), 377–388. doi: 10.1016/S0044-8486(00)00408-7
Wu L., Gao A., Li L., Chen J., Li J., Ye J. (2021). A single-cell transcriptome profiling of anterior kidney leukocytes from Nile tilapia (Oreochromis niloticus). Front. Immunol. 12. doi: 10.3389/fimmu.2021.783196
Wu Y., Liu Y., Zhang H., Wang X., Lin Q. (2021). Expression patterns of alpha-2 macroglobulin reveal potential immune functions in brood pouch of the lined seahorse hippocampus erectus. Aquaculture. 533, 736064. doi: 10.1016/j.aquaculture.2020.736064
Wu Y., Zhang H., Zhang B., Lin Q., Liu Y. (2021). Molecular characterization of TLR22 and its role in immunological modification of the brood pouch of the lined seahorse, hippocampus erectus. Aquaculture. 539, 736628. doi: 10.1016/j.aquaculture.2021.736628
Xiao W., Chen Z., Zhang Y., Wu Y., Jiang H., Zhang H., et al. (2022). Hepcidin gene Co-option balancing paternal immune protection and Male pregnancy. Front. Immunol. 13, 884417. doi: 10.3389/fimmu.2022.884417
Xiao W., Qin G., Zhang Y., Liu Y., Zhang H., Chen Z., et al. (2022). The characterization of secretory phospholipase A2 group IB and its functional profiles during male pregnancy of lined seahorse hippocampus erectus. Aquaculture 553, 738065. doi: 10.1016/j.aquaculture.2022.738065
Xie J., Bu L., Jin S., Wang X., Zhao Q., Zhou S., et al. (2020). Outbreak of vibriosis caused by vibrio harveyi and vibrio alginolyticus in farmed seahorse hippocampus kuda in China. Aquaculture 523, 735168. doi: 10.1016/j.aquaculture.2020.735168
Yakimoff W., Gousseff W. (1936). Eimeria syngnathi n. sp., a new coccidium from the great pipe fish (Syngnathus nigrolineatus). J. R Microsc Soc. 56 (4), 376.
Yang Q., Zheng L., Huang Z., Lin Q., Lu Z., Zhou C. (2017). Identification and characterization of pathogen vibrio rotiferianus, a pathogen isolated from hippocampus erectus with tail-rot disease. JFSC. 24 (05), 1131–1140. doi: 10.3724/SP.J.1118.2017.16310
Zenclussen A. C., Gerlof K., Zenclussen M. L., Ritschel S., Zambon Bertoja A., Fest S., et al. (2006). Regulatory T cells induce a privileged tolerant microenvironment at the fetal-maternal interface. Eur. J. Immunol. 36 (1), 82–94. doi: 10.1002/eji.200535428
Zhang Y., Qin G., Lin J., Lin Q. (2015). Growth, survivorship, air-bubble disease, and attachment of feeble juvenile seahorses, hippocampus kuda (Bleeker, 1852). J. World Aquac Soc 46 (3), 292–300. doi: 10.1111/jwas.12193
Zhang Y.-H., Ravi V., Qin G., Dai H., Zhang H.-X., Han F.-M., et al. (2020). Comparative genomics reveal shared genomic changes in syngnathid fishes and signatures of genetic convergence with placental mammals. Natl. Sci. Rev. 7 (6), 964–977. doi: 10.1093/nsr/nwaa002
Zhang B., Zhang H., Qin G., Liu Y., Han X., Yin J., et al. (2019). TLR2 gene in seahorse brood pouch plays key functional roles in LPS-induced antibacterial responses. J. Fish Dis. 42 (7), 1085–1089. doi: 10.1111/jfd.13006
Keywords: syngnathidae, immunity, male pregnancy, review, seahorse, pipefish, genome, evolution
Citation: Parker J, Dubin A and Roth O (2023) Genome rearrangements, male pregnancy and immunological tolerance – the curious case of the syngnathid immune system. Front. Mar. Sci. 10:1099231. doi: 10.3389/fmars.2023.1099231
Received: 15 November 2022; Accepted: 13 January 2023;
Published: 26 January 2023.
Edited by:
Antonio Figueras, Spanish National Research Council (CSIC), SpainReviewed by:
Patricia Pereiro, Spanish National Research Council (CSIC), SpainMagalí Rey-Campos, Institute of Marine Research (CSIC), Spain
Copyright © 2023 Parker, Dubin and Roth. This is an open-access article distributed under the terms of the Creative Commons Attribution License (CC BY). The use, distribution or reproduction in other forums is permitted, provided the original author(s) and the copyright owner(s) are credited and that the original publication in this journal is cited, in accordance with accepted academic practice. No use, distribution or reproduction is permitted which does not comply with these terms.
*Correspondence: Jamie Parker, anBhcmtlckB6b29sb2dpZS51bmkta2llbC5kZQ==