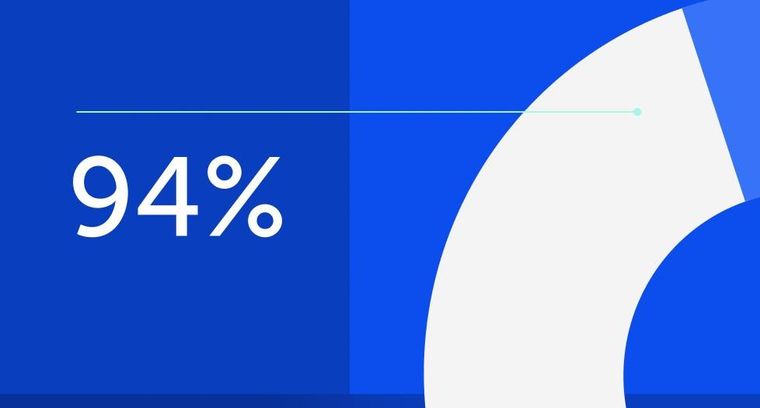
94% of researchers rate our articles as excellent or good
Learn more about the work of our research integrity team to safeguard the quality of each article we publish.
Find out more
ORIGINAL RESEARCH article
Front. Mar. Sci., 18 January 2023
Sec. Marine Molecular Biology and Ecology
Volume 10 - 2023 | https://doi.org/10.3389/fmars.2023.1087411
This article is part of the Research TopicDeep-sea Chemosynthetic Ecosystems: Living in Extreme EnvironmentsView all 13 articles
Introduction: Marine metal contamination caused by deep-sea mining activities has elicited great concern from both social and scientific communities. Among the various metals deep-sea organisms might encounter, cadmium (Cd) is a widely detected metal that in very small amounts is nonetheless capable of severe toxicity. Yet due to both remoteness and technical challenges, insights into the effects of metal exposure resulting from mining activities upon deep-sea organisms are limited.
Methods: Here, we investigated Cd’s toxicological effects on deep-sea mussels of Gigantidas platifrons exposed to 100 or 1000 g/L of Cd for 7 days; an integrated approach was used that incorporated proteomics and metabolomics along with traditional approaches (metal concentrations, metal subcellular distribution, and anti-oxidative and immune-related biochemical indexes).
Results and Discussion: Results showed that Cd exposure caused significant Cd’s accumulation in mussel gills and redistribution of Cd among subcellular compartments, with cellular debris being the primary binding site. Although anti-oxidative enzymes activities (superoxide dismutase and catalase) were not significantly altered in mussel gills of both exposed groups, the markedly increased level of glutathione S-transferase detected via proteomic technique clearly evinced that deep-sea mussels suffered from oxidative stress under Cd exposure. Besides, altered activities of acid phosphatase and alkaline phosphatase assayed by traditional methods along with the predominant presence of largely altered immune-related proteins detected by proteomic data strongly revealed an immune response of deep-sea mussels elicited by Cd. In addition, results of proteomics combined with those of non-targeted metabolomics demonstrated that Cd could exert toxicity by disrupting cytoskeleton structure, ion homeostasis, and primary metabolisms of energy, lipid, and nucleotide in deep-sea mussels. As demonstrated in this study, proteomics and metabolomics can be used in tandem to provide valuable insights into the molecular mechanisms of deep-sea organisms’ response to Cd exposure and for helping to discover potential biomarkers for application during deep-sea mining assessments.
The deep-sea floor harbors a wealthy of valuable mineral resources which are necessary components of a variety of low-carbon energy technologies, such as solar panels, wind turbines, and geothermal capture systems (Carlsson and Gade, 1986). As mineral resources on land are gradually depleted and deep-sea equipment and technology are further developed, deep-sea mineral resources have emerged as a new target for extraction and acquisition by humans, with deep-sea mining now entering a highly active period. The contract numbers for exploring deep-sea mineral resources has soared, from just seven in 2010 to thirty-one in 2021, of which seven mining contracts are for the exploration of hydrothermal areas (ISA).
However, deep-sea mining is a large-scale human activity. In the process of transporting metal minerals from hundreds or even thousands of meters of seabed up to the water surface, a mining operation will inevitably result in the release of toxic substances including copper, cadmium, zinc, lead, and iron. When excessive amounts of these metals are absorbed by local organisms, it can cause acute or chronic damage to deep-sea species, inhibiting their growth and reproduction, affecting the local food chain, and eventually leading to ecosystem shutdown (Boschen et al., 2013; Hauton et al., 2017; Martins et al., 2017). Hindered by both its remoteness and technical challenges, the deep sea is the least explored biome on the planet. As a result, there is very little knowledge of how deep-sea mining impacts deep-sea organisms in terms of their physiology and population dynamics. To establish guidelines and frameworks for sustainable mining practices as soon as possible, it is imperative that metal toxicological research of deep-sea organisms be conducted, which provide a timely empirical basis that can also inform theory for the establishment of sensitive biomarkers for the monitoring of deep-sea mining activities.
The deep-sea bivalve Gigantidas platifrons is a cosmopolitan mussel that can be found in most deep-sea chemosynthetic ecosystems of the West Pacific Ocean. As stationary filter feeders, individuals of this species live in the benthic environment, where pollutants are deposited and accumulated (Zhou et al., 2020; Zhou et al., 2021). These mussels are very sensitive to environmental fluctuations and can serve as sentinels to monitor the deep-sea environment (Zhou et al., 2020; Zhou et al., 2021). Among the various metals deep-sea life may come into contact with, Cd is a nonessential element in deep-sea mussels’ physiology, which can be readily absorbed, transported, and accumulated in their bodies (Company et al., 2010; Zhou et al., 2021). Furthermore, Cd does not biodegrade and can persist in organisms for a long time, causing serious toxic effects via trace amounts on organisms at their physiological, cellular, subcellular and molecular levels. Experimental studies with deep-sea organisms under metal exposure have shown that Cd could cause general cellular injury, impair lysosomal and plasma membrane integrity, induce oxidative stress and overproduction of reactive oxygen species (ROS), disrupt cellular osmoregulation as well as disordering core metabolic pathways of amino acids, carbohydrates, and lipids (Company et al., 2019; Zhou et al., 2021). In deep-sea hydrothermal ecosystems, Cd levels tend to be high, capable of reaching 1.5 μM and 3950 ppm in hydrothermal fluids and metallic mineral deposits, respectively (Zeng, 2011).
Previously, researchers have investigated the toxic effects of deep-sea mining upon deep-sea fauna by using shallow-water taxa as proxies for deep-sea taxa (Brown et al., 2017b; Mestre et al., 2019; Pinheiro et al., 2021). However, such studies are controversial because they ignore the complexity of the deep-sea environment and species differences between organisms in shallow and deep water habitats (Brown et al., 2017a). A recent study of deep-sea organisms exposed to metals found that the expression of some traditional metal-related biochemical indexes (e.g., metallothioneins) or metabolites (e.g., D-allose) were not significantly induced or exhibited a pattern opposite to that found in shallow-water (Zhou et al., 2021), implying that deep-sea organisms might undergo different biochemical and molecular responses when exposed to metals. Besides, when compared with the vast amount of toxicological research in costal organisms, corresponding research on deep-sea organisms far from comprehensive and limited to the responses of a known class of toxicity-responsive genes or particular enzyme activity (Company et al., 2010; Bougerol et al., 2015; Martins et al., 2017). The response mechanisms of deep-sea organisms induced by stress from different metals are not exactly the same, and they depend on a complex genomic regulatory network involving multiple genes and pathways (del Rio, 2016; Wong et al., 2015). Therefore, systematical toxicological work carried out using multiple approaches at once, to unravel these biological effects and responsive mechanisms, can truly reflect the ongoing and expected life processes in those parts of the deep sea incurring mining perturbations.
Recently, with dramatic advances in high-throughput molecular tools, ‘omic’ technologies including proteomics and metabolomics have been extensively used in toxicological studies of marine environments (Sanchez et al., 2011; Xu et al., 2019). Proteomics theoretically characterizes the entire protein pool at the organelle, cellular or tissue level, and is capable of detecting significantly different expressed proteins (DEPs) caused by exogenous factors in organisms (Tomanek, 2011; Campos et al., 2012). Meanwhile, metabolomics systematically examines the entire range of endogenous low-molecular weight metabolites (< 1000 Da) in biological samples (Ji et al., 2015). It offers the opportunity to compare the metabolites and then detect alterations in their expression profiles in organisms. When used in tandem, proteomics and metabolomics could provide plenty of information concerning the molecular responses of living organisms to contamination. In combination, these two ‘omic’ approaches would ideally complement each other, offering opportunities to decode novel molecular mechanisms involved in stress response, and being useful for discovering reliable biomarkers of metal exposure and its effects. To our best knowledge, however, no attempt has yet been made to study the toxic effects of metals on deep-sea organisms simultaneously at the protein and metabolite levels.
In this study, to gain a comprehensive understanding of the underlying toxicological effects associated with metal exposure in deep-sea organisms, the deep-sea mussel G. platifrons was selected as the model organism and exposed to cadmium (100 and 1000 μg/L) for 7 days, and the response of the treated deep-sea mussels then evaluated using both traditional toxicological methods (i.e., metal accumulation, metal subcellular distribution, biochemical indexes) and ‘omic’ techniques (proteomics and metabolomics). The objectives of this study were (i) to determine the subcellular distribution and biochemical response of G. platifrons gill tissue under Cd stress; (ii) to identify key proteins/metabolites that are differentially expressed in G. platifrons under Cd stress, and their involved essential pathways; (iii) to uncover the molecular mechanisms by which G. platifrons responds to Cd. This study will contribute to bridging a glaring knowledge gap regarding the potential impacts of deep-sea mining on deep-sea organisms, as well as identifying some important responsive candidate biomarkers associated Cd stress for the monitoring of deep-sea mining.
Mussels of G. platifrons were collected from the seabed of the South China Sea at seawater depths below 1119 m, by using the remotely operated vehicle Faxian (ROV Faxian), it mounted to the research vessel Kexue. ROV Faxian brought the mussels to the sea surface after first placing them in an insulated container. Once aboard, mussels were immediately transferred to a specially designed aquarium system, used for culturing deep-sea organisms. In this system, mussels were cultured in surrounding seawater at 4°C with a pressure of 1 atm. Water was half-renewed daily and any dead mussels were immediately discarded. Mussels received methane gas twice a day for 30 min, as a food and energy source.
Following acclimatization period of 48 hours, thirty mussels (93.2 ± 5.65 mm in shell length) were randomly placed in the three high-density polypropylene tanks (10 mussels per tank; rearing density: one mussel per liter of seawater). Mussels were exposed to nominal 100 μg/L or 1000 μg/L of Cd for 7 days, with clean filtered seawater serving as a control. The exposure concentrations of Cd chosen in this study reflect those in deep-sea environments (Zeng, 2011). Cadmium was prepared with CdCl2•2.5H2O (Sigma-Aldrich, analytical grade). The exposed concentrations were environmentally relevant and reported for hydrothermal systems (Zeng, 2011). The filtered seawater and exposed metal solution were renewed thoroughly on a daily basis. During this exposure experiment, other conditions were consistent with those of the acclimatization period, and no mussel mortality was observed.
All of thirty mussels were sacrificed after 7 days of exposure. Immediately after dissecting each with plastic tools, the mussel gills were flash frozen in liquid nitrogen, and then stored at –80°C for further analysis. Mussel gill of each individual was separated into five sets for their metal contents’ determination, metal subcellular distribution, biochemical analysis, and metabolic and proteomic assessments.
To quantify the gills’ Cd accumulation, inductively coupled plasma mass spectrometry was used as previously described (ICP-MS, Thermo-Scientific iCAPQc, Bremen, Germany) (Zhou et al., 2020). A detailed description of these procedures can be found in the Supplementary Material (S1.1).
Metal subcellular distribution in mussel gills was determined according to a well-established protocol reported in several studies (Wallace and Luoma, 2003; Pan and Wang, 2008; Yu et al., 2013; Ma and Wang, 2020). Briefly, each gill tissue sample was individually homogenized in a freshly prepared 30 mM Tris-NaCl buffer (pH 8.0, 0.15 M NaCl, 5 mM 2-mercaptoehanol, with 0.1 mM of freshly prepared phenylmethylsufonyl fluoride) using an Ultra-Turrax homogenizer (Jing Xin, China) for 30 sec. The homogenized tissue was first fractionated by centrifugation at 1450× g for 15 min. The collected pellets were digested in 1 N NaOH. After the mixture had been heated at 80°C for 10 min in a water bath, a second centrifugation at 5000× g for 10 min was carried out. Next, the metal-rich granule fraction (MRG) was obtained from the pellets, while cellular debris was obtained from the supernatant. A third centrifugation at 100 000× g for 60 min was performed on the supernatant from the first centrifugation. From this, the resulting pellet resulting was treated as the organelle fraction, and a 10-min water bath at 80°C was used to heat the ensuing supernatant. After doing another centrifugation at 50 000× g for 30 min, the heat-sensitive protein fraction (HSPF) was obtained from the supernatant, while metallothionein-like protein fraction (MTLP) was derived from the pellet. All procedures were conducted at 4°C unless otherwise stated. The experiment was conducted with blank samples lacking any tissue addition. Following the freeze-drying of each fraction, the fractions were digested with 2 mL of 15.6 M HNO3 at 80°C for 48 h. The obtained digested mixture was then diluted to 5 mL with deionized water and the Cd concentration in each fraction likewise measured according to the above procedure. The percentages of Cd metal in each fraction were defined as the metal subcellular distributions.
Six biochemical indexes—acid phosphatase (ACP), alkaline phosphatase (AKP), superoxide dismutase (SOD), catalas (CAT), reduced glutathione (GSH) and lipid peroxidation (LPO)—were measured in the mussel gill samples. All these biochemical indexes were quantified using commercial kits from the Nanjing Jiancheng Bioengineering Institute (China). AKP and ACP activity were determined according to the method King (1965). SOD was measured by the method of McCord and Fridovich (1969). CAT activity was quantified by the method of Goth (1991). Reduced GSH was determined following the method of Jollow et al. (1974). LPO was assessed by the method of Korobeĭnikova (1989). A detailed description of these procedures can be found in the Supplementary Material (S1.2).
Three mussel gill tissues were pooled into one biological replicate, and three biological replicates were analyzed for each group. This analysis using 4D Label free proteomics was conducted in collaboration with Jingjie PTM BioLabs (Hangzhou, China) (Ding et al., 2022). The main procedures included protein extraction, protein alkylation, trypsin digestion, LC-MS/MS analysis, and the bioinformatics analysis. Briefly, a lysis buffer containing 8 M urea and 1% protease inhibitor cocktail was used to extract the mussel gill proteins, which were then digested with trypsin. The tryptic peptide was dissolved in mobile phase A of liquid chromatography, and separated by a NanoElute ultra-high-performance liquid chromatography system (Bruker, Germany). Protein profiles were generated by timsTOF Pro (Bruker Daltonics) mass spectrometry after separation with the ultra-performance liquid system. The resulting MS/MS data was analyzed using the MaxQuant search engine (v1.6.15.0). Tandem mass spectra were searched against deep-sea mussel G. platifrons genome data concatenated with the reverse decoy database. The reference genome of G. platifrons employed in this study was originally published by Sun et al. (2017) and updated by Wang et al. (GenBank accession no. JAOEFJ000000000, unpublished data). The FDR (false discovery rate) was adjusted to < 1%.
Proteins with a fold-change > 1.2 or < 0.83 and a P < 0.05 were designated as being significantly differentially expressed. These proteins were classified and grouped using Gene Ontology (GO) and KEGG databases. A detailed description of these procedures can be found in the Supplementary Material (S1.3).
Metabolites in mussel gills were extracted as previously described (Zhou et al., 2021). After that, an ultrahigh performance liquid chromatography instrument (UHPLC) (1290 Infinity LC, Agilent Technologies, Santa Clara, CA, USA), equipped with a quadruple time-of-flight mass spectrometer (AB Sciex TripleTOF 6600), was used to generate the untargeted metabolic profiles. This analysis was supported by Shanghai Applied Protein Technology Co., Ltd, APTBIO. The procedures involved are described in detail in the Supplementary Material (S1.4).
The statistical analysis was implemented in SPSS v19 software for Windows (IBM, Armonk, NY, USA). For biochemical indices and metal concentrations, their means and standard deviations were calculated. Before their formal statistical analysis, data of fitted residuals were checked for homogeneity of variance and normality. Analyses of variance (ANOVA) and Kruskal–Wallis tests were applied to parametric and nonparametric variables, respectively. When a significant difference among the three groups was detected, or Tukey’s range test or Mann–Whitney U tests were respectively applied in pairwise manner to identify which treatment or control groups differed from each other.
Accumulated Cd concentrations and the corresponding subcellular Cd distribution in mussel gills are depicted in Figure 1. Mussel gills can significantly accumulate Cd from the surrounding environment, with the higher Cd exposure concentration resulting in a higher Cd concentration in mussel gills (Figure 1A). Cd concentrations within the subcellular fractions (i.e., MTLP, organelle, HSPF, MRG, cellular debris) of the mussel gills were also significantly impacted by exposure concentration (Figure 1B). In the control group, HSPF harbored the most Cd. As the exposure concentration increased, Cd was transferred to MTLP, organelle, and cellular debris, the last being where Cd was predominantly stored in deep-sea mussels.
Figure 1 Mean metal concentrations (mg/kg) and subcellular distribution of Cd in gills of the deep-sea mussel Gigantidas platifrons after 7 days of exposure to 100 or 1000 μg/L Cd (n = 10 per group, mean ± SD). Values followed by the same letter are not statistically different (P > 0.05) between the treatment groups. Pie charts show subcellular distribution of Cd in the gills of G. platifrons. L-Cd: 100 μg/L Cd; H-Cd: 1000 μg/L Cd; MTLP: metallothionein-like protein; MRG: metal-rich granules; HSPF: heat-sensitive protein.
Most biochemical parameters analyzed in this study were affected by the exposure concentrations of Cd (Figure 2). The activity of ACP decreased as the concentration of Cd increased. Conversely, AKP activity and the levels of GSH and LPO all increased with an increased Cd concentration. The activity of SOD or CAT did not differ significantly among the treatments and control group. The ACP activity and LPO level were statistically similar in both low- and high-dose group. The GSH level only raised in mussel gills of the high-dose group, being approximately three times higher that of the control group.
Figure 2 The activity of acid phosphatase (ACP), alkaline phosphatase (AKP), superoxide dismutase (SOD) and catalase (CAT), and the levels of glutathione (GSH), and lipid peroxidation (LPO) in the gills of Gigantidas platifrons after 7 days of exposure to 100 or 1000 μg/L Cd (n = 10 per group, mean ± SD). Values followed by the same letter are not statistically different (P > 0.05) between the treatment groups.
A total of 5260 quantifiable proteins were identified in the deep-sea mussel gills (Supplementary Materials S2.1). Of those, in the low dose-Cd exposure group, 162 were significantly differentially expressed proteins (DEPs) consisting of 87 up-regulated (53.7%) and 75 down-regulated (46.3%) induced DEPs; in the high dose-Cd exposure group 222 DEPs were found induced, these consisting of 144 up-regulated (64.9%) and 78 down-regulated (35.1%) DEPs (Figure 3A). Venn diagram analysis showed that 47 DEPs were altered in common (30 down-regulated and 17 up-regulated) by the two Cd-exposure treatments (Figure 3B). Detailed information on all significantly regulated proteins is provided in Table 1 and Supplementary Materials Table S1.
Figure 3 Quantitative proteomics data. (A) Significantly up- and down-regulated proteins in mussel gills of low- and high-dose groups compared with the control group. (B) Venn diagram showing the number of DEPs (differently expressed proteins) uniquely and commonly altered in the groups exposed to 100 and 1000 μg/L of Cd. (C) The GO (Gene Ontology) classification of proteins that were expressed in 100 and 1000 μg/L groups indicated by pink and blue bars, respectively.
Table 1 The key differentially expressed proteins (DEPs) responsive to cadmium in deep-sea mussel gills.
The DEPs were classified into three categories based on their GO annotations: biological process (BP), molecular function (MF), and cellular component (CC). Under the BP category, most DEPs were involved in cellular process, biological regulation, and metabolic process in both treatment groups. In the MF category, most DEPs detected were involved in catalytic activity and binding in both treatment groups. In the CC category, most DEPs were located in cell and intracellular, in both treatment groups (Figure 3C). A detailed description of the GO enrichment results for the DEPs is provided in Table S2 of the Supplementary Materials.
The most representative KEGG pathways for the down- and up-regulated DEPs are shown in Figure 4. In the low-dose Cd exposed group, O-glycan biosynthesis, apoptosis, the wnt signaling pathway, and protein processing in the endoplasmic reticulum were the most statistically significant pathways for the up-regulated DEPs. For the down-regulated DEPs, the most significant pathway was galactose metabolism. In the high dose-Cd exposure group, both mismatch repair and spliceosome were significantly enriched for the up-regulated DEPs; vitamin digestion and absorption, linoleic acid metabolism, glycolysis/gluconeogenesis and necroptosis pathway were enriched for the down-regulated DEPs.
Figure 4 KEGG pathway enrichment analyses of up and low-regulated differentially expressed proteins (DEPs) detected in gill mussels of Gigantidas platifrons treated with LCd (100 µg/L Cd) or HCd (1000 µg/L Cd). (A) up-regulated DEPs, LCd; (B) up-regulated DEPs, HCd; (C) down-regulated DEPs, LCd; (D) down-regulated DEPs, HCd.
Compared with the control group, 21 and 68 metabolic features were significantly altered in the low dose- and high dose-Cd exposure groups, respectively (Table 2). These differential metabolic profiles demonstrated that deep-sea mussels relied on differing metabolic strategies when exposed to Cd at different exposure concentrations. Importantly, only eight metabolites in total were commonly altered by both Cd exposure treatments. The metabolite classes identified included amino acids, carbohydrates, nucleotides, and lipids, as well as others. KEGG enrichment analysis demonstrated seven pathways (ABC transporters, protein digestion and absorption, aminoacyl-tRNA biosynthesis, valine, leucine and isoleucine biosynthesis, choline metabolism in cancer, glycine, serine and threonine metabolism, and mineral absorption) were commonly enriched when comparing each of the Cd-exposed group with the control group (Figure 3S). Specially, pathways of lysine degradation, fructose and mannose metabolism, proximal tubule bicarbonate reclamation were uniquely enriched in low-dose group, and pathways of purine metabolism, glucosinolate biosynthesis, mTOR signaling pathway, amino sugar and nucleotide sugar metabolism were enriched in high-dose group (Figure 3S).
Table 2 Significantly expressed metabolites in the studied deep-sea mussel in response to a low and high dose of cadmium exposure (Note: Symbol ↑ indicates the metabolite is upregulated;Symbol ↓ indicates the metabolite is downregulated).
The accumulation of Cd in deep-sea mussels increased with increasing exposure concentrations, indicating that deep-sea mussels are robust indicator organisms of environmental changes caused by metal pollution (Martins et al., 2017; Zhou et al., 2021). The subcellular compartmentalization of metals reflects dynamic processes that occur during metal accumulation and provides insights into temporary metal-binding ligands and the detoxification process in organisms (Wallace et al., 2003; Pan and Wang, 2008). As the exposure concentrations increased, the proportion of Cd in its major binding site (HSPF) significantly decreased, whereas Cd’s proportions in other fractions (mainly cellular debris) increased correspondingly. A similar pattern was also found in the scallop Chlamys nobilis under Cd or Zn exposure (Pan and Wang, 2008). This phenomenon is called the spillover effect, namely because once the major binding pool is saturated and its binding capacity now exceeded, metal(s) may subsequently become associated with other fractions (Pan and Wang, 2008). Cellular debris contains tissue fragments, cell membranes, and other cellular components without any known function. Under Cd exposure, newly accumulated Cd in deep-sea mussel gills tended to bind to less sensitive fractions in cellular debris, preventing it from binding to sensitive fractions in the cell and thus decreasing Cd’s toxicity (Lucu and Obersnel, 1996). Interestingly, there was a very small proportion of Cd (< 9%) eliminated into MTLP, whether in the control or either exposure group, suggesting that MTLP could play a minor role in Cd detoxification. This result is in line with previous findings that MTLP is probably not relied upon for a major metal detoxification strategy in deep-sea bivalves (Hardivillier et al., 2004; Zhou et al., 2021).
Apart from detoxification by binding with cellular debris other than MTLPs, deep-sea mussels also can express detoxification enzymes for adaption and survival under Cd stress. In this study, exposure to Cd resulted in the significant up-regulation of glutathione transferases (GSTs) in deep-sea mussel gills. The GSTs are phase II detoxification enzymes responsible for catalyzing the conjugation of glutathione into electrophilic compounds that eventually lead to their excretion (McDonagh and Sheehan, 2006). GSTs also play a role in defense against oxidative stress by metabolizing reactive products formed during lipid peroxidation (Canesi et al., 1999). Furthermore, another detoxification enzyme, cytochrome P450 2C31 (CYP450[2C31]), was found uniquely down-regulated in deep-sea mussels of high dose-Cd exposure group. In a toxicological study with clam Mactra chinensis exposed to Cd, CYP450 (2C31) was inhibited by a low Cd concentration (0.69 mg/L), yet induced by high Cd exposure concentrations (1.38 and 2.76 mg/L) (Zhang et al., 2016). The current results bring forth new and relevant information regarding the metal detoxification response of deep-sea mussels.
Oxidative stress is a major mechanism underpinning Cd toxicity, because its overload could disrupt the redox balance at a cellular scale and stimulate the generation of ROS (Valko et al., 2005). To counter oxidative stress, an antioxidant cascade that includes SOD, CAT, and GSH could be invoked. Among these antioxidants, SOD is responsible for the disproportionation of superoxide anions into hydrogen peroxide and dioxygen, meanwhile CAT can act as a scavenger of hydrogen peroxide. This SOD-CAT system provides the first line of defense against Cd toxicity (Li et al., 2011). However, in the current study, both SOD and CAT activity were inactivated in either Cd exposure treatment, implying that they might not be suitable for use as sensitive biomarkers in deep-sea mussels during the monitoring of Cd pollution. Altered GSH levels could also be serve as a biomarker for oxidative stress (Valko et al., 2005). Actually, the patterns of change in GSH tend to differ under different experimental conditions: GSH was reportedly depleted substantially during acute Cd exposure while being markedly augmented following chronic Cd exposure (Liu et al., 2009). Our results show that exposure to a low dose of Cd slightly raised the GSH in deep-sea mussels whereas a high dose of it significantly induced GSH in them. Besides, as a major consequence of Cd-induced oxidative stress (Liu et al., 2009), LPO was increased exclusively in deep-sea mussels belonging to the high-dose group. The altered GSH and increased LPO together suggest oxidative stress in mussels of the high-dose group. According to the biochemical data, a low dose of Cd exposure seems unlikely to have caused overt changes to the mussel’s cellular oxidative status and lipid peroxidation. Nevertheless, the markedly augmented level of GST detected via the proteomic technique clearly evidenced that these deep-sea mussels in the low dose-Cd exposure group also incurred oxidative stress. Therefore, the proteome can convey a more complete picture of the biochemical changes that deep-sea mussels undergo when faced with Cd stress, and it is more sensitive than traditional approaches for discovering novel biomarkers.
From the proteomic profiles, Cd exposure altered 11 proteins that are linked to stress responses. Among these proteins, major vault protein (MVP) was uniquely induced in deep-sea mussel gills of low-dose group. MVP is the main component of the ubiquitous, vault particles that exceed the size of ribosomes. Although the exact role of MVP remains unclear, research has shown that vault complexes are involved in cellular responses to environmental toxins by directly binding and excreting conjugated metabolites, thereby precluding a metal’s accumulation and toxic effects (Berger et al., 2009). In addition, because MVP can play a strong regulatory role in apoptosis by binding to several stress-induced effectors of signaling cascades, it has been proposed as a candidate target for modulating apoptosis resistance in aging modulation and cancer treatments (Lara et al., 2011). The up-regulated expression of MVP in mussels exposed to Cd’s low dose suggested an anti-apoptotic response to offset potential toxic effects of Cd. Expression of seven stress-related proteins belonging to the class of heat shock protein 70 (HSP70) family were significantly altered in deep-sea mussel gills of both Cd-exposure groups. HSP70 is a group of highly conserved and abundant proteins that help organisms deal with abiotic stressors (heat shock, salinity change, acidification and contaminants) by refolding, sorting, translocating, and degrading deformed proteins, as well as being co-chaperones and regulating the biologically active form of other proteins (Kim and Schöffl, 2002; Chaudhary et al., 2019; Yusof et al., 2022). A field investigation of deep-sea mussel Bathymodiolus azoricus from Atlantic hydrothermal vents confirmed that that this species could express and induce a mixture of constitutive heat-shock protein isoforms, ranging from 64 and 76 kDa, enabling it to survive despite toxic vent fluids (Pruski and Dixon, 2007). Accordingly, HSP70 can be set up as a general stress biomarker in deep-sea mussels to monitor deep-sea environmental changes caused by Cd leakage during mining activities.
Eighteen proteins associated with cytoskeleton were altered by Cd exposure. In constituting the system of fibrillar structures in the cytoplasm of eukaryotic cells, the cytoskeleton is primarily composed of three types of fibrils: microtubules, actin filaments, and intermediate filaments. These fibrils could serve as ‘weak’ elements during episodes of metal stress, inducing the expression of several molecular chaperones, including small HSP and HSP70, to protect the cytoskeleton (Liang and MacRae, 1997). Besides, there is compelling evidence that cytoskeletal proteins could be among the first targets of oxidative stress and that they participate in the homeostasis of oxidative stress (Dalle-Donne et al., 2001). Given that cytoskeletal proteins are crucial for a cell’s structure and intracellular organization, any disruption in the architecture of one of the three cytoskeletal networks is liable to cause adverse effects to cells’ integrity and functioning (Fletcher and Mullins, 2010). Cytoskeletal injuries in bivalves induced by metal pollution have been repeatedly confirmed (Gomes et al., 2013; Xu et al., 2016). And these cytoskeletal-related DEPs are generally contain actins, tubulins, and myosins in marine bivalves (Xu et al., 2019), a finding consist with our study’s results. Consequently, the significantly altered cytoskeletal proteins demonstrate that oxidative stress and subsequent disturbed cytoskeleton and cell structures can be induced by Cd in deep-sea mussels.
The immune system of deep-sea mussels was also affected by their exposure to Cd, as evinced by the altered ACP and AKP activity as well as the thirty regulated proteins (17 DEPs in the low-dose group, 20 DEPs in the high-dose group) involved in the GO term “immune system process”. Both ACP and AKP are ubiquitous enzymes in organisms that hydrolyze phosphate-containing compounds and therefore can serve as effective biomarkers of immune responses to environmental contamination (Mazorra et al., 2002). AKP activity is reliably indicative of cell membrane’s integrity and is involved in the osmoregulation (Lovett et al., 1994), immune defense (Revel et al., 2019), and calcification process of bivalves (Hohagen and Jackson, 2013). Any perturbation (i.e., altered temperature, increased exposure to metals, microplastics exposure) to the membrane properties could result in AKP’s altered activity (Jiang et al., 2012; Huang et al., 2021; Sun et al., 2021). In the present study, a significant increase in AKP activity resulted from increasing the Cd exposure concentrations, this implying a strong immune response of mussels under Cd exposure to resist metal toxicity, or disturbance in osmoregulation from disrupted membrane integrity. ACP has been proven to be associated with lysosomal functionality (Pampanin et al., 2002). Upon entering body, toxic chemicals such as metals can be sequestered within the lysosome, causing increased toxicity and eventually affecting the permeability, integrity or structure of the lysosome (Moore, 1994). Under certain circumstances when lysosomes become overburdened, hydrolases burst out of their leaky membranes and are released into the extracellular environment, with the possibility of cause cellular injury or even death (Kohler et al., 2002). Hence, the decreased ACP activity in mussels of the high-dose group might due to enzyme escape from damaged lysosomes (Jiang et al., 2012).
When studying the effects of metals on the immune functioning of marine bivalves, researchers have mainly examined total and differential hemocyte counts and phagocytic activity (Hoher et al., 2012). By using proteomics, we discerned the predominant presence of largely altered immune-related proteins in deep-sea mussel gills under Cd stress. These significantly expressed proteins have never been reported before in deep-sea invertebrates in response to metal exposure. For instance, interleukin enhancer-binding factor 2 (ILF2) homolog, stimulator of interferon genes (STING) protein 2, and E3 ubiquitin-protein ligase TRIM56-like were the three of the most prominently regulated proteins in deep-sea mussels when exposed to either Cd treatment, indicating that Cd can disturb the immune homeostasis in deep-sea mussels. As one of the six categories of cytokines that are inducible immune regulatory proteins, interleukin could play pivotal roles in modulating cell communication, growth, development, and differentiation to maintain immune system homeostasis (Yang et al., 2022). Although there are few reports of interleukin and its analogues in invertebrates, ILF has been reported to exist in invertebrates where it functions as regulator in some innate immune responses by affecting the Toll signaling pathway and cell apoptosis (Venier et al., 2011; Yang et al., 2022). As for STING, it is well known for inducing the generation of interferon and proinflammatory cytokines. Being an endoplasmic reticulum membrane protein anchored by several transmembrane domains at its N-terminal region, STING can function as a cGMP-AMP synthase downstream signal adapter in the innate immune cytosolic DNA-sensing pathway (McKnight et al., 2020; Zhang et al., 2020). Concerning E3 ubiquitin-protein ligase TRIM56, it can promote the function of STING by binding to the latter’s C-terminal domain and serve as a powerful modulator of innate immunity (Tsuchida et al., 2010). Overall, the significant alterations of the aforementioned biochemical indexes and molecules strongly suggest that multiple mechanisms are involved in mobilizing the immune response against Cd stress in the studied mussel species.
Metals can impair energy homeostasis in marine organisms because the latter require more energy for mounting one or more stress responses, detoxification steps, or damage repair mechanisms (Sokolova et al., 2012). Glycolysis is the process of decomposing glucose into pyruvate and lactate, leading to the generation of ATP (Satyanarayana, 2021). Herein, five glycolysis-related proteins (phosphoglucomutase-1, enolase 4-like isoform X4, aldose 1-epimerase isoform X1, cinnamyl alcohol dehydrogenase 8-like isoform X2, and phosphoenolpyruvate carboxykinase) were found to be significantly changed in both Cd-exposure groups. Of them, four proteins were down-regulated significantly, suggesting inhibition of glycolysis in deep-sea mussels from exposure to low or high doses of Cd. Concurrently, metabolomics data revealed that all metabolites (α-D-glucose, β-D-fructose 6-phosphate, D-glucose 6-phosphate) associated with glycolysis were down-regulated, which further confirmed that Cd exposure inhibited glycolysis in deep-sea mussels, as noted above. Previous research with the sea cucumber Apostichopus japonicas revealed that its glycolysis could be promoted in the initial phase of exposure to Cd, but then became inhibited as the duration of metal stress was prolonged. Inhibition effects tend to occur earlier if the exposure concentrations are higher (Li et al., 2016). In addition, we also detected isocitrate dehydrogenase (IDH) as commonly down-regulated in both Cd-exposure groups. IDH is an indispensable enzyme that regulates the citric acid cycle (TCA), catalyzing the conversion of isocitrate to oxalosuccinate and then to α-ketoglutarate (Satyanarayana, 2021). Thus the decreased IDH and glycolysis-associated proteins together suggests that Cd toxicity is able to impair the energy metabolism in deep-sea mussels.
Notably, we found disparate effects of Cd on the oxidative phosphorylation of deep-sea mussels in the different treatment groups. Linked to the mitochondrial electron transport chain (ETC), oxidative phosphorylation is the major source of ATP production that takes place in the mitochondria of aerobic organisms. In the low-dose group, the NADH dehydrogenase [ubiquinone] 1 alpha subcomplex subunit 5 (NDUFA5), which is responsible for converting NAD from reduced form (NADH) into oxidized form (NAD+) during oxidative phosphorylation, was down-regulated relative to the control group. This reduction in NDUFA5 might have been caused by the reduced IDH activity in the TCA cycle. Metals can reportedly influence key enzymes such as IDH in the TCA cycle, leading to less generated NADPH and NADH, which can then negatively affect the normal function of ETC and ultimately lead to lower ATP production (Sun et al., 2022). In the high-dose group, however, we found that NDUFA5 was unaltered, but all the proteins associated with oxidative phosphorylation (mitochondrial ATP synthase subunit d precursor and V-type proton ATPase 16 kDa proteolipid subunit) were up-regulated and related to ATP synthase, implying an increased demand for ATP production in deep-sea mussels. Increased oxidative phosphorylation was reported in the rock fish Sebastes schlegelii under arsenic exposure and the mussel Mytilus galloprovincialis under nano-Ag exposure, both revealing a process of energetic re-adjustment to counteract the effects of arsenic toxicity (Gomes et al., 2013; Xu et al., 2019). In particular, to compensate for the diminished energy supply caused by the inhibition of glycolysis and the TCA, deep-sea mussels bolstered amino acid levels in their body to protect themselves from more damage caused by high-dose Cd exposure (Table 2). By serving as precursors for acetyl CoA and glucose synthesis, amino acids can also be degraded and utilized as energy sources in the body (Satyanarayana, 2021). Using metabolomics, a similar phenomenon has been extensively documented in marine bivalves when they are exposed to toxic chemicals (Viant et al., 2003; Zhou et al., 2021). Overall, our findings clearly show that Cd could alter mitochondrial metabolism and thereby risk impairment of ATP generation in deep-sea mussel gills. Collectively, these changes in energy metabolism coupled with a disordered cytoskeleton and lipid peroxidation would lead to stark physical changes in the membrane, affecting the respiratory, oxidative and calcium buffer capacity of mitochondria in gills of deep-sea mussels (Gomes et al., 2013).
As the principal concentrated fuel reserve of organisms, lipids play important roles in maintaining cellular structure and regulating cellular metabolism (Satyanarayana, 2021). Alterations to lipid metabolism reportedly serve as protective strategies of organisms in response to environmental stress (Liu et al., 2016; Meng et al., 2018; Zhou et al., 2021). A metal exposure study using blue mussel Mytilus edulis demonstrated that Cd could significantly disturb its lipid metabolism by changing lipid structures and the fatty acid composition in mussels (Fokina et al., 2013). Furthermore, a metal exposure experiment with the crab Chiromantes dehaani showed that Cd could disturb lipid metabolism by weakening the ability of lipid digestion, transportation and synthase, thereby affecting the normal growth and development of that organism (Liu et al., 2016). Here, two proteins associated with lipid metabolism, phosphoethanolamine N-methyltransferase 3 isoform X1 and monoglyceride lipase-like isoform X1, were found uniquely up-regulated in low-dose group. Monoglyceride lipase catalyzes the final step of phospholipid and triglyceride breakdown by hydrolyzing monoacylglycerols into fatty acids and glycerol molecules (Aschauer et al., 2016). These products can be used for energy production or synthetic reactions. Hence, the up-regulated monoglyceride lipase-like isoform X1 is further evidence of a disturbed energy homeostasis in deep-sea mussels caused by Cd exposure. In plants, phosphoethanolamine N-methyltransferase has been suggested as one key bottleneck for the de novo synthesis of phosphatidylcholine, the chief component of plasma membrane phospholipids in most eukaryotes, which has been shown to be up-regulated under abiotic stress conditions (Jost et al., 2009). We also observed augmented levels of glycerophosphocholine in the metabolic profiles of mussels in the low-dose group. Glycerophosphocholines are critical components of biological membranes and contribute to lipid bilayer asymmetry. Therefore, those up-regulated proteins and metabolites in mussels of the low dose-Cd exposure group might together reflect an alteration of biological membranes. In the high dose-Cd exposure group, the phospholipase B1 membrane-associated-like was down-regulated exclusively. Phospholipase B1 (PLB1) can hydrolyze ester linkages on membrane phospholipids and liberate fatty acids (Chayakulkeeree et al., 2011). It is involved in membrane homeostasis and remodeling in fungi (Siafakas et al., 2007) and could be modulated under various environmental and physiological conditions (Mukherjee et al., 2003). As gleaned from the metabolic profiles, fatty acids including adrenic acid, cis-9-palmitoleic acid, and valeric acid were up- or down-regulated. One explanation for those altered proteins and metabolites is that deep-sea mussels may modify their cell membrane’s composition in order to maintain/rebuild its stability and integrity. Another possibility is that Cd exposure resulted in damaged membranes of deep-sea mussels due to lipid peroxidation (He et al., 2020). Therefore, our results demonstrate that Cd has the potential to alter the membrane state of mussel gills, directly or indirectly, by disrupting membrane lipid biosynthesis and fatty acid composition.
Perturbed nucleotide metabolism is often reported in bivalves exposed to pollutants (Jones et al., 2008; Fasulo et al., 2012; Zhou et al., 2021). In the current study, a total of 21 nucleotides or their derivatives (Table 2) were found altered in mussels of high-dose group, with only two nucleotides changed in low-dose group, indicating dose-dependent toxicity effects of Cd. As structural components of DNA, RNA as well as many coenzymes, the availability of nucleotides underpins diverse metabolic reactions (Satyanarayana, 2021). Changed nucleotides profiles could be linked to neurotransmitter processes and cell signaling given that nucleosides (e.g., guanosine and adenosine), bases (e.g., guanine and adenine), and their metabolic products (e.g., xanthine and hypoxanthine) can be discharged into the extracellular space as intercellular signaling molecules (Rathbone et al., 1999). Apart from their function as signaling molecules, adenine and its analogues and inosine can also act as immunomodulatory molecules, exerting cellular protective and multiple anti-inflammatory effects in organisms (Haskó et al., 2000). That these purine metabolites increased in deep-sea mussels suggests their immune and inflammatory responses were enhanced under Cd exposure. Changing nucleotide profiles may also have contributed to peroxisome proliferation in rats (Ringeissen et al., 2003). As common oxidative organelles in eukaryotes, peroxisomes can function to protect the cell from toxic substances, contributing to the detoxification process of organisms. Thus, the increased levels of nucleotides identified in this study may point to elevated antioxidant enzyme activities and other catalytic activities during the metal detoxification process (Leonard et al., 2014).
Calcium binding proteins (CaBPs) have been identified as molecular targets for cadmium binding and cadmium toxicity. Due to the similarity between Cd2+ and Ca2+ in both structure and size, Cd2+ can not only enter cells via the Ca2+ uptake pathway but also compete with Ca2+ for the binding sites in CaBPs, in this way inhibiting the calcium influx of organisms (Choong et al., 2014), which can eventually affect cellular Ca2+ homeostasis. Here, CaBPs of the EF-Hand superfamily (EF-CaBPs) including calmodulin, calmodulin-like protein 4, EF-hand calcium-binding domain-containing protein 5-like isoform X1, EF-hand calcium-binding domain-containing protein 6-like isoform X2, and zinc finger ZZ-type and EF-hand domain-containing protein 1-like isoform X2, were all significantly altered in both Cd exposure groups. EF-CaBPs regulate all aspects of cellular calcium signaling pathways by binding to Ca2+, including Ca2+ gating control, modulation of the amplitude and duration of Ca2+ signals, and transduction of Ca2+ signals into biochemical responses (Girard et al., 2015). Therefore, the abnormal expression of EF-CaBPs indicated that Ca2+-triggered signaling could be involved in how deep-sea mussels overcome a metal challenge.
Deep-sea mussel gills are in direct contact with the ambient environment, and serve as an important organ for regulating cellular osmoregulation. We found that certain membrane transport proteins belonging to the solute carrier (SLC) family, such as sodium- and chloride-dependent taurine transporter-like in low-dose Cd-exposed group and sodium-dependent phosphate transport protein 2B-like isoform X1, phosphate carrier protein, and calcium-binding mitochondrial carrier protein SCaMC-2-like were significantly altered in high dose-Cd exposure group. Specifically, for these treated deep-sea mussels of high-dose group, the 16 kDa proteolipid (subunit c) of V-ATPase involved in translocating protons across lipid bilayers was significantly up-regulated. V-ATPase is capable of ensuring intracellular pH homeostasis, protecting cells against endogenous oxidative stress induced by divalent heavy metals, and inhibiting ROS production (Techo et al., 2020). Similar findings were found for the shrimp Litopenaeus vannamei under copper exposure (Guo et al., 2021). Meanwhile, the metabolic profiles of deep-sea mussels exposed to a high dose of Cd revealed that large amounts of amino acids were significantly induced. Previous research has shown that marine invertebrates such as deep-sea mussels can utilize intracellular amino acids to maintain their osmolality in balance with their surroundings (Zhou et al., 2021). Collectively then, the accumulated amino acids, dysregulated SLCs, and V-ATPase (subunit c) further suggest that Cd disturbed the ion exchange function of gills in the studied deep-sea mussel.
This study took a holistic perspective, incorporating both traditional approaches and ‘omic’ techniques to study the toxicological effects of Cd upon a keystone deep-sea bivalve Gigantidas platifrons. To do this, G. platifrons was exposed for 7 days to Cd at concentrations of 100 or 1000 μg/L, and metal accumulation, metal distribution in the subcellular compartments, anti-oxidative and immune-related biochemical parameters, and protein and metabolic profiles were evaluated as response variables. Under Cd exposure, the deep-sea mussel G. platifrons heavily accumulated Cd in its gills, where this Cd tended to bind to cellular debris other than MTLP, suggesting that MTLP might not be a major detoxification strategy in deep-sea bivalves. Parallel proteomic and metabolomics analyses revealed that the two doses of Cd evoked similar cellular responses, namely the elicitation of stress and immune responses and the disruption of the cytoskeleton structure, ion homeostasis, and primary metabolism processes in deep-sea mussels. A potential toxicological mechanism of Cd on deep-sea organisms is schematically illustrated in Figure 5. Overall, with deep-sea mining activities predicted to significantly impact marine ecosystems, the current results provide much-needed critical insights into how deep-sea organisms respond to metals as well as contributing to global information for identifying early responses of deep-sea organisms to metal pollution.
Figure 5 Potential mechanism schematic of Cd toxicology on deep-sea mussel G. platifrons according to KEGG (100μg/L Cd, A and 1000μg/L Cd, B). The altered proteins and metabolites were shown by marking the names in red (up-regulated) or blue (down-regulated) color. Abbreviations: ACP, acid phosphatase; AKP, alkaline phosphatase; IAP, baculoviral IAP repeat-containing protein 7-B isoform X3; STING, stimulator of interferon protein 2; AP-2, AP-2 complex subunit alpha-2-like; IEB, interleukin enhancer-binding factor 2 homolog; SVA, superkiller viralicidic activity 2-like 2; TRIM56, E3 ubiquitin-protein ligase TRIM56-like; NTF, nuclear transport factor 2-like; CTSL: shell fibrous prismatic cathepsin-like protein 1; Myosin, unconventional myosin-XVI-like isoform X10; IST1, IST1 homolog; PXN, leupaxin-like isoform X2; DNAH, dynein heavy chain 6, axonemal-like isoform X3; DNAI1, dynein intermediate chain 2, ciliary isoform X2; TUBB, tubulin beta chain-like; DNAH10, dynein heavy chain 10, axonemal-like isoform X2; DNAH5, dynein heavy chain 5, axonemal-like isoform X2; TCTP, translationally-controlled tumor protein homolog; ZZEF, zinc finger ZZ-type and EF-hand domain-containing protein 1-like isoform X2; TSC, tubulin-specific chaperone A; 3’-AMP, Adenosine 3’-AMP monophosphate; 2’,3’-cyclic AMP, Adenosine 2’,3’-cyclic monophosphate; D-Ribulose-5P, Ribulose 5’-phosphate; PRPP, phosphoribosyl diphosphate; ATP5H, mitochondrial ATP synthase subunit d precursor; ATPeV0C, V-type proton ATPase 16 kDa proteolipid subunit; IDH3, isocitrate dehydrogenase [NAD] subunit beta, mitochondrial-like; NDUFA5, NADH dehydrogenase [ubiquinone] 1 alpha subcomplex subunit 5; ADH, cinnamyl alcohol dehydrogenase 8-like isoform X2; GPC, Glycerophosphocholine; PEA, phosphoethanolamine; Cyt P450, cytochrome P450 2C31; GST, glutathione S-transferase; HSP70, heat-shock protein 70 family; CALM. Calmodulin; pgm, Phosphoglucomutase-1; GALM, aldose 1-epimerase isoform X1; ENO, enolase 4-like isoform X4; AACS, acetoacetyl-CoA synthetase-like; NMT, phosphoethanolamine N-methyltransferase 3 isoform X1; MGLL, monoglyceride lipase-like isoform X1; PCK, phosphoenolpyruvate carboxykinase, cytosolic [GTP] isoform X1; NMDA, glutamate receptor ionotropic; NPT2b, sodium-dependent phosphate transport protein 2B-like isoform X1; GPCR, 5-hydroxytryptamine receptor 7; PSMD4, 26S proteasome non-ATPase regulatory subunit 4-like; PSMF1, Proteasome inhibitor PI31 subunit; PSME3, proteasome activator complex subunit 3-like.
The datasets presented in this study can be found in online repositories. The names of the repository/repositories and accession number(s) can be found in the article/Supplementary Material.
LZ contributed to conception, methodology, software, writing of the study. ML, ZZ, HC, and CL carried out the experiments. MW, HW, HZ, and LC organized the database. CLL directed the manuscriptrevision. All authors contributed to the article and approved the submitted version.
This research was financially supported by the National Key R&DProgram of China (2022YFC2804003), the Strategic Priority Research Program of the Chinese Academy of Sciences (grant number XDB42020401), the National Natural Science Foundation of China (grant number 42276153, 41906103, 41906124,42030407), the Key Research Program of Frontier Sciences, CAS (ZDBS-LY-DQC032), the Open Research Project of National Major Science & Technology Infrastructure (RV KEXUE) (grant number NMSTI-KEXUE2017K01).
The authors thank to Lingling Sun for metal analyzing.
The authors declare that the research was conducted in the absence of any commercial or financial relationships that could be construed as a potential conflict of interest.
All claims expressed in this article are solely those of the authors and do not necessarily represent those of their affiliated organizations, or those of the publisher, the editors and the reviewers. Any product that may be evaluated in this article, or claim that may be made by its manufacturer, is not guaranteed or endorsed by the publisher.
The Supplementary Material for this article can be found online at: https://www.frontiersin.org/articles/10.3389/fmars.2023.1087411/full#supplementary-material
Aschauer P., Rengachari S., Lichtenegger J., Schittmayer M., Das K. M. P., Mayer N., et al. (2016). Crystal structure of the saccharomyces cerevisiae monoglyceride lipase Yju3p. Biochim. Biophys. Acta (BBA) Mol Cell Biol. Lipids 1861, 462–470. doi: 10.1016/j.bbalip.2016.02.005
Berger W., Steiner E., Grusch M., Elbling L., Micksche M. (2009). Vaults and the major vault protein: novel roles in signal pathway regulation and immunity. Cell. Mol. Life Sci. 66, 43–61. doi: 10.1007/s00018-008-8364-z
Boschen R. E., Rowden A. A., Clark M. R., Gardner J. P. (2013). Mining of deep-sea seafloor massive sulfides: a review of the deposits, their benthic communities, impacts from mining, regulatory frameworks and management strategies. Ocean Coast. Manage. 84, 54–67. doi: 10.1016/j.ocecoaman.2013.07.005
Bougerol M., Boutet I., LeGuen D., Jollivet D., Tanguy A. (2015). Transcriptomic response of the hydrothermal mussel Bathymodiolus azoricus in experimental exposure to heavy metals is modulated by the pgm genotype and symbiont content. Mar. Genomics 21, 63–73. doi: 10.1016/j.margen.2014.11.010
Brown A., Thatje S., Hauton C. (2017a). The effects of temperature and hydrostatic pressure on metal toxicity: insights into toxicity in the deep sea. Environ. Sci. Technol. 51, 10222–10231. doi: 10.1021/acs.est.7b02988
Brown A., Wright R., Mevenkamp L., Hauton C. (2017b). A comparative experimental approach to ecotoxicology in shallow-water and deep-sea holothurians suggests similar behavioural responses. Aquat. Toxicol. 191, 10–16. doi: 10.1016/j.aquatox.2017.06.028
Campos A., Tedesco S., Vasconcelos V., Cristobal S. (2012). Proteomic research in bivalves: Towards the identification of molecular markers of aquatic pollution. J. Proteomics 75, 4346–4359. doi: 10.1016/j.jprot.2012.04.027
Canesi L., Viarengo A., Leonzio C., Filippelli M., Gallo G. (1999). Heavy metals and glutathione metabolism in mussel tissues. Aquat. Toxicol. 46, 67–76. doi: 10.1016/S0166-445X(98)00116-7
Carlsson K.-H., Gade G. (1986). Metabolic adaptation of the horseshoe crab, Limulus polyphemus, during exercise and environmental hypoxia and subsequent recovery. Biol. Bull. 171, 217–235. doi: 10.2307/1541919
Chaudhary R., Baranwal V. K., Kumar R., Sircar D., Chauhan H. (2019). Genome-wide identification and expression analysis of Hsp70, Hsp90, and Hsp100 heat shock protein genes in barley under stress conditions and reproductive development. Funct. Integr. Genomics 19, 1007–1022. doi: 10.1007/s10142-019-00695-y
Chayakulkeeree M., Johnston S. A., Oei J. B., Lev S., Williamson P. R., Wilson C. F., et al. (2011). SEC14 is a specific requirement for secretion of phospholipase B1 and pathogenicity of cryptococcus neoformans. Mol. Microbiol. 80, 1088–1101. doi: 10.1111/j.1365-2958.2011.07632.x
Choong G., Liu Y., Templeton D. M. (2014). Interplay of calcium and cadmium in mediating cadmium toxicity. Chemico Biol Interact. 211, 54–65. doi: 10.1016/j.cbi.2014.01.007
Company R., Antunez O., Cosson R. P., Serafim A., Shillito B., Cajaraville M., et al. (2019). Protein expression profiles in Bathymodiolus azoricus exposed to cadmium. Ecotoxicol Environ. Saf. 171, 621–630. doi: 10.1016/j.ecoenv.2019.01.031
Company R., Serafim A., Cosson R. P., Fiala-Medioni A., Camus L., Serrao-Santos R., et al. (2010). Sub-Lethal effects of cadmium on the antioxidant defence system of the hydrothermal vent mussel Bathymodiolus azoricus. Ecotoxicol Environ. Saf. 73, 788–795. doi: 10.1016/j.ecoenv.2010.01.003
Dalle-Donne I., Rossi R., Milzani A., Di Simplicio P., Colombo R. (2001). The actin cytoskeleton response to oxidants: from small heat shock protein phosphorylation to changes in the redox state of actin itself. Free Radical Biol. Med. 31, 1624–1632. doi: 10.1016/S0891-5849(01)00749-3
del Rio G. F. (2016). Transcriptomic approach of the response to metals in the hydrothermal mussel bathymodiolus azoricus. (Doctoral dissertation, Université Pierre et Marie Curie-Paris VI).
Ding P., Xu Y., Li L., Lv X., Li L., Chen J., et al. (2022). Intracellular complement C5a/C5aR1 stabilizes β-catenin to promote colorectal tumorigenesis. Cell Rep. 39, 110851. doi: 10.1016/j.celrep.2022.110851
Fasulo S., Iacono F., Cappello T., Corsaro C., Maisano M., D’Agata A., et al. (2012). Metabolomic investigation of Mytilus galloprovincialis (Lamarck 1819) caged in aquatic environments. Ecotoxicol Environ. Saf. 84, 139–146. doi: 10.1016/j.ecoenv.2012.07.001
Fletcher D. A., Mullins R. D. (2010). Cell mechanics and the cytoskeleton. Nature 463, 485–492. doi: 10.1038/nature08908
Fokina N. N., Ruokolainen T. R., Nemova N. N., Bakhmet I. N. (2013). Changes of blue mussels Mytilus edulis l. lipid composition under cadmium and copper toxic effect. Biol. Trace Element Res. 154, 217–225. doi: 10.1007/s12011-013-9727-3
Girard F., Venail J., Schwaller B., Celio M. R. (2015). The EF-hand Ca2+-binding protein super-family: a genome-wide analysis of gene expression patterns in the adult mouse brain. Neuroscience 294, 116–155. doi: 10.1016/j.neuroscience.2015.02.018
Gomes T., Pereira C. G., Cardoso C., Bebianno M. J. (2013). Differential protein expression in mussels Mytilus galloprovincialis exposed to nano and ionic Ag. Aquat. Toxicol. 136, 79–90. doi: 10.1016/j.aquatox.2013.03.021
Goth L. (1991). A simple method for determination of serum catalase activity and revision of reference range. Clinica Chimica Acta 196, 143–151. doi: 10.1016/0009-8981(91)90067-M
Guo H., Chen T., Liang Z., Fan L., Shen Y., Zhou D. (2021). iTRAQ and PRM-based comparative proteomic profiling in gills of white shrimp litopenaeus vannamei under copper stress. Chemosphere 263, 128270. doi: 10.1016/j.chemosphere.2020.128270
Hardivillier A., Leignel V., Denis F., Uguen G., Cosson R., Laulier M. (2004). Do organisms living around hydrothermal vent sites contain specific metallothioneins? the case of the genus bathymodiolus (Bivalvia, mytilidae). Comp. Biochem. Physiol. C Toxicol Pharmacol. 139, 111–118.
Haskó G., Kuhel D. G., Németh Z. H., Mabley J. G., Stachlewitz R. F., Virág L., et al. (2000). Inosine inhibits inflammatory cytokine production by a posttranscriptional mechanism and protects against endotoxin-induced shock. J. Immunol. 164, 1013–1019. doi: 10.4049/jimmunol.164.2.1013
Hauton C., Brown A., Thatje S., Mestre N. C., Bebianno M. J., Martins I., et al. (2017). Identifying toxic impacts of metals potentially released during deep-sea mining–a synthesis of the challenges to quantifying risk. Front. Mar. Sci. 4. doi: 10.3389/fmars.2017.00368
He E. K., Qiu R. L., Cao X. D., Song L., Peijnenburg W. J. G. M., Qiu H. (2020). Elucidating toxicodynamic differences at the molecular scale between ZnO nanoparticles and ZnCl2 in Enchytraeus crypticus via nontargeted metabolomics. Environ. Sci. Technol. 54, 3487–3498. doi: 10.1021/acs.est.0c00663
Hohagen J., Jackson D. J. (2013). An ancient process in a modern mollusc: early development of the shell in Lymnaea stagnalis. BMC Dev. Biol. 13. doi: 10.1186/1471-213X-13-27
Hoher N., Kohler A., Strand J., Broeg K. (2012). Effects of various pollutant mixtures on immune responses of the blue mussel (Mytilus edulis) collected at a salinity gradient in Danish coastal waters. Mar. Environ. Res. 75, 35–44. doi: 10.1016/j.marenvres.2011.11.003
Huang W., Wang X. H., Chen D. Y., Xu E. G., Luo X., Zeng J. N., et al. (2021). Toxicity mechanisms of polystyrene microplastics in marine mussels revealed by high-coverage quantitative metabolomics using chemical isotope labeling liquid chromatography mass spectrometry. J. Hazard Mater 417. doi: 10.1016/j.jhazmat.2021.126003
Jiang H. X., Yang H. M., Kong X. H., Wang S. P., Liu D. Q., Shi S. J. (2012). Response of acid and alkaline phosphatase activities to copper exposure and recovery in freshwater fish Carassius auratus gibelio var. Life Sci. J Acta Zhengzhou Univ. Overseas Edition 9, 233–245.
Ji C. L., Cao L. L., Li F. (2015). Toxicological evaluation of two pedigrees of clam Ruditapes philippinarum as bioindicators of heavy metal contaminants using metabolomics. Environ. Toxicol. Pharmacol. 39, 545–554. doi: 10.1016/j.etap.2015.01.004
Jollow D., Mitchell J., Zampaglione N. A., Gillette J. (1974). Bromobenzene-induced liver necrosis. protective role of glutathione and evidence for 3, 4-bromobenzene oxide as the hepatotoxic metabolite. Pharmacology 11, 151–169. doi: 10.1159/000136485
Jones O. A. H., Dondero F., Viarengo A., Griffin J. L. (2008). Metabolic profiling of Mytilus galloprovincialis and its potential applications for pollution assessment. Mar. Ecol. Prog. Ser. 369, 169–179. doi: 10.3354/meps07654
Jost R., Berkowitz O., Shaw J., Masle J. (2009). Biochemical characterization of two wheat phosphoethanolamine n-methyltransferase isoforms with different sensitivities to inhibition by phosphatidic acid. J. Biol. Chem. 284, 31962–31971. doi: 10.1074/jbc.M109.022657
Kim B. H., Schöffl F. (2002). Interaction between arabidopsis heat shock transcription factor 1 and 70 kDa heat shock proteins. J. Exp. Bot. 53, 371–375. doi: 10.1093/jexbot/53.367.371
King J. (1965). “The hydrolases-acid and alkaline phosphatases,” in Practical clinical enzymology. Ed. King J. (London: Van Nostrand Company Ltd), 191–208.
Kohler A., Wahl E., Soffker K. (2002). Functional and morphological changes of lysosomes as prognostic biomarkers of toxic liver injury in a marine flatfish (Platichthys flesus (L.)). Environ. Toxicol. Chem. 21, 2434–2444. doi: 10.1002/etc.5620211124
Korobeĭnikova E. N. (1989). Modification of the determination of lipid peroxidation products in a reaction with thiobarbituric acid. Laboratornoe delo, 8–10.
Lara P. C., Pruschy M., Zimmermann M., Henriquez-Hernandez L. A. (2011). MVP and vaults: a role in the radiation response. Radiat. Oncol. 6. doi: 10.1186/1748-717X-6-148
Leonard J. A., Cope W. G., Barnhart M. C., Bringolf R. B. (2014). Metabolomic, behavioral, and reproductive effects of the synthetic estrogen 17 alpha-ethinylestradiol on the unionid mussel Lampsilis fasciola. Aquat. Toxicol. 150, 103–116. doi: 10.1016/j.aquatox.2014.03.004
Liang P., MacRae T. H. (1997). Molecular chaperones and the cytoskeleton. J. Cell Sci. 110, 1431–1440. doi: 10.1242/jcs.110.13.1431
Li L., Tian X., Yu X., Dong S. (2016). Effects of acute and chronic heavy metal (Cu, cd, and zn) exposure on sea cucumbers (Apostichopus japonicus). BioMed. Res. Int. 2016.
Liu Z. Q., Lv W. W., Huang Y. H., Fan B., Li Y. M., Zhao Y. L. (2016). Effects of cadmium on lipid metabolism in female estuarine crab, Chiromantes dehaani. Comp. Biochem. Physiol. C Toxicol Pharmacol. 188, 9–16.
Liu J., Qu W., Kadiiska M. B. (2009). Role of oxidative stress in cadmium toxicity and carcinogenesis. Toxicol. Appl. Pharmacol. 238, 209–214. doi: 10.1016/j.taap.2009.01.029
Li Z. H., Zlabek V., Velisek J., Grabic R., Machova J., Kolarova J., et al. (2011). Acute toxicity of carbamazepine to juvenile rainbow trout (Oncorhynchus mykiss): Effects on antioxidant responses, hematological parameters and hepatic EROD. Ecotoxicol Environ. Saf. 74, 319–327. doi: 10.1016/j.ecoenv.2010.09.008
Lovett D. L., Towle D. W., Faris J. E. (1994). Salinity sensitive alkaline-phosphatase activity in gills of the blue crab, Callinectes sapidus rathbun. Comp. Biochem. Physiol. B Biochem Mol. Biol. 109, 163–173.
Lucu Č., Obersnel V. (1996). Cadmium influx across isolated carcinus gill epithelium interaction of lanthanum and calcium with cadmium influxes. J. Comp. Physiol. B 166, 184–189. doi: 10.1007/BF00263981
Martins I., Goulart J., Martins E., Morales-Román R., Marín S., Riou V., et al. (2017). Physiological impacts of acute Cu exposure on deep-sea vent mussel Bathymodiolus azoricus under a deep-sea mining activity scenario. Aquat. Toxicol. 193, 40–49. doi: 10.1016/j.aquatox.2017.10.004
Ma L., Wang W.-X. (2020). Subcellular metal distribution in two deep-sea mollusks: Insight of metal adaptation and detoxification near hydrothermal vents. Environ. pollut. 266, 115303. doi: 10.1016/j.envpol.2020.115303
Mazorra M. T., Rubio J. A., Blasco J. (2002). Acid and alkaline phosphatase activities in the clam Scrobicularia plana: kinetic characteristics and effects of heavy metals. Comp. Biochem. Physiol. Part B: Biochem. Mol. Biol. 131, 241–249.
McCord J. M., Fridovich I. (1969). Superoxide dismutase an enzymic function for erythrocuprein (hemocuprein). J. Biol. Chem. 244, 6049–6055. doi: 10.1016/S0021-9258(18)63504-5
McDonagh B., Sheehan D. (2006). Redox proteomics in the blue mussel Mytilus edulis: carbonylation is not a pre-requisite for ubiquitination in acute free radical-mediated oxidative stress. Aquat. Toxicol. 79, 325–333. doi: 10.1016/j.aquatox.2006.06.020
McKnight K. L., Swanson K. V., Austgen K., Richards C., Mitchell J. K., McGivern D. R., et al. (2020). Stimulator of interferon genes (STING) is an essential proviral host factor for human rhinovirus species a and c. Proc. Natl. Acad. Sci. U.S.A. 117, 27598–27607. doi: 10.1073/pnas.2014940117
Meng X.-L., Li S., Qin C.-B., Zhu Z.-X., Hu W.-P., Yang L.-P., et al. (2018). Intestinal microbiota and lipid metabolism responses in the common carp (Cyprinus carpio l.) following copper exposure. Ecotoxicol Environ. Saf. 160, 257–264. doi: 10.1016/j.ecoenv.2018.05.050
Mestre N., Auguste M., De Sá L., Fonseca T., Cardoso C., Brown A., et al. (2019). Are shallow-water shrimps proxies for hydrothermal-vent shrimps to assess the impact of deep-sea mining? Mar. Environ. Res. 151, 104771.
Moore M. N. (1994). Reactions of molluscan lysosomes as biomarkers of pollutant-induced cell injury. contaminants in the environment. a multidiscplinary assessment of risks to man and other organisms (Boca Raton: CRC Press, Lewis Publishers), 111–123.
Mukherjee P. K., Chandra J., Kuhn D. M., Ghannoum M. A. (2003). Differential expression of Candida albicans phospholipase b (PLB1) under various environmental and physiological conditions. Microbiology-Sgm 149, 261–267. doi: 10.1099/mic.0.25829-0
Pampanin D. M., Ballarin L., Carotenuto L., Marin M. G. (2002). Air exposure and functionality of Chamelea gallina haemocytes: effects on haematocrit, adhesion, phagocytosis and enzyme contents (vol 131, pg 605, 2002). Comp. Biochem. Physiol. A Mol Integr. Physiol. 133, 199–199. doi: 10.1016/S1095-6433(02)00114-9
Pan K., Wang W. X. (2008). The subcellular fate of cadmium and zinc in the scallop Chlamys nobilis during waterborne and dietary metal exposure. Aquat. Toxicol. 90, 253–260. doi: 10.1016/j.aquatox.2008.09.010
Pinheiro M., Oliveira A., Barros S., Alves N., Raimundo J., Caetano M., et al. (2021). Functional, biochemical and molecular impact of sediment plumes from deep-sea mining on Mytilus galloprovincialis under hyperbaric conditions. Environ. Res. 195, 110753. doi: 10.1016/j.envres.2021.110753
Pruski A. M., Dixon D. R. (2007). Heat shock protein expression pattern (HSP70) in the hydrothermal vent mussel Bathymodiolus azoricus. Mar. Environ. Res. 64, 209–224. doi: 10.1016/j.marenvres.2007.01.003
Rathbone M. P., Middlemiss P. J., Gysbers J. W., Andrew C., Herman M. A. R., Reed J. K., et al. (1999). Trophic effects of purines in neurons and glial cells. Prog. Neurobiol. 59, 663–690. doi: 10.1016/S0301-0082(99)00017-9
Revel M., Lagarde F., Perrein-Ettajani H., Bruneau M., Akcha F., Sussarellu R., et al. (2019). Tissue-specific biomarker responses in the blue mussel mytilus spp. exposed to a mixture of microplastics at environmentally relevant concentrations. Front. Environ. Sci. 7.
Ringeissen S., Connor S. C., Brown H. R., Sweatman B. C., Hodson M. P., Kenny S. P., et al. (2003). Potential urinary and plasma biomarkers of peroxisome proliferation in the rat: identification of n-methylnicotinamide and n-methyl-4-pyridone-3-carboxamide by 1H nuclear magnetic resonance and high performance liquid chromatography. Biomarkers 8, 240–271. doi: 10.1080/1354750031000149124
Sanchez B. C., Ralston-Hooper K., Sepulveda M. S. (2011). Review of rRecent proteomic applications in aquatic toxicology. Environ. Toxicol. Chem. 30, 274–282. doi: 10.1002/etc.402
Siafakas A. R., Sorrell T. C., Wright L. C., Wilson C., Larsen M., Boadle R., et al. (2007). Cell wall-linked cryptococcal phospholipase B1 is a source of secreted enzyme and a determinant of cell wall integrity. J. Biol. Chem. 282, 37508–37514. doi: 10.1074/jbc.M707913200
Sokolova I. M., Frederich M., Bagwe R., Lannig G., Sukhotin A. A. (2012). Energy homeostasis as an integrative tool for assessing limits of environmental stress tolerance in aquatic invertebrates. Mar. Environ. Res. 79, 1–15. doi: 10.1016/j.marenvres.2012.04.003
Sun Q. Y., Li Y., Shi L. J., Hussain R., Mehmood K., Tang Z. X., et al. (2022). Heavy metals induced mitochondrial dysfunction in animals: Molecular mechanism of toxicity. Toxicology 469, 153136. doi: 10.1016/j.tox.2022.153136
Sun Y., Liu Y., Yu X. B., Wang T., Wang Q., Yao W. Z., et al. (2021). Combined effects of ration levels and temperature on immune responses of the triangle sail mussel Hyriopsis cumingii. Aquacult Res Toxicology. 469, 153136.
Techo T., Jindarungrueng S., Tatip S., Limcharoensuk T., Pokethitiyook P., Kruatrachue M., et al. (2020). Vacuolar h+-ATPase is involved in preventing heavy metal-induced oxidative stress in saccharomyces cerevisiae. Environ. Microbiol. 22, 2403–2418. doi: 10.1111/1462-2920.15022
Tomanek L. (2011). Environmental proteomics: changes in the proteome of marine organisms in response to environmental stress, pollutants, infection, symbiosis, and development. Ann. Rev. Mar. Sci. 3, 373–399. doi: 10.1146/annurev-marine-120709-142729
Tsuchida T., Zou J. A., Saitoh T., Kumar H., Abe T., Matsuura Y., et al. (2010). The ubiquitin ligase TRIM56 regulates innate immune responses to intracellular double-stranded DNA. Immunity 33, 765–776. doi: 10.1016/j.immuni.2010.10.013
Valko M., Morris H., Cronin M. T. D. (2005). Metals, toxicity and oxidative stress. Curr. Medicinal Chem. 12, 1161–1208. doi: 10.2174/0929867053764635
Venier P., Varotto L., Rosani U., Millino C., Celegato B., Bernante F., et al. (2011). Insights into the innate immunity of the Mediterranean mussel Mytilus galloprovincialis. BMC Genomics 12. doi: 10.1186/1471-2164-12-69
Viant M. R., Rosenblum E. S., Tjeerdema R. S. (2003). NMR-based metabolomics: a powerful approach for characterizing the effects of environmental stressors on organism health. Environ. Sci. Technol. 37, 4982–4989. doi: 10.1021/es034281x
Wallace W. G., Lee B. G., Luoma S. N. (2003). Subcellular compartmentalization of cd and zn in two bivalves. i. significance of metal-sensitive fractions (MSF) and biologically detoxified metal (BDM). Mar. Ecol. Prog. Ser. 249, 183–197. doi: 10.3354/meps249183
Wallace W. G., Luoma S. N. (2003). Subcellular compartmentalization of cd and zn in two bivalves. II. significance of trophically available metal (TAM). Mar. Ecol. Prog. Ser. 257, 125–137. doi: 10.3354/meps257125
Wong Y. H., Sun J., He L. S., Chen L. G., Qiu J. W., Qian P. Y. (2015). High-throughput transcriptome sequencing of the cold seep mussel Bathymodiolus platifrons. Sci. Rep. 5, 16597. doi: 10.1038/srep16597
Xu L., Ji C., Wu H., Tan Q., Wang W.-X. (2016). A comparative proteomic study on the effects of metal pollution in oysters Crassostrea hongkongensis. Mar. pollut. Bull. 112, 436–442. doi: 10.1016/j.marpolbul.2016.07.009
Xu L. L., Lu Z., Ji C. L., Cong M., Li F., Shan X. J., et al. (2019). Toxicological effects of as (V) in juvenile rockfish Sebastes schlegelii by a combined metabolomic and proteomic approach. Environ. pollut. 255. doi: 10.1016/j.envpol.2019.113333
Yang B. B., Lin S. H., Li B., Wei Z., Li Q. Q., Shen X. L., et al. (2022). Interleukin enhancer binding factor 2 (IEBF 2) was involved in the regulation of the antibacterial immune reactions in fresh water crayfish, Procambarus clarkii. Dev. Comp. Immunol. 126. doi: 10.1016/j.dci.2021.104226
Yu X. J., Pan K., Liu F. J., Yan Y., Wang W. X. (2013). Spatial variation and subcellular binding of metals in oysters from a large estuary in China. Mar. pollut. Bull. 70, 274–280. doi: 10.1016/j.marpolbul.2013.02.036
Yusof N. A., Masnoddin M., Charles J., Thien Y. Q., Nasib F. N., Wong C. M. V. L., et al. (2022). Can heat shock protein 70 (HSP70) serve as biomarkers in Antarctica for future ocean acidification, warming and salinity stress? Polar Biol., 1–24.
Zhang J., Li H., Qin Y., Ye S., Liu M. (2016). Identification of functional genes involved in Cd2+ response of Chinese surf clam (Mactra chinensis) through transcriptome sequencing. Environ. Toxicol. Pharmacol. 41, 113–120. doi: 10.1016/j.etap.2015.11.006
Zhang H., You Q. D., Xu X. L. (2020). Targeting stimulator of interferon genes (STING): A medicinal chemistry perspective. J. Medicinal Chem. 63, 3785–3816. doi: 10.1021/acs.jmedchem.9b01039
Zhou L., Cao L., Wang X., Wang M., Wang H., Zhong Z., et al. (2020). Metal adaptation strategies of deep-sea bathymodiolus mussels from a cold seep and three hydrothermal vents in the West pacific. Sci. Total Environ. 707, 136046. doi: 10.1016/j.scitotenv.2019.136046
Keywords: metal, toxicology, Mytilidae, deep-sea, omics, environment monitor
Citation: Zhou L, Li M, Zhong Z, Chen H, Wang M, Lian C, Wang H, Zhang H, Cao L and Li C (2023) Toxicological effects of cadmium on deep-sea mussel Gigantidas platifrons revealed by a combined proteomic and metabolomic approach. Front. Mar. Sci. 10:1087411. doi: 10.3389/fmars.2023.1087411
Received: 02 November 2022; Accepted: 04 January 2023;
Published: 18 January 2023.
Edited by:
Yu Zhang, Shanghai Jiao Tong University, ChinaReviewed by:
Liqiang Zhao, Guangdong Ocean University, ChinaCopyright © 2023 Zhou, Li, Zhong, Chen, Wang, Lian, Wang, Zhang, Cao and Li. This is an open-access article distributed under the terms of the Creative Commons Attribution License (CC BY). The use, distribution or reproduction in other forums is permitted, provided the original author(s) and the copyright owner(s) are credited and that the original publication in this journal is cited, in accordance with accepted academic practice. No use, distribution or reproduction is permitted which does not comply with these terms.
*Correspondence: Chaolun Li, bGNsQHFkaW8uYWMuY24=
Disclaimer: All claims expressed in this article are solely those of the authors and do not necessarily represent those of their affiliated organizations, or those of the publisher, the editors and the reviewers. Any product that may be evaluated in this article or claim that may be made by its manufacturer is not guaranteed or endorsed by the publisher.
Research integrity at Frontiers
Learn more about the work of our research integrity team to safeguard the quality of each article we publish.